- 1Guang’anmen Hospital, China Academy of Chinese Medical Sciences, Beijing, China
- 2Beijing University of Chinese Medicine, Beijing, China
- 3College of Traditional Chinese Medicine, Changchun University of Traditional Chinese Medicine, Changchun, China
The global pandemic caused by the SARS-CoV-2 virus has had a profound impact on the onset, progression, and management of diabetes, posing significant challenges to healthcare systems worldwide. This review elucidates the multifaceted impact of SARS-CoV-2 on diabetes mellitus, emphasizing the increased complexity of glycemic management in patients with SARS-CoV-2 infection following viral infection in the postpandemic era. In this study, we examined the diverse effects of the SARS-CoV-2 virus on individuals with diabetes. These effects included an elevated risk of morbidity, erratic fluctuations in blood glucose levels, the emergence of complications associated with diabetes, and the emergence of challenges related to self-management of the disease. From a mechanistic perspective, we investigated the following factors: SARS-CoV-2-mediated direct damage to islet beta cells, dysregulation of the RAAS system, impairment of islet function by oxidative stress, and the effects of the integrated stress response, stress response, and reduced adiponectin levels on insulin utilization efficiency and glucose metabolism. Furthermore, viral effects extend to diabetic complications and cardiovascular risk factors, such as coagulation abnormalities, hypertension, and lipid metabolism. This results in an exacerbation of the development of diabetic complications. This review highlights the urgent need for refined management strategies for patients with diabetes during the pandemic and in the later stages of COVID-19. Additionally, there is a need for integrated management strategies to mitigate the impact of COVID-19 on the long-term outcomes of patients with diabetes.
1 Introduction
Although the acute threat of the coronavirus disease 2019 (COVID-19) pandemic has diminished, its shadow lingers in the form of post-acute sequelae (PASC), posing unprecedented challenges to chronic disease management—particularly for diabetes mellitus (DM), a metabolic disorder characterized by chronic hyperglycemia and multisystem comorbidities (1). DM has a significant impact on the quality of life and overall health of patients and places a burden on national healthcare resources. The bidirectional relationship between severe acute respiratory syndrome coronavirus 2 (SARS-CoV-2) and diabetes remains alarming: while diabetic patients face elevated risks of severe COVID-19 outcomes (2), emerging evidence implicates the virus as a catalyst for new-onset diabetes and glycemic destabilization (3). This includes an increased risk of morbidity, fluctuating blood glucose levels, an increased likelihood of diabetic complications, and self-management challenges, which will be described in greater detail later. This review delves into several key aspects of its mechanism, including the direct effects of the virus on pancreatic beta cells, as well as the broader metabolic dysregulation associated with infection. A meta-analysis of 11 retrospective cohorts spanning 47.1 million subjects globally underscores this peril, revealing a 64% increased risk of incident diabetes post-COVID-19 (4). As global healthcare systems are now coping with the long-term complexities of the aftermath of COVID-19, there is a pressing need to investigate the mechanisms through which the virus affects DM from various perspectives and to develop a comprehensive management strategy to address the distinctive challenges encountered by individuals with DM. The objective of this review is to provide a comprehensive examination of the complex interrelationship between SARS-CoV-2 and DM, emphasizing the multifaceted influence of viral infection on glycemic control and DM management.
2 Impact of COVID-19 on the development and clinical management of DM
2.1 COVID-19 increased the incidence of DM
2.1.1 The impact of SARS-CoV-2 infection on the incidence of DM
There is a bidirectional relationship between SARS-CoV-2 infection and DM. On the one hand, diabetic patients tend to develop more severe clinical symptoms after contracting COVID-19. Conversely, inadequate glycemic control in individuals with newly diagnosed DM and in those with preexisting DM has also been reported in SARS-CoV-2-infected individuals, which may be one of the complications of COVID-19 (5, 6). The varying degrees of glucose intolerance that patients develop after COVID-19 are referred to as ‘long COVID’ or ‘post-COVID syndrome’ (7). Statistical analysis indicated that the risk of developing DM may be increased by 60% in individuals infected with SARS-CoV-2. However, the incidence of DM is greater than that of respiratory infections caused by other viruses (8). The glycemic abnormalities caused by COVID-19 include both type 1 DM (T1DM) and type 2 DM (T2DM). Consequently, it is imperative to prioritize the investigation of T1DM in pediatric populations (9). A meta-analysis of 11 retrospective cohorts (47.1 million participants) revealed that patients with COVID-19 had a 64% greater risk of developing DM (RR = 1.64, 95% CI: 1.51–1.79), including both T1DM and T2DM, than non-COVID-19 controls did (4). In addition, a prospective cohort study of 181,280 infected individuals over 352 days revealed an increased risk (HR 1.40, 95% CI 1.36 - 1.44) and excess burden (13.46, 95% CI 12.11 - 14.84, per 1000 people at 12 months) of DM in COVID-19 patients compared with controls (10).
COVID-19 is characterized by the overproduction of inflammatory factors such as interleukins (ILs), interferons (IFNs), chemokines and tumor necrosis factors (TNFs). Viral infection leads to overactivation of immune cells and the production of proinflammatory cytokines, known as a cytokine storm, which further leads to severe COVID-19 symptoms and patient death (11). In addition, this inflammatory storm damages organs throughout the body (including major glucose-metabolizing organs) and increases insulin resistance. The pancreas is an important target for SARS-CoV-2 because angiotensin-converting enzyme 2 (ACE2) is expressed in both exocrine glands and pancreatic islets (12), and inflammation of pancreatic beta cells in COVID-19 patients also contributes to new-onset DM (13). These possible reasons for the increased incidence of DM after SARS-CoV-2 infection will be discussed in more detail later.
2.1.2 The impact of COVID-19 treatment on the incidence of DM
In addition to the damage to glucose metabolism caused by viral infection, treatment for COVID-19 can also cause blood glucose abnormalities. Diabetic drugs such as corticosteroids, which are often used in patients with severe COVID-19, are major causes of DM (14). Dexamethasone reduces mortality and the length of hospital stay in patients with severe COVID-19 (15), but it increases blood glucose levels, making it not recommended for patients with mild COVID-19 and DM (16). In addition to dexamethasone, some studies have suggested that the antiviral drug Remdesivir also leads to high blood glucose levels, which should be taken into account when caring for patients with both DM and COVID-19 (17). Lifestyle changes during the COVID-19 pandemic may also contribute to DM. During the COVID-19 epidemic, the population adopted unhealthy diets and reduced physical activity as a result of widespread confinement. These lifestyle changes contribute to obesity, exacerbating insulin resistance, which may accelerate the progression to T2DM in individuals with preexisting β-cell dysfunction (18). This increase in the prevalence of obesity during the COVID-19 pandemic has also been reported in other studies (12). Notably, just 2 weeks of reduced daily physical activity in healthy young adults resulted in decreased muscle mass, increased visceral fat mass, insulin resistance and increased plasma triglyceride levels (19).
2.2 COVID-19 affects glucose levels in diabetic patients
COVID-19 itself is thought to trigger new-onset diabetes and hyperglycemia but also to worsen glycemic control in patients with pre-existing diabetes. COVID-19 is not uncommon in patients with established diabetes mellitus in the context of the SARS-CoV-2 pandemic (14). Poor glycemic control was observed in both T1DM and T2DM patients after SARS-CoV-2 infection, and the reasons for these two conditions were different. The former is attributed to a cytokine storm caused by the abnormal release of all kinds of hormones and various proinflammatory cytokines, and the latter may be due to the massive destruction of pancreatic beta cells by the virus (20). People with DM after COVID-19 experience both hyperglycemia and blood glucose fluctuations.
2.2.1 COVID-19 causes elevated blood glucose in patients with DM
Following infection with SARS-CoV-2, the onset of hyperglycemia may precede symptoms of the disease itself (20). A study of 29 patients with T2DM reported that 12% of diabetic patients experienced frequent hyperglycemia after infection (21). This was associated with higher rates of hospitalization and mortality (22). The presence of corticosteroids, cytokine storms, damage to pancreatic beta cells, or a combination of these factors has been demonstrated to result in more severe hyperglycemia and increased ketoacidosis in individuals with DM (23). In addition, the strategies implemented by governments during the pandemic also contributed to elevated blood glucose levels. The results of a systematic review and meta-analysis demonstrated that the implementation of a lockdown due to the pandemic resulted in statistically significant increases in HbA1c, fasting glucose, and body mass index in patients with T2DM (p < 0.05) (24).
2.2.2 COVID-19 causes fluctuations in blood glucose in patients with DM
In the management of blood glucose in individuals with DM, preventing hyperglycemia and maintaining a stable blood glucose level are equally important. Hypoglycemia is a common complication of DM and is more likely to result in adverse clinical outcomes, including mortality, than is hyperglycemia. Typically, hypoglycemia is attributed to substance abuse (especially insulin use) and poor dietary management. However, this effect appears to be intensified in critically ill patients in the intensive care unit (ICU) (25). The majority of available evidence indicates that the severity and mortality of SARS-CoV-2 infection are greater in individuals with DM, particularly when accompanied by fluctuations in blood glucose levels (26). Notably, some studies have reported a correlation between insulin use and mortality in hospitalized patients (27). Hypoglycemia incidence is elevated in hospitalized COVID-19 patients with diabetes, likely due to erratic oral intake, altered drug metabolism, and cytokine-mediated insulin resistance (28).
2.3 The impact of COVID-19 on the development of diabetic complications
Following a 12-week period during which the patient has been infected with SARS-CoV-2, a number of clinical symptoms may still be observed, including fatigue, dyspnea, myalgia, weakness, headache and cognitive retardation. These symptoms are collectively known as post-COVID-19 syndrome. The incidence of microvascular dysfunction and organ damage is increased in individuals with DM following a diagnosis of SARS-CoV-2 infection. Combined with hospitalization, protein deficiency, and corticosteroid therapy, there is also a loss of muscle mass and increased fatigue, especially in the elderly and women (29). Furthermore, the incidence of complications has been shown to be elevated in individuals with DM following a diagnosis of SARS-CoV-2 infection (30).
