- 1Department of Pharmaceutical Sciences, College of Pharmacy, Oregon State University, Corvallis, OR, United States
- 2Vaccine and Gene Therapy Institute, Oregon Health and Science University, Beaverton, OR, United States
Expanding efforts to develop preventive gonorrhea vaccines is critical because of the serious health consequences combined with the prevalence and the dire possibility of untreatable gonorrhea. Reverse vaccinology, which includes genome and proteome mining, has proven successful in the discovery of vaccine candidates against many pathogenic bacteria. Here, we describe proteomic applications including comprehensive, quantitative proteomic platforms and immunoproteomics coupled with broad-ranging bioinformatics that have been applied for antigen mining to develop gonorrhea vaccine(s). We further focus on outlining the vaccine candidate decision tree, describe the structure-function of novel proteome-derived antigens as well as ways to gain insights into their roles in the cell envelope, and underscore new lessons learned about the fascinating biology of Neisseria gonorrhoeae.
Introduction
Worldwide, over 78 million people are estimated to acquire the sexually transmitted infection gonorrhea every year (1). Female reproductive health is disproportionately affected by this disease and half of infected women show no symptoms (2–4). Serious health consequences are associated with untreated or insufficiently treated gonorrhea, including pelvic inflammatory disease, inflammation of the fallopian tubes, pre-term delivery, miscarriage, or ectopic pregnancy (5–7). Additionally, infants born vaginally to infected mothers are exposed to the disease in the birth canal and are thus at risk of developing a sight-threatening conjunctivitis (8). Although the health consequences to men are not as severe as for women and predominantly manifest as uncomplicated urethritis accompanied by a neutrophil-rich exudate (6, 9), gonorrhea can ascend to the epididymis or the testes and may require surgical removal of the infected site (10–12). Infertility can occur in both females and males without proper treatment (9, 13).
The bacterium responsible for gonorrhea, Neisseria gonorrhoeae (Ng), is a highly adaptable pathogen. Its natural competence and plastic genome have contributed to the extensive spread of antibiotic resistance. Through a number of horizontally acquired genes and chromosomal mutations, Ng has become resistant to every antibiotic used for its treatment (14–16). The Centers for Disease Control and Prevention (CDC) in the United States currently recommend a dual therapy of intramuscular ceftriaxone combined with oral azithromycin as a first-line treatment for uncomplicated gonorrhea (17, 18). However, the first isolates resistant to this combination therapy have begun to emerge (19). Three new therapeutics for gonorrhea treatment are being evaluated in clinical trials (20), but considering the speed with which the gonococcus develops antibiotic resistance (15), new drugs will not provide a long-term solution. The development and introduction of a protective vaccine against gonorrhea should therefore be prioritized to limit its spread.
Thus far, only two gonorrhea vaccines, using either killed whole organisms or purified pilin protein, have progressed to clinical trials. Despite robust antibody responses in both trials, neither vaccine provided protection against acquiring the disease after immunization (21–24). These failures are likely due to a number of factors. Pilin proteins undergo extensive antigenic variation through frequent recombination with transcriptionally silent pilS gene cassettes (25–28). Experimental infections have demonstrated that multiple pilin variants are isolated from a single individual, and that these variants are antigenically distinct from the inoculating parent strain (29–31). Further, pilin proteins are subjected to phase variation, where protein expression transitions between “on” and “off” states through slipped-strand repair of upstream repeat regions (32). Antigenic and phase variation of pilin during infection likely contributed to the failure of both vaccine trials. Another factor that may have led to the whole cell vaccine's inability to protect from infection is the presence of the reduction modifiable protein (Rmp; also known as protein III) in the vaccine. Localized to the outer membrane, Rmp is highly conserved and immunogenic, yet antibodies induced by this antigen actively prevent assembly of the complement membrane attack complex in immune serum (33, 34). These challenges illustrated the necessity for new approaches in gonorrhea vaccine development.
In the intervening years, vaccine progress has been slow. One of the difficulties is that Ng infection rarely, if ever, leads to an adaptive immune response (35–38). For this reason, mechanisms of protection against gonorrhea are unknown (24), which makes the evaluation of the potential efficacy of vaccine candidates prior to expensive immunization studies challenging. The serum bactericidal activity of antibodies generated during an immune response strongly predicts protection for vaccines against N. meningitidis [Nm; (39, 40)], a frequent causative agent of meningitis, so the ability of gonococcal antigens to elicit bactericidal antibodies is currently used as a surrogate mechanism of protection (41). Based on this criterion, 14 Ng antigens with functions in colonization and invasion, nutrient acquisition, and immune evasion have been proposed for inclusion in a gonorrhea vaccine [reviewed in (41)]. Immunization with each of the candidate proteins, cyclic loop peptides, or lipooligosaccharide epitope mimics elicited bactericidal antibodies, although studies for seven of the antigens were performed only in Nm (41).
Despite the difficulties in developing a vaccine against gonorrhea, several recent advances suggest that a protective vaccine is now within reach. The first was the development of a female mouse model of lower genital tract infection, in which mice are treated with 17-β estradiol and a cocktail of antibiotics to increase susceptibility to Ng and to reduce the overgrowth of vaginal commensal bacteria, respectively (42). This model has enabled the study of the immune response to gonococcal infection in a whole organism for which extensive genetic and immunological tools are available (24, 43, 44). A series of elegant studies, combining information gathered from experimental murine infections and tissue culture experiments, demonstrated Ng actively suppresses the generation of a productive adaptive immune response. Both mouse splenic mononuclear cells and human dendritic cells infected with Ng produced elevated levels of interleukin (IL)-6, tumor necrosis factor-α (TNF-α), IL-1β, and IL-23, a set of cytokines that promote terminal differentiation of T-cells toward T helper 17 (Th17) cells (45, 46). Production of IL-17 is a characteristic marker of a Th17 response and promotes neutrophil recruitment through the induction of granulocyte-colony stimulating factor and chemokines (45). In support of gonorrhea promoting Th17 differentiation during an active infection, elevated levels of IL-17 were discovered in female mice challenged with Ng (46). Gonococci are also able to divert T-cell differentiation away from an adaptive Th1/Th2 response by inducing the production of transforming growth factor (TGF)-β and IL-10 (47–49). Furthermore, Ng stimulates the differentiation of macrophages toward a regulatory phenotype and prevents macrophage antigen display. Through these immunosuppressive activities, the gonococcus is able to further inhibit the generation of a protective T-cell response (50, 51). The knowledge gained through these studies into the sophisticated methods Ng uses to promote its own survival and prevent triggering an adaptive immune response will enable a more informed strategy for vaccine development and help avoid the failures of the past.
In studies making use of the insights gathered from a better knowledge of the immune evasion strategies employed by the gonococcus, mice treated intravaginally with micro-encapsulated IL-12 and either infected with a common laboratory strain, FA1090, or immunized with membrane vesicles (MVs) collected from FA1090 were protected against subsequent infections up to 6 months after the initial treatment, even when challenged with heterologous strains (52, 53). IL-12 treatment promoted a Th1 response, as well as enhancing serum immunoglobulin A (IgA) and vaginal IgA and IgG levels (54).
Lessons can also be learned from the successful development of the licensed four-component Nm serogroup B vaccine, 4CMenB (BEXSERO; GlaxoSmithKline). This bacterium presented a daunting vaccination challenge for a number of years due to the polysaccharide capsule surrounding group B meningococci, which is structurally identical to the polysialic acid carbohydrate found on the surface of many human cells. Because of this similarity, immunization with the group B capsule is minimally immunogenic and/or may result in the generation of autoantibodies (55). To circumvent this problem, a subunit vaccine was developed by identifying conserved open reading frames in the whole genome sequence of Nm serogroup B, a strategy termed reverse vaccinology (55–59). Out of nearly 600 vaccine candidates identified with this strategy, 350 were successfully expressed and purified from Escherichia coli, 28 elicited bactericidal antibodies, and only three recombinant proteins—two of which are composed of a fusion of two proteins—were combined with MVs to formulate 4CMenB (59, 60). Finally, a retrospective study found that immunization with another Nm serogroup B vaccine, MeNZB, containing the same MVs as 4CMenB, was 31% effective at preventing gonorrhea (61, 62). The MeNZB vaccine is no longer available, but these seminal studies provide strong evidence that a protective gonorrhea vaccine is possible.
A comparison of the number of antigens evaluated for the serogroup B vaccine with the number currently being investigated for a gonorrhea vaccine illustrates how far gonorrhea research lags behind meningitis research and emphasizes that new strategies are necessary to increase the pool of vaccine candidates under consideration. An innovative way to address this gap was to perform reverse vaccinology antigen mining using subcellular fractionation coupled with high-throughput quantitative proteomics followed by bioinformatics (63, 64), which identified numerous stably expressed proteins and suggested that formulation of a subunit vaccine against gonorrhea would be successful. Both genome- and proteome-based reverse vaccinology approaches have become more prevalent since the technique was introduced (56). Candidate vaccine antigens have been identified through whole-genome screens of a number of medically important pathogens (65–69). As the availability of bacterial genome sequences has increased, more detailed analyses have become possible, including comparative genomics. One weakness of using whole genome sequences to search for vaccine candidate antigens is that the pathogens do not necessarily express the proteins discovered through this approach. Transcriptome analysis provides a way to circumvent this limitation but a low correlation between transcriptomic and proteomic data has been well established [reviewed in (70)]. For this reason, we have chosen to pursue proteomic-based reverse vaccinology, as proteomic studies reveal the biologically relevant population of proteins expressed during exposure to the conditions under examination (63, 64, 71). Proteomic approaches also have the potential to specifically identify surface-exposed proteins without the need for extensive bioinformatic predictions (72).
In this article, we provide an overview of proteomic and bioinformatic approaches that have been utilized for gonorrhea antigen mining. Our focus will also be on functional and structural characterization of proteome-derived antigens to determine their role in gonococcal pathogenesis and physiology as well as to inform the development of next generation vaccines based on structural vaccinology.
Proteomic Technologies Applied for Gonorrhea Antigen Mining
Surface-localized proteins represent attractive vaccine candidates, as they are important foci for the immune system and play pivotal roles in bacterial physiology as well as host-pathogen interactions. Naturally elaborated MVs (NeMVs) and MVs extracted from cell evelopes (CE) by either lithium or deoxycholate treatment (LeOMVs or DeOMVs, respectively) contain surface-localized proteins, other outer membrane and periplasm-derived proteins, and commonly cytoplasmic proteins (73–79). In addition to proteins, MVs contain lipopolysaccharide and DNA of chromosomal, plasmid, or phage origin, as well as RNA (73, 75, 80). NeMVs are purified and concentrated from culture supernatants by separating intact cells from already-formed NeMVs (Figure 1). Le-MVS and De-MVs are extracted from bacterial cells with detergent, reducing the content of reactogenic LPS/LOS and also many lipoproteins (75, 81, 82). Including different types of MVs in vaccine formulations has led to some of the most effective vaccines against bacterial diseases (61, 81, 83). Remarkably, following a nationwide implementation of 4CMenB, a recent study showed >80% vaccine-mediated protection against current Nm B strains in the United Kingdom (84, 85).
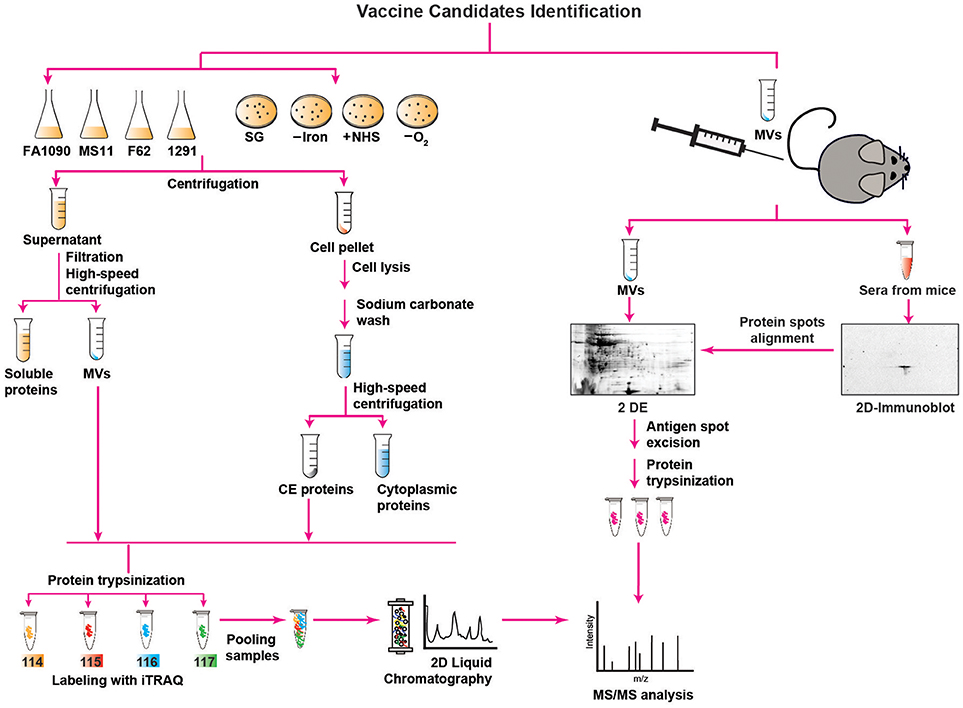
Figure 1. Outline of proteomics approaches used for mining of Neisseria gonorrhoeae vaccine antigens. Description is provided in the main text. SG, standard growth conditions; –Iron, iron deprivation; +NHS, addition of normal human serum; –O2, anaerobic growth conditions; MVs, membrane vesicles; CE, CE; 2 D, two-dimensional; 2 DE, two-dimensional electrophoresis; iTRAQ, isobaric tagging for absolute quantitation; MS/MS, mass spectrometry analysis.
For all these reasons, the identification of CE and MV antigens is a key objective of proteomics-driven vaccinology. It is however, a difficult task, because membrane proteins are commonly low abundant, hydrophobic, have a basic charge, and can be of high molecular weight. Therefore, comprehensive antigen mining of bacterial CE and MVs necessitates extensive subcellular fractionation procedures coupled with high-throughput quantitative proteomics and extensive bioinformatics (Figure 1). In addition, multidimensional protein identification technology combats these challenges by using a combination of two different kinds of liquid chromatography (2D-LC) that separates proteins prior to their identification, greatly diminishing the complexity of the sample at the peptide level and resulting in the identification of a superior number of proteins (75, 86).
Accordingly, to interrogate the Ng CE and MVs for new antigens, three independent proteomic technologies and experimental designs have been applied for the first time in the gonorrhea field (52, 63, 64). In the earliest proteomic mining, four common laboratory Ng strains (FA1090, MS11, F62, and 1291) were cultured under standard growth conditions in liquid medium to mid-logarithmic phase and subjected to fractionation, sodium carbonate extraction, and ultracentrifugation steps to isolate NeMVs and CE proteins [Figure 1; (63)]. Subsequently, these subproteomes were trypsinized and labeled with four different isobaric tags (114, 115, 116, and 117) targeting ε-amine group of lysine in peptides for relative and absolute quantitation (iTRAQ). After labeling, the samples were pooled and the peptide mixture was subjected to fractionation by 2D-LC followed by MS/MS for protein detection and quantitation. This multiplexed high-throughput proteomics approach enabled identification of 533 and 168 common proteins in the CE and MVs, respectively, in all four Ng strains in biological replicate experiments. Strain FA1090 was arbitrarily selected as the reference strain for calculating the protein abundance. After applying rigorous criteria, Zielke et al. (63) eliminated up to 68% of identified proteins. Among these proteins, 305 and 46 were uniformly present in the CE and MVs, respectively, in four strains. A total of 22 proteins were present at different levels in both analyzed subproteomes of these strains. Overall, these studies led to identification of a plethora of proteins that were either novel or had not been characterized in Ng. In this group were ubiquitous proteins localized to the CE and MVs: LPS-transport protein LptD (OstA, Imp), BamA, BamE, a predicted extracellular protein NGO1063 (SliC), and outer membrane proteins NGO1205 (ZnuD), NGO1344 (AsmA), NGO1956 (TamA), NGO1985 (BamG), NGO2111 (Slam2), NGO2121 (MlaA), NGO2139 (MetQ), and NGO2054 (63, 64). We focus on these proteome-derived antigens in the later sections of this article.
