- Department of Pediatric Surgery, University Medical Center Hamburg-Eppendorf (UKE), Hamburg, Germany
Background: Neutrophil extracellular traps (NETs) are a defense mechanism in which neutrophils cast a net-like structure in response to microbial infection. NETs consist of decondensed chromatin and about 30 enzymes and peptides. Some components, such as neutrophil elastase (NE) and myeloperoxidase (MPO), present antimicrobial but also cytotoxic properties, leading to tissue injury. Many inflammatory diseases are associated with NETs, and their final role has not been identified. Pulmonary surfactant is known to have immunoregulatory abilities that alter the function of adaptive and innate immune cells. The aim of this study was to investigate the hypothesis that natural surfactant preparations inhibit the formation of NETs.
Methods: The effect of two natural surfactants (Alveofact® and Curosurf®) on spontaneous and phorbol-12-myristate-13-acetate–induced NET formation by neutrophils isolated by magnetic cell sorting from healthy individuals was examined. NETs were quantitatively detected by absorption and fluorometric-based assays for the NET-specific proteins (NE, MPO) and cell-free DNA. Immunofluorescence microscopy images were used for visualization.
Results: Both surfactant preparations exerted a dose-dependent inhibitory effect on NET formation. Samples treated with higher concentrations and with 30 min pre-incubation prior to stimulation with phorbol-12-myristate-13-acetate had significantly lower levels of NET-specific proteins and cell-free DNA compared to untreated samples. Immunofluorescence microscopy confirmed these findings.
Conclusions: The described dose-dependent modulation of NET formation ex vivo suggests an interaction between exogenous surfactant supplementation and neutrophil granulocytes. The immunoregulatory effects of surfactant preparations should be considered for further examination of inflammatory diseases.
Introduction
Neutrophil granulocytes play a major role in defending the lung against invading pathogens (1). As part of the innate immune system, they reach the site of infection as one of the first immune cells to attack microorganisms by using defense mechanisms such as phagocytosis and degranulation (2). A particular form of host defense performed by these cells is neutrophil extracellular traps (NETs). To form NETs, neutrophils undergo a specific form of programmed cell death, termed NETosis, which leads to the release of web-like decondensed DNA and antimicrobial granular proteins into the extracellular space (3). Besides the beneficial effect of entrapping infectious agents, an inordinate amount of NETs leads to tissue injury due to their cytotoxic components such as histones, neutrophil elastase (NE), myeloperoxidase (MPO), and other neutrophil proteases, thus contributing to many inflammatory lung diseases (4–6). NETosis may be triggered by a variety of stimuli such as bacteria, viruses, fungi, and protozoa, among others. In vitro NETosis can be elicited through treatment with phorbol 12-myristate 13-acetate (PMA) or interleukin (IL)-8 (1, 7–10). Due to the involvement of NETs in many inflammatory and chronic lung diseases, they might serve as therapeutic targets, however this has not been explored in detail so far (4, 11, 12).
Pulmonary surfactant is a composition of mostly phospholipids (80%), cholesterol (10%), and the four surfactant proteins (SP): SP-A, SP-B, SP-C, and SP-D (10%). It is secreted by alveolar type II cells, lining the alveolar epithelium and thereby reducing the surface tension at the air–liquid interface. This function is important for ensuring the compliance of the lung, supporting gas exchange, and preventing alveolar collapse during exhalation (13). Consequently, a lack of surfactant leads to respiratory failure in premature infants, causing respiratory distress syndrome, which requires mechanical ventilation and exogenous surfactant replacement (14). In Germany, there are two typical clinically used animal-derived (natural) surfactants. Bovine-derived Alveofact® and porcine-derived Curosurf®. Both consist of multiple components and differ significantly in composition. While Alveofact® holds a larger portion of both SP-B and SP-C, Curosurf® contains more Dipalmitoylphosphatidylcholine, a major phospholipid in surfactant (15). Recommended dosage of Alveofact® with 50–100 mg/kg differs from recommendations for Curosurf® with 100–200 mg/kg. However, the cumulative dosage of both surfactants may differ from the recommendations due to clinically necessary repeated applications (16, 17). In terms of in vivo effectiveness, animal-derived (natural) surfactants containing SP-B and SP-C have proven to be superior to synthetic animal protein-free surfactants (18). Apart from the commonly known biophysical functions, the role of pulmonary surfactant in the innate immune system has been elucidated in the last two decades (19). The immunoregulatory properties are primarily exerted by the surfactant proteins, especially SP-A and SP-D. These hydrophilic proteins belong to the collectin family and are able to interact with a variety of pathogens and other targets (neutrophils, surfactant phospholipids, and DNA) via a carbohydrate recognition domain (CRD; lectin domain) and further collectin receptors (20). They are not only able to opsonize pathogens via their CRDs and thus enhance phagocytosis by immune cells, but can also modulate and even inhibit the functions of these cells through anti-inflammatory signals (21). For example, SP-A and SP-D deficiency renders mice incapable of terminating neutrophilic inflammation (22, 23). Moreover, SP-D can diminish lipopolysaccharide (LPS)-induced NET formation (24). The hydrophobic surfactant proteins SP-B and SP-C play a crucial role in reducing surface tension (13, 25). However, more recent studies find that they and other surfactant phospholipids also contribute to immunomodulation (26–29).
In the present study, the effect of two common clinically used surfactants (Alveofact®, Curosurf®) on spontaneous and PMA-induced NET formation was investigated. Alveofact® and Curosurf® contain exclusively SP-B and SP-C, along with phospholipids, but no SP-A or SP-D that have been proven to be effective against neutrophilic inflammation and NET formation (23, 24, 30). We were able to assess the immunoregulatory effects of these natural surfactants on NET formation in neutrophil granulocytes.
Materials and Methods
Surfactant Preparations and Chemokines
Two natural surfactant preparations common in clinical use were examined: Curosurf® (Chiesi, Hamburg, Germany) is prepared from minced porcine lungs and supplied as a ready-to-use intratracheal suspension. Alveofact® (Lyomark Pharma, Oberhaching, Germany) is a surfactant isolated from bovine lung lavage and prepared as a lyophilized powder that has to be reconstituted with the provided solvent prior to use. The surfactants were used and stored according to the manufacturers’ recommendations. To obtain approximately similar concentrations in vitro we covered a range of concentrations from 0.001–2.5 mg/ml.
Moreover, phorbol-12-myristate-13-acetate (PMA), a common chemical stimulator for NETosis (10), from Cayman Chemical (USA) was used.
Isolation of Neutrophils
Blood was obtained with informed, signed consent from healthy local donors following approval by the Ethics Committee of the Hamburg Medical Association (PV5921). The blood was collected in EDTA-K monovettes (Sarstedt, Nümbrecht, Germany). Neutrophil granulocytes were isolated using the MACSxpress® Whole Blood Neutrophil Isolation Kit according to the manufacturer’s protocol (Miltenyi Biotec, Bergisch Gladbach, Germany). Briefly: 8ml of whole blood was mixed with 4ml MACSxpress® Isolation Mix, incubated for 5 min at room temperature (RT) in the MACSmix™ Tube Rotator at 12 rpm, and magnetically separated with the MACSxpress® Separator for 15 min. After centrifugation of the target cell fraction at 300 × g for 10 min at 4°C, residual erythrocytes were lysed at 4°C by resuspending the cell pellet in 2 ml of H2O for 30 s prior to adding 0.7 ml of 0.6 M KCl (Sigma-Aldrich, Saint Louis, MO, USA). Neutrophils were washed with phosphate-buffered saline and resuspended in RPMI 1640 (Thermo Fischer, Waltham, MA, USA) containing 1% bovine serum albumin (Sigma-Aldrich, Saint Louis, MO, USA).
Purity of the extracted neutrophils (>95%) was assured via fluorescence-activated cell sorting (FACS) using anti-CD15-FITC (mAb HI98, IgM) and anti-CD16-PerCP (mAb 3G8, IgG1) antibodies (BioLegend, San Diego, CA, USA). Cell morphology was analyzed by hematoxylin and eosin staining.
