- 1Department of Neonatal and Pediatric Intensive Care, John Paul II Center for Child and Family Health, Sosnowiec, Poland
- 2Department of Pediatrics and Pediatric Endocrinology, Medical University of Silesia, Katowice, Poland
- 3Laboratory for the Diagnosis of Metabolic Disorders and Steroidogenesis, The Children's Memorial Health Institute, Warsaw, Poland
- 4Department of Paediatric and Adolescent Endocrinology, Jagiellonian University Medical College, University Children's Hospital, Krakow, Poland
Introduction: Adrenal steroidogenesis plays a pivotal role in neonatal adaptation, and advanced steroid profiling offers novel insights into disease risks and personalized management strategies. This study aimed to identify adrenal steroid metabolomic clusters in neonates and to correlate them with clinical outcomes.
Methods: In a prospective observational design (June 2021–July 2022), 50 neonates (12 early preterm, 18 late preterm, and 20 full-term) admitted with respiratory distress underwent continuous 24-hour urine collection via an urinary catheter. Steroid profiles were analyzed by gas chromatography–mass spectrometry. K-means clustering was employed to classify the metabolomic data, which were subsequently correlated with mortality, bronchopulmonary dysplasia (BPD), small for gestational age (SGA), and intraventricular hemorrhage (IVH).
Results: K-means analysis delineated three distinct metabolic clusters. Cluster 1 displayed a profoundly suppressed steroidogenesis (low C19 and C21 excretion, diminished 3β-hydroxysteroid dehydrogenase and 5α-reductase activities), correlating with an increased incidence of BPD, high mortality risk scores, and significant rates of SGA/intrauterine growth restriction. Cluster 2 exhibited adrenal hyperactivation with elevated cortisol/cortisone derivatives, moderately increased C19/C21 metabolites, and partial 3β-HSD deficits, associated with a heightened risk of IVH and mortality. Cluster 3 showed robust steroidogenesis (high C19/C21 excretion and high 3β-HSD/5α-reductase activities), accompanied by the lowest mortality rates and absence of BPD or SGA/IUGR.
Conclusions: Suppressed steroidogenesis increased BPD, SGA, and mortality, while excessive cortisol output in Cluster 2 was associated with a higher risk of IVH. Robust steroidogenesis supported favorable outcomes, highlighting the potential for metabolome-guided interventions.
Introduction
Steroids play a pivotal role in the regulation of a multitude of physiological processes during fetal development. The synthesis of these steroids is predominantly undertaken by the fetal zone (FZ) and the definitive zone (DZ), which are crucial regions within the developing fetal adrenal cortex. Dehydroepiandrosterone (DHEA), synthesized in the FZ, undergoes 16α-hydroxylation in the fetal liver and is subsequently converted stepwise to estriol within the feto-placental unit. The synthesis of glucocorticoids and mineralocorticoids occurs in the definitive zone and is determined by the activity of 3β-hydroxysteroid dehydrogenase type 2 (HSD3B2), which facilitates the conversion of pregnenolone and 17-hydroxypregnenolone into their active forms (1–3). Furthermore, recent research has identified the involvement of alternative androgen pathways, such as the 11-oxy pathways, in the production of bioactive steroids, thereby contributing to a more complex understanding of adrenal steroidogenesis (4, 5).
The exact physiological and teleological roles of both estrogens and fetal zone steroids (FZS) in fetal development remain unclear. Nonetheless, recent studies indicate that estradiol, along with FZS such as DHEA, may exert neuroprotective effects. Indeed, the fetal brain expresses estrogen receptors and contains aromatase, an enzyme responsible for local estrogen production (6). Meanwhile, glucocorticoids play an essential role in organ maturation during fetal development, as well as in regulating metabolism and the stress response postnatally. In contrast, mineralocorticoids are crucial for maintaining fluid and electrolyte homeostasis (1–3).
The expansion of the fetal zone commences at approximately 8 to 9 weeks post-fertilization, with steroidogenesis in the DZ developing gradually throughout gestation (7). In the initial stages, the fetal adrenal glands predominantly produce androgens, particularly DHEA, due to the limited expression of the enzyme 3β-hydroxysteroid dehydrogenase type 2 (3β-HSD2) (8). The full capacity for glucocorticoid synthesis is reached during the latter stages of the second trimester, while the significance of mineralocorticoids, such as aldosterone, becomes apparent predominantly in the third trimester (1). As birth approaches, the fetal-placental unit is disrupted and adrenal cortical activity is expected to reach a state of functional maturity. In preterm infants ongoing estriol production from fetal zone DHEA would necessitate the involvement of other peripheral tissues beyond the liver, though the specifics of this process remain unknown (6). Following the expected birth date, the fetal zone of the adrenal cortex gradually undergoes involution (9).
Given the aforementioned characteristics, premature neonates are defined by the immaturity of the adrenal glands, which limits their ability to produce steroids at required levels. Concurrently, there is a high demand for steroids during this intensive perinatal development phase, further complicated by critical conditions associated with premature birth (10). This imbalance in steroid production, particularly in cortisol synthesis, is partially managed by mechanisms such as the balance between 11β-HSD1 and 11β-HSD2, which regulate local cortisol availability in tissues (11).
In practice, the neonatal assessment of the adrenal glands is primarily limited to the exclusion of congenital adrenal hyperplasia (CAH) during screening tests. This involves the assessment of 17 OHP in dried blood spots on filter paper, with further confirmation in a short steroid profile in the same blood sample. This newborn screening enables the exclusion of the most common forms of CAH, including 21-hydroxylase deficiency and 11β-hydroxylase deficiency. In some countries, where screening has not been implemented, exclusion is based solely on clinical suspicion (12). In many cases, genetic analysis is essential to determine the precise cause of primary adrenal insufficiency (PAI), which is of significant importance for the long-term management of the condition, including the personalization of therapy (13). It is also important to note that initial screening diagnostics, involving the measurement of 17-hydroxyprogesterone (17-OHP) levels from blood collected on filter paper from the second day of life, is limited by high false positive rates, and low positive predictive values (14). Proper steroid activity is crucial from birth and disturbances in steroidogenesis have been identified in neonatal disorders such as bronchopulmonary dysplasia (BPD), intraventricular hemorrhage (IVH), and small for gestational age (SGA) (15–20).
The recent literature has focused on the assessment of the steroid metabolome in newborns using gas chromatography-mass spectrometry (GC-MS), given the lack of a commercially available reliable immunoassay for measuring typical neonatal steroids (6, 19, 21). The application of steroid metabolomics allows for the phenotyping of individuals at a chemical level and facilitates the development of cluster-specific therapeutic strategies, which may be particularly beneficial for addressing neonatal disorders. This unique steroid metabolome, often referred to as a metabolic fingerprint, provides insight into specific steroid patterns among metabolites involved in steroidogenesis. By linking these patterns to gestational age or clinical conditions, it enables personalized diagnostics and the tailoring of treatments (19, 22).