In the second proteomics-driven antigen mining approach for gonorrhea vaccine(s), our group was interested in discovering antigens induced in response to host-relevant environmental stimuli as they may represent novel protective antigens in distinct niches in the human host (64). Therefore, the model Ng strain FA1090 was subjected to aerobic (SG) and anaerobic (–O2) conditions, iron deprivation (–Iron), and exposure to normal human sera (+NHS; Figure 1), followed by CE protein extraction, trypsinization, iTRAQ labeling, and 2D-LC MS/MS (64). Three biological experiments yielded 751 common proteins with 17, 32, and 367 proteins with altered expression compared to SG in the presence of NHS, upon iron deprivation, and during anaerobic growth, respectively. In addition, 259 proteins were ubiquitously expressed under all conditions. There were many newly identified ubiquitous and differentially expressed CE proteins and potential new antigens including Slam2 and NGO1688 (both positively regulated by low iron), and ZnuD, which was induced under oxygen limitation (64).
In addition to the aforementioned approaches, we applied classical immunoproteomics to identify potential cross-reactive antigens in native MVs derived from Ng FA1090 that were intravaginally inoculated concurrently with interleukin-12 (Figure 1) and showed protection against heterologous Ng strains in the female mouse model of lower genital tract infection (52). Our approach relied on 2 DE SDS-PAGE separation of Ng native MVs coupled with immunoblotting with sera from MV-immunized mice (Figure 1). The overall MV proteome maps were created by staining proteins in a fluorescent stain. After the superimposition of antigenic maps (2D Immunoblot) with proteome maps, matching spots were excised and the proteins were subjected to trypsin digestion and MS/MS-based identification. The blotted protein maps consistently showed two spots of masses corresponding to 45 kDa and 43 kDa that were identified by MS/MS as translation elongation factor-Tu (EF-Tu) and a putative periplasmic polyamine-binding protein, PotF3, respectively. Supporting these findings, both proteins were also identified in our quantitative proteomic profiling of MVs derived from four common gonococcal isolates (63).
Bioinformatics for Gonorrhea Vaccines
After the mining and discovery of potential new antigens, insights can be gained into their suitability in a vaccine formulation through bioinformatic analyses to predict their function, subcellular localization, and post-translational modifications (Figure 2). In our studies, we employed PSORTb (87, 88), SOSUI-GramN (89), and CELLO (90, 91) algorithms to infer protein localization within the cell and to identify proteins discovered in the CE or MVs that localized to the outer membrane (63, 64). While each method considers the physiochemical properties of the amino acids that make up the protein, follow up computations differ between algorithms. To increase the accuracy of the subcellular localization predictions in our studies, we utilized a majority-votes strategy in which proteins were assigned to cellular compartments based on the results of at least two of the three methods (63, 64). To provide additional confidence in the localization predictions, searches for signal sequences recognized by signal peptidase (SPase) I or SPaseII can also be performed. The SignalP 4.1 server1 can be used as a tool to detect signal peptides (SP; Figure 3) associated with targeting to secretory pathways and cleaved by SPaseI (92, 93), while the LipoP 1.0 server2 detects lipoprotein signal peptides (LSP; Figure 3) associated with cleavage by SPaseII and further processing through the lipoprotein maturation pathway (94). Lipoproteins are characterized by an invariant cysteine residue that is modified with two or three acyl chains, which allows a hydrophilic protein to remain anchored to the membrane (95). Depending on secondary sorting signals, which are not well understood, lipoproteins may be anchored to either the inner or outer membrane and may face the periplasm or the extracellular milieu. Surface-exposed lipoproteins may be involved in nutrient acquisition, cell wall homeostasis, and adhesion to host cells (95), properties which we will discuss in more detail below. Finally, lipoproteins exposed to the extracellular milieu act as ligands for Toll-like receptor (TLR) 2 and may thus contribute to activating an adaptive immune response (95), a feature which makes them intriguing targets for vaccine development.
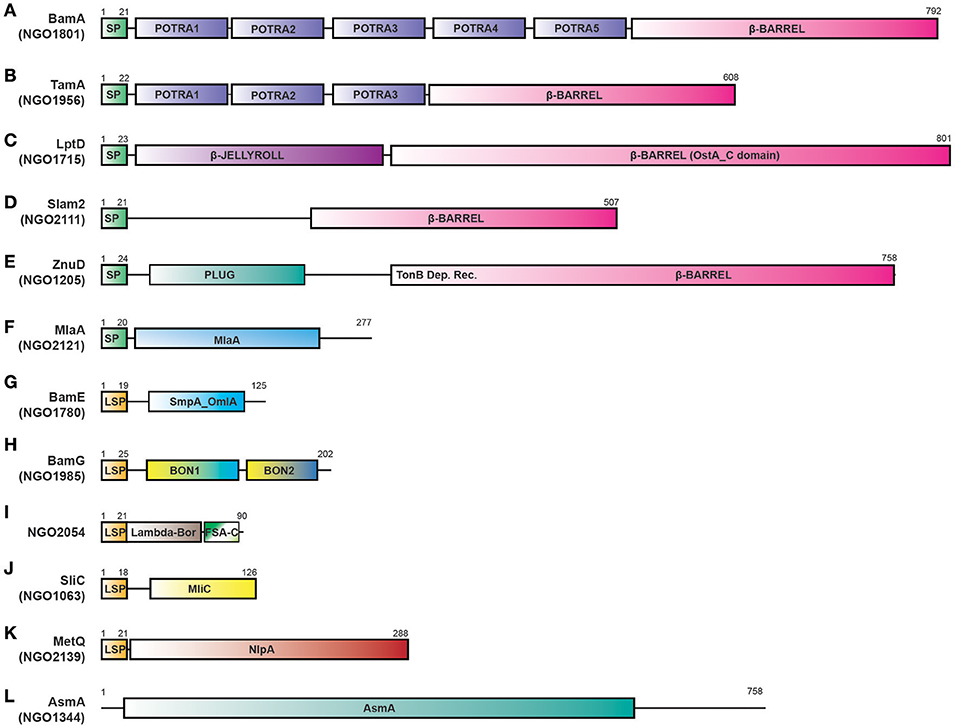
Figure 3. Proteome-derived antigen domain schematics. ORFs were examined for the presence of domains using KEGG, NCBI, UNIPROT, and literature searches. The presence of signal peptides was determined using the SignalP 4.1 and LipoP 1.0 servers. (A) BamA with a signal peptide, five POTRA domains, and an OMP β-barrel domain. (B) TamA protein with a signal peptide, three POTRA domains and an OMP β-barrel domain. (C) LptD with a signal peptide, N terminal β-JELLYROLL domain, and β-barrel OstA_C domain. (D) Slam 2 with a signal peptide and C terminal β-barrel domain (a DUF560 domain). (E) ZnuD comprises a signal peptide, plug domain, a Ton-B dependent receptor domain, and an OMP β-barrel domain. (F) MlaA with a signal peptide and predicted MlaA domain. (G) BamE containing a lipoprotein signal peptide and SmpA_OmlA domain. (H) BamG is composed of a lipoprotein signal peptide and two BON domains. (I) NGO2054 contains a Lambda-Bor domain that overlaps with a lipoprotein signal peptide, as well as a Fragile Site Associated protein C-terminus (FSA-C). (J) SliC containing a lipoprotein signal peptide and MliC protein family domain. (K) MetQ comprises a lipoprotein signal peptide and a NlpA domain (L) AsmA includes an AsmA domain. Schematics are not to scale. SP, signal peptide; LSP, lipoprotein signal peptide; BON, bacterial OsmY and nodulation; FSA-C, Fragile site-associated protein C-terminus; OMP, outer membrane protein.
An additional analysis that can be performed to complement the prediction of subcellular localization includes functional category predictions through searches for Clusters of Orthologous Groups (COG). This analysis involves a phylogenetic comparison of the proteome-derived vaccine candidates to a database of proteins with known or predicted functions to determine to which cellular process(es) the protein is likely to contribute (96, 97). The results are grouped into four broad categories—Cellular Processes and Signaling, Information Storage and Processing, Metabolism, and Poorly Characterized—each of which can then be broken down further. Altogether, proteins can be clustered into one or more of 25 categories. Although the NCBI COG database3 does not contain information for Ng, COG data is available for Nm, which allows for functional analysis using a closely related organism. Insights gained into the function of the proteome-derived vaccine candidates will enable a more rational vaccine design approach, in which candidate antigens involved in multiple functions or in functional categories critical to bacterial fitness can be preferentially chosen in the vaccine decision tree and evaluated for their protective capabilities (Figure 2).
The proliferation of genomic data also benefits proteomics-driven reverse vaccinology. Of particular utility to gonorrhea vaccine research is the publically available Neisseria Multi Locus Sequence Typing database4 [Neisseria PubMLST, developed by Keith Jolley and sited at the University of Oxford; (98)], which has collected whole or partial genome sequence data from nearly 47,000 Neisseria isolates as of August 19, 2018. Annotation of the Neisseria pan-genome is underway, which would further facilitate vaccine development by identifying antigens that are present across the known population of Ng isolates, as well as proteins that are uniquely associated with highly antibiotic resistant strains. The wealth of information available from this database enables bioinformatic analyses of antigen conservation across numerous sequenced isolates. For example, in our characterization of a novel surface exposed lysozyme inhibitor of C-type lysozyme encoded by the ngo1063 open reading frame, SliC, use of the Neisseria PubMLST database revealed the existence of only 10 closely related alleles with 9 single nucleotide polymorphic sites across nearly 5,000 Ng isolates. Further, 98% of isolates in the database possessed a single sliC allele (99), indicating that this protein is highly conserved and its inclusion in a vaccine could provide broad protection without the need for multiple antigenic variants.
Another analysis made possible by the information present in the PubMLST database is to map polymorphisms to available structural data (Figure 2). If highly prevalent polymorphisms are found on surface-exposed portions of the proteins, multiple recombinant proteins that incorporate the most common variants may need to be included in a vaccine to provide protection against a broader range of strains. While structural data will not necessarily be available immediately for all vaccine candidates, another computational analysis method may still enable prediction of surface exposed polymorphisms. Prediction of transmembrane helices by hidden Markov modeling using the TMHMM 2.0 server5 can reveal protein regions predicted to be internal, transmembrane, or external (100). Combining data from PubMLST with the results of TMHMM predictions can suggest which polymorphisms are likely to be surface exposed. Importantly, this analysis must be accompanied by surface-exposure assessments, as we discuss below, and may not be accurate for lipoproteins where the lipid anchor could be the only portion of the protein embedded in the membrane.
Additionally relevant to vaccine research are bioinformatic tools designed to predict the immunogenicity and protective capability of candidate antigens. Depending on whether the desired immune response is humoral (B-cell mediated) or cellular (T-cell mediated), numerous tools exist to predict whether antigen-derived peptides possess epitopes that are likely to be recognized by either of the major histocompatibility complex (MHC) class I or II proteins, the transporter associated protein (TAP) responsible for translocating peptides across the endoplasmic reticulum to MHC molecules for surface display, or B cell receptors. MHC I predictive tools have been estimated to be 90–95% accurate, although MHC II predictions are less reliable. Structural information is required for accurate B cell receptor binding predictions, as B cells and antibodies recognize the protein's native form. Certain tools use biochemical properties of the protein's constituent amino acids to predict likely B cell receptor binding sites. More accurate techniques require the protein's 3D structure as input [prediction tools extensively reviewed in (101)]. These prediction strategies may be useful to filter out antigens that are not likely to generate an immune response. However, follow-up immunological testing in the mouse as described below will be required to establish whether the response is protective or whether, as in the case of Rmp, the immunogenic response actively blocks the action of the adaptive immune system (33, 34).
Vaccine Decision Tree
Subsequent to proteomic identification and bioinformatic analysis, candidate antigens should be assessed for their suitability for inclusion in a vaccine. Here, we suggest a decision tree for the evaluation of vaccine candidate proteins (Figure 2). To determine the expression characteristics of each vaccine candidate, they should initially be expressed and purified from a heterologous host, such as E. coli, and used to immunize rabbits or mice to collect polyclonal immune serum specific to the protein under investigation. These sera can then be used for several informative studies, such as confirmation of localization predictions by probing subcellular fractions to determine in which compartment(s) the protein is predominantly located. Immune sera can be used to interrogate the abundance of proteins after proteolytic shaving of intact cells, an experiment which will demonstrate whether proteins are accessible to external proteases (64). Protein accessibility to antibodies can also be directly investigated by immunoblotting analysis of intact cells spotted onto membranes and comparison of the signal to lysed cells using known surface-exposed proteins as controls (64). Antisera generated against vaccine candidates coupled with fluorescently labeled secondary antibodies can also be used to establish antigenic surface exposure through fluorescence-activated cell sorting analyses (68). Furthermore, primary antisera coupled with gold nanoparticle-labeled secondary antibodies can be employed in electron microscopy studies of surface exposure. With this type of study, not only can surface exposure be confirmed, but the localization, distribution and overall abundance of surface proteins can also be directly observed (102, 103). Serum raised against a candidate antigen additionally enables studies to accompany conservation predictions performed during initial bioinformatic analyses. In our assessments of candidate antigens, we employed a panel of at least 36 genetically, geographically, and temporally distinct Ng isolates, including the 2016 WHO reference strains (104), to examine whether the expression of each antigen is consistent across heterogeneous strains, as well as to determine whether the epitope(s) recognized by the antiserum are conserved in diverse gonococci and meningococci (64, 79, 99). Finally, to determine the likelihood that immunization with vaccine candidate antigens will be protective, antiserum raised against each antigen can be used to assess the protein's ability to elicit bactericidal and/or opsonophagocytic antibodies (24, 43). As mentioned previously, it is unknown whether antibodies' bactericidal or opsonophagocytic properties will predict their protective capabilities (24, 41). However, one or both characteristics is likely to contribute to a productive adaptive immune response, so this examination is useful in the absence of established protective mechanisms.
Studies that can be performed in tandem with antiserum-enabled investigations involve characterizations of the antigens' function(s) in gonococcal physiology and pathogenesis (Figure 2). We discuss these investigations in greater detail in a later section. In broad terms, the experiments associated with functional characterization are designed to assess the effects of conditional or isogenic knockout mutations on the ability of Ng to thrive in vitro under standard conditions as well as during exposure to stimuli relevant to infection, its ability to adhere to and invade epithelial cells, and its ability to colonize female mice during experimental infection in the murine model of lower genital tract infection or transgenic mice (41, 42). The overarching goal of these experiments is to determine the effects of the loss of each vaccine candidate, as antibodies are known to block protein function. In Ng, neutralizing antibodies against the nitrite reductase AniA inhibited its enzymatic activity (105). Neutralizing antibodies were also demonstrated to prevent MetQ-mediated gonococcal adhesion to epithelial cells (106), and were able to abrogate in vitro lysozyme inhibition by the Neisserial adhesin complex protein (ACP), a dual-function protein involved in adhesion and defense against lysozyme attack (99, 107–109). Additionally, Nm and Ng IgA1 protease-mediated IgA cleavage was inhibited by sera collected from both acute and convalescent meningitis patients (110). More specifically, antibodies that block protein function are elicited by immunization with each of the recombinant protein components of the 4CMenB vaccine—factor H binding protein (fHbp), Neisserial heparin binding antigen (NHBA), and Neisseria adhesin A (NadA). Antibodies against fHbp abrogate factor H binding and thus enhance meningococcal serum sensitivity (111). Both NHBA and NadA are involved in bacterial adherence, and neutralizing antibodies against either protein reduce the ability of Nm to adhere to epithelial cells (112, 113). Non-neutralizing antibodies provide protection against viruses (114, 115); however, similar data are scarce for bacteria (114). Targeting bacterial virulence factors or physiologically important proteins with a vaccine could discourage mutations that would allow the protein to evade immune detection, thereby improving the vaccine's success. The exclusive focus on targeting virulence factors in a vaccine is not vital, however, as demonstrated by immunization with the S. agalactiae surface immunogenic protein (Sip), which induces a strong protective response (116) but has no reported function in the pathogenesis of group B streptococci. It is possible that the antigens eventually formulated into a successful gonorrhea vaccine will not generate neutralizing antibodies, but evidence suggests antibodies that block protein function to some extent are elicited in the majority of immune responses effective at protecting against bacterial pathogens.