Cell Treatments
Neutrophils were resuspended in Gibco® RPMI 1640 media containing 1% bovine serum albumin (BSA) for the MPO assay and in Gibco ® RPMI 1640 media containing 1% BSA and 0.1% calcium chloride for the NE and cell-free DNA (cfDNA) assay. For the MPO assay, the cells were seeded into a 96-well cell culture plate at a density of 2×105 cells per well. Neutrophils undergoing NE and cfDNA assay were seeded in a 24-well cell culture plate at a concentration of 1×106 cells/well. After 2-h incubation at 37°C, the cell culture plates were treated with Curosurf® (0.001, 0.1, 1 mg/ml) or Alveofact® (0.025, 0.25, 2.5 mg/ml) at different PMA incubation time points to investigate a possible preventive and/or therapeutic effect on NETosis. The cells were treated 30 min prior, simultaneously, and 10 min after stimulation with 100 nM PMA per well. After 3-h incubation at 37°C, NETosis assays were performed.
Assays for Measurement of Neutrophil Extracellular Trap Formation
To quantify the amount of NETs, three different assays that measured NET-specific proteins and cfDNA were performed. The neutrophil MPO activity assay kit (MPO) and NETosis assay kit for measuring NET-derived MPO and NE, respectively from Cayman Chemicals were used according to the manufacturer’s instructions. The cfDNA assay was performed following the protocol of Fuchs et al. (31). To measure the effect of timing and dosage, we investigated three treatment time points (30 min before, simultaneously, and 10 min after activation with PMA) and three concentrations of Curosurf® (1 µg/ml, 100 µg/ml, 1 mg/ml) and Alveofact® (25 µg/ml, 250 µg/ml, 2.5 mg/ml). Untreated and unactivated cells were used as the negative control; untreated but activated cells served as the positive control.
For the MPO assay, cells were seeded and treated in a 96-well plate. Then, the plate was centrifuged at 1200 rpm for 10 min at RT, followed by transfer of the supernatants into a new plate. After adding 50 µl 3,3′,5,5′-tetramethylbenzidine (TMB), the absorbance of the supernatants was measured at 375 nm. The measured absorbance was proportional to the amount of MPO in the sample. Using the NETosis assay kit, only NE and DNA bound to the NETs were measured as the supernatant was washed out twice after treatment, leaving only adhered NETs on the bottom of the well. S7 nuclease was added to cleave the NETs and release NE and DNA into the supernatant, which could be used for both the NE assay and cfDNA assay.
For the NE assay, the supernatant was transferred into a 96-well plate and incubated with 100 µl 500 nM N-methoxysuccinyl-Ala-Ala-Pro-Val p-nitroanilide for 2 h at 37°C before the absorbance was read at 405 nm.
Cell-free DNA was detected by mixing 50 µl 1:2 diluted supernatant with 50 µl SYTOX Orange or 50 µl dilution buffer (0.1% BSA and 2 mM EDTA in DPBS). Fluorescence measurement was performed immediately afterwards at excitation, 544 nm; emission, 590 nm; cut off, 570 nm.
Enzyme-Linked Immunosorbent Assay
The concentration of cytosolic PAD4 in neutrophils was measured using the Human PADI4 ELISA kit (MyBioSource, San Diego, USA) according to the manufacturer’s instructions. Protein extraction was carried out using RIPA Buffer with cOmplete™ Mini Protease Inhibitor Cocktail (Merck KGaA, Darmstadt, Germany). The protein levels were estimated from a generated standard curve. The optical densities were examined at a 450-nm wavelength using a microplate reader (Molecular Devices, Sunnyvale, USA).
Immunostaining and Imaging
Neutrophils (2×105/well) were seeded into 12-well plates containing coverslips and incubated for 2 h at 37°C. Medium was removed and replaced with or without surfactant solution 30 min before, simultaneously, and 10 min after stimulation with 100 nM PMA per well. The surfactant concentrations were analogous to that of the other assays (Curosurf®, 0.001, 0.1, and 1 mg/ml; Alveofact®, 0.025, 0.25, 2.5 mg/ml). After 3-h incubation at 37°C, the cells were washed twice with DPBS and fixed overnight at -20°C with 99% methanol. After fixation, the cells were immunostained. Cells were washed twice with DPBS, blocked with 1% BSA–DPBS for 1 h at RT, and incubated with either primary specific or isocontrol antibody solution for 1 h at RT. The following antibodies were used: 1:200 anti-NE antibody (Abcam, UK), 1:100 anti-MPO antibody (Abcam) and anti–citrullinated histone H3 R2+R8+R17 (H3cit) antibody (Abcam). Subsequently, the cells were incubated with secondary antibodies for 1 h at RT after washing twice with DPBS. NE and H3cit detection were assessed with an anti-rabbit secondary antibody conjugated with Alexa Fluor 647 (1:200, Abcam), MPO was detected with FITC-conjugated anti-mouse secondary antibody (1:1000, Abcam). After a three-wash step, the cells were counterstained with 1:1000 4′,6-diamino-2-phenylindole (DAPI) and mounted with Fluoromount-G (SouthernBiotech, USA). Microscopy images were taken using Apotome.2 by Zeiss (Carl Zeiss AG, Oberkochen, Germany) at ×40 magnification and processed using ImageJ software (Version 1.51, NIH, USA).
Flow Cytometry
A total of 1x106 neutrophils were treated with indicated doses at different timepoints, supernatant was collected and adherent cells were detached by incubation with Accutase (Capricorn Scientific, Ebsdorfergrund, Germany) for 30–60 min. Cells were washed twice with PBS (Thermo Fischer, Waltham, MA, USA) and labeled with propidium iodide (PI) and Annexin-V-FITC (Becton, Dickinson and Company, Franklin Lakes, NJ, USA) or anti-CD11b-VioBlue (mAb REA713, IgG1) and anti-CD66b-APC (mAb REA306, IgG1) antibodies (Miltenyi Biotec, Bergisch Gladbach, Germany) or anti-P2Y6 Receptor-FITC (Alomone Labs, Jerusalem, Israel) as described in the manufacturer’s protocols. Analysis was performed with a flow cytometer (FACSCanto™ II, Becton, Dickinson, and Company, Franklin Lakes, NJ, USA). Data were analyzed using BD FACSDiva™ (Becton, Dickinson and Company, Franklin Lakes, NJ, USA).
Determination of Intracellular Levels of Reactive Oxygen Species
The intracellular ROS levels were determined as described previously by Balaiya et al. using the oxidation-sensitive fluorescent DHR-123 (ThermoFisher Scientific, Massachusetts, USA) (32, 33). Cells were seeded at a density of 0,5x105 into black/flat clear bottom 96-well plates (Corning Incorporated, New York, NY, USA), treated as described and loaded with 5 μM DHR-123. Cells were then incubated for 30 min in the dark at culture condition and 30 min on the plate shaker at 35 rpm at RT. Fluorescence was measured at excitation/emission =505/534 nm using a microplate reader (Flex Station® 3, Molecular Devices, San Jose, CA, USA).
Intracellular Calcium Mobilization
To detect intracellular calcium mobilization, the Fluo-8 No Wash Calcium Assay kit was used, following manufacturer’s protocol (ab112129, Abcam, Cambridge, Great Britain). Briefly, 1x105 cells per well were seeded in black/flat clear bottom 96-well plates (Corning Incorporated, New York, NY, USA) and settled at 37°C with 5% CO2. Cells were treated as described before. Fluo-8 stock solution was added together with PMA stimulation. Cells were incubated for 30 min at culture condition (37°C, 5% CO2) and afterwards 30 min at room temperature. Fluorescence was measured at excitation/emission = 490/525nm continuously every 15 min for 2 h using a microplate reader (Flex Station® 3). Peak results for Calcium influx at 2 h after stimulation are displayed as normalized data on PMA-stimulated controls.
Statistical Analysis
Each assay was performed in three biological replicates (n = 3). All data were analyzed with SPSS Statistics 26 (IBM, Ehningen, Germany) and GraphPad Prism 8 (San Diego, USA). Differences between groups were calculated using ANOVA with Dunnett’s correction for repeated measurements. Data are presented as mean ± SD of values from three independent experiments. Significance level was set as p<0.05 (*<0.05, **<0.005, ***<0.0005, ****<0.0001).
Results
Curosurf® and Alveofact® Generate No Pro-Inflammatory Effect
Neither Curosurf® nor Alveofact® cause activation in neutrophils as indicated by staining with CD11b and CD66b antibodies (Figure 1D). Furthermore, they do not induce apoptosis or necrosis in neutrophils (Figures 1A, B) with a consistent ability of neutrophils to generate reactive oxygen species (Figure 1C). Also, we could not find a significant release of NETs in the NE, MPO or cfDNA assay either. (data not shown). This was tested by incubating the cells with the same dosages of Curosurf® and Alveofact® in the absence of PMA as a stimulator for NETosis. Compared to spontaneous NET formation, higher levels of MPO, NE, or cfDNA were not detectable.
