- Department of Microbiology, Immunology and Molecular Genetics, Long School of Medicine, The University of Texas Health Science Center at San Antonio, San Antonio, TX, United States
Malaria remains a significant contributor to the global burden of disease, with around 40% of the world’s population at risk of Plasmodium infections. The development of an effective vaccine against the malaria parasite would mark a breakthrough in the fight to eradicate the disease. Over time, natural infection elicits a robust immune response against the blood stage of the parasite, providing protection against malaria. In recent years, we have gained valuable insight into the mechanisms by which IgG acts to prevent pathology and inhibit parasite replication, as well as the potential role of immunoglobulin M (IgM) in these processes. Here, we discuss recent advances in our understanding of the mechanisms, acquisition, and maintenance of naturally acquired immunity, and the relevance of these discoveries for the development of a potential vaccine against the blood stage of Plasmodium falciparum.
Introduction
Malaria is a deadly disease caused predominantly by the parasite Plasmodium falciparum. In 2018, an estimated 228 million cases occurred globally, resulting in 405,000 deaths, of which most were children (1). With the distribution of long-lasting insecticide-treated bed nets, increased insecticide spraying, and earlier diagnosis and treatment, major progress has been made in reducing morbidity and mortality since 2010. However, malaria continues to be a major global public health challenge and have devastating socioeconomical impact, mainly in Sub-Saharan Africa. Because of the spread of drug-resistant parasites and insecticide-resistant mosquitoes, as well as lack of access to treatment, vaccine development remains the most promising avenue for the eradication of malaria.
Vaccine development efforts have focused on multiple stages of the parasite life cycle, including the pre-erythrocytic stages and the asexual blood stage (Figure 1). While the development of sporozoite vaccines is in a more advanced stage than blood stage vaccines [reviewed in (2, 3)], strategies against both stages still face multiple hurdles. Therefore, the ultimate malaria vaccine may need to target both life cycle stages to reach sufficient vaccine efficacy. The Plasmodium asexual blood stage is responsible for symptomatic disease and can elicit a robust immune response [reviewed in (4)]. Over the course of multiple infections, antibody responses against blood stage parasites broaden and reach a level that protects against malaria (5–7). However, eliciting a long-lasting, protective antibody response by vaccination has proven a difficult task. Among the many reasons for the failures of historical blood stage vaccine candidates are i) sequence variation in vaccine targets that resulted in parasite strain-specific responses (8, 9), ii) inability of the vaccine to elicit sufficiently high antibody titers necessary for protection (10, 11), and iii) quick waning of elicited immune responses (6, 12–15). In parallel to these obstacles for vaccine development, many questions about the nature of naturally acquired immunity remain. For example, it is not fully understood why immunity against malaria develops relatively slowly, how long naturally acquired antibody responses are maintained in the absence of re-exposure, whether antibody responses are strain-transcending or a combination of strain-specific responses, and which (combinations of) antigen(s) should be prioritized for vaccine development.
