- 1Center for Reproductive Medicine, XuZhou Central Hospital, Xuzhou, Jiangsu, China
- 2Department of Obstetrics and Gynecology, The Second Affiliated Hospital of Soochow University, Suzhou, Jiangsu, China
- 3Jiangsu Key Laboratory of New Drug Research and Clinical Pharmacy, Xuzhou Medical University, Xuzhou, Jiangsu, China
- 4Department of Obstetrics and Gynecology, XuZhou Central Hospital, Xuzhou, Jiangsu, China
Objective: Proteomic analysis was conducted on human follicular fluid (FF) using the 4D label-free method to identify proteins potentially influencing oocyte quality in hyperandrogenic (HA) polycystic ovary syndrome (PCOS) patients.
Methods: FF was collected from 3 different groups: HA PCOS patients, non-hyperandrogenic (NHA) PCOS patients, and controls. Protein profiles of FF from HA PCOS patients (n = 10) were constructed utilizing 4D label-free proteomics technology. Differentially expressed proteins were identified by comparing these profiles with those from NHA PCOS (n = 10) and control patients (n = 10). In addition, FF was collected from 34 HA, 33 NHA, and 23 control patients for enzyme-linked immunosorbent assay (ELISA) validation of differentially expressed proteins. Associations between the levels of differentially expressed proteins in FF and various embryonic outcome indicators were evaluated.
Results: The HA group demonstrated significantly reduced normal cleavage rates, D3 available embryo rates, D3 high-quality embryo rates, available blastocyst rates, and high-quality blastocyst rates compared to the NHA and control groups (HA vs. NHA vs. Control, 88.3 vs. 93.6 vs. 94.23, 70.57 vs. 81.76 vs. 83.77, 42.49 vs. 56.39 vs. 61.83, 55.0 vs. 65.96 vs. 67.26, 27.62 vs. 45.19 vs. 44.75, respectively), with statistically significant differences (P < 0.05). 23 differentially expressed proteins were identified in FF profiles of the HA group relative to the control group, while 9 differentially expressed proteins were noted in comparison with the NHA group. Cross-comparison highlighted three potential target proteins: insulin-like growth factor binding protein 5 (IGFBP5), lysosomal-associated membrane protein 2 (LAMP2), and cadherin-5 (CDH5). Adjusting for age and body mass index (BMI), IGFBP5 levels in FF exhibited negative correlations with normal cleavage rate, D3 high-quality embryo rate, available blastocyst rate, and high-quality blastocyst rate (P ≤ 0.05). Similarly, LAMP2 levels were negatively correlated with normal cleavage rate, D3 available embryo rate, D3 high-quality embryo rate, and high-quality blastocyst rate (P < 0.05). CDH5 levels demonstrated positive correlations with D3 high-quality embryo rate and high-quality blastocyst rate (P < 0.05).
Conclusion: The proteins IGFBP5, LAMP2, and CDH5 may contribute to the mechanisms underlying the adverse effects of hyperandrogenism on oocyte quality in PCOS patients.
Introduction
Polycystic ovary syndrome (PCOS) is a common endocrine metabolic disorder, which is characterized by oligo/anovulation, clinical or biochemical hyperandrogenism, and/or polycystic ovaries. It is known to affect approximately 6 to 10% of women of reproductive age (1). Although the international guidelines have stated PCOS as a risk factor for infertility only in the presence of oligo-ovulation or anovulation and recommend in-vitro fertilization-embryo transfer (IVF-ET) as a last solution, a high proportion of women with PCOS fail to conceive after ovulation and require hospital visits for infertility and IVF treatment 8 and 10 times more frequently, respectively than those without PCOS (1). Previous studies have demonstrated that even if patients with PCOS have undergone IVF to assist conception and can achieve abundant oocytes during the ovulation-promoting process of IVF, the quality of their oocytes is usually poor. In addition, the rates of normal fertilization of oocytes, egg cleavage, high-quality embryos, and blastocyst formation are significantly lower than those in the general control population (2). Therefore, in addition to ovulation disorders, decreased oocyte quality is likely to affect the fertility of patients with PCOS.
Hyperandrogenism, one of the three clinical features of the Rotterdam Criteria for PCOS, has been implicated in the pathophysiologic mechanisms underlying PCOS (3). The administration of exogenous androgen alone is the most commonly used method to establish animal models of PCOS (4). Previous studies have demonstrated that patients with PCOS without hyperandrogenism, similar to controls, have significantly higher pregnancy rates and live birth rates compared to those with hyperandrogenism (5). Thus, hyperandrogenic status reduces the pregnancy and live birth rates in patients with PCOS. It remains unknown if reduced pregnancy rate and live birth rate in patients with hyperandrogenic PCOS are related to their poor oocyte quality. Furthermore, specific mechanisms by which hyperandrogenism affects oocyte quality remain elusive.
Follicular fluid (FF), present in the lumen of growing follicles, constitutes an immediate microenvironment for oocyte development and maturation (6), thus reflecting the status of oocytes to a certain extent. Because it is ethically challenging to acquire normal oocytes and FF is a by-product that can be easily obtained during IVF egg retrieval, researchers usually study FF to assess the status of oocytes. Recently, researchers have investigated the pathogenesis of PCOS by comparing the differences in the protein profiles of FF from patients with and without PCOS (6–8), screened for biomarkers, and elucidated the possible pathogenesis of PCOS at the molecular protein level. However, no previous FF proteomics studies have addressed hyperandrogenic versus non-hyperandrogenic phenotypes within the PCOS population, with considerably few studies linking them to oocyte quality.
In the present study, we applied the 4D label-free proteomics technology to construct an FF protein profile of patients with hyperandrogenic PCOS and subsequently explore the possible mechanisms by which hyperandrogenism affects the oocyte quality of these patients. In addition, we identified possible targets for clinical diagnosis and treatment to improve the oocyte quality of patients with hyperandrogenic PCOS.
2 Materials and methods
2.1 Patient selection and stimulation protocol
Patients recruited for this study underwent IVF-ET at Xuzhou City Central Hospital between March 2021 and May 2024. These patients received superovulation treatment using an antagonist regimen and were subsequently fertilized via IVF. The study was approved by the Biomedical Research Ethics Review Committee of Xuzhou Central Hospital (approval number: XZXY-LK-20200331-027). All patients signed an informed consent form before participating.
Patients were diagnosed with PCOS according to the Rotterdam criteria (9). They were divided into the hyperandrogenic (HA) PCOS and non-hyperandrogenic (NHA) PCOS groups. The diagnosis of hyperandrogenism was based on clinical hyperandrogenism (modified FG score ≥ 8) or biochemical hyperandrogenism (serum total testosterone [TT] ≥ 2.6 nmol/L; or free androgen index (FAI ≥ 6.4, FAI = T [nmol/L] × 100/SHBG [nmol/L]); or free testosterone (FT) ≥ 20.82 pmol/L (9, 10); or DHE-S ≥ 10.6 µmol/L. The control group included patients undergoing IVF for tubal or male factor infertility. Exclusion criteria included age > 40 years, chromosomal abnormalities, history of recurrent miscarriages, ovarian tumors, history of surgery, history of malignancy, immune system disorders, history of more than three IVF failures, comorbidities, such as endometriosis, dysfunction of thyroid, adrenal, and pituitary, and the use of any medication affecting blood glucose, lipids, and sex hormones within 3 months. A total of 267 PCOS patients (159 HA patients and 108 NHA patients) and 203 control patients were recruited.
2.2 Sample collection
FF samples were collected from 10 patients with HA, 10 patients with NHA, and 10 controls for proteomic analysis. FF samples were also collected from an additional 34 HA, 33 NHA, and 23 control patients for enzyme-linked immunosorbent assay (ELISA) validation of differentially expressed proteins.
FF was collected by transvaginal ultrasound-guided aspiration on 35 to 37 h after the administration of human chorionic gonadotropin (HCG) (LiZhu Pharma; Zhuhai, China). Only clear FF samples without macroscopic blood contamination were included. After oocyte isolation, FF samples were centrifuged at 3000 rpm for 10 min to remove cells and insoluble particles. Subsequently, the supernatant was separated and stored at −80°C for further use.
2.3 4D label-free relative quantitative proteomics based on FF sample detection
2.3.1 Mass spectrometry experimental procedures
2.3.1.1 Protein extraction and digestion
Proteins were extracted from the FF of 30 patients. The SDT buffer (4% sodium dodecyl sulfate [SDS], 100 mM Tris-HCl, pH 7.6) was used for sample lysis and protein extraction. Protein quantification was performed using the bicinchoninic acid (BCA) protein assay kit (Bio-Rad, USA). Briefly, 20 µg of protein for each sample was mixed with 5× loading buffer and boiled for 5 min. Proteins were separated on a 4% to 20% SDS-PAGE gel (constant voltage: 180V, 45 min). Protein bands were visualized by Coomassie Blue R-250 staining.
An appropriate amount of protein was collected from each sample and trypsinized using the filter-aided proteome preparation (FASP) method described by Matthias Mann. The digested peptides of each sample were desalted on a C18 cartridge (Empore™ SPE Cartridges C18 [standard density], bed I.D. 7 mm, volume 3 mL, Sigma), concentrated by vacuum centrifugation and reconstituted in 40 µL of 0.1% (v/v) formic acid. The peptide content was estimated by ultraviolet (UV) light spectral density at 280 nm using an extinction coefficient of 1.1 for 0.1% (g/L) solution calculated from the abundance of tryptophan and tyrosine in vertebrate proteins.