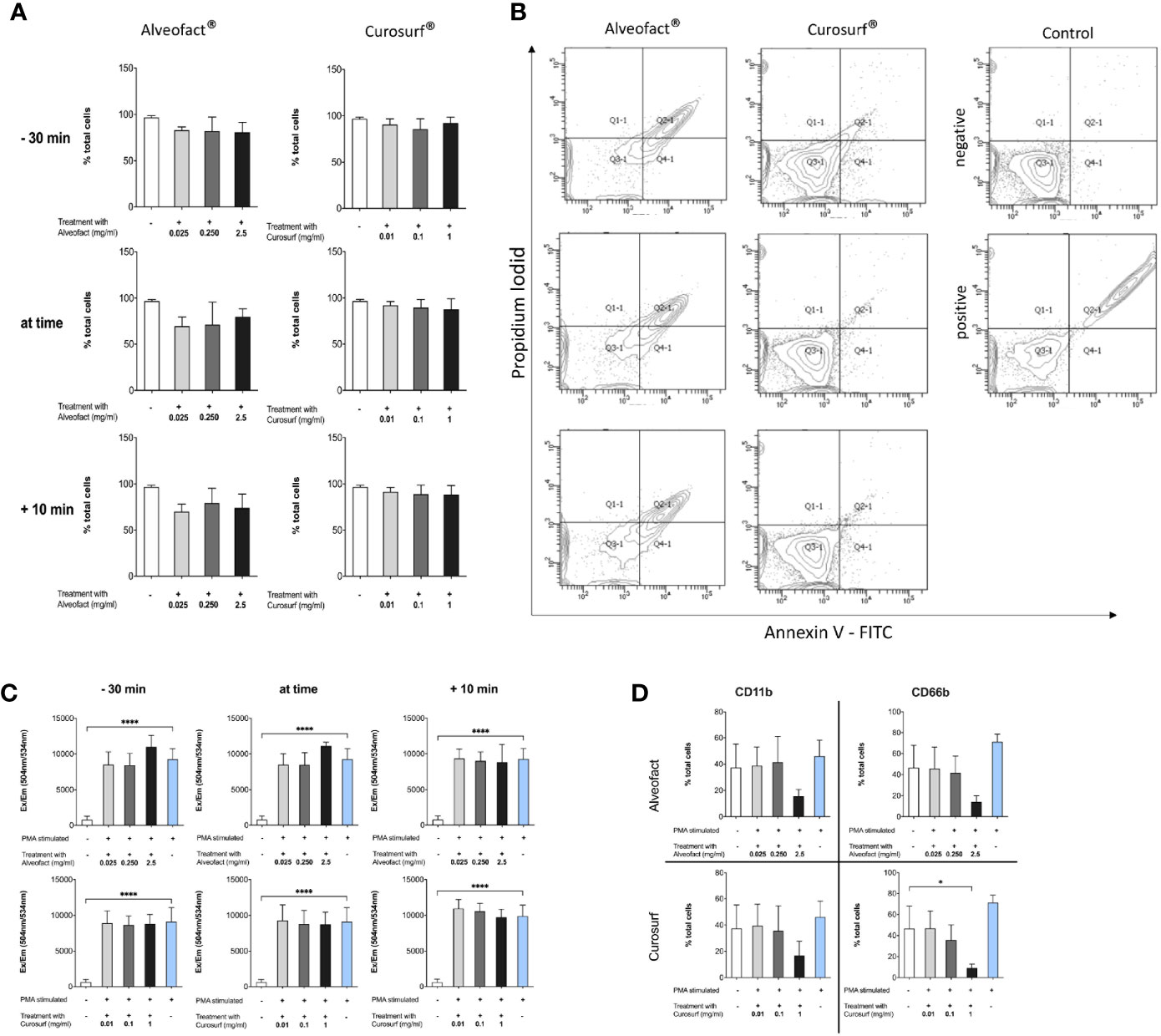
Figure 1 Neutrophils are not altered by animal derived surfactants. (A, B) Treatment with surfactant does not change survival of neutrophils. (A) Cells were treated and stained with PI and Annexin-V. Double negative cells are counted as living cells and displayed as mean+-SD of three independent experiments with no significant changes between untreated and unstimulated cells versus cells treated with surfactant at different timepoints and different doses. (B) Representative blot of 30 min pretreatment with surfactant. (C) Production of reactive oxygen species measured with Dihydrorhodamin-123. Treatment with surfactant does not alter the ability of neutrophils to produce ROS. (D) Neutrophils show no change in activation compared to untreated control. Figure shows 30 min pretreatment and is representative for all other timepoints. Only maximum dose of Curosurf® shows a decrease of the activation marker CD66b. Significance level was set as p<0.05 (*<0.05, **<0.005, ***<0.0005, ****<0.0001).
Curosurf®
The highest concentration of Curosurf® (1 mg/ml) paired with the longest incubation time, i.e., 30 min prior to activation with PMA, significantly reduced NE (p = 0.0076), MPO (p = 0.0027) and cfDNA (p = 0.0026) (Figures 2A, D, G). In the simultaneous activation/treatment and 10-min treatment after activation groups, samples treated with 1 mg/ml of Curosurf® had significantly decreased MPO (Figures 2E, F) and cfDNA (Figures 2H, I), whereas NE (Figures 2B, C) exhibited no significant changes.
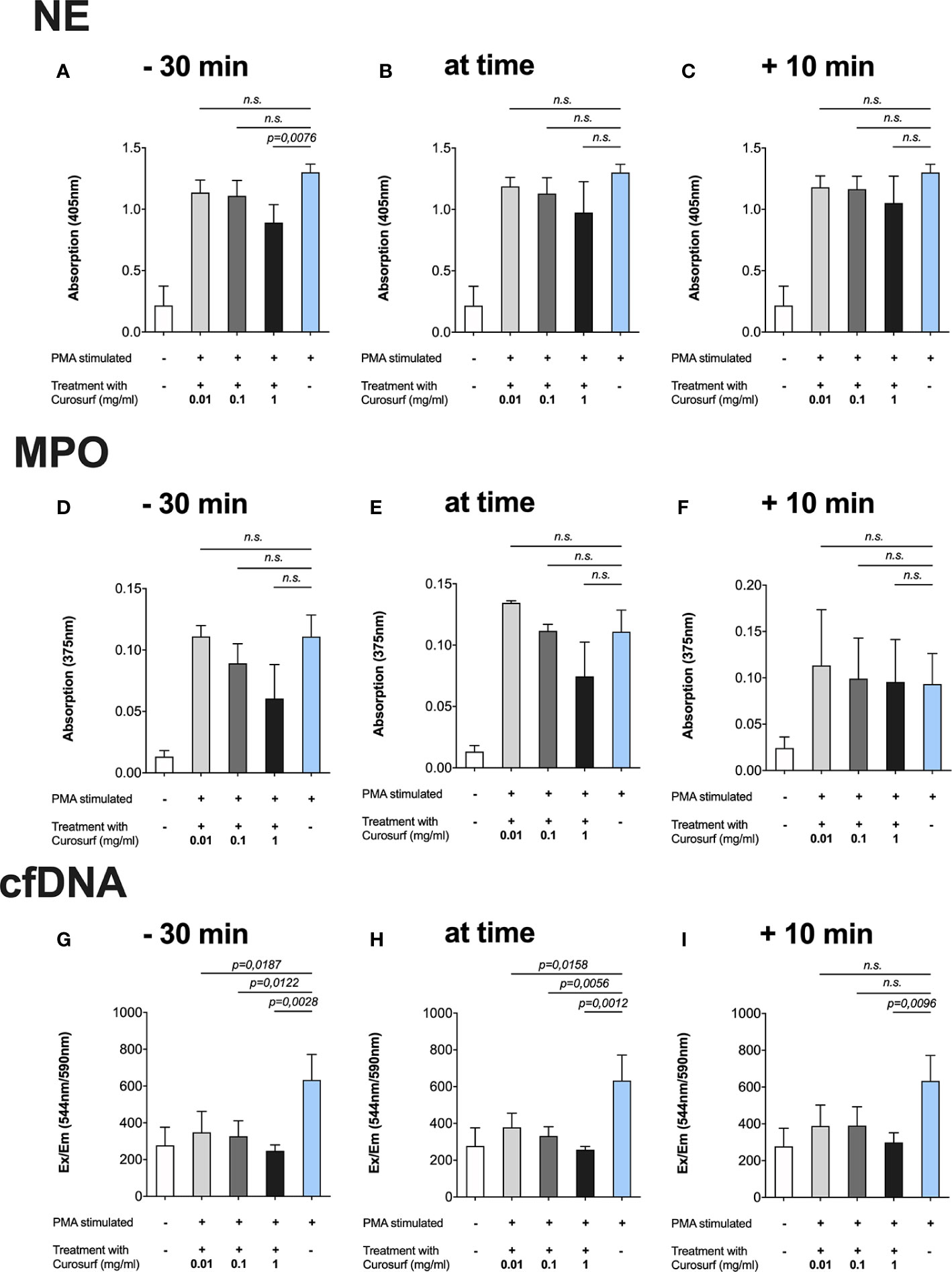
Figure 2 Curosurf® reduces PMA-induced formation of NETs. Neutrophils were incubated with Curosurf® at three different time points in relation to activation with 100 nm PMA, and then afterwards the NET-specific components were measured in three different assays. Cells that were untreated but stimulated with 100 nm PMA served as positive control in each assay. (A–C) There was a trend toward a dose- and time-dependent decrease in NE, but only the highest dose of 1 mg/ml paired with the longest incubation time of 30 min showed a significant result. (D–F) Proportionate intensity of the blue color emitted by TMB as a chromogenic substrate represented the amount of MPO and showed a significant decrease of NETs for the highest dose at each time point. (G–I) SYTOX Orange assessment of the amount of cfDNA. Significantly less cfDNA was observed in almost every variant of time and dose.