Identification of various metabolic patterns is possible through data clustering methods in analysis. One of the most common approaches is the k-means algorithm, which involves dividing a dataset into k clusters, where k is a number predetermined by the user. The earliest mentions of this method date back to the 1960s. The algorithm first assigns all data and then points to randomly selected k cluster centroids and repeats this process until the centroids no longer change, indicating convergence (23). K-means clustering has already been used in steroid analysis for classifying subunits of certain conditions, which aims to personalize the therapeutic approach (22, 24, 25).
By using a k-means clustering algorithm we aimed to compare prospectively the identified steroid metabolomes and the clinical phenotypes of newborns hospitalized in the Neonatal Intensive Care Unit (NICU). Furthermore, the research aimed to elucidate the specific conditions that are characteristic of the steroid patterns identified in preterm and term infants.
Patients and methods
Patients
The study population comprised a consecutive series of 50 newborns, including 12 early preterm infants (24%; gestational age (GA): 31 - 31.9), 18 late preterm infants (36%; GA: 34 - 36.7) and 20 full-term infants (40%; GA: 38 - 41.6). The infants were admitted to the NICU within the first 48 hours of life between June 2021 and July 2022. On the third day of life, all patients underwent a 24-hour urine collection to analyze individual steroid metabolites. All patients were routinely catheterized with a Foley catheter due to the need to monitor daily diuresis, making the study non-invasive as the urine collected in this way was used for analysis. Comprehensive data on prenatal growth, labor, and medical histories were gathered from the NICU files to correlate these with the steroid profile outcomes.
The study was conducted in accordance with the Helsinki declaration and the ethical standards set forth by the Ethics Committee of Silesian Medical University in Katowice (PCN/CBN/0052/KB1/99/I/21/22/23) and informed written parental consent was obtained. Given the non-invasive nature of the study, no parental objections to participation were encountered. Previous exclusions included antenatal steroid therapy (n=2) and chromosomal aberration (trisomy 21, n=1). The exclusion criteria encompassed congenital adrenal hyperplasia (CAH), which was not observed. Gestational age was verified using the expanded Ballard score and/or obstetrical dating.
In line with the guidelines of the Pradhan Mantri Surakshit Matritva Abhiyan (PMSMA) for classification of high-risk pregnancy (26), a total of 25 pregnancies in our cohort were classified as high-risk. These included two twin gestations, five cases of urinary tract infections, two cases of preeclampsia, two maternal infections (COVID-19 and pyelonephritis), four cases of placental abruption, three unmonitored pregnancies, three cases of uterine rupture or threat thereof, one case of Hashimoto's thyroiditis, one case of hypothyroidism, as well as additional instances of asthma and polycystic ovary syndrome (PCOS). Cesarean deliveries were categorized based on urgency using a three-tier classification system as described by Torloni et al. (27). Among the 50 neonates included in this study, 35 were delivered via cesarean section, of which 16 were elective, 9 were urgent, and 10 were classified as emergency procedures.
Children were admitted to the NICU due to various conditions, including Infantile Respiratory Distress Syndrome (n = 28), congenital pneumonia (n = 4), respiratory failure (n = 8), low birth weight (n = 7), epilepsy (n = 2), and apnea (n = 1). Broncho-Pulmonary Dysplasia (BPD) was diagnosed according to the 2006 National Institute of Health (US) criteria for BPD (28). Intraventricular hemorrhage (IVH) was evaluated using cranial ultrasound and only grades 1 and 2 were observed (29). SGA was defined as a birth weight below 10th percentile for gestational age (30). Intrauterine Growth Restriction (IUGR) was defined based on prenatal ultrasound criteria as estimated fetal weight (EFW) or abdominal circumference (AC) below the 3rd percentile, or meeting at least two out of three conditions: EFW or AC below the 10th percentile, AC or EFW crossing more than two quartiles, and cerebroplacental ratio (CPR) below the 5th percentile or umbilical artery pulsatility index (UA-PI) above the 95th percentile (31). Both patent foramen ovale (PFO) and pulmonary hypertension (PH) were assessed using transthoracic echocardiography (TNE), following the recommendations of the American Society of Echocardiography (32). TNE was performed within 72 hours after birth in extremely preterm infants and within the first week of life in late preterm and full-term neonates, depending on clinical indications. Sepsis was defined as one positive blood culture with non–coagulase negative staphylococci or one positive blood culture with coagulase-negative staphylococci in combination with a C-reactive protein level greater than 10 mg/L within 2 days of blood culture or two positive blood cultures with coagulase-negative staphylococci drawn within 2 days (33). The severity of illness was assessed within the first 12 hours after admission to the NICU using the Score for Neonatal Acute Physiology Extension-II (SNAPPE-II) (34). All of the patients received both enteral and parenteral feeding.
Urine collection procedure
Urine samples were collected continuously for a 24-hour period, commencing at 7 a.m. on the third day of life and concluding at 7 a.m. the following day. At six-hour intervals, the urine samples were stored at a temperature of 6 degrees Celsius until the conclusion of the collection period. Upon completion, the total volume of urine collected was recorded, and the samples were then preserved at a temperature of -24 degrees Celsius in a freezer until they were transported in a refrigerated container to the laboratory for analysis.
Laboratory analysis
The steroid profiles in urine were determined using gas chromatography-mass spectrometry (GC-MS) at the Laboratory for the Diagnosis of Metabolism and Steroidogenesis Disorders with several decades of experience in the analysis of steroid metabolome. The analysis encompassed the monitoring of 30 metabolites in both term and preterm infants (Table 1). Steroid analysis was conducted using a Hewlett-Packard HP 6890 Series GC System, which was equipped with a Hewlett-Packard 5973 Mass Selective Detector. The separation was conducted on a 12-meter quartz capillary column, namely the HP Ultra 1. The operational parameters were optimized with the objective of achieving the highest possible resolution and peak sharpness for the steroid compounds. The identification of steroid peaks was achieved by comparing the retention times of the analytes with those of standard steroid compounds under identical chromatographic conditions. This comparison ensured accurate identification based on retention time congruence. Quantitative determinations were conducted by comparing the area of each steroid peak to that of an internal standard, stigmasterol. In cases where compound separation was challenging due to matrix complexity, the Selected Ion Monitoring (SIM) method was employed.
Statistical analysis and metabolomic data
The study applied the concept of a "metabolome fingerprint", which was based on the quantitative data obtained from the analysis of urinary steroids by GC-MS over a 24-hour period (35). Peer Group Normalization (PGN) was employed to standardize metabolite values against appropriate reference groups. The selected demographic features included sex, age, body length and body weight, as each significantly influences metabolic profiles. Initially, correlations between these features and metabolites were calculated using Pearson's correlation coefficient to determine their impact. Statistically significant correlations (p < 0.05) formed the basis for assigning weights to each feature. Age was assigned a weight proportional to its number of significant correlations (7), body length with 12 significant correlations, while body weight, with 17 significant correlations, was given the highest weight. Peer groups were constructed considering sex to account for biological differences. For each participant, distances in the feature space (age, body length and body weight) were computed with the inclusion of the assigned weights. Within each peer group, the 10 closest neighbors of the same sex were selected to form the reference cohort, ensuring biological consistency. Normalization was performed by calculating Z-scores for each metabolite based on the mean and standard deviation within the peer group.