2.3.1 Cardiovascular and cerebrovascular complications
The SARS-CoV-2 virus can accelerate the development of T2DM, which in turn can lead to long-term complications of T2DM (31). SARS-CoV-2 infection has been demonstrated to cause damage to vascular endothelial cells, thus increasing the risk of cardiovascular and cerebrovascular disease among diabetic patients. Compared with cardiovascular and cerebrovascular complications caused by DM alone, these complications are more likely to occur with the additional presence of SARS-CoV-2 infection (12). In patients with DM and concomitant infection with the SARS-CoV-2 virus, hyperglycemia, hypoxia and proinflammatory environments lead to vascular endothelial dysfunction and thrombosis, thereby increasing the risk of cardiovascular and cerebrovascular complications (32). Patients with basal metabolic dysfunction, such as T2DM and obesity, are at increased risk of developing complications from SARS-CoV-2 infection, including multiple organ dysfunction and cellular energy deprivation secondary to immune response disorders (33). At present, the long-term effects of COVID-19 on cardiovascular and cerebrovascular complications remain unclear because of the absence of high-quality clinical studies.
2.3.2 Chronic complications of DM
The chronic complications of DM include diabetic retinopathy (DR), diabetic nephropathy, diabetic peripheral neuropathy (DPN) and diabetic foot. While case reports suggest potential associations between SARS-CoV-2 infection and worsening microvascular complications, robust evidence linking direct viral mechanisms to retinopathy, nephropathy, or neuropathy remains limited. The observed increases in complications may reflect pandemic-related disruptions in care, psychological stress, or indirect metabolic dysregulation rather than direct viral effects. The monitoring and treatment of DR during the current pandemic are facing significant challenges. During the pandemic, there has been a notable decline in the administration of intravitreal injections for DR worldwide, with reductions ranging from approximately 30% to nearly 100% (34). To date, no research has been conducted to confirm the effect of the SARS-CoV-2 virus on the development of DR; however, a number of case reports have mentioned this possibility (35, 36). A five-year retrospective study revealed a notable surge in the incidence and severity of DR following the onset of the pandemic. The authors posit that this may be related to the psychological impact of the pandemic rather than the direct effects of the virus itself (37). Furthermore, studies have reported the effects of the SARS-CoV-2 virus on DPN (38), with evidence suggesting that vaccines may contribute to this phenomenon (39). Diabetic nephropathy (40) and diabetic foot (41) have also been mentioned, but these studies lack supporting clinical evidence.
2.3.3 Acute complications of DM
Individuals with diabetes infected with the SARS-CoV-2 are more susceptible to developing severe acute complications of DMI, including diabetic ketoacidosis and hyperosmolar hyperglycemia (42). The entry receptor of SARS-CoV-2, ACE2, is expressed in several extrapulmonary tissues and may cause hyperglycemia and ketosis (43). Metformin and sodium–glucose transport protein 2 inhibitors (SGLT2is) may be discontinued in patients with severe COVID-19 because of their association with lactic acidosis and ketoacidosis (20). A study of 658 hospitalized patients with confirmed SARS-CoV-2 infection demonstrated the occurrence of ketosis in 42 patients. Among these patients, three presented with diabetic ketoacidosis, and four died (44).
2.4 COVID-19 affects self-management in diabetic patients
The results of a systemic review show that DM patients who maintained or increased physical activity during the COVID-19 pandemic had lower plasma glucose and HbA1c levels. Some authors suggested that COVID-19 promoted deleterious metabolic adjustments in DM patients and that physical activity followed by exercise would have beneficial effects on insulin sensitivity and lipid metabolism homeostasis (45). The implementation of lockdown measures during an epidemic has adverse effects on the medical treatment and exercise regimens of diabetic patients, which in turn impedes the control of blood glucose levels. During the lockdown, all individuals, including those with T2DM, are permitted only those activities deemed essential. Such a change in lifestyle, including diet, exercise, insulin adjustment, mood, stress, social relationships and work activities, can have a significant impact on both physical and mental health, potentially affecting self-management and glycemic control in individuals with DM (18). Furthermore, for those with DM complications, the ongoing pandemic may also result in delays or even the cancellation of planned treatments (34).
2.5 Impact of COVID-19 on clinical management decisions in diabetic patients
2.5.1 Increased rates of hospitalization and risk of death
Patients with T1DM or T2DM have a markedly elevated risk of severity and mortality from SARS-CoV-2 infection, particularly those with poor glycemic control (26). Reports show that patients with DM concurrently infected with the SARS-CoV-2 virus are at an elevated risk of mortality (46). Existing data indicate that elevated blood glucose levels facilitate local viral replication in the lungs, impair the antiviral immune response, and ultimately result in severe illness in patients with COVID-19 (47). A meta-analysis involving 45,775 hospitalized COVID-19 patients revealed that the weighted prevalence of mortality in hospitalized COVID-19 patients with DM (20.0%, 95% CI: 15.0–26.0) was 82% higher than that in non-DM patients (11.0%, 95% CI: 5.0–16.0) (22).
2.5.2 Drug treatments
Insulin is a key therapy for the treatment of diabetes and is used in patients suffering from severe COVID-19 accompanied by hyperglycemia (14). However, some studies have mentioned an increase in mortality in these patients to whom insulin therapy has been applied (48). A retrospective study of 3,305 cases from Wuhan, China, indicated that insulin treatment for patients with COVID-19 comorbid with T2DM was associated with a significant increase in mortality (27.2% versus 3.5%) (28). Insulin therapy has the potential to cause a hypoglycemic response. While mortality was not reduced in insulin-treated COVID-19 patients even in the absence of hypoglycemia (27). It is important to note that insulin remains the cornerstone therapy for hyperglycemic ICU patients, including those with undiagnosed diabetes mellitus, as it is effective in relieving acute hyperglycemia and ketoacidosis. The association between insulin use and mortality observed in the retrospective study may reflect confounding by indication, as COVID-19 patients to whom insulin is applied are usually critically ill patients with severe hyperglycemia or comorbidities (e.g., cardiovascular disease). Therefore, the reasons for the increased mortality rate may be complex, with increased systemic inflammation and damage to vital organs as potential causes. It is recommended that insulin use be measured and fully evaluated by clinicians.
Other oral hypoglycemic agents have different effects on diabetic patients with COVID-19, and some may be more suitable for diabetic patients with concurrent COVID-19. Current evidence indicates that drugs commonly used for the treatment of DM, including dipeptidyl peptidase 4 (DPP-4) inhibitors, glucagon-like peptide-1 receptor agonists (GLP-1RAs), SGLT-2is and metformin, may influence the progression of SARS-CoV-2 infection. Therefore, it is necessary to determine the efficacy and safety of these agents in the treatment of DM patients with SARS-CoV-2 infection (49). The administration of peroxisome proliferator-activated receptor γ (PPARγ) activators and GLP-1RA has been demonstrated to upregulate ACE2 in animal models, which may increase the risk of SARS-CoV-2 infection (50). Metformin has been proven to reduce lung damage and is therefore regarded as a promising treatment for patients with DM and COVID-19 because of its dual activity (51). DPP-4 inhibitors, such as gliptins, may have the potential to exert a positive pleiotropic effect on inflammatory diseases and could therefore be repurposed as salutary drugs against COVID-19 syndrome, even in nondiabetic subjects (52). SGLT2i has also been suggested to be beneficial for COVID-19 outcomes; however, these potentially beneficial agents remain controversial, with an insufficient number of clinical trials (53). In addition, herbs such as curcumin and propolis may have adjunctive antiviral, anti-inflammatory, insulin-resistance-reducing, and immunomodulatory effects and show promise in assisting the management of COVID-19 and DM comorbidity (54). Specific herbs and traditional formulations have potential benefits in controlling symptoms, regulating blood glucose levels, and improving immune function, but there is insufficient clinical evidence to support their use as stand-alone therapies (55).
2.6 Evaluation and management
During the COVID-19 pandemic, the assessment and management of people with DM has been affected (56). The outbreak had an enormous impact on national health services, particularly on the diagnosis and monitoring of chronic diseases such as DM (57). In the postepidemic era, this impact is also subtly changing the management model for DM patients. During the pandemic, the pace of development of digital services began to increase exponentially. As actual doctor–patient access is limited, telemedicine offers the most convenient opportunity to communicate with and maintain the care of people with DM (58). In fact, telemedicine is the most useful and commonly used tool for maintaining contact between patients and doctors when physical distance is limited by confinement (59), and telemedicine is more appropriate for the assessment and management of chronic diseases such as DM. Nevertheless, there are still significant challenges in telemedicine, such as a fragmented approach to health technology assessment and reimbursement, a lack of eHealth education and literacy, particularly among healthcare professionals, a lack of data integration, and concerns about electronic health records, patient consent and privacy (60). However, such measures are beneficial for the management of people with DM and may prove invaluable in the event of a similar outbreak in the future. Clinical studies on the effects of COVID-19 on DM are summarized in Table 1.
3 Mechanisms of action of SARS-CoV-2 in the etiology of DM
3.1 Impairment of pancreatic islet function leads to insufficient insulin production
3.1.1 Direct damage to pancreatic beta cells caused by SARS-CoV-2, with both acute and sustained effects
New-onset hyperglycemia after SARS-CoV-2 infection may be attributed to the ability of the virus to successfully invade beta cells, abduct their intracellular mechanisms and disrupt their insulin production. SARS-CoV-2 gains entry into beta cells via viral receptors, as well as subsequent damage due to direct acute viral injury or the long-term persistence of uncleared virus. In both instances, SARS-CoV-2 directly induces β-cell dysfunction and death (61, 62).