Similarly, only cfDNA was significantly decreased after treatment with lower concentrations of Curosurf® (1 µg/ml, 100 µg/ml) and treatment simultaneously or 30 min prior to activation (Figures 2G, H). Although no statistical significance was observed for NE and MPO following treatment with lower Curosurf® concentrations, a tendency of time- and concentration-dependence was observed, especially in the MPO assay (Figures 2D–F).
Alveofact®
Even more evident results were obtained after treating the cells with Alveofact® at the same time points described above. The 30 min incubation with the highest concentration (2.5 mg/ml) prior to activation with PMA generated the lowest measurements of NE (Figure 3A), MPO (Figure 3D), and cfDNA (Figure 3G). In simultaneous treatment with activation and treatment 10 min after activation, the highest concentration (2.5 mg/ml) also resulted in a significant reduction of NE (Figures 3B, C), MPO (Figures 3E, F), and cfDNA (Figures 3H, I). Unlike Curosurf®, the intermediate dose of 250 µg/ml Alveofact® significantly reduced the amount of NE (Figures 3A–C) and cfDNA (Figures 3G, H), but not MPO (Figures 3E, F), at all timepoints, indicating a stronger effect of Alveofact®. The lowest concentration of 25 µg/ml generally had no significant effect on any of the measured factors, with one exception in the cfDNA assay for simultaneous treatment and activation (Figure 3H).
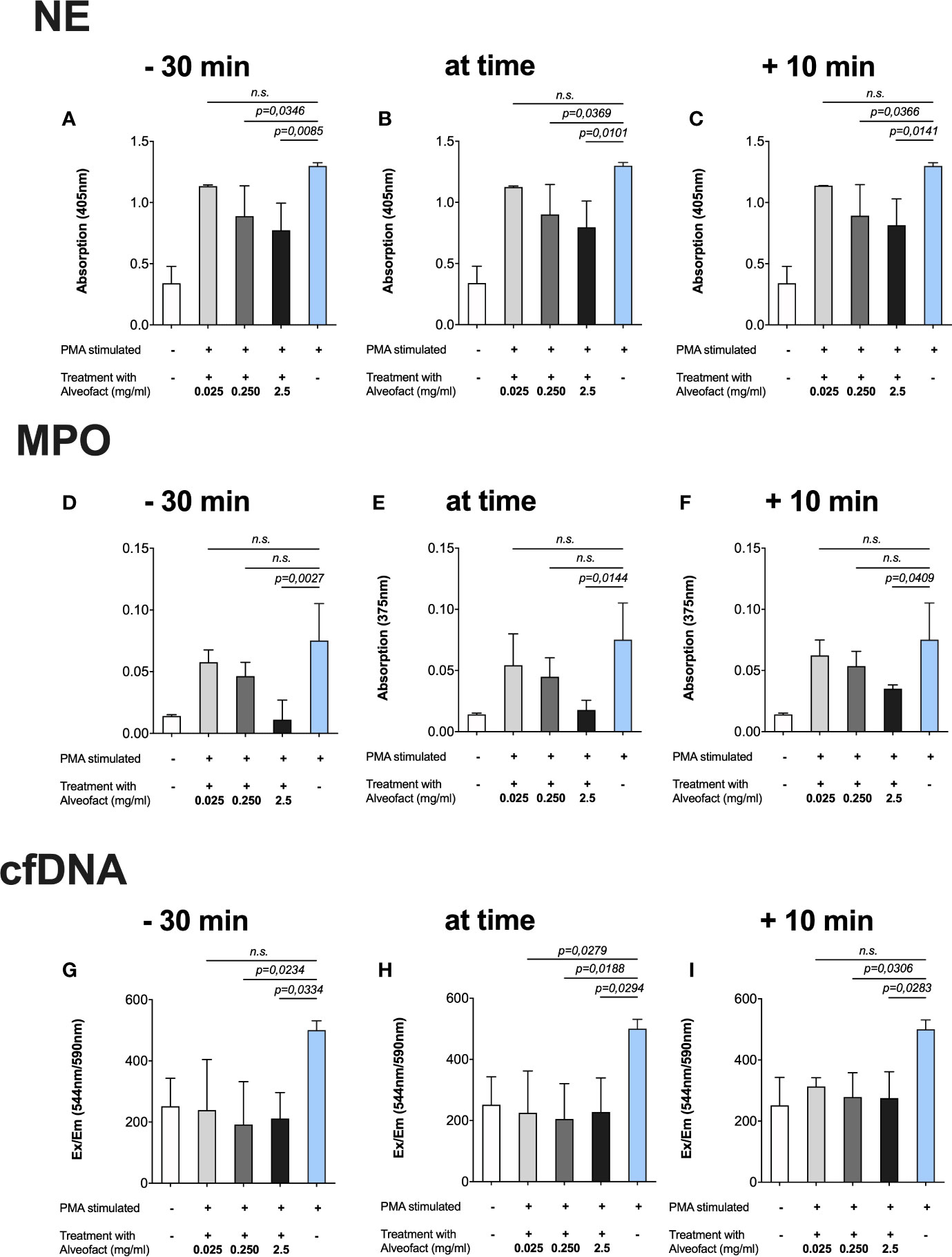
Figure 3 Alveofact® reduces extracellular NE, MPO, and cfDNA. Neutrophils were incubated with Alveofact® at three different time points in relation to activation with 100 nm PMA, and then afterwards the NET-specific components were measured in three different assays. Cells that were untreated but stimulated with 100 nm PMA served as positive control in each assay. (A–C) Concentrations of 250 µg/ml and 2.5 mg/ml could reduce the amounts of NE significantly at all time points. (D–F) The amounts of MPO were approximately as low as that in the untreated control when 2.5 mg/ml Alveofact® was applied at 30 min prior to simultaneous activation with PMA. (G–I) The levels of cfDNA were about as high as that in the untreated and unstimulated control, regardless of timing and concentration of Alveofact®.
Immunostained Microscopy Images Confirm the Inhibition of NETosis by Curosurf® and Alveofact®
We used immunofluorescence staining to visualize and verify the suppression of NETosis by Curosurf® or Alveofact®. To detect NETs, neutrophils were treated with either Curosurf® or Alveofact® and stimulated with PMA. Untreated and unstimulated neutrophils were used as the negative control, showing a DNA signal (blue) in the nucleus (Figures 4B, D). On the contrary, neutrophils incubated with PMA alone showed the common net-like DNA structure concomitant to NE (red), MPO (green), and H3cit (red) (Figures 4B, D). Concordantly with the results reported above, immunofluorescence staining showed decreased NE and MPO (Figure 4C) and H3cit (Figure 4A) signals with treatment involving the highest concentration of Curosurf® (1 mg/ml) and Alveofact® (2.5 mg/ml) 30 min prior to PMA incubation. Even under simultaneous activation with PMA, the highest dose nevertheless achieved a definite reduction of the H3cit (Figure 4A), NE, and MPO signals (data not shown). In contrast, the lower concentrations of both surfactant solutions exerted no relevant inhibitory effect on the neutrophils, as exemplified for NE and MPO (Figure 4C), and seemed to be time-independent. Thus, the microscopy images confirmed the assay findings.