An additional study that can be used to inform vaccine design involves antigen structural elucidation, either alone or in complex with antibodies directed against the target protein. We discuss structural studies that have been performed on proteome-derived vaccine candidates in a subsequent section. Not only does the structure of a protein give insights into its function, but it also enables allele mapping, as discussed in the previous bioinformatics section (Figure 2). Traditionally, structural characterization is performed through X-ray crystallography (117), although advances in nuclear magnetic resonance spectroscopy also allow for the structural elucidation of small proteins (118, 119). Co-crystallization with antibodies can reveal the mechanism(s) of action of the antibodies against the target protein (120–122). This strategy requires the use of antigen binding fragments of monoclonal antibodies (mAbs), which are more technically challenging, time-consuming, and expensive to produce than polyclonal antibodies (123). For this reason, co-crystallization is likely to be pursued only after further evaluation of the antigen's immunogenicity and protective capabilities as part of a vaccine. A complementary technique that can be pursued for difficult-to-crystallize antigen/antibody complexes is cryo-electron microscopy, in which single molecules embedded in a flash-frozen matrix are visualized with an electron microscope. This technique was successfully employed to elucidate the structure of an integral membrane ion channel protein (124), as well as the interaction interface between an antibody fragment that successfully neutralized a range of influenza virus variants and the receptor site of the influenza virus hemagglutinin protein (125). One limitation of cryo-electron microscopy is that structural resolution tends to be poorer than with the use of other techniques. However, recent technical advances have been able to improve acquired structures to near-atomic resolution [<4Å; (126)]. Although cryo-electron microscopy may circumvent some limitations of X-ray crystallography or nuclear magnetic resonance, mAbs will still be required for evaluating antigen/antibody interactions.
Finally, if the results of the evaluation studies suggest that a protein may be a suitable vaccine component, its immunogenicity and ability to elicit a protective response will be investigated through protection and clearance studies performed in the female mouse model of gonorrhea (Figure 2). To date, only an extremely limited number of studies have been published examining the ability of vaccine formulations to accelerate clearance and protect against subsequent infection in the mouse model. These studies include intranasal immunization with MVs, which was associated with enhanced clearance in one study (127), but not in another (24). However, intravaginal immunization with MVs combined with microencapsulated IL-12 both accelerated gonococcal clearance and protected mice from subsequent infection (52). Active intraperitoneal immunization with a peptide mimic of a conserved LOS epitope recognized by the 2C7 mAb or passive immunization with the mAb itself both shortened disease duration (128). Finally, mice immunized in the rear footpads with viral replicon particles (VRPs; viral derivatives that deliver antigens after a single replication cycle) loaded with the outer membrane porin PorB, combined with a subsequent booster immunization comprised of recombinant refolded PorB and an adjuvant, cleared experimental infections significantly more quickly than control mice (24). Follow up experiments suggested that this protection was likely associated with the adjuvant effect of the VRP itself, rather than a specific protective effect of the PorB antigen (24). These studies, and their paucity, reveal the need to accelerate and expand systematic immunization studies that examine the protective effects of antigens individually and in combination. Additionally, the effects of the presence or absence of adjuvants with each antigen formulation should be examined, as different adjuvants can drive specific adaptive immune responses (Figure 2). Currently, vaccines that induce a balanced Th1/Th2 responses are considered optimal [reviewed in (129)] and are important in defending against gonorrhea (52, 53). Examples of FDA-approved adjuvants that should be evaluated based on these considerations include alum, which drives a Th2 response; monophosphoryl lipid A, a detoxified LPS derivative that acts as a TLR4 agonist to stimulate a Th1 response; and oligonucleotides enriched in cytosine phosphoguanine (CpG) islands (129, 130), which are TLR9 agonists that mediate Th1 and CD8 cellular immune responses but also increase antibody titers (131). To optimize the chance of developing an effective vaccine, the route of immunization, as well as different antigen delivery systems that potentiate immune responses, should be considered and investigated. Numerous delivery systems are in clinical and pre-clinical stages of development, including virus-like particles, emulsions, liposomes, polymer-based systems, hydrogels, and implants [reviewed in (129, 130)]. Finally, evaluation of subunit vaccines beyond their ability to accelerate clearance and protect against subsequent infection will include quantification of antigen-specific IgG and IgA antibody titers in serum and vaginal mucosal secretions, evaluation of antibodies' bactericidal and opsonophagocytic activities, and examination of the cellular immune response.
While we recognize that the decision tree presented here may appear daunting, it is important to remember that traditional vaccinology has failed to deliver a successful gonorrhea vaccine. Development of new vaccines is not trivial. The challenges inherent in protecting against this highly adaptable pathogen necessitate creative and flexible approaches. The decision tree and the generation of effective gonorrhea vaccines will be shaped by lessons learned from the Ng biology and epidemiology as well as new delivery systems and technologies. A strength of our proposed decision tree is that, regardless of whether an antigen is ultimately formulated into a vaccine, more information will be gained into Ng pathophysiology and the gonorrhea research field will be accelerated.
Elucidation of Function of the Proteome-Derived Vaccine Candidates
We propose a vaccine-induced immune response that targets antigens important for CE homeostasis, bacterial pathogenesis, or overall viability may not only protect against the acquisition of subsequent gonorrhea infections but may also enhance immune system efficacy by weakening the gonococcus, thus accelerating the clearance of ongoing infections. A vaccine that employs this strategy could conceivably be used for therapeutic interventions in addition to preventative purposes. Studies evaluating the effectiveness of a vaccine against herpes simplex virus type 2 (HSV-2) provide a precedent for a therapeutic vaccine. In both guinea pigs (132) and humans (133, 134), vaccination against HSV-2 tended to decrease the lesion rate and reduced viral shedding for at least 12 months—an evaluation criterion for efficacy of HSV-2 antiviral agents (134). A neutralizing antibody response was key for the vaccine's immunotherapeutic activity (132). For this reason, determining the function of antigens proposed for inclusion in a vaccine is useful to predict the potential physiological effects of an immune response that blocks protein function. The scientific literature contains a wealth of information that can be used as a starting point to facilitate functional characterization. Numerous gonococcal proteins have homologs that have been investigated in E. coli or Nm. However, these studies should be approached with a modicum of caution, as protein function may differ between species. For example, we determined that LptD is essential in Ng (63), similar to findings in E. coli (135, 136). In contrast, this protein is dispensable for Nm (137). Furthermore, fHbp, which is a surface-exposed protein that contributes to Nm serum resistance, has no signal peptide for outer membrane localization in gonococci, nor does loss of Ng fHbp alter bacterial susceptibility to human serum (138). The potential for distinct protein function, even among closely related species, emphasizes the importance of performing independent studies to examine the role of homologous proteins in the organism being targeted by the vaccine.
A common tool for the study of protein function is a bacterial strain with a knockout mutation in the protein of interest. This can be accomplished through homologous recombination-mediated allelic replacement of the genetic locus with an antibiotic resistance marker, as we have performed in our studies (63, 64, 99), or through gene inactivation by targeted transposon insertion mutagenesis (139). Of course, attempting to knock out an essential gene with this strategy will be unsuccessful, as transformation efforts will not result in any colonies, or off-target mutations may occur that lead to antibiotic resistance but do not affect the target gene. Neither outcome is desirable. Our strategy to circumvent this difficulty has been to place the gene of interest at an unlinked locus under the control of an inducible promoter, then to replace the native gene with an antibiotic resistance cassette while inducing protein expression from the heterologous locus (64, 140, 141). With this technique, the effects of protein depletion, as well as protein stability, can be studied for essential genes. Using knockout mutant strains of non-essential genes, protein function can be assessed by exposing bacteria to different stress conditions and monitoring for growth. We evaluate bacterial survival under conditions relevant to human infection, including iron starvation, exposure to human serum, and anoxia, with the hypothesis that bacteria deficient in proteins important to a rapid or appropriate response to any of the conditions will be non-viable or will not grow as robustly as wild type bacteria (79, 99, 142). CE permeability and stress can be evaluated by exposure to different antibiotics, either with the use of Etest antimicrobial test strips6 or serial dilutions of bacteria inoculated onto solid medium supplemented with antibiotics (63, 79, 99). Additionally, overproduction of MVs is a general marker of CE stress (143–145), so a comparison of MV production between wild type and mutant bacteria can suggest the level of CE stress resulting from the loss of a protein (79). Qualitative or quantitative proteomic profiling of supernatants collected from liquid cultures can give insights into the extent of membrane leakage or cellular lysis associated with deficiency of each vaccine candidate (146, 147). Finally, in vitro tissue culture experiments can inform whether the protein under investigation is involved in, or has substrates that contribute to, the ability of Ng to adhere to, invade, and survive within human cervical epithelial cells (99, 106, 148, 149).
An innovative technique that we adapted for the first study of its kind in Ng is the use of phenotype microarrays [PMs; (147)]. Developed by Biolog7, each PM is a 96-well microtiter plate pre-formulated with varying concentrations of numerous diverse compounds to assess bacterial nutritional requirements and sensitivity to chemical agents, including antibiotics and osmolytes (150, 151). Ng nutritional requirements are well established. Glucose, pyruvate, and lactate are the only carbon sources the gonococcus is able to utilize (152). Therefore, we focused solely on chemical sensitivity PMs to assess the ability of seven vaccine candidates to defend against osmotic shock, as well as their physiological roles during exposure to CE-perturbing agents including metals, antimicrobial peptides, small hydrophobic molecules, and dyes (147). In our investigation with a comprehensive screen of over 1,000 conditions, we discovered 323 conditions that affected at least one of the mutant strains tested. Using these data, we generated a dendrogram based on the similarity between the effects of the loss of each protein, which revealed that the defects associated with knockout mutations of BamG or MlaA were the most distinct from the other five strains tested. The results of PM screening suggested these two antigens would be the most suitable to include in a vaccine, due to the extensive chemical sensitivities associated with the loss of either protein (147).
Although in vitro experiments are useful for predicting protein function, it is impossible to simultaneously account for all factors that will be encountered during infection of the host. For this reason, infection studies in the female mouse model are invaluable (Figure 2). Experimental infections with a single strain can be used to determine whether the bacterial load or infection duration is altered in the absence of a target protein (42, 153). Alternatively, competitive infections between the mutant strain and wild type bacteria can be performed to minimize mouse-to-mouse variation and to directly associate fitness phenotypes with the loss of a protein (153). Finally, to determine whether the protein under investigation contributes to bacterial response to hormones, ovariectomized mice can be utilized to decrease hormonal influence over infection characteristics (43, 153).
The studies outlined above will facilitate vaccine development by identifying antigens with roles in maintaining gonococcal fitness under stress conditions, including during active infections.
Function of Proteomic-Derived Antigens in CE Homeostasis and Nutrient Acquisition
In the following sections, we discuss insights into function-structure of 12 proteomics-derived antigens (Figure 3). We have already verified surface-exposure for a majority of these vaccine candidates, as well as their expression and conservation among diverse Ng isolates. We have also established that BamA, TamA, LptD, MetQ, and NGO2054 elicit bactericidal antibodies that cross-react with heterologous Ng strains (64). Our initial characterization of these vaccine candidates in Ng, in addition to studies of the functions of homologous proteins in Nm show that many of them play different roles in the CE homeostasis or in nutrient acquisition. Maintaining the integrity of the OM, as well as acquiring nutrients, are critical for bacterial survival in hostile environments, such as those encountered during infection of the host. As a gram-negative bacterium, Ng possesses a typical CE, composed of a cytoplasmic or inner membrane, a cell wall made up of peptidoglycan, and an asymmetric OM (Figure 4). The outer membrane of Neisseria spp contains LOS, rather than the more typical LPS, which reflects their niche as mucosal pathogens (154). LOS acts as a buffer region for protection against environmental insult and contributes to pathogenesis through several mechanisms (154, 155).
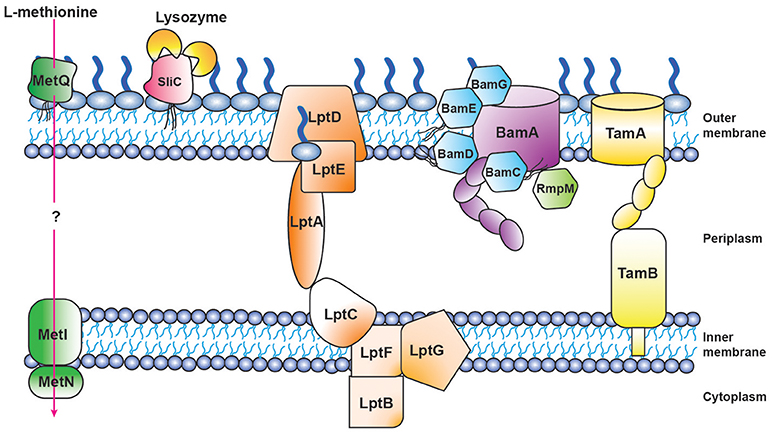
Figure 4. Schematic of proteomics-derived antigens MetQ, SliC, LptD, BAM, and TAM function-structure and organization in Neisseria gonorrhoeae.
Three of our antigens (BamA, BamE, and BamG) are components of the β-barrel assembly machinery (BAM) system (63, 79, 156), which is responsible for folding and inserting β-barrel proteins into the bacterial outer membrane (157, 158). Until recently this critical protein complex has been primarily investigated in E. coli, where it consists of the central β-barrel “Omp85” protein BamA, assisted by four lipoproteins (BamB-E). In contrast to E. coli, gonococcal BAM lacks BamB and contains a surface-displayed BamE [Figure 4; (79)]. Further, Neisseria possess an additional non-essential accessory protein RmpM (159). Similarly to other gram-negative bacteria, BamA and BamD are essential for Ng cell viability, and depletion of either BAM component results in OMP misfolding, as well as defects in protein stability and assembly (64, 160–162). BamA is responsible for folding and inserting virtually all β-barrel OMPs in the OM (158). In contrast, BamE is not essential, which enables study of its effects on the CE. Our comprehensive analyses, including PMs, showed that the loss of BamE renders Ng susceptible to a wide range of compounds including detergents, antimicrobial peptides, other membrane-perturbing agents, and antibiotics (79, 147). Enhanced production of MVs is a marker of CE stress (143). Accordingly, the ΔbamE mutant produced significantly increased amounts of MVs in comparison to WT bacteria. Additionally, the MV protein profile exhibited distinct alterations, suggesting that certain proteins were specifically targeted for packaging into MVs (79). Finally, removal of BamE appeared to destabilize the BamA-D interaction, which resulted in BamD localized on the cell surface of BamE-deprived Ng (79). The aberrant BamD localization in the absence of BamE could further increase membrane perturbations by interference with proper OMP insertion by BamA.
We recently discovered that one of our proteome-derived vaccine candidates, NGO1985, is a previously unrecognized accessory lipoprotein within the BAM complex and therefore renamed this protein BamG (156). Included in BamG is a lipoprotein signal peptide, which targets the protein for surface display (156), as well as two bacterial OsmY and nodulation (BON) domains (Figure 3H). BON domains are proposed to bind phospholipids based on the finding that E. coli OsmY prevents the inner membrane from shrinking during osmotic shock, although this hypothesis was not experimentally verified (163–165). In Ng, our initial probing with detergents and PM analyses revealed an extensive sensitivity phenome associated with lack of BamG, suggesting that BamG, through its interaction with the BAM complex, plays a critical function in CE biogenesis (63, 147, 156).