2.3.1.2 Liquid chromatography-tandem mass spectrometry (LC-MS/MS) data analysis
Liquid chromatography-mass spectrometry (LC-MS/MS) was performed on a timsTOF Pro mass spectrometer (Bruker) coupled to a Nanoelute (Bruker). The peptides were applied to a C18-reversed-phase analytical column (Thermo Scientific Easy column; 25 cm long, 75 μm inner diameter, 1.9 μm resin) in 95% buffer A (0.1% aqueous formic acid solution) connected to a linear gradient separation in buffer B (99.9% acetonitrile and 0.1% formic acid) at a flow rate of 300 nL/min. The mass spectrometer was operated in the positive ion mode. The applied electrospray voltage was 1.5 kV. Precursors and fragments were analyzed on a TOF detector with a mass range of m/z 100 to 1700. The timsTOF Pro was operated in parallel accumulation serial fragmentation (PASEF) mode; data acquisition in the PASEF mode was based on the following parameters: ion mobility coefficient (1/K0) value was set from 0.6 to 1.6 Vs cm2; 1 MS and 10 MS/MS PASEF scans. Active exclusion was enabled with a release time of 24 s.
2.3.2 Bioinformatics analysis
The raw MS data of each sample were retrieved, identified, and quantitatively analyzed using the MaxQuant 1.6.14 software. The database was (Swissprot_Homo_sapiens_20376_20220104.fasta). Subcellular localization was predicted using the CELLO method (http://cello.life.nctu.edu.tw/). For selected genes, functional classification and enrichment analyses were performed using the ToppGene online tool (https://toppgene.cchmc.org/enrichment.jsp). The overall molecular function (MF), biological process (BP) and cellular component (CC) of the genes were analyzed using the built-in GO triple classification annotation data of ToppGene, and the Reactome data of the genes were used to analyze the regulatory pathways in which the gene clusters were mainly involved. We also used ToppGene’s built-in Reactome data to analyze the regulatory pathways in which the gene clusters are mainly involved. Finding direct and indirect interactions between target proteins based on information in the STRING (http://string-db.org/) database.
2.4 ELISA validation
The differential abundance of the three selected proteins was verified by ELISA using FF samples from patients in HA, NHA, and control groups. ELISA was performed on FF samples, namely, LAMP2, IGFBP5, and CDH5, according to the manufacturer’s instructions. The dilution ratios were 1:2, 1:200, and 1:1000.
2.5 Statistics analysis
All data were analyzed using the statistical software SPSS version 24.0 for Windows. Continuous variables are described as mean ± standard deviation ( ± s). Differences between the groups were determined by Student’s t-test or one-way analysis of variance (ANOVA). Partial correlation analysis was conducted to investigate the connections between the selected protein and various embryo quality assessment indices after adjusting for age, body mass index (BMI). A statistically significant difference was defined as p ≤ 0.05 (two-tailed).
3 Results
3.1 Comparison of general conditions among three groups of patients
We collected data from 203 control, 108 NHA, and 159 HA patients. A comparison of baseline characteristics and ovulation promotion outcomes in the three groups is shown in Table 1.
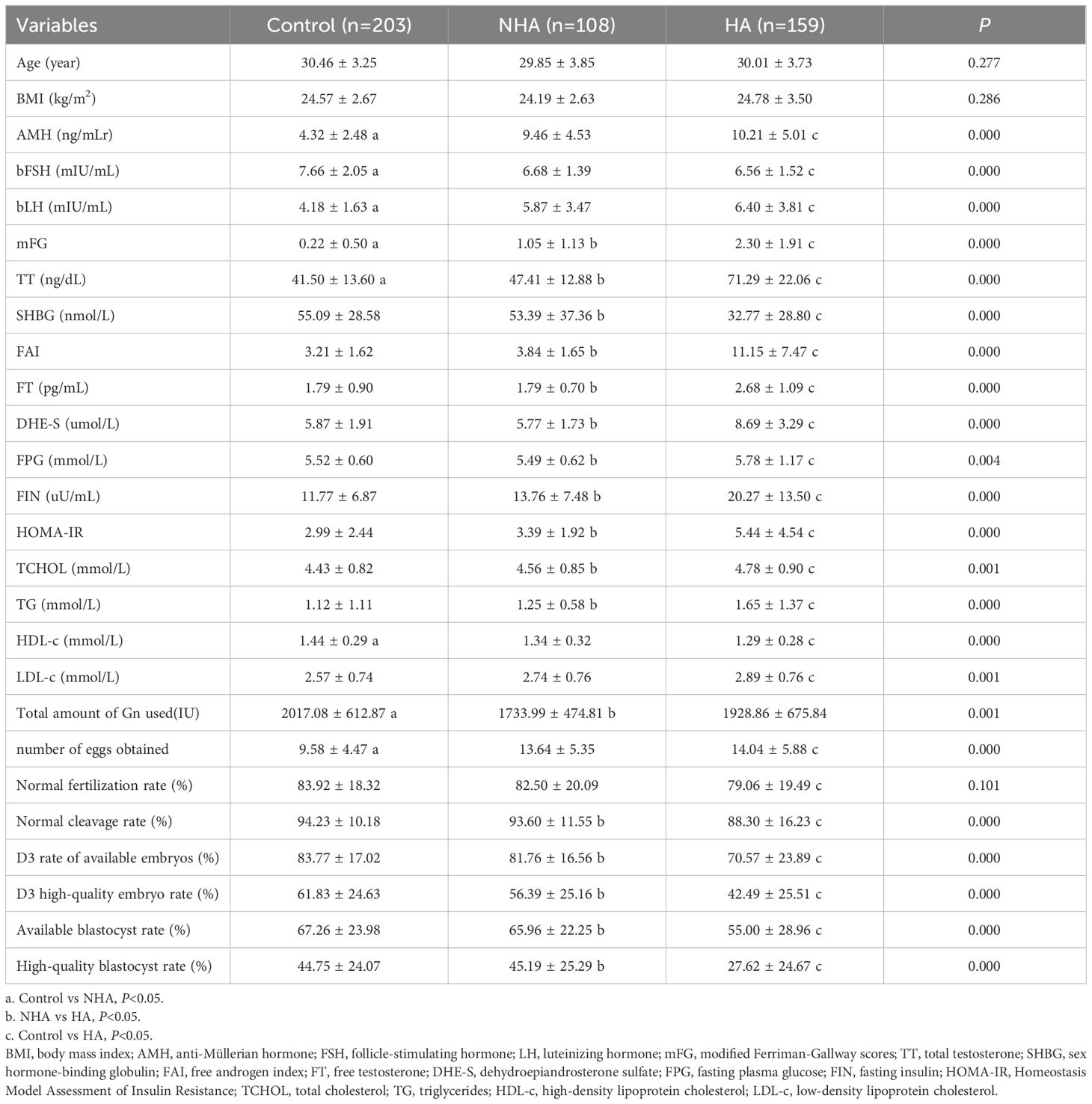
Table 1. Comparison of clinical, hormonal and biochemical characteristics and embryonic conditions in three groups of patients.
3.2 Quantitative FF protein profiling
We performed 4D label-free relative quantitative proteomic analysis on 30 samples, which identified 537 proteins, of which 435 proteins had quantitative information. A fold change (FC) value of more than 1.5-fold change and P < 0.05 were used as the screening criteria, and 23 differentially expressed proteins were screened between HA and control groups (Figure 1A), and nine differentially expressed proteins were screened between HA and NHA groups (Figure 1B). Information on annotation and quantification of differentially expressed proteins is listed in Tables 2 and 3. The subcellular localization of differentially expressed proteins was primarily concentrated in the extracellular region (HA/C: 53.33%, HA/NHA: 58.33%).