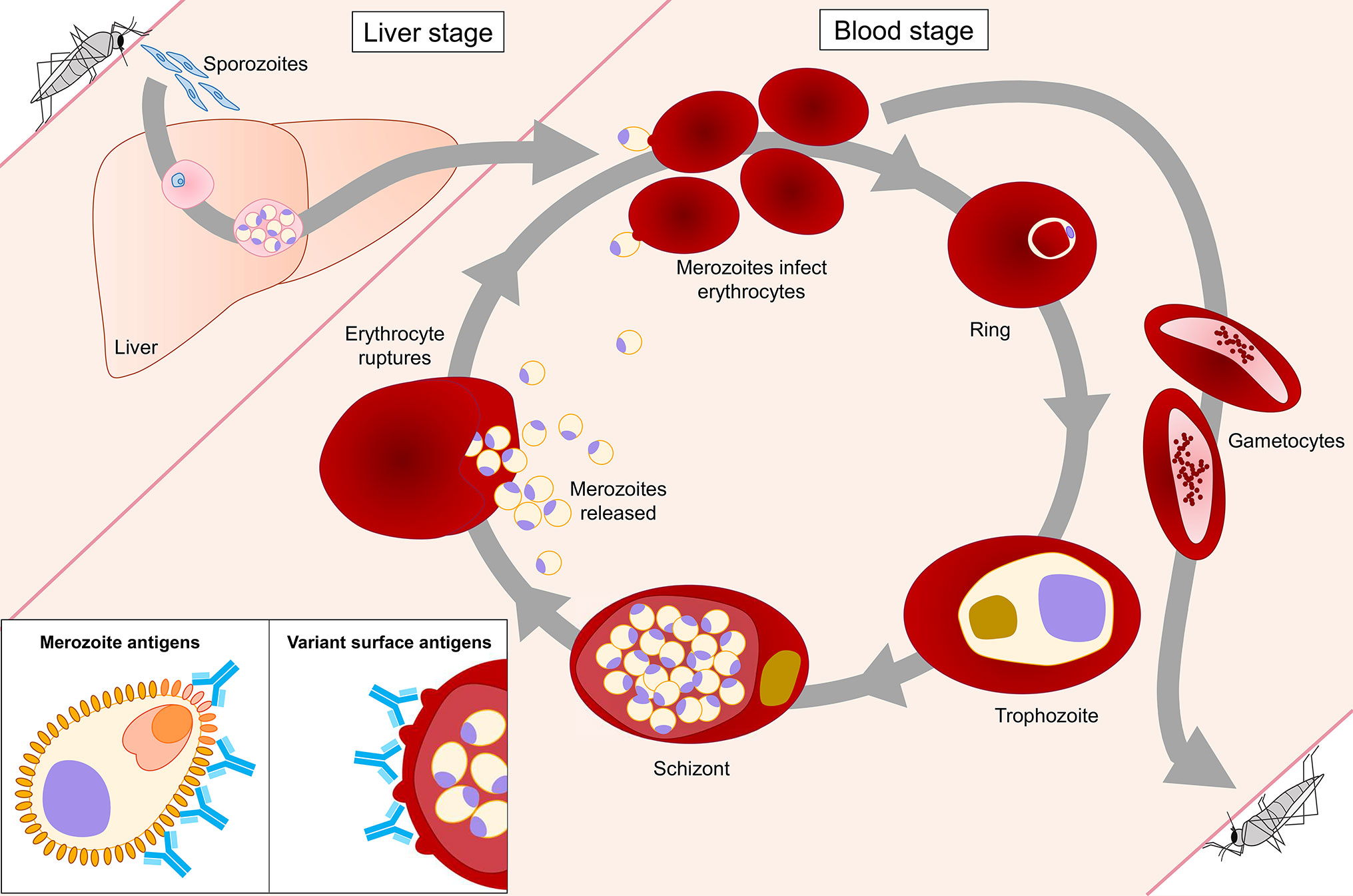
Figure 1 Plasmodium falciparum life cycle stages in the human host. The blood stage of P. falciparum is the only life cycle stage responsible for disease in the human host. During the intraerythrocytic developmental cycle (IDC), merozoites invade erythrocytes, followed by development and replication of the parasite through ring, trophozoite, and schizont stages, until new merozoites egress and the cycle repeats. Antibody responses that protect against malaria are directed against two classes of antigens expressed during the IDC. Merozoite antigens, such as MSP1 and AMA1, are common targets of antibodies generated during natural P. falciparum infection. Antibodies against these antigens prevent merozoite invasion of erythrocytes via various effector mechanisms, including neutralization, opsonic phagocytosis, and complement activation. Variant surface antigens (VSAs) are expressed on the surface of infected erythrocytes. Antibodies against VSAs, including PfEMP1, RIFINs, and STEVORs, prevent cytoadherence of infected erythrocytes to vascular endothelium and rosetting, thereby promoting clearance of parasite-infected erythrocytes by the spleen.
The main antigenic targets of blood stage parasites can be divided into two categories: i) parasite variant surface antigens on the cell membrane of infected erythrocytes and ii) proteins that are located on the merozoite surface or secreted by merozoites during erythrocyte invasion (Figure 1). Recent studies examining naturally acquired immunity have revealed important insights into antibody responses against these two groups of P. falciparum antigens, including their development and maintenance, key molecular and immunological mechanisms of parasite inhibition, and a potential role for immunoglobulin M (IgM) in the protective response. This review provides an overview of these discoveries and highlights their relevance for vaccine development.
Plasmodium falciparum Variant Surface Antigens
During the mature stages of the asexual blood stage, P. falciparum expresses variant surface antigens (VSAs) on the cell membrane of the infected erythrocyte. These proteins play crucial roles in both malaria pathogenesis and immune evasion. VSAs belong to multigenic families, most notably P. falciparum erythrocyte membrane protein 1 (PfEMP1), repetitive interspersed repeats (RIFIN), and subtelomeric variant open reading frame (STEVOR). PfEMP1 can bind to specific host receptors lining the vascular endothelium, resulting in sequestration of infected erythrocytes in capillaries, thereby preventing parasite clearance by the spleen (16–18) (Figure 2). In addition, members of all three VSA families mediate rosetting, the formation of a cluster of uninfected erythrocytes around an infected erythrocyte, which enhances microvascular obstruction (19–22). Rosetting and sequestration of infected erythrocytes can contribute to disease by reducing capillary perfusion and promoting parasite survival by preventing splenic clearance [reviewed in (23)]. Antibodies against VSAs may function by countering these immune evasion strategies of the parasite (24–26). In addition, anti-VSA antibodies may contribute to directly killing infected erythrocytes through the induction of opsonic phagocytosis (27) or antibody-dependent cellular cytotoxicity by natural killer (NK) cells (28).