The patient clustering was conducted using MetaboAnalyst, an online platform for metabolomic data analysis (https://metaboanalyst.ca) developed by the Xia Laboratory at McGill University in Montreal, Canada. Three clusters were determined by visual inspection of the heatmap. The k-means clustering algorithm was employed to create three distinct clusters among neonates (Figure 1A). The PERMANOVA (Permutational Multivariate Analysis of Variance) and a principal component analysis (PCA) were conducted (Figure 1B). Obtained metabolomic fingerprints and clinical data were subjected to statistical analysis using Statistica version 13.0 (StataCorp. LLC, USA), followed by ANOVA and post-hoc analysis or Mann Whitney's U Test. The chi-square test or Fisher's exact test was employed to assess the existence of statistically significant clinical differences between the clusters, with a p-value of less than 0.05 considered to indicate such a difference. In order to compare the daily excretion rates of single steroid metabolites between clusters, the Bonferroni adjustment was employed to correct for multiple testing. The metabolites were grouped according to their carbon structure. Table 1 provides the detailed classification and chemical structures of these metabolites. To calculate the excretion of steroid subgroups, the raw results were aggregated and subsequently, the Z-score was calculated.
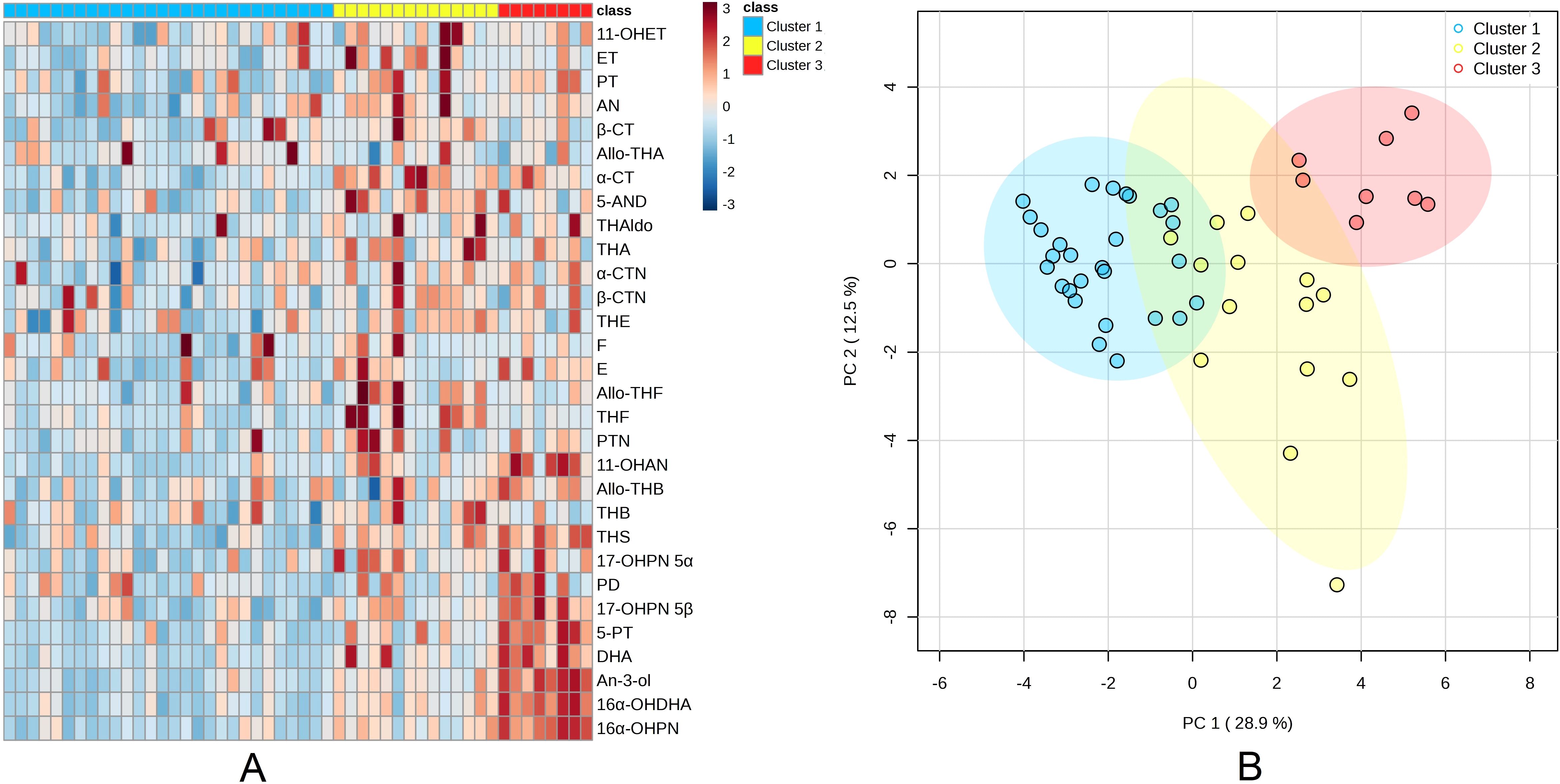
Figure 1. Analysis of steroid metabolite excretion in neonates (A) Heatmap and metabolite clusters: The columns correspond to individual patients, while the rows represent the z-transformed 24-hour excretion rates of 30 steroid metabolites. The colour scale employed for the heatmap ranges from blue (indicative of low excretion rates) to red (indicative of high excretion rates). The top marginal row indicates the assigned clusters, (B) Principal component (PC) analysis: The dots represent samples from 50 neonates, projected onto the plane defined by the first and second principal components. The colours of the dots correspond to the classification groups of the subjects.
Enzyme activity calculation methods
In the present study, the enzymatic activities involved in steroidogenesis—specifically 11β-HSD-1, 11β-HSD-2, 5α-reductase, and 3β-HSD—were calculated using established ratios between selected precursor and product metabolites, derived from the quantitative GC-MS data. These ratios function as surrogate markers of enzymatic function, thereby enabling inference of pathway activity without the necessity of data normalization. (8, 36).
Results
The application of the k-means clustering method resulted in the categorization of all neonates into three distinct clusters: Cluster 1 (n = 28), Cluster 2 (n = 14) and Cluster 3 (n = 8). A subsequent investigation revealed that no statistically significant differences were observed between the neonatal clusters with regard to gestational age (GA), sex or body weight (p > 0.05; Table 2). Notably, emergency cesarean sections were significantly more frequent in Cluster 1 compared to Cluster 3 (7 vs. 0 cases, respectively; p = 0.015). Figure 2 depicts the 'steroid metabolomic signatures' of the three identified clusters. The PERMANOVA analysis demonstrated significant differences between each of the groups in their PCA scores (p = 0.001). The greatest variability among the clusters was related to DHEA and 17-hydroxyprogesterone metabolites. The concentrations of steroid metabolites (raw and normalized data) are presented in Supplementary Tables 1, 2.
The post-hoc Bonferroni tests demonstrated statistically significant differences between each cluster for C19 and C21 (p < 0.05). A decreased excretion for both C19 and C21 was observed in Cluster 1, an increased excretion in Cluster 3, and moderately elevated excretion in Cluster 2. With regard to cortisol and cortisone derivatives, cluster 2 exhibited high levels of excretion, while cluster 3 demonstrated an intermediate level of excretion; however, cluster 1 presented a significantly decreased result, with a statistical disparity when compared with cluster 2 (p = 0.026; Table 3).