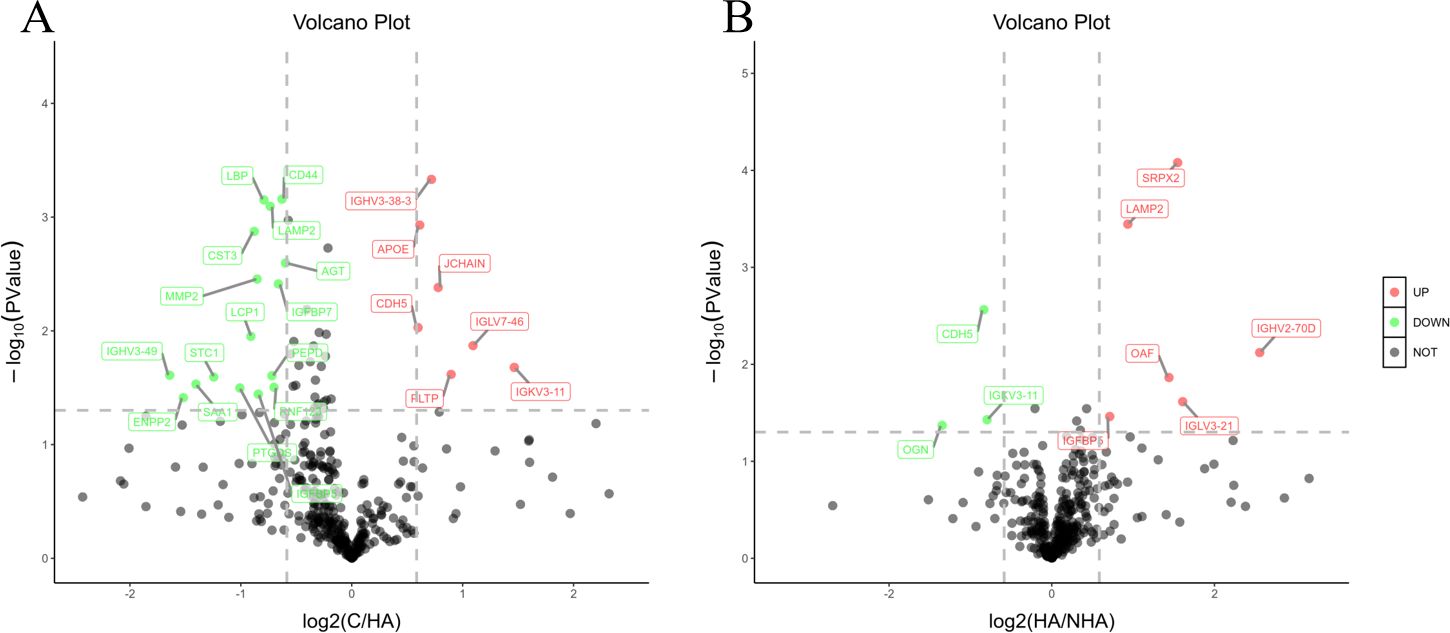
Figure 1. Volcano plot of differentially expressed proteins between different groups. Green points in the graph are differential proteins with down-regulated expression, red points are differential proteins with up-regulated expression, and gray points are proteins with no differential change; vertical coordinates are P-values (logarithmic with base 10), and horizontal coordinates are multiplicity of differences (logarithmic with base 2). (A): C/HA (control group vs. hyperandrogenic PCOS group); (B): HA/NHA (hyperandrogenic PCOS group vs. non-hyperandrogenic PCOS group).
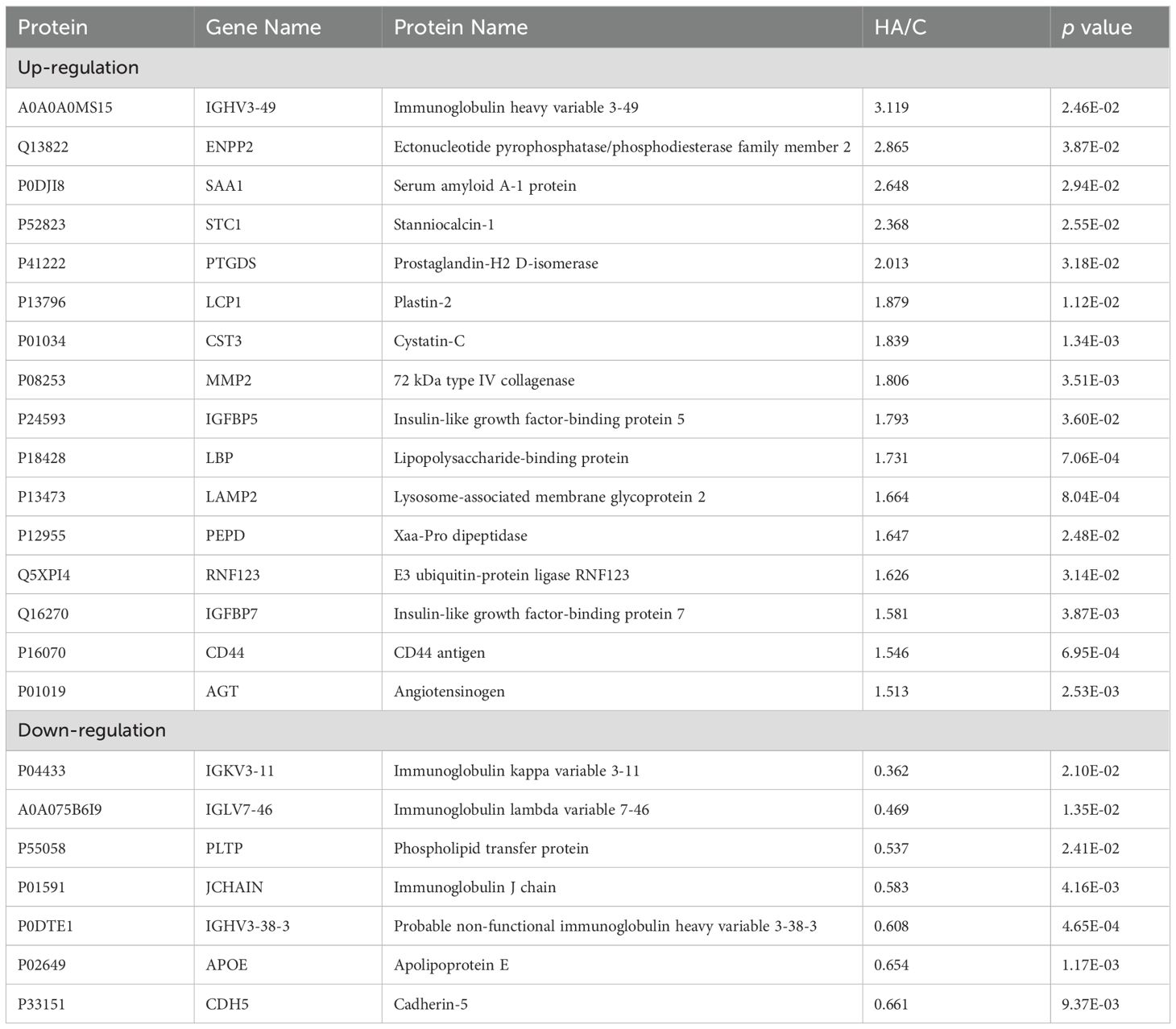
Table 2. Annotation and quantitative information of differentially expressed proteins in the follicular fluid of the HA and Control groups.
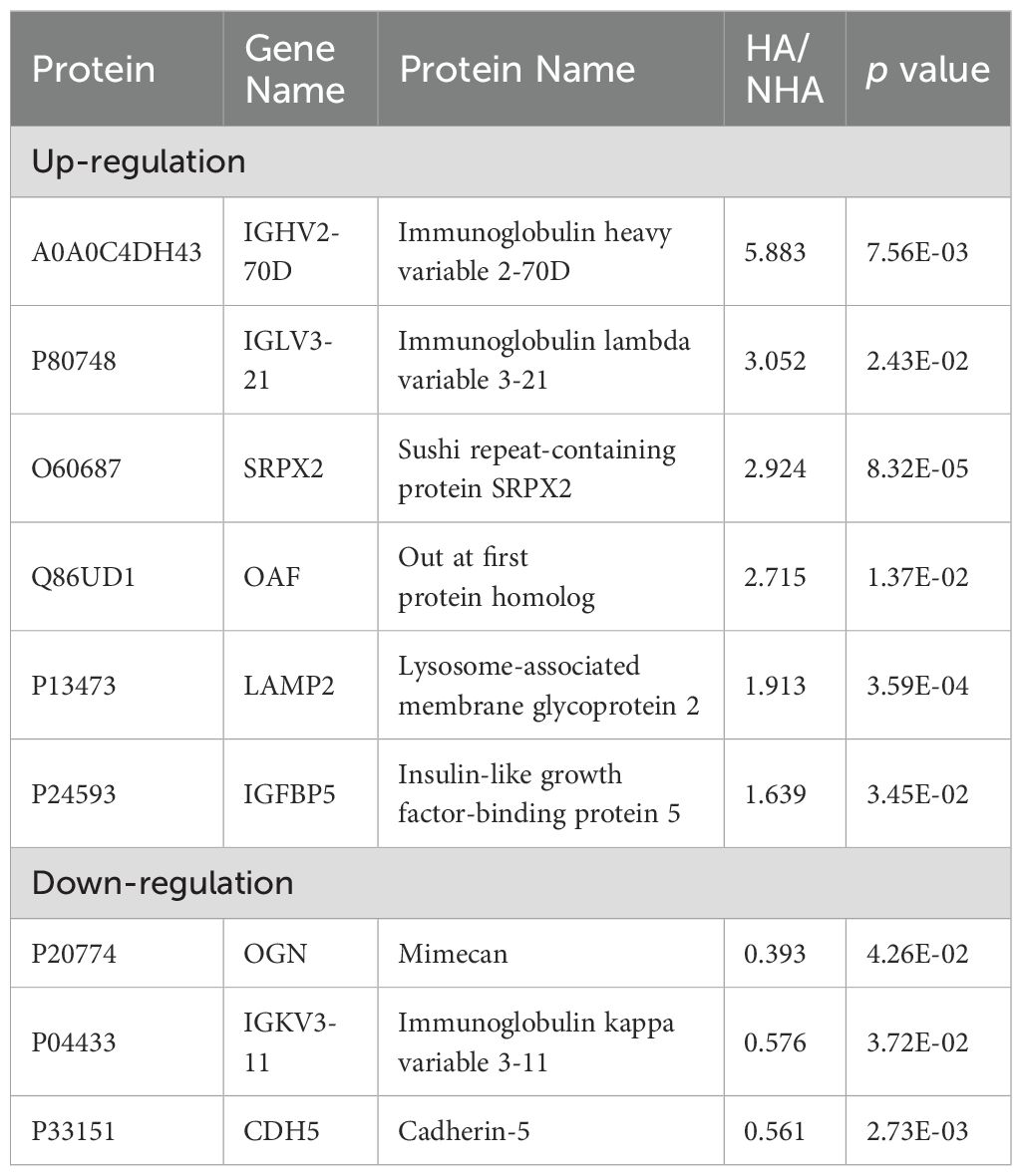
Table 3. Annotation and quantitative information of differentially expressed proteins in the follicular fluid of the HA and NHA groups.