In vitro studies have demonstrated a notable reduction in the prevalence of SARS-CoV-2 in other pancreatic α and δ cells, as well as in endothelial cells. Conversely, human pancreatic beta cells exhibit heightened vulnerability to SARS-CoV-2 (63). Trajectory analysis demonstrated that SARS-CoV-2 induces eIF2 pathway-mediated beta cell transdifferentiation, resulting in decreased insulin gene expression in beta cells. Concurrently, glucagon and other alpha cells and acinar cell markers are upregulated in beta cells (11, 64). Autopsy samples from COVID-19 patients demonstrated a reduction in insulin expression and the abnormal presence of trypsin/insulin double-positive cells, confirming that SARS-CoV-2 induces such transdifferentiation of beta cells (11). SARS-CoV-2 has been demonstrated to directly induce β-cell killing. The SARS-CoV-2 spike (S) and nucleocapsid (N) proteins were identified following the transduction of islets in vitro (63). The autopsy results of 19 deceased COVID-19 patients demonstrated the presence of SARS-CoV-2 virus infiltration in beta cells in all patients. By immunohistochemistry and immunofluorescence staining of tissue from deceased COVID-19 patients, many viral antigen-positive cells were observed in the pancreatic islets and exocrine tissue (62). Specifically, pseudokinase mixed lineage kinase domain like (pMLKL)-positive cells were detected in a small percentage of pancreatic islet samples from all COVID-19 patients at autopsy. Since SARS-CoV-2 viral infiltration results in pMLKL positivity, this is likely related to the necrotic apoptotic signaling pathway (62). SARS-CoV-2 selectively infects human pancreatic islet beta cells, leading to dysregulation of insulin homeostasis, induction of apoptosis-related signaling pathways, and apoptosis.
However, there is insufficient evidence to support that hyperglycemia in COVID-19 is driven primarily by virus-induced β-cell death or dysfunction, resulting in acute or prolonged insulin deficiency. The direct cytopathic effect on beta cells remains controversial. Moreover, there are no clinical data on pancreatic involvement in patients with mild COVID-19 or evidence of widespread β-cell destruction (9).
3.1.2 SARS-CoV-2 regulates the renin–angiotensin–aldosterone system to affect pancreatic β-cells
The major regulator of the renin-angiotensin system, Angiotensin-Converting Enzyme 2 (ACE2), which is found in large quantities in the epithelia of the lung and small intestine in humans, is the functional receptor for SARS-CoV-2 and acts as an anti-inflammatory enzyme (65, 66). Angiotensin-converting enzyme (ACE) converts angiotensin I (AI) to angiotensin II (AII), and ACE2 cleaves AI and AII to inactive angiotensin 1–9 and angiotensin 1–7, respectively, which have vasodilatory and antifibrotic effects. SARS-CoV binding and downregulation of ACE2 expression may explain some of the uncommon sequelae observed in patients with COVID-19, including glycemic abnormalities. The interaction between the SARS-CoV-2 surface-anchored spike (S) glycoprotein and the membrane ACE2 provides the possibility for viral entry into the host cell, which is initiated via the serine protease transmembrane serine protease 2 (TMPRSS2) for S protein initiation (67, 68). ACE2 mRNA is expressed in the majority of tissues, with particularly high levels observed in both the exocrine and endocrine pancreas. There is a correlation between ACE2 and glucose regulation. ACE2 is highly expressed in pancreatic islet cells, which serve as a point of entry for SARS-CoV-2, and pancreatic beta cells are particularly susceptible to SARS-CoV-2 infection via ACE2. SARS-CoV-2 has been demonstrated to disrupt the equilibrium of ACE/ACE2 homeostasis and RAAS activation. Additionally, the mRNAs that act on ACE2 in the pancreatic endocrine and exocrine glands affect pancreatic function (69, 70). SARS-CoV infection results in acute β-cell dysfunction, leading to acute hyperglycemia and transient T2DM. Over 50% of patients develop DM during the course of their hospitalization for SARS-CoV infection, whereas only 5% remain living with DM after three years of recovery from the viral infection (71, 72).
3.1.3 Oxidative stress caused by SARS-CoV-2 infection damages beta cells
SARS-CoV-2 infection may alter mitochondrial dynamics and affect mitochondrial function at different levels, whereas mitochondria are essential for an adequate innate antiviral immune response, energy metabolism, and reactive oxygen species (ROS) production. Upon entry of SARS-CoV-2, the RNA genome is released and translated, producing structural and nonstructural proteins that interact with mitochondrial components. Subsequently, SARS-CoV-2 evades the mitochondria-mediated innate immune response and establishes an infection (73). SARS-CoV-2 has been demonstrated to induce mitochondrial damage through a number of mechanisms, including depolarization of the mitochondrial membrane, opening of the mitochondrial permeability transition pore and increased ROS release. This phenomenon is particularly evident in the mitochondrial electron transport chain, which may result in mitochondrial oxidative damage (74).
Emerging evidence indicates that COVID-19 hijacks the mitochondria of immune cells, replicates within mitochondrial structures, and disrupts mitochondrial dynamics, causing cell death (75). In addition, prolonged COVID-19 after acute infection leads to mitochondrial dysfunction, redox state imbalance, impaired energy metabolism, and chronic immune dysregulation (75). A total of 4.5% of omicron-infected individuals and 10.8% of delta variant-infected individuals experienced the debilitating syndrome of long COVID-19 (76). Proper mitochondrial function is essential for nutrient sensing and insulin secretion by beta cells. Compared with other cells, pancreatic beta cells are particularly susceptible to oxidative stress with increased DNA damage due to high ROS production and severe deficiencies in antioxidant capacity (77). These findings suggest that oxidative stress attributed to SARS-CoV-2 may play a role in β-cell failure that cannot be ignored.
3.2 Mechanisms by which COVID-19 affects glucose metabolism
In addition to the direct effects of the SARS-CoV-2 virus on the pancreas, the presence of inflammation, an unregulated immune response and stress also affect the host’s systemic metabolism and insulin efficacy, which in turn leads to abnormalities in glycemic levels. A variety of metabolic processes, including glycogenolysis, galactose degradation and glycolysis, are inhibited in SARS-CoV-2-infected individuals as a result of pathway enrichment analysis across tissues (75).
3.2.1 Insulin resistance triggered by overactivated inflammation
COVID-19 may trigger insulin resistance, leading to chronic metabolic disorders that do not exist prior to infection. Severe SARS-CoV-2 infection and its associated hyperinflammation and cytokine activation affect insulin target tissues (primarily the liver, muscle and adipose tissue), reducing insulin sensitivity and causing hyperglycemia (33, 78). Metabolic inflammation also weakens the immune system, limiting the body’s ability to respond to infection, impeding the healing process, and lengthening the recovery period (33). Moreover, SARS-CoV-2 induces a cytokine storm, an exaggerated immune response with broad-spectrum cytokine production, which creates a systemic aggressive proinflammatory state. Elevated cytokine levels inhibit insulin signaling, leading to the development of insulin resistance and increased infiltration of adipose tissue with macrophages (79). Excessive activation of additional inflammatory variables, such as elevated neutrophil, IL-6, and CRP levels, as well as immune response abnormalities, such as decreases in lymphocyte, monocyte, CD4+, and CD8+ T-cell counts, results in insufficient insulin production and systemic insulin resistance (7). These conditions lead to impaired glucose regulation and new-onset DM. A study of the cytokine profiles of patients with acute COVID-19 and post-COVID-19 revealed that the levels of macrophage inflammatory protein-1 beta (MIP-1beta) and TNF were significantly increased in the serum of patients with COVID-19 compared with healthy controls; in the serum of patients who recovered from COVID-19, the levels of IL-1beta, IL-2, IL-4, IL-7, IL-8, IL-10, IL-13, IL-17, granulocyte colony stimulating factor (G-CSF) and IFN-γ were also increased (80, 81). Overall, inflammatory scores are increased in patients with COVID-19 and in patients recovering from COVID-19, and inflammatory scores are correlated with HOMA-IR (80). The synergistic effect between COVID-19 and T2DM may amplify the inflammatory response and further downregulate the interferon response, which may lead to greater symptomatic severity and poorer glycemic control in these patients (82).
3.2.2 The integrated stress response attenuates insulin efficacy
The ISR is a central regulatory network of signals induced upon dysregulation of protein homeostasis and is capable of integrating various intracellular stresses, including dysregulation of protein homeostasis, nutritional deficiencies, viral infections, and redox imbalances (83). Viral infection triggers ISR, and its impact on metabolism-related diseases cannot be underestimated. The SARS-CoV-2 RNA fragment activates protein kinase R (PKR), which phosphorylates insulin receptor substrate 1 (IRS-1) serine, resulting in insulin resistance. During ISR, at least two of the four serine/threonine kinases activated by various stressors, PKR and PERK, can downregulate insulin receptor substrate serine phosphorylation via the insulin signaling pathway, reducing insulin action (78).
3.2.3 Stress response
Similar to other viruses, SARS-CoV-2 infection may induce a stress response that reduces insulin production, releases counterregulatory hormones, including cortisol and adrenaline, induces excessive gluconeogenesis and impairs glucose disposal, thereby causing transient hyperglycemia (84–86). Although these mechanisms may not necessarily cause DM, they should be evaluated. In acute disease, cortisol, adrenaline and glucagon are released as a stress response to stimulate gluconeogenesis in the liver, resulting in transient hyperglycemia. Hyperglycemia induced by these processes may even lead to glucotoxicity in beta cells, which further reduces insulin secretory function (86, 87). In addition, high doses of steroids and inflammatory cytokines impair insulin secretion (88).
3.2.4 Insulin resistance and reduced adiponectin levels
Obesity, metabolic syndrome, and T2DM have all been linked to dysfunctional adipokine production. Adipose tissue regulates beta cell function, mass, and insulin sensitivity in peripheral tissues (89). The lipocalin-leptin ratio is a biomarker of metabolic health and adiposity function and was found to be severely lower in the serum lipocalin group than in the ARDS-positive group. Adipsin, a hormone known to promote β-cell survival and insulin secretion, is also reduced in patients with COVID-19 (90). Therefore, it was hypothesized that COVID-19 may contribute to the development of insulin resistance and hyperglycemia by causing adipocyte dysfunction (78, 91).
