- Department of Internal Medicine and Clinical Nutrition, Institute of Medicine, Sahlgrenska Osteoporosis Centre and Centre for Bone and Arthritis Research at the Sahlgrenska Academy, University of Gothenburg, Gothenburg, Sweden
The first evidence of the existence of vitamin A was the observation 1881 that a substance present in small amounts in milk was necessary for normal development and life. It was not until more than 100 years later that it was understood that vitamin A acts as a hormone through nuclear receptors. Unlike classical hormones, vitamin A cannot be synthesized by the body but needs to be supplied by the food as retinyl esters in animal products and ß-carotene in vegetables and fruits. Globally, vitamin A deficiency is a huge health problem, but in the industrialized world excess of vitamin A has been suggested to be a risk factor for secondary osteoporosis and enhanced susceptibility to fractures. Preclinical studies unequivocally have shown that increased amounts of vitamin A cause decreased cortical bone mass and weaker bones due to enhanced periosteal bone resorption. Initial clinical studies demonstrated a negative association between intake of vitamin A, as well as serum levels of vitamin A, and bone mass and fracture susceptibility. In some studies, these observations have been confirmed, but in other studies no such associations have been observed. One meta-analysis found that both low and high serum levels of vitamin A were associated with increased relative risk of hip fractures. Another meta-analysis also found that low levels of serum vitamin A increased the risk for hip fracture but could not find any association with high serum levels of vitamin A and hip fracture. It is apparent that more clinical studies, including large numbers of incident fractures, are needed to determine which levels of vitamin A that are harmful or beneficial for bone mass and fracture. It is the aim of the present review to describe how vitamin A was discovered and how vitamin A is absorbed, metabolized and is acting as a ligand for nuclear receptors. The effects by vitamin A in preclinical studies are summarized and the clinical investigations studying the effect by vitamin A on bone mass and fracture susceptibility are discussed in detail.
Introduction
Vitamin A, present in both animal products and vegetables, is a fat-soluble compound referring to substances possessing the biological activity of retinol and characterized by unsaturated isoprenoid chain structures. It is well known that vitamin A is important for normal growth, reproduction, vision and immunity, but also for having important roles in pathological processes such as cardiovascular diseases, cancer, skin diseases and obesity (1). High levels of vitamin A have also been considered as a risk factor for secondary osteoporosis (2, 3). Biological effects by vitamin A are due to activation of nuclear receptors by the retinol metabolite all-trans-retinoic acid (ATRA) and subsequent regulation of more than 500 genes in a variety of cell types (4). Although the main aim of the present review is to discuss skeletal remodeling as a consequence of high levels of vitamin A in industrialized countries, it is important to note that nutritional deficiency of vitamin A is a huge public health problem worldwide. In developing countries, in which the prevalence of vitamin A deficiency can be as common as 60% of the population, the deficiency causes xerophthalmia and blindness in more than 4 million children and numerous numbers of early deaths due to infections (5).
Discovery
As pointed out by Semba (6), it is not possible to establish when vitamin A was discovered and who made the discovery. Important early observations were made by the Russian scientist Nicolai Lunin 1881 in experiments where adult mice were found to live well on milk, but were unable to stay alive on a diet containing protein, fat, carbohydrates, salt and water unless this food was supplemented with dried milk (7). Shortly afterwards (1891), it was found that unknown, fat-like substance(s) in egg yolk was/were necessary for good health in mice (8). Further support for the finding that milk contained important substance(s) was the observation 1909 that rats fed a diet containing the essential nutrients for life according to the prevailing dogma, i.e. protein, fat, carbohydrates and minerals, died, but stayed alive healthy if milk was added to this diet (9). The most famous experiments were then made by the British physician and biochemist Frederic Gowland Hopkins (1912), who reported that young rats given protein, starch, cane sugar, lard and minerals gained weight considerably less than rats fed the same diet with very small amounts of milk (10). He also showed that when milk was withdrawn from the food given to well growing rats, these rats stopped growing, and that the slowly growing rats started to grow fast when they were given food with milk. He concluded that an “accessory factor” present in “astongishly small amounts” in milk is essential for life, although these studies did not show which substance or substances that were important. The same year, Casmir Funk suggested to designate all minor essential nutrients in foods as ´vitamins´, with the mistaken idea that all these factors are ´vital amines´ (11), which led to that the “accessory food factor”, sometimes called “fat-soluble A”, eventually was given the name “vitamin A”.
Sir Frederic Gowland Hopkins shared the Noble Prize in Medicine and Physiology 1929 for having discovered vitamins with the Dutch physician Christiaan Eijkman, who had described that beriberi could be cured by a compound present in rice (now known as thiamin or vitamin B1). Hopkins was, however, always keen to give credit to the early observations by Nicolai Lunin (7).
By feeding vitamin A deficient mice with carotenoids, it was shown that carotene is a precursor to vitamin A (12). The Swiss chemist Paul Karrer described the chemical formula of carotenoid and later showed how carotenoids can be transformed to vitamin A, observations followed by the establishment of the chemical structure of vitamin A itself using extracts from cod-liver oil (13, 14). Paul Karrer received the Noble Prize in Chemistry 1937 for these achievements. Vitamin A was crystallized 1937 (15) and during late 1940´s vitamin A was synthesized and produced in large scale to be used for treatment of deficiency (16, 17).
During the following two decades, several groups reported that deficiency and excess of vitamin A resulted in developmental disturbances. An important finding during this time period was the demonstration that 11-cis-retinal, together with the protein opsin, makes up the light sensitive chromophore rhodopsin in the eye (18), a finding which resulted in that the American biochemist George Wald was awarded the Noble Prize in Physiology and Medicine 1967 together with the Finnish physiologist Ragnar Granit and the American physiologist Haldan Keffer Hartline. A key event in vitamin A biology was the discoveries by the American biochemist Hector deLuca that all-trans-retinoic acid (ATRA) is formed in vivo from retinol and in fact is a natural metabolite of retinol (19, 20), followed by the observation that ATRA is the most potent metabolite of vitamin A inducing differentiation of F9 embryonal carcinoma cells in vitro (21). During the 1970´s, binding proteins for retinol and retinoic acid in plasma and cells were discovered, followed by studies showing how retinoids were metabolized and transported extra- and intracellularly (22, 23). Subsequent studies showed that some intracellular binding proteins function as nuclear receptors, which when binding to DNA act as transcription factors following dimerizing with other nuclear receptors (24–26).
Beneficial effects by vitamin A
A large number of different cell types express receptors for vitamin A and thereby are target cells for this pleiotropic hormone. In many of these cells, vitamin A regulates cell growth and differentiation and is important both for embryological development, including of the skeleton (see further below “Vitamin A receptor signaling”), as well as for tissue growth and regeneration during postnatal life (27, 28). Importantly for these functions, vitamin A regulates subsets of genes in stem cells involved in their fate and differentiation (29).
In hematopoiesis, vitamin A enhances expansion of hematopoietic stem cells and induces differentiation of granulocytes from committed myeloid precursors (30). Vitamin A is important in immune processes in both innate and acquired immunity through effects on regulatory T cell growth and differentiation, B-cell maturation and antibody responses and differentiation of dendritic cells (31–33).
Vitamin A has also important effects in a variety of different functions in epithelial cells in tissues such as skin, sweat glands and salivary glands. Effects on keratinocytes involves stimulation of epithelial cells growth and differentiation during wound healing (33).
Due to effects by vitamin A on cell proliferation, differentiation and apoptosis, a lot of interest has been directed to a potential role of vitamin A in cancer and both stimulatory and inhibitory effects have been observed. However, treatment of certain cancers with different retinoids, including ATRA, is approved by FDA (33). Treatment of acute promyelocytic leukemia (APL) has turned out to be particularly successful (34). Retinoids are also used to treat skin diseases such as psoriasis, acne, seborrhea and ichthyosis (33).
Harmful effects by vitamin A
Acute harmful effects by excess of vitamin A are rare today but are well known among early Arctic explorer who suffered from vertigo, vomiting, diarrhea, headache and convulsions which could cause their death when consuming vitamin A rich liver from polar beer or from Greenland Husky sled dog. Today, hypervitaminosis A is sometimes observed in young people consuming large amounts of candy-like supplements or overdosing retinoids for treatment of acne (35).
Deleterious effects by vitamin A on the skeleton observed in preclinical studies was reported already 1934 (36). Extreme softness of bone and spontaneous fractures were observed in rats given high doses of vitamin A. During the 1940´s, limping gait and fractures associated with increased bone resorption were demonstrated in rats and guinea pigs given high amounts of vitamin A (37, 38). Similar observations were made in dogs given vitamin A (39). It was not known if the deleterious effects by vitamin A on the skeleton were caused by direct or indirect effects. Barnicot was the first to show 1948 a direct erosive effect on bone by adding fragments of crystalline vitamin A on parietal bones ex vivo, which were then transplanted to cerebral hemispheres of littermates (40). Direct effect by vitamin A on bone loss associated with osteoclasts was also noted in explant cultures of either chicken limb bone or fetal mouse long bones (41, 42). Since then, numerous studies have demonstrated increased bone resorption, decreased bone mass and enhanced fracture susceptibility in rodents given high doses of vitamin A for short period of time (2).
The first clinical reports describing effects by vitamin A on the skeleton in humans were some few case reports published during 1944 -1988 describing symptoms in patients taking intoxicating doses of vitamin A (43). These reports described bone pain, radiographic osteopenia, hypercalcemia and increased bone resorption. Early epidemiological studies during 1980 and 90’s yielded contradictory results when assessing possible associations between dietary intake of vitamin A, serum retinol and bone mass (43). These studies, however, were often low-powered with less accurate methods to assess bone mass. The first large, well designed clinical study was the investigation by Melhus et al. in which dietary intake of retinol was found to be positively related to decreased bone mass in several skeletal sites, using dual-energy X-ray absorptiometry (DXA), and to increased hip fracture risk (44). Since then, several studies assessing the potentially detrimental effect by vitamin A on bone mass and fracture risk have been published, however, with divergent results (2).
Vitamin A metabolism and receptor signaling
Since vitamin A is not produced by the body it needs to be provided in the diet either as retinyl esters in animal products or as ß-carotene in vegetables. These precursor molecules are taken up by the intestine and then delivered to the lymphatics before being released into blood. A substantial part of vitamin A is stored in the liver and serves as a reservoir in case of vitamin A deficiency. In the circulation, vitamin A is present mainly as retinol but also as retinyl esters and ß-carotene. Uptake and metabolism of vitamin A involve several binding proteins and enzymes.
To regulate target cell activities, circulating forms of vitamin A need to be converted in the cytoplasm to the biologically active compound ATRA, which acts as a ligand for vitamin A receptors in the nucleus. These receptors heterodimerize with another type of nuclear vitamin A receptor for which the ligand is elusive. The complex binds to DNA and either activate or repress gene transcription.
It is the aim of the present review to briefly describe the metabolism and cellular effects by vitamin A and to present pro and cons regarding the potential harmful effect by vitamin A on the skeleton and to argue for the need of well-powered prospective clinical studies including large numbers of incident fractures. It is also suggested that the potential effects by vitamin A on load-induced anabolic response, fracture healing and effects by bone anabolic drugs should be investigated.
Vitamin A metabolism
Since vitamin A cannot be synthesized in the body, precursor molecules need to be acquired from the food and converted to biologically active compounds through many steps in the intestine, liver and, finally, in target cells. These processes involve several enzymes, binding proteins as well as cell surface and nuclear receptors. In the food, vitamin A is present as retinyl esters (mainly retinyl palmitate) in animal products such as egg, meat, fish, fortified cereals and dairy products, and in plant-based food such as vegetables and fruits as ß-carotene.
Since retinyl esters and ß-carotene are water insoluble they are transferred in the intestinal lumen to mixed micelles, a process which is facilitated by gastric and pancreatic enzymes. Before being taken up by enterocytes, retinyl esters are hydrolyzed to retinol mainly by pancreatic lipase. The uptake of retinol and ß-carotene in the apical side of enterocytes is not known in detail but is believed to be due to both passive diffusion and facilitated transport (45) (Figure 1). Importantly, the uptake and intracellular metabolism of ß-carotene in enterocytes are under regulation of a negative feed-back loop controlled by the transcription factor ISX (intestinal-specific homeobox gene), which is induced by ATRA (46).