GO functional annotation results demonstrated that 23 differentially expressed proteins of HA/C were primarily enriched in 2,246 GO terms. Among them, 1,876 terms were related to BP, with 855 statistically significant. Differentially expressed proteins were most enriched in biological processes such as tissue morphogenesis, regulation of multicellular biological processes, remodeling of protein–lipid complexes, female pregnancy, inflammatory response, collagen catabolic process, and cell migration (Figure 2A). Furthermore, 191 were related to MF, with 101 statistically significant, and the molecular functions largely corresponded to binding functions, such as signaling receptor binding, glycosaminoglycan binding, insulin-like growth factor binding, lipoprotein particle binding, and activation of cholesterol transfer, and sterol transfer (Figure 2B). Similarly, 179 terms were related to CC, with 70 statistically significant; differentially expressed proteins were largely implicated in the formation of different lipoprotein particles, protein–lipid complexes, immunoglobulin complexes, and several extracellular matrices (Figure 2C). The pathways enriched were identified by Reactome enrichment analysis (Figure 2D). The main pathways of enrichment were immune and metabolism-related pathways.
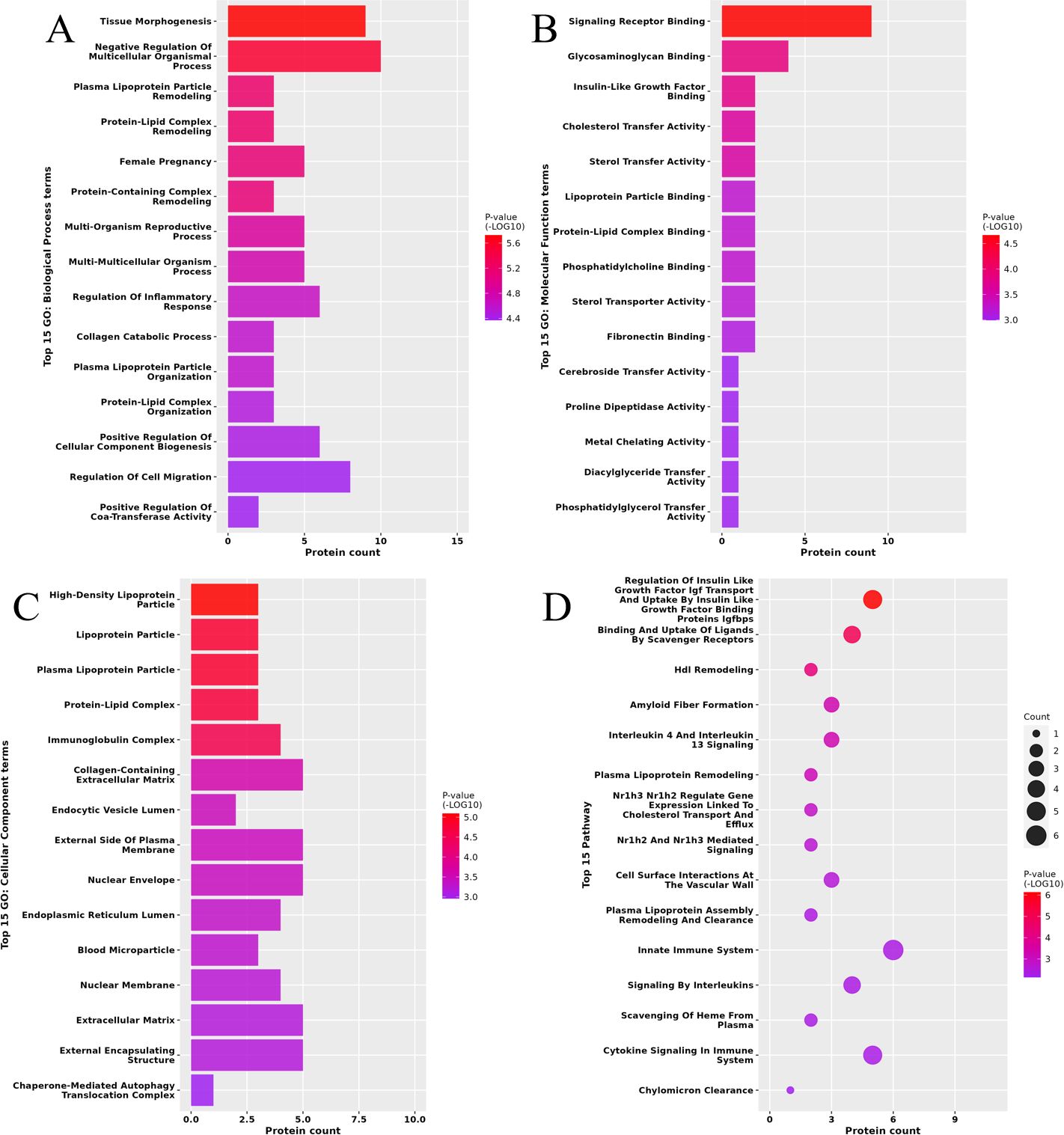
Figure 2. Functional enrichment of differentially expressed proteins (DEPs) in follicular fluid of hyperandrogenic (HA) and control patients. Biological process (BP) analysis (A). Molecular function (MF) analysis (B). Cellular component (CC) analysis (C). Reactome enrichment analysis (D).
HA/NHA differentially expressed proteins were majorly enriched in 506 GO terms. Among them, 395 were related to BP, with 139 statistically significant, and the differentially expressed proteins were largely involved in biological processes such as blood vessel lumen ensheathment, vascular endothelial cell stratification, endothelial tubular lumen expansion, and negative regulation of smooth muscle cell proliferation (Figure 3A). Furthermore, 37 were related to MF, with 19 statistically significant, and were majorly based on the binding function, including hepatocyte growth factor binding, growth factor binding, fibrinogen binding, insulin-like growth factor binding, vascular endothelial growth factor receptor binding, and BMP receptor binding function (Figure 3B). Next, we found that 74 were related to CC, with 32 statistically significant, and largely involved in the composition of autophagy translocation complex, autophagic vesicle, lysosome, immunoglobulin complex, insulin-like growth factor complex, and platelet dense granule membrane (Figure 3C). The pathways enriched were identified by Reactome enrichment analysis (Figure 3D). The main pathways of enriched were the complement cascade pathway and the inflammation-related pathway.
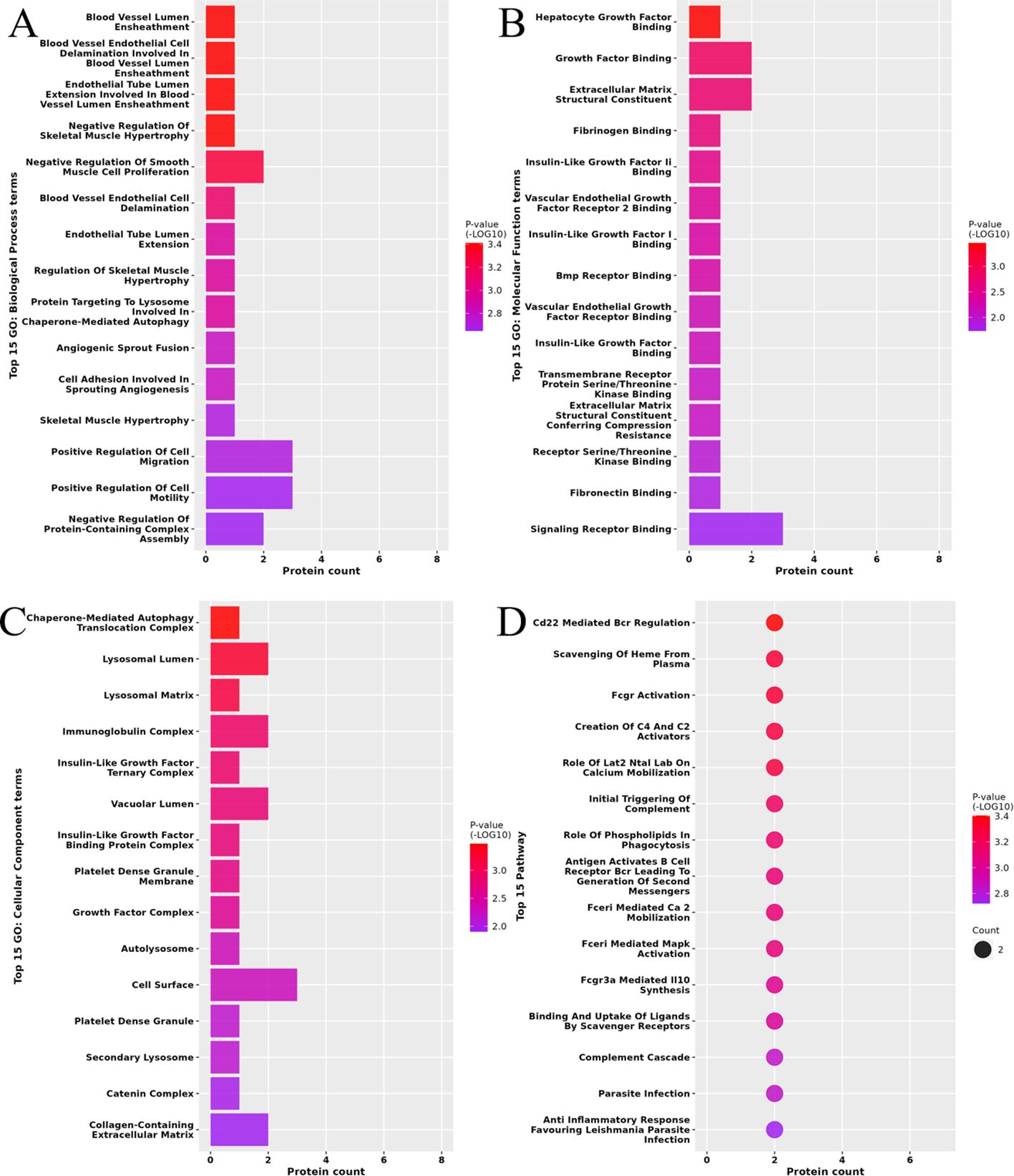
Figure 3. Functional enrichment of differentially expressed proteins (DEPs) in follicular fluid of hyperandrogenic (HA) and non-hyperandrogenic (NHA) PCOS patients. Biological process (BP) analysis (A). Molecular function (MF) analysis (B). Cellular component (CC) analysis (C). Reactome enrichment analysis (D).
