- 1Laboratorio de Biotecnología Molecular, Facultad de Ciencias de la Vida, Universidad Andrés Bello, Santiago, Chile
- 2Interdisciplinary Center for Aquaculture Research (INCAR), University of Concepción, Concepcion, Chile
- 3Laboratory of Biotechnology and Aquatic Genomics, Department of Oceanography, University of Concepción, Concepcion, Chile
- 4Centro de Investigación Marina Quintay (CIMARQ), Universidad Andrés Bello, Quintay, Chile
Background: Chronic stress is a critical challenge in fish aquaculture, adversely affecting growth, health, and overall productivity. Among the most significant chronic stressors in intensive farming is crowding, which triggers the release of cortisol, the primary stress hormone in fish. Cortisol re-allocates energy away from growth-related processes toward stress response mechanisms. Consequently, overcrowded fish often exhibit slower growth rates, and impaired skeletal muscle development. Understanding the mechanisms underlying crowding stress and their long-term effects, including epigenetic changes, is essential for optimizing farming conditions, and enhancing fish welfare.
Objective: This study aims to characterize the physiological, transcriptomic, and epigenomic responses in juvenile rainbow trout (Oncorhynchus mykiss) exposed for 30 days to high stocking densities.
Results: Crowding stress led to decreased weight in the high-density (HD) group. It also resulted in elevated cortisol levels, oxidative DNA damage, and protein carbonylation in skeletal muscle. Using RNA-seq, we identified 4,050 differentially expressed genes (DEGs), and through whole-genome bisulfite sequencing (WGBS), we detected 11,672 differentially methylated genes (DMGs). Integrative analyses revealed 263 genes with a negative correlation between upregulated expression and downregulated methylation, primarily associated with autophagy, mitophagy, and the insulin signaling pathway. Conversely, 299 genes exhibited the reverse trend, mainly linked to ATP-dependent chromatin remodeling.
Conclusion: This study offers the first detailed exploration of the molecular responses in skeletal muscle to crowding stress, integrating RNA-seq and WGBS analysis in rainbow trout, offering valuable information for improving aquaculture practices.
1 Introduction
Fish aquaculture plays a vital role in meeting the growing global demand for seafood, contributing significantly to food security, economic development, and environmental sustainability (1). Salmonid farming is among the most important sectors of fish aquaculture, making a substantial contribution to global aquatic food production (2). While Atlantic salmon (Salmo salar) dominates the global market, Rainbow trout (Oncorhynchus mykiss) farming is a critical component of salmonid aquaculture, particularly in freshwater systems (3). Renowned for its adaptability, rapid growth, and nutritional value, rainbow trout is cultivated in diverse environments supporting both small- and large-scale production (4, 5). In 2022, inland and coastal aquaculture of rainbow trout reached a volume of 959,600 tonnes, valued at USD 3.2 billion (6). Despite advances in aquaculture, intensive systems often induce stress, harming fish health, growth, and welfare (7). Common stressors include poor water quality, overcrowding, handling during transport or harvest, temperature fluctuations, and disease outbreaks (8). These challenges trigger physiological stress responses, most notably the release of cortisol, the primary stress hormone in fish (9). Chronic stress impairs both innate and adaptive immune responses, increasing the susceptibility to diseases (10). Chronic stress significantly affects the psychological and ethological condition of fish, impacting their behavior and social interactions (11, 12). It disrupts metabolism, diverting energy from growth to survival, reducing feed intake, and accelerating muscle loss (13, 14). In addition, Chronic stress weakens muscle fibers, slowing growth, reducing weight, and impairing swimming (15–19).
Chronic stress in fish can induce significant oxidative stress in skeletal muscle, adversely affecting cellular health, growth, and overall fish quality (20). Prolonged stress elevates cortisol levels, which increases metabolic rates and leads to production of ROS (reactive oxygen species). These ROS can damage critical cellular components, including lipids and DNA (21). Oxidative stress also promotes protein carbonylation, altering protein structure and disrupting essential muscle functions such as metabolism and contraction efficiency (22). Moreover, chronic stress may suppress the expression of antioxidant enzymes, rendering muscle tissue more susceptible to sustained oxidative damage (23, 24). A recent study on Rainbow trout demonstrated that chronic stress from high stocking densities elevates cortisol levels, disrupting the balance of muscle protein turnover. This imbalance affects three critical proteolytic pathways: the UPS (ubiquitin-proteasome system), the ALS (autophagy-lysosome system), and the calpain system (25). These pathways, essential for maintaining muscle integrity and supporting growth, become dysregulated under chronic stress, resulting in muscle degradation, and reduced growth rate (25). Similarly, a study on Fine flounder (Paralichthys adspersus) found that crowding stress elevated cortisol levels, disrupting growth-promoting pathways such as the growth hormone/insulin-like growth factor axis. This stress also activated muscle breakdown mechanisms through the ubiquitin-proteasome system and autophagy, further inhibiting growth (26).
Chronic stress is increasingly recognized for its influence on epigenetic memory in fish, affecting not only immediate physiological responses but also long-term gene ex-pression patterns (27, 28). Epigenetic modifications, such as DNA methylation and histone modifications, serve as mechanisms through which environmental stressors, including chronic stress, leave lasting imprints on the genome without modifying the fundamental DNA sequence (29). These stress-induced epigenetic changes can impact key biological processes, including growth, immune function, metabolism, and reproductive capacity. Furthermore, they may influence not only the stressed individual but also subsequent generations (27). By modulating gene expression in a long-lasting manner, epigenetic memory enables fish to adapt to recurring stressors. However, it can also result in maladaptive outcomes, such as reduced growth efficiency or heightened disease susceptibility. Although there are no studies specifically examining the effects of crowding on growth in aquaculture-relevant species, its epigenetic impacts have been studied in zebrafish (Danio rerio). Recent research revealed that maintaining zebrafish larvae at high densities led to changes in the methylation of promoter regions associated with DNA maintenance methylation, reproduction, and stress response (30). Similarly, in adult male zebrafish skin, a global reduction in DNA methylation levels was observed, particularly in processes linked to immune response and oxidative stress (31). Understanding how chronic stress shapes epigenetic memory in fish is essential for improving fish health and welfare in aquaculture. This knowledge paves the way for developing strategies to mitigate the long-term negative effects of stress. As sentient beings, fish experience pain and stress in ways comparable to mammals, making their welfare a critical consideration in aquaculture and research practices. In the present study, we examined the effects of crowding on juvenile Rainbow trout reared at high stocking densities using RNA-Seq and whole-genome bisulfite sequencing (WGBS). This study aims to provide a comprehensive analysis of the physiological and molecular impacts of crowding stress in rainbow trout, with a focus on the potential long-term negative effects of these farming practices. We identified numerous differentially expressed and methylated genes, many of which were associated with critical processes involved in skeletal muscle catabolism and atrophy, including autophagy/mitophagy, insulin signaling pathway, and ATP-dependent chromatin remodeling.
2 Methodology
2.1 Protocol experiment
This study followed bioethical protocol authorized by the ethical committee of the Universidad Andres Bello (protocol code 010/2023). Juvenile Rainbow trout (O. mykiss) (7.24 ± 1.35 g) were obtained from Pisciculture Rio Blanco (PUCV, Chile) and randomly assigned to six 16 L tanks with aerated, dechlorinated water at 15 ± 1°C. Four tanks contained 10 individuals, corresponding to low-density group (LD) (control condition) (10 kg/m3), and four tanks contained 30 individuals, corresponding to high-density condition (HD) (experimental condition) (30 kg/m³). Experiments were carried out in 100-cm high tanks with water turnover rate of 3.5 l/min ensuring an oxygen concentration of 7 mg/l. The Rainbow trout were fed daily with salmon pellets at 1.5% of their body weight under a 12:12 h light-dark cycle. In all groups it was verified that food consumption was the same. Fish from LD and HD groups were sampled at 30 days of crowding. Six fish per tank were randomly euthanized under anesthesia (benzocaine 300 mg/l). Time sampling and rearing density were defined based on the experience and results obtained in Valenzuela et al. (25). Blood from caudal vessel was collected using 1 mL heparinized (10 mg/mL) syringe, then centrifuged at 2000 x g for 5 min at 4°C to collect plasma, immediately stored at − 80°C. Myotome skeletal muscle was sampled from all fish, specifically a cross section from the epaxial area. Finally, samples were frozen by liquid nitrogen and stored at − 80°C until processed for RNA and DNA extraction.
2.2 Growth performance, physiological, and oxidative stress parameters
On day 0 and 30 of the trial, body weight (g) and total length (cm) of each sampled fish were measured. The condition factor (K) was calculated as K= (W/l3) x 100, where W is body weight and l is the total body length. The plasmatic levels of cortisol, creatine kinase activity, and glucose, were quantified using the Cortisol ELISA Kit (Cayman Chemical, Ann Arbor, MI, USA), Creatine Kinase Assay Kit (Abcam, Cambridge, UK), and Glucose Assay Kit (Abcam, Cambridge, UK), respectively. DNA oxidative damage, protein carbonylation, and lipid peroxidation were determined using OxiSelect Oxidative DNA Damage Quantification (Cell Biolabs, San Diego, CA, USA), OxiSelect Protein Carbonyl Spectrophotometric Assay (Cell Biolabs, San Diego, CA, USA), and OxiSelect HNE Adduct Competitive ELISA Kit (Cell Biolabs, San Diego, CA, USA) respectively. Catalase (CAT) and superoxide dismutase (SOD) activities were quantified using the Abcam catalase (Abcam, Cambridge, UK) and superoxide dismutase Activity Assay Kit (Abcam, Cambridge, UK), respectively. The use of this methodology in fish tissue has been previously validated (32). The parameters measured and the catalog code used are summarized in the Supplementary Table S1.