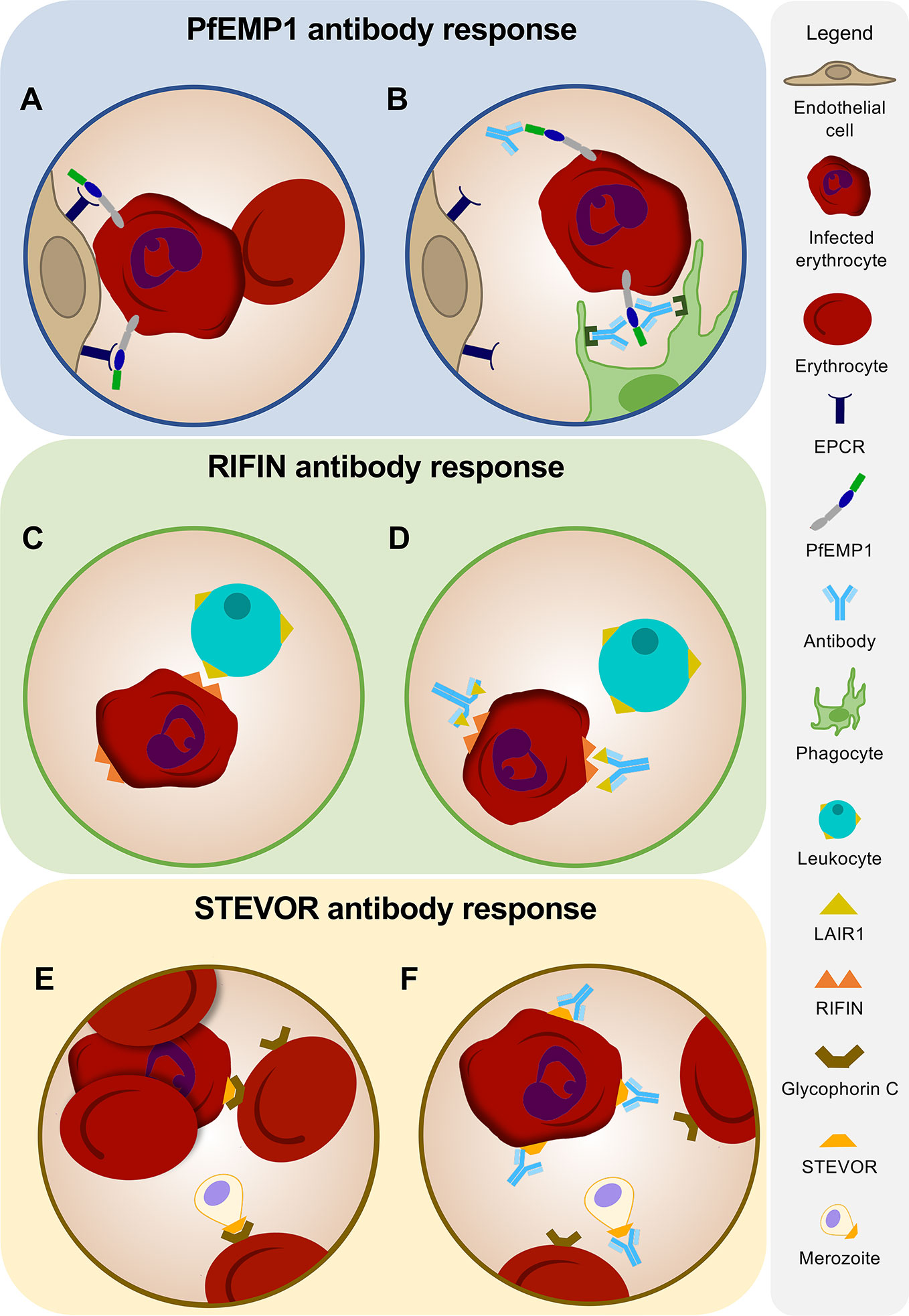
Figure 2 Antibodies against variant surface antigens. (A) PfEMP1 on the surface of an infected erythrocyte facilitates binding to receptors on human vascular endothelium. (B) Humoral immune responses against PfEMP1 inhibit attachment of infected erythrocytes to host endothelium and induce opsonic phagocytosis of infected erythrocytes. (C) Immune evasion of infected erythrocytes is mediated by RIFINs expressed on the surface of the erythrocyte. Binding of RIFINs to LAIR1 on B cells and natural killer (NK) cells results in suppression of immune responses. (D) Antibodies against RIFINs may function in preventing the binding of infected erythrocytes to LAIR1 on leukocytes. Broadly reactive antibodies to RIFINs were found to contain an insertion of a fragment of LAIR1 in the arm of the antibody heavy chain. (E) STEVORs mediate rosette formation and may play a role in merozoite invasion by adhering to glycophorin C on the erythrocyte surface. (F) Antibodies targeting STEVORs can prevent rosetting and inhibit attachment of merozoites to erythrocytes, thereby potentially limiting parasite replication and survival.
Antibody Responses Against PfEMP1
Each P. falciparum parasite encodes a diverse set of approximately 60 PfEMP1 proteins, but only one of these antigens will be expressed in a single parasite [reviewed in (29)]. PfEMP1 proteins display a high degree of sequence variation, both among the different variants encoded within a single parasite and among those expressed by different parasite strains. Switching PfEMP1 variant expression therefore contributes to quick and efficient evasion of host humoral immune responses by the parasite (18). Unfortunately, the extreme sequence diversity among PfEMP1 variants has hampered our ability to study naturally acquired immunity against these antigens, as well as the development of a PfEMP1-based vaccine.