LptD belongs to the low abundant, large and complex class of BAM substrates that are also heavily dependent on SurA, Skp and FkpA chaperones (166). LptD, together with OM-localized LptE (Figure 4), are constituents of the lipopolysaccharide transport (LptA-F) complex, and are crucial for inserting LPS into the outer leaflet of the E. coli outer membrane (167). The Lpt system appears not to function in a completely conserved manner in gram-negative bacteria, as both Ng and E. coli LptD are essential (63), whereas Nm can survive without LOS and none of the Lpt components are important for bacterial viability (168).
For the bacterial CE to exert its barrier function, the outer leaflet of the outer membrane must be a homogeneous layer of LOS. If this LOS layer is disrupted, phospholipids may diffuse from the inner leaflet and weaken the barrier function of the outer membrane. The Mla (maintenance of lipid asymmetry) system, which is conserved across gram-negative bacteria and is composed of MlaA-F, removes misplaced phospholipids from the outer leaflet and re-integrates them into the inner membrane to mitigate the detrimental effects of altered membrane asymmetry. The outer membrane component of the Mla system, MlaA, has primarily been studied in E. coli, where its deletion enhances bacterial susceptibility to membrane perturbing agents (169). In Ng, MlaA is encoded by the ngo2121 locus and comprises a signal peptide and a MlaA domain (Figure 3F). Gonococcal MlaA is annotated as a lipoprotein, although we have previously noted that it does not contain the invariant cysteine residue required for lipid modification (147). However, a lipoprotein signal peptide is present in homologs from E. coli, K. pneumoniae, and S. marcescens (169, 170). We are currently investigating the implications of the lack of MlaA lipidation in Ng. Combining antibiotic susceptibility testing using Etests with PM screening, we have established that compounds targeting the CE, including antimicrobial peptides, are more effective against Ng lacking MlaA than wild type bacteria. Resistance to antibiotics acting against cytoplasmic targets was unaltered (63, 147, 171), suggesting a specific outer membrane defect (169). Additionally, the ΔmlaA knockout showed increased vulnerability to oxidative stress-inducing compounds (147) and produced more MVs than wild type bacteria (171). Together, these findings suggest that MlaA is required for maintenance of outer membrane homeostasis and is involved in MV biogenesis (172).
Bacterial lipoproteins play numerous roles in cellular physiology, adhesion to host cells, modulation of inflammatory processes, and transport of virulence factors into host cells. Proper lipoprotein localization is critical for protein function (173). The proteome-derived vaccine candidates BamE, BamG, SliC, MetQ, and NGO2054 are newly described Ng lipoproteins (Figure 3). NGO2111 is homologous to the Nm surface lipoprotein assembly modulator Slam2, which is involved in translocating the hemoglobin-haptoglobin utilization protein to the cell surface (174). Slam2 is highly conserved amongst Neisseria isolates and is not found in E. coli (175, 176). Ng Slam2 contains a signal peptide and a 14-stranded β-barrel domain, which has also been annotated as a DUF560 domain (Figure 3D; 147, 176). In our PM study, we found no conditions that uniquely affected a Δslam2 mutant, although 36 compounds were either beneficial or detrimental to the growth of this strain, in common with one or more of 6 other mutants (147). These results suggested that Slam2 exerts an indirect effect on CE integrity, potentially through an undiscovered lipoprotein substrate.
Another gonorrhea vaccine candidate we investigated in our PM study was NGO1344, which contains an AsmA domain (Figure 3L) and is homologous to the AsmA protein from E. coli and Nm. In E. coli, loss of AsmA decreased LPS synthesis (177); however, a similar phenotype was not observed in a Nm ΔasmA mutant (178). Our analysis of cell lysis indicated that loss of Ng AsmA resulted in elevated levels of several cytoplasmic proteins in culture supernatants, especially when grown in the chemically-defined Graver-Wade liquid medium (147). Fifty-three membrane-perturbing compounds affected the viability of a ΔasmA mutant, including polymyxin B and bile salts (63, 147). Clustering analyses suggested that the loss of AsmA resulted in phenotypes similar to those observed upon BamE deletion (147). Our results indicate that gonococcal AsmA contributes to CE integrity.
We also examined the role of the small lipoprotein NGO2054 in CE homeostasis. This protein is comprised of a lipoprotein signal peptide, a Lambda-Bor motif that contributes to serum resistance in E. coli (179), and a region with homology to the C-terminus of the fragile site-associated protein involved in adipocyte differentiation in mammalian cells [Figure 3I; (180)]. A Δngo2054 mutant was the least affected in our PM screen; only 27 compounds altered its growth (147). Thus, although we have established that NGO2054 is surface exposed, well conserved, and elicits bactericidal antibodies (64), its function within the CE remains enigmatic.
Metal co-factors such as zinc and iron are critical to facilitate cellular and enzymatic processes within pathogenic bacteria. During infection, bacteria rely on scavenging these metals from the host. To combat against bacterial pathogens, host organisms sequester available metals at the site of infection, a strategy termed nutritional immunity. The Neisserial outer membrane zinc uptake protein ZnuD overcomes host-imposed zinc depletion (181). In Ng, ZnuD is encoded by the ngo1205 locus, which includes an amino terminal signal peptide, a plug domain, as well as a TonB β-barrel (Figure 3E). In support of its role as a zinc uptake protein, a Nm ΔznuD mutant was more sensitive to the bactericidal effects of neutrophil extracellular traps (182), which contain calprotectin that sequesters zinc ions (183). Additionally, meningococci deficient in ZnuD were attenuated in a systemic infection model (181), although ΔznuD gonococci exhibited no survival defect during intracellular infection of cervical epithelial cells (184). We performed our PM analysis on Ng ΔznuD bacteria to examine the contribution of ZnuD to CE integrity. Thirty-seven conditions altered the growth of bacteria lacking ZnuD, including the divalent cation chelator ethylenediaminetetraacetic acid (147). Our results provide support for the role of ZnuD as a zinc uptake protein and suggest that downstream effects to the CE occur from the decreased ability to acquire zinc.
Translocation of solutes across the CE is mediated by ABC transporters, which utilize the hydrolysis of ATP to transport molecules. A substrate binding protein is necessary to capture the substrate. Our proteome-derived vaccine candidate MetQ (63, 64), which was highly conserved in Ng as well as Nm (55, 64), is homologous to the E. coli methionine binding protein MetQ [also known as NlpA; (106)] and is annotated with a lipoprotein signal peptide and a NlpA domain (Figure 3K). The presence of an operon upstream from metQ, composed of an ATP-binding protein (MetN) and a transmembrane permease (MetI) (106), provides additional support for the role of MetQ as the substrate binding protein in an ABC transport system. Surface plasmon resonance experiments demonstrated that Ng MetQ binds L-methionine with nanomolar affinity (106). These findings, in combination with our experiments demonstrating that Ng MetQ is surface exposed, highly conserved, and elicits strongly bactericidal antibodies (64), suggest MetQ is a promising vaccine candidate. Targeting MetQ could interfere with methionine transport and result in downstream protein synthesis defects.
The studies described here illustrate the myriad cellular processes in which our proteome-derived vaccine candidates participate. A subunit vaccine composed of several of these antigens has the potential to compromise gonococcal fitness independent of factors beyond a neutralizing antibody response.
Function of SliC and MetQ in Pathogenesis
Bacterial pathogenicity is influenced by the number of infecting bacteria, the route of entry, the presence of host defense barriers, and bacterial virulence factors. Ng pathogenesis involves a collection of factors: pili, Opa proteins, LOS, and peptidoglycan all contribute to infection and are important for optimal pathogenesis (6). The study of protein function has the potential to reveal previously unrecognized virulence factors. A perfect example is our discovery of the surface-exposed inhibitor of C-type lysozyme, SliC (99). Through bioinformatic analyses, we determined SliC, encoded by the ngo1063 locus, contained a lipoprotein signal peptide and a domain similar to membrane-bound lysozyme inhibitors of C-type lysozyme (MliC domain; Figure 3J). Bacterial proteinaceous lysozyme inhibitors protect the cell wall against host lysozyme attack during infection (185). Lysozyme inhibitor proteins were not known to exist in Neisseria until the recent discovery that the Neisserial adhesin complex protein (ACP) is inhibitory toward human (HL) and hen egg white lysozyme (HEWL), both of which are C-type lysozymes (108). The activity of SliC as a lysozyme inhibitor was comprehensively examined using an assay that involved fluorescently labeled peptidoglycan. Pre-incubation of wild type SliC with HL or HEWL completely obstructed peptidoglycan hydrolysis. In contrast, SliC mutated in two residues predicted to be key for the protein's interaction with lysozyme (S83A and K103A) showed no inhibition of cell wall hydrolysis with the addition of HL or HEWL (99). Subsequently, bio-layer interferometry was used to assess the kinetic interaction between SliC and lysozyme. This analysis revealed moderate binding between SliC and HL in vitro (KD 11 μM), with one SliC molecule binding two HL molecules [Figure 4; (99)]. Surprisingly, cells that lacked SliC showed no difference to wild type when exposed to increasing levels of HL in vitro. However, a double SliC/ACP knockout was strongly attenuated during HL exposure (99, 109), suggesting that one lysozyme inhibitor compensates for the lack of the other protein. Despite this compensatory activity, Ng bacteria lacking SliC were up to 250-fold less fit during a competitive infection with WT bacteria in the mouse model (99). Further, bacteria complemented with a SliC S83A/K103A mutant were also significantly attenuated during competitive infections (99). In vitro adhesion and invasion assays revealed no difference between ΔsliC and WT bacteria, which indicated that the attenuation observed in the mouse was not due to defects in the mutant's ability to adhere to or invade epithelial cells. Finally, ΔsliC bacteria were as fit as WT during competitive infections in a lysozyme-deficient mouse, which provided conclusive evidence that the inhibitory activity of SliC against host lysozyme is critical for gonococcal colonization (99).
Substrate binding components of ABC transporters may be localized to the outer surface and may contribute to cell adherence (186, 187). MetQ from Ng was therefore assessed for its ability to mediate bacterial adhesion and invasion of cervical epithelial cells. Gonococci lacking MetQ exhibited a 2.4-fold decrease in adherence and a 1.5-fold lower level of invasion compared to the wild type strain. These findings were comparable to the level of adherence and invasion within transformed primary cervical epithelial cells (106). Additionally, bacterial survival in primary monocytes and macrophages was evaluated, to determine whether MetQ exerts a protective role against immune cells or other similar factors. Cells lacking MetQ displayed a 2.3-fold reduction of viability in primary monocytes and a 1.5-fold decline in macrophages. MetQ-deficient gonococci were also significantly attenuated upon exposure to human serum (106). The results of this study indicated MetQ, in addition to its role as a methionine transport protein, contributes to the ability of Ng to adhere to and invade epithelial cells and protects against the innate immune system.
The study of vaccine candidates' contributions to bacterial pathogenesis benefits vaccine development by revealing potential vulnerabilities that can be exploited through rational vaccine design to cripple the invading bacterial pathogen.
Structure of Proteomic-derived Antigens
Structure-based antigen design offers new possibilities in vaccine development and improvement by delivering novel immunogens and informing about protective epitopes (188). This approach, in combination with sequencing data and computational biology studies (189), can drive rational optimization of vaccines as we discussed above (Figure 2). In this section, we will focus on summarizing structural investigations that have been performed on our proteome-derived vaccine candidates. To this end, only the structures of Ng BamA and BamE have been solved (79, 190). However, Nm crystal structures of MetQ and ZnuD are available in addition to LptD, BamA, BamE, and TamA obtained from different bacterial species, all of which provide information about antigen architecture and conformational conservation.
The first BamA crystal structure was solved from Ng and revealed a 16-strand β-barrel domain within the outer membrane, connected to five N-terminal periplasmic polypeptide transport-associated (POTRA) domains [Figure 3A; (190)]. The BamA crystal structure from E. coli displayed a high level of flexibility between POTRA5 and the β-barrel domain, suggesting that the POTRA domains assist in transferring the substrate to the β-barrel domain of BamA (191). A prominent difference was found in BamA conformation in Ng compared to BamA in Haemophilus ducreyi, where the last β-strands within the barrel are tightly meshed with hydrogen bonds, providing more rigidity. However, in Ng, the last β-strand is bound to the first by only two hydrogen bonds, which allows the pore itself to twist. The POTRA5 domain of BamA in Ng is positioned closely to the barrel, with periplasmic loops 3, 4, 5, and 7 stabilizing the closed conformation. This is highly different in H. ducreyi, as the POTRA region hinges outwards ~70°, which does not allow POTRA5 to interact with the β-barrel periplasmic loops (190). The difference in the interaction between POTRA5 and the β-barrel may act as a secondary mechanism to prevent unregulated solute entry into the pore and may compensate for the lack of hydrogen bonding in Ng BamA. Further analysis of the Ng BamA crystal structure revealed the hydrophobic belt along the C-terminal strand was narrower (~9Å) than the opposite side of the barrel [~20 Å; (190)]. The authors of this study hypothesize that this reduced width may disrupt the lipid membrane environment and act to allow easier insertion of the OMP into the membrane (190). Based on the configuration of BamA, two hypotheses have been proposed for the mechanism of OMP folding and insertion, depending on the complexity of the substrate protein. Complex proteins take advantage of a lateral opening event facilitated by a conformational switch of loop 6 and the gating motion of the POTRA domains. The nascent OMP is threaded through the β-barrel and uses exposed strands of BamA as a template for proper barrel formation through a transient OMP-BamA complex until the new OMP buds off into the OM. Simple substrates may bypass the BamA β-barrel completely and may be inserted directly into the destabilized portions of the membrane through their interactions with the POTRA domains (190). Recent studies suggest that OMP binding to BamD induces conformational changes in the extracellular loops of BamA for substrate folding and membrane insertion (192). Thus, antibodies against these extracellular loops may interfere with proper OM biogenesis and cause severe downstream effects to OM integrity.
Gonococcal BamE includes a lipoprotein signal peptide, as well as a predicted SmpA_OmlA domain [Figure 3G; (193)] In E. coli, X-ray crystallography showed BamE interacts exclusively with BamA, and does not contact other accessory lipoproteins. Instead, BamE directly assists OMP folding through its interaction with BamA. In contrast to E. coli, where the native form of BamE appears to be a periplasmic monomer (194), Ng BamE is a surface-exposed dimer that also includes an additional C-terminal helix not present in other solved structures of BamE (79). Isolation of proteins from native membranes should definitively establish which conformation is the active state. The dimeric form of BamE possesses structural homology to β-lactamase inhibitors, which has led to the hypothesis that BamE has a secondary function as a β-lactamase inhibitor (195, 196). Surface-exposed dimers of BamE in Ng may therefore act as a first line of defense against β-lactam antimicrobials. In support of this secondary function of BamE, Ng ΔbamE mutants were more sensitive to several different β-lactam antimicrobials (79, 147). Further investigation will be required to determine whether BamE contributes to antibiotic resistance. If BamE does in fact possess dual functions, a neutralizing immune response could both interfere with OM integrity as well as enhance antibacterial efficacy.
Autotransporter biogenesis relies on a passenger-transport complex to assist in the translocation of autotransporters across the outer membrane. The translocation and assembly module (TAM) comprises a two-membrane complex containing a β-barrel OMP TamA and inner membrane protein TamB, a nanomachine required for virulence of pathogenic bacteria [Figure 4; (197)]. This complex facilitates the folding and insertion of the autotransporter domain and has been hypothesized to assist in proper effector domain folding. Effector domains are responsible for protein activity and frequently contribute to virulence (198). Consistent with its role in translocation, TamA contains a β-barrel structure homologous to BamA (199) and has been hypothesized to be the result of a duplication event that arose from the evolutionary convergence of BamA and TamB (200). In E.coli, the TamA structure was determined at 2.25 Å resolution, revealing a sixteen stranded β-barrel ring structure from amino acids 265-577 on the C-terminus, and three POTRA domains between amino acids 22-264 on the N-terminus [Figure 3; (201)]. The POTRA domains wind ~50Å, oriented toward the periplasm in a semi-circle arrangement (201). The initial contact between substrate and TamA is facilitated by interactions between TamB and the POTRA domains, which act as a hinge mechanism (197, 200). Additional crystal structure analysis showed a kink in the C-terminal β-strand pointing inward, which weakened the lateral wall, implying a possible gate for substrates to route toward the lipid bilayer (201). The kink resulted in a weak β-strand pair of strand 1 and 16, because of three main chain hydrogen bonds. The kink on β-strand 16 results in a large gap between the ends of strands 1 and 15 near the POTRA3 attachment site. This cleft promotes insertion of the substrate, mediated by interactions between the β-barrel and the POTRA domains. The folding and insertion process is hypothesized to begin when an autotransporter's β-barrel domain engages with POTRA3 within the periplasm and is guided toward the barrel of TamA. The structure of TamA also includes a closed extracellular lid from amino acids 456-495. Salt bridge interactions between the lid and the β-barrel are mediated by Arg477 in the lid and Asp521 in the barrel (201). Targeting the epitopes responsible for stabilizing the interaction between lid and β-barrel with an immune response could interfere with proper TamA function.
