- 1Key Laboratory of Respiratory Diseases of the Ministry of Health, Department of Respiratory and Critical Care Medicine, Tongji Medical College, Union Hospital, Huazhong University of Science and Technology, Wuhan, China
- 2Department of Thoracic Surgery, Tongji Medical College, Union Hospital, Huazhong University of Science and Technology, Wuhan, China
With the development of nanotechnology, significant progress has been made in the design, and manufacture of nanoparticles (NPs) for use in clinical treatments. Recent increases in our understanding of the central role of macrophages in the context of inflammation and cancer have reinvigorated interest in macrophages as drug targets. Macrophages play an integral role in maintaining the steady state of the immune system and are involved in cancer and inflammation processes. Thus, NPs tailored to accurately target macrophages have the potential to transform disease treatment. Herein, we first present a brief background information of NPs as drug carriers, including but not limited to the types of nanomaterials, their biological properties and their advantages in clinical application. Then, macrophage effector mechanisms and recent NPs-based strategies aimed at targeting macrophages by eliminating or re-educating macrophages in inflammation and cancer are summarized. Additionally, the development of nanocarriers targeting macrophages for disease diagnosis is also discussed. Finally, the significance of macrophage-targeting nanomedicine is highlighted, with the goal of facilitating future clinical translation.
Introduction
Macrophages are components of innate immunity and are divided into two types according to their phenotype and function, classically activated macrophages (M1 macrophages), and alternatively activated macrophages (M2 macrophages), that secrete multiple cytokines and express respective surface markers after polarization (1). M1 macrophages express the markers CD86, nitric oxide synthase (NOS) 2, tumor necrosis factor (TNF)-α, and IL-1β. In addition, M2 macrophages highly express the scavenger receptors CD163 and CD200R and release IL-10 (2). M1 macrophages mainly mediate pro-inflammatory processes that protect against the invasion of foreign bodies and play important roles in antitumor immunity in the tumor microenvironment (TME). Furthermore, M2 macrophages possess anti-inflammatory activity in inflammatory diseases but have also been shown to develop protumor characteristics and promote tumor growth and metastasis (3, 4). Macrophages existing in the TME are also called tumor-associated macrophages (TAMs), which suppress antitumor immunity (5). Mononuclear cells in the blood migrate to the tumor site and transform into TAMs, whose phenotype is similar to that of M2 macrophages (2, 6, 7). Therefore, novel therapeutics targeting M1 macrophages in inflammation and M2 macrophages in cancer that efficiently cure these diseases are desirable strategies that should be developed.
Nanomedicine, which has been used in the generation of therapeutic agents, is anticipated to help researchers solve more clinical problems. Drug delivery systems based on nanoparticles (NPs) have been widely used after several decades of technological developments (8). NPs constitute a large family of materials; synthetic NPs with various structures have been produced using a wide range of materials, including liposomes (9, 10), chitosan (11), poly(lactic-coglycolic acid) (PLGA) (12, 13), dextran (14), silica (15), and metals such as iron oxide or gold (16). These materials share several features, including their size range, hydrophilic properties and charge characteristics, which allow them to function as carriers for the delivery of drugs. Moreover, the use of NPs as drug carriers is a novel method to treat certain diseases and has several advantages. First, synthetic nanoscale materials, which are non-human components, are usually avirulent and can easily be formed into capsules and films. Second, NPs can penetrate physiological barriers, such as the blood-brain barrier (17), because of their small diameter and sufficient design, which enable their stability in circulation. Third, NPs and their drug loads can be co-encapsulated stably with high consistency. Fourth, NPs can carry engineered (polymeric NPs) antibodies or aptamers based on the target, which enables them to recognize specific cells. These features all support the use of NPs for drug delivery (8, 12, 18).
In this review, we first discuss the properties of NPs as drug carriers. We then introduce the selection of nanomaterials based on clinical need and the benefits of using nano drugs to treat clinical diseases. Afterwards, we highlight the dual roles of macrophage subtypes in inflammation and cancer. The negative impacts of macrophages are mostly attributed to M1 macrophages that modulate inflammation and M2 macrophages that contribute to cancer. Finally, we illustrate how NPs act as carriers to deliver therapeutic agents to macrophage subtypes in a targeted manner to cure inflammation and cancer.
NPs as Potential Therapeutic Agents
Types and Characteristics of NPs
NPs are tiny particles (smaller than 100 nanometers) made of materials such as latex, polymers, ceramic particles, metal particles, and carbon particles. NPs are increasingly being used in medical applications due to their physicochemical properties, including their chemical composition, size, shape, structure, morphology, and surface properties (Figure 1). Their surfaces are hydrophilic or hydrophobic and exhibit surface charge and specific ligands, which are reference factors for the selection of nanomaterials used to treat clinical diseases (19). Therefore, NPs play an active role in transporting drugs to the targeted cell and constitute a drug delivery system. Different NPs have been studied by scientists to explore their value for clinical applications.
Basis for NPs as Carriers of Therapeutic Agents
When selecting materials for NPs designed to carry therapeutic agents, several limitations of traditional drugs, such as their non-specific distribution, potential toxicity, lack of targeting capability, poor solubility in water and low therapeutic index, need to be taken into account and overcome. NPs must produce metabolites or degradation products that do not harm the human body or are decomposed and eliminated by the body. The degradation products of PLGA-NPs, a relatively mature NP that is currently being explored, are lactic acid and hydroxyacetic acid, which are also byproducts of human metabolic pathways, suggesting that PLGA-NPs can be used in medical applications as a biological material that does not produce toxic side effects. This method has been widely used in the field of biomedicine. Bowerman et al. used PLGA-docetaxel-NPs to treat breast cancer and examined their biodegradability and biocompatibility (20).
Additionally, therapeutic agents loaded into NPs must be designed with optimal sizes, shapes, and surface properties to improve their biodistribution, increase their solubility, enhance their stability, and reduce their immunogenicity (19). For instance, silica-NPs are important nanometer materials, and mesoporous silica NPs (MSNs) are currently being widely investigated. These NPs are non-toxic, tasteless, and do not produce pollution levels similar to those of non-metallic materials, and their high absorptivity and good plasticity make them suitable for disease treatment in the medical field. However, the exploitation of MSNs is currently limited to mouse models for preclinical testing, and the metabolism of silica-NPs is still a problem that hinders successful clinical translation and needs to be researched more deeply (21). In the study by Lu et al., silica was developed into MSNs that were loaded with the antitumor drug oxaliplatin and a specific indoleamine 2,3-dioxygenase inhibitor, which increased the drug concentration at the targeted orthotopic pancreatic ductal adenocarcinoma site in mouse models (22). In the studies by Man et al. and Patel et al., Au-NPs were designed to act as a bidentate ligand to enhance the stability of N-heterocyclic carbene (NHC) ligands (23), and liposomes were exploited for packaging non-water soluble drugs because of their excellent water solubility. These NPs are relatively efficient carriers of short genetic sequences that directly insert genes into cells for assembly (24). A recent phase III trial investigating a lipid NPs (LNPs) siRNA formulation to treat transthyretin (TTR)-induced amyloidosis was successful. Optimized ionizable cationic lipids are a significant factor for the clinical success of LNPs-siRNA. LNPs are the most advanced delivery vehicle, and the Food and Drug Administration (FDA) approved LNPs for the treatment of the hereditary condition TTR-mediated amyloidosis in 2018. Currently, numerous ionizable lipids are available for packaging nucleic acids, which are under clinical investigation for the treatment of various diseases, including cancer, and viral infections (25).
Moreover, designing appropriate NPs for different environments is crucial. For instance, altered pH dynamics, leaky vasculature, and hypoxia are major features in the TME. Nano drugs for acidic environments should be developed to suit different pathophysiological conditions, such as the spatial variations in the TME pH. Chitosan molecules contain free amino acids, can easily form salts in acidic solutions and are cationic, making them useful as drug carriers for antitumor drugs in an acidic TME and for drug release after degradation (11).
Clinical Application Prospects of Nano Drugs
Compared to traditional treatments, nano drugs are more beneficial for the treatment of clinical diseases. First, these drugs have an extended plasma half-life in systemic circulation and a prolonged pharmaceutical effect due to their stability in harsh environments, such as the high levels of proteases or other enzymes in the blood stream and the highly acidic environment in the stomach (26). Second, they can alleviate systemic side effects due to their unique mode of drug delivery, which is cell- (such as macrophage) or tissue-specific, and the treatment efficacy can be maximized (27). Finally, nano drugs can control drug release over a manageable period at precise doses (28). Nanomaterials can be combined into groups with different properties to carry different medications for combination therapy, which has the potential to overcome multidrug resistance (19, 29). With the technological development of drug-loaded NPs, the pharmacokinetics and bioavailability analyses of nano drugs indicate that they exhibit characteristics such as vascular permeability, slowed excretion, and mononuclear phagocyte uptake, which is important in the application of NPs-mediated drug delivery systems for disease treatment (30).
The application of these nanomaterials in their respective fields plays a very strong role, and these nanomaterials were designed to emphasize biological compatibility and biodegradable properties. However, we must admit that due to the complex internal environment in the body, most studies performed to date are verified in only animal models, and few are registered in clinical research. Therefore, the application of these materials in clinical medicine needs further research.