2.3 RNA extraction, RNA sequencing, and transcriptome analysis
Total RNA was extracted from Rainbow trout skeletal muscle of the LD and HD groups. RNA extraction was performed using the Trizol Reagent (Ambion, Carlsbad, CA, USA), following the manufacturer’s instructions. RNA integrity was confirmed by electrophoresis in agarose gel (1.2%). The isolated RNA’s quality, purity, and quantity were measured in TapeStation 2200 (Agilent Technologies Inc., Santa Clara, CA, USA). Three individuals from the LD group and three individuals from the HD group were randomly selected, for cDNA libraries construction. cDNA libraries were generated using the TruSeq RNA Sample Preparation kit v2 (Illumina, USA). NGS sequencing was performed by the Macrogen Inc. (Seoul, Korea) company on the Novaseq Illumina platform. Using a paired-end technique (2 x101 bp). Raw reads were trimmed removing sequences of low quality (Q20) and those less than 40 pb. Trimmed reads were mapped onto the reference rainbow trout genome USDA_OmykA_1.1 (RefSeq GCF_013265735.2) using CLC genomic workbench software v23 (CLC bio - Qiagen, TX, USA). The settings were minimum length fraction = 0.8 and minimum similarity fraction (long reads) = 0.8; the expression value was established as transcripts per million reads (TPM). The distance metric was calculated with the Manhattan method, and Kal’s test was used to compare gene expression levels in fold change (P = 0.0005, FDR corrected). Differential expression analysis was conducted for tissue samples and filtered by absolute fold-change values ≥ 2 and FDR corrected p-value < 0.05. The identification of GO and KEGG enrichment analysis of DEGs was previously described (33).
2.4 DNA extraction, WGBS sequencing, and methylome analysis
Genomic DNA was extracted using DNeasy Blood & Tissue Kit (Qiagen, TX, USA) following the manufacturer’s recommendations. DNA concentration and integrity were determined by Qubit dsDNA BR Assay (Invitrogen, CA, USA) and agarose Gel Electrophoresis, respectively. Genomic DNAs were fragmented into 100-300 bp by Sonication (Covaris, Massachusetts, USA) followed by bisulfite processing. 6 DNA library were prepared by Zymo-Seq WGBS Library Kit (ZymoResearch, USA), using 100 ng of input DNA. Sequencing was performed using a paired-end strategy (2 x 150 bp) with the Novaseq (Illumina) platform of Macrogen (Seul, Korea). Raw reads were trimmed removing sequences of low quality (Q20) and those less than 40 pb. Trimmed reads were analyzed using the Bisulfite sequencing tools on using CLC genomic workbench software v23 (CLC bio-Qiagen, TX, USA). Bisulfite data were mapped to Rainbow trout genome USDA_OmykA_1.1 (RefSeq GCF_013265735.2) to locate the CG, CHG, and CHH markers. The methylation levels were determined with the tool Call Methylation levels of CLC Genomics Workbench v23 (CLC bio-Qiagen, TX, USA). Finally, the differentially methylated nearby genes were extracted from the O. mykiss genome using the CLC Genomics Workbench v23 (CLC bio-Qiagen, TX, USA). The identification of GO and KEGG enrichment analysis of DMGs was previously described (34).
2.5 RT- qPCR validation
RNA extraction was performed using the Trizol Reagent (Ambion, Carlsbad, CA, USA), following the manufacturer’s instructions. RNA integrity was confirmed by electrophoresis in agarose gel (1.2%). The RNA was quantified using NanoDrop technology (BioTek Instruments, VT, USA). For cDNA synthesis, 1 μg of RNA was reverse transcribed into cDNA using 5X All-In-One RT MasterMix (Applied Biological Materials Inc., Richmond, BC, Canada) under manufacturer recommendations. The real-time PCR (qPCR) was performed in a MX3000P thermocycler (Agilent Technologies, CA, USA), following the previously described protocol (35). Relative expression level of mRNA was calculated using the 2−ΔΔCt method. Relative expression analysis was calculated using geNorm software (https://genorm.cmgg.be/), and the results are expressed as the fold induction compared with control group and using beta-actin (actβ) and 40S ribosomal protein S30 (fau) as housekeeping genes. We used Primer3 software (36) for primer design (Supplementary Table S2).
2.6 Statistical analysis
Growth performance data were analyzed by the two-way ANOVA followed by Tukey’s multiple range tests. Physiological and oxidative stress data were analyzed by a non-parametric Student’s t-test. Data was presented as average ± standard error, and the statistical significance was set at P < 0.05. Data for RT-qPCR validation were analyzed by one-way ANOVA and Tukey’s honestly significant difference as a post hoc test. Correlation analysis between RNA-seq and Q-RT-PCR was performed using Pearson’s correlation coefficient. All statistical analyses were performed with Graph Prism 7.0 software (GraphPad Software, Inc., San Diego, CA).
3 Results
3.1 Physiological analysis of crowding stress
To evaluate the effects of crowding on Rainbow trout growth performance, total weight (g), body length (cm), and condition factor (K) were measured. After 30 days of crowding stress, the high-density (HD) group exhibited significantly lower weight (p-value = 0.0285) (Figure 1A) compared to the low-density (LD) group. No significant differences in length (Figure 1B) were observed between the HD and LD groups at 30 days crowding stress (p-value = 0.9970). No significant differences in condition factor (p-value = 0.9207) (Figure 1C) were observed between the HD and LD groups.
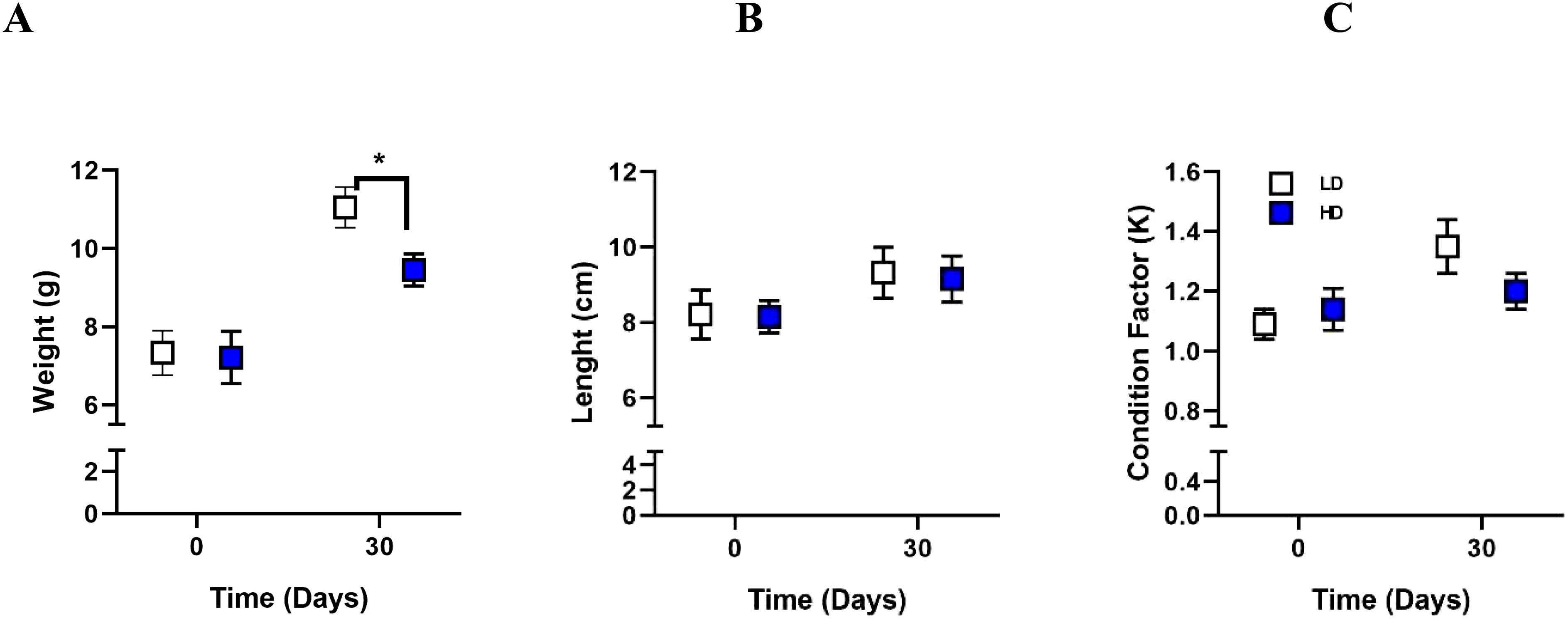
Figure 1. Assessment of growth performance to crowding. Weight (A), length (B), and condition factor K (C) were assessed in Rainbow trout at 30 days under Low Density (LD) and High Density (HD) conditions. The results are expressed as means ± standard error (n = 4). Differences between LD and HD groups are shown in *p < 0.05.
To assess stress levels in the LD and HD groups, plasma cortisol, glucose, and creatine kinase (CK) activity were measured. After 30 days, cortisol levels (p-value = 0.0231) (Figure 2A) were significantly higher in the HD group compared to the LD group. No significant differences in plasma glucose levels (p-value = 0.5502) (Figure 2B) and CK activity (p-value = 0.3152) (Figure 2C).