3.2.5 Mechanisms by which therapeutic agents for COVID-19 affect blood glucose
Glucocorticoids have anti-inflammatory and immunomodulatory effects, while disruption of glucose metabolism when applied for long periods of time or in large amounts is a common side effect (92). Dexamethasone, a long-acting glucocorticoid, remains the most effective treatment for patients with severe SARS-CoV-2 infection requiring oxygen therapy or ventilation. However, glucocorticoids increase hepatic glucose production and inhibit peripheral glucose uptake by activating glucocorticoid receptors in multiple tissues, leading to interorgan crosstalk and inducing the expression of hepatic gluconeogenic genes (93). In addition, dexamethasone induces the apoptosis of pancreatic beta cells by upregulating the expression of the TNF-related apoptosis-inducing ligand (TRAIL) pathway and TRAIL death receptor (94). Among the 254 nondiabetic patients receiving dexamethasone for COVID-19, approximately half developed hyperglycemia (95). In particular, the risk of hyperglycemia is increased in patients receiving high doses (12–24 mg per day) of dexamethasone (96). The mechanism of action of SARS-CoV-2 in causing DM is shown in Figure 1.
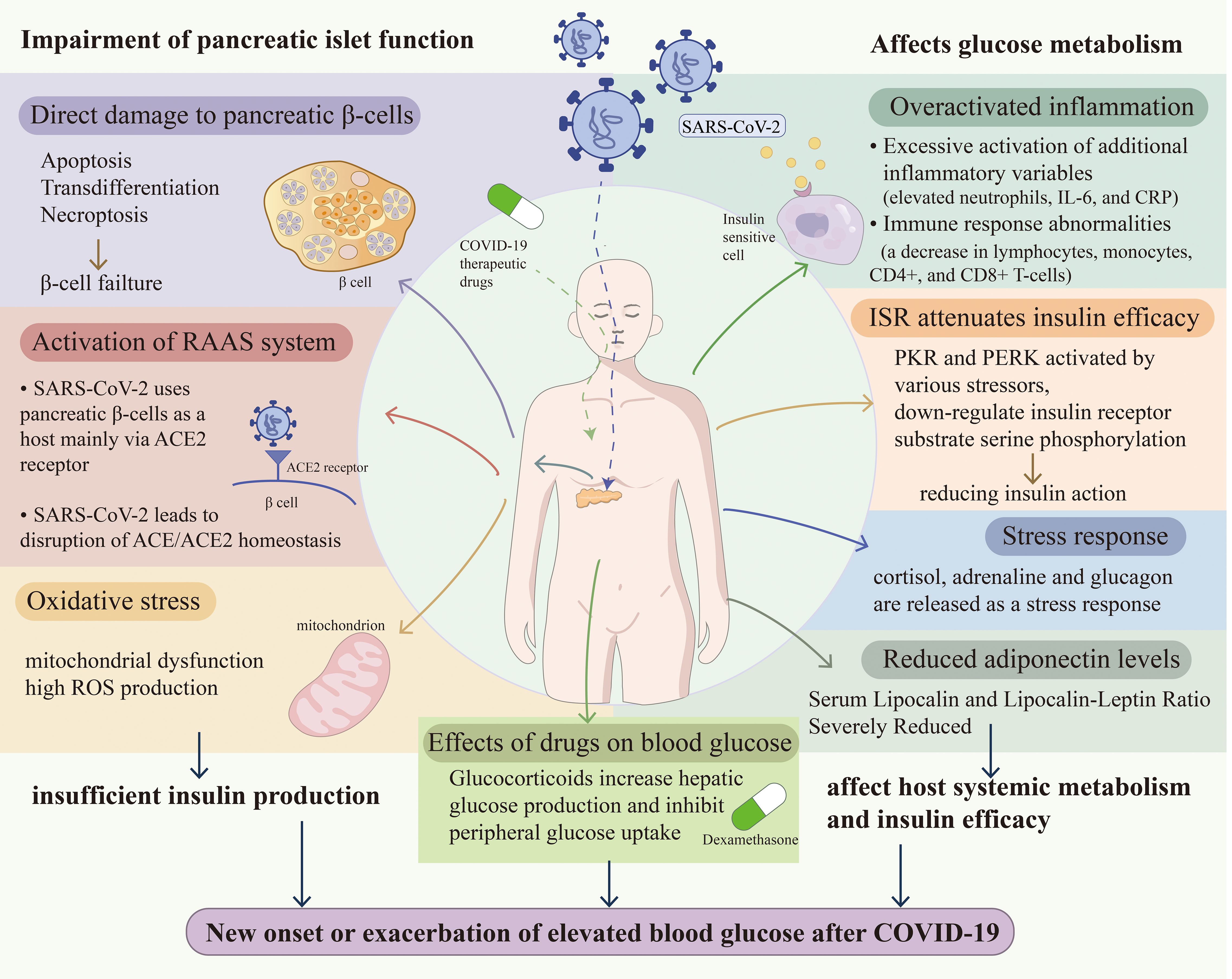
Figure 1. Demonstrates the mechanism by which COVID-19 contributes to poor glycemic control in new-onset patients and exacerbates DM by affecting pancreatic islet function and glucose metabolism.
3.3 Impact of COVID-19 on cardiovascular risk factors and complications of DM
3.3.1 Impact of COVID-19 on cardiovascular risk factors
3.3.1.1 Effect of COVID-19 on coagulation function
There are systemic dysregulations of coagulation, angiogenesis, and fibrosis in COVID-19 patients. Evidence suggests that COVID-19 infection significantly increases the likelihood of thromboembolic events, which are the leading cause of death (97, 98). The first evidence of abnormalities in coagulation parameters associated with COVID-19 infection appeared in early reports from China, as follows. The baseline characteristics of the first 99 patients hospitalized in Wuhan revealed that activated partial thromboplastin kinase time was elevated in 6% of patients, plasminogen time was elevated in 5%, and D-dimer was elevated in 36% (99). The results of another study demonstrated that patients who died from SARS-CoV-2 infection presented significantly elevated levels of D-dimer and fibrin degradation products. In this study, which included 183 Chinese patients with COVID-19 infection, 71.4% of the deaths met the criteria for diffuse intravascular coagulation, whereas only 0.6% of the survivors died (100). Notably, the incidence of venous thromboembolism is greater in patients diagnosed with SARS-CoV-2 infection (101). The long-term consequences of a fibrotic/thrombotic pancreas may also indirectly result in beta cell dysfunction, which could lead to the development of late-onset DM in COVID-19 patients (102). The mechanism behind this may be that SARS-CoV-2 infection induces endothelial injury and systemic inflammation, leading to the release of prothrombotic cytokines (e.g., IL-6, TNF-α). These cytokines upregulate tissue factor expression, activate platelets, and inhibit fibrinolysis via increased plasminogen activator inhibitor-1 (PAI-1), collectively predisposing to a hypercoagulable state (103, 104) Recent studies have found that fibrin binds to the SARS-CoV-2 spike protein, forming a pro-inflammatory clot that leads to systemic thromboinflammation in COVID-19 (105).
3.3.1.2 COVID-19-related hypertension
COVID-19 elevates patients’ blood pressure, may lead to new-onset hypertension, and worsens blood pressure control in those with preexisting hypertension, which is a risk factor for its associated adverse outcomes (106, 107). A study revealed that COVID-19 patients had significantly higher systolic and diastolic blood pressures at discharge than at admission (106). In the long run, COVID-19 also increases systolic and diastolic blood pressure in nonhospitalized patients, as in a study in which more than one in six patients with new or worsening hypertension was reported at the one-year follow-up after COVID-19 rehabilitation (108). The receptor protein for SARS-CoV-2, ACE2, negatively regulates RAAS activation primarily by converting angiotensin 1 and 2 to angiotensin 1–9 and 1–7, respectively. The increase in blood pressure after COVID-19 may be due to the downregulation of ACE2 as well as the concomitant elevation of prohypertensive angiotensin 2 levels in COVID-19 patients (109). In addition, reduced physical activity, sleep disturbances, unhealthy diets, psychological stress and anxiety states, and a lack of access to healthcare during the pandemic may all be linked to worsening blood pressure regulation (110).
3.3.1.3 Effects of COVID-19 on lipid metabolism
Cellular cholesterol plays a crucial role in SARS-CoV-2 entry and replication. Patients with severe COVID-19 have low levels of cholesterol, high-density lipoprotein and low-density lipoprotein. The rich lipid stores in adipocytes not only promote lipid raft formation on the cell membrane but also promote ACE2 expression, thereby facilitating viral entry. Thus, SARS-CoV-2 enables rapid adipocyte replication and expansion, leading to adipose tissue dysfunction and insulin resistance (90, 111). Metabolomic analyses suggested that patients with a history of SARS-CoV infection continued to exhibit dysregulated lipid metabolism after 12 years. The serum concentrations of free fatty acids, lysophosphatidylcholine, lysophosphatidylethanolamine, and phosphatidylglycerol are significantly greater in patients with a history of SARS-CoV infection than in individuals without a history of infection (112).
3.3.2 Impact of COVID-19 on cardiovascular complications
The ACE2 receptor, which provides a direct infection pathway for SARS-CoV-2, is highly expressed in the heart. Virus-induced inflammatory cell infiltration and the production of proinflammatory cytokines (including monocyte chemoattractant protein-1, IL-1beta; IL-6; and TNF-α) may impair cardiac function; thus, patients hospitalized in the acute phase of infection may present with myocarditis-induced heart damage and elevated serum cardiac troponin and NT-proBNP levels (113, 114). SARS-CoV-2 can cause long-term effects on the cardiovascular system, with cardiovascular symptoms of long COVID-19, such as fatigue, chest pain, tachycardia, and palpitations. After the first 30 days of infection, COVID-19 patients are more susceptible to cardiovascular disease, which includes cerebrovascular disease, cardiac arrhythmias, ischemic and nonischemic heart disease, pericarditis, myocarditis, heart failure, and thromboembolic disease. The risk of cardiovascular disease in patients with SARS-CoV-2 has been shown to increase in the first 30 days of infection (115). Prolonged chronic inflammation and cellular damage prompt fibroblasts to secrete extracellular matrix molecules and collagen, leading to fibrosis. SARS-CoV-2 infection produces acute cardiac injury and pathological responses to viral myocarditis, such as endothelial damage and microthrombosis, which can lead to the development of coagulation dysfunction and increase the risk of coronary intravascular thrombosis (113). In addition, chronic hypoxia caused by COVID-19 and increased pulmonary artery pressure and ventricular strain may further accelerate the development of cardiac injury in patients with neocoronary pneumonia (109, 116).