PfEMP1 proteins are composed of two to ten cysteine-rich interdomain regions (CIDRα, β, γ, and δ) and Duffy binding-like domains (DBLα, β, γ, δ, ϵ, ζ, and x) that can be further divided into 147 subtypes (30–32). These different domains can bind to different host receptors, including endothelial protein C receptor (EPCR), intercellular adhesion molecule 1 (ICAM1), and CD36. These host endothelial receptors are not expressed at similar levels in all organs (33, 34). For example, CD36 expression is low in brain vascular endothelium, while expression of EPCR is high and expression of ICAM1 is upregulated under conditions of inflammation (33, 34). Pathogenesis, disease symptoms, and the development of severe malaria are therefore partially dependent on which PfEMP1 variant is expressed by the parasite.
Severe malaria is mainly seen in children under 5 years of age and includes life-threatening complications such as cerebral malaria and severe anemia. These severe disease manifestations have been associated with the expression of specific subtypes of CIDR and DBL domains, including CIDRα1, DBLα1, DBLα2, and DBLβ (35, 36). Protection from severe malaria is acquired early in life after only a limited number of infections (37). In the past few years, multiple independent cohort studies have revealed the role of PfEMP1 IgG antibodies in this protective response and have shed light on which PfEMP1 domains are the main targets of protective antibodies. Although all PfEMP1 domains appear to be immunogenic (38), subjects with uncomplicated malaria showed higher anti-PfEMP1 IgG titers and harbored IgGs that target PfEMP1 variants associated with severe malaria (27, 39–42). In a cohort of 448 young children (29–56 months old) in Papua New Guinea, children with uncomplicated malaria had higher levels of anti-PfEMP1 IgGs than children with severe malaria (27). The ability of these antibodies to induce opsonic phagocytosis suggests that direct killing of infected erythrocytes may contribute to protection. However, it remains to be definitively established whether cellular immune mechanisms or the prevention of cytoadherence is the main mechanism of action of these antibodies. Similar to these results, a protein microarray analysis of serum reactivity against 170 PfEMP1 fragments showed that children in Mali with severe malaria had lower PfEMP1 antibody levels and these antibodies recognized fewer PfEMP1 variants than antibodies in control subjects (41). In this study, participants were age and residence matched to minimize differences in parasite exposure between groups. This similarity in exposure levels was experimentally confirmed by the observation that children with cerebral malaria and healthy controls showed only minor differences in immune responses against intracellular PfEMP1 domains and merozoite antigens. However, a limitation of this study is that only PfEMP1 domains from the reference P. falciparum strain 3D7 were used, which does not include several known PfEMP1 domains that are associated with severe malaria and does not necessarily match the parasite strains that these children have been exposed to. An extensive study of antibody responses against 456 DBLα domain variants from local parasite isolates among 232 children (0.9–3.2 years old) in Papua New Guinea showed that IgGs against 85 DBLα1 and DBLα2 variants were associated with 70–100% reduction in severe malaria (40). In this study, protection against severe malaria could be further narrowed down to antibody responses against 17 DBLα variants, many of which were physically linked to EPCR-binding CIDRα1 domains (see below). These results suggest that immunity against severe malaria is the result of antibodies targeting a relatively small panel of conserved PfEMP1 domains (less than 5% of the full PfEMP1 repertoire), providing support for developing a vaccine based on these pathogenic PfEMP1 domains. Similarly, Tuju et al. reported that children in Kenya with mild or severe disease (n = 36) showed distinct serological IgG profiles against DBLα domain variants and a similar, but weaker, qualitative difference for IgM (42). The underlying mechanisms that give rise to these different serological outcomes remain to be further investigated and will be informative for vaccine development.
CIDRα1 is a PfEMP1 domain type that may play a dominant role in the development of protection against severe malaria. CIDRα1 domains have been shown to bind to EPCR, and results from several studies suggest that this interaction plays an important role in the development of severe malaria, in particular cerebral malaria (43–45). In cross-sectional studies in two independent cohorts, IgG responses against EPCR-binding CIDRα1 domains were found to develop earlier in life than antibodies against other PfEMP1 CIDR domains (46, 47), highlighting their potential role in protection against clinical disease. In addition, a longitudinal analysis in the Malian cohort showed an ordered acquisition of IgGs against different CIDRα1 domains, starting with IgGs against CIDRα1.7 and CIDRα1.8 (46). This could signify the ordered expression of PfEMP1 variants by P. falciparum, potentially in response to the immune status of the host. Alternatively, it could reflect differences in parasite fitness and transmissibility, resulting in early infections preferentially caused by parasites circulating at higher prevalence (46).
Given the high degree of sequence variation among PfEMP1 variants, one would expect that many PfEMP1 antibodies are strain-specific. However, CIDRα1 domains were shown to have conserved structural features that allow many different PfEMP1 variants to bind to the same host receptor (48). This structural conservation of the EPCR binding site may allow the immune system to produce antibodies that are cross-reactive against many different CIDRα1 domains. Indeed, IgGs isolated from serum by affinity purification using a peptide comprising the EPCR binding site of one CIDRα1 domain were able to bind to other CIDRα1 domains and prevented interaction of the domain with EPCR (48). These results suggest that the structural conservation of CIDRα1 domains may provide an opportunity for the development of a vaccine that elicits protection against severe malaria.