3.3 Validation of three differentially expressed proteins
The clinical data revealed the proteins with statistically significant differences between the HA and NHA groups, and between the HA and control groups, and those with statistically insignificant differences between the NHA and control groups were considered target proteins. Finally, three target proteins were screened, namely, LAMP2, IGFBP5, and CDH5. ELISA results are shown in Table 4. As demonstrated in Table 4, patients in the HA group exhibited notably elevated IGFBP5 and LAMP2 levels in FF, while CDH5 levels were considerably reduced compared to those in the NHA and control groups (P < 0.05).
3.4 Correlation analysis between three differentially expressed proteins in follicular fluid and embryonic outcome
After correcting for age and BMI, the levels of IGFBP5 in the FF were negatively correlated with the normal cleavage rate, D3 high-quality embryo rate, available blastocyst rate, and high-quality blastocyst rate (P ≤ 0.05). Similarly, the levels of LAMP2 in the FF were negatively correlated with the normal cleavage rate, D3 available embryo rate, D3 high-quality embryo rate, and high-quality blastocyst rate (P < 0.05). Furthermore, the levels of CDH5 within the FF were positively correlated with the rate of D3 high-quality embryos and the rate of high-quality blastocysts in patients (P < 0.05, Table 5).
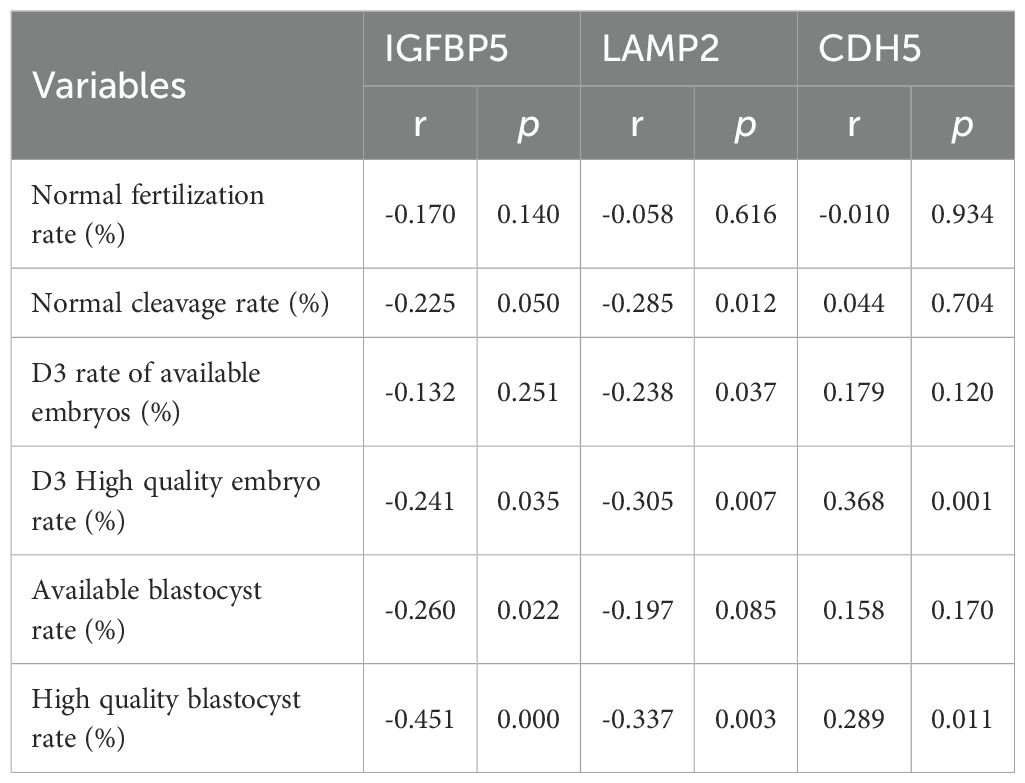
Table 5. Correlation analysis of IGFBP5, LAMP2, and CDH5 levels in follicular fluid with embryonic outcome after correction for age and BMI.
3.5 Interactions between the three target proteins
Finding direct and indirect interactions between target proteins (LAMP2, IGFBP5, and CDH5) based on information in the STRING database. The interacting protein networks of the three target proteins were identified using the STRING database and the networks were visualized (Figure 4). 20 interactors were identified in the graph. There were no direct interactions between the three proteins as shown in Figure 4. ABCB9, FBXO27 and SELE were found to interact directly with LAMP2. PCDH12, SELE, CDH24, CDH26, IGF1, PTPRB, and ESAM were found to interact directly with CDH5. CLEC17A, IGF2, IGF1, IGFBP3, IGFALS, IGFBP6, PAPPA2, and PAPPA are found to interact directly with IGFBP5. Other indirectly interacting proteins include STC2, LGALS13, IGFBP4 and IGFBP2.
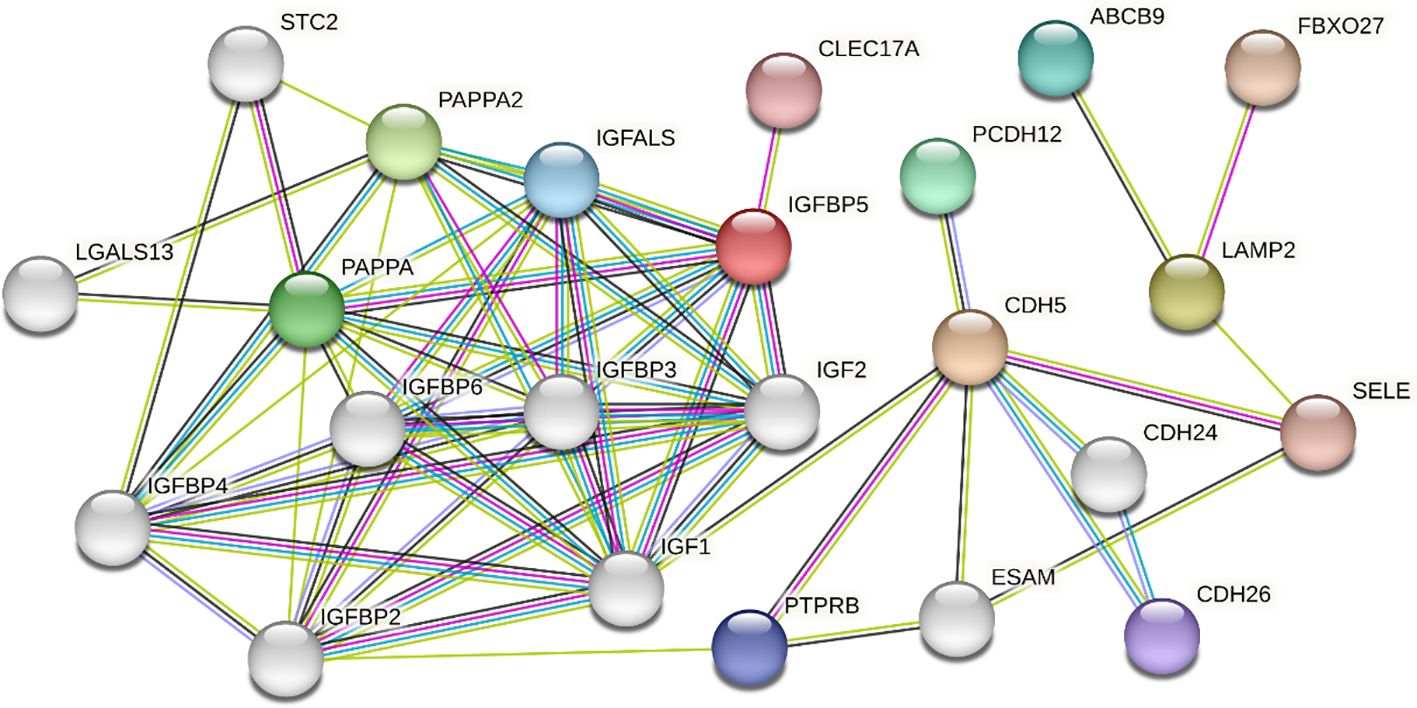
Figure 4. Analysis of direct and indirect interactions between three proteins based on information from the STRING database.