The crystal structures of the core LPS assembly LptDE protein complexes from several bacteria, including medically important pathogens, have been obtained, with only two full-length structures of Shigella flexneri and Klebsiella pneumoniae LptDE (167, 202). Overall, these studies revealed a strong structural conservation of the two-protein plug and barrel assembly and demonstrated that LptE was integrated within the 26-stranded, C-shaped β-barrel architecture of LptD. All LptDE structures have a negatively charged lumen which may facilitate LPS/LOS insertion (202). Additionally, LptD contains a periplasmic β-jellyroll domain, which is structurally similar to LptA and facilitates LPS/LOS transit through the periplasm [Figure 3C; (167)]. The N terminal domain of LptD undergoes a 21° rotation, which may aid assembly or influence flexibility of the LptCAD scaffold (202). The LptD β-barrel is large compared to other β-barrel OMPs. Its dimensions accommodate large substrates and facilitate efficient transport. Further, a kink was present in the first two β-strands of the β-barrel due to two proline residues in β1 and β2 (167), and they have been experimentally verified to play a pivotal function in the lateral opening of the barrel (202). The interaction between LptD and LptE is dependent on polar connections within the LptD β-barrel inner cavity; thus, LptE supports LptD structural maintenance, as well as export of LPS to the outer leaflet. Disruption of the interaction between LptD and LptE would be catastrophic to proper Lpt complex function (167). Due to the significance of LOS, immune system interference with LptD, and thus LOS transport, would severely alter gonococcal CE composition, weakening the bacterium and reducing its pathogenic capabilities.
The crystal structure of the outer membrane component of the MlaA-F system, MlaA, was recently attained from X-ray crystallography of MlaA proteins from Serratia marcescens and K. pneumoniae. MlaA is a monomeric α-helical OMP. Its six amphipathic α-helices facilitate the transport of polar phospholipid headgroups while residing within the hydrophobic interior of the outer membrane. The structure of MlaA allows diffusion of outer leaflet phospholipids exclusively (170). It is unclear how the phospholipid is transferred to MlaC, as the MlaA pore does not appear to permit the passage of acyl chains. Interactions between MlaA and MlaC have been hypothesized to induce a conformational change in MlaA, potentially by shifting helix 6 through a gate opening mechanism to enable phospholipid transfer to occur (170). K. pneumoniae and S. marcescens MlaA proteins were found in complex with the outer membrane pore OmpF in a 3:3 or a 1:3 ratio, respectively (170). The MlaA-porin interplay, mediated by van der Waals interactions, does not appear to significantly influence porin, although the presence of the porin appears to prevent MlaA aggregation (170). Normal function is based on proper architecture of the interaction and linkage of these two proteins. Targeting the maintenance of OM lipid asymmetry through a vaccine could sensitize bacteria to components of the immune system that target the outer membrane.
Neisserial MetQ was originally believed to bind to D-methionine (203, 204). However, structural elucidation from Nm revealed L-methionine in the binding pocket, which could not be displaced, even when the protein was heterologously expressed in E. coli in minimal medium containing only D-methionine. In support of these findings, and in contrast to the protein's annotation as a D-methionine binding protein, concrete data indicate that D-amino acids are not incorporated into proteins during ribosomal synthesis; L-amino acids are required (205). Biologically, aminoacyl-tRNA synthetases distinguish cognate L-amino acids against noncognate proteinogenic L-amino acids and also nonproteinogenic D-amino acids, thus regulating components for protein biosynthesis (206). However, E. coli MetQ is able to bind both D- and L-enantiomers with high affinity (207). Structural comparisons revealed similarities between Nm MetQ and L-methionine binding protein Tp32 from Treponema pallidum as well as the dipeptide GlyMet-binding protein Pg110 from Staphylococcus aureus, despite low sequence similarity (204). Nm MetQ is made up of 15 alpha helices and 10 β-strands, split into two domains, I and II, corresponding to residues 43-119 and 236-281; and 120-235, respectively. The two domains are connected by a hinge region and display a Venus flytrap-like structure, which is typical of periplasmic substrate binding proteins. The methionine binding site is within the crevasse of these two globular lobes (204). Residues critical for proper methionine coordination within the binding pocket include Arg156, which forms a salt bridge with the carboxyl group of L-methionine; Asn215, which forms one of two hydrogen bonds to the nitrogen of L-methionine; and Asn 238, which forms the second hydrogen bond and appears to be responsible for the stereospecificity of Neisserial MetQ (204). MetQ is a surface-displayed lipoprotein (64, 106) and thus it remains to be elucidated how L-methinonine is transported through the periplasm to the inner membrane (Figure 4).
The structure of ZnuD, a member of the TonB-dependent receptor (TbdR) family, was first crystalized in Nm by single isomorphous replacement, combined with opposing signals for native and seleno-derived ZnuD crystals (181). The crystal structure of ZnuD revealed a 22-stranded β-barrel pore architecture similar to that of siderophore domains common to the TbdR family, which includes the amino-terminal plug domain between residues 1-147 and its pore-forming domain from residues 148-734 on the carboxyl terminus [Figure 3E; (181)]. The plug domain of ZnuD is required for normal function of TonB in the inner membrane. In contrast to most TbdR proteins, which are involved in iron and cobalamin uptake, ZnuD was the first zinc TbdR structure solved (208). Due to the importance of metal co-factor acquisition, other gram-negative bacteria like E.coli use ZnuD ABC transporters to overcome zinc-deficient conditions (209). A key feature of meningococcal ZnuD is the extracellular loops. These loops are arranged in a way that displays a “comb” scaffold, allowing the uptake of zinc. Substrate binding can induce multiple conformational changes that are reversed upon zinc release (181). Key residues assisting in coordination of the zinc ion are Asp99, His100, Glu340 and His499. All four residues are highly conserved within the binding pocket across three intermediate structures (native ZnuD, ZnuD co-crystallized with cadmium, and ZnuD soaked with zinc). The binding pocket is sealed through interactions between the apical loop of the plug and extracellular loop 6. Due to the flexibility of ZnuD, remodeling of the alpha helices and β-strands occurs throughout different binding states. Zinc transport through the β-barrel channel usually requires TonB activation (181). Molecular dynamic simulations found TonB exclusively interacts with the plug domain, which is unfolded upon TonB activation (181). Further investigation using X-ray absorption spectroscopy was performed to determine whether ZnuD specifically binds zinc, or if it interacts with heme as well (181). Superimposition of the ZnuD crystal framework with the hemophore receptor HasR found a similar “lock-key” feature, although ZnuD was determined not to be a heme uptake protein (181, 210). Importantly, meningococcal ZnuD stimulates a bactericidal antibody response that recognizes peptides 233-309, 430-459, and 706-722. These peptides correspond to extracellular loops 3, 6, and 11. Loop 3 is the most immunogenic and performs a pivotal role in blocking access to the zinc binding pocket. A secondary feature of loop 3 is that it shifts to a rigid β-strand conformation upon zinc binding and back to a flexible alpha helix when the substrate is released (181). Despite the significant Nm ZnuD conformational changes observed upon zinc binding, its ability to elicit bactericidal antibodies suggests it may be an appropriate vaccine candidate for gonorrhea as well.
The structural studies we have discussed here not only give context to the function of our proteome-derived vaccine candidates, but also give insights into critical, surface-exposed portions of the proteins that can be targeted through structural vaccinology approaches.
Concluding Remarks
• Proteomics-driven vaccinology for gonorrhea has begun to deliver novel antigens and determined the contents of NeMVs from four different Ng isolates, which can further inform the vaccine development and manufacturing processes.
• Proteomics-driven antigen discovery should be paired with comprehensive bioinformatic analyses to enable more informed decisions for rational development of subunit vaccines and facilitate the inclusion of highly conserved surface-exposed proteins with important functions.
• Our proposed approach to vaccine candidate evaluation may facilitate development of the most effective vaccine against gonorrhea in a systematic and cost-effective way suitable for an academic setting.
• The proteomics-derived antigens described participate in essential CE processes as well as pathogenesis. A subunit vaccine composed of several of these antigens has the potential to severely compromise Ng fitness.
• Our discovery of SliC as a previously uncharacterized virulence factor illustrates that new lessons can still be learned about Ng biology and also highlights the importance of considering that infection occurs in a living host and involves numerous elements that cannot be replicated in vitro.
• Structural vaccinology for gonorrhea is in its infancy and thus enhanced efforts should be dedicated to solving structures of all potential vaccine candidates.
Author Contributions
FM, BB, and AS wrote the manuscript. BB and AS made final edits. AS provided illustrations.
Funding
Funding was provided to AS by grant R01-AI117235 from the National Institute of Allergy & Infectious Diseases, National Institutes of Health.
Conflict of Interest Statement
The authors declare that the research was conducted in the absence of any commercial or financial relationships that could be construed as a potential conflict of interest.
The handling Editor declared a past co-authorship with one of the authors AS.
Footnotes
1. ^http://www.cbs.dtu.dk/services/SignalP/
2. ^http://www.cbs.dtu.dk/services/LipoP/
3. ^https://www.ncbi.nlm.nih.gov/COG/
4. ^https://pubmlst.org/neisseria/
5. ^http://www.cbs.dtu.dk/services/TMHMM/
6. ^http://www.biomerieux-usa.com/clinical/etest
7. ^https://biolog.com/products-portfolio-overview/phenotype-microarrays-for-microbial-cells/
References
1. Newman L, Rowley J, Vander Hoorn S, Wijesooriya NS, Unemo M, Low N, et al. Global estimates of the prevalence and incidence of four curable sexually transmitted infections in 2012 based on systematic review and global reporting. PLoS ONE (2015) 10:e0143304. doi: 10.1371/journal.pone.0143304
2. Farley TA, Cohen DA, Elkins W. Asymptomatic sexually transmitted diseases: the case for screening. Prev Med (2003) 36:502–9. doi: 10.1016/S0091-7435(02)00058-0
4. WHO (2011). Prevalence and Incidence of Selected Sexually Transmitted Infections. Chlamydia Trachomatis, Neisseria Gonorrhoeae, Syphilis and Trichomonas vaginalis. Methods and Results Used by WHO to Generate 2005 Estimates. WHO, 36.
5. Yvert F, Frost E, Walter P, Gass R, Ivanoff B. Prepartal infection of the placenta with Neisseria gonorrhoeae. Genitourin Med. (1985) 61:103–5. doi: 10.1136/sti.61.2.103
6. Edwards JL, Apicella MA. The molecular mechanisms used by Neisseria gonorrhoeae to initiate infection differ between men and women. Clin Microbiol Rev. (2004) 17:965–81. doi: 10.1128/CMR.17.4.965-981.2004
7. Coutanceau B, Boujenah J, Poncelet C. Gonococcal chorioamnionitis with antepartum fetal death in utero. Case Rep Obstet Gynecol. (2015) 2015:451247. doi: 10.1155/2015/451247
8. Woods CR. Gonococcal infections in neonates and young children. Semin Pediatr Infect Dis (2005) 16:258–70. doi: 10.1053/j.spid.2005.06.006
9. Johnson PJ, Stringer VA, Shafer WM. Off-target gene regulation mediated by transcriptional repressors of antimicrobial efflux pump genes in Neisseria gonorrhoeae. Antimicrob Agents Chemother. (2011) 55:2559–65. doi: 10.1128/AAC.00010-11
10. Campbell MF. The surgical pathology of epididymitis. Ann Surg. (1928) 88:98–111. doi: 10.1097/00000658-192807000-00012
11. Trojian TH, Lishnak TS, Heiman D. Epididymitis and orchitis: an overview. Am Fam Physician (2009) 79:583–7.
13. Miettinen A, Heinonen PK, Teisala K, Hakkarainen K, Punnonen R. Serologic evidence for the role of Chlamydia trachomatis, Neisseria gonorrhoeae, and Mycoplasma hominis in the etiology of tubal factor infertility and ectopic pregnancy. Sex Transm Dis. (1990) 17:10–4. doi: 10.1097/00007435-199017010-00003
14. Tapsall JW. Antibiotic resistance in Neisseria gonorrhoeae. Clin Infect Dis. (2005) 41(Suppl. 4):S263–268. doi: 10.1086/430787
15. Unemo M, Shafer WM. Antimicrobial resistance in Neisseria gonorrhoeae in the 21st century: past, evolution, and future. Clin Microbiol Rev. (2014) 27:587–613. doi: 10.1128/CMR.00010-14
16. Unemo M, Del Rio C, Shafer WM. Antimicrobial resistance expressed by Neisseria gonorrhoeae: a major global public health problem in the 21st century. Microbiol Spectr. (2016a) 4:213–37. doi: 10.1128/microbiolspec.EI10-0009-2015
17. CDC. Gonococcal Infections - 2015 STD Treatment Guidelines [Online]. (2015). Available online at: https://www.cdc.gov/std/tg2015/gonorrhea.htm
18. Workowski KA. Centers for disease control and prevention sexually transmitted diseases treatment guidelines. Clin Infect Dis. (2015) 61(Suppl. 8):S759–762. doi: 10.1093/cid/civ771
19. Fifer H, Natarajan U, Jones L, Alexander S, Hughes G, Golparian D, et al. Failure of dual antimicrobial therapy in treatment of gonorrhea. N Engl J Med. (2016) 374:2504–6. doi: 10.1056/NEJMc1512757
20. Wi T, Lahra MM, Ndowa F, Bala M, Dillon JR, Ramon-Pardo P, et al. Antimicrobial resistance in Neisseria gonorrhoeae: global surveillance and a call for international collaborative action. PLoS Med. (2017) 14:e1002344. doi: 10.1371/journal.pmed.1002344
21. Greenberg L, Diena BB, Ashton FA, Wallace R, Kenny CP, Znamirowski R, et al. Gonococcal vaccine studies in Inuvik. Can J Public Health (1974) 65:29–33.