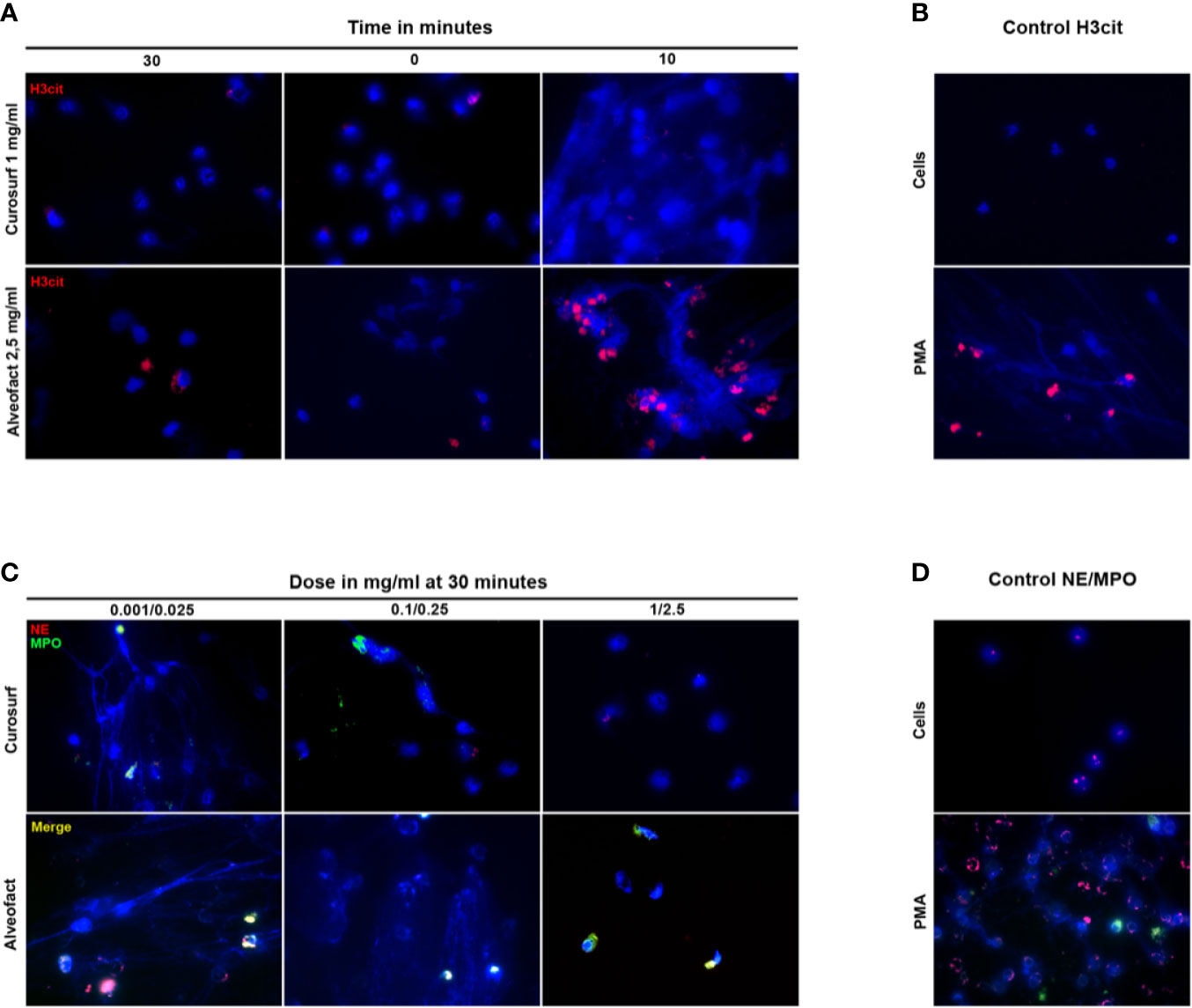
Figure 4 Immunostaining confirms the time- and dose-dependent suppression of NETosis. (A) Neutrophils were activated with PMA 30 min before, simultaneously, or 10 min after treatment with the highest dose of Curosurf® (1 mg/ml) or Alveofact® (2.5 mg/ml), and immunostained for H3cit (red) and for DNA with DAPI (blue). Treatment 10 min after stimulation showed no suppression of NETosis and a comparable picture to the positive control (B), while the 0- and 30-min time points showed suppressed NETosis. (B) Negative control with untreated and unstimulated cells had no sign of NETosis. PMA-stimulated cells as positive control showed H3cit formation. (C) Neutrophils were treated with three different concentrations of Curosurf® (0.001, 0.1, and 1 mg/ml) and Alveofact® (0.025, 0.25 and 2.5 mg/ml) 30 min before activation with PMA and were immunostained for NE (red) and MPO (green), and stained for DNA with DAPI (blue). The suppression of NETosis was strongest in samples with the highest dose of surfactant and was barely or not detectable at the lower doses. (D) Negative controls for NE/MPO showed intact untreated and unstimulated cells with only slight NE signal; positive controls with PMA-stimulated cells showed massive NET formation with explicit NE and MPO signals co-localized to NET DNA (×40 magnification).
Downregulation of the P2Y6 Receptor Expression and Decreased Calcium Mobilization Without Change of Intracellular PAD4 Levels
P2Y6 receptor was investigated by Flow Cytometry using a P2Y6-FITC antibody. Neutrophils were used as control and showed the same expression patterns of the receptor as PMA stimulated neutrophils. Surfactant-treated cells showed a significantly decreased expression of the extracellular receptor (Figure 5A). Accordingly, to the findings of decreased receptor detection, calcium mobilization was markedly reduced compared to stimulated controls (Figure 5B). All findings were independent of timing or dosing for Alveofact®. For Curosurf®, a significant decrease in calcium mobilization in all concentrations was observed only at 30 min pretreatment. Interestingly PAD4 levels did not change for time or concentration (Figure 5C).
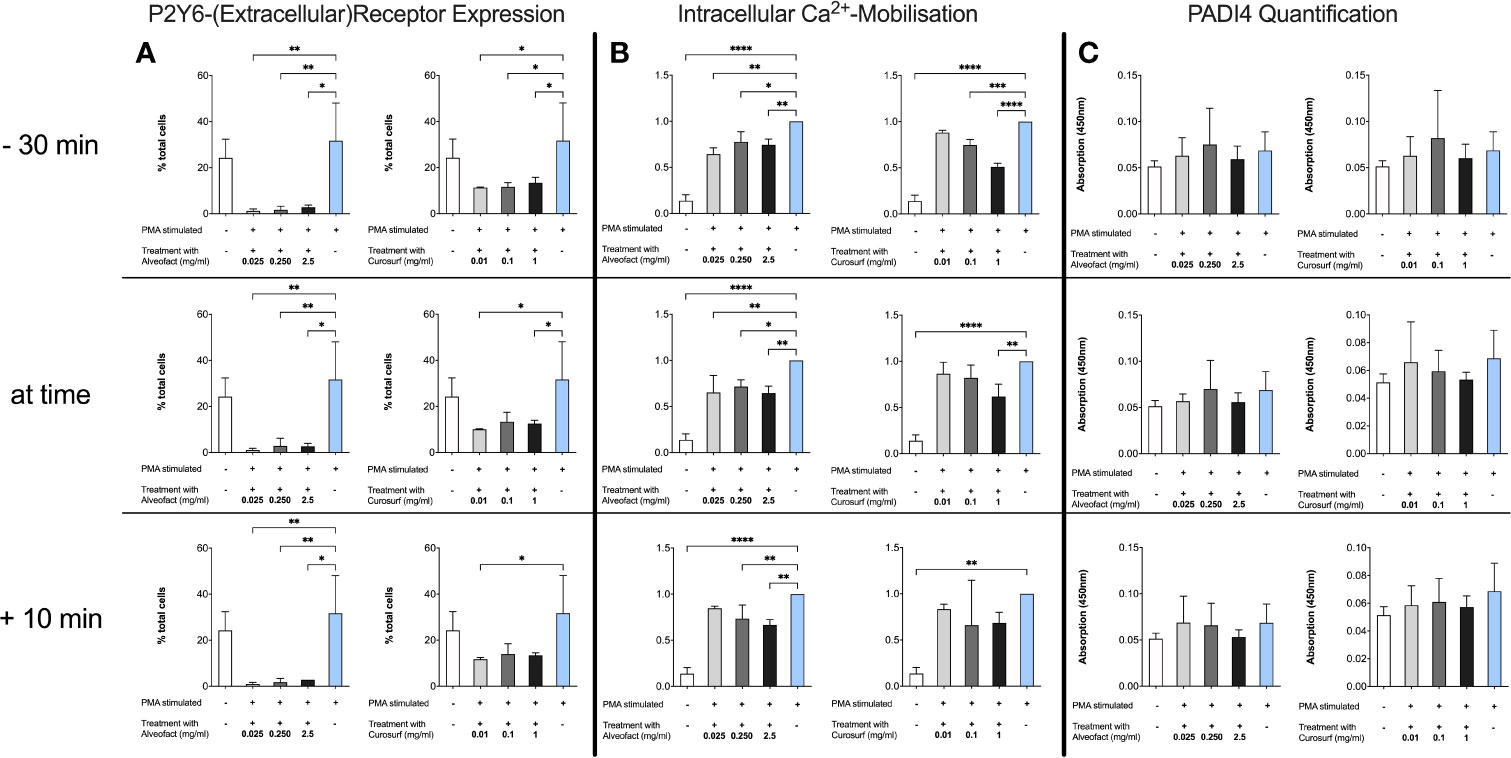
Figure 5 Decrease of P2Y6 Receptor and Calcium mobilization after treatment without change in intracellular PAD4 levels. (A) Neutrophils were treated as described before. P2Y6 was measured by flow cytometry with a FITC-conjugated antibody. Treatment with Alveofact® decreases extracellular expression of the P2Y6 receptor independent of timing or dosing, while Curosurf® does not exhibit this effect in every dosing. (B) Calcium levels at 3 h after treatment were used. Data were normalized to stimulated PMA control due to the heterogeneity in basic calcium levels and the response of the neutrophils to stimulation. Results are similar to the findings of the P2Y6 receptor expression. (C) Intracellular PAD4 levels were determined by ELISA using cell lysates of 1×106 cells. Interestingly, PAD4 levels did not change after treatment with surfactant compared to PMA-stimulated neutrophils. Significance level was set as p<0.05 (*<0.05, **<0.005,***<0.0005, ****<0.0001).