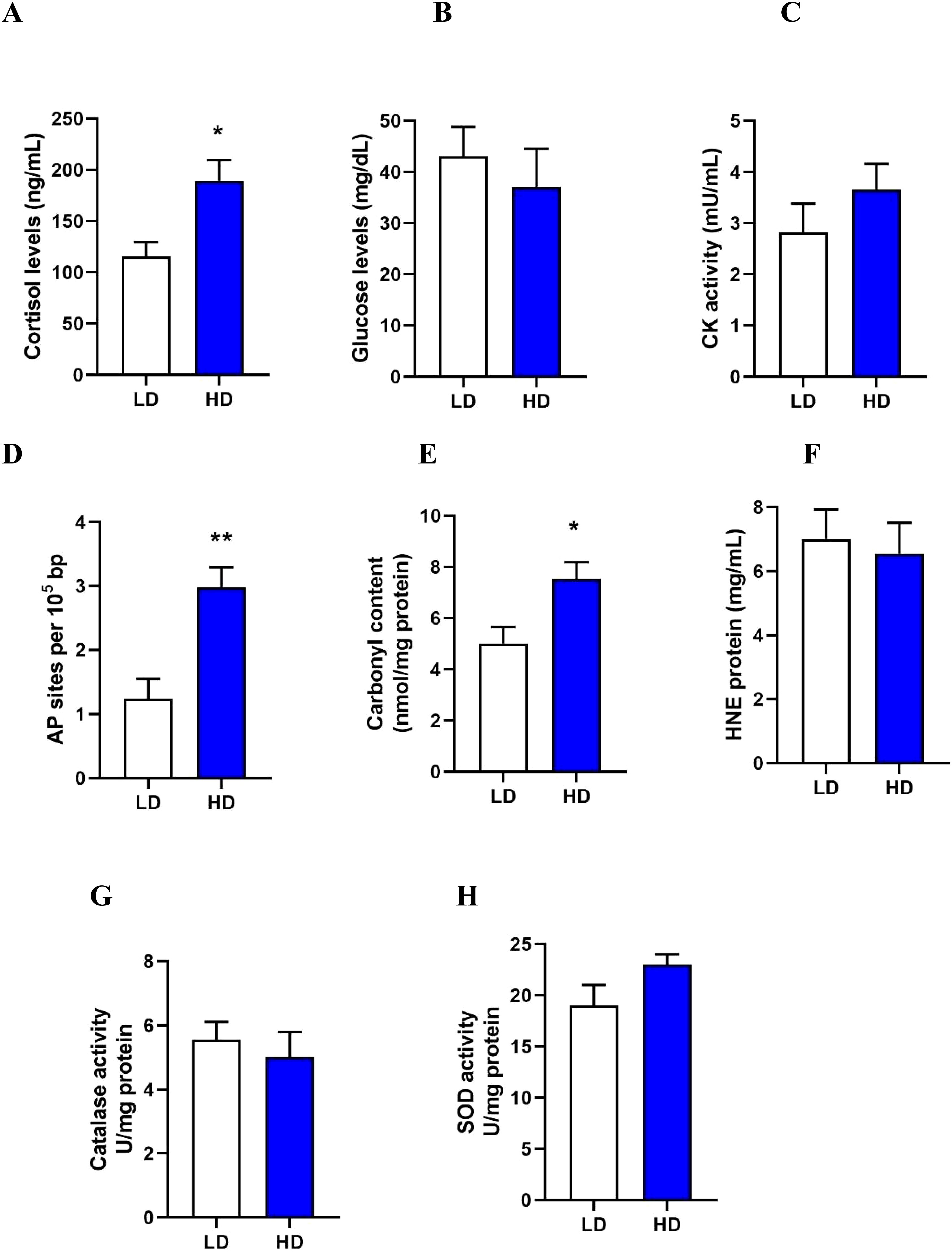
Figure 2. Assessment of stress response to crowding (A-C). Cortisol (A), glucose (B), and creatine kinase activity (C) in plasma. Evaluation of oxidative damage in the skeletal muscle to crowding (D-F). DNA oxidation as AP sites (D), protein carbonylation (E), and lipid peroxidation as HNE adducts (F). Evaluation of antioxidant activity in the skeletal muscle to crowding (G-H). Level of catalase activity (G), and superoxide dismutase (SOD) activity (H). All measurement were assessed in rainbow trout at 30 days under Low Density (LD) and High Density (HD) conditions. The results are expressed as a means + standard error (n= 4). Differences between LD and HD groups are shown in *p < 0.05, **p < 0.01.
To determine the impact of crowding on oxidative stress in the skeletal muscle of Rainbow trout, markers of oxidative damage, including DNA oxidation, protein carbonylation, and lipid peroxidation, were evaluated. Crowding stress caused oxidative stress in the skeletal muscle, evidenced by a significant increase in DNA oxidation (p-value = 0.0074) (Figure 2D) and protein carbonylation (p-value = 0.0318) (Figure 2E) in the HD group after 30 days of stress. No significant differences were observed in lipid peroxidation (p-value = 0.7452) (Figure 2F).
To assess the antioxidant response to crowding stress, the activities of catalase and superoxide dismutase (SOD) in skeletal muscle were measured. No significant differences in catalase activity (p-value = 0.5854) (Figure 2G) and SOD activity (p-value = 0.1238) (Figure 2H) were detected at 30 days of crowding stress.
3.2 Transcriptomic analysis of crowding stress
RNA sequencing was conducted using skeletal muscle samples collected 30 days after treatment from both the low density (LD) and high density (HD) groups. A total of 335,693,902 paired-end reads were generated from six cDNA libraries. The raw sequencing data have been deposited in the NCBI database under BioProject code PRJNA1189086. After filtering out adapter sequences and low-quality reads, 330,223,996 clean reads were retained for transcriptomic analysis (Supplementary Table S3). We identified 4,050 differentially expressed genes (DEGs) between the HD and LD groups, comprising 2,204 upregulated and 1,846 downregulated genes (Supplementary Table S4). GO and KEGG enrichment analyses were performed. For the upregulated DEGs, the most highly enriched Gene Ontology (GO) terms in biological processes (BP) included autophagy of mitochondria, autophagosome assembly, and protein ubiquitination (Figure 3). In the molecular function (MF) and cellular component (CC) categories, the most enriched GO terms were protein binding and autophagosome, respectively (Figure 3). KEGG pathway analysis revealed significant enrichment in autophagy, mitophagy, and lysosome pathways. Other interesting enriched KEGG pathways include Ubiquitin mediated proteolysis, mTOR signaling pathway, and FoxO signaling pathway (Table 1).
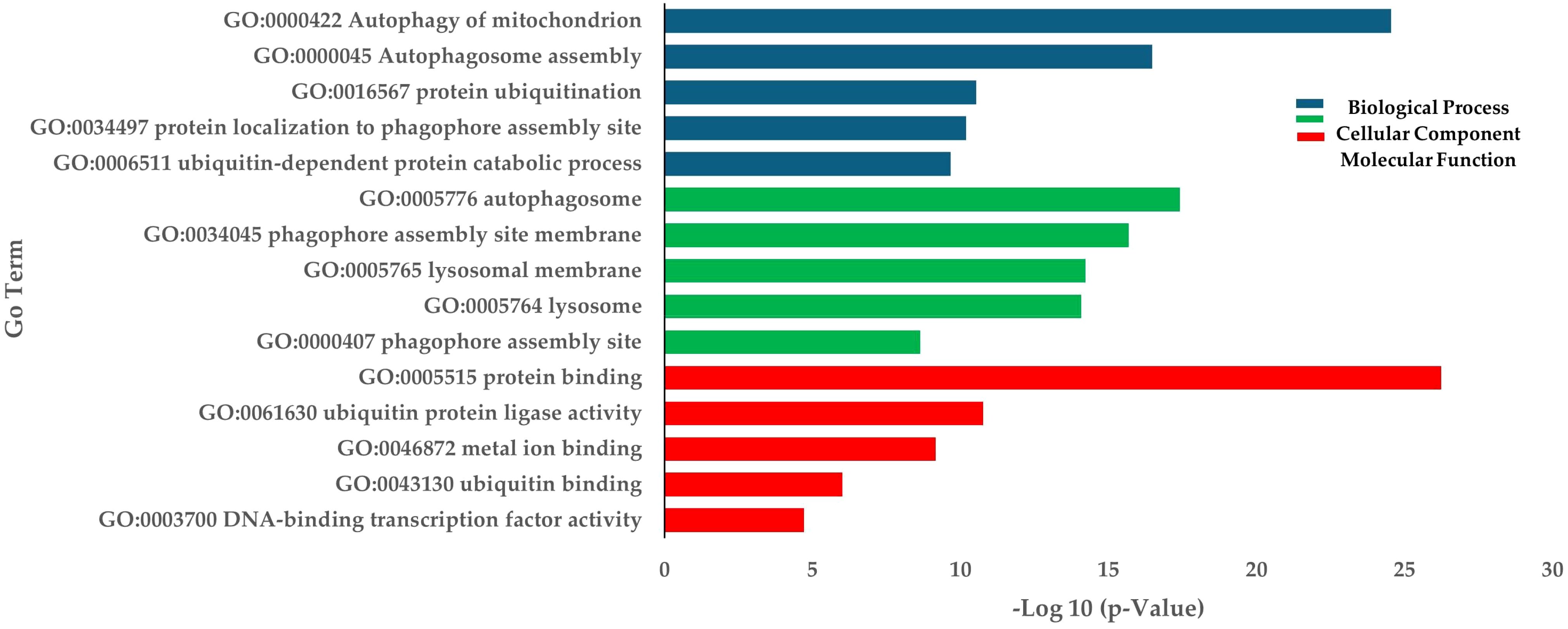
Figure 3. Enrichment of up-regulated DEGs in terms of BP (Biological process), CC (Cellular components), and MF (Molecular function) in HD group.
The downregulated DEGs were enriched in biological processes such as protein folding, protein phosphorylation, and mRNA splicing via the spliceosome (Figure 4). GO terms for down-regulated genes were associated with RNA binding (MF) and the nucleolus (CC) (Figure 4). KEGG pathway analysis indicated significant enrichment in spliceosome, nucleocytoplasmic transport, and mRNA surveillance pathways. Other interesting enriched KEGG pathways include ATP-dependent chromatin remodeling, Cysteine and methionine metabolism, and Biosynthesis of amino acids (Table 2).