Macrophages Play Different Roles in Inflammation and Cancer
Macrophages originate from hematopoietic stem cells in bone marrow, present in the blood as monocytes that migrate to tissues and differentiate into mature macrophages of different types under the stimulation of different environments in the body (Figure 2). Different macrophage subtypes have diverse activities in inflammation and cancer. M1 macrophages are key components of inflammation, and they exert their proinflammatory functions by producing high levels of proinflammatory cytokines, reactive oxygen species, inducible nitric oxide synthase (INOS), cyclooxygenase (COX)-2, and reactive nitrogen species. In addition, they also secrete cytokines such as TNF-α, IL-23, IL-1β, and IL-12, which lead to dysfunctional inflammatory responses that develop into conditions characterized by refractory or severe chronic inflammation, such as rheumatoid arthritis (RA) (31), metabolic syndrome-associated disorders (including type 2 diabetes and atherosclerosis), osteoarthritis, asthma, Crohn's disease, and Alzheimer's disease (32, 33). Thus, decreasing proinflammatory cytokine levels at the source may effectively mitigate inflammatory diseases. M1 macrophages play a role in not only proinflammatory immunity but also antitumor immunity, and they play a protective role in tumorigenesis by promoting and amplifying Th1-type responses (34) and secreting a series of cytokines, including TNFs, growth inhibitors, and antiangiogenetic factors. Therefore, M1 macrophages have potential applications as active biocarriers for anticancer drug delivery to tumors.
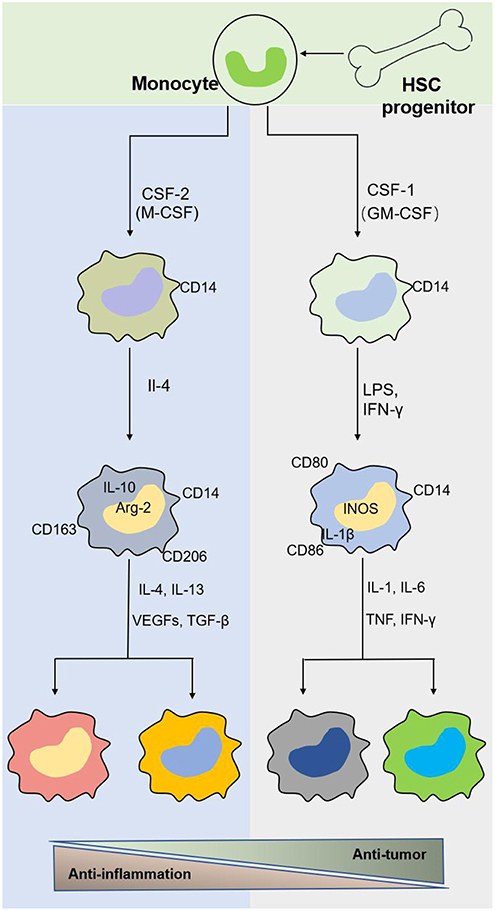
Figure 2. The origin and polarization of macrophages. Tissue macrophages are derived from hematopoietic stem cell (HSC) progenitor cells and exist in blood as monocytes under homeostatic conditions. Monocytes migrate into tissue and differentiate into different macrophages induced by physiologic stimuli, which are associated with a response to inflammatory and cancer conditions.
M2 macrophages participate in antineoplastic immunity by increasing the number of suppressor cells among myeloid-derived suppressor cells (MDSCs), TAMs, and immature monocytes. Macrophages in tumors, also called TAMs, comprise 50% of the TME population and contribute to tumor progression and poor prognosis (35–38). In addition, the percentage of TAMs is inversely proportional to the survival period, i.e., higher TAM numbers are correlated with shorter tumor patient survival (39). Furthermore, M2 macrophages secrete cytokines such as IL-4 or IL-10, IL-13, VEGFs, and TGF-β to participate in the anti-inflammatory response. The effects of macrophages on tumors and inflammation are shown in Figures 2, 3. Based on the dual role of macrophages, nano drugs mainly target negative macrophages in different diseases, delivering relevant drugs to localized areas to change the polarization conditions, thereby leading to a positive conversion between macrophage subtypes.
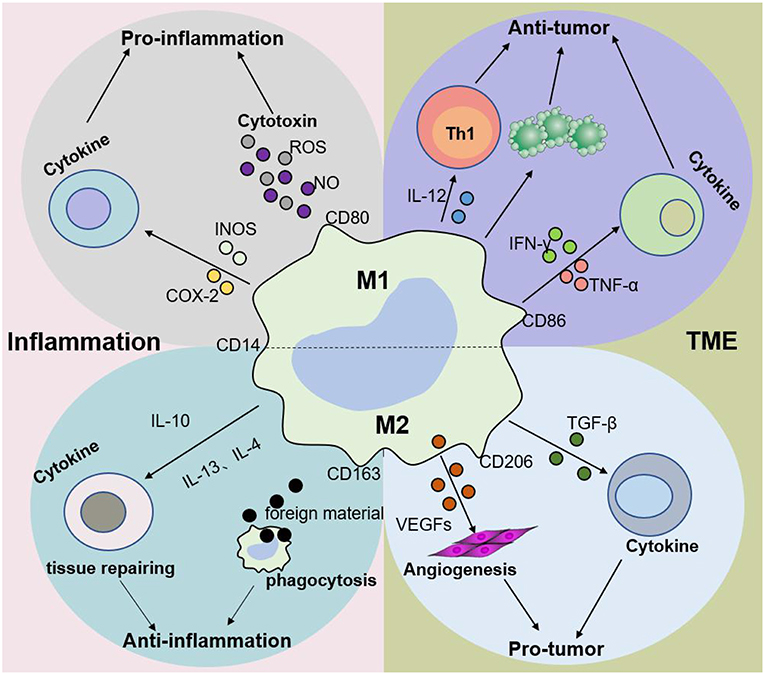
Figure 3. Macrophage subtypes play different roles in inflammation and cancer. In response to various stimuli, such as inflammation, M1 macrophages promote the progression of inflammation, while M2 macrophages mainly play an anti-inflammatory role. Nevertheless, M1 macrophages are mainly involved in antitumor immunity, while M2 macrophages promote tumor growth and invasion in the tumor microenvironment (TME). The dynamic balance between M1 and M2 macrophages jointly determines the evolution of inflammation and tumors.
Although NPs development is progressing quickly, many obstacles, and challenges remain regarding NPs drug delivery systems, which we will now discuss. The first problem that should be addressed is the mononuclear phagocyte system (MPS), which is a family of cells, including bone marrow precursors, blood monocytes, and tissue macrophages (40). The MPS enables the elimination of NPs through phagocytosis. Interestingly, Rodriguez et al. attached “don't eat-me” marker CD47 “self” peptides to the surface of NPs to avoid phagocytosis. As part of the MPS, macrophages become the popular target for its passivity, representing a potential area for elucidating more macrophage-targeting therapeutic methods. After MPS phagocytosis, NPs will enter the blood circulation and must then be cleared (41). Many solutions have been proposed to circumvent this problem, such as the novel multistage delivery vector (MSV) proposed by Blanco et al. aimed at successful delivery in the blood vessel (42, 43). NPs cannot exert their therapeutic effects, involving extravasation, cellular membrane traversal and cellular internalization, until they reached their intended target (44). As the mechanism of NPs drug delivery systems becomes increasingly clear, these obstacles need to be further researched to develop different methods for modifying NPs that can overcome these obstacles.
Strategies for Targeting M1 Macrophages by NPs to Treat Inflammation
Depletion of M1 Macrophages
Based on the negative effect of M1 macrophages on inflammation caused by large numbers of macrophages accumulating at the lesion site, leading to imbalanced inflammation, strategies targeting M1 macrophages in the inflamed tissue with drug-loaded NPs are desirable. In this section, drug-loaded NPs targeting M1 macrophages in the inflammatory environment are discussed. Furthermore, macrophages can quickly and directionally migrate to pathological sites where specific chemokines are being secreted, which allows them to serve as vehicles for the targeted delivery of drugs (45). The strategies of NPs targeting M1 macrophages by delivering specific ligands are illustrated in Figure 4. Two methods currently exist for targeting macrophages: macrophage depletion by NPs-loaded drugs and macrophage re-education by NPs carrying specific cytokines to the microenvironments (46). Thus, in inflammatory disorders, downregulating M1, or repolarizing M1 macrophages to M2 macrophages are two major approaches to relieve inflammation. One example is to use NPs composed of poly(lactic acid)-poly(ethylene glycol) block copolymer (PLA-PEG) to deliver a TNF-α siRNA to M1 macrophages in the model of inflammatory bowel disease (IBD). NPs have been shown to be more powerful and efficient when the Fab' portion of the F4/80 Ab (Fab'-bearing) is grafted onto the NPs surfaces and to attenuate colitis more effectively (47). Interestingly, although LNPs have been extensively studied as delivery systems for nucleic acid therapy, there are also several reports of their application in IBD therapy and targeting macrophages in particular. Recently, Veiga et al. (48) used the Anchored Secondary scFv Enabling Targeting (ASSET) platform to fabricate mRNA-loaded LNPs, which targeted gene expression in Ly6C+ inflammatory leukocytes. The authors then determined the potential therapeutic efficacy of IL10 cytokine expression in Ly6C+ inflammatory leukocytes in a dextran sodium sulfate (DSS) colitis model. All research results indicated that the novel therapeutic strategy of using mRNA-LNPs to specifically target anti-inflammatory cytokines in inflammation-related cells for the treatment of inflammation-related diseases has a promising development prospect (48). Increasing evidence has shown that macrophages, which produce proinflammatory cytokines such as TNF-α that are conducive to disease progression and/or maintenance, play an important role in the pathogenesis of chronic inflammation. Therefore, TNF-α has become a popular target for IBD therapy. Xiao et al. successfully synthesized a macrophage-targeted bioreducible PPM conjugate and utilized it to fabricate NPs carrying sodium triphosphate (TPP) and siRNA by electrostatic interaction. Macrophages can efficiently assimilate these NPs and release high-level RNAi, which decreases TNF-α expression and exerts anti-inflammatory effects in vitro and ex vivo (49). Aouadi et al. developed β1,3-D-glucan-encapsulated siRNA particles (GeRPs) as delivery vehicles that silence genes of mouse macrophages. GeRPs can inhibit the production of TNF-α and IL-4 in macrophages by silencing Map4k4, an unknown mediator of cytokine expression in macrophages (50).