Discussion
Commercial surfactant preparations consist of the lipophilic components of animal surfactants (30) but lack SP-A and SP-D. It is assumed that the primary immunoregulatory effect of surfactant is exerted by these proteins. Recent studies have shown that phospholipids and the hydrophobic proteins SP-B and SP-C, which are essential for biophysical functions, also have anti-inflammatory and antibacterial functions. They may mediate the shown effects (26–29, 34).
Our study finds that the two natural surfactants, Curosurf® and Alveofact®, have a time- and dose-dependent inhibitory effect on NETosis in vitro when neutrophils have been stimulated with PMA. The stronger inhibitory effect in samples that were treated before activation with PMA implies a rather preventive than therapeutic effect on NETosis. The results suggest that the tested preparations reduce the activation of neutrophil granulocytes. Other immunologic cells like macrophages might be affected as well (20).
Regarding the underlying mechanisms of these anti-inflammatory properties, SP-B and SP-C have been demonstrated to reduce LPS-induced inflammation (35, 36). A potential mechanism of action may be based on the capability of SP-C to bind LPS via its N-terminal region (24). A novel synthetic surfactant, CHF5633, which contains SP-B and SP-C analogs showed to reduce LPS-induced tumor necrosis factor (TNF)-α and IL-1β cytokine production in human neonatal monocytes (37).
Several studies have investigated the potential effects of the surfactant components in transgenic knockout mice. SP-B deficiency leads to reversible pulmonary inflammation and induces neutrophil migration to the lung (27). Mice lacking SP-C have an excessive and persistent immune response of macrophages to viral and bacterial lung infections, leading to the conclusion that SP-C is a factor that minimizes inflammation. SP-D showed to inhibit LPS-induced NETosis by opsonizing pathogens (24). Other authors (38) already described the inhibitory effect of SP-D after stimulation with PMA on NETosis. Unlike SP-A and SP-D as part of the collectin family, SP-C does not opsonize pathogens, but absent SP-C results in impaired phagocytosis by alveolar macrophages (39). We found an inhibitory effect on NETosis independently of SP-D using PMA as a neutrophil activator.
Not only the proteins in pulmonary surfactant are relevant to host defense as studies on surfactant phospholipids have found. Kuronoma et al. discovered that anionic lipids of pulmonary surfactant attenuate LPS-induced inflammatory response in alveolar macrophages via the toll-like receptor 4–interacting proteins MD-2 and CD14 (29). Similar experiments by the same research group have revealed that the minor surfactant phospholipid palmitoyl-oleoyl-phosphatidyl-glycerol suppressed viral-elicited secretion of IL-6 and IL-8 by bronchial epithelial cells (28). Animal models and in vivo studies by van Rensburg et al. and Bezerra et al. have shown that exogenous surfactant administration leads to lower levels of proinflammatory biomarkers such as TNF-α, interferon (IFN)-γ, and IL-2 in the bronchoalveolar lavage fluid of mice and children (40, 41). Concomitantly there was increased expression of the anti-inflammatory cytokines IL-10 and IL-12 (41).
Dipalmitoylphosphatidylcholine, a major component of surfactant lipids, downregulates protein kinase C (PKC) and thereby reduces oxidative burst in human monocytes (42). As PMA leads to PKC-dependent activation of NADPH (nicotinamide adenine dinucleotide phosphate) oxidase in neutrophils and subsequently to NET formation, this may be a potential mechanism for the suppression of NETosis by surfactant (3). Sil et al. hypothesized that the P2Y6 receptor, a G protein-coupled receptor that transduces its signal by intracellular calcium levels, could potentially mediate NET formation by regulating IL-8 mediated neutrophil migration (43, 44). Our results, which show a decreased intracellular calcium mobilization along with decreased extracellular P2Y6 receptor expression support this statement. P2Y6 activation leads indirectly to the activation of the store-operated Ca2+ entry (SOCE) mechanism, which is the main mechanism of calcium signaling in neutrophils during activation (45). Researchers speculate that the SOCE inhibitor might diminish NADPH oxidase activity and by that might reduce NET formation (46). However, our study cannot provide further information whether the receptor is blocked or other mechanisms such as down-regulation or internalization of the receptor may play a role. There is currently no indication that PMA mediates its effects via the P2Y6 receptor. Therefore further studies are necessary to investigate the role of the P2Y6 receptor with regard to NET formation.
According to Proquitté et al., there is no significant clinical difference between Alveofact® and Curosurf® in terms of immediate response after administration and long term outcome parameters of treated premature infants (17). However, we observed a stronger inhibitory effect of Alveofact® on NETosis, which may be attributed to the different composition. Curosurf® is prepared from minced pig lungs, while Alveofact® is derived from bovine lung lavage, which is less contaminated with plasmatic and tissue residue due to its manufacturing process (47). As reported in a comparative study, Alveofact® contains a larger fraction of both SP-B and SP-C compared to Curosurf® (15). Another possible explanation for the differing results may be the higher concentrations used for Alveofact®.
It is well known that surfactant proteins are also present in extrapulmonary sites such as the skin and mucosal surfaces, which has already been substantiated in previous studies (48, 40). The accelerated skin wound healing by Alveofact® was attributed to downregulated gene expression of TNF-α and IL-1β as well as decreased levels of macrophages (50).
In summary, pulmonary surfactant minimizes inflammation through diverse mechanisms targeting various cell types. Many studies that have investigated these mechanisms are based on observations of alveolar macrophages and monocytes. Here, we provide the first data on the inhibition on neutrophil extracellular trap formation exerted by the two natural surfactants Alveofact® and Curosurf®. We found evidence that the P2Y6 receptor may play a role in the inhibition of NET formation via interacting in the calcium pathway. Further studies are needed to decipher the details of this pathway and the underlying molecular mechanisms.
Potential limitations to this study are the lack of synthetic surfactant or individual components of the composite formulation as controls. Nonetheless Alveofact® and Curosurf® could provide a preventive treatment option against the formation of NETs. As NETs are of great relevance in the pathogenesis of many inflammatory lung diseases, our study indicates new perspectives on how pulmonary surfactant may be a potential candidate for attenuating inflammation.
Data Availability Statement
The raw data supporting the conclusions of this article will be made available by the authors, without undue reservation.