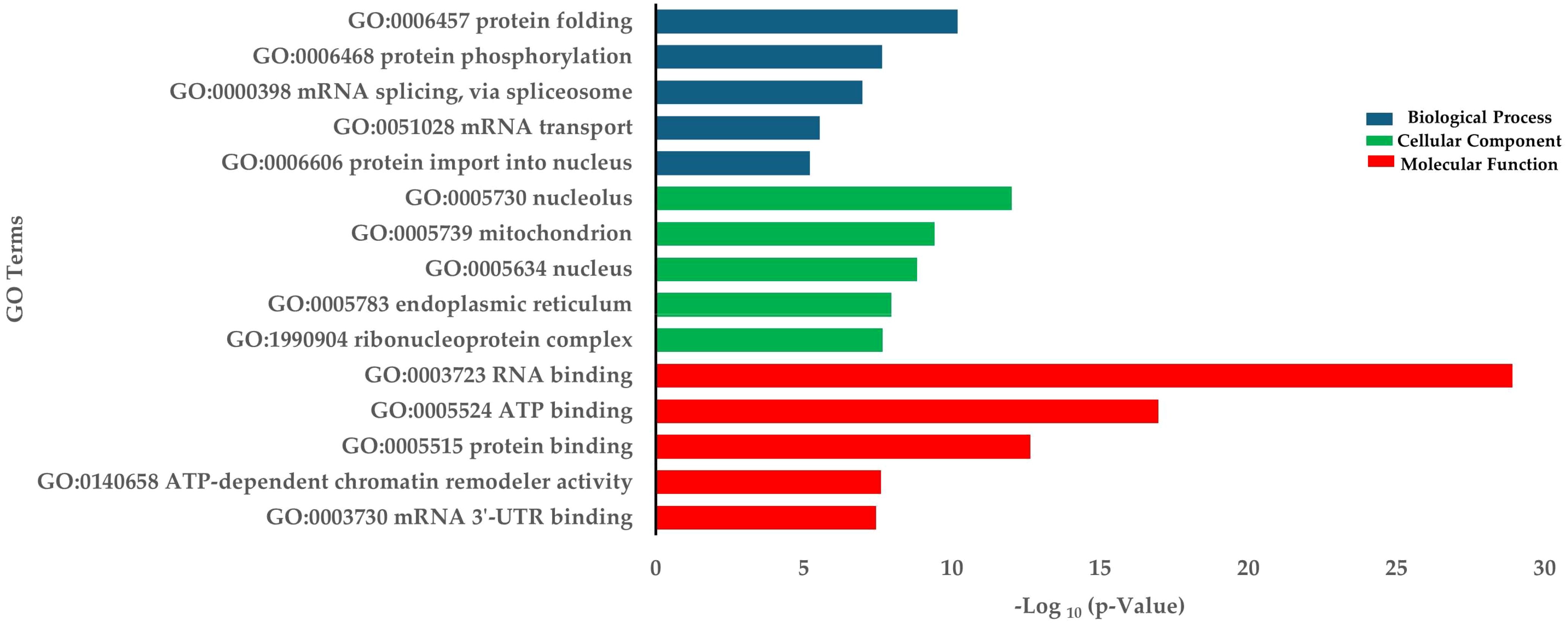
Figure 4. Enrichment of down-regulated DEGs in terms of BP (Biological process), CC (Cellular components), and MF (Molecular function) in HD group.
3.3 DNA methylation analysis of crowding stress
To evaluate the impact of crowding stress on DNA methylation in Rainbow trout, bisulfite-treated DNA and genomic libraries were prepared from the skeletal muscle samples collected from the low-density (LD) and high-density (HD) groups at 30 days. A total of 455,885,911 reads were generated across six whole-genome bisulfite sequencing (WGBS) libraries. After filtering out low-quality reads, 455,077,237 clean reads were retained for methylation analysis. The bisulfite conversion was on average 99%, ensuring high data reliability (Supplementary Table S5). The average methylation level of CG was 85.3%. The rest of the cytosine methylations were found in the context CHG and CHH with 7.6%, and 7.1%, respectively (Supplementary Table S6). Differential DNA methylation analysis was conducted in the context of CG methylation. The analysis identified 76,749 differentially methylated regions (DMRs) in the rainbow trout genome, 41,178 regions up-methylated (Supplementary Table S7), and 35,571 down-methylated (Supplementary Table S8). The distribution of DMRs in the 32 rainbow trout chromosomes can be visualized in Supplementary Table S9. On average, 38% of DMRs are located at intergenic regions, 6% in promoter regions, 18% in exonic regions, and 38% in intronic regions. The annotation of these DMRs revealed 6,039 hyper-methylated genes and 5,633 hypomethylated genes. In total, 11,672 differentially methylated genes (DMGs) were identified. Enrichment analysis of DMGs highlighted biological processes (BPs) such as protein phosphorylation, multicellular organism development, and regulation of GTPase activity (Figure 5). Gene Ontology (GO) terms associated with DMGs were primarily linked to protein binding (MF) and the Cytoplasm (CC) (Figure 5). KEGG pathway analysis revealed significant enrichment in pathways such as glycerolipid metabolism, regulation of the actin cytoskeleton, and adherens junctions. Other interesting enriched KEGG pathways include Wnt signaling pathway, Focal adhesion, and Cytoskeleton in muscle cells (Table 3).
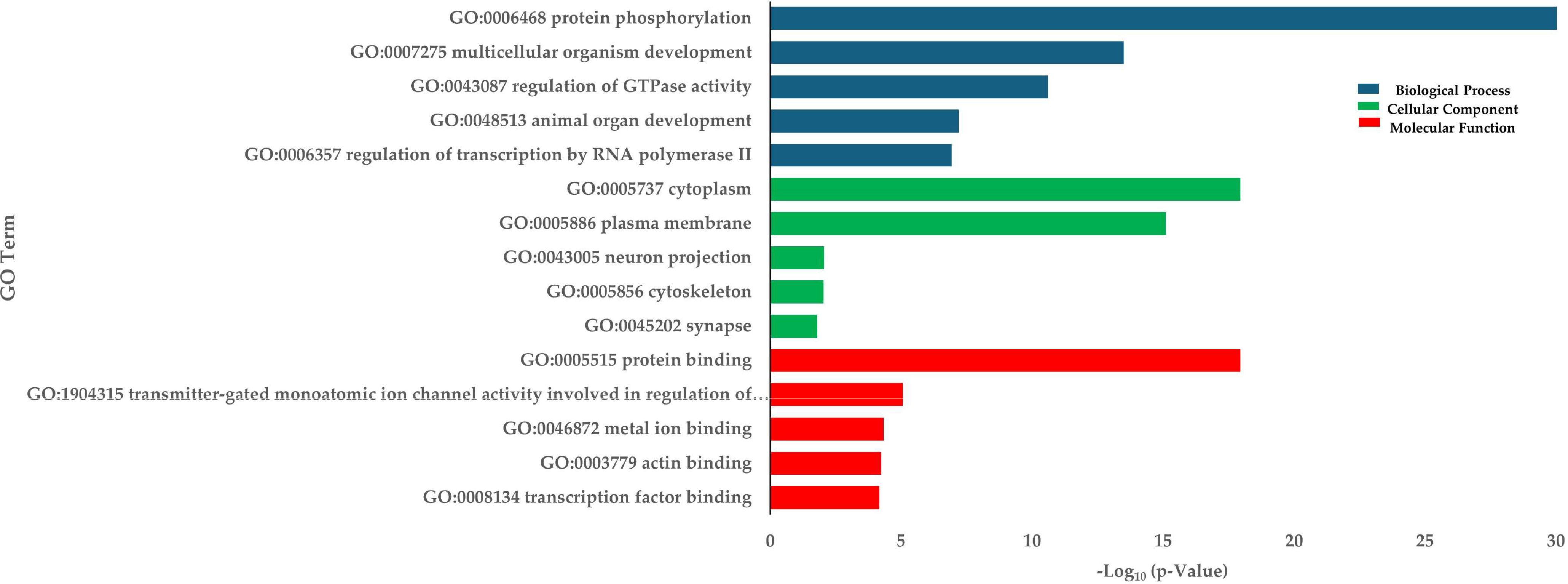
Figure 5. Enrichment of DMGs in terms of BP (Biological process), CC (Cellular components), and MF (Molecular function) in HD group.
3.4 Integrative analysis of transcriptomic and epigenomic response of crowding
Integrative findings of RNA-seq and WGBS showed an overlap of 263 genes between 2,204 up-regulated expressed genes and 5,633 down-methylated genes. GO enrichment analysis of these genes, revealed that protein phosphorylation, regulation of transcription by RNA polymerase II, and endosome to lysosome transport were overrepresented in biological process (Supplementary Table S10). In cellular component and molecular function, cytoskeleton and protein binding were significantly enriched, respectively (Supplementary Table S10). The KEGG pathways analysis revealed that Autophagy, Mitophagy, Insulin signaling pathway were highly represented (Table 4). In the other hand, an overlap of 299 genes between 1,846 down-regulated expressed genes and 6,039 up-methylated genes were identified. GO enrichment analysis of these genes revealed that regulation of translation, auditory receptor cell development, and mRNA transport were highly represented (Supplementary Table S11). In cellular component and molecular function, cytoplasm and mRNA 3’-UTR binding were significantly enriched, respectively (Supplementary Table S11). The KEGG pathways analysis revealed that ATP-dependent chromatin remodeling, D-Amino acid metabolism, and Nucleocytoplasmic transport were overrepresented (Table 4).
For validation, we selected Bcl-2 interacting protein 3 (bnip3) and Autophagy Related 9B (atg9b) genes involved in autophagy and mitophagy. RAF proto-oncogene serine/threonine-protein kinase (raf1a) and Phosphodiesterase 3B (pde3b) genes, involved in insulin signaling pathway. Brahma-related gene 1 (brg1) and Metastasis-associated protein 2 (mta2) genes, involved in ATP-dependent chromatin remodeling. Our findings demonstrate a strong correlation (r = 0.9156) between gene expression levels obtained through RNA-seq and validated using qPCR. (Figure 6).