3.3.3 Effects of COVID-19 on diabetic nephropathy
The effect of ACE2 on chronic kidney disease (CKD) remains controversial. In the case of COVID-19, diabetic patients have an approximately twofold increase in the mean ACE2 mRNA level, which may increase the severity and/or risk of SARS-CoV-2 renal infection (117). In contrast, some believe that ACE2 has the potential to slow the progression of experimental diabetic chronic kidney disease. In patients with AKI, triggering the ACE2/angiotensin (1–7)/MasR axis may be nephroprotective; however, in some cases, it may accelerate kidney injury in CKD and AKI (40, 118). A comparison of the renal histopathology of COVID-19 patients and non-COVID-19 patients revealed that in the renal cortex, the proximal renal tubules exhibited significant acute tubular injury, as evidenced by the absence of the brush border and necrosis of epithelial cells in the lumen of the tubules, and microthrombi were observed in the peritubular capillaries and glomeruli (119).
3.3.4 Microvascular injury due to COVID-19
DM-induced endothelial dysfunction is a key and initiating factor in the development of macrovascular and microvascular complications in DM, with mechanisms associated with decreased NO release, increased oxidative stress, increased production of inflammatory factors, abnormal angiogenesis and impaired endothelial repair (120). In addition to lung infection, severe COVID-19 is associated with systemic microvascular dysfunction, especially acute endothelial injury, venous thrombosis and increased capillary permeability. This is probably related to the proinflammatory cytokine storm caused by SARS-CoV-2 infection (110, 121). Owing to the severe negative effects that DM has on blood vessels at all levels throughout the body, these circulatory impairments may contribute to the development of diabetic complications, including diabetic retinopathy, neuropathy and nephropathy. Landecho et al. investigated new retinal changes in patients hospitalized for COVID-19-associated pneumonia and reported that new retinal changes were observed in 22% of patients 14 days after discharge, as assessed by fundoscopy, optical coherence tomography (OCT) and OCT angiography (122). In addition, the impact of COVID-19 on complications such as diabetic foot and diabetic retinopathy has been associated with untimely screening and insufficient resources for care in the context of epidemic control. While SARS-CoV-2-induced endothelial dysfunction and systemic inflammation may exacerbate microvascular injury, the primary drivers of diabetic microvascular complications—chronic hyperglycemia, advanced glycation end products (AGEs), and oxidative stress—remain central. COVID-19 likely amplifies existing metabolic derangements rather than directly initiating microangiopathy. The effects of COVID-19 on cardiovascular risk factors and diabetic complications are illustrated in Figure 2.
4 Conclusion
In conclusion, the global epidemic of COVID-19 has had a significant effect on the management of DM, with increased morbidity and challenges to glycemic control. The intricate interplay between SARS-CoV-2 and DM highlights the urgent need for in-depth mechanistic investigations and customized management strategies. Despite the recent observation that the symptoms of SARS-CoV-2 infection are becoming less severe, the long-term consequences of the disease, also known as long-term COVID-19, are gradually becoming more apparent and attracting attention. Furthermore, the issue of glycemic management after infection by SARS-CoV-2 continues to present a challenge for clinical practitioners. In the future, research efforts should focus on elucidating the mechanisms by which long-term COVID-19 affects blood glucose levels and the long-term impact on DM prognosis. Additionally, innovative, personalized therapeutic protocols for these patients are needed.
Author contributions
XZ: Writing – review & editing, Formal analysis, Resources, Writing – original draft, Visualization. LJ: Visualization, Resources, Writing – review & editing, Writing – original draft. WS: Writing – review & editing, Methodology, Validation. ST: Investigation, Validation, Writing – review & editing, Data curation. XK: Validation, Data curation, Formal analysis, Writing – review & editing. QG: Writing – review & editing, Methodology. ZL: Methodology, Writing – review & editing, Data curation. XA: Conceptualization, Validation, Funding acquisition, Supervision, Writing – review & editing. FL: Conceptualization, Funding acquisition, Supervision, Writing – review & editing.
Funding
The author(s) declare that financial support was received for the research and/or publication of this article. This study is sponsored by the National Natural Science Foundation of China (Project No. T2341018), the Science and Technology Innovation Project of the Chinese Academy of Traditional Chinese Medicine (Project No. CI2023C049YLL), the National Key Research and Development Programme (Project No. 2020YFC0845000) and the Escorting Project of Guang’anmen Hospital, China Academy of Traditional Chinese Medicine (Project No. GAMHH9324001).
Conflict of interest
The authors declare that the research was conducted in the absence of any commercial or financial relationships that could be construed as a potential conflict of interest.
Generative AI statement
The author(s) declare that no Generative AI was used in the creation of this manuscript.
Publisher’s note
All claims expressed in this article are solely those of the authors and do not necessarily represent those of their affiliated organizations, or those of the publisher, the editors and the reviewers. Any product that may be evaluated in this article, or claim that may be made by its manufacturer, is not guaranteed or endorsed by the publisher.
References
1. American Diabetes Association. Diagnosis and classification of diabetes mellitus. Diabetes Care. (2013) 36:S67–74. doi: 10.2337/dc13-S067
2. Kandinata SG, Soelistijo SA, Pranoto A, and Triyono EA. Random blood glucose, but not hbA1c, was associated with mortality in COVID-19 patients with type 2 diabetes mellitus-A retrospective study. Pathophysiology. (2023) 30:136–43. doi: 10.3390/pathophysiology30020012
3. Khunti K, Del Prato S, Mathieu C, Kahn SE, Gabbay RA, and Buse JB. COVID-19, hyperglycemia, and new-onset diabetes. Diabetes Care. (2021) 44:2645–55. doi: 10.2337/dc21-1318
4. Lai H, Yang M, Sun M, Pan B, Wang Q, Wang J, et al. Risk of incident diabetes after COVID-19 infection: A systematic review and meta-analysis. Metabolism. (2022) 137:155330. doi: 10.1016/j.metabol.2022.155330
5. Rubino F, Amiel SA, Zimmet P, Alberti G, Bornstein S, Eckel RH, et al. New-onset diabetes in covid-19. N Engl J Med. (2020) 383:789–90. doi: 10.1056/NEJMc2018688
6. Harding JL, Oviedo SA, Ali MK, Ofotokun I, Gander JC, Patel SA, et al. The bidirectional association between diabetes and long-COVID-19 - A systematic review. Diabetes Res Clin Pract. (2023) 195:110202. doi: 10.1016/j.diabres.2022.110202
7. Rizvi AA, Kathuria A, Al Mahmeed W, Al-Rasadi K, Al-Alawi K, Banach M, et al. Post-COVID syndrome, inflammation, and diabetes. J Diabetes Complic. (2022) 36:108336. doi: 10.1016/j.jdiacomp.2022.108336
8. Wong R, Lam E, Bramante CT, Johnson SG, Reusch J, Wilkins KJ, et al. Does COVID-19 infection increase the risk of diabetes? Current evidence. Curr Diabetes Rep. (2023) 23:207–16. doi: 10.1007/s11892-023-01515-1
9. Hirani D, Salem V, Khunti K, and Misra S. Newly detected diabetes during the COVID-19 pandemic: What have we learnt? Best Pract Res Clin Endocrinol Metab. (2023) 37:101793. doi: 10.1016/j.beem.2023.101793
10. Xie Y and Al-Aly Z. Risks and burdens of incident diabetes in long COVID: a cohort study. Lancet Diabetes Endocrinol. (2022) 10:311–21. doi: 10.1016/S2213-8587(22)00044-4
11. Tang X, Uhl S, Zhang T, Xue D, Li B, Vandana JJ, et al. SARS-CoV-2 infection induces beta cell transdifferentiation. Cell Metab. (2021) 33:1577–91.e7. doi: 10.1016/j.cmet.2021.05.015
12. Stefan N, Birkenfeld AL, and Schulze MB. Global pandemics interconnected - obesity, impaired metabolic health and COVID-19. Nat Rev Endocrinol. (2021) 17:135–49. doi: 10.1038/s41574-020-00462-1
13. Michalakis K and Ilias I. COVID-19 and hyperglycemia/diabetes. World J Diabetes. (2021) 12:642–50. doi: 10.4239/wjd.v12.i5.642
14. Unnikrishnan R and Misra A. Diabetes and COVID19: a bidirectional relationship. Nutr Diabetes. (2021) 11:21. doi: 10.1038/s41387-021-00163-2