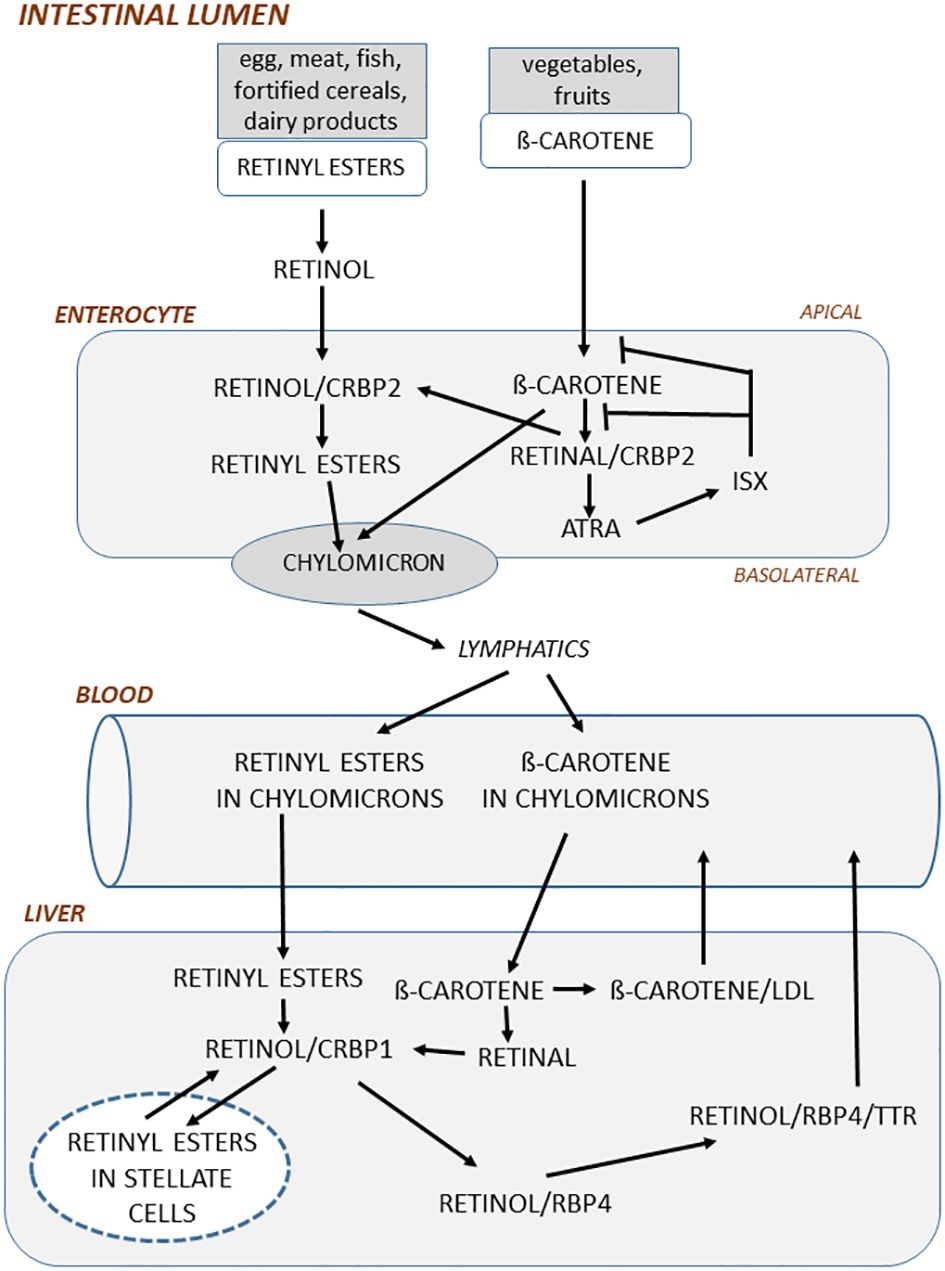
Figure 1 In the intestinal lumen, retinyl esters in food is hydrolyzed to retinol mainly by pancreatic enzymes. Retinol is taken up by enterocytes in the apical side of enterocytes and then bound to cellular retinol-binding protein 2 (CRBP2) and subsequently esterified by lechitin retinol acyltransferase to retinyl esters. ß-carotene in the food is taken up by enterocytes and then oxidized to retinal by ß-carotene-15,15´-monooxygenase. Retinal bound to CRBP2 is then either converted to retinol by retinal reductases or oxidized to ATRA by retinal aldehyde dehydrogenase. ATRA induces the transcription factor ISX, which acts as a negative feedback regulator by inhibiting both conversion of ß-carotene to retinal and the uptake of ß-carotene. In the basolateral side of enterocytes, retinyl esters and non-converted ß-carotene are incorporated into chylomicrons, which through the lymphatics are delivered to blood. Retinyl esters in chylomicrons are taken up by the liver and there hydrolyzed to retinol by retinyl ester hydrolase. Retinol bound to CRBP1 is then either converted to retinyl esters by lechitin retinol acyltransferase and stored in lipid droplets in stellate cells or transported to the site where retinol is bound to retinol-binding protein 4 (RBP4). Before being delivered to blood, retinol/RBP4 is bound to transthyretin. ß-carotene taken up by liver is converted to retinal by ß-carotene oxygenase 1 and then further to retinol or bound to very low-density lipoprotein and released to blood.
In the enterocytes, retinol binds to CRBP2 (cellular retinol-binding protein 2), a binding protein solely expressed in intestine (47), and is then esterified to retinyl esters, which is necessary for the incorporation in chylomicrons (small particles of triglycerides, phospholipids, proteins, and cholesterol), whereas ß -carotene is first converted to retinal, then to retinol and subsequently to retinyl esters (48) (Figure 1). The retinyl esters and some non-converted ß-carotene are packed in chylomicrons, which are delivered through the basolateral part of enterocytes to the lymphatics and then released into blood. ATRA is also formed in the enterocytes which induces ISX through nuclear receptors (see further below).
A unique feature of vitamin A is that, unlike other vitamins, vitamin A can be stored in the body. Although several organs and cells can store vitamin A, including fat cells, the main part (70%) of vitamin A is stored in the liver (23). Storage of vitamin A in the body can protect from vitamin A insufficiencies for several months. Following uptake of chylomicrons in the liver, retinyl esters and ß-carotene are released into hepatocytes, where retinyl esters are hydrolyzed to retinol and bound to CRBP1 (49) (Figure 1). Retinol is then either bound to RBP4 (retinol-binding protein 4) or transported to fat-storing hepatic stellate cells and esterified to retinyl esters (50, 51). 70-90% of vitamin stored in the liver is as retinyl esters in stellate cells. How ß-carotene is metabolized in the liver is less known, but it is assumed that it is converted to retinal and then to retinol and retinyl esters and subsequently either stored in hepatic cells or bound to RBP4 as retinol. Secretion of vitamin A from the liver is mainly as retinol/RBP4, which is also bound to the transporter protein TTR (transthyretin) to stabilize the complex and to reduce filtration through the kidneys (52).
In the circulation, a variety of different forms of vitamin A are present, including retinol, retinyl esters, carotenoids and retinoic acid, which concentrations can vary at fasting state and during postprandial periods (23). The concentration of retinol/RBP4/TTR is tightly regulated and varies between 2 and 4 µM, with the exception of in vitamin A deficient individuals in whom the concentration can be significantly increased after a vitamin A reach meal (23, 53–55). Serum levels of retinol <0.7 µM indicate vitamin A deficiency, whereas levels >2.1 µM are suggested to indicate hypervitaminosis A (33). Similar to retinol, ß-carotene in blood is regulated and the concentration is 5-8 µM (56). The concentration of retinyl esters in blood, however, varies considerably from 100-200 nM during fasting conditions up to 5-10 µM at postprandial periods (57). The ratio of fasting serum retinyl esters to total serum vitamin A (retinol+retinyl esters) has also been suggested to be a marker of vitamin A status. A ratio >10% has been suggested as a marker of potential vitamin A toxicity (58, 59). Retinoic acid is present in blood at 1-3 nM, but can increase for a short period of time to 80-90 nM after a vitamin A rich meal (60). As discussed in more detail by Borel and Desmarchelier (2017), the concentrations of different retinoids in blood are modulated by a large number of proteins, which participate in the metabolism of chylomicrons and other lipoproteins transporting ß-carotene and retinyl esters and by those proteins which are important for liver secretion and blood metabolism of retinol (48). Moreover, the bioavailability of vitamin A is affected more by vitamin A stores than by serum retinol levels (61) and is also affected by genetic variability as assessed by genome-wide association studies (48).
In target cells regulated by vitamin A, there is some evidence that retinol/RBP4 is taken up by the cells through diffusion (62–64), but there is also evidence indicating that uptake can be facilitated through a receptor named STRA6 (stimulated by retinoic acid 6) (65, 66) (Figure 2). This multimembrane domain protein binds RBP4 and increases cellular uptake of retinol (65). The expression of STRA6 is crucial for the formation of rhodopsin in the eye, as shown by the decreased visual response in Stra6-deficient mice (67), similar to mice lacking Rbp4 (68). STRA6, however, seems not to be important for uptake of retinol in all cell types, since although Stra6 is expressed in several cell types, there are several cells which lack Stra6 expression. Human bone marrow stromal cells have been shown to express STRA6 mRNA and the expression seemed to be higher in cells isolated from osteoporotic patients compared to cells from osteopenic or healthy individuals (69). The authors also reported that Stra6 mRNA was expressed in the mouse embryonic fibroblast-like cell line C3H10T1/2 and the mouse calvarial osteoblast cell line MC3T3/E1. Some evidence was presented indicating that Stra6 could regulate osteoblast differentiation, but the results are difficult to interpret since no information is given whether retinol/RBP4 was present in the culture media. Therefore, the role of STRA6 for retinoid-dependent effects on bone cells is not known.
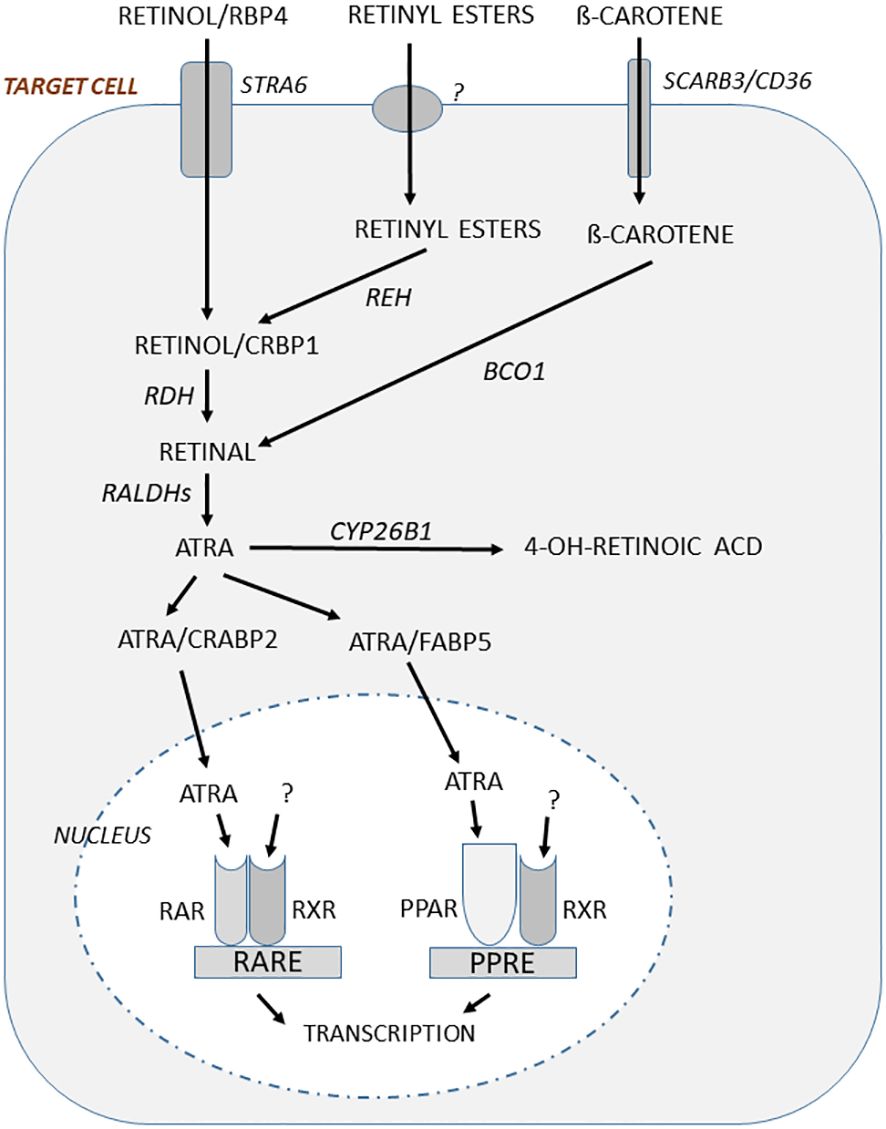
Figure 2 In target cells responding to regulation by vitamin A, retinol bound to RBP4 is taken up through the receptor STRA6 and then bound to CRBP1, whereas ß-carotene uptake is mediated by SCARB3/CD36. Retinyl esters are also taken up, but the mechanism is not known. The retinyl esters are hydrolyzed to retinol by retinyl ester hydrolase (REH). Retinol is oxidized by retinol dehydrogenase (RDH) to retinal which then is oxidized in a two-step processes using retinal aldehyde dehydrogenases (RALDH1, RALDH2) to form ATRA. Retinal is also formed by conversion of ß-carotene through ß-carotene-15,15´-monooxygenase (BCO1). Binding of ATRA to cellular retinoid acid-binding protein 2 (CRABP2) facilitates translocation to nucleus where ATRA binds to RARs. This complex heterodimerize with RXRs for which the ligand is not known, but may be 9-cis-13,14-dihydroretinoic acid. This dimeric ligand-inducible transcription factor will increase or decrease gene transcription through retinoic acid responsive elements (RAREs). ATRA can also bind to fatty acid-binding protein 5 (FABP5) and this complex facilitates translocation to the nucleus and binding to peroxisome proliferator-activated receptors (PPAR), which after heterodimerizing with RXRs act as a transcription factor increasing or decreasing gene transcription through peroxisome proliferator response element (PPRE).