4 Discussion
“Heterogeneity” is a highly distinctive feature of PCOS and inevitably contributes to its onset and progression. Therefore, it is necessary to characterize the changes in oocyte quality in PCOS and the mechanisms influencing them according to the type of PCOS. Previously, limited studies have focused on first categorizing patients by type before investigating the relationship between PCOS and embryonic outcomes, yet the findings of these studies have generally been consistent. For instance, patients with PCOS with phenotype A (coexistence of hyperandrogenism, ovulation disorders, and polycystic ovaries) or those with a combination of phenotypes, such as obesity, insulin resistance (IR), and metabolic syndrome, are at a significantly increased risk for decreased oocyte quality (1, 5).
The findings of the present study align with those of previous studies because, among the four phenotypes of PCOS, patients with phenotype D (i.e., uncomplicated hyperandrogenic status) have the lowest risk and degree of metabolic abnormalities (11). A study by Huang et al. concluded hyperandrogenism is a key factor contributing to impaired IR and other metabolic conditions (12). Consistent with the results of this study, patients in the hyperandrogenic PCOS group in the present study showed a significantly impaired glucose and lipid metabolism compared with those in the non-hyperandrogenic PCOS and control groups. Therefore, hyperandrogenic status may be one of the reasons for the decreased oocyte quality in patients with hyperandrogenic PCOS. The results from a few previous studies support this conclusion (5, 13–15). Statistical analysis of certain clinical data revealed that hyperandrogenism can affect the quality of oocytes in patients with PCOS, thereby significantly reducing the fertilization rate, rate of formation of high-quality embryos, rate of blastocyst formation, and rate of clinical pregnancy (5). In addition, animal studies have demonstrated that excess androgens can affect the quality of oocytes (13–15).
For the first time, protein profiles of FF in patients with hyperandrogenic PCOS were compared to those with non-hyperandrogenic PCOS and age- and BMI-matched general control patients using 4D label-free relative quantitative proteomics.
The FF protein profiles of hyperandrogenic PCOS patients were compared with those of control patients. The findings indicated that the proteins expressed differentially were primarily associated with immunity, inflammation and metabolism. Serum amyloid A-1 protein (SAA1), a marker of inflammation produced mainly by the liver, is significantly increased in peripheral blood, granulosa cells, FF, and endometrium of patients with PCOS, and its elevated expression can promote the occurrence of IR and miscarriage in PCOS patients (16, 17). Özdemir Başer and colleagues reported a significant increase in serum cystatin C levels among PCOS patients, which correlated positively with interleukin 6, an inflammatory factor (18). Additionally, matrix metalloproteinases (MMPs), which influence ovarian tissue remodeling and are involved in PCOS pathogenesis (19), were found to be elevated in PCOS patients, with higher serum levels of MMP9 and MMP2 leading to follicular dysplasia (20).
The insulin-like growth factor-binding proteins (IGFBPs) family modulates the bioavailability of insulin and insulin-like growth factor (IGF) through binding, thereby facilitating their respective physiological functions (21). Prior research has indicated that both IGFBP7 and IGFBP5 have significant roles in the progression of type 2 diabetes and its related complications (22, 23). Lysosome-associated membrane glycoprotein 2 (LAMP2), an autophagy-linked protein, has been linked to the development of various inflammatory diseases due to its altered expression. Notably, autophagy levels are markedly increased in ovarian tissues and granulosa cells of patients with PCOS or in PCOS model rats (24, 25). Lipopolysaccharide (LPS) stimulates the release of inflammatory factors, and its key ligand, lipopolysaccharide-binding protein (LBP), activates the inflammatory response by interacting with LPS. Studies have shown that LBP levels are significantly elevated in the serum of PCOS patients and correlate independently with IR in this condition (26). High endothelial permeability is pivotal in inflammatory and immune responses, and CDH5 serves as a crucial marker of endothelial permeability; lower CDH5 expression levels correspond to higher endothelial permeability (27).
Apolipoprotein E (ApoE) plays a crucial role in lipid clearance, and its dysregulation can contribute to the development of atherosclerosis and cardiovascular disease. In experimental studies of atherosclerosis, ApoE-/- mice are among the most commonly used transgenic models (28). Phospholipid transfer protein (PLTP), a key player in lipoprotein conversion and metabolism, has been positively linked to the risk of cardiovascular diseases, including atherosclerosis (29). Nevertheless, studies have revealed that PLTP deficiency can worsen glucose intolerance and inflammatory conditions induced by a high-fat diet (30). It’s noteworthy that plasma PLTP activity can be diminished by insulin infusion or hyperinsulinaemia (31, 32). Remarkably, elevated circulating PLTP levels notably enhance glucose tolerance and insulin sensitivity (33). Our current investigation demonstrates a significantly reduced PLTP expression in the hyperandrogenic PCOS group compared to the control group.
The proteins expressed differentially in the comparison between the hyperandrogenic and non-hyperandrogenic groups were likewise linked to immunity, inflammation, and metabolism. This finding indicates that a significant androgen elevation may intensify immune, inflammatory, and metabolic disruptions in individuals with PCOS. Previous studies by Kumtornrut et al. and Quartier et al. have shown that the introduction of androgens elevates the gene expression of IGFBP5 (34, 35). Significant increases in autophagy-related markers have been observed in granulosa cells upon exposure to specific concentrations of dihydrotestosterone or testosterone propionate (36, 37). Furthermore, hypogonadal men undergoing testosterone replacement therapy have demonstrated notable elevations in the count of peripheral blood monocytes and dendritic cells, as well as enhanced LAMP2 expression (38). Although no prior research has explored the connection between hyperandrogenism and CDH5 expression, patients with hyperandrogenic PCOS face a notably elevated risk of ovarian hyperstimulation syndrome (OHSS) and exhibit significantly heightened vascular endothelial cell permeability during hyperovulation. This observation offers indirect corroboration for our study’s findings. Furthermore, previous studies have demonstrated that androgen excess can increase food intake and promote obesity by suppressing insulin and leptin signaling in the hypothalamus and upregulating the expression of pro-phagocytic genes (39). Mimecan, recently recognized as a novel satiety hormone (40), showed significantly reduced expression in the FF of the hyperandrogenic PCOS group.
Numerous preceding investigations align with the findings of our current study. Zhang and colleagues revealed an imbalance in immune and inflammatory responses within the FF of individuals with PCOS (41). Gaberšček et al. determined that PCOS is marked by persistent low-grade inflammation accompanied by autoimmune sequelae (42). Furthermore, Ascani et al. illustrated that elevated androgen levels can modify the frequency of B lymphocytes and elevate circulating immunoglobulin M titers (43).
By cross-referencing, our study pinpointed three proteins—IGFBP5, LAMP2, and CDH5—that are differentially expressed and may mediate the effects of hyperandrogenism on oocyte quality. We subsequently confirmed their expression levels in FF via ELISA, finding concordance with our proteomics data. Correlation analyses indicated that higher IGFBP5 and LAMP2 levels, coupled with lower CDH5 levels, correlate with reduced oocyte quality. These observations align with previous research (44–48).
Kulus M et al. demonstrated that IGFBP5 is primarily expressed in atretic follicles and serves as a marker of senescence in ovarian granulosa cells cultured in vitro (44). When IGFBP5 levels rise significantly in FF, the likelihood of oocytes developing into blastocysts within follicles notably diminishes (45). Furthermore, Peng et al. and Zhong et al. reported that increased IGFBP5a transcription levels are linked to the embryotoxic effects of antioxidants in zebrafish (46, 47). Exposure to aflatoxin B1, high temperature, and nonylphenol, all known to harm oocyte mass, leads to an elevation in LAMP2 expression (48–50). Previous research has rarely explored the association between CDH5 and oocyte or embryo development. Xu and colleagues found that, following in vitro maturation (IVM) of immature oocytes, the expression of CDH5 was notably elevated in the granulosa cells adjacent to oocytes capable of developing into blastocysts, compared to those unable to progress to this stage (51).
Although so many studies have shown a correlation between alterations in the three and oocyte quality, the exact mechanism of action is not currently elucidated in detail. The results of protein interactions analyses showed no direct interactions among the three proteins. Further analysis of their indirect interactions revealed that the three proteins may work together to influence oocyte quality through co-regulation of cell proliferation, inflammatory response and other processes. The specific mechanism will be determined by further studies in the future.
In summary, IGFBP5, LAMP2, and CDH5 may be involved in the process by which hyperandrogenism affects oocyte quality in PCOS patients. In future studies, we plan to further explore these differential proteins to reveal the pathogenesis of hyperandrogenic PCOS and provide new ideas to improve oocyte quality of hyperandrogenic PCOS patients.