The prevalence of SGA was documented in 32.1% of infants in Cluster 1, while IUGR was reported in 25% of pregnancies within this cluster. Notably, no cases of IUGR were observed in Cluster 3 (p=0.021 and p=0.046, respectively). Furthermore, the prevalence of bronchopulmonary dysplasia (BPD) was significantly higher in Cluster 1 (46.4%) compared to Cluster 3 (p = 0.024). Conversely, IVH was observed with the lowest frequency in Cluster 1 (17.8%), exhibiting a substantial difference compared to Cluster 2 (50%; p = 0.033) but not to Cluster 3 (37.5%; p > 0.05). Two early preterm girls from Cluster 3 (25%) exhibited features of androgen excess, evidenced by clitoral enlargement. Cluster 3 was distinguished by the lowest mortality rate, with a median SNAPPE-II Score of 5 (range 5 - 24), exhibiting a significant difference compared to Cluster 1 (23; 5 - 72; p = 0.036) and a trend towards Cluster 2 (23.5; 5 - 67; p = 0.08). The duration of hospitalization was comparable across all groups (p > 0.05).
As demonstrated in Table 4, a number of statistically significant differences in steroid metabolite ratios were identified among the neonatal clusters. The highest activity of 5α-reductase and 3β-HSD was observed in cluster 3 (p < 0.002), and increased levels of androgen precursors were also identified (p < 0.002) when compared to clusters 1 and 2. Conversely, the activity of 11β-HSD-1 was higher in cluster 2 than in clusters 1 and 3 (p = 0.021 and p = 0.038, respectively). The activity of 11β-HSD-2 was greater in cluster 1 compared to cluster 2 (p = 0.022).
Discussion
This study utilized a steroid metabolomic signature approach in neonates admitted to the NICU, implementing a rigorous 24-hour urine collection via catheterization in newborns and subsequent analysis by GC-MS (21). Although similar analyses have been conducted using adhesive urine bags or diaper extraction methods (19, 37), these less invasive approaches often face difficulties in ensuring a complete 24-hour sample collection. This highlights the necessity for a standardized sampling technique to accurately capture daily fluctuations in steroid excretion, thereby ensuring the reliability and validity of the metabolomic data.
K-means clustering successfully distinguished three clusters among all neonates that differed in all adrenal steroid synthesis pathways. During gestation, the fetal zone of the adrenal glands predominantly secretes C19 steroid precursors, notably DHEA and its hydroxylated derivatives (9, 38). Consequently, in our dataset, the greatest variation among clusters centered on these C19 metabolites, such as 16α-hydroxy-DHEA and An-3-ol, likely mirroring differences in the pace of fetal zone involution immediately after birth (6). The observed variability in C19 intermediates may reflect distinct adaptive trajectories among neonates, given the established role of the fetal zone in shaping the early postnatal endocrine milieu (9, 38).
In the context of the present study, it was observed that neonates in Cluster 1 exhibited a marked reduction across all three steroid groups, indicating a profoundly diminished adrenal output. In particular, they showed low 3β-HSD activity, as evidenced by minimal downstream products from DHEA (C19) and pregnenolone (C21). Concurrently, diminished 5α-reductase activity curtailed the conversion of androgenic precursors (e.g., testosterone) and the 5α-reduction of cortisol to tetrahydrocortisol. Furthermore, elevated 11β-HSD2 activity indicated heightened placental inactivation of cortisol (11, 39, 40). Collectively, these enzymatic patterns suggest a global suppression of neonatal steroidogenesis in Cluster 1.
Clinically, neonates in Cluster 1 also exhibited elevated SNAPPE-II scores, indicative of an elevated mortality risk relative to Cluster 3. This finding is consistent with previous studies demonstrating that insufficient glucocorticoid availability impairs the stress response in critically ill neonates (41, 42). Given the low excretion of both cortisol and cortisone derivatives in this group, an inadequate adrenal reserve may underlie their higher vulnerability to severe neonatal complications. Such deficiencies in adrenal steroid output appear to compound the risk associated with other perinatal stressors frequently encountered in the NICU. Furthermore, the clinical profile of Cluster 1 was marked by a significantly higher number of emergency cesarean deliveries compared to Cluster 3, suggesting that neonates in this group may have been exposed to more acute perinatal stress. This difference aligns with the overall impression of a less favorable intrauterine and peripartum environment in Cluster 1.
In addition, a significant incidence of bronchopulmonary dysplasia (BPD) was observed in Cluster 1 neonates, with no cases detected in Cluster 3, suggesting a substantially elevated risk in the former group. This observation is consistent with the notion that endogenous glucocorticoids play a pivotal role in lung maturation and the regulation of pulmonary inflammation (6, 16) (28). Consequently, a suboptimal adrenal reserve in these neonates may be a contributing factor to their predisposition for BPD. This finding is consistent with the hypothesis that low cortisol production in preterm infants is associated with poorer respiratory outcomes, and that adequate postnatal steroid levels may be necessary to mitigate the multifactorial pathogenesis of BPD (6, 16).
The findings highlight the potential benefits of targeted steroid therapy as part of a precision medicine approach. Antenatal corticosteroids (ACS) remain the gold standard for pregnancies at risk of preterm delivery, improving neonatal respiratory function and shaping metabolic profiles (43–45). Nevertheless, an effective and safe postnatal regimen to prevent bronchopulmonary dysplasia (BPD) remains elusive. The use of hydrocortisone therapy has yielded equivocal outcomes, with the timing and dosing of treatment proving to be of critical importance (46–49). Initiating treatment beyond 50 days of life is less effective in reducing BPD incidence (50), whereas high-dose glucocorticoids given too early may raise concerns about neurodevelopment (51).
In this context, we propose that individualized postnatal steroid replacement regimens could be optimized using each neonate's steroid metabolomic fingerprint, moving beyond uniform protocols based solely on gestational age. For neonates in Cluster 1, characterized by globally suppressed steroidogenesis, and markedly reduced 3β-HSD and 5α-reductase activity, early low-dose hydrocortisone may serve as a targeted intervention. This concept is supported by the PREMILOC trial, which demonstrated that early low-dose hydrocortisone reduces the incidence of BPD in extremely preterm infants (46). However, our data suggest that neonates with a steroid metabolomic profile indicative of global adrenal insufficiency may particularly benefit from such therapy, if introduced judiciously.
Conversely, in Cluster 2 neonates—who demonstrate elevated cortisol and cortisone levels and a higher risk of intraventricular hemorrhage—the decision to initiate steroid treatment may need to be delayed or withheld, as exogenous glucocorticoids could exacerbate cerebrovascular instability. In these infants, metabolomic screening could serve as a tool for exclusion, helping to prevent unnecessary or harmful intervention.
In cluster 3, where steroidogenesis appears to be preserved and clinical outcomes are favourable, routine postnatal steroid therapy may not be necessary. However, careful long-term monitoring could be considered, particularly in preterm females with signs suggestive of in utero androgen excess, given the potential for future metabolic or reproductive complications.