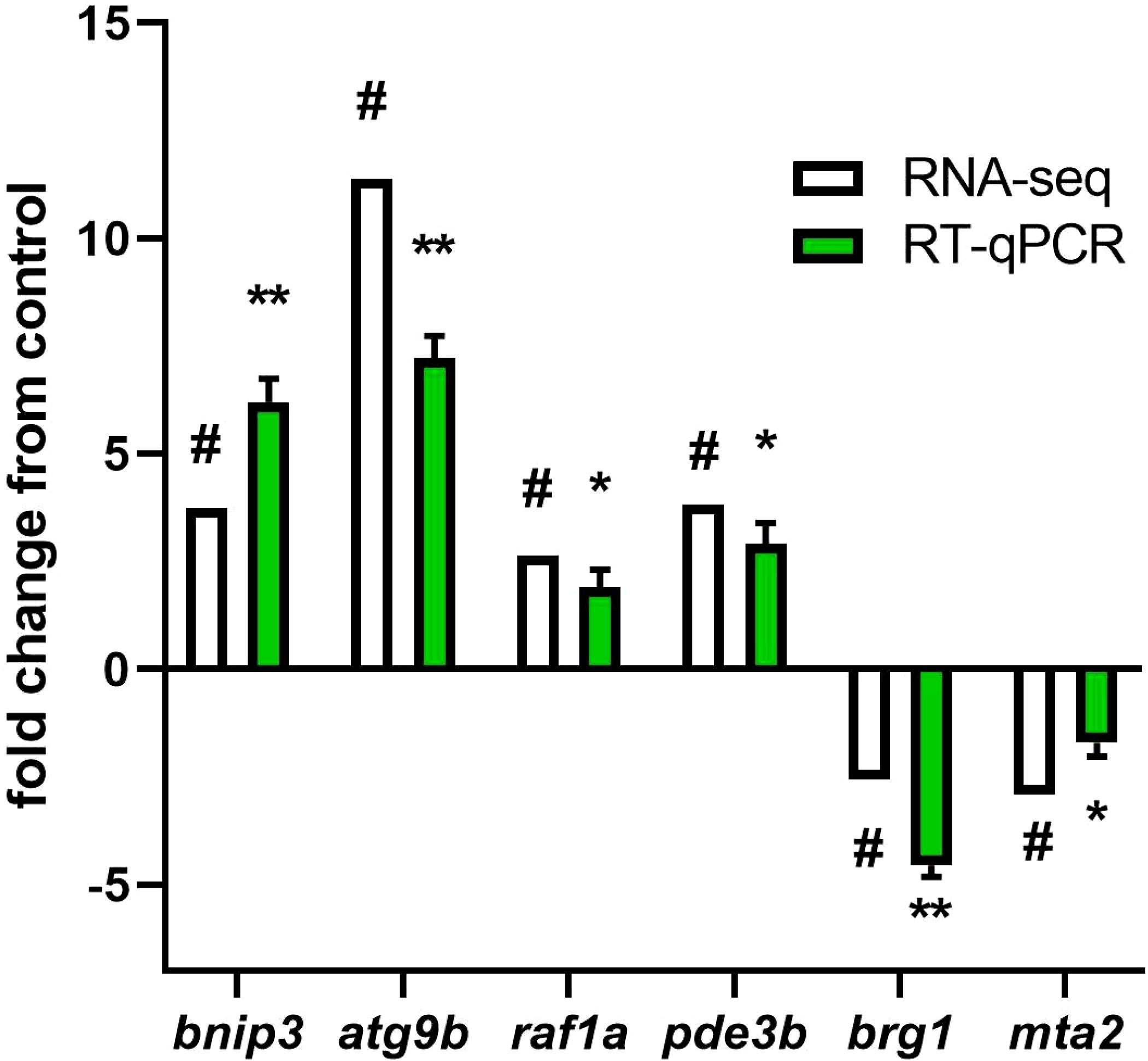
Figure 6. RT-qPCR validation of DEGs and DMGs of representative pathways. The genes selected for the RT-PCR validation were bnip3, atg9b, raf1a, pde3b, brg1, and mat2. For RNA-seq, in white, “#” indicates a log2 fold change ≥2.0 and FDR <0.05. For RT-qPCR, in green, relative expression was normalized against fau and actβ. The results are expressed as means ± standard errors (n=4). Differences are shown in *p < 0.05, and **p < 0.01.
4 Discussion
Crowding stress significantly impacts on the welfare and growth performance of farmed fish. Fish, like other vertebrates, can experience psychological and ethological suffering under chronic stress, particularly in intensive aquaculture settings. Chronic stress, typically caused by prolonged exposure to environmental stressors, affects both their mental state and natural behaviors (12). Excessive stocking density may lead to suppressed growth, increased susceptibility to diseases, and higher mortality rates (37). In addition, crowding stress can enhance cannibalism in the aquaculture of some fish species due to resource competition, stress-induced aggression, and restricted movement (38). Our study aimed to assess the growth, physiological, and molecular impacts of stocking densities on rainbow trout, revealing those higher densities significantly impaired growth rates, physiological and oxidative status, as well as transcriptomic and epigenetic responses in skeletal muscle. Our results indicate that crowding stress causes a significant decrease in weight and condition factor at 30 days, accompanied by increased plasma levels of cortisol and creatine kinase. Numerous studies have shown that growth is negatively affected in various fish species when density levels exceed optimal thresholds, leading to significant declines in performance and overall health. According to FAO, intensive commercial farms for rainbow trout typically operate at maximum stocking density of 40–50 kg/m3 (5). For instance, in juvenile Rainbow trout, body weight and plasma cortisol levels were significantly altered at a stocking density of 30 kg/m³ after 45 days compared to fish reared at 10 kg/m³ (25). Similarly, a study conducted on the same species found that cortisol levels were significantly higher in fish reared at 40 kg/m³ and 80 kg/m³ compared to those kept at 10 kg/m³ for 30 days, although growth parameters were not quantified (39). In Atlantic salmon (S. salar), farming at 53 kg/m³ for 66 days led to decreased growth and increased plasma cortisol levels compared to 18 kg/m³ densities (40). Similar reports exist for non-salmonid species. In Fine flounder (P. adspersus), weight and condition factor were significantly reduced after seven weeks of crowding at 17 kg/m³ compared to fish reared at 7 kg/m³, with significant differences in cortisol levels observed only at week four (26). In Nile tilapia (Oreochromis niloticus), significant changes in weight and cortisol levels have also been reported with high-density farming (41). In summary, all these studies highlight a close relationship between crowding stress and elevated cortisol levels in plasma.
Increasing evidence suggests that elevated stocking densities can disrupt intracellular redox balance, leading to oxidative stress and cellular damage in various fish species (42). In our study, we determined that crowding stress induced oxidative damage in DNA and proteins in the skeletal muscle, without altering the antioxidant activity of catalase and superoxide dismutase (SOD). These observations are consistent with similar studies. For example, a recent study on Largemouth black bass (Micropterus salmoides) found that high farming densities (120 g/m³) induced oxidative stress and li-pid peroxidation in plasma and liver tissues after 90 days (43). Increasing Rainbow trout density to 25 kg/m³ for 70 days induced oxidative stress and up-regulated cytokine gene expression, although no variations were observed in catalase and SOD activity in the intestine, similar to our findings (44). Other studies suggest that crowding may increase antioxidant activity, indicating a possible response by the antioxidant system to stress (45). Oxidative damage associated with stress is not limited to crowding but also involves other stressors. For example, thermal stress has been shown to cause oxidation of proteins, nucleic acids, and lipids in the marine teleost red cusk-eel (Genypterus chilensis) (46). Interestingly, our research group recently described the effects of temperature stress in Rainbow trout, showing an increase in autophagy in skeletal muscle, but without linking it to oxidative damage (47). The relationship between stress and oxidative damage in fish skeletal muscle may be primarily regulated by cortisol and its non-genomic pathway. In Rainbow trout myotubes, cortisol has been shown to induce a rapid increase in plasma levels of reactive oxygen species (ROS), triggering a series of events associated with the ERK1/2 MAP kinase pathway (48).