15. Abubakar AR, Sani IH, Godman B, Kumar S, Islam S, Jahan I, et al. Systematic review on the therapeutic options for COVID-19: clinical evidence of drug efficacy and implications. Infect Drug Resist. (2020) 13:4673–95. doi: 10.2147/idr.S289037
16. Ahmed MH and Hassan A. Dexamethasone for the treatment of coronavirus disease (COVID-19): a review. SN Compr Clin Med. (2020) 2:2637–46. doi: 10.1007/s42399-020-00610-8
17. Vallianou NG, Evangelopoulos A, Kounatidis D, Stratigou T, Christodoulatos GS, Karampela I, et al. Diabetes mellitus and SARS-coV-2 infection: pathophysiologic mechanisms and implications in management. Curr Diabetes Rev. (2021) 17:e123120189797. doi: 10.2174/1573399817666210101110253
18. Heshmati HM. Interactions between COVID-19 infection and diabetes. Front Endocrinol (Lausanne). (2023) 14:1306290. doi: 10.3389/fendo.2023.1306290
19. Olsen RH, Krogh-Madsen R, Thomsen C, Booth FW, and Pedersen BK. Metabolic responses to reduced daily steps in healthy nonexercising men. Jama. (2008) 299:1261–3. doi: 10.1001/jama.299.11.1259
20. Orioli L, Hermans MP, Thissen JP, Maiter D, Vandeleene B, and Yombi JC. COVID-19 in diabetic patients: Related risks and specifics of management. Ann Endocrinol (Paris). (2020) 81:101–9. doi: 10.1016/j.ando.2020.05.001
21. Zhou J and Tan J. Diabetes patients with COVID-19 need better blood glucose management in Wuhan, China. Metabolism. (2020) 107:154216. doi: 10.1016/j.metabol.2020.154216
22. Saha S, Al-Rifai RH, and Saha S. Diabetes prevalence and mortality in COVID-19 patients: a systematic review, meta-analysis, and meta-regression. J Diabetes Metab Disord. (2021) 20:939–50. doi: 10.1007/s40200-021-00779-2
23. Misra A, Ghosh A, and Gupta R. Heterogeneity in presentation of hyperglycaemia during COVID-19 pandemic: A proposed classification. Diabetes Metab Syndr. (2021) 15:403–6. doi: 10.1016/j.dsx.2021.01.018
24. Ojo O, Wang XH, Ojo OO, Orjih E, Pavithran N, Adegboye ARA, et al. The effects of COVID-19 lockdown on glycaemic control and lipid profile in patients with type 2 diabetes: A systematic review and meta-analysis. Int J Environ Res Public Health. (2022) 19(3). doi: 10.3390/ijerph19031095
25. Finfer S, Chittock DR, Su SY, Blair D, Foster D, Dhingra V, et al. Intensive versus conventional glucose control in critically ill patients. N Engl J Med. (2009) 360:1283–97. doi: 10.1056/NEJMoa0810625
26. Singh AK and Khunti K. COVID-19 and diabetes. Annu Rev Med. (2022) 73:129–47. doi: 10.1146/annurev-med-042220-011857
27. Donath MY. Glucose or insulin, which is the culprit in patients with COVID-19 and diabetes? Cell Metab. (2021) 33:2–4. doi: 10.1016/j.cmet.2020.11.015
28. Yu B, Li C, Sun Y, and Wang DW. Insulin treatment is associated with increased mortality in patients with COVID-19 and type 2 diabetes. Cell Metab. (2021) 33:65–77.e2. doi: 10.1016/j.cmet.2020.11.014
29. Raveendran AV and Misra A. Post COVID-19 syndrome (“Long COVID”) and diabetes: challenges in diagnosis and management. Diabetes Metab Syndr. (2021) 15:102235. doi: 10.1016/j.dsx.2021.102235
30. Metwally AA, Mehta P, Johnson BS, Nagarjuna A, and Snyder MP. COVID-19-induced new-onset diabetes: trends and technologies. Diabetes. (2021) 70:2733–44. doi: 10.2337/dbi21-0029
31. Hayden MR. An immediate and long-term complication of COVID-19 may be type 2 diabetes mellitus: the central role of β-cell dysfunction, apoptosis and exploration of possible mechanisms. Cells. (2020) 9(11). doi: 10.3390/cells9112475
32. Li M, Wu X, Shi J, and Niu Y. Endothelium dysfunction and thrombosis in COVID-19 with type 2 diabetes. Endocrine. (2023) 82:15–27. doi: 10.1007/s12020-023-03439-y
33. Batabyal R, Freishtat N, Hill E, Rehman M, Freishtat R, and Koutroulis I. Metabolic dysfunction and immunometabolism in COVID-19 pathophysiology and therapeutics. Int J Obes (Lond). (2021) 45:1163–9. doi: 10.1038/s41366-021-00804-7
34. Ahmed I and Liu TYA. The impact of COVID-19 on diabetic retinopathy monitoring and treatment. Curr Diabetes Rep. (2021) 21:40. doi: 10.1007/s11892-021-01411-6
35. Lyons CE, Zhu I, and Gill MK. Accelerated progression of diabetic retinopathy following severe COVID-19 infection. Am J Ophthalmol Case Rep. (2023) 32:101911. doi: 10.1016/j.ajoc.2023.101911
36. Rego Lorca D, Rouco Fernandez A, Jimenez Santos M, Saenz-Frances F, Burgos-Blasco B, and Donate Lopez J. Bilateral retinal vein occlusion and diabetic retinopathy after COVID-19. Acta Ophthalmol. (2021) 99:e1246–e8. doi: 10.1111/aos.14718
37. Bhatta S, Pant N, and Keteca TB. Assessing the impact of COVID-19 on diabetic retinopathy in Fiji: A 5-year review. Clin Exp Ophthalmol. (2024) 52(6):684–6. doi: 10.1111/ceo.14378
38. Oh KE, Kim YJ, Oh YR, Kang E, Nam HK, Rhie YJ, et al. Commentary on "The prevalence of diabetic peripheral neuropathy in youth with diabetes mellitus". Ann Pediatr Endocrinol Metab. (2023) 28:275–82. doi: 10.6065/apem.2244214.107
39. Alrawaili MS, Abuzinadah AR, AlShareef AA, Hindi EA, Bamaga AK, Alshora W, et al. Serum SARM1 levels and diabetic peripheral neuropathy in type 2 diabetes: correlation with clinical neuropathy scales and nerve conduction studies and impact of COVID-19 vaccination. Vaccines (Basel). (2024) 12(2). doi: 10.3390/vaccines12020209
40. Mourad D, Azar NS, and Azar ST. Diabetic nephropathy and COVID-19: the potential role of immune actors. Int J Mol Sci. (2021) 22(15). doi: 10.3390/ijms22157762
41. Atri A, Kocherlakota CM, and Dasgupta R. Managing diabetic foot in times of COVID-19: time to put the best ‘foot’ forward. Int J Diabetes Dev Ctries. (2020) 40:321–8. doi: 10.1007/s13410-020-00866-9
42. de Sá-Ferreira CO, da Costa CHM, Guimarães JCW, Sampaio NS, Silva LML, de Mascarenhas LP, et al. Diabetic ketoacidosis and COVID-19: what have we learned so far? Am J Physiol Endocrinol Metab. (2022) 322:E44–e53. doi: 10.1152/ajpendo.00244.2021
43. Gupta A, Madhavan MV, Sehgal K, Nair N, Mahajan S, Sehrawat TS, et al. Extrapulmonary manifestations of COVID-19. Nat Med. (2020) 26:1017–32. doi: 10.1038/s41591-020-0968-3
44. Li J, Wang X, Chen J, Zuo X, Zhang H, and Deng A. COVID-19 infection may cause ketosis and ketoacidosis. Diabetes Obes Metab. (2020) 22:1935–41. doi: 10.1111/dom.v22.10
45. Souza E, Meneses-Santos D, Santos JC, Aidar FJ, Carvalho CRO, Santos JLD, et al. Does physical exercise promote health benefits for diabetic patients during the COVID-19 pandemic?”: A systematic review. Sports (Basel). (2023) 11(10). doi: 10.3390/sports11100192
46. Rajpal A, Rahimi L, and Ismail-Beigi F. Factors leading to high morbidity and mortality of COVID-19 in patients with type 2 diabetes. J Diabetes. (2020) 12:895–908. doi: 10.1111/1753-0407.13085
47. Vidhya Rekha U, Anita M, Bhuminathan S, and Sadhana K. Known data on CoVid-19 infection linked to type-2 diabetes. Bioinformation. (2021) 17:772–5. doi: 10.6026/97320630017772
48. Pranata R, Henrina J, Raffaello WM, Lawrensia S, and Huang I. Diabetes and COVID-19: The past, the present, and the future. Metabolism. (2021) 121:154814. doi: 10.1016/j.metabol.2021.154814
49. Bielka W, Przezak A, and Pawlik A. Therapy of type 2 diabetes in patients with SARS-coV-2 infection. Int J Mol Sci. (2021) 22(14). doi: 10.3390/ijms22147605
50. Shao S, Yang Q, Pan R, Yu X, and Chen Y. Interaction of severe acute respiratory syndrome coronavirus 2 and diabetes. Front Endocrinol (Lausanne). (2021) 12:731974. doi: 10.3389/fendo.2021.731974
51. Petakh P, Kamyshna I, Nykyforuk A, Yao R, Imbery JF, Oksenych V, et al. Immunoregulatory intestinal microbiota and COVID-19 in patients with type two diabetes: A double-edged sword. Viruses. (2022) 14(3). doi: 10.3390/v14030477
52. Pantanetti P, Cangelosi G, and Ambrosio G. Potential role of incretins in diabetes and COVID-19 infection: a hypothesis worth exploring. Intern Emerg Med. (2020) 15:779–82. doi: 10.1007/s11739-020-02389-x
53. Popovic DS, Papanas N, Pantea Stoian A, Rizvi AA, Janez A, and Rizzo M. Use of novel antidiabetic agents in patients with type 2 diabetes and COVID-19: A critical review. Diabetes Ther. (2021) 12:3037–54. doi: 10.1007/s13300-021-01170-3
54. Yusuf AP, Zhang JY, Li JQ, Muhammad A, and Abubakar MB. Herbal medications and natural products for patients with covid-19 and diabetes mellitus: Potentials and challenges. Phytomed Plus. (2022) 2:100280. doi: 10.1016/j.phyplu.2022.100280
55. Rasmi Y, di Bari I, Faisal S, Haque M, Aramwit P, da Silva A, et al. Herbal-based therapeutics for diabetic patients with SARS-Cov-2 infection. Mol Biol Rep. (2024) 51:316. doi: 10.1007/s11033-024-09291-1