23. Boslego JW, Tramont EC, Chung RC, McChesney DG, Ciak J, Sadoff JC, et al. Efficacy trial of a parenteral gonococcal pilus vaccine in men. Vaccine (1991) 9:154–62. doi: 10.1016/0264-410X(91)90147-X
24. Zhu W, Chen CJ, Thomas CE, Anderson JE, Jerse AE, Sparling PF. Vaccines for gonorrhea: can we rise to the challenge? Front Microbiol. (2011) 2:124. doi: 10.3389/fmicb.2011.00124
25. Hagblom P, Segal E, Billyard E, So M. Intragenic recombination leads to pilus antigenic variation in Neisseria gonorrhoeae. Nature (1985) 315:156–8. doi: 10.1038/315156a0
26. Haas R, Veit S, Meyer TF. Silent pilin genes of Neisseria gonorrhoeae MS11 and the occurrence of related hypervariant sequences among other gonococcal isolates. Mol Microbiol. (1992) 6:197–208. doi: 10.1111/j.1365-2958.1992.tb02001.x
27. Criss AK, Kline KA, Seifert HS. The frequency and rate of pilin antigenic variation in Neisseria gonorrhoeae. Mol Microbiol. (2005) 58:510–9. doi: 10.1111/j.1365-2958.2005.04838.x
28. Hill SA, Davies JK. Pilin gene variation in Neisseria gonorrhoeae: reassessing the old paradigms. FEMS Microbiol Rev (2009) 33:521–30. doi: 10.1111/j.1574-6976.2009.00171.x
29. Swanson J, Robbins K, Barrera O, Corwin D, Boslego J, Ciak J, et al. Gonococcal pilin variants in experimental gonorrhea. J Exp Med. (1987a) 165:1344–57. doi: 10.1084/jem.165.5.1344
30. Swanson J, Robbins K, Barrera O, Koomey JM. Gene conversion variations generate structurally distinct pilin polypeptides in Neisseria gonorrhoeae. J Exp Med. (1987b) 165:1016–25. doi: 10.1084/jem.165.4.1016
31. Seifert HS, Wright CJ, Jerse AE, Cohen MS, Cannon JG. Multiple gonococcal pilin antigenic variants are produced during experimental human infections. J Clin Invest. (1994) 93:2744–9. doi: 10.1172/JCI117290
32. Segal E, Billyard E, So M, Storzbach S, Meyer TF. Role of chromosomal rearrangement in N. gonorrhoeae pilus phase variation. Cell (1985) 40:293–300. doi: 10.1016/0092-8674(85)90143-6
33. Rice PA, Vayo HE, Tam MR, Blake MS. Immunoglobulin G antibodies directed against protein III block killing of serum-resistant Neisseria gonorrhoeae by immune serum. J Exp Med. (1986) 164:1735–48. doi: 10.1084/jem.164.5.1735
34. Li G, Xie R, Zhu X, Mao Y, Liu S, Jiao H, et al. Antibodies with higher bactericidal activity induced by a Neisseria gonorrhoeae Rmp deletion mutant strain. PLoS ONE (2014) 9:e90525. doi: 10.1371/journal.pone.0090525
35. Kraus SJ, Brown WJ, Arko RJ. Acquired and natural immunity to gonococcal infection in chimpanzees. J Clin Invest. (1975) 55:1349–56. doi: 10.1172/JCI108054
36. Plummer FA, Simonsen JN, Chubb H, Slaney L, Kimata J, Bosire M, et al. Epidemiologic evidence for the development of serovar-specific immunity after gonococcal infection. J Clin Invest. (1989) 83:1472–6. doi: 10.1172/JCI114040
37. Fox KK, Thomas JC, Weiner DH, Davis RH, Sparling PF, Cohen MS. Longitudinal evaluation of serovar-specific immunity to Neisseria gonorrhoeae. Am J Epidemiol. (1999) 149:353–8. doi: 10.1093/oxfordjournals.aje.a009820
38. Schmidt KA, Schneider H, Lindstrom JA, Boslego JW, Warren RA, Van de Verg L, et al. Experimental gonococcal urethritis and reinfection with homologous gonococci in male volunteers. Sex Transm Dis. (2001) 28:555–564. doi: 10.1097/00007435-200110000-00001
39. Frasch CE, Borrow R, Donnelly J. Bactericidal antibody is the immunologic surrogate of protection against meningococcal disease. Vaccine (2009) 27(Suppl. 2):B112–116. doi: 10.1016/j.vaccine.2009.04.065
40. Granoff DM. Relative importance of complement-mediated bactericidal and opsonic activity for protection against meningococcal disease. Vaccine (2009) 27(Suppl. 2):B117–125. doi: 10.1016/j.vaccine.2009.04.066
41. Rice PA, Shafer WM, Ram S, Jerse AE. Neisseria gonorrhoeae: drug resistance, mouse models, and vaccine development. Annu Rev Microbiol. (2017) 71:665–86. doi: 10.1146/annurev-micro-090816-093530
42. Jerse AE. Experimental gonococcal genital tract infection and opacity protein expression in estradiol-treated mice. Infect Immun. (1999) 67:5699–708.
43. Jerse AE, Wu H, Packiam M, Vonck RA, Begum AA, Garvin LE. Estradiol-treated female mice as surrogate hosts for Neisseria gonorrhoeae genital tract infections. Front Microbiol. (2011) 2:107. doi: 10.3389/fmicb.2011.00107
44. Jerse AE, Deal CD. Vaccine research for gonococcal infections: where are we? Sex Transm Infect. (2013) 89(Suppl. 4):iv63–68. doi: 10.1136/sextrans-2013-051225
45. Ouyang W, Kolls JK, Zheng Y. The biological functions of T helper 17 cell effector cytokines in inflammation. Immunity (2008) 28:454–67. doi: 10.1016/j.immuni.2008.03.004
46. Feinen B, Jerse AE, Gaffen SL, Russell MW. Critical role of Th17 responses in a murine model of Neisseria gonorrhoeae genital infection. Mucosal Immunol. (2010) 3:312–21. doi: 10.1038/mi.2009.139
47. Liu Y, Russell MW. Diversion of the immune response to Neisseria gonorrhoeae from Th17 to Th1/Th2 by treatment with anti-transforming growth factor beta antibody generates immunological memory and protective immunity. MBio (2011) 2:e00095–00011. doi: 10.1128/mBio.00095-11
48. Liu Y, Islam EA, Jarvis GA, Gray-Owen SD, Russell MW. Neisseria gonorrhoeae selectively suppresses the development of Th1 and Th2 cells, and enhances Th17 cell responses, through TGF-beta-dependent mechanisms. Mucosal Immunol. (2012) 5:320–31. doi: 10.1038/mi.2012.12
49. Liu Y, Liu W, Russell MW. Suppression of host adaptive immune responses by Neisseria gonorrhoeae: role of interleukin 10 and type 1 regulatory T cells. Mucosal Immunol. (2014) 7:165–76. doi: 10.1038/mi.2013.36
50. Escobar A, Candia E, Reyes-Cerpa S, Villegas-Valdes B, Neira T, Lopez M, et al. Neisseria gonorrhoeae induces a tolerogenic phenotype in macrophages to modulate host immunity. Mediators Inflamm. (2013) 2013:127017. doi: 10.1155/2013/127017
51. Ortiz MC, Lefimil C, Rodas PI, Vernal R, Lopez M, Acuña-Castillo C, et al. Neisseria gonorrhoeae modulates immunity by polarizing human macrophages to a M2 profile. PLoS ONE (2015) 10:e0130713. doi: 10.1371/journal.pone.0130713
52. Liu Y, Hammer LA, Liu W, Hobbs MM, Zielke RA, Sikora AE, et al. Experimental vaccine induces Th1-driven immune responses and resistance to Neisseria gonorrhoeae infection in a murine model. Mucosal Immunol (2017a) 10:1594–608. doi: 10.1038/mi.2017.11
53. Liu Y, Perez J, Hammer LA, Gallagher HC, De Jesus M, Egilmez NK, et al. Intravaginal administration of interleukin 12 during genital gonococcal infection in mice induces immunity to heterologous strains of neisseria gonorrhoeae. mSphere (2018) 3:e00421–17. doi: 10.1128/mSphere.00421-17
54. Liu Y, Egilmez NK, Russell MW. Enhancement of adaptive immunity to Neisseria gonorrhoeae by local intravaginal administration of microencapsulated interleukin 12. J Infect Dis. (2013) 208:1821–9. doi: 10.1093/infdis/jit354
55. Pizza M, Scarlato V, Masignani V, Giuliani MM, Arico B, Comanducci M, et al. Identification of vaccine candidates against serogroup B meningococcus by whole-genome sequencing. Science (2000) 287:1816–20. doi: 10.1126/science.287.5459.1816
56. Rappuoli R. Reverse vaccinology. Curr Opin Microbiol. (2000) 3:445–50. doi: 10.1016/S1369-5274(00)00119-3
57. Tettelin H, Saunders NJ, Heidelberg J, Jeffries AC, Nelson KE, Eisen JA, et al. Complete genome sequence of Neisseria meningitidis serogroup B strain MC58. Science (2000) 287:1809–1815. doi: 10.1126/science.287.5459.1809
58. Rappuoli R. Reverse vaccinology, a genome-based approach to vaccine development. Vaccine (2001) 19:2688–91. doi: 10.1016/S0264-410X(00)00554-5
59. Seib KL, Zhao X, Rappuoli R. Developing vaccines in the era of genomics: a decade of reverse vaccinology. Clin Microbiol Infect. (2012) 18(Suppl. 5):109–16. doi: 10.1111/j.1469-0691.2012.03939.x
60. Serruto D, Bottomley MJ, Ram S, Giuliani MM, Rappuoli R. The new multicomponent vaccine against meningococcal serogroup B, 4CMenB: immunological, functional and structural characterization of the antigens. Vaccine (2012) 30(Suppl. 2):B87–97. doi: 10.1016/j.vaccine.2012.01.033
61. Petousis-Harris H, Paynter J, Morgan J, Saxton P, McArdle B, Goodyear-Smith F, et al. Effectiveness of a group B outer membrane vesicle meningococcal vaccine against gonorrhoea in New Zealand: a retrospective case-control study. Lancet (2017) 390:1603–10. doi: 10.1016/S0140-6736(17)31449-6
62. Unemo M, Sikora AE. Infection: proof of principle for effectiveness of a gonorrhoea vaccine. Nat Rev Urol (2017) 14:643–4. doi: 10.1038/nrurol.2017.139
63. Zielke RA, Wierzbicki IH, Weber JV, Gafken PR, Sikora AE. Quantitative proteomics of the Neisseria gonorrhoeae cell envelope and membrane vesicles for the discovery of potential therapeutic targets. Mol Cell Proteomics (2014b) 13:1299–317. doi: 10.1074/mcp.M113.029538
64. Zielke RA, Wierzbicki IH, Baarda BI, Gafken PR, Soge OO, Holmes KK, et al. Proteomics-driven antigen discovery for development of vaccines against gonorrhea. Mol Cell Proteomics (2016) 15:2338–55. doi: 10.1074/mcp.M116.058800
65. Ross BC, Czajkowski L, Hocking D, Margetts M, Webb E, Rothel L, et al. Identification of vaccine candidate antigens from a genomic analysis of Porphyromonas gingivalis. Vaccine (2001) 19:4135–42. doi: 10.1016/S0264-410X(01)00173-6
66. Wizemann TM, Heinrichs JH, Adamou JE, Erwin AL, Kunsch C, Choi GH, et al. Use of a whole genome approach to identify vaccine molecules affording protection against Streptococcus pneumoniae infection. Infect Immun. (2001) 69:1593–8. doi: 10.1128/IAI.69.3.1593-1598.2001
67. Etz H, Minh DB, Henics T, Dryla A, Winkler B, Triska C, et al. Identification of in vivo expressed vaccine candidate antigens from Staphylococcus aureus. Proc Natl Acad Sci USA (2002) 99:6573–8. doi: 10.1073/pnas.092569199
68. Montigiani S, Falugi F, Scarselli M, Finco O, Petracca R, Galli G, et al. Genomic approach for analysis of surface proteins in Chlamydia pneumoniae. Infect Immun. (2002) 70:368–79. doi: 10.1128/IAI.70.1.368-379.2002
69. Srinivasa Rao PS, Lim TM, Leung KY. Functional genomics approach to the identification of virulence genes involved in Edwardsiella tarda pathogenesis. Infect Immun. (2003) 71:1343–51. doi: 10.1128/IAI.71.3.1343-1351.2003
70. Haider S, Pal R. Integrated analysis of transcriptomic and proteomic data. Curr Genomics (2013) 14:91–110. doi: 10.2174/1389202911314020003
71. Baarda BI, Sikora AE. Proteomics of Neisseria gonorrhoeae: the treasure hunt for countermeasures against an old disease. Front Microbiol. (2015) 6:1190. doi: 10.3389/fmicb.2015.01190
72. Doro F, Liberatori S, Rodriguez-Ortega MJ, Rinaudo CD, Rosini R, Mora M, et al. Surfome analysis as a fast track to vaccine discovery: identification of a novel protective antigen for Group B Streptococcus hypervirulent strain COH1. Mol Cell Proteomics (2009) 8:1728–37. doi: 10.1074/mcp.M800486-MCP200
73. Dorward DW, Garon CF, Judd RC. Export and intercellular transfer of DNA via membrane blebs of Neisseria gonorrhoeae. J Bacteriol. (1989) 171:2499–505. doi: 10.1128/jb.171.5.2499-2505.1989
74. Ferrari G, Garaguso I, Adu-Bobie J, Doro F, Taddei AR, Biolchi A, et al. Outer membrane vesicles from group B Neisseria meningitidis Δgna33 mutant: proteomic and immunological comparison with detergent-derived outer membrane vesicles. Proteomics (2006) 6:1856–66. doi: 10.1002/pmic.200500164
75. Lee EY, Choi DS, Kim KP, Gho YS. Proteomics in gram-negative bacterial outer membrane vesicles. Mass Spectrom Rev. (2008) 27:535–55. doi: 10.1002/mas.20175
76. Choi DS, Kim DK, Choi SJ, Lee J, Choi JP, Rho S, et al. Proteomic analysis of outer membrane vesicles derived from Pseudomonas aeruginosa. Proteomics (2011) 11:3424–9. doi: 10.1002/pmic.201000212
77. Zielke RA, Gafken PR, Sikora AE. Quantitative proteomic analysis of the cell envelopes and native membrane vesicles derived from gram-negative bacteria. Curr Protoc Microbiol. (2014a) 34:1F 3 1–1F 3 16. doi: 10.1002/9780471729259.mc01f03s34
78. Zielke RA, Sikora AE. Isolation of cell envelopes and naturally released membrane vesicles of Neisseria gonorrhoeae. Curr Protoc Microbiol. (2014) 34:4A 3 1–4A 3 17. doi: 10.1002/9780471729259.mc04a03s34
79. Sikora AE, Wierzbicki IH, Zielke RA, Ryner RF, Korotkov KV, Buchanan SK, et al. Structural functional insights into the role of BamD and BamE within the beta-barrel assembly machinery in Neisseria gonorrhoeae. J Biol Chem. (2018) 293:1106–19. doi: 10.1074/jbc.RA117.000437
80. Post DM, Zhang D, Eastvold JS, Teghanemt A, Gibson BW, Weiss JP. Biochemical and functional characterization of membrane blebs purified from Neisseria meningitidis serogroup B. J Biol Chem. (2005) 280:38383–94. doi: 10.1074/jbc.M508063200
81. Holst J, Oster P, Arnold R, Tatley MV, Naess LM, Aaberge IS, et al. Vaccines against meningococcal serogroup B disease containing outer membrane vesicles (OMV): lessons from past programs and implications for the future. Hum Vaccin Immunother. (2013) 9:1241–53. doi: 10.4161/hv.24129
82. van der Pol L, Stork M, van der Ley P. Outer membrane vesicles as platform vaccine technology. Biotechnol J. (2015) 10:1689–706. doi: 10.1002/biot.201400395
83. Delany I, Rappuoli R, Seib KL. Vaccines, reverse vaccinology, and bacterial pathogenesis. Cold Spring Harb Perspect Med. (2013) 3:a012476. doi: 10.1101/cshperspect.a012476
84. Basta NE, Christensen H. 4CMenB vaccine effectiveness: reasons for optimism. Lancet (2016) 388:2719–21. doi: 10.1016/S0140-6736(16)32061-X
85. Parikh SR, Andrews NJ, Beebeejaun K, Campbell H, Ribeiro S, Ward C, et al. Effectiveness and impact of a reduced infant schedule of 4CMenB vaccine against group B meningococcal disease in England: a national observational cohort study. Lancet (2016) 388:2775–82. doi: 10.1016/S0140-6736(16)31921-3
86. Poetsch A, Wolters D. Bacterial membrane proteomics. Proteomics (2008) 8:4100–22. doi: 10.1002/pmic.200800273
87. Gardy JL, Laird MR, Chen F, Rey S, Walsh CJ, Ester M, et al. PSORTb v.2.0: expanded prediction of bacterial protein subcellular localization and insights gained from comparative proteome analysis. Bioinformatics (2005) 21:617–23. doi: 10.1093/bioinformatics/bti057
88. Yu NY, Wagner JR, Laird MR, Melli G, Rey S, Lo R, et al. PSORTb 3.0: improved protein subcellular localization prediction with refined localization subcategories and predictive capabilities for all prokaryotes. Bioinformatics (2010) 26:1608–15. doi: 10.1093/bioinformatics/btq249
89. Imai K, Asakawa N, Tsuji T, Akazawa F, Ino A, Sonoyama M, et al. SOSUI-GramN: high performance prediction for sub-cellular localization of proteins in gram-negative bacteria. Bioinformation (2008) 2:417–21. doi: 10.6026/97320630002417
90. Yu CS, Lin CJ, Hwang JK. Predicting subcellular localization of proteins for Gram-negative bacteria by support vector machines based on n-peptide compositions. Protein Sci. (2004) 13:1402–6. doi: 10.1110/ps.03479604
91. Yu CS, Chen YC, Lu CH, Hwang JK. Prediction of protein subcellular localization. Proteins (2006) 64:643–51. doi: 10.1002/prot.21018
92. Nielsen H, Engelbrecht J, Brunak S, von Heijne G. Identification of prokaryotic and eukaryotic signal peptides and prediction of their cleavage sites. Protein Eng. (1997) 10:1–6. doi: 10.1093/protein/10.1.1
93. Petersen TN, Brunak S, von Heijne G, Nielsen H. SignalP 4.0: discriminating signal peptides from transmembrane regions. Nat Methods (2011) 8:785–6. doi: 10.1038/nmeth.1701
94. Juncker AS, Willenbrock H, Von Heijne G, Brunak S, Nielsen H, Krogh A. Prediction of lipoprotein signal peptides in Gram-negative bacteria. Protein Sci. (2003) 12:1652–62. doi: 10.1110/ps.0303703
95. Nakayama H, Kurokawa K, Lee BL. Lipoproteins in bacteria: structures and biosynthetic pathways. FEBS J. (2012) 279:4247–68. doi: 10.1111/febs.12041
96. Tatusov RL, Galperin MY, Natale DA, Koonin EV. The COG database: a tool for genome-scale analysis of protein functions and evolution. Nucleic Acids Res. (2000) 28:33–6. doi: 10.1093/nar/28.1.33
97. Galperin MY, Makarova KS, Wolf YI, Koonin EV. Expanded microbial genome coverage and improved protein family annotation in the COG database. Nucleic Acids Res. (2015) 43:D261–9. doi: 10.1093/nar/gku1223
98. Jolley KA, Maiden MC. BIGSdb: Scalable analysis of bacterial genome variation at the population level. BMC Bioinformatics (2010) 11:595. doi: 10.1186/1471-2105-11-595
99. Zielke RA, Le Van A, Baarda BI, Herrera MF, Acosta CJ, Jerse AE, et al. SliC is a surface-displayed lipoprotein that is required for the anti-lysozyme strategy during Neisseria gonorrhoeae infection. PLoS Pathog. (2018) 14:e1007081. doi: 10.1371/journal.ppat.1007081
100. Krogh A, Larsson B, von Heijne G, Sonnhammer EL. Predicting transmembrane protein topology with a hidden Markov model: application to complete genomes. J Mol Biol. (2001) 305:567–80. doi: 10.1006/jmbi.2000.4315
101. Soria-Guerra RE, Nieto-Gomez R, Govea-Alonso DO, Rosales-Mendoza S. An overview of bioinformatics tools for epitope prediction: implications on vaccine development. J Biomed Inform. (2015) 53:405–14. doi: 10.1016/j.jbi.2014.11.003
102. Layh G, Schmitt S, Buchanan TM. Surface immunolabeling of Neisseria gonorrhoeae employing monoclonal antibodies to proteins IA and IB. Zentralbl Bakteriol Mikrobiol Hyg A (1988) 269:34–42. doi: 10.1016/S0176-6724(88)80082-8
103. Paques M, Teppema JS, Beuvery EC, Abdillahi H, Poolman JT, Verkleij AJ. Accessibility of gonococcal and meningococcal surface antigens: immunogold labeling for quantitative electron microscopy. Infect Immun. (1989) 57:582–9.