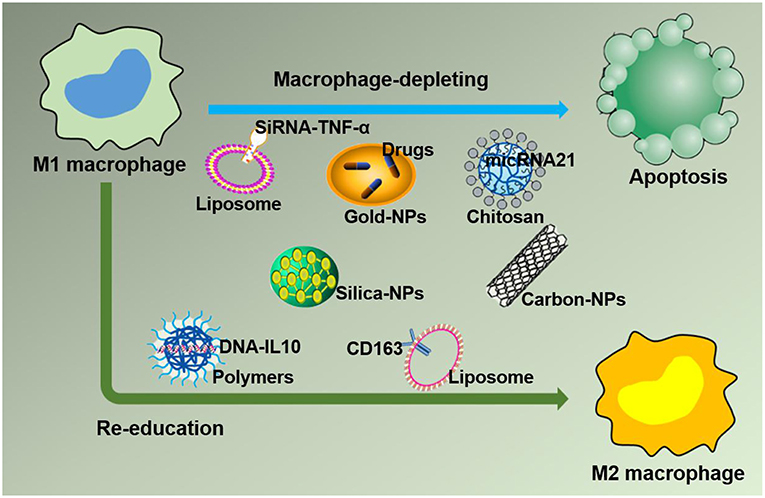
Figure 4. Strategies for nanoparticles packaged with therapeutic agents to target M1 macrophages in inflammation leading to M1 macrophage depletion and re-education.
In a recent study by Bejerano et al., miRNA-21-loaded NPs delivered miRNA-21 to cardiac M1 macrophages after myocardial infarction and subsequently increased angiogenesis, reduced the number of apoptotic cells, and improved cardiac healing by downregulating the expression of TNF-α and iNOS. This study highlighted a new therapeutic strategy to target M1 macrophages using the NPs-mediated delivery of miRNA-21 to resolve inflammation (51). These methods directly target M1 macrophages with nano drugs to decrease the levels of proinflammatory cytokines and have been proven to be an effective strategy to treat diseases in preclinical model (52). This NPs-based approach should significantly benefit patients suffering from inflammatory diseases when the technology is applied clinically in the future.
Re-education of M1 Macrophages
Another novel treatment strategy for chronic inflammatory diseases is repolarizing macrophages from an M1 to an M2 phenotype (Figure 4). RA, an autoimmune disease, manifests as the accumulation of macrophages in the arthritic synovium, which limits drug access and renders RA difficult to treat. Jain et al. attempted to encapsulate the anti-inflammatory (IL-10) cytokine encoding plasmid DNA into non-condensing alginate NPs and then modify the tuftsin peptide to the surface of the nanocarriers to actively target macrophages. This technology enabled nano drugs to easily enter the arthritic synovium to deliver drugs to macrophages and successfully reprogrammed the macrophage phenotype from M1 to M2, which led to the downregulation of proinflammatory cytokine (IL-6, IL-1β, and TNF-α) expression in systemic and joint tissues and eventually prevented the progression of inflammation and joint damage in arthritic rat models (53).
Importantly, NPs expressing targeting ligands themselves or the addition of targeting ligands to the surface enables NPs to specifically target cells through selective binding to the receptors overexpressed on the cell surface. Dextrin may serve as a representative targeting molecule and has been applied as a plasma volume expander in clinical applications due to its high biocompatibility. The development of nanotechnology has increased the potential applications of dextran for the treatment of inflammatory diseases through the synthesis of dextran-NPs that can target macrophages (14). The selective and high efficiency of dextran-NPs at targeting macrophages is due to the expression of dextran-binding C-type lectins and scavenger receptors on their surface, and these NPs are excreted due to metabolic processing (18). Jain et al. already developed novel carriers to transport IL-10 into inflammatory environments to repolarize macrophages from an M1 to an M2 state, which could serve as a novel therapeutic strategy for the treatment of chronic inflammatory diseases (53). Polyethylenimine NPs carrying the gene for CD163 (an M2 macrophage marker) grafted with a mannose ligand can target cells with a monocytic origin, especially M1 macrophages, thereby converting M1 macrophages into M2 macrophages, leading to the release of anti-inflammatory factors to resolve inflammation and the alleviation of inflammatory disease progression (54). Overall, nano drugs are a novel platform for clinical treatments.
Tactics for Using NPs to Target M2 Macrophages in Cancer Treatment
Depletion of TAMs
In regard to TAM targeting, strategies can be divided into two categories: depletion of TAMs and reprogramming of TAMs [(55, 56); Figure 5]. Advanced methods to deplete TAMs include the inhibition of colony-stimulating factor 1 (CSF1)–CSF1 receptor (CSF1R) signaling, which contributes to the apoptosis of a large proportion of TAMs (57). Many clinical treatment strategies that target CSF1R have been developed, including the employment of small molecules and antiCSF1R mAbs, and are summarized in Muhammad Ovais' review (58). Another approach to deplete TAMs is blockading the recruitment of circulating inflammatory monocytes to the tumor site. This process is highly dependent on CC-chemokine ligand 2 (CCL2)–CC-chemokine receptor 2 (CCR2) signaling. By inhibiting the CCL2–CCR2 signaling pathway, mononuclear cells remain in the bone marrow, which leads to a decrease in recruitment to tumor primary and metastatic lesions (59–62). Preclinical trials on the CCL2–CCR2 blockade approach are ongoing. Anti-CCL2 antibodies in combination with chemotherapeutics were proven to be effective for advanced metastatic prostate cancer in murine models (63). In addition, a small molecule CCR2 inhibitor (PF-04136309) was applied in a preclinical pancreatic cancer mouse model, which resulted in reduced tumor growth and fewer liver metastases by reducing the recruitment of inflammatory monocytes to the tumors (64).
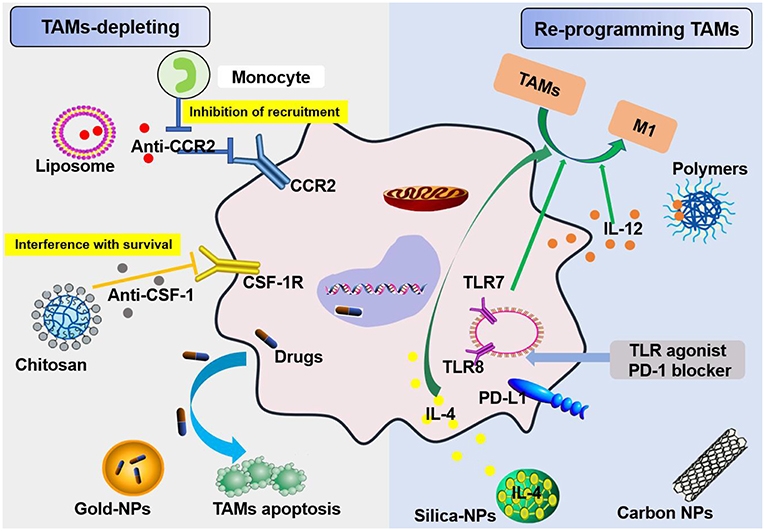
Figure 5. Schemes for nanoparticles loaded with specific agents to target TAM signaling pathways. Nanoparticles target TAMs in the TME via two mechanisms, TAM depletion and TAM reprogramming. The former includes the inhibition of recruitment by blocking the CCL2-CCR2 signaling pathway and survival interference via inhibiting the CSF1-CSF1R signaling pathway. The latter mainly involves cellular re-education to change TAMs into M1 macrophages.
Recent research suggests that TAMs hinder antitumor therapies by expressing PD-1 and inhibiting acquired immunity, like T cells, as well as by inhibiting innate immunity, similar to macrophages. TAMs that produce PD-1 and exhibit poor phagocytosis are much worse at engulfing tumor cells (65). In addition, the TME downregulates CD47 expression on TAMs, and this molecule was recently shown to be involved in an immune checkpoint. Thus, immunotherapies targeting TAMs are very promising strategies for fighting cancer along with current immunotherapies, including chimeric antigen receptor T cell therapy (CAR-T), T cell receptor therapy (TCR-T), cytotoxic T lymphocyte-associated protein (CTLA)4 antibody therapy, programmed death (PD)-1 therapy, and programmed death-ligand (PD-L)1 antibody therapy (66). Furthermore, paclitaxel is currently one of the best natural anticancer drugs and has been developed as a nano drug and approved for clinical use in the treatment of breast cancer, ovarian cancer, and lung cancer. Paclitaxel-loaded NPs can penetrate tumor microvessels in solid tumors via binding a high-affinity LFA-1 I domain with cross-linked amphiphilic copolymers. The LFA-1 I domain can specifically identify ICAM-1, which is overexpressed in inflammatory and neoplastic conditions in areas such as the vasculature and TAMs. The amphiphilic copolymer acts as a drug carrier due to its hydrophilic corona, which produces stability, and its hydrophobic core, which allows encapsulation of paclitaxel because it is not highly soluble in water. Ultimately, nano drug delivery of paclitaxel targets markers prevalent in carcinomas and an inflamed TME, such as TAMs (67). In 2019, Rybakova et al. (68) used an in vitro-transcribed mRNA (IVT-mRNA) system for the in vivo delivery of a humanized anti-HER2 (also known as ERBB2) antibody, trastuzumab, and packaged IVT-mRNA into LNPs. This approach not only ensures anticancer activity and efficient in vivo delivery but also delays degradation of the mRNA (68). Rafique et al. encapsulated calcitriol into LNPs and developed near-infrared calcitriol PEGylated NPs [PEG-LNP(Cal)] by a microfluidic mixing technique. The results showed that calcitriol can be effectively targeted to macrophages (69). Nanotechnology systems for the targeted delivery of drugs to TAMs are effective methods for mitigating tumor promotion (46). These systems also substantially increase the efficiency of chemotherapy and overcome drug resistance in treating cancer (70).