Ethics Statement
The studies involving human participants were reviewed and approved by the medical research ethics committee of the medical chamber of Hamburg.
Author Contributions
AS and JT conceptualized the study. AS, JT, MT, BA, LR, and JK conducted the investigation. AS, JT, MB, and JK conducted the data curation and performed the formal analysis. AS and JT wrote the original draft. AS, LPR, IK, KR, MB, JT, and JPK wrote, reviewed, edited, and revised the manuscript. All authors contributed to the article and approved the submitted version.
Conflict of Interest
The authors declare that the research was conducted in the absence of any commercial or financial relationships that could be construed as a potential conflict of interest.
Acknowledgments
We thank the UKE microscopy imaging facility for providing microscopy services and the UKE FACS Sorting Core Unit for the flow cytometry services. Copyright permissions: Copyright permission was obtained for Curosurf® and Alveofact®.
Abbreviations
NETs, Neutrophil extracellular traps; NE, neutrophil elastase; MPO, myeloperoxidase; cfDNA, cell-free DNA; H3cit, citrullinated histone H3; SP-A, surfactant protein A; SP-B, surfactant protein B; SP-C, surfactant protein C; SP-D, surfactant protein D; PMA, phorbol-12-myristate-13-acetate; BSA, bovine serum albumin; DPBS, Dulbecco’s phosphate-buffered saline; RT, room temperature; DAPI, 4′,6-diamino-2-phenylindole; LPS, lipopolysaccharide; TNF, tumor necrosis factor; IL, interleukin; IFN, interferon; PKC, protein kinase C; CD, cluster of differentiation; NADPH, nicotinamide adenine dinucleotide phosphate; CRD, carbohydrate recognition domain; FACS, fluorescence-activated cell sorting; TMB, 3,3′,5,5′-tetramethylbenzidine; SOCE, store-operated Ca2+ entry.
References
1. Brinkmann V, Reichard U, Goosmann C, Fauler B, Uhlemann Y, Weiss DS, et al. Neutrophil extracellular traps kill bacteria. Science (2004) 303(5663):1532–5. doi: 10.1126/science.1092385
2. Segal AW. How neutrophils kill microbes. Annu Rev Immunol (2005) 23:197–223. doi: 10.1146/annurev.immunol.23.021704.115653
3. Fuchs TA, Abed U, Goosmann C, Hurwitz R, Schulze I, Wahn V, et al. Novel cell death program leads to neutrophil extracellular traps. J Cell Biol (2007) 176(2):231–41. doi: 10.1083/jcb.200606027
4. Porto BN, Stein RT. Neutrophil Extracellular Traps in Pulmonary Diseases: Too Much of a Good Thing? Front Immunol (2016) 7:311. doi: 10.3389/fimmu.2016.00311
5. Saffarzadeh M, Juenemann C, Queisser MA, Lochnit G, Barreto G, Galuska SP, et al. Neutrophil extracellular traps directly induce epithelial and endothelial cell death: a predominant role of histones. PloS One (2012) 7(2):e32366. doi: 10.1371/journal.pone.0032366
6. Downey DG, Bell SC, Elborn JS. Neutrophils in cystic fibrosis. Thorax (2009) 64(1):81–8. doi: 10.1136/thx.2007.082388
7. Hiroki CH, Toller-Kawahisa JE, Fumagalli MJ, Colon DF, Figueiredo LTM, Fonseca B, et al. Neutrophil Extracellular Traps Effectively Control Acute Chikungunya Virus Infection. Front Immunol (2019) 10:3108. doi: 10.3389/fimmu.2019.03108
8. Bruns S, Kniemeyer O, Hasenberg M, Aimanianda V, Nietzsche S, Thywissen A, et al. Production of extracellular traps against Aspergillus fumigatus in vitro and in infected lung tissue is dependent on invading neutrophils and influenced by hydrophobin RodA. PloS Pathog (2010) 6(4):e1000873. doi: 10.1371/journal.ppat.1000873
9. Abi Abdallah DS, Lin C, Ball CJ, King MR, Duhamel GE, Denkers EY. Toxoplasma gondii triggers release of human and mouse neutrophil extracellular traps. Infect Immun (2012) 80(2):768–77. doi: 10.1128/IAI.05730-11
10. Takei H, Araki A, Watanabe H, Ichinose A, Sendo F. Rapid killing of human neutrophils by the potent activator phorbol 12-myristate 13-acetate (PMA) accompanied by changes different from typical apoptosis or necrosis. J Leukoc Biol (1996) 59(2):229–40. doi: 10.1002/jlb.59.2.229
11. Wright TK, Gibson PG, Simpson JL, McDonald VM, Wood LG, Baines KJ. Neutrophil extracellular traps are associated with inflammation in chronic airway disease. Respirology (2016) 21(3):467–75. doi: 10.1111/resp.12730
12. Németh T, Sperandio M, Mócsai A. Neutrophils as emerging therapeutic targets. Nat Rev Drug Discov (2020) 19(4):253–75. doi: 10.1038/s41573-019-0054-z
13. Rugonyi S, Biswas SC, Hall SB. The biophysical function of pulmonary surfactant. Respir Physiol Neurobiol (2008) 163(1-3):244–55. doi: 10.1016/j.resp.2008.05.018
14. Polin RA, Carlo WA. Surfactant replacement therapy for preterm and term neonates with respiratory distress. Pediatrics (2014) 133(1):156–63. doi: 10.1542/peds.2013-3443
15. Bernhard W, Mottaghian J, Gebert A, Rau GA, von Der HH, Poets CF. Commercial versus native surfactants. Surface activity, molecular components, and the effect of calcium. Am J Respir Crit Care Med (2000) 162(4 Pt 1):1524–33. doi: 10.1164/ajrccm.162.4.9908104
16. Baroutis G, Kaleyias J, Liarou T, Papathoma E, Hatzistamatiou Z, Costalos C. Comparison of three treatment regimens of natural surfactant preparations in neonatal respiratory distress syndrome. Eur J Pediatr (2003) 162(7-8):476–80. doi: 10.1007/s00431-002-1144-0
17. Proquitte H, Dushe T, Hammer H, Rudiger M, Schmalisch G, Wauer RR. Observational study to compare the clinical efficacy of the natural surfactants Alveofact and Curosurf in the treatment of respiratory distress syndrome in premature infants. Respir Med (2007) 101(1):169–76. doi: 10.1016/j.rmed.2006.03.033
18. Ardell S, Pfister RH, Soll R. Animal derived surfactant extract versus protein free synthetic surfactant for the prevention and treatment of respiratory distress syndrome. Cochrane Database Syst Rev (2015) 8:Cd000144. doi: 10.1002/14651858.CD000144.pub2
19. Wright JR. Immunomodulatory functions of surfactant. Physiol Rev (1997) 77(4):931–62. doi: 10.1152/physrev.1997.77.4.931
20. Wright JR. Immunoregulatory functions of surfactant proteins. Nat Rev Immunol (2005) 5(1):58–68. doi: 10.1038/nri1528
21. Chroneos ZC, Sever-Chroneos Z, Shepherd VL. Pulmonary surfactant: an immunological perspective. Cell Physiol Biochem (2010) 25(1):13–26. doi: 10.1159/000272047
22. Ikegami M, Scoville EA, Grant S, Korfhagen T, Brondyk W, Scheule RK, et al. Surfactant protein-D and surfactant inhibit endotoxin-induced pulmonary inflammation. Chest (2007) 132(5):1447–54. doi: 10.1378/chest.07-0864
23. LeVine AM, Kurak KE, Bruno MD, Stark JM, Whitsett JA, Korfhagen TR. Surfactant protein-A-deficient mice are susceptible to Pseudomonas aeruginosa infection. Am J Respir Cell Mol Biol (1998) 19(4):700–8. doi: 10.1165/ajrcmb.19.4.3254
24. Arroyo R, Khan MA, Echaide M, Pérez-Gil J, Palaniyar N. SP-D attenuates LPS-induced formation of human neutrophil extracellular traps (NETs), protecting pulmonary surfactant inactivation by NETs. Commun Biol (2019) 2(1):470. doi: 10.1038/s42003-019-0662-5
25. Melton KR, Nesslein LL, Ikegami M, Tichelaar JW, Clark JC, Whitsett JA, et al. SP-B deficiency causes respiratory failure in adult mice. Am J Physiol Lung Cell Mol Physiol (2003) 285(3):L543–9. doi: 10.1152/ajplung.00011.2003