By integrating steroid metabolomic signatures with clinical stratification, this approach supports a personalized model of postnatal glucocorticoid therapy, optimizing timing, dosage, and indication based on objective biochemical markers, rather than generalized treatment algorithms. Such strategies may ultimately enhance respiratory adaptation, reduce complications, and minimize adverse neurodevelopmental outcomes.
Beyond mortality and respiratory concerns, Cluster 1 demonstrated a significant occurrence of SGA and IUGR, although only a small percentage of pregnancies within this cluster were complicated by preeclampsia. Since more than 60% of SGA cases remain idiopathic (38, 52, 53), an attenuated fetal adrenal function, exacerbated by excessive maternal glucocorticoids, could contribute to restricted in utero growth (11, 39, 40). Whilst a number of investigations have reported increased DHEA metabolites in IUGR neonates (20, 52), a recent study by Heckmann et al. (2024) similarly identified SGA infants within the cluster of globally reduced steroid excretion, thus reinforcing the notion that broadly suppressed steroidogenesis may help explain the growth deficits observed in Cluster 1 (19).
In the subsequent analysis, neonates in Cluster 2 demonstrated moderately elevated excretion of both C19 and C21 metabolites, in addition to high excretion F and E derivatives, distinguishing them from both Clusters 1 and 3. Alongside this heightened adrenal output, Cluster 2 exhibited a partial deficit in 3β-HSD activity and the highest incidence of IVH. These findings underscore that enhanced steroid synthesis does not necessarily confer neuroprotection in preterm infants. Indeed, while some data suggest that certain FZ steroids may possess neuroprotective capacity—particularly via estrogenic or progestogenic pathways (54, 55)—human evidence remains inconclusive. Del Valle et al. identified robust DHEA production in the fetal zone in the absence of 3β-HSD2 (1, 56, 57), but did not confirm a protective role for these steroids. In contrast, Heckmann et al. established a correlation between heightened FZ activity and an augmented risk of IVH (19). Moreover, an excess or dysregulation of cortisol secretion has been demonstrated to be associated with hemorrhagic complications in preterm populations, underscoring the tenuous equilibrium between beneficial and deleterious steroid effects (41, 42, 58).
From a mechanistic standpoint, elevated cortisol/cortisone derivatives have the potential to destabilize the fragile germinal matrix vasculature, which is highly susceptible to hemorrhage in very preterm infants (29). In this cluster, the high E and F excretion could be both a driver of cerebrovascular fragility and a response to the systemic stress of emerging IVH. Beyond IVH, neonates in Cluster 2 also faced a relatively high mortality risk, suggesting that hyperactivation of the adrenal axis may have broader clinical repercussions. Thus, the adrenal hyperactivation and imbalanced steroid production distinctive of Cluster 2 may, in practice, be more detrimental than protective, raising questions about the roles of backdoor steroid pathways and 11-oxo androgens in shaping cerebral perfusion and vascular integrity. Further research is warranted to clarify whether modulating these pathways could mitigate the cerebrovascular instability observed in this subgroup of preterm neonates.
Finally, neonates in Cluster 3 exhibited the highest excretion of C19 and C21 metabolites, along with intermediate levels of cortisol/cortisone derivatives. This robust steroidogenesis was sustained by elevated 3β-HSD and 5α-reductase activities, hinting at a vigorous yet relatively coordinated adrenal response. This endocrine profile is consistent with the observed low mortality risk in Cluster 3 infants, with no incidences of BPD or SGA/IUGR, thereby supporting the hypothesis that adequate steroid production promotes healthier neonatal adaptation (6, 16) (48),
With regard to IVH, it still occurred in Cluster 3, indicating that any potential neuroprotective influence of robust fetal zone steroidogenesis – such as the proposed protective effects of estrogens or other neuroactive steroids (54, 55) – may not be sufficiently potent to prevent IVH outright. Nevertheless, the overall clinical outlook for Cluster 3 remained favorable, highlighting that this group's balanced adrenal output helps mitigate many of the major complications seen in other clusters.
Beyond short-term outcomes, the distinct adrenal steroid profiles identified in our study may carry important implications for long-term health trajectories. Preterm neonates in Cluster 1, exhibiting globally reduced adrenal steroidogenesis and diminished 3β-HSD and 5α-reductase activity, may be at risk of impaired stress responses and suboptimal neurodevelopment. Although a prospective cohort of ELBW infants found no direct association between low early cortisol levels and neurodevelopmental impairment (41), theoretical concerns persist that prolonged hypoactivity of the hypothalamic–pituitary–adrenal (HPA) axis during critical periods may adversely affect brain maturation and long-term adaptive capacity.
In contrast, Cluster 2 neonates, characterized by elevated cortisol and cortisone levels, may be subject to endogenous glucocorticoid overexposure. Such hormonal profiles have previously been linked to an increased risk of intraventricular hemorrhage and cerebral palsy (41). Moreover, fetal glucocorticoid excess has been implicated in long-lasting alterations of HPA axis regulation, potentially predisposing to hypertension, insulin resistance, and behavioral disturbances in later life (11).
Lastly, although Cluster 3 infants demonstrated favorable early outcomes and preserved steroidogenesis, signs of androgen excess were noted, particularly among preterm females. Two such infants exhibited mild, non-progressive clitoromegaly, suggestive of in utero androgen excess. Elevated androgen levels in preterm infants have been associated with accelerated catch-up growth and increased risk of metabolic syndrome later in life (2). In females, these exposures may further predispose to polycystic ovary syndrome (PCOS) or insulin resistance, underscoring the potential long-term implications of early hyperandrogenism. Thus, Cluster 3 exemplifies how a robust and apparently well-regulated steroid profile may favor short-term clinical stability while still conferring select endocrine risks that merit ongoing surveillance.
in uteroThe principal strength of our study lies in its comprehensive coverage of the entire gestational age range, from early preterm to term, in conjunction with a thorough clinical characterization. A further advantage is the 24-hour urinary collection via Foley catheters and GC–MS analysis, a method that allows for complete metabolite assessment and serves to minimize sampling bias.
This study adopted an observational approach, which constrained the capacity to establish definitive causal relationships between steroid profiles and clinical outcomes. Additionally, while the sample population encompassed a diverse demographic, expanding it in future multicenter studies would enhance statistical reliability. Lastly, although clinical and metabolomic data were meticulously collected and analyzed, further prospective validation in larger cohorts is imperative to ascertain the practical applicability of the identified steroid signatures in neonatal care.
The findings of this study demonstrate the potential of metabolomic signatures to facilitate the stratification of neonates according to their adrenal steroid profiles, thus offering a promising avenue for the delivery of personalized neonatal care. However, in order to establish which steroid patterns correlate with specific conditions or outcomes, the execution of larger-scale studies is indispensable. In addition, the application of advanced computational methods, such as artificial intelligence (AI) and neural network approaches, which extend beyond K-means clustering, may provide further insight into these complex metabolic patterns. By leveraging AI-driven analytics, clinicians could more accurately identify, classify, and predict high-risk neonatal phenotypes, thus enhancing diagnostic precision and ultimately improving patient outcomes (59).