Antibody Responses Against RIFINs
RIFINs are encoded by a set of 150 to 200 genes and are highly antigenically varied (49). Following the pattern of the PfEMP1 family in P. falciparum, a single RIFIN is expressed by each parasite (19). Members of the RIFIN family preferably bind to blood group A erythrocytes to form large rosettes and induce cytoadherence, thereby potentially contributing to severe disease (19). In addition, it has recently been shown that RIFINs can bind to inhibitory receptors expressed on B cells, T cells, and NK cells, including leukocyte immunoglobulin-like receptor B1 (LILRB1) and leukocyte-associated immunoglobulin-like receptor 1 (LAIR1) (50) (Figure 2). Binding of these receptors to their natural ligands results in the suppression of immune responses (51–53). RIFINs bind the same region of LILRB1 as MHC-I, its natural ligand, and mimic the function of MHC-I by co-localizing with LILRB1 in the immunological synapse and inhibiting NK cell activation (54). In addition, it has been demonstrated that binding of RIFIN to LILRB1 inhibited IgM production in primary human B cells and reduced NK-mediated killing of target cells (55). A direct effect of RIFIN-binding to LAIR1-expressing mouse T cell hybridoma reporter cells has not been observed, but the effect of the interaction between RIFIN and LAIR1 has yet to be tested under more relevant experimental conditions, such as using primary human leukocytes (55). Based on these observations, it has been hypothesized that one of the functions of RIFINs is to down-modulate immune responses against P. falciparum through the binding to these inhibitory receptors.
Interestingly, it has been discovered that the human immune system exploits the ability of RIFINs to bind LAIR1 to generate broadly reactive IgM and IgG antibodies to RIFINs (56, 57). These antibodies have highly unusual structures, caused by an insertion of a human LAIR1 exon into the antibody heavy chain variable region CDR3 or into the switch region (Figure 2). Gene insertions in switch regions showed higher prevalence in class-switched memory B cells as compared to naive B cells, suggesting a role for activation-induced cytidine deaminase in facilitating such insertions (56, 57). Through somatic hypermutation, these LAIR1 domains lost the ability to bind to their natural ligand, collagen, thereby preventing the generation of auto-antibodies, but retained high affinity for RIFINs. These LAIR1 antibodies were found in 5–10% of malaria-experienced individuals, whereas only 0.3% of European donors had LAIR1-containing IgG (56, 57). However, the presence of LAIR1 antibodies did not correlate with protection against malaria. Thus, while LAIR1 antibodies are testament to the exceptionally strong selection pressure of P. falciparum on the immune response of infected individuals, the question remains whether antibodies against RIFINs contribute to protection.
IgG antibodies against RIFINs (irrespective of a LAIR1 insert) were detected in children in Tanzania as early as 1 year after birth, but levels did not increase with age (19). No difference was observed in serum IgG reactivity against three RIFINs on a protein microarray between children (1–6 years old) and adults (18–55 years old) in Mali (58). IgGs in the sera from the adults in this cohort bound to more RIFIN peptides in both semi-conserved and hypervariable domains than did the antibodies in the sera from the children (58). However, there was no difference in seroreactivity between A-type RIFINs, expressed on the surface of the infected erythrocyte, and B-type RIFINs, localized inside the parasite, suggesting that the increased antibody reactivity in adults reflects the higher cumulative exposure to the malaria parasite as compared to the children, but not protection (58, 59). In line with this observation, in children in Kenya, IgGs against PfEMP1 were associated with protection from malaria, while antibodies against other VSAs were not (60), suggesting that PfEMP1 proteins are the main target of protective immune responses against parasite proteins expressed on the erythrocyte surface.
Antibody Responses Against STEVORs
Of the VSA families discussed in this review, STEVORs were the last to be identified and remain the least characterized. STEVORs represent a family of approximately 40 polymorphic proteins encoded by genes found in subtelomeric regions of the P. falciparum genome (61). RNA-sequencing analysis of the P. falciparum 3D7 strain revealed a biphasic expression profile of STEVORs within the intraerythrocytic cycle, peaking in early trophozoites and late schizonts/merozoites (62). Additionally, STEVOR expression has been identified in the sporozoite and gametocyte stage of the parasite (63). Localization of STEVORs in all three stages is distinct, suggesting different functions based on the life cycle stage. In both trophozoites and gametocytes, STEVORs can be found on the infected erythrocyte membrane (63), where they likely play a role in parasite sequestration [reviewed in (64, 65)]. In addition, STEVORs have been detected on the apical surface of merozoites (66). STEVORs can bind to glycophorin C on the erythrocyte surface, leading to rosette formation of erythrocytes infected with mature parasites (67) and attachment of merozoite to the erythrocyte membrane (20). Given the widespread distribution of STEVORs throughout the P. falciparum life cycle, antibody responses against these targets may inhibit parasite replication at various stages. Indeed, STEVOR-targeting monoclonal antibodies showed a reduction in rosetting (67) and inhibition of merozoite invasion (20).