104. Unemo M, Golparian D, Sanchez-Buso L, Grad Y, Jacobsson S, Ohnishi M, et al. The novel 2016 WHO Neisseria gonorrhoeae reference strains for global quality assurance of laboratory investigations: phenotypic, genetic and reference genome characterization. J Antimicrob Chemother. (2016b) 71:3096–108. doi: 10.1093/jac/dkw288
105. Shewell LK, Ku SC, Schulz BL, Jen FE, Mubaiwa TD, Ketterer MR, et al. Recombinant truncated AniA of pathogenic Neisseria elicits a non-native immune response and functional blocking antibodies. Biochem Biophys Res Commun. (2013) 431:215–20. doi: 10.1016/j.bbrc.2012.12.132
106. Semchenko EA, Day CJ, Seib KL. MetQ of Neisseria gonorrhoeae is a surface-expressed antigen that elicits bactericidal and functional blocking antibodies. Infect Immun. (2017) 85:e00898–16. doi: 10.1128/IAI.00898-16
107. Hung MC, Heckels JE, Christodoulides M. The adhesin complex protein (ACP) of Neisseria meningitidis is a new adhesin with vaccine potential. MBio (2013) 4:e00041–13. doi: 10.1128/mBio.00041-13
108. Humbert MV, Awanye AM, Lian LY, Derrick JP, Christodoulides M. Structure of the Neisseria Adhesin Complex Protein (ACP) and its role as a novel lysozyme inhibitor. PLoS Pathog. (2017) 13:e1006448. doi: 10.1371/journal.ppat.1006448
109. Ragland SA, Humbert MV, Christodoulides M, Criss AK. Neisseria gonorrhoeae employs two protein inhibitors to evade killing by human lysozyme. PLoS Pathog. (2018) 14:e1007080. doi: 10.1371/journal.ppat.1007080
110. Lomholt H, Lind I, Kilian M. Neisseria gonorrhoeae IgA1 proteases share epitopes recognized by neutralizing antibodies. Vaccine (1995) 13:1213–9. doi: 10.1016/0264-410X(95)00057-8
111. Madico G, Welsch JA, Lewis LA, McNaughton A, Perlman DH, Costello CE, et al. The meningococcal vaccine candidate GNA1870 binds the complement regulatory protein factor H and enhances serum resistance. J Immunol (2006) 177:501–10. doi: 10.4049/jimmunol.177.1.501
112. Tavano R, Capecchi B, Montanari P, Franzoso S, Marin O, Sztukowska M, et al. Mapping of the Neisseria meningitidis NadA cell-binding site: relevance of predicted {alpha}-helices in the NH2-terminal and dimeric coiled-coil regions. J Bacteriol (2011) 193:107–15. doi: 10.1128/JB.00430-10
113. Vacca I, Del Tordello E, Gasperini G, Pezzicoli A, Di Fede M, Rossi Paccani S, et al. Neisserial Heparin Binding Antigen (NHBA) Contributes to the Adhesion of Neisseria meningitidis to Human Epithelial Cells. PLoS ONE (2016) 11:e0162878. doi: 10.1371/journal.pone.0162878
114. Forthal DN. Functions of antibodies. Microbiol Spectr. (2014) 2:AID-0019-2014. doi: 10.1128/microbiolspec.AID-0019-2014
115. Bootz A, Karbach A, Spindler J, Kropff B, Reuter N, Sticht H, et al. Protective capacity of neutralizing and non-neutralizing antibodies against glycoprotein B of cytomegalovirus. PLoS Pathog. (2017) 13:e1006601. doi: 10.1371/journal.ppat.1006601
116. Brodeur BR, Boyer M, Charlebois I, Hamel J, Couture F, Rioux CR, et al. Identification of group B streptococcal Sip protein, which elicits cross-protective immunity. Infect Immun. (2000) 68:5610–8. doi: 10.1128/IAI.68.10.5610-5618.2000
117. Su XD, Zhang H, Terwilliger TC, Liljas A, Xiao J, Dong Y. Protein crystallography from the perspective of technology developments. Crystallogr Rev. (2015) 21:122–53. doi: 10.1080/0889311X.2014.973868
118. Shin J, Lee W, Lee W. Structural proteomics by NMR spectroscopy. Expert Rev Proteomics (2008) 5:589–601. doi: 10.1586/14789450.5.4.589
119. Zhuravleva A, Korzhnev DM. Protein folding by NMR. Prog Nucl Mag Resonan Spectr. (2017) 100:52–77. doi: 10.1016/j.pnmrs.2016.10.002
120. Stura EA, Fieser GG, Wilson IA. Crystallization of antibodies and antibody-antigen complexes. ImmunoMethods (1993) 3:164–79. doi: 10.1006/immu.1993.1051
121. Obmolova G, Malia TJ, Teplyakov A, Sweet R, Gilliland GL. Promoting crystallization of antibody-antigen complexes via microseed matrix screening. Acta Crystallogr D Biol Crystallogr. (2010) 66:927–33. doi: 10.1107/S0907444910026041
122. Malia TJ, Obmolova G, Luo J, Teplyakov A, Sweet R, Gilliland GL. Crystallization of a challenging antigen-antibody complex: TLR3 ECD with three noncompeting Fabs. Acta Crystallogr Sect F Struct Biol Cryst Commun. (2011) 67:1290–5. doi: 10.1107/S1744309111030983
123. Lipman NS, Jackson LR, Trudel LJ, Weis-Garcia F. Monoclonal versus polyclonal antibodies: distinguishing characteristics, applications, and information resources. ILAR J (2005) 46:258–68. doi: 10.1093/ilar.46.3.258
124. Liao M, Cao E, Julius D, Cheng Y. Structure of the TRPV1 ion channel determined by electron cryo-microscopy. Nature (2013) 504:107–12. doi: 10.1038/nature12822
125. Liu Y, Pan J, Jenni S, Raymond DD, Caradonna T, Do KT, et al. CryoEM structure of an influenza virus receptor-binding site antibody-antigen interface. J Mol Biol. (2017b) 429:1829–39. doi: 10.1016/j.jmb.2017.05.011
126. Earl LA, Falconieri V, Milne JL, Subramaniam S. Cryo-EM: beyond the microscope. Curr Opin Struct Biol. (2017) 46:71–8. doi: 10.1016/j.sbi.2017.06.002
127. Plante M, Jerse A, Hamel J, Couture F, Rioux CR, Brodeur BR, et al. Intranasal immunization with gonococcal outer membrane preparations reduces the duration of vaginal colonization of mice by Neisseria gonorrhoeae. J Infect Dis. (2000) 182:848–55. doi: 10.1086/315801
128. Gulati S, Zheng B, Reed GW, Su X, Cox AD, St Michael F, et al. Immunization against a saccharide epitope accelerates clearance of experimental gonococcal infection. PLoS Pathog. (2013) 9:e1003559. doi: 10.1371/journal.ppat.1003559
129. Karch CP, Burkhard P. Vaccine technologies: From whole organisms to rationally designed protein assemblies. Biochem Pharmacol. (2016) 120:1–14. doi: 10.1016/j.bcp.2016.05.001
130. Bobbala S, Hook S. Is there an optimal formulation and delivery strategy for subunit vaccines? Pharm Res. (2016) 33:2078–97. doi: 10.1007/s11095-016-1979-0
131. Lee S, Nguyen MT. Recent advances of vaccine adjuvants for infectious diseases. Immune Netw. (2015) 15:51–7. doi: 10.4110/in.2015.15.2.51
132. Awasthi S, Hook LM, Shaw CE, Friedman HM. A trivalent subunit antigen glycoprotein vaccine as immunotherapy for genital herpes in the guinea pig genital infection model. Hum Vaccin Immunother. (2017) 13:2785–93. doi: 10.1080/21645515.2017.1323604
133. Bernstein DI, Wald A, Warren T, Fife K, Tyring S, Lee P, et al. Therapeutic vaccine for genital herpes simplex virus-2 infection: findings from a randomized trial. J Infect Dis. (2017) 215:856–64. doi: 10.1093/infdis/jix004
134. Van Wagoner N, Fife K, Leone PA, Bernstein DI, Warren T, Panther L, et al. Effects of different doses of GEN-003, a therapeutic vaccine for genital herpes simplex virus-2, on viral shedding and lesions: results of a randomized placebo-controlled trial. J Infect Dis. (2018) 218:1890–9. doi: 10.1093/infdis/jiy415
135. Braun M, Silhavy TJ. Imp/OstA is required for cell envelope biogenesis in Escherichia coli. Mol Microbiol. (2002) 45:1289–302. doi: 10.1046/j.1365-2958.2002.03091.x
136. Ruiz N, Kahne D, Silhavy TJ. Transport of lipopolysaccharide across the cell envelope: the long road of discovery. Nat Rev Microbiol. (2009) 7:677–83. doi: 10.1038/nrmicro2184
137. Bos MP, Tefsen B, Geurtsen J, Tommassen J. Identification of an outer membrane protein required for the transport of lipopolysaccharide to the bacterial cell surface. Proc Natl Acad Sci USA (2004) 101:9417–22. doi: 10.1073/pnas.0402340101
138. Welsch JA, Ram S. Factor H and neisserial pathogenesis. Vaccine (2008) 26(Suppl. 8):I.40–45. doi: 10.1016/j.vaccine.2008.11.060
139. Wu SC, Maragathavally KJ, Coates CJ, Kaminski JM. Steps toward targeted insertional mutagenesis with class II transposable elements. Methods Mol Biol. (2008) 435:139–51. doi: 10.1007/978-1-59745-232-8_10
140. Zielke RA, Wierzbicki IH, Baarda BI, Sikora AE. The Neisseria gonorrhoeae Obg protein is an essential ribosome-associated GTPase and a potential drug target. BMC Microbiol. (2015) 15:129. doi: 10.1186/s12866-015-0453-1
141. Wierzbicki IH, Zielke RA, Korotkov KV, Sikora AE. Functional and structural studies on the Neisseria gonorrhoeae GmhA, the first enzyme in the glycero-manno-heptose biosynthesis pathways, demonstrate a critical role in lipooligosaccharide synthesis and gonococcal viability. Microbiologyopen (2017) 6:e00432. doi: 10.1002/mbo3.432
142. Sikora AE, Mills RH, Weber JV, Hamza A, Passow BW, Romaine A, et al. Peptide Inhibitors Targeting the Neisseria gonorrhoeae Pivotal anaerobic respiration factor AniA. Antimicrob Agents Chemother. (2017) 61:e00186–17. doi: 10.1128/AAC.00186-17
143. Kulp A, Kuehn MJ. Biological functions and biogenesis of secreted bacterial outer membrane vesicles. Annu Rev Microbiol. (2010) 64:163–84. doi: 10.1146/annurev.micro.091208.073413
144. Kulkarni HM, Jagannadham MV. Biogenesis and multifaceted roles of outer membrane vesicles from Gram-negative bacteria. Microbiology (2014) 160:2109–21. doi: 10.1099/mic.0.079400-0
145. Haurat MF, Elhenawy W, Feldman MF. Prokaryotic membrane vesicles: new insights on biogenesis and biological roles. Biol Chem. (2015) 396:95–109. doi: 10.1515/hsz-2014-0183
146. Wang X, Jiang F, Zheng J, Chen L, Dong J, Sun L, et al. The outer membrane phospholipase A is essential for membrane integrity and type III secretion in Shigella flexneri. Open Biol. (2016) 6:160073. doi: 10.1098/rsob.160073
147. Baarda BI, Emerson S, Proteau PJ, Sikora AE. Deciphering the function of new gonococcal vaccine antigens using phenotypic microarrays. J Bacteriol. (2017) 199:1–24. doi: 10.1128/JB.00037-17
148. Wu HJ, Seib KL, Edwards JL, Apicella MA, McEwan AG, Jennings MP. Azurin of pathogenic Neisseria spp. is involved in defense against hydrogen peroxide and survival within cervical epithelial cells. Infect Immun. (2005) 73:8444–8. doi: 10.1128/IAI.73.12.8444-8448.2005
149. Cole JG, Jerse AE. Functional characterization of antibodies against Neisseria gonorrhoeae opacity protein loops. PLoS ONE (2009) 4:e8108. doi: 10.1371/journal.pone.0008108
150. Bochner BR, Gadzinski P, Panomitros E. Phenotype microarrays for high-throughput phenotypic testing and assay of gene function. Genome Res. (2001) 11:1246–55. doi: 10.1101/gr.186501
151. Shea A, Wolcott M, Daefler S, Rozak DA. Biolog phenotype microarrays. Methods Mol Biol. (2012) 881:331–73. doi: 10.1007/978-1-61779-827-6_12
152. Morse SA. The biology of the gonococcus. CRC Crit Rev Microbiol. (1978) 7:93–189. doi: 10.3109/10408417909083071
153. Jerse AE, Sharma ND, Simms AN, Crow ET, Snyder LA, Shafer WM. A gonococcal efflux pump system enhances bacterial survival in a female mouse model of genital tract infection. Infect Immun. (2003) 71:5576–82. doi: 10.1128/IAI.71.10.5576-5582.2003
154. Preston A, Mandrell RE, Gibson BW, Apicella MA. The lipooligosaccharides of pathogenic gram-negative bacteria. Crit Rev Microbiol. (1996) 22:139–80. doi: 10.3109/10408419609106458
155. Silhavy TJ, Kahne D, Walker S. The bacterial cell envelope. Cold Spring Harb Perspect Biol. (2010) 2:a000414. doi: 10.1101/cshperspect.a000414
156. Wierzbicki IH, ZR A, Jerse AE, Begum AA, Unemo M, Sikora AE. NGO1985 is a surface-exposed outer membrane lipoprotein involved in Neisseria gonorrhoeae cell envelope homeostasis and a novel gonorrhea vaccine candidate. In: International Pathogenic Neisseria Conference (2016).