Re-education of TAMs
TAMs are responsible for tumor promotion and malignant tumor development, which is usually associated with poor prognosis. Because TAMs are highly plastic, reprogramming these cells toward a tumoricidal phenotype has been a popular therapeutic strategy.
TAMs promote tumor development through the secretion of immunosuppressive agents. Therefore, TAMs are potential targets for cancer therapy by nano drugs that can reprogram TAMs into antitumorigenic macrophages (71). Reprogramming of TAMs, also called re-education of TAMs, is currently the most attractive strategy for cancer treatment and can reverse the protumor phenotype into the antitumor phenotype (55, 58). This method can slow or stop cancer growth by activating the antitumor functions of M1 macrophages and stimulating the activity of Th1-type cytotoxic T cells and other effector cells (72). In recent years, more researchers have focused on small molecules and NPs formulations for macrophage repolarization, such as Toll-like receptor (TLR) agonists, cytokines, antibodies, and RNAs (73). In addition to the polarization methods mentioned, we summarize the common polarization targets in Table 1. Here, we describe some specific studies that have been performed regarding TAM depolarization. Polymeric NPs have been designed and synthesized with an IL-12 payload to re-educate TAMs, eventually promoting macrophage conversion from the M2 to the M1 phenotype, demonstrating the promise of nanomaterials as a platform for cancer immunotherapy (78). TLR agonists are a potential method to polarize TAMs into M1-like cells (82). NPs loaded with TLR agonists targeting TAMs are currently being tested as antitumoral agents in animal models. In 2018, Rodell et al. established that R848-loaded β-cyclodextrin NPs (CDNP-R848) contributed to efficient drug delivery to TAMs. The authors capitalized on β-cyclodextrin (CD), which enables drug solubilization, and R848, an agonist of TLR7 and TLR8 that can target TAMs and eventually shift TAMs to an M1 phenotype, thereby improving cancer immunotherapy and controlling tumor growth. This method has been utilized in animal models, protecting the animals against tumor rechallenge and improving immunotherapeutic responses when used in combination with the immune checkpoint inhibitor anti-PD-1. This treatment strategy represents a tool to transform TAMs into an M1-like phenotype and subsequently promote tumor regression and increase the efficacy of immune checkpoint blockade (83, 84). Another successful example is the encapsulation of miR-125b into CD44 targeting hyaluronic acid-poly(ethylenimine) (HA-PEI)-based NPs by Parayath et al. After abundant scientific experiments, the authors determined that this type of NPs successfully enables the transfection of TAMs and demonstrated its contribution to TAM repolarization in anticancer immunotherapy (85).
More than that, carbohydrates like mannose can also employ to target macrophages. The expression of mannose receptors in the cells of the immune system was preferential which made mannose become a popular ligand targeting macrophages (86). Lots of new methods using mannose as targeting ligand have been proposed in recent months. Wang et al. developed twin-like core shell NPs (TCN) for targeting delivery of sorafenib and TAMs re-polarization agents IMD-0354 to cancer cells and TAMs which could enhance tumor-localized chemoimmunotherapy. Mannose, as a targeting ligand was anchored to IMD-0354 for selective targeting delivery to TAMs (87). Zhao et al. synthesized the albumin NPs modified with dual ligands, a transferrin receptor (TfR)-binding peptide T12 and mannose. They proved that this system efficiently inhibited the glioma cell proliferation and successfully “re-educated” the protumor M2 toward antitumor M1 (88). Most of mannose as ligand targeting macrophages now are applied for macrophage re-polarization. It is helpful and of great potential to be used in tumor-localized chemoimmunotherapy. Additionally, TAMs can activate the nuclear factor (NF)-κB pathway by producing ligands to target protumor genes such as CXCL12 and VEGFC via the CD40 receptor (89). Interestingly, CD40 blockage also leads to the upregulation of IL12, which can repolarize TAMs into M1 macrophages. For instance, NPs loaded with a corresponding inhibitor can block the NF-κB signaling pathway, which can switch TAMs into M1 macrophages that are toxic to tumor cells; this approach has a bright future in cancer treatment (90). Additionally, for successful nanocarrier drug delivery to macrophages, different drugs and their individual physicochemical properties should be considered. The encapsulation strategies, including the covalent stimulus-responsive covalent linking of drugs to the carrier and different release rates, are summarized in Table 2. In all, NPs deliver specific ligands to M2 macrophages to modulate the function in tumors, as summarized in Figure 5.
However, when trying to target TAMs in the TME, the infiltration of nano drugs should be considered. To avoid the uptake of infiltrated nano drugs by normal macrophages and promote the retention of nano drugs in tissues, the following methods may be used. First, high interstitial fluid pressure (IFP) is one of the most important features of the TME, as it contributes to NPs accumulation in tumors and restricts their extravasation and penetration (108). Another strategy is utilizing a pH-sensitive design for NPs. Because acidosis is a typical physiological factor of the TME and the tumor pH ranges from 6.5 to 6.8 while the pH in healthy tissues is 7.4, NPs designed to be pH-sensitive can release drugs in tumors and exist stably in normal tissues (19, 109). For instance, polymers, including poly(acryl amide) (PAAm), micelles and liposomes, can release drugs by changing structures in the TME through protonation or deprotonation (110–113). These approaches may prevent NPs from participating in humoral circulation and being ingested by normal tissue macrophages.
Although advanced cancer research has revealed that the TME affects cancer progression and metastasis (71), some specific TME conditions could be used to promote NPs uptake by TAMs in tumors. For example, the enhanced permeability and retention (EPR) effect is a typical characteristic of the TME (114). EPR may be related to the difference between tumor vasculature and normal blood vessels, with leaky capillary gaps of the former being ~100–780 nm and those of the physiological blood vessels being ~5–8 nm (115–117). Many studies have shown that NPs accumulate more in tumors than in other tissues due to the heterogeneity of the tumor vasculature and EPR effects. In addition, the half-life of the drug in the NPs system is prolonged for escapement from renal clearance (118). These two conditions, which encompass the blood circulation stability of NPs and the EPR effect, provide an avenue for drugs to reach and combat tumors. The EPR effect makes NPs extravasate from the tumor vasculature and transport into tumor cells and stroma, which leads to the accumulation of antitumor drugs in the TME at high concentrations and a reduction in systemic side effects. Exploiting this EPR effect and active targeting moieties was shown to be beneficial for the success of NPs-based therapy and for coping with delivery-related drug resistance (119). Therapeutic agents based on NPs targeting TAMs may represent a new approach to curing cancer in the clinic.
We have thus far summarized two methods for targeting macrophages with NPs in these two environments, depletion and re-education of macrophages. Polarizing active M1 macrophages into the immunosuppressive M2 phenotype in an inflammatory environment and repolarizing M2 macrophages into the immunoactive M1 state in the TME appear to be most effective in different circumstances. In fact, beyond the consumption of M1 macrophages in inflammation and TAMs in cancer, their main disadvantage is the loss of their potential immune stimulatory effects as major phagocytes and specialized antigen presenting cells. Therefore, the functional repolarization of macrophages to enhance their beneficial function and limit their detrimental properties is currently the most attractive strategy for disease treatment.
Diagnostic Purposes of Targeting Macrophages
As stated above, targeting different macrophage subtypes by different NPs can efficiently cure inflammation and cancer in animal models. In addition, macrophage targeting might be used for disease diagnosis, especially for malignant lesions, beyond the delivery of TAM-targeting therapeutic drugs by NPs. However, this approach is linked to the design, development, and application of NPs (120). Based on the type of NPs, the PET, CT, MRI imaging modalities, and their combinations can be used to detect the accumulation of NPs in macrophages (121). For example, MRI-compatible nanomaterials, such as iron oxide NPs, can be rapidly recognized and phagocytosed by macrophages, which leads to a positive MR signal effect on T1-weighted MR images, and the signal strength is proportional to the number of macrophages, thereby providing a non-invasive method to assess the progression and prognosis of diseases and facilitating treatment-related decisions by quantifying TAMs in tumors (122). Interestingly, abnormal patterns of NPs accumulation are helpful for the accurate detection of lymph node metastases in prostate cancer, which is judged by unique MRI features (123, 124). The clinical trial (NCT01770353) of MM-398 (nanoliposomal irinotecan, Nal-IRI) assessing the practicability of ferumoxytol by imaging TAMs to predict patient response is currently ongoing. Furthermore, the binding of various molecular markers of macrophage subtypes to NPs surfaces allows specific recognition by macrophages (125). For instance, NPs-labeled Ly-6Chigh monocyte biomarkers are more efficient than their Ly-6Clow counterparts in mice (126). Therefore, developing non-invasive methods for imaging macrophages holds substantial promise.