Data availability statement
The datasets presented in this study can be found in online repositories. The names of the repository/repositories and accession number(s) can be found below: http://www.proteomexchange.org/, PXD060939.
Ethics statement
The studies involving humans were approved by Biomedical Research Ethics Review Committee of Xuzhou Central Hospital. The studies were conducted in accordance with the local legislation and institutional requirements. The participants provided their written informed consent to participate in this study.
Author contributions
QY: Data curation, Formal Analysis, Writing – original draft, Funding acquisition. JZ: Data curation, Formal Analysis, Investigation, Writing – review & editing. YC: Data curation, Formal Analysis, Writing – review & editing. XY: Funding acquisition, Writing – review & editing. HZ: Formal Analysis, Funding acquisition, Project administration, Supervision, Writing – review & editing.
Funding
The author(s) declare financial support was received for the research, authorship, and/or publication of this article. Scientific research project of Jiangsu Provincial Health and Health Commission (Z2023081); Open grant project of Jiangsu Provincial Key Laboratory of New Drug Research and Clinical Pharmacology (XZSYSKF2023012); Scientific research project of Jiangsu Maternal and Child Health Association (FYX202322); Science and Technology Development Fund of Affiliated Hospital of Xuzhou Medical University (XYFY202205); and National Natural Science Foundation of China (81873835, 81571405).
Acknowledgments
The authors thank all the subjects who participated in the study and the hospital staffs for their contribution in sample and data collection.
Conflict of interest
The authors declare that the research was conducted in the absence of any commercial or financial relationships that could be construed as a potential conflict of interest.
Generative AI statement
The author(s) declare that no Generative AI was used in the creation of this manuscript.
Publisher’s note
All claims expressed in this article are solely those of the authors and do not necessarily represent those of their affiliated organizations, or those of the publisher, the editors and the reviewers. Any product that may be evaluated in this article, or claim that may be made by its manufacturer, is not guaranteed or endorsed by the publisher.
References
1. Palomba S, Daolio J, and La Sala GB. Oocyte competence in women with polycystic ovary syndrome. Trends Endocrinol Meta. (2017) 28:186–98. doi: 10.1016/j.tem.2016.11.008
2. Tang K, Wu L, Luo Y, and Gong B. In vitro fertilization outcomes in women with polycystic ovary syndrome: A meta-analysis. Eur J Obstet Gynecol Reprod Bio. (2021) 259:146–52. doi: 10.1016/j.ejogrb.2021.02.023
3. Wang J, Wu D, Guo H, and Li M. Hyperandrogenemia and insulin resistance: The chief culprit of polycystic ovary syndrome. Life Sc. (2019) 236:116940. doi: 10.1016/j.lfs.2019.116940
4. Corrie L, Gulati M, Singh SK, Kapoor B, Khursheed R, Awasthi A, et al. Recent updates on animal models for understanding the etiopathogenesis of polycystic ovarian syndrome. Life Sc. (2021) 280:119753. doi: 10.1016/j.lfs.2021.119753
5. Ramezanali F, Ashrafi M, Hemat M, Arabipoor A, Jalali S, and Moini A. Assisted reproductive outcomes in women with different polycystic ovary syndrome phenotypes: the predictive value of anti-Müllerian hormone. Reprod BioMed Online. (2016) 32:503–12. doi: 10.1016/j.rbmo.2016.01.010
6. Ambekar AS, Kelkar DS, Pinto SM, Sharma R, Hinduja I, Zaveri K, et al. Proteomics of follicular fluid from women with polycystic ovary syndrome suggests molecular defects in follicular development. J Clin Endocrinol Meta. (2015) 100:744–53. doi: 10.1210/jc.2014-2086
7. Domingues TS, Bonetti TC, Pimenta DC, Mariano DOC, Barros B, Aquino AP, et al. Proteomic profile of follicular fluid from patients with polycystic ovary syndrome (PCOS) submitted to in vitro fertilization (IVF) compared to oocyte donors. JBRA Assist Repro. (2019) 23:367–91. doi: 10.5935/1518-0557.20190041
8. Sim YJ, Ryu AR, and Lee MY. Proteomic analysis of human follicular fluid from polycystic ovary syndrome patients. Biotechnol Appl Bioche. (2022) 69:289–95. doi: 10.1002/bab.2108
9. Yin Q, Yan X, Cao Y, and Zheng J. Evaluation of novel obesity- and lipid-related indices as predictors of abnormal glucose tolerance in Chinese women with polycystic ovary syndrome. BMC Endocr Disor. (2022) 22:272. doi: 10.1186/s12902-022-01179-0
10. Zhou Z, Ni R, Hong Y, Li Y, Wang Y, Zhao X, et al. Defining hyperandrogenaemia according to the free androgen index in Chinese women: a cross-sectional study. Clin Endocrinol (Oxf). (2012) 77:446–52. doi: 10.1111/j.1365-2265.2012.04395.x
11. Dumesic DA, Padmanabhan V, and Abbott DH. Polycystic ovary syndrome and oocyte developmental competence. Obstet Gynecol Surv. (2008) 63:39–48. doi: 10.1097/OGX.0b013e31815e85fc
12. Huang R, Zheng J, Li S, Tao T, Ma J, and Liu W. Characteristics and contributions of hyperandrogenism to insulin resistance and other metabolic profiles in polycystic ovary syndrome. Acta Obstet Gynecol Scan. (2015) 94:494–500. doi: 10.1111/aogs.12612
13. Ravisankar S, Murphy MJ, Redmayne-Titley N, Davis B, Luo F, Takahashi D, et al. Long-term hyperandrogenemia and/or western-style diet in rhesus macaque females impairs preimplantation embryogenesis. Endocrinolog. (2022) 163:bqac019. doi: 10.1210/endocr/bqac019
14. Bertoldo MJ, Caldwell ASL, Riepsamen AH, Lin D, Gonzalez MB, Robker RL, et al. A hyperandrogenic environment causes intrinsic defects that are detrimental to follicular dynamics in a PCOS mouse model. Endocrinolog. (2019) 160:699–715. doi: 10.1210/en.2018-00966
15. Chappell NR, Zhou B, Hosseinzadeh P, Schutt A, Gibbons WE, and Blesson CS. Hyperandrogenemia alters mitochondrial structure and function in the oocytes of obese mouse with polycystic ovary syndrome. F S S. (2021) 2:101–12. doi: 10.1016/j.xfss.2020.12.001
16. Zhu Q, Wang Y, Xu L, Shi M, Meng Y, Shao C, et al. Role of SAA1 in endometrial extracellular matrix remodeling in polycystic ovary syndrome: implication for pregnancy loss. J Clin Endocrinol Met. (2025) 110:658–67. doi: 10.1210/clinem/dgae596
17. Zhu Q, Yao Y, Xu L, Wu H, Wang W, He Y, et al. Elevated SAA1 promotes the development of insulin resistance in ovarian granulosa cells in polycystic ovary syndrome. Reprod Biol Endocrin. (2022) 20:4. doi: 10.1186/s12958-021-00873-3
18. Özdemir Başer Ö, Göçmen AY, and Aydoğan Kırmızı D. The role of inflammation, oxidation and Cystatin-C in the pathophysiology of polycystic ovary syndrome. Turk J Obstet Gyneco. (2022) 19:229–35. doi: 10.4274/tjod.galenos.2022.29498
19. Ranjbaran J, Farimani M, Tavilani H, Ghorbani M, Karimi J, Poormonsefi F, et al. Matrix metalloproteinases 2 and 9 and MMP9/NGAL complex activity in women with PCOS. Reproductio. (2016) 151:305–11. doi: 10.1530/rep-15-0340
20. Chen Z, Wei H, Zhao X, Xin X, Peng L, Ning Y, et al. Metformin treatment alleviates polycystic ovary syndrome by decreasing the expression of MMP-2 and MMP-9 via H19/miR-29b-3p and AKT/mTOR/autophagy signaling pathways. J Cell Physio. (2019) 234:19964–76. doi: 10.1002/jcp.28594
21. Duan C and Allard JB. Insulin-like growth factor binding protein-5 in physiology and disease. Front Endocrinol (Lausanne). (2020) 11:100. doi: 10.3389/fendo.2020.00100
22. López-Bermejo A, Khosravi J, Fernández-Real JM, Hwa V, Pratt KL, Casamitjana R, et al. Insulin resistance is associated with increased serum concentration of IGF-binding protein-related protein 1 (IGFBP-rP1/MAC25). Diabetes. (2006) 55:2333–9. doi: 10.2337/db05-1627
23. Lee JH, Kim DY, Pantha R, Lee EH, Bae JH, Han E, et al. Identification of pre-diabetic biomarkers in the progression of diabetes mellitus. Biomedicines. (2021) 10:72. doi: 10.3390/biomedicines10010072
24. Li D, You Y, Bi FF, Zhang TN, Jiao J, Wang TR, et al. Autophagy is activated in the ovarian tissue of polycystic ovary syndrome. Reproduction. (2018) 155:85–92. doi: 10.1530/rep-17-0499