157. Hagan CL, Silhavy TJ, Kahne D. β-Barrel membrane protein assembly by the Bam complex. Annu Rev Biochem. (2011) 80:189–210. doi: 10.1146/annurev-biochem-061408-144611
158. Ricci DP, Silhavy TJ. The Bam machine: a molecular cooper. Biochim Biophys Acta (2012) 1818:1067–84. doi: 10.1016/j.bbamem.2011.08.020
159. Volokhina EB, Beckers F, Tommassen J, Bos MP. The β-barrel outer membrane protein assembly complex of Neisseria meningitidis. J Bacteriol. (2009) 191:7074–85. doi: 10.1128/JB.00737-09
160. Voulhoux R, Bos MP, Geurtsen J, Mols M, Tommassen J. Role of a highly conserved bacterial protein in outer membrane protein assembly. Science (2003) 299:262–5. doi: 10.1126/science.1078973
161. Gentle I, Gabriel K, Beech P, Waller R, Lithgow T. The Omp85 family of proteins is essential for outer membrane biogenesis in mitochondria and bacteria. J Cell Biol. (2004) 164:19–24. doi: 10.1083/jcb.200310092
162. Onufryk C, Crouch ML, Fang FC, Gross CA. Characterization of six lipoproteins in the sigmaE regulon. J Bacteriol. (2005) 187:4552–61. doi: 10.1128/JB.187.13.4552-4561.2005
163. Liechty A, Chen J, Jain MK. Origin of antibacterial stasis by polymyxin B in Escherichia coli. Biochim Biophys Acta (2000) 1463:55–64. doi: 10.1016/S0005-2736(99)00178-9
164. Oh JT, Cajal Y, Skowronska EM, Belkin S, Chen J, Van Dyk TK, et al. Cationic peptide antimicrobials induce selective transcription of micF and osmY in Escherichia coli. Biochim Biophys Acta (2000) 1463:43–54. doi: 10.1016/S0005-2736(99)00177-7
165. Yeats C, Bateman A. The BON domain: a putative membrane-binding domain. Trends Biochem Sci. (2003) 28:352–5. doi: 10.1016/S0968-0004(03)00115-4
166. Mahoney TF, Ricci DP, Silhavy TJ. Classifying beta-Barrel assembly substrates by manipulating essential bam complex members. J Bacteriol. (2016) 198:1984–92. doi: 10.1128/JB.00263-16
167. Qiao S, Luo Q, Zhao Y, Zhang XC, Huang Y. Structural basis for lipopolysaccharide insertion in the bacterial outer membrane. Nature (2014) 511:108–11. doi: 10.1038/nature13484
168. Bos MP, Tommassen J. The LptD chaperone LptE is not directly involved in lipopolysaccharide transport in Neisseria meningitidis. J Biol Chem. (2011) 286:28688–96. doi: 10.1074/jbc.M111.239673
169. Malinverni JC, Silhavy TJ. An ABC transport system that maintains lipid asymmetry in the gram-negative outer membrane. Proc Natl Acad Sci USA (2009) 106:8009–14. doi: 10.1073/pnas.0903229106
170. Abellon-Ruiz J, Kaptan SS, Basle A, Claudi B, Bumann D, Kleinekathofer U, et al. Structural basis for maintenance of bacterial outer membrane lipid asymmetry. Nat Microbiol. (2017) 1:1616–23. doi: 10.1038/s41564-017-0046-x
171. Baarda BI, Zielke RA, Le Van A, Jerse AE, Sikora AE. A novel outer membrane protein, NGO2121, influences membrane vesicle production and virulence of Neisseria gonorrhoeae. In: International Pathogenic Neisseria Conference (2016).
172. Roier S, Zingl FG, Cakar F, Durakovic S, Kohl P, Eichmann TO, et al. A novel mechanism for the biogenesis of outer membrane vesicles in Gram-negative bacteria. Nat Commun. (2016) 7:10515. doi: 10.1038/ncomms10515
173. Kovacs-Simon A, Titball RW, Michell SL. Lipoproteins of bacterial pathogens. Infect Immun. (2011) 79:548–61. doi: 10.1128/IAI.00682-10
174. Hooda Y, Lai CC, Judd A, Buckwalter CM, Shin HE, Gray-Owen SD, et al. Slam is an outer membrane protein that is required for the surface display of lipidated virulence factors in Neisseria. Nat Microbiol. (2016) 1:16009. doi: 10.1038/nmicrobiol.2016.9
175. Stabler RA, Marsden GL, Witney AA, Li Y, Bentley SD, Tang CM, et al. Identification of pathogen-specific genes through microarray analysis of pathogenic and commensal Neisseria species. Microbiology (2005) 151:2907–22. doi: 10.1099/mic.0.28099-0
176. Tommassen J, Arenas J. Biological functions of the secretome of Neisseria meningitidis. Front Cell Infect Microbiol. (2017) 7:256. doi: 10.3389/fcimb.2017.00256
177. Deng M, Misra R. Examination of AsmA and its effect on the assembly of Escherichia coli outer membrane proteins. Mol Microbiol. (1996) 21:605–12. doi: 10.1111/j.1365-2958.1996.tb02568.x
178. Putker F, Grutsch A, Tommassen J, Bos MP. Ght protein of Neisseria meningitidis is involved in the regulation of lipopolysaccharide biosynthesis. J Bacteriol. (2014) 196:780–9. doi: 10.1128/JB.00943-13
179. Barondess JJ, Beckwith J. A bacterial virulence determinant encoded by lysogenic coliphage lambda. Nature (1990) 346:871–4. doi: 10.1038/346871a0
180. Wei Y, Lin-Lee YC, Yang X, Dai W, Zhao S, Rassool FV, et al. Molecular cloning of Chinese hamster 1q31 chromosomal fragile site DNA that is important to mdr1 gene amplification reveals a novel gene whose expression is associated with spermatocyte and adipocyte differentiation. Gene (2006) 372:44–52. doi: 10.1016/j.gene.2005.12.024
181. Calmettes C, Ing C, Buckwalter CM, El Bakkouri M, Chieh-Lin Lai C, Pogoutse A, et al. The molecular mechanism of Zinc acquisition by the neisserial outer-membrane transporter ZnuD. Nat Commun. (2015) 6:7996. doi: 10.1038/ncomms8996
182. Lappann M, Danhof S, Guenther F, Olivares-Florez S, Mordhorst IL, Vogel U. In vitro resistance mechanisms of Neisseria meningitidis against neutrophil extracellular traps. Mol Microbiol. (2013) 89:433–49. doi: 10.1111/mmi.12288
183. Urban CF, Ermert D, Schmid M, Abu-Abed U, Goosmann C, Nacken W, et al. Neutrophil extracellular traps contain calprotectin, a cytosolic protein complex involved in host defense against Candida albicans. PLoS Pathog. (2009) 5:e1000639. doi: 10.1371/journal.ppat.1000639
184. Hagen TA, Cornelissen CN. Neisseria gonorrhoeae requires expression of TonB and the putative transporter TdfF to replicate within cervical epithelial cells. Mol Microbiol. (2006) 62:1144–57. doi: 10.1111/j.1365-2958.2006.05429.x
185. Callewaert L, Van Herreweghe JM, Vanderkelen L, Leysen S, Voet A, Michiels CW. Guards of the great wall: bacterial lysozyme inhibitors. Trends Microbiol. (2012) 20:501–10. doi: 10.1016/j.tim.2012.06.005
186. Lefevre P, Braibant M, de Wit L, Kalai M, Roeper D, Grotzinger J, et al. Three different putative phosphate transport receptors are encoded by the Mycobacterium tuberculosis genome and are present at the surface of Mycobacterium bovis BCG. J Bacteriol. (1997) 179:2900–6. doi: 10.1128/jb.179.9.2900-2906.1997
187. Kovacs-Simon A, Leuzzi R, Kasendra M, Minton N, Titball RW, Michell SL. Lipoprotein CD0873 is a novel adhesin of Clostridium difficile. J Infect Dis. (2014) 210:274–84. doi: 10.1093/infdis/jiu070
188. Dormitzer PR, Grandi G, Rappuoli R. Structural vaccinology starts to deliver. Nat Rev Microbiol. (2012) 10:807–13. doi: 10.1038/nrmicro2893
189. Liljeroos L, Malito E, Ferlenghi I, Bottomley MJ. Structural and computational biology in the design of immunogenic vaccine antigens. J Immunol Res. (2015) 2015:156241. doi: 10.1155/2015/156241
190. Noinaj N, Kuszak AJ, Gumbart JC, Lukacik P, Chang H, Easley NC, et al. Structural insight into the biogenesis of beta-barrel membrane proteins. Nature (2013) 501:385–90. doi: 10.1038/nature12521
191. Albrecht R, Schütz M, Oberhettinger P, Faulstich M, Bermejo I, Rudel T, et al. Structure of BamA, an essential factor in outer membrane protein biogenesis. Acta Crystallogr D Biol Crystallogr. (2014) 70:1779–89. doi: 10.1107/S1399004714007482
192. Lee J, Sutterlin HA, Wzorek JS, Mandler MD, Hagan CL, Grabowicz M, et al. Substrate binding to BamD triggers a conformational change in BamA to control membrane insertion. Proc Natl Acad Sci USA (2018) 115:2359–64. doi: 10.1073/pnas.1711727115
193. Ryan KR, Taylor JA, Bowers LM. The BAM complex subunit BamE (SmpA) is required for membrane integrity, stalk growth and normal levels of outer membrane β-barrel proteins in Caulobacter crescentus. Microbiology (2010) 156:742–56. doi: 10.1099/mic.0.035055-0
194. Knowles TJ, Browning DF, Jeeves M, Maderbocus R, Rajesh S, Sridhar P, et al. Structure and function of BamE within the outer membrane and the beta-barrel assembly machine. EMBO Rep. (2011) 12:123–8. doi: 10.1038/embor.2010.202
195. Albrecht R, Zeth K. Structural basis of outer membrane protein biogenesis in bacteria. J Biol Chem. (2011) 286:27792–803. doi: 10.1074/jbc.M111.238931
196. Kim KH, Kang HS, Okon M, Escobar-Cabrera E, McIntosh LP, Paetzel M. Structural characterization of Escherichia coli BamE, a lipoprotein component of the beta-barrel assembly machinery complex. Biochemistry (2011) 50:1081–90. doi: 10.1021/bi101659u
197. Shen HH, Leyton DL, Shiota T, Belousoff MJ, Noinaj N, Lu J, et al. Reconstitution of a nanomachine driving the assembly of proteins into bacterial outer membranes. Nat Commun. (2014) 5:5078. doi: 10.1038/ncomms6078
198. Henderson IR, Nataro JP. Virulence functions of autotransporter proteins. Infect Immun. (2001) 69:1231–43. doi: 10.1128/IAI.69.3.1231-1243.2001
199. Selkrig J, Leyton DL, Webb CT, Lithgow T. Assembly of beta-barrel proteins into bacterial outer membranes. Biochim Biophys Acta (2014) 1843:1542–50. doi: 10.1016/j.bbamcr.2013.10.009
200. Heinz E, Selkrig J, Belousoff MJ, Lithgow T. (2015). Evolution of the translocation and assembly module (TAM). Genome Biol Evol. 7, 1628–43. doi: 10.1093/gbe/evv097
201. Gruss F, Zahringer F, Jakob RP, Burmann BM, Hiller S, Maier T. The structural basis of autotransporter translocation by TamA. Nat Struct Mol Biol. (2013) 20:1318–20. doi: 10.1038/nsmb.2689
202. Botos I, Majdalani N, Mayclin SJ, McCarthy JG, Lundquist K, Wojtowicz D, et al. Structural and functional characterization of the LPS transporter LptDE from gram-negative pathogens. Structure (2016) 24:965–76. doi: 10.1016/j.str.2016.03.026
203. Peng J, Yang L, Yang F, Yang J, Yan Y, Nie H, et al. Characterization of ST-4821 complex, a unique Neisseria meningitidis clone. Genomics (2008) 91:78–87. doi: 10.1016/j.ygeno.2007.10.004
204. Yang X, Wu Z, Wang X, Yang C, Xu H, Shen Y. Crystal structure of lipoprotein GNA1946 from Neisseria meningitidis. J Struct Biol. (2009) 168:437–43. doi: 10.1016/j.jsb.2009.09.001
205. Cava F, Lam H, de Pedro MA, Waldor MK. Emerging knowledge of regulatory roles of D-amino acids in bacteria. Cell Mol Life Sci. (2011) 68:817–31. doi: 10.1007/s00018-010-0571-8
206. Sankaranarayanan R, Moras D. The fidelity of the translation of the genetic code. Acta Biochim Pol. (2001) 48:323–35.
207. Hondorp ER, Matthews RG. Methionine. EcoSal Plus (2006) 2:1–36. doi: 10.1128/ecosalplus.3.6.1.7
208. Noinaj N, Guillier M, Barnard TJ, Buchanan SK. TonB-dependent transporters: regulation, structure, and function. Annu Rev Microbiol. (2010) 64:43–60. doi: 10.1146/annurev.micro.112408.134247
209. Ma L, Terwilliger A, Maresso AW. Iron and zinc exploitation during bacterial pathogenesis. Metallomics (2015) 7:1541–54. doi: 10.1039/C5MT00170F
Keywords: Neisseria gonorrhoeae, vaccine, proteomics, antigen, reverse vaccinology, bioinformatics, protein structure-function, membrane vesicles
Citation: Baarda BI, Martinez FG and Sikora AE (2018) Proteomics, Bioinformatics and Structure-Function Antigen Mining For Gonorrhea Vaccines. Front. Immunol. 9:2793. doi: 10.3389/fimmu.2018.02793
Received: 31 August 2018; Accepted: 13 November 2018;
Published: 04 December 2018.
Edited by:
Michael W. Russell, University at Buffalo, United StatesReviewed by:
Joseph U. Igietseme, Centers for Disease Control and Prevention (CDC), United StatesTrevor F. Moraes, University of Toronto, Canada
Copyright © 2018 Baarda, Martinez and Sikora. This is an open-access article distributed under the terms of the Creative Commons Attribution License (CC BY). The use, distribution or reproduction in other forums is permitted, provided the original author(s) and the copyright owner(s) are credited and that the original publication in this journal is cited, in accordance with accepted academic practice. No use, distribution or reproduction is permitted which does not comply with these terms.
*Correspondence: Aleksandra E. Sikora, YWxla3NhbmRyYS5zaWtvcmFAb3JlZ29uc3RhdGUuZWR1