The uptake in target cells of ß-carotene present in blood in chylomicrons or bound to other lipoproteins is mediated by SCARB3 (scavenger receptors class B), also known as CD36 and the related SCARB1. It is not known if retinyl esters in chylomicron or bound to other lipoproteins are taken up through a receptor. It has, however, been suggested that retinyl esters can be taken up in target cells by a “flip-flop” process across the phospholipid bilayer in cell membranes (70) (Figure 2).
In the target cells, retinol delivered by uptake or formed after conversion of retinyl esters through retinyl ester hydrolase (REH), is bound to CRBP1 and then oxidized to retinal by retinol dehydrogenase (RDH) (Figure 2). Retinal is formed also by conversion of ß-carotene through ß-carotene-15,15´-monooxygenase (BCO1). Retinal is then converted by two-step oxidation processes through retinal aldehyde dehydrogenases (RALDH1, RALDH2) to ATRA, which is the biologically active form of vitamin A. After binding to CRABP2 (cellular retinoid acid-binding protein 2) ATRA is shuttled to the nuclei where it binds to retinoid acid receptors (RARs). Global deletion of Raldh1 in mice has been reported to result in increased cortical thickness and a slight enhanced trabecular bone mass, indicating a deleterious effect by vitamin A on the skeleton (71). ATRA can also be shuttled to the nuclei after being bound to FABP5 (fatty acid-binding protein 5) and then ligated to PPARß/γ (peroxisome proliferator-activated receptor), another nuclear receptor which similar to RARs (see further below) dimerizes with retinoid X receptors (RXRs) (Figure 2).
The intracellular concentrations of ATRA are not only dependent on biosynthesis, but also on degradation. Enzymes in the cytochrome P450 family such as the enzyme CYP26B1 (cytochrome P450 family 26 subfamily B member 1) catalyze the degradation of ATRA to inactive, hydroxylated metabolites (72, 73)(Figure 2). Global deletion of Cyp26b1 in mice, or mutation of Cyp26b1 in zebrafish, causes multiple skeletal and craniofacial deformities (74, 75). Interestingly, some case reports from patients with different missense mutations in the CYP26B1 gene have shown the importance of this enzyme for skeletal and joint morphogenesis with a large number of skeletal and craniofacial deformities described in humans (75, 76). Several mutations are lethal, but some individuals survive beyond infancy and in these individuals, also the presence of gracile long bones have been described (77). Thus, mutations rendering CYP26B1 less catalytically active causing accumulation of ATRA intracellularly cause skeletal phenotypes similar to hypervitaminosis A.
Vitamin A receptor signaling
The discovery of vitamin A receptors followed studies investigating how other fat soluble hormones such as estrogen and glucocorticoids bind to intracellular proteins and DNA (78). Initially, a receptor which could bind retinoic acid (RAR) and with the capacity to function as a ligand-inducible transcription factor was described 1987 independently by Pierre Chambon and Ronald Evans (79, 80). Recently, the background for the discoveries have been described in two fascinating papers by each group (25, 26). Shortly afterwards, Pierre Chambon and Magnus Phfahl cloned a second RAR (81, 82). The initially described receptor was given the name RARα and the second RARß. Then, Chambon and colleagues cloned a third receptor designated RARγ (83). These three receptors are encoded by different genes in different chromosomes and due to alternative splicing several isoforms exist. Subsequent studies have shown the presence also of three related receptors, designated RXRα (84), RXRß (85) and RXRγ, which similar to RARs are encoded by different genes (86). The ligand binding domains in RARs and RXRs have only restricted homology, which explains why ATRA, the natural ligand for RARs, does not bind to RXR. 9-cis-retinoic acid is a high affinity ligand for both RARs and RXRs (87, 88), but since this compound is either undetectable or present in too low concentrations to activate RXR, 9-cis-retinoic acid is not likely to be an endogenous ligand for RXR. Recently, however, 9-cis-13,14-dihydroretinoic acid has been demonstrated to be an endogenous and physiologically relevant retinoid capable of activating RXRs (89). Interestingly, the two distinct retinoid receptor subfamilies were found to be closely related mechanistically since it was found that RXR was necessary for the DNA binding and transactivation by RAR. Not only was RXRs necessary for the activity of RARs but also for other nuclear receptors since RXRs act as co-factors for RARs, vitamin D receptor (VDR) and thyroid receptors (TR) by making up heterodimers with these receptors, a process necessary for DNA binding and transactivation of RARs, VDR and TR (90, 91). Later, it has been shown that RXRs make up heterodimers also with other nuclear receptors such as PPAR, Liver X receptor (LXR) and farnesoid X receptor (FXR) (92). This means that RXRs play a critical role for the activity of not only retinoids but also for certain other hormones since there will be a competition between ligands and receptors requiring RXRs as accessory factors.
The importance of the RARs for the physiological functions of vitamin A in vivo is supported by observations showing that mice deficient of Rara or Rarb (93, 94), as well as mice with Rara/Rarg double knockout (95) exhibit similar phenotypes as vitamin A deficient mice. The phenotypes observed in mice with deletion of Rara/Rarg is more severe and extensive compared to those found in vitamin A deficient mice, probably because of difficulties achieving absolute deprivation of vitamin A. In agreement with the well documented role for vitamin A in skeletal development, Rara/Rarb deficient mice have several abnormalities in craniofacial skeleton including lack of incisors, as well as malformations in vertebra and the appendicular skeleton (95). The phenotypes generated by deletion of Rxrs, however, have been difficult to interpret since RXRs heterodimerize with several other nuclear receptors such as RARs, PPARs, VDR, TR and LXR.
Experimental studies on effects by vitamin A on bone resorption and bone mass
Effects by vitamin A on bone resorption
As mentioned in the introduction, there are several studies in rodents showing that large amounts of retinoids in the chow, or retinoids administered orally, or by s.c. injections in rats (96–101) or mice (99, 102) result in decreased cortical thickness, periosteal circumference and strength in the long bones. The loss of bone has been attributed to an increased formation of periosteal osteoclasts (Figure 3A), which together with findings of enhanced levels of hydroxyproline in urine and TRAP5b in serum show that the loss of bone is due to increased bone resorption. Mechanistically, enhanced bone resorption is caused by increased RANKL/OPG ratio induced by ATRA (103). Decreased cortical bone mass caused by increased amounts of vitamin A is also indicted by the observation that deletion in mice of Raldh, the intracellular enzyme responsible for the final step in formation of ATRA, results in increased cortical thickness, although it could not be established if this was due to decreased bone resorption or enhanced bone formation (71). Interestingly, increased numbers of osteoclasts have been observed in a patient with homozygous mutation of CYB26B1, the enzyme responsible for degradation of ATRA (104).
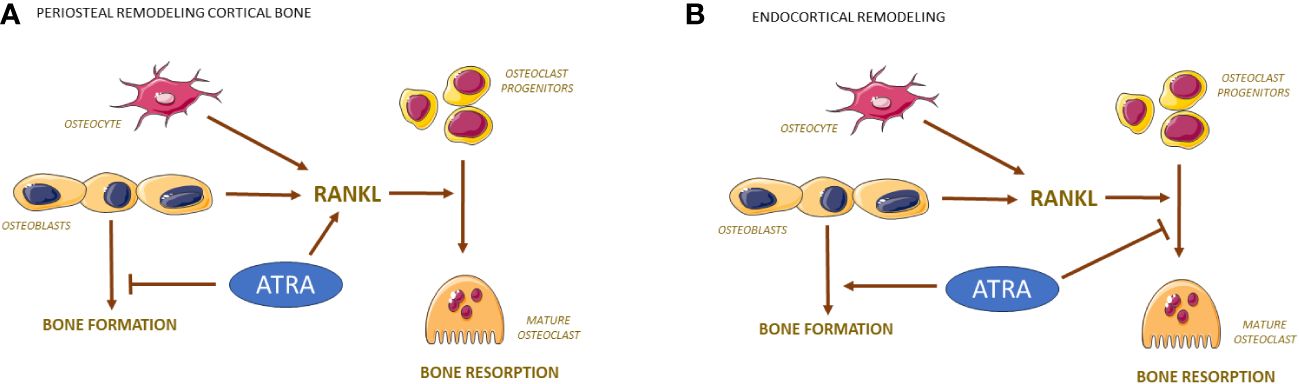
Figure 3 Bone resorption and formation are regulated differently by ATRA on periosteal and endocranial surfaces of bone. RANKL produced by osteocytes/osteoblasts activates the differentiation of mononuclear osteoclast progenitor cells to mature osteoclasts. On periosteal surfaces, ATRA induces the expression of RANKL causing enhanced number of osteoclasts while inhibiting the bone forming effects by osteoblasts (A). On endocortical surfaces, ATRA inhibits the differentiation of osteoclast progenitors causing decreased number of osteoclasts while stimulating bone formation by osteoblasts (B).
Studies aiming to elucidate if hypervitaminosis A also affects trabecular bone have yielded conflicting results. In rats, two studies have shown decreased trabecular bone mass in femur and tibia (100, 101), whereas three studies have not been able to observe any effect on trabecular bone mass in humerus or tibia (98, 99, 105). In mice fed with an increased vitamin A diet, Yorgan et al. observed reduced trabecular bone mass in vertebrae, associated with increased numbers of osteoclasts and decreased numbers of osteoblasts (106), whereas Lionikaite et al. could not observe any effect on trabecular bone mass in femur, tibia or vertebra in mice on increased vitamin A diet, although cortical bone mass was decreased (102, 107).
In only one study was investigated if hypervitaminosis A may affect also skull bones (108). The authors demonstrated that high dose of retinoids decreased the thickness of calvaria in rats, an effect due to both increased numbers of endocranial osteoclasts and decreased pericranial bone formation rate. Interestingly, also increased osteocyte lacuna was observed indicating that hypervitaminosis A may increase osteocytic osteolysis. In agreement with these experimental observations, thin skull bones has been described in young children suffering from vitamin A intoxication (109).
These studies unequivocally show that vitamin A strongly affects long bones causing decreased cortical bone mass, smaller bone with decreased diaphyseal diameter and decreased bone strength in rodents treated with high doses of retinoids for short period of time. It could, however, be questioned to what extent these results are of relevance for potential effects by vitamin A in humans with modest increases of vitamin A. With the aim to assess if also a lower dose of vitamin A would affect the skeleton, Johansson et al. fed rats with retinyl palmitate and retinyl acetate for seven days at a dose (1200 IU vitamin A/g pellet instead of 1700 IU/g pellet) which resulted in 18 times increased serum levels of retinyl ester from 270 to 5300 nM. It was observed that cortical bone area was still significantly decreased (98). In a more recent study, mice were fed with retinyl palmitate for 4 and 10 weeks with two doses of retinyl palmitate resulting in an increase of retinyl esters from 65 nM to 130 and 230 nM, respectively, after 10 weeks (102). By increasing serum retinyl esters 2 and 3.5 times, respectively, a dose- and time-dependent decrease of cortical thickness and periosteal circumference was observed, which was statistically significant at the highest dose. Linear regression analyses showed that cortical thickness in femur and periosteal circumference were significantly associated with the dosage of vitamin A. Since the effects were more pronounced after 10 than 4 weeks, the possibility exists that even larger effects could have been obtained if treatment time had been extended more than 10 weeks. Since the physiological levels of retinyl esters in humans are in the range of 50-200 nM (58, 59) these experiments indicate that clinically relevant doses of vitamin A have deleterious effects on the skeleton in mice.