Although it is clear that STEVORs are involved in host-pathogen interactions, their role in disease pathogenesis is incompletely understood. Several cohort studies have attempted to unravel this role by analyzing serum antibody responses against STEVORs. In a cohort of Ugandan individuals aged 6–22 years old, seroprevalence of antibodies against STEVORs was lower as compared to anti-RIFIN antibodies (68). Grouping of participants based on risk of febrile malaria revealed only a single STEVOR commonly targeted by the humoral immune system of protected individuals (68). In this study, seroreactivity was tested using a library of 30 unique STEVOR recombinant proteins, each expressed as 1 or 2 truncated fragments (68). As a result, it is possible that antibody reactivity was missed because of misfolding of these protein fragments. Future studies will therefore need to evaluate host immune responses to full length STEVOR proteins. A similar study took a closer look at seroreactivity in children versus adults in a Malian cohort using a peptide array (58). Child and adult sera were able to bind all three RIFINs tested in this panel. While adult antibodies also reacted with all six STEVORs, children only showed reactivity to four of the six (58). A peptide array of these six STEVORs revealed that IgG from adults bound significantly more peptides and had more reactivity to semi-conserved and hypervariable regions as compared to IgG from children (58). When comparing sera obtained from children before and 90 days after a single clinical malaria episode, double the amount of STEVOR peptides were recognized by antibodies in the sera at the post-malaria time point, which more closely resembled the epitope profile targeted by adults (58). This study is limited in the repertoire of STEVOR proteins tested and only measured antibody responses against linear epitopes, but it does highlight how quickly antibodies to a single protein can develop over the course of a single infection. These findings are in line with the rapid acquisition of antibodies contributing to protection against severe disease after only a few clinical episodes.
Additional analyses will be required to distinguish if anti-STEVOR antibodies contribute to protection or merely correlate with antigen exposure. Future studies will require a more extensive analysis of STEVOR expression in clinical isolates, similar to what has been accomplished for PfEMP1. The use of 3D7-based antigens to measure anti-STEVOR antibody responses is a limiting factor when trying to understand the contribution of antibody response to protection, since analyses of clinical isolates have revealed regions within STEVORs with high sequence variation (69) and variability in STEVOR expression between fresh isolates and laboratory-adapted strains (66). Using STEVOR sequences from clinical isolates along with cohorts of individuals who are at risk of or clinically protected from malaria will provide a deeper understanding of variants involved in disease pathogenesis, anti-STEVOR antibody responses that are associated with protection, and potential targets for vaccine design.
Plasmodium falciparum Merozoite Antigens
Erythrocyte invasion by merozoites is an essential step in the asexual replication cycle of Plasmodium parasites, a process that can be blocked by antibodies [reviewed in (70)]. As a result, merozoite antigens have been the subject of intensive research over the past decades, which unfortunately has not (yet) translated into a successful blood stage vaccine [reviewed in (71)]. Merozoites express a large repertoire of proteins on their surface and in specialized secretory organelles, micronemes, and rhoptries, that are released during the invasion process [reviewed in (70)]. Titers of antibodies against members of the merozoite surface protein (MSP) family are typically among the highest detected in malaria-experienced individuals (72, 73), most likely because of their abundance and exposed nature. However, associations between antibody specificities in serum and protection against malaria are generally weaker for merozoite surface proteins than for microneme or rhoptry proteins (74, 75). Because of genetic polymorphisms and functional redundancies among merozoite proteins, it is still unclear which antigens are the best candidates for vaccine development (8, 9). Delineating the key merozoite antigens and the mechanisms by which antibodies against these antigens confer protection therefore remain important areas of research for the development of a blood stage vaccine.
Dissecting Protective Antibody Responses to Merozoite Antigens
The prioritization of antigens for vaccine development has been hampered by multiple issues, including our lack of understanding which immunological measures correlate with protection and which mechanisms of antibody-mediated parasite inhibition are most relevant for immunity. Large-scale efforts will therefore be needed to systematically compare antibody responses across different cohorts using multiple readouts. One of the most high-throughput approaches has been to compare antibody specificities between protected and susceptible people using protein microarrays. These analyses have shown that a large proportion of the P. falciparum proteome is immunogenic and that the breadth and durability of IgG responses increases with cumulative exposure (5–7). In addition, protected individuals have higher levels of IgG against a broad range of antigens, with unique serological profiles for each individual (5–7). The difference between protection from and susceptibility to malaria cannot simply be explained by the presence or absence of one or a small number of antibody specificities. To better understand the high complexity of such data sets and move beyond analyzing IgG responses to individual antigens, recent studies have used machine learning approaches that are able to assess combinations of responses and their association with protection (76, 77). An analysis of protein microarray data comprising antibody reactivity against more than 1,000 antigens in children (2–10 years old) and young adults (18–25 years old) showed that protection was associated with a qualitative shift in antibody responses, with an increase in antibody titers against VSAs and a decrease in antibody titers against antigens enriched for conserved proteins with unknown function (77). Proietti et al. identified an immune signature of IgG responses against 15 antigens that predicted protection from malaria across two cohorts (76). Interestingly, most of these proteins were localized intracellularly and were expressed at different stages of the P. falciparum life cycle, making them unlikely direct targets of parasite-inhibitory antibodies. However, these results may highlight the importance of other components of the adaptive immune system, such as T cell responses, in driving protective B cell immunity. In addition, this immune signature can be used to predict disease susceptibility, monitor the acquisition of naturally acquired immunity over time, and assess the impact of malaria interventions.