56. Verma AK, Beg MMA, Bhatt D, Dev K, Alsahli MA, Rahmani AH, et al. Assessment and management of diabetic patients during the COVID-19 pandemic. Diabetes Metab Syndr Obes. (2021) 14:3131–46. doi: 10.2147/DMSO.S285614
57. Carr MJ, Wright AK, Leelarathna L, Thabit H, Milne N, Kanumilli N, et al. Impact of COVID-19 on diagnoses, monitoring, and mortality in people with type 2 diabetes in the UK. Lancet Diabetes Endocrinol. (2021) 9:413–5. doi: 10.1016/S2213-8587(21)00116-9
58. Rosta L, Menyhart A, Mahmeed WA, Al-Rasadi K, Al-Alawi K, Banach M, et al. Telemedicine for diabetes management during COVID-19: what we have learnt, what and how to implement. Front Endocrinol (Lausanne). (2023) 14:1129793. doi: 10.3389/fendo.2023.1129793
59. de Kreutzenberg SV. Telemedicine for the clinical management of diabetes; implications and considerations after COVID-19 experience. High Blood Press Cardiovasc Prev. (2022) 29:319–26. doi: 10.1007/s40292-022-00524-7
60. Choudhary P, Bellido V, Graner M, Altpeter B, Cicchetti A, Durand-Zaleski I, et al. The challenge of sustainable access to telemonitoring tools for people with diabetes in europe: lessons from COVID-19 and beyond. Diabetes Ther. (2021) 12:2311–27. doi: 10.1007/s13300-021-01132-9
61. Geravandi S, Mahmoudi-Aznaveh A, Azizi Z, Maedler K, and Ardestani A. SARS-CoV-2 and pancreas: a potential pathological interaction? Trends Endocrinol Metab. (2021) 32:842–5. doi: 10.1016/j.tem.2021.07.004
62. Steenblock C, Richter S, Berger I, Barovic M, Schmid J, Schubert U, et al. Viral infiltration of pancreatic islets in patients with COVID-19. Nat Commun. (2021) 12:3534. doi: 10.1038/s41467-021-23886-3
63. Wu CT, Lidsky PV, Xiao Y, Lee IT, Cheng R, Nakayama T, et al. SARS-CoV-2 infects human pancreatic β cells and elicits β cell impairment. Cell Metab. (2021) 33:1565–76.e5. doi: 10.1016/j.cmet.2021.05.013
64. Drucker DJ. Diabetes, obesity, metabolism, and SARS-CoV-2 infection: the end of the beginning. Cell Metab. (2021) 33:479–98. doi: 10.1016/j.cmet.2021.01.016
65. Hikmet F, Méar L, Edvinsson Å, Micke P, Uhlén M, and Lindskog C. The protein expression profile of ACE2 in human tissues. Mol Syst Biol. (2020) 16:e9610. doi: 10.15252/msb.20209610
66. Lumpuy-Castillo J, Lorenzo-Almorós A, Pello-Lázaro AM, Sánchez-Ferrer C, Egido J, Tuñón J, et al. Cardiovascular damage in COVID-19: therapeutic approaches targeting the renin-angiotensin-aldosterone system. Int J Mol Sci. (2020) 21(18). doi: 10.3390/ijms21186471
67. Kuba K, Yamaguchi T, and Penninger JM. Angiotensin-converting enzyme 2 (ACE2) in the pathogenesis of ARDS in COVID-19. Front Immunol. (2021) 12:732690. doi: 10.3389/fimmu.2021.732690
68. Chen F, Zhang Y, Li X, Li W, Liu X, and Xue X. The impact of ACE2 polymorphisms on COVID-19 disease: susceptibility, severity, and therapy. Front Cell Infect Microbiol. (2021) 11:753721. doi: 10.3389/fcimb.2021.753721
69. Rao S, Lau A, and So HC. Exploring diseases/traits and blood proteins causally related to expression of ACE2, the putative receptor of SARS-coV-2: A mendelian randomization analysis highlights tentative relevance of diabetes-related traits. Diabetes Care. (2020) 43:1416–26. doi: 10.2337/dc20-0643
70. Szlachcic WJ, Dabrowska A, Milewska A, Ziojla N, Blaszczyk K, Barreto-Duran E, et al. SARS-CoV-2 infects an in vitro model of the human developing pancreas through endocytosis. iScience. (2022) 25:104594. doi: 10.1016/j.isci.2022.104594
71. Bornstein SR, Dalan R, Hopkins D, Mingrone G, and Boehm BO. Endocrine and metabolic link to coronavirus infection. Nat Rev Endocrinol. (2020) 16:297–8. doi: 10.1038/s41574-020-0353-9
72. Yang JK, Lin SS, Ji XJ, and Guo LM. Binding of SARS coronavirus to its receptor damages islets and causes acute diabetes. Acta Diabetol. (2010) 47:193–9. doi: 10.1007/s00592-009-0109-4
73. Srinivasan K, Pandey AK, Livingston A, and Venkatesh S. Roles of host mitochondria in the development of COVID-19 pathology: Could mitochondria be a potential therapeutic target? Mol BioMed. (2021) 2:38. doi: 10.1186/s43556-021-00060-1
74. Shang C, Liu Z, Zhu Y, Lu J, Ge C, Zhang C, et al. SARS-coV-2 causes mitochondrial dysfunction and mitophagy impairment. Front Microbiol. (2021) 12:780768. doi: 10.3389/fmicb.2021.780768
75. Noonong K, Chatatikun M, Surinkaew S, Kotepui M, Hossain R, Bunluepuech K, et al. Mitochondrial oxidative stress, mitochondrial ROS storms in long COVID pathogenesis. Front Immunol. (2023) 14:1275001. doi: 10.3389/fimmu.2023.1275001
76. Antonelli M, Pujol JC, Spector TD, Ourselin S, and Steves CJ. Risk of long COVID associated with delta versus omicron variants of SARS-CoV-2. Lancet. (2022) 399:2263–4. doi: 10.1016/s0140-6736(22)00941-2
77. Eguchi N, Vaziri ND, Dafoe DC, and Ichii H. The role of oxidative stress in pancreatic β Cell dysfunction in diabetes. Int J Mol Sci. (2021) 22(4). doi: 10.3390/ijms22041509
78. Santos A, Magro DO, Evangelista-Poderoso R, and Saad MJA. Diabetes, obesity, and insulin resistance in COVID-19: molecular interrelationship and therapeutic implications. Diabetol Metab Syndr. (2021) 13:23. doi: 10.1186/s13098-021-00639-2
79. Oronsky B, Larson C, Hammond TC, Oronsky A, Kesari S, Lybeck M, et al. A review of persistent post-COVID syndrome (PPCS). Clin Rev Allergy Immunol. (2023) 64:66–74. doi: 10.1007/s12016-021-08848-3
80. Montefusco L, Ben Nasr M, D’Addio F, Loretelli C, Rossi A, Pastore I, et al. Acute and long-term disruption of glycometabolic control after SARS-CoV-2 infection. Nat Metab. (2021) 3:774–85. doi: 10.1038/s42255-021-00407-6
81. Huang C, Wang Y, Li X, Ren L, Zhao J, Hu Y, et al. Clinical features of patients infected with 2019 novel coronavirus in Wuhan, China. Lancet. (2020) 395:497–506. doi: 10.1016/s0140-6736(20)30183-5
82. Steenblock C, Schwarz PEH, Ludwig B, Linkermann A, Zimmet P, Kulebyakin K, et al. COVID-19 and metabolic disease: mechanisms and clinical management. Lancet Diabetes Endocrinol. (2021) 9:786–98. doi: 10.1016/S2213-8587(21)00244-8
83. Lu HJ, Koju N, and Sheng R. Mammalian integrated stress responses in stressed organelles and their functions. Acta Pharmacol Sin. (2024) 45(6):1095–114. doi: 10.1038/s41401-023-01225-0
84. Atkinson MA and Powers AC. Distinguishing the real from the hyperglycaemia: does COVID-19 induce diabetes? Lancet Diabetes Endocrinol. (2021) 9:328–9. doi: 10.1016/s2213-8587(21)00087-5
85. Laurenzi A, Caretto A, Molinari C, Mercalli A, Melzi R, Nano R, et al. No evidence of long-term disruption of glycometabolic control after SARS-coV-2 infection. J Clin Endocrinol Metab. (2022) 107:e1009–e19. doi: 10.1210/clinem/dgab792
86. Wu R, Mumtaz M, Maxwell AJ, Isaacs SR, Laiho JE, Rawlinson WD, et al. Respiratory infections and type 1 diabetes: Potential roles in pathogenesis. Rev Med Virol. (2023) 33:e20429. doi: 10.1002/rmv.v33.2
87. Dungan KM, Braithwaite SS, and Preiser JC. Stress hyperglycaemia. Lancet. (2009) 373:1798–807. doi: 10.1016/s0140-6736(09)60553-5
88. Landstra CP and de Koning EJP. COVID-19 and diabetes: understanding the interrelationship and risks for a severe course. Front Endocrinol (Lausanne). (2021) 12:649525. doi: 10.3389/fendo.2021.649525
89. Gómez-Banoy N, Guseh JS, Li G, Rubio-Navarro A, Chen T, Poirier B, et al. Adipsin preserves beta cells in diabetic mice and associates with protection from type 2 diabetes in humans. Nat Med. (2019) 25:1739–47. doi: 10.1038/s41591-019-0610-4
90. Reiterer M, Rajan M, Gómez-Banoy N, Lau JD, Gomez-Escobar LG, Ma L, et al. Hyperglycemia in acute COVID-19 is characterized by insulin resistance and adipose tissue infectivity by SARS-CoV-2. Cell Metab. (2021) 33:2174–88.e5. doi: 10.1016/j.cmet.2021.09.009
91. Shoelson SE, Herrero L, and Naaz A. Obesity, inflammation, and insulin resistance. Gastroenterology. (2007) 132:2169–80. doi: 10.1053/j.gastro.2007.03.059
93. Li JX and Cummins CL. Fresh insights into glucocorticoid-induced diabetes mellitus and new therapeutic directions. Nat Rev Endocrinol. (2022) 18:540–57. doi: 10.1038/s41574-022-00683-6
94. Kutpruek S, Suksri K, Maneethorn P, Semprasert N, Yenchitsomanus PT, and Kooptiwut S. Imatinib prevents dexamethasone-induced pancreatic β-cell apoptosis via decreased TRAIL and DR5. J Cell Biochem. (2023) 124:1309–23. doi: 10.1002/jcb.v124.9
95. Rhou YJJ, Hor A, Wang M, Wu YF, Jose S, Chipps DR, et al. Dexamethasone-induced hyperglycaemia in COVID-19: Glycaemic profile in patients without diabetes and factors associated with hyperglycaemia. Diabetes Res Clin Pract. (2022) 194:110151. doi: 10.1016/j.diabres.2022.110151