Cortisol plays a central function in modulating both genomic and epigenomic responses to stress (49). It exerts its effects through genomic mechanisms by binding to glucocorticoid receptors, which act as transcription factors that directly regulate the expression of genes involved in stress responses, metabolism, and growth (50). Recent research has also shown that cortisol induces epigenomic changes, such as DNA methylation, which can influence long-term gene expression. These epigenetic changes may create a “stress memory” within the organism, meaning that fish exposed to chronic stress may have altered responses to subsequent stressors, potentially affecting growth, immune responses, and behavior (34). In the present study, we found that after 30 days of crowding stress, the expression of four thousand genes was altered, primarily associated with up-regulated pathways like autophagy, mitophagy, and the ubiquitin-proteasome system, and down-regulated pathways such as the spliceosome, nucleocytoplasmic transport, and mRNA surveillance. These results align with data published by our group, which described how crowding stress induces skeletal muscle protein degradation through upregulation of the UPS and autophagy pathways, while downregulating IGF-1 components during the stress response (25, 26). The involvement of cortisol in the regulation of gene expression related to protein catabolism in skeletal muscle has been demonstrated through various approaches (50). However, there is no evidence showing that changes in the expression of autophagy genes can be modulated by epigenomic remodeling. To explore this further, we examined the dynamics of DNA methylation in response to crowding stress using whole-genome bisulfite sequencing (WGBS). Methylation analysis revealed 76,748 differentially methylated regions (DMRs) in the Rainbow trout genome, with CG methylation being far more abundant than CHG and CHH methylation, which is consistent with other reports (51, 52). GC methylation was evenly distributed across all chromosomes, predominantly located in gene bodies and promoter regions near the transcription start site, similar to previous studies (53, 54). To assess the influence of DNA methylation on biological processes associated with crowding stress, we conducted a negative correlation analysis between differentially expressed genes (DEGs) and genes with altered methylation levels in their promoter regions or gene bodies (DMGs). We identified 263 genes showing a negative correlation between up-regulated expression and down-methylation, primarily related to autophagy, mitophagy, and insulin signaling pathways. Additionally, 299 genes showed a negative correlation between down-regulated expression and up-methylation, mainly linked to ATP-dependent chromatin remodeling, D-amino acid metabolism, and Nucleocytoplasmic transport. Few studies have explored the effects of stress on skeletal muscle in fish, particularly examining how methylation patterns and gene expression are differentially influenced. To our knowledge, no studies have analyzed the effects of crowding stress on genome methylation and epigenetic remodeling in teleosts. However, there are some interesting reports on multi-stressor and acute stress. A study on early life stressors, like cold shock and air exposure, showed that DNA methylation patterns and gene expression were altered in Atlantic salmon (S. salar), highlighting the role of environmental factors in shaping the methylome (55). Similarly, whole-genome bisulfite sequencing has been used to investigate DNA methylation changes in Atlantic salmon under acute (cold-shock during embryogenesis) and chronic stress (lack of tank enrichment during larval stages), revealing contrasting effects on DNA methylation linked to immune responses and gene expression (56). The same technical approach (WGBS) has been used to demonstrate differential methylation of sperm in Atlantic salmon (S. salar) in response to captivity, revealing how environmental factors influence gamete DNA methylation and contribute to transgenerational plasticity in this species (57). Our research group recently explored the effects of environmental factors on epigenomic and transcriptomic responses in Atlantic salmon during infection by sea lice (Caligus rogercresseyi) (58) and the effects of cortisol-mediated stress on transcriptomic and epigenomic responses in rainbow trout skeletal muscle (34). Both studies demonstrate a strong relationship between DNA methylation, gene expression, and the type of stress.
To validate our findings, we selected six genes that exhibited a negative correlation between methylation levels and gene expression, related to autophagy, the insulin pathway, and ATP-dependent chromatin remodeling. BCL2 interacting protein 3 (bnip3) is a gene involved in autophagy, mitophagy, and cell death (59). Overexpression of bnip3 under high-density farming has been reported in Fine flounder (P. adspersus) (26) and Red claw crayfish (Cherax quadricarinatus) (60). Although no reports indicate changes in the methylation of this gene due to crowding stress, promoter region methylation of bnip3 has been described under hypoxic conditions in Rainbow trout (61). Atg9b, a crucial autophagy-related gene, plays a pivotal role in initiating autophagy by facilitating the formation and trafficking of autophagosomes. Unlike other autophagy-related genes, ATG9B is a transmembrane protein, making it essential for membrane delivery to expanding autophagosomes (62). Interestingly, its expression has been shown to regulate autophagy during immune responses in fish muscle cells challenged with the intracellular pathogen P. salmonis (63). Although there are no reports of methylation of this gene in aquatic organisms, its epigenetic regulation is essential for controlling autophagy in breast carcinoma, increasing the degradation of cellular proteins, similar to our observations (64). Raf1, also known as RAF proto-oncogene ser-ine/threonine-protein kinase gene, is a kinase that primarily functions as part of the MAPK/ERK signaling pathway, playing an essential role in regulating cell growth, differentiation, and survival. In the context of autophagy, raf1 has a dual role, as it can both promote and inhibit autophagic processes depending on cellular conditions and stimuli (65). Its expression has been upregulated as a mediator of temperature stress-induced autophagy in rainbow trout (47). Phosphodiesterase 3B (pde3b) is an enzyme that breaks down cyclic AMP (cAMP), a molecule that plays a key role in cell signaling, including autophagy regulation. In the context of autophagy, PDE3B’s activity indirectly influ-ences autophagic processes through its modulation of the cAMP/protein kinase A (PKA) pathway (66). To our knowledge, there are no previous reports about methylation of these genes in teleost under stress. Finally, we validated the downregulation of brg1 and mta2 involved in ATP-dependent chromatin remodeling. The Metastasis-Associated (mta) gene family, particularly mta2, is involved in chromatin remodeling and has a substantial role in regulating gene expression. The proteins encoded by mta genes are core components of the nucleosome remodeling and histone deacetylation (NuRD) complex, a multi-protein complex that modifies chromatin structure by both remodeling nucleosomes and deacetylating histones (67). Brg1 (Brahma-related gene 1) is a critical ATPase and a central component of the SWI/SNF chromatin remodeling complex, which plays a crucial role in regulating chromatin structure and gene expression. This protein facilitates the ATP-dependent alteration of nucleosome positioning, making DNA more or less accessible for transcription and other nuclear processes (68). There are no studies that analyze its expression under stress conditions in fish, and even less its epigenetic regulation. Cortisol’s role in gene methylation is not fully understood, but studies in mammals show that glucocorticoids can alter DNA methylation through modulation in the expression of methyltransferases. DNA methylation occurs when DNA methyltransferases (DNMTs) add methyl groups to cytosine, creating 5-methylcytosine (5mC), which suppresses gene expression. In contrast, ten-eleven translocation (TET) proteins convert 5mC to 5-hydroxymethylcytosine (5hmC), promoting gene expression by leading to unmethylated cytosine (69, 70). Our future studies will investigate how cortisol and stress regulate differential gene methylation and expression, increasing the number of biological and technical replicates per treatment while addressing the failure to measure incidental variables that are essential for a broader understanding of our results.
5 Conclusion
This study highlights the significant impact of crowding stress on the growth and skeletal muscle development of juvenile rainbow trout in aquaculture. Crowding, a common chronic stressor, triggers the release of cortisol, redirecting energy from growth to stress response mechanisms, which ultimately impairs growth and muscle quality. The molecular analysis presented here reveals extensive physiological, oxidative, and epigenetic changes induced by crowding, including alterations in gene expression and DNA methylation. Notably, genes related to autophagy, mitophagy, and insulin signaling were found to be affected by crowding stress, while chromatin remodeling pathways were also implicated in the stress response. These findings provide novel insights into the molecular mechanisms underlying stress-induced growth impairments and emphasize the importance of optimizing farming conditions to improve fish welfare. By integrating transcriptomic and epigenetic data, this research paves the way for more sustainable aquaculture practices that can mitigate the detrimental effects of chronic stress on farmed fish. Future research should focus on investigating the mechanisms through which cortisol modulates differential gene methylation, increasing the number of biological and technical replicates per treatment.
Data availability statement
The data presented in the study are deposited in the NCBI database repository, accession number BioProject code PRJNA1189086.
Ethics statement
This study followed bioethical protocol authorized by the ethical committee of the Universidad Andres Bello (protocol code 010/2023). The study was conducted in accordance with the local legislation and institutional requirements.
Author contributions
DA-C: Conceptualization, Formal Analysis, Investigation, Validation, Writing – original draft. VV-M: Funding acquisition, Software, Supervision, Validation, Writing – review & editing. CG-E: Formal Analysis, Funding acquisition, Methodology, Visualization, Writing – review & editing. AM: Data curation, Project administration, Resources, Writing – original draft. JV: Conceptualization, Data curation, Formal Analysis, Funding acquisition, Investigation, Methodology, Project administration, Resources, Software, Supervision, Validation, Visualization, Writing – original draft, Writing – review & editing.
Funding
The author(s) declare that financial support was received for the research and/or publication of this article. This research was funded by the Fondo Nacional de Desarrollo Científico y Tecnológico (FONDECYT) under grant numbers 1230794 (to Juan Antonio Valdés), 11220307 (to Valentina Valenzuela-Muñoz), and 1210852 (to Cristian Gallardo-Escarate); Fondo de Financiamiento de Centros de Investigación en Áreas Prioritarias (FONDAP) grants INCAR FONDAP 1522A0004 (2023) and 1523A0007 (2024). Daniela Aravena-Canales acknowledges support received from ANID (Ph.D. scholarship 21220218).
Conflict of interest
The authors declare that the research was conducted in the absence of any commercial or financial relationships that could be construed as a potential conflict of interest.
The author(s) declared that they were an editorial board member of Frontiers, at the time of submission. This had no impact on the peer review process and the final decision.
Generative AI statement
The author(s) declare that Generative AI was used in the creation of this manuscript. ChatGPT (https://chat.openai.com/) was used to edit English orthography, grammar, and redaction in the manuscripts.
Publisher’s note
All claims expressed in this article are solely those of the authors and do not necessarily represent those of their affiliated organizations, or those of the publisher, the editors and the reviewers. Any product that may be evaluated in this article, or claim that may be made by its manufacturer, is not guaranteed or endorsed by the publisher.