One shortcoming of these large-scale protein microarray studies is that they only assess antibody binding, not antibody effector functions such as opsonization or complement fixation. Antibodies that bind to merozoite antigens do not necessarily inhibit erythrocyte invasion. For example, it has been shown that the activity of inhibitory anti-MSP1 IgG can be blocked by non-inhibitory antibodies that compete for binding to overlapping epitopes or bind to more distal regions and presumably prevent binding of inhibitory antibodies through steric hindrance (78). Assays that assess different antibody effector functions are therefore essential to measure the ability of antibodies to inhibit parasite replication or induce parasite killing by immune cells. In recent years, the importance of antibody effector functions in parasite inhibition has been demonstrated and several studies have started to delineate the contribution of antibodies to individual antigens in this process. In the following sections, we summarize recent advances in our understanding of the nature, and mechanisms of acquired antibody-mediated protection against P. falciparum merozoite invasion.
Antibody Responses Against Combinations of Merozoite Antigens
Recent studies on naturally acquired immunity have provided the important insight that the breadth of antigen recognition is more predictive of immunity than antibody reactivity against any single antigen (74, 75, 79–82). This would suggest that a vaccine based on a single antigen may not be successful in eliciting a fully protective antibody response. To better understand how many different antigens should be targeted to achieve protection and whether antibody responses against certain combinations of antigens perform better than others, the cumulative protective effect of individual antibody specificities has been analyzed. In both Sub-Saharan African and Indian populations, combinations of IgGs against four or five antigens provided nearly full protection against malaria, with only limited contributions from antibodies against additional antigens (80, 82). The most effective combinations were reported to target antigens presented during distinct stages of the invasion process, thereby working synergistically to inhibit invasion (79). In some of these combinatorial analyses, IgG responses against several uncharacterized proteins that do not have a functional annotation but are referred to by their gene identifiers (for example, PF3D7_1136200 and PF3D7_0606800) were associated with the highest levels of protection. These findings support the approach to develop a multi-antigen vaccine and encourage further evaluation of several novel protective antigens as potential vaccine candidates.
Strain-Transcending Immunity
Circulating P. falciparum strains are genetically diverse (8, 9). One of the challenges for vaccine development is to elicit immunity against these genetically distinct parasite strains. Passive immunization studies in the 1960s and 1990s showed that the transfer of IgG from immune individuals living in West Africa to malaria patients in East Africa or Thailand resulted in almost complete clearance of parasitemia in the absence of other treatment (83, 84). These results suggest that IgG from immune individuals can inhibit the replication of different P. falciparum strains from diverse geographical regions. However, the question remained whether this protective effect was the result of a combination of strain-specific antibodies targeting multiple genetically diverse antigens, or if this was due to one or more antibodies targeting conserved epitopes and thereby conferring strain-transcending immunity. A recent study by Hill et al. sought to answer this question by measuring opsonic phagocytosis of merozoites from 15 different parasite strains induced by serum antibodies from semi-immune children (5–14 years old) living in Papua New Guinea (85). Children with serum IgGs that promoted phagocytosis of merozoites from all strains tested showed an 85% decrease in the risk of developing malaria during 6 months of follow-up as compared to children with narrow opsonic phagocytosis activity in serum. Depletion of serum antibodies that bound to one merozoite strain drastically reduced opsonization of merozoites from other P. falciparum strains. These results suggest that opsonic phagocytosis activity against merozoites from different strains is mediated by the same antibodies. However, it is important to mention that P. falciparum exposure levels were not matched between groups and it can therefore not be ruled out that differences in opsonic phagocytosis activity between these groups were driven by differences in antibody titers. More research will be required to fully understand the dynamics of strain-transcending immunity and to identify the conserved epitopes targeted by this response.
Antibody Effector Functions Associated With Protection
One of the priorities for malaria vaccine development is the establishment of correlates of protection and the standardization of in vitro assays to measure these immunological parameters. It is therefore important to better understand the molecular and immunological mechanisms by which antibodies inhibit merozoite invasion. In general, antibodies can act by blocking interactions between molecules (i.e., neutralization), or by promoting Fc-mediated effector functions, including complement activation, opsonic phagocytosis, and antibody-dependent cellular cytotoxicity.
Antibodies against several merozoite antigens have been shown to act by neutralization. For example, IgG binding to EBA-175 prevented attachment of this protein to glycophorin A on the erythrocyte surface, thereby inhibiting invasion (86). Similarly, anti-PfRH5 IgG engineered to not engage in any Fc-mediated effector functions was able to protect against P. falciparum challenge, demonstrating that neutralization is likely to be the primary mechanism of action (87). Although the growth inhibition assay has traditionally been the gold standard in vitro assessment of antibody neutralization, it generally does not correlate well with naturally acquired protection against malaria and may therefore be considered less valuable for assessing naturally acquired immune responses than other, more recently developed, assays (74). However, the growth inhibition assay seems well suited to measure the activity of antibodies that are known to mediate their effect through neutralization. We speculate that these antibodies most likely target antigens that are less abundant on the merozoite surface and are involved in essential interactions with ligands on the erythrocyte (Figure 3). The growth inhibition assay may therefore be the assay of choice to evaluate vaccine-elicited antibody responses against EBA-175, PfRH5, and potentially other antigens.