Conclusions
Metabolomic clustering revealed distinct adrenal steroid profiles associated with neonatal health outcomes, highlighting the complex interplay between steroidogenesis and clinical risks. Profoundly suppressed adrenal activity, characterized by low C19 and C21 steroid excretion and diminished 3β-HSD and 5α-reductase activity, was linked to increased risks of mortality, BPD, and growth restrictions (SGA/IUGR), emphasizing the importance of addressing global deficits in steroidogenesis. Conversely, adrenal hyperactivation, marked by elevated cortisol and cortisone derivatives alongside moderately increased C19 and C21 metabolites and partial 3β-HSD deficits, was associated with a heightened risk of IVH and mortality, suggesting that excessive glucocorticoid output can exacerbate neonatal vascular fragility. The most favorable short-term outcomes were demonstrated by neonates with robust steroidogenesis, as indicated by high C19 and C21 excretion and high 3β-HSD and 5α-reductase activity, who exhibited the lowest mortality rates and absence of BPD or SGA/IUGR. These findings emphasize the potential of metabolomic signatures to guide personalized interventions, improving both immediate and future neonatal outcomes.
Limitations
The study was conducted in a highly specific clinical population—neonates requiring intensive care and urinary catheterization during the first 24 hours of life—which limits the applicability of results to healthy term infants. Furthermore, the absence of a healthy control group precludes direct comparisons with physiological reference patterns. Finally, although 24-hour urine collection offers high diagnostic value, its feasibility is limited to selected clinical settings. These aspects underscore the pilot nature of the study and provide a foundation for future prospective validation.
Data availability statement
The original contributions presented in the study are included in the article/Supplementary Material, further inquiries can be directed to the corresponding author.
Ethics statement
The studies involving humans were approved by Ethics Committee of Silesian Medical University in Katowice (PCN/CBN/0052/KB1/99/I/21/22/23). The studies were conducted in accordance with the local legislation and institutional requirements. Written informed consent for participation in this study was provided by the participants' legal guardians/next of kin.
Author contributions
ML: Formal Analysis, Investigation, Methodology, Visualization, Writing – original draft, Writing – review & editing. TS: Data curation, Resources, Software, Writing – original draft. AS: Data curation, Resources, Software, Writing – original draft. JF: Investigation, Resources, Writing – original draft. DJ: Conceptualization, Supervision, Validation, Writing – original draft. AG: Conceptualization, Funding acquisition, Project administration, Supervision, Validation, Writing – original draft.
Funding
The author(s) declare that financial support was received for the research and/or publication of this article. This study was supported by the research project No. PCN-1-165/N/2/0, under agreement BNW-NWN/3116/2023, conducted in the Department of Pediatrics and Pediatric Endocrinology, Medical University of Silesia, Katowice, Poland.
Conflict of interest
The authors declare that the research was conducted in the absence of any commercial or financial relationships that could be construed as a potential conflict of interest.
Generative AI statement
The author(s) declare that Generative AI was used in the creation of this manuscript. The language editing process for this manuscript involved the utilisation of ChatGPT-1 (version 1.0, GPT-based language model, source: OpenAI), with the ultimate responsibility for the content of the manuscript lying exclusively with the authors.
Publisher’s note
All claims expressed in this article are solely those of the authors and do not necessarily represent those of their affiliated organizations, or those of the publisher, the editors and the reviewers. Any product that may be evaluated in this article, or claim that may be made by its manufacturer, is not guaranteed or endorsed by the publisher.
Supplementary material
The Supplementary Material for this article can be found online at: https://www.frontiersin.org/articles/10.3389/fendo.2025.1569355/full#supplementary-material
References
1. Del Valle I, Young MD, Kildisiute G, Ogunbiyi OK, Buonocore F, Simcock IC, et al. An integrated single-cell analysis of human adrenal cortex development. JCI Insight. (2023) 8(14):e168177. doi: 10.1172/jci.insight.168177
2. Greaves RF, Wudy SA, Badoer E, Zacharin M, Hirst JJ, Quinn T, et al. A tale of two steroids: The importance of the androgens DHEA and DHEAS for early neurodevelopment. J Steroid Biochem Mol Biol. (2019) 188:77–85. doi: 10.1016/j.jsbmb.2018.12.007
3. Schiffer L, Barnard L, Baranowski ES, Gilligan LC, Taylor AE, Arlt W, et al. Human steroid biosynthesis, metabolism and excretion are differentially reflected by serum and urine steroid metabolomes: A comprehensive review. J Steroid Biochem Mol Biol. (2019) 194:105439. doi: 10.1016/j.jsbmb.2019.105439
4. Liimatta J, du Toit T, Voegel CD, Jääskeläinen J, Lakka TA, and Flück CE. Multiple androgen pathways contribute to the steroid signature of adrenarche. Mol Cell Endocrinol. (2024) 592:112293. doi: 10.1016/j.mce.2024.112293
5. du Toit T Swart AC BL. Back where it belongs: 11β-hydroxyandrostenedione compels the re-assessment of C11-oxy androgens in steroidogenesis. Mol Cell Endocrinol. (2021) 525:111189. doi: 10.1016/j.mce.2021.111189
6. Flück CE, Kuiri-Hänninen T, Silvennoinen S, Sankilampi U, and Groessl M. The androgen metabolome of preterm infants reflects fetal adrenal gland involution. J Clin Endocrinol Metab. (2022) 107:3111–9. doi: 10.1210/clinem/dgac482
7. Miller WL and Flück CE. CHAPTER 13 - Adrenal cortex and its disorders. In: Sperling MA, editor. Pediatric Endocrinology, 4th ed. Philadelphia: Elsevier. (2014). p. 471–532.e1.
8. Storbeck K-H, Schiffer L, Baranowski ES, Chortis V, Prete A, Barnard L, et al. Steroid metabolome analysis in disorders of adrenal steroid biosynthesis and metabolism. Endocr Rev. (2019) 40:1605–25. doi: 10.1210/er.2018-00262
9. Ishimoto H and Jaffe RB. Development and function of the human fetal adrenal cortex: a key component in the feto-placental unit. Endocr Rev. (2011) 32:317–55. doi: 10.1210/er.2010-0001
10. Van den Berghe G, Téblick A, Langouche L, and Gunst J. The hypothalamus-pituitary-adrenal axis in sepsis- and hyperinflammation-induced critical illness: Gaps in current knowledge and future translational research directions. EBioMedicine. (2022) 84:104284. doi: 10.1016/j.ebiom.2022.104284
11. Zhu P, Wang W, Zuo R, and Sun K. Mechanisms for establishment of the placental glucocorticoid barrier, a guard for life. Cell Mol Life Sci. (2019) 76:13–26. doi: 10.1007/s00018-018-2918-5
12. Committee UKNS. External review against programme appraisal criteria for the UK National Screening Committee (2023). Available online at: https://www.gov.uk/government/publications/external-review-against-programme-appraisal-criteria (Accessed Aug 17, 2024).