Effects by vitamin A on bone formation
Most of the experimental studies in rodents have focused on effects by vitamin A on bone resorption. There are, however, some studies also assessing effects on bone formation. The decreased periosteal circumference by increased levels of vitamin A seems to be due not only to increased periosteal bone resorption, but also to decreased bone formation (Figure 3A). Dynamic histomorphometry has demonstrated decreased periosteal bone formation rate in rats given high doses of vitamin A (110), and in mice treated with considerably smaller doses of vitamin A (102).
Site specific effects by vitamin A on bone resorption and formation
Interestingly, vitamin A seems to have site specific effects on both bone formation and osteoclastogenesis. Decreased endocortical circumference and bone marrow area are observed in femur and tibia in both rats and mice with high and moderately increased doses of vitamin A (100, 102). This is to a large extent dependent on increased endocortical bone formation as assessed by both histology and dynamic histomorphometry (102, 104), but decreased osteoclast numbers on endocortical surfaces also contribute (Figure 3B) (100, 102). In contrast to the increase of endocortical bone formation, vitamin A decreases bone formation on periosteal surfaces on long bones and skull bones without affecting bone formation on endocranial and intracranial surfaces of the skull bones (102, 108, 110). The decrease of endocortical osteoclasts contrasts with the increased numbers of osteoclasts observed at periosteal surfaces in appendicular skeleton and endocranially in skull bones (99, 100, 102). Interestingly, vitamin A has no effect on osteoclasts numbers intracranially (108) in contrast to TNF-α, IL-1ß, PTH and 1,25(OH)2-vitamin D3, which decrease calvarial bone mass by increasing osteoclast numbers intracranially (111). Currently, it is not known the reason why vitamin A differently affects the response in osteoblasts and osteoclast in different parts of the skeleton.
Effects by vitamin A on loading induced bone mass
In one study, the possibility has been assessed that vitamin A may affect the increase of bone mass caused by mechanical loading (107). For this purpose, axial loading of tibiae in mice was applied on alternate days for two weeks resulting in an 43% increase of trabecular bone mass. This response was substantially reduced by feeding the mice with retinoid palmitate at a dose causing a modest increase of retinyl esters. Loading increased also cortical thickness and pericortical circumference and both responses were decreased by increasing the dosage of vitamin A. The reduced anabolic response in mice with increased dosage of vitamin A was due to decreased bone formation rate (Figure 4). These findings may have implications for the gain of bone mass achieved by physical activity in humans. The observations should prompt studies investigating if vitamin A may affect bone formation during fracture healing or induced by anabolic drugs used for treatment of osteoporosis.
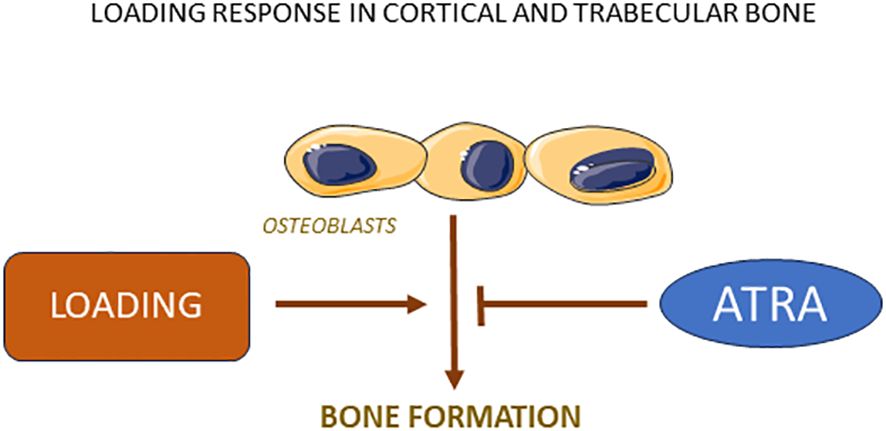
Figure 4 Loading stimulates bone formation in both cortical and trabecular bone due to increased osteoblast activity, a response which is robustly inhibited by ATRA.
Summary
In long bones, increasing amounts of vitamin A in rodents cause enhanced bone resorption and decreased bone formation on periosteal surfaces and decreased bone resorption and increased bone formation on endocortical surfaces, resulting in decreased cortical thickness, decreased diaphyseal diameter and enhanced fragility. In skull bones, bone density is decreased due to enhanced endocranial bone resorption, decreased precranial bone formation and enhanced osteocytic osteolysis. Increased bone mass caused by mechanical loading of long bones is reduced by vitamin A.
Although the relevance of experimental observations in rodents for human physiology and pathology should be discussed, the deleterious effects by increasing amounts of vitamin A for the skeleton in rats and mice, even at clinically relevant amounts, should be taken into account when evaluating possible associations between enhanced levels of vitamin A and osteoporosis in humans.
Epidemiological studies investigating effects by vitamin A on the skeleton
Pioneering studies in Sweden showed that increased dietary intake of retinol and increased serum levels of retinol were associated with decreased bone mineral density (BMD) and increased susceptibility to fractures (44, 112). Since then, many studies have been performed to investigate whether these studies can be confirmed in other cohorts, but the results are divergent. Some studies have reproduced the initial studies reporting a detrimental effect by vitamin A on bone, but other have found either no association or a beneficial effect (2, 113–115).
Vitamin A status is best assessed by quantifying liver stores, but for obvious reasons such assessments are not useful for clinical studies. In most studies, serum concentrations of retinol are used as a marker for vitamin A status, although retinol levels in serum are tightly regulated and best used only for assessments of deficiency or excess of vitamin A (116, 117). For these reasons, analysis of serum retinol is not recommended for use on an individual basis but is considered useful at the population basis (48). A more relevant marker of vitamin A status is serum levels of retinyl esters, but these analyses are less frequently used (118). Also analyses of ß-carotene in serum have been used in several studies, often in comparison to analyses of retinol. In addition to serum markers, several studies are based on assessments using questionnaires of dietary intake of retinoids, although the intake of vitamin A is difficult to assess since it may be associated with intake of many other nutrients which may not always be easy to adjust for.
Epidemiological studies indicating a harmful effect by vitamin A on the skeleton
Studies indicating a negative association between intake of vitamin A and bone mineral density
The first clinical investigation indicating a negative association between intake of vitamin A and BMD was the study by Melhus et al. showing that intake of retinol in 175 women (28-71 yrs) was associated with decreased BMD in femoral neck, lumbar spine and total body (Table 1) (44). Three other studies have also observed a negative association between intake of retinol and BMD or decrease of BMD over time in either femoral neck or lumbar spine, although this was not consistently found for all sites examined (Table 1) (119, 120, 122). In one study, the association was lost when vitamin A supplements were added (119). In another study was observed that in participants not using vitamin A supplements there was an positive association between dietary vitamin A intake and BMD, and changes of BMD in hip and lumbar spine during the 4-years follow-up, whereas intake of vitamin A was inversely related to BMD and BMD changes in those which used supplemental retinol (120).
In a Norwegian study enrolling 3,052 women, BMD in forearm was found to be higher in women who had not been given cod liver oil during childhood, compared to those which had been forced to consume the oil (Table 1) (121).
Interestingly, one study found that not only high intake of retinol, but also low intake of retinol was associated with low BMD and greater change of BMD over time (Table 1) (120).
Studies indicating a negative association between intake of vitamin A and fracture risk
Although low BMD, as assessed by DXA, is a well-established risk factor for osteoporotic fractures, the incidence of low-trauma fractures in spine, hip and forearm are the clinically most relevant symptoms, with hip fractures being the most serious event. In one study enrolling 1,124 women with 247 hip fracturs, high intake of retinol was associated with increased risk for fracture (Table 2) (44). In another study enrolling 1,221 men with 111 fractures at any site, those with highest quantile of retinol intake had increased risk for fracture compared to those in the lowest quantile (112). In the Nurse´s Health study enrolling 72,337 postmenopausal women with 603 incident hip fractures, highest quantile of total vitamin A intake or retinol intake was significantly associated with increased fracture rate (135). The risk was further enhanced in those taking vitamin A supplements. Lim et al. have also observed an increased hip fracture risk in those using vitamin A supplements although the intake of vitamin A or retinol was not associated with increased risk of hip fracture (140).
Studies indicating a negative association between serum levels of vitamin A and bone mineral density
Two studies have observed that high serum levels of retinol are associated with low BMD in lumbar spine, femoral neck or hip (Table 3) (141, 142). In one study, high maternal retinol was associated with low total body mineral content and bone area in offspring but, however, not with BMD (143).
Studies indicating a negative association between serum levels of vitamin A and fracture risk
A Swedish study enrolling 2,322 men and an American study enrolling 2,799 postmenopausal women, expiring 84 and 172 hip fractures, respectively, found that high serum retinol increased the risk of hip fractures (Table 4) (112, 149). Interestingly, an increased risk for hip fracture was also observed in one of the studies with low levels of serum retinol (149).
Epidemiological studies indicating no harmful effect by vitamin A on the skeleton
Studies indication no association between intake of vitamin A and bone mineral density
In three studies enrolling 2,016, 11,068 and 917 individuals, respectively, no association between intake of retinol or vitamin A and BMD at several sites (femoral neck, lumbar spine, total hip, total body) or decrease of BMD over time was observed (Table 1) (123–125). No association between retinol intake and BMD was also found in three studies with low number of individuals (126–128).
Total hip BMD was not found to be associated with multivitamin supplements in a study of 2,606 women older than 75 years of age (148). Similarly, Sowers et al. did not find any association between radial bone mass and use of vitamin A supplements (152).
In several studies, intake of ß-carotene has been separately assessed. In a metanalysis was concluded that ß-carotene intake was not associated with the overall risk of osteoporosis (most frequently assessed by analysis of BMD, but in some studies by fracture risk). In a subanalysis, however, there was a negative association between ß-carotene intake and osteoporosis in Asian women and men, which was not observed in the Western population (153).
Studies indicating no association between intake of vitamin A and fracture risk
In three studies enrolling 34,703, 75,747 and 34,696 individuals, respectively, there was no association between intake of retinol or vitamin A and fracture risk in hip or with non-hip fractures (Table 2) (136–138). Similar finding was made in a fourth smaller study (123). In one study, there was a 17% increased risk for hip fractures, but not for non-hip fractures, among the 12,293 vitamin A supplement users (136).
A Danish study did not find any increased risk of total fractures or fractures in forearm, hip and spine in patients treated with vitamin A analogues (isotretinoin, acitretin) (154).
Studies indicating no association between increased serum levels of vitamin A and bone mineral density
In one large study enrolling 5,790 women and men and in a smaller study with 59 postmenopausal women analyzing vitamin A status as retinyl esters as % of total vitamin A (retinol + retinyl esters) in serum, no association between vitamin A and BMD in lumbar spine and proximal femur could be observed (Table 3) (128, 144), similar to an old study analyzing serum retinol (152).
No association between maternal serum retinol and total body BMD in offsprings analyzed two weeks after birth (143).
Serum retinol and serum RBP4 have been analyzed in 78 young men 23 years of age and found not to be associated with BMD total body, lumbar spine or hip, indicating no association between serum levels of vitamin A and peak bone mass (145).
Studies indicating no association between serum levels of vitamin A and fracture risk
In an old study by Sowers et al., no association between serum levels of retinol and osteoporotic fractures was found (152). Three subsequent studies were also unable to find that increased serum levels of retinol are associated with increased risk for hip fracture och osteoporotic fractures in general (Table 4) (148, 150, 151). In two of the studies, the number of fractures were modest with 92 hip fractures and 54 osteoporotic fractures (148, 150) but the third study included 1,154 hip fractures (151). In the study by Holvik et al., however, there was a modest increased risk of hip fracture (RR 1.41) in the lowest quantile compared to the middle quantile of serum retinol (151).
Epidemiological studies investigating the relative roles of vitamin A and vitamin D för bone mineral density and fracture risk
In some studies, the potential interaction between vitamin A and D on bone mass and fracture has been investigated. One reason is that low levels of vitamin D are well recognized to be associated with decreased BMD and enhanced fracture risk. Another reason is that vitamin A interferes with vitamin D-dependent intestinal absorption of calcium (155). Since the receptor for vitamin D (VDR) and the RARs binding the biologically active metabolite of vitamin A (ATRA) dimerize with the same receptors (RXR) for regulating DNA transcription (2), it might be that vitamin A and D may interact to regulate BMD and fracture risk.
Although data from the Iowa Women´s Health Initiative Study enrolling 34,703 post-menopausal women did not find an association between retinol intake and fracture risk, such an association was observed in those with highest intake of retinol and low vitamin D (140). Similar observations were made by in a large cohort of 75,747 post-menopausal women participating in the Women´s Health Initiative Observational Study (137). In a Spanish study of 232 postmenopausal women, those with vitamin D-deficiency (25(OH)-D3 <20 ng/ml) and high serum retinol had substantially lower BMD compared to those with low serum retinol (136). In contrast, Holvik et al. could not observe any significant interaction between serum concentrations of 25(OH)-D3 and serum retinol on hip fractures (n=1,154) in a cohort of 21,774 Norwegian women and men (65-79 yrs) (151).