25. Xu B, Dai W, Liu L, Han H, Zhang J, Du X, et al. Metformin ameliorates polycystic ovary syndrome in a rat model by decreasing excessive autophagy in ovarian granulosa cells via the PI3K/AKT/mTOR pathway. Endocr. (2022) 69:863–75. doi: 10.1507/endocrj.EJ21-0480
26. Zhu Q, Zhou H, Zhang A, Gao R, Yang S, Zhao C, et al. Serum LBP is associated with insulin resistance in women with PCOS. PloS On. (2016) 11:e0145337. doi: 10.1371/journal.pone.0145337
27. Sekulic M, Giaglis S, Chatelain N, Bodmer D, and Petkovic V. Neutrophil extracellular traps affect human inner ear vascular permeability. Int J Mol Sc. (2024) 25:9766. doi: 10.3390/ijms25189766
28. Zhang Y, Fatima M, Hou S, Bai L, Zhao S, and Liu E. Research methods for animal models of atherosclerosis (Review). Mol Med Re. (2021) 24:871. doi: 10.3892/mmr.2021.12511
29. Zheng J, Zhang K, Li Z, Worgall TS, and Jiang XC. PLTP deficiency-mediated atherosclerosis regression could be related to sphinogosine-1-phosphate reduction. Atherosclerosis. (2022) 356:53–5. doi: 10.1016/j.atherosclerosis.2022.07.007
30. Lebrun LJ, Pallot G, Nguyen M, Tavernier A, Dusuel A, Pilot T, et al. Increased weight gain and insulin resistance in HF-fed PLTP deficient mice is related to altered inflammatory response and plasma transport of gut-derived LPS. Int J Mol Sc. (2022) 23:13226. doi: 10.3390/ijms232113226
31. Riemens SC, van Tol A, Sluiter WJ, and Dullaart RP. Plasma phospholipid transfer protein activity is lowered by 24-h insulin and acipimox administration: blunted response to insulin in type 2 diabetic patients. Diabetes. (1999) 48:1631–7. doi: 10.2337/diabetes.48.8.1631
32. Van Tol A, Ligtenberg JJ, Riemens SC, van Haeften TW, Reitsma WD, and Dullaart RP. Lowering of plasma phospholipid transfer protein activity by acute hyperglycaemia-induced hyperinsulinaemia in healthy men. Scand J Clin Lab Invest. (1997) 57:147–57. doi: 10.1080/00365519709056383
33. Sponton CH, Hosono T, Taura J, Jedrychowski MP, Yoneshiro T, Wang Q, et al. The regulation of glucose and lipid homeostasis via PLTP as a mediator of BAT-liver communication. EMBO Re. (2020) 21:e49828. doi: 10.15252/embr.201949828
34. Kumtornrut C, Yamauchi T, Koike S, Aiba S, and Yamasaki K. Androgens modulate keratinocyte differentiation indirectly through enhancing growth factor production from dermal fibroblasts. J Dermatol Sc. (2019) 93:150–8. doi: 10.1016/j.jdermsci.2019.01.007
35. Quartier A, Chatrousse L, Redin C, Keime C, Haumesser N, Maglott-Roth A, et al. Genes and pathways regulated by androgens in human neural cells, potential candidates for the male excess in autism spectrum disorder. Biol Psychiatr. (2018) 84:239–52. doi: 10.1016/j.biopsych.2018.01.002
36. Xing Y, Liu YX, Liu X, Wang SL, Li P, Lin XH, et al. Effects of Gui Zhu Yi Kun formula on the P53/AMPK pathway of autophagy in granulosa cells of rats with polycystic ovary syndrome. Exp Ther Me. (2017) 13:3567–73. doi: 10.3892/etm.2017.4384
37. Yi S, Zheng B, Zhu Y, Cai Y, Sun H, and Zhou J. Melatonin ameliorates excessive PINK1/Parkin-mediated mitophagy by enhancing SIRT1 expression in granulosa cells of PCOS. Am J Physiol Endocrinol Meta. (2020) 319:E91–e101. doi: 10.1152/ajpendo.00006.2020
38. Corrales JJ, Almeida M, Martín-Martín L, Miralles JM, and Orfao A. Testosterone replacement therapy in hypogonadal men is associated with increased expression of LAMP-2 (CD107b) by circulating monocytes and dendritic cells. Clin Endocrinol (Oxf). (2014) 80:577–84. doi: 10.1111/cen.12338
39. Liu Y, Xu YC, Cui YG, Jiang SW, Diao FY, Liu JY, et al. Androgen excess increases food intake in a rat polycystic ovary syndrome model by downregulating hypothalamus insulin and leptin signaling pathways preceding weight gain. Neuroendocrinology. (2022) 112:966–81. doi: 10.1159/000521236
40. Su B, Zhang QY, Li XS, Yu HM, Li P, Ma JH, et al. The expression of mimecan in adrenal tissue plays a role in an organism’s responses to stress. Aging (Albany NY). (2021) 13:13087–107. doi: 10.18632/aging.202991
41. Zhang X, Xu X, Li P, Zhou F, Kong L, Qiu J, et al. TMT based proteomic analysis of human follicular fluid from overweight/obese and normal-weight patients with polycystic ovary syndrome. Front Endocrinol (Lausanne). (2019) 10:821. doi: 10.3389/fendo.2019.00821
42. Gaberšček S, Zaletel K, Schwetz V, Pieber T, Obermayer-Pietsch B, and Lerchbaum E. Mechanisms in endocrinology: thyroid and polycystic ovary syndrome. Eur J Endocrino. (2015) 172:R9–21. doi: 10.1530/eje-14-0295
43. Ascani A, Torstensson S, Risal S, Lu H, Eriksson G, Li C, et al. The role of B cells in immune cell activation in polycystic ovary syndrome. Elife. (2023) 12:e86454. doi: 10.7554/eLife.86454
44. Kulus M, Kranc W, Sujka-Kordowska P, Mozdziak P, Jankowski M, Konwerska A, et al. The processes of cellular growth, aging, and programmed cell death are involved in lifespan of ovarian granulosa cells during short-term IVC - Study based on animal model. Theriogenology. (2020) 148:76–88. doi: 10.1016/j.theriogenology.2020.02.044
45. Nicholas B, Alberio R, Fouladi-Nashta AA, and Webb R. Relationship between low-molecular-weight insulin-like growth factor-binding proteins, caspase-3 activity, and oocyte quality. Biol Repro. (2005) 72:796–804. doi: 10.1095/biolreprod.104.036087
46. Peng W, Liu C, Chen D, Duan X, and Zhong L. Exposure to N-(1,3-dimethylbutyl)-N’-phenyl-p-phenylenediamine (6PPD) affects the growth and development of zebrafish embryos/larvae. Ecotoxicol Environ Saf. (2022) 232:113221. doi: 10.1016/j.ecoenv.2022.113221
47. Zhong L, Peng W, Liu C, Gao L, Chen D, and Duan X. IPPD-induced growth inhibition and its mechanism in zebrafish. Ecotoxicol Environ Saf. (2022) 239:113614. doi: 10.1016/j.ecoenv.2022.113614
48. Hu LL, Chen S, Shen MY, Huang QY, Li HG, Sun SC, et al. Aflatoxin B1 impairs porcine oocyte quality via disturbing intracellular membrane system and ATP production. Ecotoxicol Environ Saf. (2023) 263:115213. doi: 10.1016/j.ecoenv.2023.115213
49. Lee SH, Sun MH, Zhou D, Jiang WJ, Li XH, Heo G, et al. High temperature disrupts organelle distribution and functions affecting meiotic maturation in porcine oocytes. Front Cell Dev Bio. (2022) 10:826801. doi: 10.3389/fcell.2022.826801
50. Hu LL, Li HG, Li XM, Xu Y, Pang YQ, Wang B, et al. Nonylphenol exposure-induced oocyte quality deterioration could be reversed by melatonin supplementation in mice. Environ Pollu. (2022) 305:119317. doi: 10.1016/j.envpol.2022.119317
Keywords: polycystic ovary syndrome, hyperandrogenism, oocyte, follicular fluid, proteomics
Citation: Yin Q, Zheng J, Cao Y, Yan X and Zhang H (2025) Proteomic analysis of human follicular fluid based on the 4D label free method to identify proteins that may affect oocyte quality in hyperandrogenic PCOS patients. Front. Endocrinol. 16:1579469. doi: 10.3389/fendo.2025.1579469
Received: 19 February 2025; Accepted: 24 April 2025;
Published: 15 May 2025.
Edited by:
Duan Xing, Southeast University, ChinaReviewed by:
Chenyun Miao, Zhejiang Chinese Medical University, ChinaQingrui Zhuan, Air Force General Hospital PLA, China
Xia Zhao, Southeast University, China
Copyright © 2025 Yin, Zheng, Cao, Yan and Zhang. This is an open-access article distributed under the terms of the Creative Commons Attribution License (CC BY). The use, distribution or reproduction in other forums is permitted, provided the original author(s) and the copyright owner(s) are credited and that the original publication in this journal is cited, in accordance with accepted academic practice. No use, distribution or reproduction is permitted which does not comply with these terms.
*Correspondence: Hong Zhang, emhhbmdob25neXFxQDE2My5jb20=; Xiaonan Yan, eWFueGlhb25hbkAxMjYuY29t
†These authors have contributed equally to this work and share first authorship
‡ORCID: Qianqian Yin, orcid.org/0000-0002-9185-7004
Xiaonan Yan, orcid.org/0000-0001-8886-7423
Hong Zhang, orcid.org/0000-0003-4943-1859