Concluding Remarks
In summary, we report the current status of macrophage-targeting NPs in the context of inflammatory disorders and cancers. Together with the facts that macrophages are naturally involved in and crucial for the pathogenesis of inflammation/cancer and that current nanotechnologies can design and generate NPs that specifically target macrophages, the rich variety of examples discussed above makes us confident that well-engineered NPs targeting macrophages demonstrate a new paradigm in a wide range of therapies for cancer and inflammatory disorders, such as acute lung injury, RA, stroke, atherosclerosis and myocardial infarction.
While NPs targeting macrophages have shown potential in disease treatment, many interesting questions and challenges still need to be addressed. First, as we know, the time course of macrophage infiltration is always dependent on the pathogenesis of the disease and its stages; thus, studies on the dynamics of macrophage activation and trafficking in cancer and inflammatory disorders are needed for optimization of the best time to deliver NPs to better target infiltrated macrophages. Second, recent studies have highlighted the complexity and phenotypic heterogeneity of inflammatory monocyte-derived cells or resident macrophage populations in different tissues and disease contexts (127). As their variability extends far beyond the simplistic classifications of M1 and M2 phenotypes, NPs must be engineered to achieve selective targeting by clearly elucidating and differentiating the specific subtypes. Last but not least, due to the heterogeneity of the inflammatory/tumor microenvironment, NPs–cell interactions are also vital mechanisms that must be elucidated. These NPs–cell interactions, including but not limited to the internalization and processing of nanomaterials by macrophages and other immune cells, are only partially understood and play an important role in both therapeutic and imaging efficacy.
Initial data from macrophage-targeted pharmacological interventions in clinical trials indicate that macrophage-targeting strategies can be successfully translated into novel clinical treatment options (128, 129). However, for the clinical translation of NPs-based macrophage-targeting therapies, further work is required to explore the safety of NPs, patient-specific responses, disease-type specificity, and precise targeting/imaging technology to close the gap between the bench and clinic.
Author Contributions
GH and MG wrote the draft. YJ reviewed and edited the manuscript before submission. JX, FW, JF, QH, GY, ZL, and XW commented and added extra information.
Funding
This paper was supported by the National Natural Science Foundation of China (nos. 81572942 and 81770096), the State Project for Essential Drug Research and Development, China (no. 2018ZX09301001001), the Natural Science Foundation of Hubei Province (no. 2014CFA057), the Special Fund for Technological Innovation of Hubei (no. 2017ACA094), the Health and Planning Commission Fund of Hubei Province (no. WJ2017M098), the Science and Technology Support Program of Hubei Province (no. YSF2015001294), the Wuhan Planning Project of Science and Technology (no. 2014060101010036), and the Special Fund for Industrial Transformation and Upgrading.
Conflict of Interest Statement
The authors declare that the research was conducted in the absence of any commercial or financial relationships that could be construed as a potential conflict of interest.
References
1. Cros J, Cagnard N, Woollard K, Patey N, Zhang SY, Senechal B, et al. Human CD14dim monocytes patrol and sense nucleic acids and viruses via TLR7 and TLR8 receptors. Immunity. (2010) 33:375–86. doi: 10.1016/j.immuni.2010.08.012
2. Gordon S, Taylor PR. Monocyte and macrophage heterogeneity. Nat Rev Immunol. (2005) 5:953–64. doi: 10.1038/nri1733
3. Murray PJ, Wynn TA. Obstacles and opportunities for understanding macrophage polarization. J Leukocyte Biol. (2011) 89:557–63. doi: 10.1189/jlb.0710409
4. Murray PJ, Wynn TA. Protective and pathogenic functions of macrophage subsets. Nat Rev Immunol. (2011) 11:723–37. doi: 10.1038/nri3073
5. Mosser DM, Edwards JP. Exploring the full spectrum of macrophage activation. Nat Rev Immunol. (2008) 8:958–69. doi: 10.1038/nri2448
6. Sutterwala FS, Noel GJ, Clynes R, Mosser DM. Selective suppression of interleukin-12 induction after macrophage receptor ligation. J Exp Med. (1997) 185:1977–85. doi: 10.1084/jem.185.11.1977
7. Sutterwala FS, Noel GJ, Salgame P, Mosser DM. Reversal of proinflammatory responses by ligating the macrophage Fcgamma receptor type I. J Exp Med. (1998) 188:217–22. doi: 10.1084/jem.188.1.217
8. Moghimi SM, Hunter AC, Murray JC. Nanomedicine: current status and future prospects. FASEB J. (2005) 19:311–30. doi: 10.1096/fj.04-2747rev
9. Nguyen TX, Huang L, Gauthier M, Yang G, Wang Q. Recent advances in liposome surface modification for oral drug delivery. Nanomedicine. (2016) 11:1169–85. doi: 10.2217/nnm.16.9
10. Ren H, He Y, Liang J, Cheng Z, Zhang M, Zhu Y, et al. Role of liposome size, surface charge, and PEGylation on rheumatoid arthritis targeting therapy. ACS Appl Mater Interfaces. (2019) 11:20304–15. doi: 10.1021/acsami.8b22693
11. Rao W, Wang H, Han J, Zhao S, Dumbleton J, Agarwal P, et al. Chitosan-decorated doxorubicin-encapsulated nanoparticle targets and eliminates tumor reinitiating cancer stem-like cells. ACS Nano. (2015) 9:5725–40. doi: 10.1021/nn506928p
12. Danhier F, Ansorena E, Silva JM, Coco R, Le Breton A, Preat V. PLGA-based nanoparticles: an overview of biomedical applications. J Controlled Release. (2012) 161:505–22. doi: 10.1016/j.jconrel.2012.01.043
13. Acharya S, Sahoo SK. PLGA nanoparticles containing various anticancer agents and tumour delivery by EPR effect. Adv Drug Deliv Rev. (2011) 63:170–83. doi: 10.1016/j.addr.2010.10.008
14. Ma L, Liu TW, Wallig MA, Dobrucki IT, Dobrucki LW, Nelson ER, et al. Efficient targeting of adipose tissue macrophages in obesity with polysaccharide nanocarriers. ACS Nano. (2016) 10:6952–62. doi: 10.1021/acsnano.6b02878
15. Diab R, Canilho N, Pavel IA, Haffner FB, Girardon M, Pasc A. Silica-based systems for oral delivery of drugs, macromolecules and cells. Adv Colloid Interface Sci. (2017) 249:346–62. doi: 10.1016/j.cis.2017.04.005
16. Mody VV, Siwale R, Singh A, Mody HR. Introduction to metallic nanoparticles. J Pharmacy Bioall Sci. (2010) 2:282–9. doi: 10.4103/0975-7406.72127
17. Pang L, Qin J, Han L, Zhao W, Liang J, Xie Z, et al. Exploiting macrophages as targeted carrier to guide nanoparticles into glioma. Oncotarget. (2016) 7:37081–91. doi: 10.18632/oncotarget.9464
18. Pustylnikov S, Sagar D, Jain P, Khan ZK. Targeting the C-type lectins-mediated host-pathogen interactions with dextran. J Pharm Pharm Sci. (2014) 17:371–92. doi: 10.18433/J3N590
19. Sun T, Zhang YS, Pang B, Hyun DC, Yang M, Xia Y. Engineered nanoparticles for drug delivery in cancer therapy. Angew Chemie. (2014) 53:12320–64. doi: 10.1002/anie.201403036
20. Bowerman CJ, Byrne JD, Chu KS, Schorzman AN, Keeler AW, Sherwood CA, et al. Docetaxel-loaded PLGA nanoparticles improve efficacy in taxane-resistant triple-negative breast cancer. Nano Lett. (2017) 17:242–8. doi: 10.1021/acs.nanolett.6b03971
21. Kwon D, Cha BG, Cho Y, Min J, Park EB, Kang SJ, et al. Extra-large pore mesoporous silica nanoparticles for directing in vivo M2 macrophage polarization by delivering IL-4. (2017) 17:2747–56. doi: 10.1021/acs.nanolett.6b04130
22. Lu J, Liu X, Liao YP, Salazar F, Sun B. Nano-enabled pancreas cancer immunotherapy using immunogenic cell death and reversing immunosuppression. Nat Comm. (2017) 8:1811. doi: 10.1038/s41467-017-01651-9
23. Man RWY, Li CH, MacLean MWA, Zenkina OV, Zamora MT, Saunders LN, et al. Ultra stable gold nanoparticles modified by bidentate N-heterocyclic carbene ligands. J Am Chem Soc. (2017) 140:1576–9. doi: 10.1021/jacs.7b08516
24. Patel S, Ashwanikumar N, Robinson E, DuRoss A, Sun C, Murphy-Benenato KE, et al. Boosting intracellular delivery of lipid nanoparticle-encapsulated mRNA. Nano Lett. (2017) 17:5711–8. doi: 10.1021/acs.nanolett.7b02664
25. Kulkarni JA, Cullis PR, van der Meel R. Lipid nanoparticles enabling gene therapies: from concepts to clinical utility. Nucl Acid Ther. (2018) 28:146–57. doi: 10.1089/nat.2018.0721
26. Matsumura Y, Maeda H. A new concept for macromolecular therapeutics in cancer chemotherapy: mechanism of tumoritropic accumulation of proteins and the antitumor agent smancs. Cancer Res. (1986) 46(12 Pt 1):6387–92.