Epidemiological studies indicating a beneficial effect of vitamin A on the skeleton
Studies indicating a positive effect by vitamin A intake and bone mineral density
In contrast to studies showing a harmful or no effect by increased vitamin A on the skeleton, there are several studies reporting a beneficial effect (Table 1). In a large prospective cohort, The Rotterdam study with 2,172 men and 3,116 women older than 55 years, a positive association between femoral neck BMD and risk of all fractures in the subjects with highest quintile of total vitamin A or retinol intake was observed (129). Such association was not observed with the intake of ß-carotene. The positive association between vitamin A intake and fracture risk was lost after adjustment for BMD. Interestingly, the association between vitamin A intake and fracture risk was seen only in overweight subjects (BMI>25 kg/m2). It was speculated that vitamin A may be stored in fat cells and thereby protect the skeleton from harmful effects by vitamin A, but it was also suggested that subjects with high BMI have larger BMD due to more mechanical loading. No significant associations were observed between dietary intake of vitamin D, or plasma levels of vitamin D, and BMD or fracture risk.
Similar to the findings by de Jonge et al., a positive association between vitamin A intake and BMD of total hip, femoral neck and lumbar spine has been observed in two other studies enrolling 6,481 and 3,154 individuals, respectively(Table 1) (130, 131). Also in four other studies with fewer number of individuals, a positive effect on BMD by vitamin A intake has been observed (126, 132–134).
Studies indicating a positive effect by vitamin A intake and fracture risk
One study has found that high intake of retinol is associated with decreased hip fracture risk (Table 2) (139).
Studies indicating a positive effect by increased serum retinol on bone mineral density
There are two large studies enrolling 2,606 women and 3,154 women and men, respectively, which have found that serum retinol is positively associated with total hip BMD (Table 3) (131, 148). Also two smaller study have observed that serum retinol is associated with femoral neck BMD (146, 147).
Meta-analyses of the association between vitamin A and fracture susceptibility
In one meta-analysis examining the relationship between fracture risk and vitamin A (156), eight prospective studies were included in which data for intake of vitamin A, retinol or ß-carotene were available (44, 123, 135, 137, 138, 140, 157, 158). In two of these studies, only data for intake of ß-carotene was available (157, 158). In the four studies where intake of vitamin A or retinol had been recorded the numbers of hip fractures were 247, 603, 525 and 588 (44, 135, 137, 140). In three studies, the numbers of total fractures were 163, 1040 and 1898 (123, 137, 138). The analyses showed that high intake of vitamin A and retinol increased the risk of hip fracture with an adjusted relative risk of 1.29 and 1.40, respectively, whereas high intake of ß-carotene did not increase the risk of hip fracture. High intake of vitamin A and retinol did not affect the risk of total fracture.
Four prospective studies were identified in which serum retinol levels and fractures were available (112, 148–150). In three studies in which serum retinol and hip fractures were available, the numbers of hip fractures were 84, 92 and 172 (112, 148, 149) and in three studies data for serum retinol and total numbers of fractures (123, 266 and 312) were available (112, 148, 150). When analyzing relationship between serum levels of retinol and hip fracture the results demonstrated a U-shaped relationship with increased relative risk at both low (RR 1.56) and high (RR 1.87) levels of retinol, with no association for total fractures. The authors calculate that serum retinol concentration between 1.99 and 2.31 µmol/l seems to be optimal for bone health.
In a second meta-analyses by Zhang et al. (159), published three years after the study by Wu et al. (156), also eight studies examining the relationship between fracture risk and intake of vitamin A, retinol and ß-carotene were included (44, 123, 129, 135, 137, 138, 140, 158). One of the studies in the meta-analysis by Wu et al. was missing in this study (157), but a study by de Jonge et al. was included (published after the meta-analyses by Wu et al.) (129). In the meta-analyses by Zhang et al., higher intake of vitamin A and retinol was found to be associated with an increase of hip fracture risk (RR 1.29 and 1.40, respectively), similar to the analyses by Wu et al. In contrast, high intake of vitamin A and retinol was associated with a slightly decrease of total fracture risk (RR 0.94 and 0.95, respectively); no such association was observed by Wu et al.
The analysis of a relationship between fracture risk and serum levels of retinol included five studies (112, 148–151) of which four were identical to the studies included by Wu et al. (156). One more study by Holvik et al. was included (151), which was published after the analyses by Wu et al. Like Wu et al., the authors found that low levels of serum retinol slightly increased risk of total fracture (RR 1.11) or hip fracture (RR 1.27), but in contrast to Wu et al., no significant association between high serum retinol and fracture risk was found which may be due to the inclusion of the large study by Holvik et al. with 1,154 fractures which did not find any association between serum high serum retinol and hip fracture risk but a modest increased risk at low serum retinol (151).
Interestingly, subgroup analysis of retinol intake and total fracture risk showed that women have a larger fracture risk compared to a subgroup of men and mixed gender and that Europeans have a lower fracture risk than Americans.
Comments
It is apparent that there is no consensus regarding the potential role of dietary intake of vitamin A and BMD. One problem with these studies is that there is usually a substantial difference in time between the dietary assessments and the measurements of BMD. Another issue is that intake of vitamin A may be associated with intake of other nutrients which are difficult to adjust for. Some studies indicate that the intake of supplements containing retinol affects the outcome and this is not taken into consideration in all studies. Meta-analyses have found that also low intake of vitamin A is associated with low BMD although this has not been observed in all studies. This brings into question if differences between studies might be influenced by differences in the use of supplements and the degree of fortification of cereals and dairy products in different countries. The absorption and metabolism of retinyl esters and ß-carotene as well as the response in target cells are regulated by several enzymes, binding proteins and receptors which all are modulated by genetic variations in the genes encoding these proteins which all most likely influence an interindividual variability in the bioavailability and response to vitamin A. Although not very much studied, genetic variations in genes encoding RBP4, TTR and BCO1 have been shown to be associated with fasting serum retinol levels (48).
Although preclinical studies have clearly demonstrated the harmful effect on cortical bone mass and strength, it is also apparent that there is no consensus regarding the associations between serum retinol and BMD or hip fracture risk in clinical studies. Two meta-analyses have found that low serum retinol is associated with increased hip fracture susceptibility, but in only one of these analyses an association between hip fracture risk and high serum retinol was observed. It should be pointed out that these meta-analyses are based upon few numbers of publications with rather few numbers of hip fractures included. There is obviously a need for more well-powered investigations, which also would make it possible to perform stratified analyses and to determine possible sex and age specific associations and to analyze if there is a threshold for serum retinol and putative negative associations with fracture susceptibility. Since observed associations are very informative but cannot prove causality, there is also a need to use genetic instruments for the serum levels of retinol and to perform 2-sample Mendelian Randomization studies to determine their causal association with BMD and fracture risk.
The observation demonstrating that the bone anabolic response to mechanical loading in mice is decreased by vitamin A should be confirmed in other studies and extended by studies aiming to elucidate the potential effect by vitamin A on the response in the skeleton to physical activities and to investigate if vitamin A affects forced bone formation induced during bone fracture healing or by treatment of osteoporotic patients with bone anabolic drugs.
Although one study failed to observe an association between serum retinol and BMD in young men (23 yrs of age) (145), the importance for the growing skeleton of an adequate intake of vitamin A is indicated by recent observations in 426 Chinese children (6-9 yrs) showing that serum retinol and BMD are related by an invert U-shaped association. The existence of a reflection point shows the importance of not overdosing the intake of vitamin A (160).
Author contributions
UL: Conceptualization, Writing – original draft, Writing – review & editing.
Funding
The author(s) declare financial support was received for the research, authorship, and/or publication of this article. Studies in the author´s laboratory have been supported by fundings from the Swedish Research Council, the Marie Curie Initial Training Network (FP7-People-2013-INT::#607446), the ALF/LUA research grant from the Sahlgrenska University Hospital in Gothenburg, the IngaBritt and Arne Lundberg Foundation.
Conflict of interest
The author declares that the research was conducted in the absence of any commercial or financial relationships that could be construed as a potential conflict of interest.
The author(s) declared that they were an editorial board member of Frontiers, at the time of submission. This had no impact on the peer review process and the final decision.
Publisher’s note
All claims expressed in this article are solely those of the authors and do not necessarily represent those of their affiliated organizations, or those of the publisher, the editors and the reviewers. Any product that may be evaluated in this article, or claim that may be made by its manufacturer, is not guaranteed or endorsed by the publisher.
References
1. Olsen T, Blomhoff R. Retinol, retinoic acid, and retinol-binding protein 4 are differentially associated with cardiovascular disease, type 2 diabetes, and obesity: an overview of human studies. Adv Nutr. (2020) 11:644–66. doi: 10.1093/advances/nmz131
2. Conaway HH, Henning P, Lerner UH. Vitamin a metabolism, action, and role in skeletal homeostasis. Endocr Rev. (2013) 34:766–97. doi: 10.1210/er.2012-1071
3. Henning P, Conaway HH, Lerner UH. Retinoid receptors and their role in bone remodeling. Front Endocrinol (Lausanne). (2015). 6:31. doi: 10.3389/fendo.2015.00031
4. Balmer JE, Blomhoff R. Gene expression regulation by retinoic acid. J Lipid Res. (2002) 43:1773–808. doi: 10.1194/jlr.R100015-JLR200
5. Wirth JP, Petry N, Tanumihardjo SA, Rogers LM, McLean E, Greig A, et al. Vitamin A supplementation programs and country-level evidence of vitamin A deficiency. Nutrients. (2017) 9:1–18. doi: 10.3390/nu9030190
6. Semba RD. On the 'discovery' of vitamin A. Ann Nutr Metab. (2012) 61:192–8. doi: 10.1159/000343124
7. Lunin N. Über die Bedeutung der anorganischen Salze für die Ernährung des Thieres. Zeitsch Physiol Chimie. (1881) 5:31–9.
8. Socin A. In welcher Form wird das Eisen resorbirt? Zeitsch Physiol Chimie. (1891) 15:93–139. doi: 10.1515/bchm1.1891.15.2.93
9. Knapp P. Experimenteller Beitrag zur Ernährung von Ratten mit künstlicher Nahrung und zum Zusammenhang von Ernährungsstörungen mit Erkrankungen der Conjunctiva. Zeitsch Exp Pathol Therap. (1909) 5:147–69.
10. Hopkins FG. Feeding experiments illustrating the importance of accessory factors in normal dietaries. J Physiol. (1912) 44:425–60. doi: 10.1113/jphysiol.1912.sp001524
11. Funk C. The etiology of the deficiency diseases, Beri-beri, polyneuritis in birds, epidemic dropsy, scurvy, experimental scurvy in animals, infantile scurvy, ship beri-beri, pellagre. J State Med. (1912) 20:341–68.