13. Buonocore F, Maharaj A, Qamar Y, Koehler K, Suntharalingham JP, Chan LF, et al. Genetic analysis of pediatric primary adrenal insufficiency of unknown etiology: 25 years' Experience in the UK. J Endocr Soc. (2021) 5:bvab086. doi: 10.1210/jendso/bvab086
14. Held PK, Bird IM, and Heather NL. Newborn screening for congenital adrenal hyperplasia: review of factors affecting screening accuracy. Screening. (2020) 6:67. doi: 10.3390/ijns6030067
15. Vidarsdottir H, Thorkelsson T, Halldorsson TI, Bjarnason R, Geirsson RT, Rinaldo P, et al. Does metabolomic profile differ with regard to birth weight? Pediatr Res. (2021) 89:1144–51. doi: 10.1038/s41390-020-1033-0
16. Lal CV, Bhandari V, and Ambalavanan N. Genomics, microbiomics, proteomics, and metabolomics in bronchopulmonary dysplasia. Semin Perinatol. (2018) 42:425–31. doi: 10.1053/j.semperi.2018.09.004
17. Fanos V, Pintus MC, Lussu M, Atzori L, Noto A, Stronati M, et al. Urinary metabolomics of bronchopulmonary dysplasia (BPD): preliminary data at birth suggest it is a congenital disease. J Matern Fetal Neonatal Med. (2014) 27 Suppl 2:39–45. doi: 10.3109/14767058.2014.955966
18. Hübner S, Reich B, and Heckmann M. Role of sex steroids and their receptors in human preterm infants: Impacts on future treatment strategies for cerebral development. Biochem Pharmacol. (2015) 98:556–63. doi: 10.1016/j.bcp.2015.08.093
19. Heckmann M, Runkel AS, Sunny DE, Hartmann MF, Ittermann T, and Wudy SA. Steroid metabolomic signature in term and preterm infants. Biomolecules. (2024) 14:5–15. doi: 10.3390/biom14020235
20. Priante E, Verlato G, Stocchero M, Giordano G, Pirillo P, Bonadies L, et al. Metabolomic profiling of intrauterine growth-restricted preterm infants: a matched case-control study. Pediatr Res. (2023) 93:1599–608. doi: 10.1038/s41390-022-02292-5
21. Honour JW, Conway E, Hodkinson R, and Lam F. The evolution of methods for urinary steroid metabolomics in clinical investigations particularly in childhood. J Steroid Biochem Mol Biol. (2018) 181:28–51. doi: 10.1016/j.jsbmb.2018.02.013
22. Gawlik A, Shmoish M, Hartmann MF, Malecka-Tendera E, Wudy SA, and Hochberg Z. Steroid metabolomic disease signature of nonsyndromic childhood obesity. J Clin Endocrinol Metab. (2016) 101:4329–37. doi: 10.1210/jc.2016-1754
23. Ikotun AM, Ezugwu AE, Abualigah L, Abuhaija B, and Heming J. K-means clustering algorithms: A comprehensive review, variants analysis, and advances in the era of big data. Inf Sci. (2023) 622:178–210. doi: 10.1016/j.ins.2022.11.139
24. Kamrath C, Friedrich C, Hartmann MF, and Wudy SA. Metabotypes of congenital adrenal hyperplasia in infants determined by gas chromatography-mass spectrometry in spot urine. J Steroid Biochem Mol Biol. (2023) 231:106304. doi: 10.1016/j.jsbmb.2023.106304
25. Ye J, Zhuang X, Li X, Gong X, Sun Y, Wang W, et al. Novel metabolic classification for extrahepatic complication of metabolic associated fatty liver disease: A data-driven cluster analysis with international validation. Metabolism. (2022) 136:155294. doi: 10.1016/j.metabol.2022.155294
26. Kuppusamy P, Prusty RK, and Kale DP. High-risk pregnancy in India: Prevalence and contributing risk factors - a national survey-based analysis. J Glob Health. (2023) 13:04116. doi: 10.7189/jogh.13.04116
27. Torloni MR, Betran AP, Souza JP, Widmer M, Allen T, Gulmezoglu M, et al. Classifications for cesarean section: a systematic review. PloS One. (2011) 6:e14566. doi: 10.1371/journal.pone.0014566
28. Kinsella JP, Greenough A, and Abman SH. Bronchopulmonary dysplasia. Lancet. (2006) 367:1421–31. doi: 10.1016/S0140-6736(06)68615-7
29. Bowerman RA, Donn SM, Silver TM, and Jaffe MH. Natural history of neonatal periventricular/intraventricular hemorrhage and its complications: sonographic observations. AJR Am J Roentgenol. (1984) 143:1041–52. doi: 10.2214/ajr.143.5.1041
30. Battaglia FC and Lubchenco LO. A practical classification of newborn infants by weight and gestational age. J Pediatr. (1967) 71:159–63. doi: 10.1016/S0022-3476(67)80066-0
31. Gordijn SJ, Beune IM, Thilaganathan B, Papageorghiou A, Baschat AA, Baker PN, et al. Consensus definition of fetal growth restriction: a Delphi procedure. Ultrasound Obstet Gynecol. (2016) 48:333–9. doi: 10.1002/uog.1588
32. McNamara PJ, Jain A, El-Khuffash A, Giesinger R, Weisz D, Freud L, et al. Guidelines and recommendations for targeted neonatal echocardiography and cardiac point-of-care ultrasound in the neonatal intensive care unit: An update from the American Society of echocardiography. J Am Soc Echocardiogr. (2024) 37:171–215. doi: 10.1016/j.echo.2023.11.016
33. Singh M, Alsaleem M, and Gray CP. Neonatal sepsis. In: StatPearls [Internet]. Treasure Island (FL): StatPearls Publishing. (2022). Available from: https://www.ncbi.nlm.nih.gov/books/NBK531478/.