27. Minko T, Kopeckova P, Kopecek J. Comparison of the anticancer effect of free and HPMA copolymer-bound adriamycin in human ovarian carcinoma cells. Pharm Res. (1999) 16:986–96. doi: 10.1023/A:1018959029186
28. Couvreur P. Nanoparticles in drug delivery: past, present and future. Adv Drug Deliv Rev. (2013) 65:21–3. doi: 10.1016/j.addr.2012.04.010
29. Bao G, Mitragotri S, Tong S. Multifunctional nanoparticles for drug delivery and molecular imaging. Ann Rev Biomed Eng. (2013) 15:253–82. doi: 10.1146/annurev-bioeng-071812-152409
30. Matoba T, Koga JI, Nakano K, Egashira K, Tsutsui H. Nanoparticle-mediated drug delivery system for atherosclerotic cardiovascular disease. J Card. (2017) 70:206–11. doi: 10.1016/j.jjcc.2017.03.005
31. Wong SH, Lord JM. Factors underlying chronic inflammation in rheumatoid arthritis. Arch Immunol Ther Exp. (2004) 52:379–88.
32. Libby P. Inflammation and cardiovascular disease mechanisms. Am J Clin Nutr. (2006) 83:456s−60s. doi: 10.1093/ajcn/83.2.456S
33. Okin D, Medzhitov R. Evolution of inflammatory diseases. Curr Biol. (2012) 22:R733–40. doi: 10.1016/j.cub.2012.07.029
34. Biswas SK, Mantovani A. Macrophage plasticity and interaction with lymphocyte subsets: cancer as a paradigm. Nat Immunol. (2010) 11:889–96. doi: 10.1038/ni.1937
35. Casulo C, Arcila M, Bohn OL, Teruya-Feldstein J, Maragulia J, Moskowitz CH. Tumor associated macrophages in relapsed and refractory Hodgkin lymphoma. Leukem Res. (2013) 37:1178–83. doi: 10.1016/j.leukres.2013.03.021
36. Ahn GO, Tseng D, Liao CH, Dorie MJ, Czechowicz A, Brown JM. Inhibition of Mac-1. (CD11b/CD18) enhances tumor response to radiation by reducing myeloid cell recruitment. Proc Natl Acad Sci USA. (2010) 107:8363–8. doi: 10.1073/pnas.0911378107
37. Pollard JW. Tumour-educated macrophages promote tumour progression and metastasis. Nat Rev Cancer. (2004) 4:71–8. doi: 10.1038/nrc1256
38. Morrison C. Immuno-oncologists eye up macrophage targets. Nat Rev Drug Disc. (2016) 15:373–4. doi: 10.1038/nrd.2016.111
39. Steidl C, Lee T, Shah SP, Farinha P, Han G, Nayar T, et al. Tumor-associated macrophages and survival in classic Hodgkin's lymphoma. N Engl J Med. (2010) 362:875–85. doi: 10.1056/NEJMoa0905680
40. Jenkins SJ, Hume DA. Homeostasis in the mononuclear phagocyte system. Trends Immunol. (2014) 35:358–67. doi: 10.1016/j.it.2014.06.006
41. Rodriguez PL, Harada T, Christian DA, Pantano DA, Tsai RK, Discher DE. Minimal “Self” peptides that inhibit phagocytic clearance and enhance delivery of nanoparticles. Science. (2013) 339:971–5. doi: 10.1126/science.1229568
42. Ferrari M. Frontiers in cancer nanomedicine: directing mass transport through biological barriers. Trends Biotechnol. (2010) 28:181–8. doi: 10.1016/j.tibtech.2009.12.007
43. Tasciotti E, Liu X, Bhavane R, Plant K, Leonard AD, Price BK, et al. Mesoporous silicon particles as a multistage delivery system for imaging and therapeutic applications. Nat Nanotechnol. (2008) 3:151–7. doi: 10.1038/nnano.2008.34
44. Blanco E, Shen H, Ferrari M. Principles of nanoparticle design for overcoming biological barriers to drug delivery. Nat Biotechnol. (2015) 33:941–51. doi: 10.1038/nbt.3330
45. Mantovani A, Sica A, Sozzani S, Allavena P, Vecchi A, Locati M. The chemokine system in diverse forms of macrophage activation and polarization. Trends Immunol. (2004) 25:677–86. doi: 10.1016/j.it.2004.09.015
46. Ngambenjawong C, Gustafson HH, Pun SH. Progress in tumor-associated macrophage (TAM)-targeted therapeutics. Adv Drug Deliv Rev. (2017) 114:206–21. doi: 10.1016/j.addr.2017.04.010
47. Laroui H, Viennois E, Xiao B, Canup BS, Geem D, Denning TL, et al. Fab'-bearing siRNA TNFalpha-loaded nanoparticles targeted to colonic macrophages offer an effective therapy for experimental colitis. J Control Release. (2014) 186:41–53. doi: 10.1016/j.jconrel.2014.04.046
48. Veiga N, Goldsmith M, Granot Y, Rosenblum D, Dammes N, Kedmi R, et al. Cell specific delivery of modified mRNA expressing therapeutic proteins to leukocytes. Nat Comm. (2018) 9:4493. doi: 10.1038/s41467-018-06936-1
49. Xiao B, Laroui H, Ayyadurai S, Viennois E, Charania MA, Zhang Y, et al. Mannosylated bioreducible nanoparticle-mediated macrophage-specific TNF-alpha RNA interference for IBD therapy. Biomaterials. (2013) 34:7471–82. doi: 10.1016/j.biomaterials.2013.06.008
50. Aouadi M, Tesz GJ, Nicoloro SM, Wang M, Chouinard M, Soto E, et al. Orally delivered siRNA targeting macrophage Map4k4 suppresses systemic inflammation. Nature. (2009) 458:1180–4. doi: 10.1038/nature07774
51. Bejerano T. Nanoparticle delivery of miRNA-21 mimic to cardiac macrophages improves myocardial remodeling after myocardial infarction. Nano Lett. (2018) 18:5885–91. doi: 10.1021/acs.nanolett.8b02578
52. Singh A, Talekar M, Raikar A, Amiji M. Macrophage-targeted delivery systems for nucleic acid therapy of inflammatory diseases. J Control Release. (2014) 190:515–30. doi: 10.1016/j.jconrel.2014.04.021
53. Jain S, Tran TH, Amiji M. Macrophage repolarization with targeted alginate nanoparticles containing IL-10 plasmid DNA for the treatment of experimental arthritis. Biomaterials. (2015) 61:162–77. doi: 10.1016/j.biomaterials.2015.05.028
54. Alvarado-Vazquez PA, Bernal L, Paige CA, Grosick RL, Moracho Vilrriales C, Ferreira DW, et al. Macrophage-specific nanotechnology-driven CD163 overexpression in human macrophages results in an M2 phenotype under inflammatory conditions. Immunobiology. (2017) 222:900–12. doi: 10.1016/j.imbio.2017.05.011
55. DeNardo DG, Ruffell B. Macrophages as regulators of tumour immunity and immunotherapy. Nat Rev Immunol. (2019) 19:369–82. doi: 10.1038/s41577-019-0127-6
56. Ostuni R, Kratochvill F, Murray PJ, Natoli G. Macrophages and cancer: from mechanisms to therapeutic implications. Trends Immunol. (2015) 36:229–39. doi: 10.1016/j.it.2015.02.004
57. Pyonteck SM, Akkari L, Schuhmacher AJ, Bowman RL, Sevenich L, Quail DF, et al. CSF-1R inhibition alters macrophage polarization and blocks glioma progression. Nat Med. (2013) 19:1264–72. doi: 10.1038/nm.3337
58. Ovais M, Guo M, Chen C. Tailoring nanomaterials for targeting tumor-associated macrophages. Adv Mater. (2019) 31:e1808303. doi: 10.1002/adma.201808303
59. Nywening TM, Wang-Gillam A, Sanford DE, Belt BA, Panni RZ, Cusworth BM, et al. Targeting tumour-associated macrophages with CCR2 inhibition in combination with FOLFIRINOX in patients with borderline resectable and locally advanced pancreatic cancer: a single-centre, open-label, dose-finding, non-randomised, phase 1b trial. Lancet Oncol. (2016) 17:651–62. doi: 10.1016/S1470-2045(16)00078-4
60. Lim SY, Yuzhalin AE, Gordon-Weeks AN, Muschel RJ. Targeting the CCL2-CCR2 signaling axis in cancer metastasis. Oncotarget. (2016) 7:28697–710. doi: 10.18632/oncotarget.7376
61. Zhang J, Patel L, Pienta KJ. CC chemokine ligand 2 (CCL2) promotes prostate cancer tumorigenesis and metastasis. Cytokine Growth Factor Rev. (2010) 21:41–8. doi: 10.1016/j.cytogfr.2009.11.009
62. Qian BZ, Li J, Zhang H, Kitamura T, Zhang J, Campion LR, et al. CCL2 recruits inflammatory monocytes to facilitate breast-tumour metastasis. Nature. (2011) 475:222–5. doi: 10.1038/nature10138
63. Kirk PS, Koreckij T, Nguyen HM, Brown LG, Snyder LA, Vessella RL, et al. Inhibition of CCL2 signaling in combination with docetaxel treatment has profound inhibitory effects on prostate cancer growth in bone. Int J Mol Sci. (2013) 14:10483–96. doi: 10.3390/ijms140510483