12. Moore T. Vitamin A and carotene. VI. The conversion of carotene to vitamin A in vivo. Biochem J. (1930) 24:692–702. doi: 10.1042/bj0240692
13. Karrer P, Morf R, Pflanzenfarbstoffe XXXV. Zur konstitution des {{ι}}β{{/ι}}-carotins and -hydrocarotins. Helv Chim Acta. (1931) 14:1033–6. doi: 10.1002/hlca.19310140510
14. Karrer P, Morf R, Schöpp K. Zur Kenntnis des Vitamins-A aus Fischtranen. Helv Chim Acta. (1931) 14:1036–40. doi: 10.1002/hlca.19310140511
15. Holmes HN, Corbet RE. A crystalline vitamin a concentrate. Science. (1937) 85:103. doi: 10.1126/science.85.2195.103
16. Arens JF, van Dorp DA. Synthesis of some compounds possessing vitamin A activity. Nature. (1946) 157:190–1. doi: 10.1038/157190a0
17. Isler OH, Ronco A, Kofler M. Synthese des vitamin A. Helv Chim Acta. (1947) 30:1911–21. doi: 10.1002/hlca.19470300666
18. Wald G. Molecular basis of visual excitation. Science. (1968) 162:230–9. doi: 10.1126/science.162.3850.230
19. Emerick RJ, Zile M, DeLuca HF. Formation of retinoic acid from retinol in the rat. Biochem J. (1967) 102:606–11. doi: 10.1042/bj1020606
20. Ito YL, Zile M, Ahrens H, DeLuca HF. Liquid-gel partition chromatography of vitamin A compounds; formation of retinoic acid from retinyl acetate in vivo. J Lipid Res. (1974) 15:517–24. doi: 10.1016/S0022-2275(20)36772-9
21. Strickland S, Mahdavi V. The induction of differentiation in teratocarcinoma stem cells by retinoic acid. Cell. (1978) 15:393–403. doi: 10.1016/0092-8674(78)90008-9
22. Kedishvili NY. Enzymology of retinoic acid biosynthesis and degradation. J Lipid Res. (2013) 54:1744–60. doi: 10.1194/jlr.R037028
23. O'Byrne SM, Blaner WS. Retinol and retinyl esters: biochemistry and physiology. J Lipid Res. (2013) 54:1731–43. doi: 10.1194/jlr.R037648
24. di Masi A, Leboffe L, De Marinis E, Pagano F, Cicconi L, Rochette-Egly C, et al. Retinoic acid receptors: from molecular mechanisms to cancer therapy. Mol Aspects Med. (2015) 41:1–115. doi: 10.1016/j.mam.2014.12.003
25. Giguere V, Evans RM. Chronicle of a discovery: the retinoic acid receptor. J Mol Endocrinol. (2022) 69:T1–T11. doi: 10.1530/JME-22-0117
26. Petkovich M, Chambon P. Retinoic acid receptors at 35 years. J Mol Endocrinol. (2022) 69:T13–24. doi: 10.1530/JME-22-0097
27. Germain P, Chambon P, Eichele G, Evans RM, Lazar MA, Leid M, et al. International Union of Pharmacology. LX. Retinoic acid receptors. Pharmacol Rev. (2006) 58:712–25. doi: 10.1124/pr.58.4.4
28. Maden M, Hind M. Retinoic acid, a regeneration-inducing molecule. Dev Dyn. (2003) 226:237–44. doi: 10.1002/dvdy.10222
29. Gudas LJ. Retinoids induce stem cell differentiation via epigenetic changes. Semin Cell Dev Biol. (2013) 24:701–5. doi: 10.1016/j.semcdb.2013.08.002
30. Zardo G, Cimino G, Nervi C. Epigenetic plasticity of chromatin in embryonic and hematopoietic stem/progenitor cells: therapeutic potential of cell reprogramming. Leukemia. (2008) 22:1503–18. doi: 10.1038/leu.2008.141
31. Manicassamy S, Ravindran R, Deng J, Oluoch H, Denning TL, Kasturi SP, et al. Toll-like receptor 2-dependent induction of vitamin A-metabolizing enzymes in dendritic cells promotes T regulatory responses and inhibits autoimmunity. Nat Med. (2009) 15:401–9. doi: 10.1038/nm.1925
32. Mora JR, Iwata M, von Andrian UH. Vitamin effects on the immune system: vitamins A and D take centre stage. Nat Rev Immunol. (2008) 8:685–98. doi: 10.1038/nri2378
33. Carazo A, Macakova K, Matousova K, Krcmova LK, Protti M, Mladenka P. Vitamin A update: forms, sources, kinetics, detection, function, deficiency, therapeutic use and toxicity. Nutrients. (2021) 13:1–36. doi: 10.3390/nu13051703
34. Tallman MS, Andersen JW, Schiffer CA, Appelbaum FR, Feusner JH, Ogden A, et al. All-trans-retinoic acid in acute promyelocytic leukemia. N Engl J Med. (1997) 337:1021–8. doi: 10.1056/NEJM199710093371501
35. Lam HS, Chow CM, Poon WT, Lai CK, Chan KC, Yeung WL, et al. Risk of vitamin A toxicity from candy-like chewable vitamin supplements for children. Pediatrics. (2006) 118:820–4. doi: 10.1542/peds.2006-0167
36. Davies AW, Moore T. Vitamin A and carotene: The distribution of vitamin A in the organs of the normal and hypervitaminotic rat. Biochem J. (1934) 28:288–95. doi: 10.1042/bj0280288
38. Wolbach SB. Vitamin-A deficiency and excess in relation to skeletal growth. J Bone Joint Surg Am. (1947) 29:171–92.
39. Maddock CL, Wolbach SB, Maddock S. Hypervitaminosis A in the dog. J Nutr. (1949) 39:117–37. doi: 10.1093/jn/39.1.117
40. Barnicot NA. Local action of calciferol and vitamin A on bone. Nature. (1948) 162:848. doi: 10.1038/162848a0
41. Fell HB, Mellanby E. Effects of hypervitaminosis A on foetal mouse bones cultivated in vitro; preliminary communication. Br Med J. (1950) 2:535–9. doi: 10.1136/bmj.2.4678.535
42. Fell HB, Mellanby E. The effect of hypervitaminosis A on embryonic limb bones cultivated in vitro. J Physiol. (1952) 116:320–49. doi: 10.1113/jphysiol.1952.sp004708
43. Binkley N, Krueger D. Hypervitaminosis A and bone. Nutr Rev. (2000) 58:138–44. doi: 10.1111/j.1753-4887.2000.tb01848.x
44. Melhus H, Michaelsson K, Kindmark A, Bergstrom R, Holmberg L, Mallmin H, et al. Excessive dietary intake of vitamin A is associated with reduced bone mineral density and increased risk for hip fracture. Ann Intern Med. (1998) 129:770–8. doi: 10.7326/0003-4819-129-10-199811150-00003
45. Borel P. Factors affecting intestinal absorption of highly lipophilic food microconstituents (fat-soluble vitamins, carotenoids and phytosterols). Clin Chem Lab Med. (2003) 41:979–94. doi: 10.1515/CCLM.2003.151
46. Lobo GP, Hessel S, Eichinger A, Noy N, Moise AR, Wyss A, et al. ISX is a retinoic acid-sensitive gatekeeper that controls intestinal beta,beta-carotene absorption and vitamin A production. FASEB J. (2010) 24:1656–66. doi: 10.1096/fj.09-150995
47. Ong DE, Page DL. Cellular retinol-binding protein (type two) is abundant in human small intestine. J Lipid Res. (1987) 28:739–45. doi: 10.1016/S0022-2275(20)38669-7
48. Borel P, Desmarchelier C. Genetic variations associated with vitamin A status and vitamin A bioavailability. Nutrients. (2017) 9:1–17. doi: 10.3390/nu9030246
49. Ong DE. Purification and partial characterization of cellular retinol-binding protein from human liver. Cancer Res. (1982) 42:1033–7.
50. Ong DE, Crow JA, Chytil F. Radioimmunochemical determination of cellular retinol- and cellular retinoic acid-binding proteins in cytosols of rat tissues. J Biol Chem. (1982) 257:13385–9. doi: 10.1016/S0021-9258(18)33460-4
51. Ong DE, MacDonald PN, Gubitosi AM. Esterification of retinol in rat liver. Possible participation by cellular retinol-binding protein and cellular retinol-binding protein II. J Biol Chem. (1988) 263:5789–96. doi: 10.1016/S0021-9258(18)60635-0
52. van Bennekum AM, Wei S, Gamble MV, Vogel S, Piantedosi R, Gottesman M, et al. Biochemical basis for depressed serum retinol levels in transthyretin-deficient mice. J Biol Chem. (2001) 276:1107–13. doi: 10.1074/jbc.M008091200
53. Tanumihardjo SA, Russell RM, Stephensen CB, Gannon BM, Craft NE, Haskell MJ, et al. Biomarkers of nutrition for development (BOND)-vitamin A review. J Nutr. (2016) 146:1816S–48S. doi: 10.3945/jn.115.229708
54. Herbeth B, Zittoun J, Miravet L, Bourgeay-Causse M, Carre-Guery G, Delacoux E, et al. Reference intervals for vitamins B1, B2, E, D, retinol, beta-carotene, and folate in blood: usefulness of dietary selection criteria. Clin Chem. (1986) 32:1756–9. doi: 10.1093/clinchem/32.9.1756
55. Chuwers P, Barnhart S, Blanc P, Brodkin CA, Cullen M, Kelly T, et al. The protective effect of beta-carotene and retinol on ventilatory function in an asbestos-exposed cohort. Am J Respir Crit Care Med. (1997) 155:1066–71. doi: 10.1164/ajrccm.155.3.9116988
56. Redlich CA, Chung JS, Cullen MR, Blaner WS, Van Bennekum AM, Berglund L. Effect of long-term beta-carotene and vitamin A on serum cholesterol and triglyceride levels among participants in the Carotene and Retinol Efficacy Trial (CARET). Atherosclerosis. (1999) 143:427–34. doi: 10.1016/S0021-9150(98)00303-7
57. Relas H, Gylling H, Miettinen TA. Effect of stanol ester on postabsorptive squalene and retinyl palmitate. Metabolism. (2000) 49:473–8. doi: 10.1016/S0026-0495(00)80011-6
58. Krasinski SD, Russell RM, Otradovec CL, Sadowski JA, Hartz SC, Jacob RA, et al. Relationship of vitamin A and vitamin E intake to fasting plasma retinol, retinol-binding protein, retinyl esters, carotene, alpha-tocopherol, and cholesterol among elderly people and young adults: increased plasma retinyl esters among vitamin A-supplement users. Am J Clin Nutr. (1989) 49:112–20. doi: 10.1093/ajcn/49.1.112
59. Ballew C, Bowman BA, Russell RM, Sowell AL, Gillespie C, National Health Nutrition Examination Survey. Serum retinyl esters are not associated with biochemical markers of liver dysfunction in adult participants in the third National Health and Nutrition Examination Survey (NHANES III), 1988–1994. Am J Clin Nutr. (2001) 73:934–40. doi: 10.1093/ajcn/73.5.934
60. Arnhold T, Tzimas G, Wittfoht W, Plonait S, Nau H. Identification of 9-cis-retinoic acid, 9,13-di-cis-retinoic acid, and 14-hydroxy-4,14-retro-retinol in human plasma after liver consumption. Life Sci. (1996) 59:PL169–77. doi: 10.1016/0024-3205(96)00408-0
61. Cifelli CJ, Green JB, Wang Z, Yin S, Russell RM, Tang G, et al. Kinetic analysis shows that vitamin A disposal rate in humans is positively correlated with vitamin A stores. J Nutr. (2008) 138:971–7. doi: 10.1093/jn/138.5.971
62. During A, Hussain MM, Morel DW, Harrison EH. Carotenoid uptake and secretion by CaCo-2 cells: beta-carotene isomer selectivity and carotenoid interactions. J Lipid Res. (2002) 43:1086–95. doi: 10.1194/jlr.M200068-JLR200
63. During A, Harrison EH. Mechanisms of provitamin A (carotenoid) and vitamin A (retinol) transport into and out of intestinal Caco-2 cells. J Lipid Res. (2007) 48:2283–94. doi: 10.1194/jlr.M700263-JLR200
64. During A, Harrison EH. Intestinal absorption and metabolism of carotenoids: insights from cell culture. Arch Biochem Biophys. (2004) 430:77–88. doi: 10.1016/j.abb.2004.03.024
65. Kawaguchi R, Yu J, Honda J, Hu J, Whitelegge J, Ping P, et al. A membrane receptor for retinol binding protein mediates cellular uptake of vitamin A. Science. (2007) 315:820–5. doi: 10.1126/science.1136244
66. Blaner WS. STRA6, a cell-surface receptor for retinol-binding protein: the plot thickens. Cell Metab. (2007) 5:164–6. doi: 10.1016/j.cmet.2007.02.006
67. Ruiz A, Mark M, Jacobs H, Klopfenstein M, Hu J, Lloyd M, et al. Retinoid content, visual responses, and ocular morphology are compromised in the retinas of mice lacking the retinol-binding protein receptor, STRA6. Invest Ophthalmol Vis Sci. (2012) 53:3027–39. doi: 10.1167/iovs.11-8476
68. Quadro L, Blaner WS, Salchow DJ, Vogel S, Piantedosi R, Gouras P, et al. Impaired retinal function and vitamin A availability in mice lacking retinol-binding protein. EMBO J. (1999) 18:4633–44. doi: 10.1093/emboj/18.17.4633
69. Song I, Choi YJ, Jin Y, Kim JW, Koh JT, Ji HM, et al. STRA6 as a possible candidate gene for pathogenesis of osteoporosis from RNAseq analysis of human mesenchymal stem cells. Mol Med Rep. (2017) 16:4075–81. doi: 10.3892/mmr.2017.7072
70. Noy N. The ionization behavior of retinoic acid in lipid bilayers and in membranes. Biochim Biophys Acta. (1992) 1106:159–64. doi: 10.1016/0005-2736(92)90234-D
71. Nallamshetty S, et al. Deficiency of retinaldehyde dehydrogenase 1 induces BMP2 and increases bone mass in vivo. PloS One. (2013) 8:e71307. doi: 10.1371/journal.pone.0071307
72. Duester G. Retinoic acid synthesis and signaling during early organogenesis. Cell. (2008) 134:921–31. doi: 10.1016/j.cell.2008.09.002
73. White JA, Beckett-Jones B, Guo YD, Dilworth FJ, Bonasoro J, Jones G, et al. cDNA cloning of human retinoic acid-metabolizing enzyme (hP450RAI) identifies a novel family of cytochromes P450. J Biol Chem. (1997) 272:18538–41. doi: 10.1074/jbc.272.30.18538
74. Yashiro K, Zhao X, Uehara M, Yamashita K, Nishijima M, Nishino J, et al. Regulation of retinoic acid distribution is required for proximodistal patterning and outgrowth of the developing mouse limb. Dev Cell. (2004) 6:411–22. doi: 10.1016/S1534-5807(04)00062-0