34. Richardson DK, Corcoran JD, Escobar GJ, and Lee SK. SNAP-II and SNAPPE-II: Simplified newborn illness severity and mortality risk scores. J Pediatr. (2001) 138:92–100. doi: 10.1067/mpd.2001.109608
35. Vitkin E, Ben-Dor A, Shmoish M, Hartmann MF, Yakhini Z, Wudy SA, et al. Peer group normalization and urine to blood context in steroid metabolomics: The case of CAH and obesity. Steroids. (2014) 88:83–9. doi: 10.1016/j.steroids.2014.07.003
36. Zuckerman-Levin N, Tsivlin L, Knopf C, Flor O, Shen-Orr Z, Levin M, et al. 11β–hydroxysteroid dehydrogenase type 1 activity in short small-for-GA children and in response to GH therapy. Pediatr Res. (2011) 70(2):208–12. doi: 10.1203/PDR.0b013e3182226a0c
37. Heckmann M, Hartmann MF, Kampschulte B, Gack H, Bödeker R-H, Gortner L, et al. Assessing cortisol production in preterm infants: do not dispose of the nappies. Pediatr Res. (2005) 57:412–8. doi: 10.1203/01.PDR.0000153947.51642.C1
38. Pignatti E, du Toit T, and Flück CE. Development and function of the fetal adrenal. Rev Endocr Metab Disord. (2023) 24:5–21. doi: 10.1007/s11154-022-09756-3
39. Johnston RC, Faulkner M, Carpenter PM, Nael A, Haydel D, Sandman CA, et al. Associations between placental corticotropin-releasing hormone, maternal cortisol, and birth outcomes, based on placental histopathology. Reprod Sci. (2020) 27:1803–11. doi: 10.1007/s43032-020-00182-x
40. Siemiątkowska A, Kosicka K, Szpera-Goździewicz A, Krzyścin M, Bręborowicz GH, and Główka FK. Cortisol metabolism in pregnancies with small for gestational age neonates. Sci Rep. (2019) 9:17890. doi: 10.1038/s41598-019-54362-0
41. Aucott SW, Watterberg KL, Shaffer ML, Donohue PK, and PROPHET study group. Early cortisol values and long-term outcomes in extremely low birth weight infants. J Perinatol. (2010) 30:484–8. doi: 10.1038/jp.2009.191
42. Renolleau C, Toumazi A, Bourmaud A, Benoist J-F, Chevenne D, Mohamed D, et al. Association between baseline cortisol serum concentrations and the effect of prophylactic hydrocortisone in extremely preterm infants. J pediatrics. (2021) pp:65–70.e3. doi: 10.1016/j.jpeds.2020.12.057
43. Valerio E, Meneghelli M, Stocchero M, Galderisi A, Visentin S, Bonadies L, et al. The impact of antenatal corticosteroids on the metabolome of preterm newborns: an untargeted approach. Int J Mol Sci. (2024) 25:4. doi: 10.3390/ijms25115860
44. Committee on Obstetric Practice. Committee opinion no. 713: antenatal corticosteroid therapy for fetal maturation. Obstet Gynecol. (2017) 130:e102–9. doi: 10.1097/AOG.0000000000002237
45. Baraldi E, Giordano G, Stocchero M, Moschino L, Zaramella P, Tran MR, et al. Untargeted metabolomic analysis of amniotic fluid in the prediction of preterm delivery and bronchopulmonary dysplasia. PloS One. (2016) 11:e0164211. doi: 10.1371/journal.pone.0164211
46. Baud O, Maury L, Lebail F, Ramful D, El Moussawi F, Nicaise C, et al. Effect of early low-dose hydrocortisone on survival without bronchopulmonary dysplasia in extremely preterm infants (PREMILOC): a double-blind, placebo-controlled, multicentre, randomised trial. Lancet. (2016) 387:1827–36. doi: 10.1016/S0140-6736(16)00202-6
47. Onland W, Cools F, Kroon A, Rademaker K, Merkus MP, Dijk PH, et al. Effect of hydrocortisone therapy initiated 7 to 14 days after birth on mortality or bronchopulmonary dysplasia among very preterm infants receiving mechanical ventilation: A randomized clinical trial. JAMA. (2019) 321:354–63. doi: 10.1001/jama.2018.21443
48. Watterberg KL, Walsh MC, Li L, Chawla S, D'Angio CT, Goldberg RN, et al. Hydrocortisone to improve survival without bronchopulmonary dysplasia. N Engl J Med. (2022) 386:1121–31. doi: 10.1056/NEJMoa2114897
49. Torgerson D, Guardado M, Steurer M, Chapin C, Hernandez RD, and Ballard PL. The hydrocortisone-responsive urinary metabolome of premature infants. Pediatr Res. (2023) 94:1317–26. doi: 10.1038/s41390-023-02610-5
50. Malavolti AM, Bassler D, Arlettaz-Mieth R, Faldella G, Latal B, and Natalucci G. Bronchopulmonary dysplasia-impact of severity and timing of diagnosis on neurodevelopment of preterm infants: a retrospective cohort study. BMJ Paediatr Open. (2018) 2:e000165. doi: 10.1136/bmjpo-2017-000165
51. Kelly EN, Shah VS, Levenbach J, Vincer M, DaSilva O, Shah PS, et al. Inhaled and systemic steroid exposure and neurodevelopmental outcome of preterm neonates. J Matern Fetal Neonatal Med. (2018) 31:2665–72. doi: 10.1080/14767058.2017.1350644
52. Allvin K, Ankarberg-Lindgren C, Niklasson A, Jacobsson B, and Dahlgren J. Altered umbilical sex steroids in preterm infants born small for gestational age. J Matern Fetal Neonatal Med. (2020) 33:4164–70. doi: 10.1080/14767058.2019.1598362
53. Hung T-H, Hsieh T-T, Lo L-M, Chiu T-H, Hsieh C-C, and Hsu J-J. Risk factors and perinatal outcomes associated with idiopathic small for gestational age Taiwanese newborns. Int J Gynaecol Obstet. (2013) 122:212–5. doi: 10.1016/j.ijgo.2013.03.033
54. Suzuki S, Brown CM, and Wise PM. Mechanisms of neuroprotection by estrogen. Endocrine. (2006) 29:209–15. doi: 10.1385/ENDO:29:2:209
55. Stein DG. Progesterone exerts neuroprotective effects after brain injury. Brain Res Rev. (2008) 57:386–97. doi: 10.1016/j.brainresrev.2007.06.012
56. Novoselova TV, King PJ, Guasti L, Metherell LA, Clark AJL, and Chan LF. ACTH signalling and adrenal development: lessons from mouse models. Endocr Connect. (2019) 8:R122–30. doi: 10.1530/EC-19-0190
57. Sunny DE, Krüger EL, Hammer E, Völker U, and Heckmann M. Fetal zone steroids show discrete effects on hyperoxia-induced attenuation of migration in cultured oligodendrocyte progenitor cells. Oxid Med Cell Longev. (2022) 2022:2606880. doi: 10.1155/2022/2606880
58. Heckmann M, Hartmann MF, Kampschulte B, Gack H, Bödeker R-H, Gortner L, et al. Cortisol production rates in preterm infants in relation to growth and illness: a noninvasive prospective study using gas chromatography-mass spectrometry. J Clin Endocrinol Metab. (2005) 90:5737–42. doi: 10.1210/jc.2005-0870
Keywords: neonates, adrenals, steroidogenesis, metabolomics, K-means clustering
Citation: Lorek M, Stradomska TJ, Siejka A, Fuchs J, Januś D and Gawlik-Starzyk A (2025) Metabolomic fingerprints of clustered preterm and term neonates – a pilot study. Front. Endocrinol. 16:1569355. doi: 10.3389/fendo.2025.1569355
Received: 31 January 2025; Accepted: 23 April 2025;
Published: 16 May 2025.
Edited by:
Emir Muzurovic, Clinical Center of Montenegro, MontenegroReviewed by:
Kai-Sheng Hsieh, China Medical University, TaiwanTong Wu, Purdue University Indianapolis, United States
Copyright © 2025 Lorek, Stradomska, Siejka, Fuchs, Januś and Gawlik-Starzyk. This is an open-access article distributed under the terms of the Creative Commons Attribution License (CC BY). The use, distribution or reproduction in other forums is permitted, provided the original author(s) and the copyright owner(s) are credited and that the original publication in this journal is cited, in accordance with accepted academic practice. No use, distribution or reproduction is permitted which does not comply with these terms.
*Correspondence: Miłosz Lorek, bG9yZWsubWlsb3N6QGdtYWlsLmNvbQ==