Supplementary material
The Supplementary Material for this article can be found online at: https://www.frontiersin.org/articles/10.3389/fendo.2025.1571111/full#supplementary-material
References
1. FAO. The State of World Fisheries and Aquaculture 2024 – Blue Transformation in action. Rome: Food and Agriculture Organization of the United Nations (2024). doi: 10.4060/cd0683en
2. Albrektsen S, Kortet R, Skov PV, Ytteborg E, Gitlesen S, Kleinegris D, et al. Future feed resources in sustainable salmonid production: A review. Rev Aquacult. (2022) 14:1790–812. doi: 10.1111/raq.12673
3. D’Agaro E, Gibertoni P, Esposito S. Recent trends and economic aspects in the rainbow trout (Oncorhynchus mykiss) sector. Appl Sci. (2022) 12:8773. doi: 10.3390/app12178773
4. Wind T, Schumann M, Hofer S, Schulz C, Brinker A. Life cycle assessment of rainbow trout farming in the temperate climate zone based on the typical farm concept. J Cleaner Prod. (2022) 380:134851. doi: 10.1016/j.jclepro.2022.134851
5. Woynarovich A, Hoitsy G, Moth-Poulsen T. Small-scale rainbow trout farming. Rome: FAO. Fisheries and Aquaculture Technical Paper No. (2011). 561. 81 pp. Available at: https://www.fao.org/docrep/015/i2125e/i2125e.pdf
7. Balasch JC, Tort L. Netting the stress responses in fish. Front Endocrinol. (2019) 10:62. doi: 10.3389/fendo.2019.00062
8. Ciji A, Akhtar MS. Stress management in aquaculture: a review of dietary interventions. Rev Aquacult. (2021) 13:2190–247. doi: 10.1111/raq.12565
9. Aluru N, Vijayan MM. Stress transcriptomics in fish: A role for genomic cortisol signaling. Gen Comp Endocrinol. (2009) 164:142–50. doi: 10.1016/j.ygcen.2009.03.020
10. Virtanen MI, Brinchmann MF, Patel DM, Iversen MH. Chronic stress negatively impacts wound healing, welfare, and stress regulation in internally tagged Atlantic salmon (Salmo salar). Front Physiol. (2023) 14:1147235. doi: 10.3389/fphys.2023.1147235
11. Zhang Z, Fu Y, Zhao H, Zhang X. Social enrichment affects fish growth and aggression depending on fish species: Applications for aquaculture. Front Mar Sci. (2022) 9:1011780. doi: 10.3389/fmars.2022.1011780
12. Toni M, Manciocco A, Angiulli E, Alleva E, Cioni C, Malavasi S. Review: Assessing fish welfare in research and aquaculture, with a focus on European directives. Animal. (2019) 13:161–70. doi: 10.1017/S1751731118000940
13. Pfalzgraff T, Lund I, Skov PV. Prolonged cortisol elevation alters whole body and tissue metabolism in rainbow trout (Oncorhynchus mykiss). Comp Biochem Physiol Part A: Mol Integr Physiol. (2022) 263:111098. doi: 10.1016/j.cbpa.2021.111098
14. Jerez-Cepa I, Gorissen M, Mancera JM, Ruiz-Jarabo I. What can we learn from glucocorticoid administration in fish? Effects of cortisol and dexamethasone on intermediary metabolism of gilthead seabream (Sparus aurata L.). Comp Biochem Physiol Part A: Mol Integr Physiol. (2019) 231:1–10. doi: 10.1016/j.cbpa.2019.01.010
15. Amador MHB. Multiple pathways flounder growth in frazzled fish. J Exp Biol. (2018) 221:jeb169839. doi: 10.1242/jeb.169839
16. Sadoul B, Vijayan MM. Stress and growth. In: Fish Physiology. Elsevier (2016). p. 167–205. doi: 10.1016/B978-0-12-802728-8.00005-9
17. Mwaura JG, Wekesa C, Ogutu PA, Okoth P. Whole transcriptome analysis of differentially expressed genes in cultured nile tilapia (O. niloticus) subjected to chronic stress reveals signaling pathways associated with depressed growth. Genes. (2023) 14:795. doi: 10.3390/genes14040795
18. Xu C, Su L, Qiu N, Hou M, Yu F, Zou X, et al. The effect of unpredictable chronic stress on rare minnow (Gobiocypris rarus): growth, behaviour and physiology. Biology. (2022) 11:1755. doi: 10.3390/biology11121755
19. Gallas-Lopes M, Bastos LM, Benvenutti R, Panzenhagen AC, Piato A, Herrmann AP. Ten years of unpredictable chronic stress in zebrafish: a systematic review and meta-analysis. bioRxiv (2022) 2022–12. doi: 10.1101/2022.12.12.520151
20. Martínez-Álvarez RM, Morales AE, Sanz A. Antioxidant defenses in fish: biotic and abiotic factors. Rev Fish Biol Fisheries. (2005) 15:75–88. doi: 10.1007/s11160-005-7846-4
21. Oliveira J, Oliva-Teles A, Couto A. Tracking biomarkers for the health and welfare of aquaculture fish. Fishes. (2024) 9:289. doi: 10.3390/fishes9070289
22. Chowdhury S, Saikia SK. Oxidative stress in fish: A review. J Sci Res. (2020) 12:145–60. doi: 10.3329/jsr.v12i1.41716
23. Liu Y, Tian C, Yang Z, Huang C, Jiao K, Yang L, et al. Effects of chronic heat stress on growth, apoptosis, antioxidant enzymes, transcriptomic profiles, and immune-related genes of Hong Kong catfish (Clarias fuscus). Animals. (2024) 14:1006. doi: 10.3390/ani14071006
24. Swain HS, Das BK, Upadhyay A, Ramteke MH, Kumar V, Meena DK, et al. Stocking density mediated stress modulates growth attributes in cage reared Labeo rohita (Hamilton) using multifarious biomarker approach. Sci Rep. (2022) 12:9869. doi: 10.1038/s41598-022-13570-x
25. Valenzuela CA, Ponce C, Zuloaga R, González P, Avendaño-Herrera R, Valdés JA, et al. Effects of crowding on the three main proteolytic mechanisms of skeletal muscle in rainbow trout (Oncorhynchus mykiss). BMC Vet Res. (2020) 16:294. doi: 10.1186/s12917-020-02518-w
26. Valenzuela CA, Zuloaga R, Mercado L, Einarsdottir IE, Björnsson BT, Valdés JA, et al. Chronic stress inhibits growth and induces proteolytic mechanisms through two different nonoverlapping pathways in the skeletal muscle of a teleost fish. Am J Physiology-Regulat Integr Comp Physiol. (2018) 314:R102–13. doi: 10.1152/ajpregu.00009.2017
27. Guinand B, Samaras A. Epigenetics of stress in farmed fish: an appraisal. In: Piferrer F, Wang H, editors. Epigenetics in Aquaculture. Wiley (2023). p. 263–85. doi: 10.1002/9781119821946.ch12
28. Best C, Mennigen JA, Gilmour KM. Exploring transcriptional and post-transcriptional epigenetic regulation of crf and 11βhsd2 in rainbow trout brain during chronic social stress. Comp Biochem Physiol Part A: Mol Integr Physiol. (2024) 288:111557. doi: 10.1016/j.cbpa.2023.111557
29. Abdelnour SA, Naiel MAE, Said MB, Alnajeebi AM, Nasr FA, Al-Doaiss AA, et al. Environmental epigenetics: Exploring phenotypic plasticity and transgenerational adaptation in fish. Environ Res. (2024) 252:118799. doi: 10.1016/j.envres.2024.118799
30. Valdivieso A, Caballero-Huertas M, Moraleda-Prados J, Piferrer F, Ribas L. Exploring the effects of rearing densities on epigenetic modifications in the zebrafish gonads. IJMS. (2023) 24:16002. doi: 10.3390/ijms242116002
31. Fernando GCP, Khansari AR, Tort L. Response to chronic crowding stress in shy and bold behavioral groups of male and female zebrafish. Am J Physiology-Regulat Integr Comp Physiol. (2024) 327:R275–90. doi: 10.1152/ajpregu.00041.2024
32. Tkachenko H, Kurhaluk N, Grudniewska J, Andriichuk A. Tissue-specific responses of oxidative stress biomarkers and antioxidant defenses in rainbow trout Oncorhynchus mykiss during a vaccination against furunculosis. Fish Physiol Biochem. (2014) 40:1289–1300. doi: 10.1007/s10695-014-9924-9
33. Aedo JE, Zuloaga R, Bastias-Molina M, Meneses C, Boltaña S, Molina A, et al. Early transcriptomic responses associated with the membrane-initiated action of cortisol in the skeletal muscle of rainbow trout (Oncorhynchus mykiss). Physiol Genomics. (2019) 51(11), 596–606. doi: 10.1152/physiolgenomics.00042.2019
34. Aravena-Canales D, Valenzuela-Muñoz V, Gallardo-Escarate C, Molina A, Valdés JA. Transcriptomic and epigenomic responses to cortisol-mediated stress in rainbow trout (Oncorhynchus mykiss) skeletal muscle. IJMS. (2024) 25:7586. doi: 10.3390/ijms25147586
35. Fuentes EN, Safian D, Valdés JA, Molina A. Isolation and selection of suitable reference genes for real-time PCR analyses in the skeletal muscle of the fine flounder in response to nutritional status: assessment and normalization of gene expression of growth-related genes. Fish Physiol Biochem. (2013) 39:765–77. doi: 10.1007/s10695-012-9739-5
36. Untergasser A, Cutcutache I, Koressaar T, Ye J, Faircloth BC, Remm M, et al. Primer3—new capabilities and interfaces. Nucleic Acids Res. (2012) 40:e115–5. doi: 10.1093/nar/gks596
37. Santurtun E, Broom D, Phillips C. A review of factors affecting the welfare of Atlantic salmon (Salmo salar). Anim welf. (2018) 27:193–204. doi: 10.7120/09627286.27.3.193
38. Pereira LS, Agostinho AA, Winemiller KO. Revisiting cannibalism in fishes. Rev Fish Biol Fisheries. (2017) 27:499–513. doi: 10.1007/s11160-017-9469-y
39. Yarahmadi P, Miandare HK, Fayaz S, Caipang CMA. Increased stocking density causes changes in expression of selected stress- and immune-related genes, humoral innate immune parameters and stress responses of rainbow trout (Oncorhynchus mykiss). Fish Shellfish Immunol. (2016) 48:43–53. doi: 10.1016/j.fsi.2015.11.007