75. Laue K, Pogoda HM, Daniel PB, van Haeringen A, Alanay Y, von Ameln S, et al. Craniosynostosis and multiple skeletal anomalies in humans and zebrafish result from a defect in the localized degradation of retinoic acid. Am J Hum Genet. (2011) 89:595–606. doi: 10.1016/j.ajhg.2011.09.015
76. Morton JE, Frentz S, Morgan T, Sutherland-Smith AJ, Robertson SP, et al. Biallelic mutations in CYP26B1: A differential diagnosis for Pfeiffer and Antley-Bixler syndromes. Am J Med Genet A. (2016) 170:2706–10. doi: 10.1002/ajmg.a.37804
77. Grand K, Skraban CM, Cohen JL, Dowsett L, Mazzola S, Tarpinian J, et al. Nonlethal presentations of CYP26B1-related skeletal anomalies and multiple synostoses syndrome. Am J Med Genet A. (2021) 185:2766–75. doi: 10.1002/ajmg.a.62387
78. Benbrook DM, Chambon P, Rochette-Egly C, Asson-Batres MA. History of retinoic acid receptors. Subcell Biochem. (2014) 70:1–20. doi: 10/1007/978-94-017-9050-5¬_1
79. Petkovich M, Brand NJ, Krust A, Chambon P. A human retinoic acid receptor which belongs to the family of nuclear receptors. Nature. (1987) 330:444–50. doi: 10.1038/330444a0
80. Giguere V, Ong ES, Segui P, Evans RM. Identification of a receptor for the morphogen retinoic acid. Nature. (1987) 330:624–9. doi: 10.1038/330624a0
81. Benbrook D, Lernhardt E, Pfahl M. A new retinoic acid receptor identified from a hepatocellular carcinoma. Nature. (1988) 333:669–72. doi: 10.1038/333669a0
82. Brand N, Petkovich M, Krust A, Chambon P, de The H, Marchio A, et al. Identification of a second human retinoic acid receptor. Nature. (1988) 332:850–3. doi: 10.1038/332850a0
83. Zelent A, Krust A, Petkovich M, Kastner P, Chambon P. Cloning of murine alpha and beta retinoic acid receptors and a novel receptor gamma predominantly expressed in skin. Nature. (1989) 339:714–7. doi: 10.1038/339714a0
84. Mangelsdorf DJ, Ong ES, Dyck JA, Evans RM. Nuclear receptor that identifies a novel retinoic acid response pathway. Nature. (1990) 345:224–9. doi: 10.1038/345224a0
85. Hamada K, Gleason SL, Levi BZ, Hirschfeld S, Appella E, Ozato K. H-2RIIBP, a member of the nuclear hormone receptor superfamily that binds to both the regulatory element of major histocompatibility class I genes and the estrogen response element. Proc Natl Acad Sci U.S.A. (1989) 86:8289–93. doi: 10.1073/pnas.86.21.8289
86. Mangelsdorf DJ, Borgmeyer U, Heyman RA, Zhou JY, Ong ES, Oro AE, et al. Characterization of three RXR genes that mediate the action of 9-cis retinoic acid. Genes Dev. (1992) 6:329–44. doi: 10.1101/gad.6.3.329
87. Heyman RA, Mangelsdorf DJ, Dyck JA, Stein RB, Eichele G, Evans RM, et al. 9-cis retinoic acid is a high affinity ligand for the retinoid X receptor. Cell. (1992) 68:397–406. doi: 10.1016/0092-8674(92)90479-V
88. Levin AA, Sturzenbecker LJ, Kazmer S, Bosakowski T, Huselton C, Allenby G, et al. 9-cis retinoic acid stereoisomer binds and activates the nuclear receptor RXR alpha. Nature. (1992) 355:359–61. doi: 10.1038/355359a0
89. Ruhl R, Krzyzosiak A, Niewiadomska-Cimicka A, Rochel N, Szeles L, Vaz B, et al. 9-cis-13,14-dihydroretinoic acid is an endogenous retinoid acting as RXR ligand in mice. PloS Genet. (2015) 11:e1005213. doi: 10.1371/journal.pgen.1005213
90. Yu VC, Delsert C, Andersen B, Holloway JM, Devary OV, Naar AM, et al. RXR beta: a coregulator that enhances binding of retinoic acid, thyroid hormone, and vitamin D receptors to their cognate response elements. Cell. (1991) 67:1251–66. doi: 10.1016/0092-8674(91)90301-E
91. Leid M, Kastner P, Lyons R, Nakshatri H, Saunders M, Zacharewski T, et al. Purification, cloning, and RXR identity of the HeLa cell factor with which RAR or TR heterodimerizes to bind target sequences efficiently. Cell. (1992) 68:377–95. doi: 10.1016/0092-8674(92)90478-U
92. Mangelsdorf DJ, Evans RM. The RXR heterodimers and orphan receptors. Cell. (1995) 83:841–50. doi: 10.1016/0092-8674(95)90200-7
93. Lufkin T, Lohnes D, Mark M, Dierich A, Gorry P, Gaub MP, et al. High postnatal lethality and testis degeneration in retinoic acid receptor alpha mutant mice. Proc Natl Acad Sci U.S.A. (1993) 90:7225–9. doi: 10.1073/pnas.90.15.7225
94. Luo J, Pasceri P, Conlon RA, Rossant J, Giguere V. Mice lacking all isoforms of retinoic acid receptor beta develop normally and are susceptible to the teratogenic effects of retinoic acid. Mech Dev. (1995) 53:61–71. doi: 10.1016/0925-4773(95)00424-6
95. Lohnes D, Mark M, Mendelsohn C, Dolle P, Dierich A, Gorry P, et al. Function of the retinoic acid receptors (RARs) during development (I). Craniofacial and skeletal abnormalities in RAR double mutants. Development. (1994) 120:2723–48. doi: 10.1242/dev.120.10.2723
96. Trechsel U, Stutzer A, Fleisch H. Hypercalcemia induced with an arotinoid in thyroparathyroidectomized rats. New model to study bone resorption in vivo. J Clin Invest. (1987) 80:1679–86. doi: 10.1172/JCI113257
97. Hough S, Avioli LV, Muir H, Gelderblom D, Jenkins G, Kurasi H, et al. Effects of hypervitaminosis A on the bone and mineral metabolism of the rat. Endocrinology. (1988) 122:2933–9. doi: 10.1210/endo-122-6-2933
98. Johansson S, Lind PM, Hakansson H, Oxlund H, Orberg J, Melhus H. Subclinical hypervitaminosis A causes fragile bones in rats. Bone. (2002) 31:685–9. doi: 10.1016/S8756-3282(02)00910-9
99. Kneissel M, Studer A, Cortesi R, Susa M. Retinoid-induced bone thinning is caused by subperiosteal osteoclast activity in adult rodents. Bone. (2005) 36:202–14. doi: 10.1016/j.bone.2004.11.006
100. Lind T, Lind PM, Jacobson A, Hu L, Sundqvist A, Risteli J, et al. High dietary intake of retinol leads to bone marrow hypoxia and diaphyseal endosteal mineralization in rats. Bone. (2011) 48:496–506. doi: 10.1016/j.bone.2010.10.169
101. Wray AE, Okita N, Ross AC. Cortical and trabecular bone, bone mineral density, and resistance to ex vivo fracture are not altered in response to life-long vitamin A supplementation in aging rats. J Nutr. (2011) 141:660–6. doi: 10.3945/jn.110.132126
102. Lionikaite V, Gustafsson KL, Westerlund A, Windahl SH, Koskela A, Tuukkanen J, et al. Clinically relevant doses of vitamin A decrease cortical bone mass in mice. J Endocrinol. (2018) 239:389–402. doi: 10.1530/JOE-18-0316
103. Conaway HH, Pirhayati A, Persson E, Pettersson U, Svensson O, Lindholm C, et al. Retinoids stimulate periosteal bone resorption by enhancing the protein RANKL, a response inhibited by monomeric glucocorticoid receptor. J Biol Chem. (2011) 286:31425–36. doi: 10.1074/jbc.M111.247734
104. Lind T, Lugano R, Gustafson AM, Norgard M, van Haeringen A, Dimberg A, et al. Bones in human CYP26B1 deficiency and rats with hypervitaminosis A phenocopy Vegfa overexpression. Bone Rep. (2018) 9:27–36. doi: 10.1016/j.bonr.2018.06.006
105. Green AC, Kocovski P, Jovic T, Walia MK, Chandraratna RAS, Martin TJ, et al. Retinoic acid receptor signalling directly regulates osteoblast and adipocyte differentiation from mesenchymal progenitor cells. Exp Cell Res. (2017) 350:284–97. doi: 10.1016/j.yexcr.2016.12.007
106. Yorgan TA, Heckt T, Rendenbach C, Helmis C, Seitz S, Streichert T, et al. Immediate effects of retinoic acid on gene expression in primary murine osteoblasts. J Bone Miner Metab. (2016) 34:161–70. doi: 10.1007/s00774-015-0666-2
107. Lionikaite V, Henning P, Drevinge C, Shah FA, Palmquist A, Wikstrom P, et al. Vitamin A decreases the anabolic bone response to mechanical loading by suppressing bone formation. FASEB J. (2019) 33:5237–47. doi: 10.1096/fj.201802040R
108. Lind T, Ohman C, Calounova G, Rasmusson A, Andersson G, Pejler G, et al. Excessive dietary intake of vitamin A reduces skull bone thickness in mice. PloS One. (2017) 12:e0176217. doi: 10.1371/journal.pone.0176217
109. Persson B, Tunell R, Ekengren K. Chronic vitamin a intoxication during the first half year of life; description of 5 cases. Acta Paediatr Scand. (1965) 54:49–60. doi: 10.1111/j.1651-2227.1965.tb06345.x
110. Lind T, Sundqvist A, Hu L, Pejler G, Andersson G, Jacobson A, et al. Vitamin a is a negative regulator of osteoblast mineralization. PloS One. (2013) 8:e82388. doi: 10.1371/journal.pone.0082388
111. Morony S, Capparelli C, Lee R, Shimamoto G, Boone T, Lacey DL, et al. A chimeric form of osteoprotegerin inhibits hypercalcemia and bone resorption induced by IL-1beta, TNF-alpha, PTH, PTHrP, and 1, 25(OH)2D3. J Bone Miner Res. (1999) 14:1478–85. doi: 10.1359/jbmr.1999.14.9.1478
112. Michaelsson K, Lithell H, Vessby B, Melhus H. Serum retinol levels and the risk of fracture. N Engl J Med. (2003) 348:287–94. doi: 10.1056/NEJMoa021171
113. Henning P, Conaway HH, Lerner UH. Retinoid receptors in bone and their role in bone remodeling. Front Endocrinol (Lausanne). (2015) 6:31. doi: 10.3389/fendo.2015.00031
114. Yee MMF, Chin KY, Ima-Nirwana S, Wong SK. Vitamin A and bone health: A review on current evidence. Molecules. (2021) 26:1–19. doi: 10.3390/molecules26061757
115. Jackson HA, Sheehan AH. Effect of vitamin A on fracture risk. Ann Pharmacother. (2005) 39:2086–90. doi: 10.1345/aph.1G028
116. Furr HC, Amedee-Manesme O, Clifford AJ, Bergen HR, Jones AD 3rd, Anderson DP, et al. Vitamin A concentrations in liver determined by isotope dilution assay with tetradeuterated vitamin A and by biopsy in generally healthy adult humans. Am J Clin Nutr. (1989) 49:713–6. doi: 10.1093/ajcn/49.4.713
117. Penniston KL, Tanumihardjo SA. The acute and chronic toxic effects of vitamin A. Am J Clin Nutr. (2006) 83:191–201. doi: 10.1093/ajcn/83.2.191
118. Croquet V, Pilette C, Lespine A, Vuillemin E, Rousselet MC, Oberti F, et al. Hepatic hyper-vitaminosis A: importance of retinyl ester level determination. Eur J Gastroenterol Hepatol. (2000) 12:361–4. doi: 10.1097/00042737-200012030-00016
119. Macdonald HM, New SA, Golden MH, Campbell MK, Reid DM. Nutritional associations with bone loss during the menopausal transition: evidence of a beneficial effect of calcium, alcohol, and fruit and vegetable nutrients and of a detrimental effect of fatty acids. Am J Clin Nutr. (2004) 79:155–65. doi: 10.1093/ajcn/79.1.155
120. Promislow JH, Goodman-Gruen D, Slymen DJ, Barrett-Connor E. Retinol intake and bone mineral density in the elderly: the Rancho Bernardo Study. J Bone Miner Res. (2002) 17:1349–58. doi: 10.1359/jbmr.2002.17.8.1349
121. Forsmo S, Fjeldbo SK, Langhammer A. Childhood cod liver oil consumption and bone mineral density in a population-based cohort of peri- and postmenopausal women: the Nord-Trondelag Health Study. Am J Epidemiol. (2008) 167:406–11. doi: 10.1093/aje/kwm320
122. De Franca NA, Camargo MB, Lazaretti-Castro M, Martini LA. Antioxidant intake and bone status in a cross-sectional study of Brazilian women with osteoporosis. Nutr Health. (2013) 22:133–42. doi: 10.1177/0260106014563445
123. Rejnmark L, Vestergaard P, Charles P, Hermann AP, Brot C, Eiken P, et al. No effect of vitamin A intake on bone mineral density and fracture risk in perimenopausal women. Osteoporos Int. (2004) 15:872–80. doi: 10.1007/s00198-004-1618-1
124. Wolf RL, Cauley JA, Pettinger M, Jackson R, Lacroix A, Leboff MS, et al. Lack of a relation between vitamin and mineral antioxidants and bone mineral density: results from the Women's Health Initiative. Am J Clin Nutr. (2005) 82:581–8. doi: 10.1093/ajcn/82.3.581
125. Kaptoge S, Welch A, McTaggart A, Mulligan A, Dalzell N, Day NE, et al. Effects of dietary nutrients and food groups on bone loss from the proximal femur in men and women in the 7th and 8th decades of age. Osteoporos Int. (2003) 14:418–28. doi: 10.1007/s00198-003-1391-6
126. Kim DE, Cho SH, Park HM, Chang YK. Relationship between bone mineral density and dietary intake of beta-carotene, vitamin C, zinc and vegetables in postmenopausal Korean women: a cross-sectional study. J Int Med Res. (2016) 44:1103–14. doi: 10.1177/0300060516662402
127. Suzuki Y, Whiting SJ, Davison KS, Chilibeck PD. Total calcium intake is associated with cortical bone mineral density in a cohort of postmenopausal women not taking estrogen. J Nutr Health Aging. (2003) 7:296–9.