96. Snow TAC, Arulkumaran N, Singer M, and Choi SH. Effect of dexamethasone dose on outcomes in acute COVID-19 disease: A systematic review and meta-analysis. J Infect. (2023) 87:490–7. doi: 10.1016/j.jinf.2023.09.008
97. Polat V and Bostancı G. Sudden death due to acute pulmonary embolism in a young woman with COVID-19. J Thromb Thrombolysis. (2020) 50:239–41. doi: 10.1007/s11239-020-02132-5
98. Lim S, Bae JH, Kwon HS, and Nauck MA. COVID-19 and diabetes mellitus: from pathophysiology to clinical management. Nat Rev Endocrinol. (2021) 17:11–30. doi: 10.1038/s41574-020-00435-4
99. Chen N, Zhou M, Dong X, Qu J, Gong F, Han Y, et al. Epidemiological and clinical characteristics of 99 cases of 2019 novel coronavirus pneumonia in Wuhan, China: a descriptive study. Lancet. (2020) 395:507–13. doi: 10.1016/S0140-6736(20)30211-7
100. Tang Y, Liu J, Zhang D, Xu Z, Ji J, and Wen C. Cytokine storm in COVID-19: the current evidence and treatment strategies. Front Immunol. (2020) 11:1708. doi: 10.3389/fimmu.2020.01708
101. Moores LK, Tritschler T, Brosnahan S, Carrier M, Collen JF, Doerschug K, et al. Prevention, diagnosis, and treatment of VTE in patients with coronavirus disease 2019: CHEST guideline and expert panel report. Chest. (2020) 158:1143–63. doi: 10.1016/j.chest.2020.05.559
102. Qadir MMF, Bhondeley M, Beatty W, Gaupp DD, Doyle-Meyers LA, Fischer T, et al. SARS-CoV-2 infection of the pancreas promotes thrombofibrosis and is associated with new-onset diabetes. JCI Insight. (2021) 6(16). doi: 10.1172/jci.insight.151551
103. Tang N, Li D, Wang X, and Sun Z. Abnormal coagulation parameters are associated with poor prognosis in patients with novel coronavirus pneumonia. J Thromb Haemost. (2020) 18:844–7. doi: 10.1111/jth.14768
104. Han H, Yang L, Liu R, Liu F, Wu KL, Li J, et al. Prominent changes in blood coagulation of patients with SARS-CoV-2 infection. Clin Chem Lab Med. (2020) 58:1116–20. doi: 10.1515/cclm-2020-0188
105. Ryu JK, Yan Z, Montano M, Sozmen EG, Dixit K, Suryawanshi RK, et al. Fibrin drives thromboinflammation and neuropathology in COVID-19. Nature. (2024) 633:905–13. doi: 10.1038/s41586-024-07873-4
106. Akpek M. Does COVID-19 cause hypertension? Angiology. (2022) 73:682–7. doi: 10.1177/00033197211053903
107. Sheppard JP, Nicholson BD, Lee J, McGagh D, Sherlock J, Koshiaris C, et al. Association between blood pressure control and coronavirus disease 2019 outcomes in 45 418 symptomatic patients with hypertension: an observational cohort study. Hypertension. (2021) 77:846–55. doi: 10.1161/HYPERTENSIONAHA.120.16472
108. Azami P, Vafa RG, Heydarzadeh R, Sadeghi M, Amiri F, Azadian A, et al. Evaluation of blood pressure variation in recovered COVID-19 patients at one-year follow-up: a retrospective cohort study. BMC Cardiovasc Disord. (2024) 24:240. doi: 10.1186/s12872-024-03916-w
109. Vosko I, Zirlik A, and Bugger H. Impact of COVID-19 on cardiovascular disease. Viruses. (2023) 15(2). doi: 10.3390/v15020508
110. Basra R, Whyte M, Karalliedde J, and Vas P. What is the impact of microvascular complications of diabetes on severe COVID-19? Microvasc Res. (2022) 140:104310. doi: 10.1016/j.mvr.2021.104310
111. Wang T, Cao Y, Zhang H, Wang Z, Man CH, Yang Y, et al. COVID-19 metabolism: Mechanisms and therapeutic targets. MedComm (2020). (2022) 3:e157. doi: 10.1002/mco2.v3.3
112. Wu Q, Zhou L, Sun X, Yan Z, Hu C, Wu J, et al. Altered lipid metabolism in recovered SARS patients twelve years after infection. Sci Rep. (2017) 7:9110. doi: 10.1038/s41598-017-09536-z
113. Adeghate EA, Eid N, and Singh J. Mechanisms of COVID-19-induced heart failure: a short review. Heart Fail Rev. (2021) 26:363–9. doi: 10.1007/s10741-020-10037-x
114. Zheng YY, Ma YT, Zhang JY, and Xie X. COVID-19 and the cardiovascular system. Nat Rev Cardiol. (2020) 17:259–60. doi: 10.1038/s41569-020-0360-5
115. Xie Y, Xu E, Bowe B, and Al-Aly Z. Long-term cardiovascular outcomes of COVID-19. Nat Med. (2022) 28:583–90. doi: 10.1038/s41591-022-01689-3
116. Agricola E, Beneduce A, Esposito A, Ingallina G, Palumbo D, Palmisano A, et al. Heart and lung multimodality imaging in COVID-19. JACC Cardiovasc Imag. (2020) 13:1792–808. doi: 10.1016/j.jcmg.2020.05.017
117. Gilbert RE, Caldwell L, Misra PS, Chan K, Burns KD, Wrana JL, et al. Overexpression of the severe acute respiratory syndrome coronavirus-2 receptor, angiotensin-converting enzyme 2, in diabetic kidney disease: implications for kidney injury in novel coronavirus disease 2019. Can J Diabetes. (2021) 45:162–6.e1. doi: 10.1016/j.jcjd.2020.07.003
118. Heyman SN, Walther T, and Abassi Z. Angiotensin-(1-7)-A potential remedy for AKI: insights derived from the COVID-19 pandemic. J Clin Med. (2021) 10(6). doi: 10.3390/jcm10061200
119. Nie X, Qian L, Sun R, Huang B, Dong X, Xiao Q, et al. Multi-organ proteomic landscape of COVID-19 autopsies. Cell. (2021) 184:775–91.e14. doi: 10.1016/j.cell.2021.01.004
120. Shi Y and Vanhoutte PM. Macro- and microvascular endothelial dysfunction in diabetes. J Diabetes. (2017) 9:434–49. doi: 10.1111/jdb.2017.9.issue-5
121. Kipshidze N, Dangas G, White CJ, Kipshidze N, Siddiqui F, Lattimer CR, et al. Viral coagulopathy in patients with COVID-19: treatment and care. Clin Appl Thromb Hemost. (2020) 26:1076029620936776. doi: 10.1177/1076029620936776
122. Landecho MF, Yuste JR, Gándara E, Sunsundegui P, Quiroga J, Alcaide AB, et al. COVID-19 retinal microangiopathy as an in vivo biomarker of systemic vascular disease? J Intern Med. (2021) 289:116–20. doi: 10.1111/joim.13156
123. Rathmann W, Kuss O, and Kostev K. Incidence of newly diagnosed diabetes after Covid-19. Diabetologia. (2022) 65:949–54. doi: 10.1007/s00125-022-05670-0
124. Qeadan F, Tingey B, Egbert J, Pezzolesi MG, Burge MR, Peterson KA, et al. The associations between COVID-19 diagnosis, type 1 diabetes, and the risk of diabetic ketoacidosis: A nationwide cohort from the US using the Cerner Real-World Data. PLoS One. (2022) 17:e0266809. doi: 10.1371/journal.pone.0266809
125. Tazare J, Walker AJ, Tomlinson LA, Hickman G, Rentsch CT, Williamson EJ, et al. Rates of serious clinical outcomes in survivors of hospitalisation with COVID-19 in England: a descriptive cohort study within the OpenSAFELY platform. Wellc Open Res. (2022) 7:142. doi: 10.12688/wellcomeopenres
126. Cohen K, Ren S, Heath K, Dasmariñas MC, Jubilo KG, Guo Y, et al. Risk of persistent and new clinical sequelae among adults aged 65 years and older during the post-acute phase of SARS-CoV-2 infection: retrospective cohort study. BMJ. (2022) 376:e068414. doi: 10.1136/bmj-2021-068414
127. Birabaharan M, Kaelber DC, Pettus JH, and Smith DM. Risk of new-onset type 2 diabetes in 600 055 people after COVID-19: A cohort study. Diabetes Obes Metab. (2022) 24:1176–9. doi: 10.1111/dom.14659
128. Barrett CE, Koyama AK, Alvarez P, Chow W, Lundeen EA, Perrine CG, et al. Risk for newly diagnosed diabetes >30 days after SARS-coV-2 infection among persons aged <18 years - United States, March 1, 2020-June 28, 2021. MMWR Morb Mortal Wkly Rep. (2022) 71:59–65. doi: 10.15585/mmwr.mm7102e2
129. Ayoubkhani D, Khunti K, Nafilyan V, Maddox T, Humberstone B, Diamond I, et al. Post-covid syndrome in individuals admitted to hospital with covid-19: retrospective cohort study. BMJ. (2021) 372:n693. doi: 10.1136/bmj.n693
Keywords: COVID-19, SARS-CoV-2, diabetes, glucose metabolism, pancreatic β-cells, virus induced diabetes
Citation: Zhao X, Jiang L, Sun W, Tang S, Kang X, Gao Q, Li Z, An X and Lian F (2025) Understanding the interplay between COVID-19 and diabetes: insights for the post-pandemic era. Front. Endocrinol. 16:1599969. doi: 10.3389/fendo.2025.1599969
Received: 25 March 2025; Accepted: 29 April 2025;
Published: 21 May 2025.
Edited by:
Konstantinos Kantartzis, University of Tübingen, GermanyReviewed by:
Eleni Karlafti, Aristotle University of Thessaloniki, GreeceZisis Kontoninas, Aristotle University of Thessaloniki, Greece
Copyright © 2025 Zhao, Jiang, Sun, Tang, Kang, Gao, Li, An and Lian. This is an open-access article distributed under the terms of the Creative Commons Attribution License (CC BY). The use, distribution or reproduction in other forums is permitted, provided the original author(s) and the copyright owner(s) are credited and that the original publication in this journal is cited, in accordance with accepted academic practice. No use, distribution or reproduction is permitted which does not comply with these terms.
*Correspondence: Fengmei Lian, bGZtNTY1QHNvaHUuY29t; Xuedong An, ZG9jdG9yX2FueGRAMTYzLmNvbQ==
†These authors have contributed equally to this work and share first authorship