40. Liu B, Liu Y, Sun G. Effects of stocking density on growth performance and welfare-related physiological parameters of Atlantic salmon S almo salar L. @ in recirculating aquaculture system. Aquac Res. (2017) 48:2133–44. doi: 10.1111/are.13050
41. Zaki MAA, Alabssawy AN, Nour AE-AM, El Basuini MF, Dawood MAO, Alkahtani S, et al. The impact of stocking density and dietary carbon sources on the growth, oxidative status and stress markers of Nile tilapia (Oreochromis niloticus) reared under biofloc conditions. Aquacult Rep. (2020) 16:100282. doi: 10.1016/j.aqrep.2020.100282
42. Zhang T, Zhang L, Yin T, You J, Liu R, Huang Q, et al. Recent understanding of stress response on muscle quality of fish: From the perspective of industrial chain. Trends Food Sci Technol. (2023) 140:104145. doi: 10.1016/j.tifs.2023.104145
43. Jia R, Wang L, Hou Y, Feng W, Li B, Zhu J. Effects of stocking density on the growth performance, physiological parameters, redox status and lipid metabolism of micropterus salmoides in integrated rice–fish farming systems. Antioxidants. (2022) 11:1215. doi: 10.3390/antiox11071215
44. Hoseini SM, Yousefi M, Taheri Mirghaed A, Paray BA, Hoseinifar SH, Van Doan H. Effects of rearing density and dietary tryptophan supplementation on intestinal immune and antioxidant responses in rainbow trout (Oncorhynchus mykiss). Aquaculture. (2020) 528:735537. doi: 10.1016/j.aquaculture.2020.735537
45. Wei D. Growth performance, hematological and biochemical responses of African catfish (Clarias gariepinus) reared at different stocking densities. Afr J Agric Res. (2011) 6:233–266. doi: 10.5897/AJAR11.1278
46. Dettleff P, Toloza C, Fuentes M, Aedo J, Zuloaga R, Estrada JM, et al. Gills de novo assembly reveals oxidative stress, unfolded protein, and immune response on red cusk-eel (Genypterus Chilensis) under thermal stress. Mar Environ Res. (2024) 196:106440. doi: 10.1016/j.marenvres.2024.106440
47. Molina A, Dettleff P, Valenzuela-Muñoz V, Gallardo-Escarate C, Valdés JA. High-temperature stress induces autophagy in rainbow trout skeletal muscle. Fishes. (2023) 8:303. doi: 10.3390/fishes8060303
48. Espinoza MB, Aedo JE, Zuloaga R, Valenzuela C, Molina A, Valdés JA. Cortisol induces reactive oxygen species through a membrane glucocorticoid receptor in rainbow trout myotubes. J Cell Biochem. (2017) 118:718–25. doi: 10.1002/jcb.25676
49. Metzger DCH, Schulte PM. Epigenomics in marine fishes. Mar Genomics. (2016) 30:43–54. doi: 10.1016/j.margen.2016.01.004
50. Aedo JE, Zuloaga R, Aravena-Canales D, Molina A, Valdés JA. Role of glucocorticoid and mineralocorticoid receptors in rainbow trout (Oncorhynchus mykiss) skeletal muscle: A transcriptomic perspective of cortisol action. Front Physiol. (2023) 13:1048008. doi: 10.3389/fphys.2022.1048008
51. Blondeau-Bidet E, Banousse G, L’Honoré T, Farcy E, Cosseau C, Lorin-Nebel C. The role of salinity on genome-wide DNA methylation dynamics in European sea bass gills. Mol Ecol. (2023) 32:5089–109. doi: 10.1111/mec.17089
52. Yang J, Liu M, Zhou T, Li Q, Lin Z. Transcriptome and methylome dynamics in the gills of large yellow croaker (Larimichthys crocea) during low-salinity adaption. Front Mar Sci. (2023) 10:1082655. doi: 10.3389/fmars.2023.1082655
53. Yue Y, Wang Y, Zhang B, Zeng J, Wang Q, Wang C, et al. Whole-genome methylation sequencing of large yellow croaker (Larimichthys crocea) liver under hypoxia and acidification stress. Mar Biotechnol. (2023) 25:567–79. doi: 10.1007/s10126-023-10226-3
54. Lin YL, Zhu ZX, Ai CH, Xiong YY, De Liu T, Lin HR, et al. Transcriptome and DNA methylation responses in the liver of yellowfin seabream under starvation stress. Mar Biotechnol. (2023) 25:150–60. doi: 10.1007/s10126-022-10188-y
55. Moghadam HK, Johnsen H, Robinson N, Andersen Ø, H. Jørgensen E, Johnsen HK, et al. Impacts of early life stress on the methylome and transcriptome of atlantic salmon. Sci Rep. (2017) 7:5023. doi: 10.1038/s41598-017-05222-2
56. Uren Webster TM, Rodriguez-Barreto D, Martin SAM, Van Oosterhout C, Orozco-terWengel P, Cable J, et al. Contrasting effects of acute and chronic stress on the transcriptome, epigenome, and immune response of Atlantic salmon. Epigenetics. (2018) 13:1191–207. doi: 10.1080/15592294.2018.1554520
57. Wellband K, Roth D, Linnansaari T, Curry RA, Bernatchez L. Environment-driven reprogramming of gamete DNA methylation occurs during maturation and is transmitted intergenerationally in Atlantic Salmon. G3 Genes|Genomes|Genetics. (2021) 11:jkab353. doi: 10.1093/g3journal/jkab353
58. Valenzuela-Muñoz V, Wanamaker S, Núñez-Acuña G, Roberts S, Garcia A, Valdés JA, et al. Environmental influence on the Atlantic salmon transcriptome and methylome during sea lice infestations. Fish Shellfish Immunol. (2024) 151:109692. doi: 10.1016/j.fsi.2024.109692
59. Niemi NM, Friedman JR. Coordinating BNIP3/NIX-mediated mitophagy in space and time. Biochem Soc Trans. (2024) 52:1969–79. doi: 10.1042/BST20221364
60. Dong Y, Jia R, Hou Y, Diao W, Li B, Zhu J. Effects of stocking density on the growth performance, mitophagy, endocytosis and metabolism of Cherax quadricarinatus in integrated rice–crayfish farming systems. Front Physiol. (2022) 13:1040712. doi: 10.3389/fphys.2022.1040712
61. Veron V, Marandel L, Liu J, Vélez EJ, Lepais O, Panserat S, et al. DNA methylation of the promoter region of bnip3 and bnip3l genes induced by metabolic programming. BMC Genomics. (2018) 19:677. doi: 10.1186/s12864-018-5048-4
62. Choi J, Jang H, Xuan Z, Park D. Emerging roles of ATG9/ATG9A in autophagy: implications for cell and neurobiology. Autophagy. (2024) 20(11):1–15. doi: 10.1080/15548627.2024.2384349
63. Valenzuela CA, Azúa M, Álvarez CA, Schmitt P, Ojeda N, Mercado L. Evidence of the Autophagic Process during the Fish Immune Response of Skeletal Muscle Cells against Piscirickettsia salmonis. Animals. (2023) 13:880. doi: 10.3390/ani13050880
64. Zhang X, Li C, Wang D, Chen Q, Li C-L, Li H-J. Aberrant methylation of ATG2B, ATG4D, ATG9A and ATG9B CpG island promoter is associated with decreased mRNA expression in sporadic breast carcinoma. Gene. (2016) 590:285–92. doi: 10.1016/j.gene.2016.05.036
65. Huang Y, Zhen Y, Chen Y, Sui S, Zhang L. Unraveling the interplay between RAS/RAF/MEK/ERK signaling pathway and autophagy in cancer: From molecular mechanisms to targeted therapy. Biochem Pharmacol. (2023) 217:115842. doi: 10.1016/j.bcp.2023.115842
66. Degerman E, Ahmad F, Chung YW, Guirguis E, Omar B, Stenson L, et al. From PDE3B to the regulation of energy homeostasis. Curr Opin Pharmacol. (2011) 11:676–82. doi: 10.1016/j.coph.2011.09.015
67. Sen N, Gui B, Kumar R. Physiological functions of MTA family of proteins. Cancer Metastasis Rev. (2014) 33:869–77. doi: 10.1007/s10555-014-9514-4
68. Navickas SM, Giles KA, Brettingham-Moore KH, Taberlay PC. The role of chromatin remodeler SMARCA4/BRG1 in brain cancers: a potential therapeutic target. Oncogene. (2023) 42:2363–73. doi: 10.1038/s41388-023-02773-9
69. Paes T, Feelders RA, Hofland LJ. Epigenetic mechanisms modulated by glucocorticoids with a focus on cushing syndrome. J Clin Endocrinol Metab. (2024) 109:e1424–33. doi: 10.1210/clinem/dgae151
70. Liu W, Mohan SP, Nagaraj NR, Sundar Jaganathan S, Wen Y, Ramasubramanyan S, et al. Epigenetic alterations associated with dexamethasone sodium phosphate through DNMT and TET in RPE cells. Mol Vis. (2021) 27:643–55. Available at: http://www.molvis.org/molvis/v27/643.
Keywords: oxidative stress, aquaculture, methylome, transcriptome, growth
Citation: Aravena-Canales D, Valenzuela-Muñoz V, Gallardo-Escarate C, Molina A and Valdés JA (2025) Molecular and epigenetic responses to crowding stress in rainbow trout (Oncorhynchus mykiss) skeletal muscle. Front. Endocrinol. 16:1571111. doi: 10.3389/fendo.2025.1571111
Received: 05 February 2025; Accepted: 27 March 2025;
Published: 16 April 2025.
Edited by:
Athanasios Samaras, University of Crete, GreeceReviewed by:
Marco Albano, University of Messina, ItalyYuwen Dong, University of Pennsylvania, United States
Katerina Moutou, University of Thessaly, Greece
Copyright © 2025 Aravena-Canales, Valenzuela-Muñoz, Gallardo-Escarate, Molina and Valdés. This is an open-access article distributed under the terms of the Creative Commons Attribution License (CC BY). The use, distribution or reproduction in other forums is permitted, provided the original author(s) and the copyright owner(s) are credited and that the original publication in this journal is cited, in accordance with accepted academic practice. No use, distribution or reproduction is permitted which does not comply with these terms.
*Correspondence: Juan Antonio Valdés, anZhbGRlc0B1bmFiLmNs