128. Penniston KL, Weng N, Binkley N, Tanumihardjo SA. Serum retinyl esters are not elevated in postmenopausal women with and without osteoporosis whose preformed vitamin A intakes are high. Am J Clin Nutr. (2006) 84:1350–6. doi: 10.1093/ajcn/84.6.1350
129. de Jonge EA, Kiefte-de Jong JC, Campos-Obando N, Booij L, Franco OH, Hofman A, et al. Dietary vitamin A intake and bone health in the elderly: the Rotterdam Study. Eur J Clin Nutr. (2015) 69:1360–8. doi: 10.1038/ejcn.2015.154
130. Joo NS, Yang SW, Song BC, Yeum KJ. Vitamin A intake, serum vitamin D and bone mineral density: analysis of the Korea National Health and Nutrition Examination Survey (KNHANES, 2008-2011). Nutrients. (2015) 7:1716–27. doi: 10.3390/nu7031716
131. Chen GD, Zhu YY, Cao Y, Liu J, Shi WQ, Liu ZM, et al. Association of dietary consumption and serum levels of vitamin A and beta-carotene with bone mineral density in Chinese adults. Bone. (2015) 79:110–5. doi: 10.1016/j.bone.2015.05.028
132. Choi MJ, Park EJ, Jo HJ. Relationship of nutrient intakes and bone mineral density of elderly women in Daegu, Korea. Nutr Res Pract. (2007) 1:328–34. doi: 10.4162/nrp.2007.1.4.328
133. Karamati M, Yousefian-Sanni M, Shariati-Bafghi SE, Rashidkhani B. Major nutrient patterns and bone mineral density among postmenopausal Iranian women. Calcif Tissue Int. (2014) 94:648–58. doi: 10.1007/s00223-014-9848-5
134. Houtkooper LB, Ritenbaugh C, Aickin M, Lohman TG, Going SB, Weber JL, et al. Nutrients, body composition and exercise are related to change in bone mineral density in premenopausal women. J Nutr. (1995) 125:1229–37. doi: 10/1093/jn/125.5.1229
135. Feskanich D, Singh V, Willett WC, Colditz GA. Vitamin A intake and hip fractures among postmenopausal women. JAMA. (2002) 287:47–54. doi: 10.1001/jama.287.1.47
136. Mata-Granados JM, Cuenca-Acevedo JR, Luque de Castro MD, Holick MF, Quesada-Gomez JM. Vitamin D insufficiency together with high serum levels of vitamin A increases the risk for osteoporosis in postmenopausal women. Arch Osteoporos. (2013) 8:124. doi: 10.1007/s11657-013-0124-5
137. Caire-Juvera G, Ritenbaugh C, Wactawski-Wende J, Snetselaar LG, Chen Z. Vitamin A and retinol intakes and the risk of fractures among participants of the Women's Health Initiative Observational Study. Am J Clin Nutr. (2009) 89:323–30. doi: 10.3945/ajcn.2008.26451
138. Key TJ, Appleby PN, Spencer EA, Roddam AW, Neale RE, Allen NE. Calcium, diet and fracture risk: a prospective study of 1898 incident fractures among 34 696 British women and men. Public Health Nutr. (2007) 10:1314–20. doi: 10.1017/S1368980007696402
139. Sun LL, Li BL, Xie HL, Fan F, Yu WZ, Wu BH, et al. Associations between the dietary intake of antioxidant nutrients and the risk of hip fracture in elderly Chinese: a case-control study. Br J Nutr. (2014) 112:1706–14. doi: 10.1017/S0007114514002773
140. Lim LS, Harnack LJ, Lazovich D, Folsom AR. Vitamin A intake and the risk of hip fracture in postmenopausal women: the Iowa Women's Health Study. Osteoporos Int. (2004) 15:552–9. doi: 10.1007/s00198-003-1577-y
141. Mata-Granados JM, Cuenca-Acevedo R, Luque de Castro MD, Sosa M, Quesada-Gomez JM. Vitamin D deficiency and high serum levels of vitamin A increase the risk of osteoporosis evaluated by Quantitative Ultrasound Measurements (QUS) in postmenopausal Spanish women. Clin Biochem. (2010) 43:1064–8. doi: 10.1016/j.clinbiochem.2010.06.001
142. Navarro-Valverde C, Caballero-Villarraso J, Mata-Granados JM, Casado-Diaz A, Sosa-Henriquez M, Malouf-Sierra J, et al. High serum retinol as a relevant contributor to low bone mineral density in postmenopausal osteoporotic women. Calcif Tissue Int. (2018) 102:651–6. doi: 10.1007/s00223-017-0379-8
143. Handel MN, Moon RJ, Titcombe P, Abrahamsen B, Heitmann BL, Calder PC, et al. Maternal serum retinol and beta-carotene concentrations and neonatal bone mineralization: results from the Southampton Women's Survey cohort. Am J Clin Nutr. (2016) 104:1183–8. doi: 10.3945/ajcn.116.130146
144. Ballew C, Galuska D, Gillespie C. High serum retinyl esters are not associated with reduced bone mineral density in the Third National Health And Nutrition Examination Survey, 1988-1994. J Bone Miner Res. (2001) 16:2306–12. doi: 10.1359/jbmr.2001.16.12.2306
145. Hogstrom M, Nordstrom A, Nordstrom P. Retinol, retinol-binding protein 4, abdominal fat mass, peak bone mineral density, and markers of bone metabolism in men: the Northern Osteoporosis and Obesity (NO2) Study. Eur J Endocrinol. (2008) 158:765–70. doi: 10.1530/EJE-07-0796
146. Maggio D, Barabani M, Pierandrei M, Polidori MC, Catani M, Mecocci P, et al. Marked decrease in plasma antioxidants in aged osteoporotic women: results of a cross-sectional study. J Clin Endocrinol Metab. (2003) 88:1523–7. doi: 10.1210/jc.2002-021496
147. Maggio D, Polidori MC, Barabani M, Tufi A, Ruggiero C, Cecchetti R, et al. Low levels of carotenoids and retinol in involutional osteoporosis. Bone. (2006) 38:244–8. doi: 10.1016/j.bone.2005.08.003
148. Barker ME, McCloskey E, Saha S, Gossiel F, Charlesworth D, Powers HJ, et al. Serum retinoids and beta-carotene as predictors of hip and other fractures in elderly women. J Bone Miner Res. (2005) 20:913–20. doi: 10.1359/JBMR.050112
149. Opotowsky AR, Bilezikian JP, N.I.f.-u. study. Serum vitamin A concentration and the risk of hip fracture among women 50 to 74 years old in the United States: a prospective analysis of the NHANES I follow-up study. Am J Med. (2004) 117:169–74. doi: 10.1016/j.amjmed.2004.02.045
150. Ambrosini GL, Alfonso H, Reid A, Mackerras D, Bremner AP, Beilby J, et al. Plasma retinol and total carotenes and fracture risk after long-term supplementation with high doses of retinol. Nutrition. (2014) 30:551–6. doi: 10.1016/j.nut.2013.10.007
151. Holvik K, Ahmed LA, Forsmo S, Gjesdal CG, Grimnes G, Samuelsen SO, et al. No increase in risk of hip fracture at high serum retinol concentrations in community-dwelling older Norwegians: the Norwegian Epidemiologic Osteoporosis Studies. Am J Clin Nutr. (2015) 102:1289–96. doi: 10.3945/ajcn.115.110528
152. Sowers MF, Wallace RB. Retinol, supplemental vitamin A and bone status. J Clin Epidemiol. (1990) 43:693–9. doi: 10.1016/0895-4356(90)90040-V
153. Gao SS, Zhao Y. The effects of beta-carotene on osteoporosis: a systematic review and meta-analysis of observational studies. Osteoporos Int. (2023) 34:627–39. doi: 10.1007/s00198-022-06593-7
154. Vestergaard P, Rejnmark L, Mosekilde L. High-dose treatment with vitamin A analogues and risk of fractures. Arch Dermatol. (2010) 146:478–82. doi: 10.1001/archdermatol.2010.59
155. Johansson S, Melhus H. Vitamin A antagonizes calcium response to vitamin D in man. J Bone Miner Res. (2001) 16:1899–905. doi: 10.1359/jbmr.2001.16.10.1899
156. Wu AM, Huang CQ, Lin ZK, Tian NF, Ni WF, Wang XY, et al. The relationship between vitamin A and risk of fracture: meta-analysis of prospective studies. J Bone Miner Res. (2014) 29:2032–9. doi: 10.1002/jbmr.2237
157. Sahni S, Hannan MT, Blumberg J, Cupples LA, Kiel DP, Tucker KL. Protective effect of total carotenoid and lycopene intake on the risk of hip fracture: a 17-year follow-up from the Framingham Osteoporosis Study. J Bone Miner Res. (2009) 24:1086–94. doi: 10.1359/jbmr.090102
158. Dai Z, Wang R, Ang LW, Low YL, Yuan JM, Koh WP. Protective effects of dietary carotenoids on risk of hip fracture in men: the Singapore Chinese Health Study. J Bone Miner Res. (2014) 29:408–17. doi: 10.1002/jbmr.2041
159. Zhang X, Zhang R, Moore JB, Wang Y, Yan H, Wu Y, et al. The effect of vitamin A on fracture risk: A meta-analysis of cohort studies. Int J Environ Res Public Health. (2017) 14:1–15. doi: 10.3390/ijerph14091043
Keywords: vitamin A, bone, osteoporosis, osteoclast, osteoblast
Citation: Lerner UH (2024) Vitamin A – discovery, metabolism, receptor signaling and effects on bone mass and fracture susceptibility. Front. Endocrinol. 15:1298851. doi: 10.3389/fendo.2024.1298851
Received: 22 September 2023; Accepted: 02 April 2024;
Published: 22 April 2024.
Edited by:
Giacomina Brunetti, University of Bari Aldo Moro, ItalyReviewed by:
Vishwa Deepak, Kean University-Wenzhou, ChinaJonathan H. Tobias, University of Bristol, United Kingdom
Copyright © 2024 Lerner. This is an open-access article distributed under the terms of the Creative Commons Attribution License (CC BY). The use, distribution or reproduction in other forums is permitted, provided the original author(s) and the copyright owner(s) are credited and that the original publication in this journal is cited, in accordance with accepted academic practice. No use, distribution or reproduction is permitted which does not comply with these terms.
*Correspondence: Ulf H. Lerner, dWxmLmxlcm5lckBndS5zZQ==