26. Mulugeta S, Beers MF. Surfactant protein C: its unique properties and emerging immunomodulatory role in the lung. Microbes Infect (2006) 8(8):2317–23. doi: 10.1016/j.micinf.2006.04.009
27. Ikegami M, Whitsett JA, Martis PC, Weaver TE. Reversibility of lung inflammation caused by SP-B deficiency. Am J Physiol Lung Cell Mol Physiol (2005) 289(6):L962–70. doi: 10.1152/ajplung.00214.2005
28. Numata M, Chu HW, Dakhama A, Voelker DR. Pulmonary surfactant phosphatidylglycerol inhibits respiratory syncytial virus-induced inflammation and infection. Proc Natl Acad Sci USA (2010) 107(1):320–5. doi: 10.1073/pnas.0909361107
29. Kuronuma K, Mitsuzawa H, Takeda K, Nishitani C, Chan ED, Kuroki Y, et al. Anionic pulmonary surfactant phospholipids inhibit inflammatory responses from alveolar macrophages and U937 cells by binding the lipopolysaccharide-interacting proteins CD14 and MD-2. J Biol Chem (2009) 284(38):25488–500. doi: 10.1074/jbc.M109.040832
30. Jeon GW. Surfactant preparations for preterm infants with respiratory distress syndrome: past, present, and future. Korean J Pediatr (2019) 62(5):155–61. doi: 10.3345/kjp.2018.07185
31. Fuchs TA, Kremer Hovinga JA, Schatzberg D, Wagner DD, Lammle B. Circulating DNA and myeloperoxidase indicate disease activity in patients with thrombotic microangiopathies. Blood (2012) 120(6):1157–64. doi: 10.1182/blood-2012-02-412197
32. Balaiya S, Chalam KV. An In vitro Assay to Quantify Nitrosative Component of Oxidative Stress. J Mol Genet Med (2014) 8(3):2–4. doi: 10.4172/1747-0862.1000120
33. Henderson LM, Chappell JB. Dihydrorhodamine 123: a fluorescent probe for superoxide generation? Eur J Biochem (1993) 217(3):973–80. doi: 10.1111/j.1432-1033.1993.tb18328.x
34. Yang L, Johansson J, Ridsdale R, Willander H, Fitzen M, Akinbi HT, et al. Surfactant protein B propeptide contains a saposin-like protein domain with antimicrobial activity at low pH. J Immunol (2010) 184(2):975–83. doi: 10.4049/jimmunol.0900650
35. Epaud R, Ikegami M, Whitsett JA, Jobe AH, Weaver TE, Akinbi HT. Surfactant protein B inhibits endotoxin-induced lung inflammation. Am J Respir Cell Mol Biol (2003) 28(3):373–8. doi: 10.1165/rcmb.2002-0071OC
36. Garcia-Verdugo I, Garcia de Paco E, Espinassous Q, Gonzalez-Horta A, Synguelakis M, Kanellopoulos J, et al. Synthetic peptides representing the N-terminal segment of surfactant protein C modulate LPS-stimulated TNF-alpha production by macrophages. Innate Immun (2009) 15(1):53–62. doi: 10.1177/1753425908100500
37. Glaser K, Fehrholz M, Papsdorf M, Curstedt T, Kunzmann S, Speer CP. The new generation synthetic reconstituted surfactant CHF5633 suppresses LPS-induced cytokine responses in human neonatal monocytes. Cytokine (2016) 86:119–23. doi: 10.1016/j.cyto.2016.08.004
38. Douda DN, Grasemann H, Palaniyar N. Surfactant Protein D Regulates NETosis. B55 HOST DEFENSE IN PULMONARY INFECTION AND TUBERCULOSIS. Am Thoracic Soc Int Conf Abstracts: Am Thoracic Soc (2012) A3283–A. doi: 10.1164/ajrccm-conference.2012.185.1_MeetingAbstracts.A3283
39. Glasser SW, Senft AP, Whitsett JA, Maxfield MD, Ross GF, Richardson TR, et al. Macrophage dysfunction and susceptibility to pulmonary Pseudomonas aeruginosa infection in surfactant protein C-deficient mic e. J Immunol (2008) 181(1):621–8. doi: 10.4049/jimmunol.181.1.621
40. Bezerra FS, Ramos CO, Castro TF, Araujo N, de Souza ABF, Bandeira ACB, et al. Exogenous surfactant prevents hyperoxia-induced lung injury in adult mice. Intens Care Med Exp (2019) 7(1):19. doi: 10.1186/s40635-019-0233-6
41. van Rensburg L, van Zyl JM, Smith J, Goussard P. Effect of exogenous surfactant on Paediatric Bronchoalveolar lavage derived macrophages’ cytokine secretion. BMC Pulm Med (2019) 19(1):236. doi: 10.1186/s12890-019-1006-4
42. Tonks A, Parton J, Tonks AJ, Morris RH, Finall A, Jones KP, et al. Surfactant phospholipid DPPC downregulates monocyte respiratory burst via modulation of PKC. Am J Physiol Lung Cell Mol Physiol (2005) 288(6):L1070–80. doi: 10.1152/ajplung.00386.2004
43. Sil P, Hayes CP, Reaves BJ, Breen P, Quinn S, Sokolove J, et al. P2Y6 Receptor Antagonist MRS2578 Inhibits Neutrophil Activation and Aggregated Neutrophil Extracellular Trap Formation Induced by Gout-Associated Monosodium Urate Crystals. J Immunol (2017) 198(1):428–42. doi: 10.4049/jimmunol.1600766
44. Itagaki K, Kannan KB, Livingston DH, Deitch EA, Fekete Z, Hauser CJ. Store-operated calcium entry in human neutrophils reflects multiple contributions from independently regulated pathways. J Immunol (2002) 168(8):4063–9. doi: 10.4049/jimmunol.168.8.4063
45. Demaurex N, Nunes P. The role of STIM and ORAI proteins in phagocytic immune cells. Am J Physiol Cell Physiol (2016) 310(7):C496–508. doi: 10.1152/ajpcell.00360.2015
46. Zhang H, Clemens RA, Liu F, Hu Y, Baba Y, Theodore P, et al. STIM1 calcium sensor is required for activation of the phagocyte oxidase during inflammation and host defense. Blood (2014) 123(14):2238–49. doi: 10.1182/blood-2012-08-450403
47. Blanco O, Perez-Gil J. Biochemical and pharmacological differences between preparations of exogenous natural surfactant used to treat Respiratory Distress Syndrome: role of the different components in an efficient pulmonary surfactant. Eur J Pharmacol (2007) 568(1-3):1–15. doi: 10.1016/j.ejphar.2007.04.035
48. Madsen J, Kliem A, Tornoe I, Skjodt K, Koch C, Holmskov U. Localization of lung surfactant protein D on mucosal surfaces in human tissues. J Immunol (2000) 164(11):5866–70. doi: 10.4049/jimmunol.164.11.5866
49. Mo YK, Kankavi O, Masci PP, Mellick GD, Whitehouse MW, Boyle GM, et al. Surfactant protein expression in human skin: evidence and implications. J Invest Dermatol (2007) 127(2):381–6. doi: 10.1038/sj.jid.5700561
Keywords: neutrophil granulocytes, neutrophil extracellular traps, pulmonary surfactant, anti-inflammatory, alveofact®, curosurf®
Citation: Schulz A, Pagerols Raluy L, Kolman JP, Königs I, Trochimiuk M, Appl B, Reinshagen K, Boettcher M and Trah J (2021) The Inhibitory Effect of Curosurf® and Alveofact® on the Formation of Neutrophil Extracellular Traps. Front. Immunol. 11:582895. doi: 10.3389/fimmu.2020.582895
Received: 06 August 2020; Accepted: 30 November 2020;
Published: 19 January 2021.
Edited by:
Martin Herrmann, University Hospital Erlangen, GermanyReviewed by:
Taruna Madan, National Institute for Research in Reproductive Health (ICMR), IndiaPayel Sil, National Institute of Environmental Health Sciences (NIEHS), United States
Copyright © 2021 Schulz, Pagerols Raluy, Kolman, Königs, Trochimiuk, Appl, Reinshagen, Boettcher and Trah. This is an open-access article distributed under the terms of the Creative Commons Attribution License (CC BY). The use, distribution or reproduction in other forums is permitted, provided the original author(s) and the copyright owner(s) are credited and that the original publication in this journal is cited, in accordance with accepted academic practice. No use, distribution or reproduction is permitted which does not comply with these terms.
*Correspondence: Julian Trah, anRyYWhAdWtlLmRl