64. Sanford DE, Belt BA, Panni RZ, Mayer A, Deshpande AD, Carpenter D, et al. Inflammatory monocyte mobilization decreases patient survival in pancreatic cancer: a role for targeting the CCL2/CCR2 axis. Clin Cancer Res. (2013) 19:3404–15. doi: 10.1158/1078-0432.CCR-13-0525
65. Gordon SR, Maute RL, Dulken BW, Hutter G, George BM, McCracken MN, et al. PD-1 expression by tumour-associated macrophages inhibits phagocytosis and tumour immunity. Nature. (2017) 545:495–9. doi: 10.1038/nature22396
66. Tseng D, Volkmer JP, Willingham SB, Contreras-Trujillo H, Fathman JW, Fernhoff NB, et al. Anti-CD47 antibody-mediated phagocytosis of cancer by macrophages primes an effective antitumor T-cell response. Proc Natl Acad Sci USA. (2013) 110:11103–8. doi: 10.1073/pnas.1305569110
67. Park S, Kang S, Chen X, Kim EJ, Kim J, Kim N, et al. Tumor suppression via paclitaxel-loaded drug carriers that target inflammation marker upregulated in tumor vasculature and macrophages. Biomaterials. (2013) 34:598–605. doi: 10.1016/j.biomaterials.2012.10.004
68. Rybakova Y, Kowalski PS, Huang Y, Gonzalez JT, Heartlein MW, DeRosa F, et al. mRNA delivery for therapeutic anti-HER2 antibody expression in vivo. Mol Ther. (2019). doi: 10.1016/j.ymthe.2019.05.012
69. Rafique A, Etzerodt A, Graversen JH, Moestrup SK, Dagnaes-Hansen F, Moller HJ. Targeted lipid nanoparticle delivery of calcitriol to human monocyte-derived macrophages in vitro and in vivo: investigation of the anti-inflammatory effects of calcitriol. Int J Nanomed. (2019) 14:2829–46. doi: 10.2147/IJN.S192113
70. Silva V, Al-Jamal WT. Exploiting the cancer niche: tumor-associated macrophages and hypoxia as promising synergistic targets for nano-based therapy. J Control Release. (2017) 253:82–96. doi: 10.1016/j.jconrel.2017.03.013
71. Muntimadugu E, Kommineni N, Khan W. Exploring the potential of nanotherapeutics in targeting tumor microenvironment for cancer therapy. Pharmacol Res. (2017) 126:109–22. doi: 10.1016/j.phrs.2017.05.010
72. Mills CD, Lenz LL, Harris RA. A breakthrough: macrophage-directed cancer immunotherapy. Cancer Res. (2016) 76:513–6. doi: 10.1158/0008-5472.CAN-15-1737
73. van Dalen FJ, van Stevendaal M, Fennemann FL. Molecular repolarisation of tumour-associated macrophages. Molecules. (2018) 24:9. doi: 10.3390/molecules24010009
74. Wang C, Hu Z, Zhu Z, Zhang X, Wei Z, Zhang Y, et al. The MSHA strain of Pseudomonas aeruginosa (PA-MSHA) inhibits gastric carcinoma progression by inducing M1 macrophage polarization. Tumour Biol. (2016) 37:6913–21. doi: 10.1007/s13277-015-4451-6
75. Vidyarthi A, Khan N, Agnihotri T, Negi S, Das DK, Aqdas M, et al. TLR-3 stimulation skews M2 macrophages to M1 through IFN-alphabeta signaling and restricts tumor progression. Front Immunol. (2018) 9:1650. doi: 10.3389/fimmu.2018.01650
76. Wiehagen KR, Girgis NM, Yamada DH, Smith AA, Chan SR, Grewal IS, et al. Combination of CD40 agonism and CSF-1R blockade reconditions tumor-associated macrophages and drives potent antitumor immunity. Cancer Immunol Res. (2017) 5:1109–21. doi: 10.1158/2326-6066.CIR-17-0258
77. Zhang JQ, Zeng S, Vitiello GA, Seifert AM, Medina BD, Beckman MJ, et al. Macrophages and CD8(+) T cells mediate the antitumor efficacy of combined CD40 ligation and imatinib therapy in gastrointestinal stromal tumors. Cancer Immunol Res. (2018) 6:434–47. doi: 10.1158/2326-6066.CIR-17-0345
78. Wang Y, Lin YX, Qiao SL, An HW, Ma Y, Qiao ZY, et al. Polymeric nanoparticles promote macrophage reversal from M2 to M1 phenotypes in the tumor microenvironment. Biomaterials. (2017) 112:153–63. doi: 10.1016/j.biomaterials.2016.09.034
79. Yang FC, Chiu PY, Chen Y, Mak TW, Chen NJ. TREM-1-dependent M1 macrophage polarization restores intestinal epithelium damaged by DSS-induced colitis by activating IL-22-producing innate lymphoid cells. J Biomed Sci. (2019) 26:46. doi: 10.1186/s12929-019-0539-4
80. Qiu Q, Li C, Song Y, Shi T, Luo X, Zhang H, et al. Targeted delivery of ibrutinib to tumor-associated macrophages by sialic acid-stearic acid conjugate modified nanocomplexes for cancer immunotherapy. Acta Biomater. (2019) 92:184–95. doi: 10.1016/j.actbio.2019.05.030
81. Na YR, Yoon YN, Son D, Jung D, Gu GJ, Seok SH. Consistent inhibition of cyclooxygenase drives macrophages towards the inflammatory phenotype. PLoS ONE. (2015) 10:e0118203. doi: 10.1371/journal.pone.0118203
82. Shime H, Matsumoto M, Oshiumi H, Tanaka S, Nakane A, Iwakura Y, et al. Toll-like receptor 3 signaling converts tumor-supporting myeloid cells to tumoricidal effectors. Proc Natl Acad Sci USA. (2012) 109:2066–71. doi: 10.1073/pnas.1113099109
83. Rodell CB, Arlauckas SP, Cuccarese MF, Garris CS, Li R, Ahmed MS, et al. TLR7/8-agonist-loaded nanoparticles promote the polarization of tumour-associated macrophages to enhance cancer immunotherapy. Nat Biomed Eng. (2018) 2:578–88. doi: 10.1038/s41551-018-0236-8
84. Cully M. Cancer: re-educating tumour-associated macrophages with nanoparticles. Nat Rev Drug Disc. (2018) 17:468. doi: 10.1038/nrd.2018.102
85. Parayath NN, Parikh A, Amiji MM. Repolarization of tumor-associated macrophages in a genetically engineered nonsmall cell lung cancer model by intraperitoneal administration of hyaluronic acid-based nanoparticles encapsulating MicroRNA-125b. Nano Lett. (2018) 18:3571–9. doi: 10.1021/acs.nanolett.8b00689
86. Irache JM, Salman HH, Gamazo C, Espuelas S. Mannose-targeted systems for the delivery of therapeutics. Expert Opin Drug Deliv. (2008) 5:703–24. doi: 10.1517/17425247.5.6.703
87. Wang T, Zhang J, Hou T, Yin X, Zhang N. Selective targeting of tumor cells and tumor associated macrophages separately by twin-like core-shell nanoparticles for enhanced tumor-localized chemoimmunotherapy. Nanoscale. (2019) 11:13934–46. doi: 10.1039/C9NR03374B
88. Zhao P, Wang Y, Kang X, Wu A, Yin W, Tang Y, et al. Dual-targeting biomimetic delivery for anti-glioma activity via remodeling the tumor microenvironment and directing macrophage-mediated immunotherapy. Chem Sci. (2018) 9:2674–89. doi: 10.1039/C7SC04853J
89. Biswas SK, Lewis CE. NF-κB as a central regulator of macrophage function in tumors. J Leukocyte Biol. (2010) 88:877–84. doi: 10.1189/jlb.0310153
90. Fong CH, Bebien M, Didierlaurent A, Nebauer R, Hussell T, Broide D, et al. An antiinflammatory role for IKKbeta through the inhibition of “classical” macrophage activation. J Exp Med. (2008) 205:1269–76. doi: 10.1084/jem.20080124
91. Kita K, Dittrich C. Drug delivery vehicles with improved encapsulation efficiency: taking advantage of specific drug-carrier interactions. Expert Opin Drug Deliv. (2011) 8:329–42. doi: 10.1517/17425247.2011.553216
92. Steerenberg PA, Storm G, de Groot G, Claessen A, Bergers JJ, Franken MA, et al. Liposomes as drug carrier system for cis-diamminedichloroplatinum (II). II. Antitumor activity in vivo, induction of drug resistance, nephrotoxicity, and Pt distribution. Cancer Chemother Pharmacol. (1988) 21:299–307. doi: 10.1007/BF00264195
93. Chen Q, Luo L, Xue Y, Han J, Liu Y, Zhang Y, et al. Cisplatin-loaded polymeric complex micelles with a modulated drug/copolymer ratio for improved in vivo performance. Acta Biomater. (2019) 92:205–18. doi: 10.1016/j.actbio.2019.05.007
94. Beaumier PL, Hwang KJ. An efficient method for loading indium-111 into liposomes using acetylacetone. J Nucl Med. (1982) 23:810–5.
95. Hwang KJ, Merriam JE, Beaumier PL, Luk KF. Encapsulation, with high efficiency, of radioactive metal ions in liposomes. Biochim Biophys Acta. (1982) 716:101–9. doi: 10.1016/0304-4165(82)90207-0
96. Chang MY, Seideman J, Sofou S. Enhanced loading efficiency and retention of 225Ac in rigid liposomes for potential targeted therapy of micrometastases. Bioconj Chem. (2008) 19:1274–82. doi: 10.1021/bc700440a
97. Semple SC, Klimuk SK, Harasym TO, Dos Santos N, Ansell SM, Wong KF, et al. Efficient encapsulation of antisense oligonucleotides in lipid vesicles using ionizable aminolipids: formation of novel small multilamellar vesicle structures. Biochim Biophys Acta. (2001) 1510:152–66. doi: 10.1016/S0005-2736(00)00343-6
98. Castro GA, Coelho AL, Oliveira CA, Mahecha GA, Orefice RL, Ferreira LA. Formation of ion pairing as an alternative to improve encapsulation and stability and to reduce skin irritation of retinoic acid loaded in solid lipid nanoparticles. Int J Pharm. (2009) 381:77–83. doi: 10.1016/j.ijpharm.2009.07.025
99. Takeuchi I, Takeshita T, Suzuki T, Makino K. Iontophoretic transdermal delivery using chitosan-coated PLGA nanoparticles for positively charged drugs. Coll Surf B Bioint. (2017) 160:520–6. doi: 10.1016/j.colsurfb.2017.10.011
100. Cheng WP, Gray AI, Tetley L, Hang Tle B, Schatzlein AG, Uchegbu IF. Polyelectrolyte nanoparticles with high drug loading enhance the oral uptake of hydrophobic compounds. Biomacromolecules. (2006) 7:1509–20. doi: 10.1021/bm060130l
101. Hu X, Jing X. Biodegradable amphiphilic polymer-drug conjugate micelles. Expert Opin Drug Deliv. (2009) 6:1079–90. doi: 10.1517/17425240903158917
102. Bhadra D, Bhadra S, Jain S, Jain NK. A PEGylated dendritic nanoparticulate carrier of fluorouracil. Int J Pharm. (2003) 257:111–24. doi: 10.1016/S0378-5173(03)00132-7
103. Cerqueira P, Noro J, Moura S, Guimaraes D, Silva C, Cavaco-Paulo A, et al. PTS micelles for the delivery of hydrophobic methotrexate. Int J Pharm. (2019) 566:282–90. doi: 10.1016/j.ijpharm.2019.05.049
104. Maharjan P, Jin M, Kim D, Yang J, Maharjan A, Shin MC, et al. Evaluation of epithelial transport and oxidative stress protection of nanoengineered curcumin derivative-cyclodextrin formulation for ocular delivery. Arch Pharm Res. (2019). doi: 10.1007/s12272-019-01154-9. [Epub ahead of print].
105. Liu C, Zhang S, McClements DJ. Design of astaxanthin-loaded core-shell nanoparticles consisting of chitosan oligosaccharides and poly(lactic- co-glycolic acid): enhancement of water solubility, stability, and bioavailability. J Agricult Food Chem. (2019) 67:5113–21. doi: 10.1021/acs.jafc.8b06963
106. Varga N, Turcsanyi A, Hornok V. Vitamin E-loaded PLA- and PLGA-based core-shell nanoparticles: synthesis, structure optimization and controlled drug release. Pharmaceutics. (2019) 11:E357. doi: 10.3390/pharmaceutics11070357
107. Yang G, Liu Y, Wang H, Wilson R, Hui Y, Yu A, et al. Bioinspired core-shell nanoparticles for hydrophobic drug delivery. Angew Chem. (2019). doi: 10.1002/anie.201908357. [Epub ahead of print].
108. Yang S, Gao H. Nanoparticles for modulating tumor microenvironment to improve drug delivery and tumor therapy. Pharmacol Res. (2017) 126:97–108. doi: 10.1016/j.phrs.2017.05.004
109. Fukumura D, Jain RK. Tumor microenvironment abnormalities: causes, consequences, and strategies to normalize. J Cell Biochem. (2007) 101:937–49. doi: 10.1002/jcb.21187
110. Kost J, Langer R. Responsive polymeric delivery systems. Adv Drug Deliv Rev. (2001) 46:125–48. doi: 10.1016/S0169-409X(00)00136-8
111. Kim JO, Kabanov AV, Bronich TK. Polymer micelles with cross-linked polyanion core for delivery of a cationic drug doxorubicin. J Control Release. (2009) 138:197–204. doi: 10.1016/j.jconrel.2009.04.019
112. Lo CL, Huang CK, Lin KM, Hsiue GH. Mixed micelles formed from graft and diblock copolymers for application in intracellular drug delivery. Biomaterials. (2007) 28:1225–35. doi: 10.1016/j.biomaterials.2006.09.050
113. Borchert U, Lipprandt U, Bilang M, Kimpfler A, Rank A, Peschka-Suss R, et al. pH-induced release from P2VP-PEO block copolymer vesicles. Langmuir. (2006) 22:5843–7. doi: 10.1021/la060227t
114. Khawar IA, Kim JH, Kuh HJ. Improving drug delivery to solid tumors: priming the tumor microenvironment. J Control Release. (2015) 201:78–89. doi: 10.1016/j.jconrel.2014.12.018
115. Roberts WG, Palade GE. Neovasculature induced by vascular endothelial growth factor is fenestrated. Cancer Res. (1997) 57:765–72.
116. Dvorak AM, Kohn S, Morgan ES, Fox P, Nagy JA, Dvorak HF. The vesiculo-vacuolar organelle (VVO): a distinct endothelial cell structure that provides a transcellular pathway for macromolecular extravasation. J Leukocyte Biol. (1996) 59:100–15. doi: 10.1002/jlb.59.1.100
117. Monsky WL, Fukumura D, Gohongi T, Ancukiewcz M, Weich HA, Torchilin VP, et al. Augmentation of transvascular transport of macromolecules and nanoparticles in tumors using vascular endothelial growth factor. Cancer Res. (1999) 59:4129–35.
118. Kalyane D, Raval N, Maheshwari R, Tambe V, Kalia K, Tekade RK. Employment of enhanced permeability and retention effect (EPR): nanoparticle-based precision tools for targeting of therapeutic and diagnostic agent in cancer. Mater Sci Eng. (2019) 98:1252–76. doi: 10.1016/j.msec.2019.01.066
119. Miao L, Lin CM, Huang L. Stromal barriers and strategies for the delivery of nanomedicine to desmoplastic tumors. J Controll Release. (2015) 219:192–204. doi: 10.1016/j.jconrel.2015.08.017
120. Mahmoudi M. Debugging nano-bio interfaces: systematic strategies to accelerate clinical translation of nanotechnologies. Trends Biotechnol. (2018) 36:755–69. doi: 10.1016/j.tibtech.2018.02.014
121. Serkova NJ. Nanoparticle-based magnetic resonance imaging on tumor-associated macrophages and inflammation. Front Immunol. (2017) 8:590. doi: 10.3389/fimmu.2017.00590
122. Daldrup-Link H, Coussens LM. MR imaging of tumor-associated macrophages. Oncoimmunology. (2012) 1:507–9. doi: 10.4161/onci.19456
123. Harisinghani MG, Barentsz J, Hahn PF, Deserno WM, Tabatabaei S, van de Kaa CH, et al. Noninvasive detection of clinically occult lymph-node metastases in prostate cancer. N Engl J Med. (2003) 348:2491–9. doi: 10.1056/NEJMoa022749
124. Heesakkers RA, Jager GJ, Hovels AM, de Hoop B, van den Bosch HC, Raat F, et al. Prostate cancer: detection of lymph node metastases outside the routine surgical area with ferumoxtran-10-enhanced MR imaging. Radiology. (2009) 251:408–14. doi: 10.1148/radiol.2512071018
125. Gordon S, Martinez FO. Alternative activation of macrophages: mechanism and functions. Immunity. (2010) 32:593–604. doi: 10.1016/j.immuni.2010.05.007
126. Sica A, Mantovani A. Macrophage plasticity and polarization: in vivo veritas. J Clin Invest. (2012) 122:787–95. doi: 10.1172/JCI59643
127. Martinez FO, Helming L, Gordon S. Alternative activation of macrophages: an immunologic functional perspective. Ann Rev Immunol. (2009) 27:451–83. doi: 10.1146/annurev.immunol.021908.132532
128. Ries CH, Cannarile MA, Hoves S, Benz J, Wartha K, Runza V, et al. Targeting tumor-associated macrophages with anti-CSF-1R antibody reveals a strategy for cancer therapy. Cancer Cell. (2014) 25:846–59. doi: 10.1016/j.ccr.2014.05.016
Keywords: macrophages, nanoparticles, drug delivery, inflammation, tumor
Citation: Hu G, Guo M, Xu J, Wu F, Fan J, Huang Q, Yang G, Lv Z, Wang X and Jin Y (2019) Nanoparticles Targeting Macrophages as Potential Clinical Therapeutic Agents Against Cancer and Inflammation. Front. Immunol. 10:1998. doi: 10.3389/fimmu.2019.01998
Received: 30 May 2019; Accepted: 07 August 2019;
Published: 21 August 2019.
Edited by:
Mustafa Diken, Translationale Onkologie an der Universitätsmedizin der Johannes Gutenberg-Universität Mainz, GermanyReviewed by:
Dan Peer, Tel Aviv University, IsraelLutz Nuhn, Max-Planck-Gesellschaft (MPG), Germany
Copyright © 2019 Hu, Guo, Xu, Wu, Fan, Huang, Yang, Lv, Wang and Jin. This is an open-access article distributed under the terms of the Creative Commons Attribution License (CC BY). The use, distribution or reproduction in other forums is permitted, provided the original author(s) and the copyright owner(s) are credited and that the original publication in this journal is cited, in accordance with accepted academic practice. No use, distribution or reproduction is permitted which does not comply with these terms.
*Correspondence: Yang Jin, d2h1aGp5QDEyNi5jb20=
†These authors have contributed equally to this work