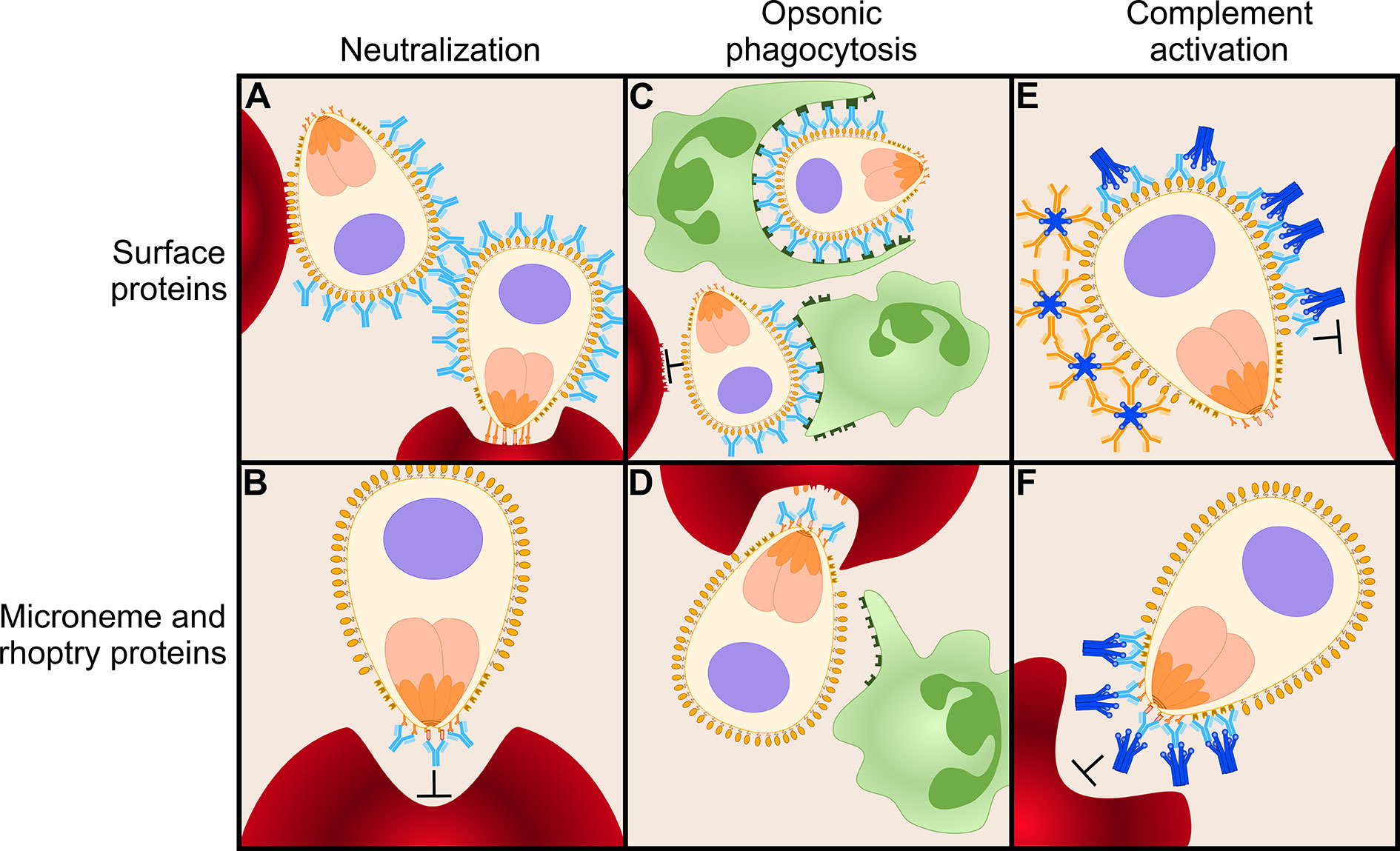
Figure 3 Antibody-mediated inhibition of merozoites. Antibodies can function in several ways to mediate inhibition of merozoite invasion into erythrocytes. Here, we present a model of various antibody effector mechanisms for two different classes of antigens: highly abundant merozoite surface proteins and relatively scarce microneme and rhoptry proteins. (A) Immunoglobulins G (IgGs; shown in light blue) targeting surface proteins are not effective mediators of inhibition via neutralization, possibly because extremely high antibody concentrations are necessary to completely block the coat of proteins on the merozoite surface. (B) IgGs can effectively neutralize merozoites when targeting relatively scarce microneme and rhoptry proteins on the apical surface, such as PfRH5 and EBA-175. (C) When targeting abundant surface proteins, opsonic phagocytosis is effectively mediated via IgG1 and IgG3 by binding of phagocytic cells (green) to their Fc domain. (D) Many microneme and rhoptry proteins are only released to the surface of the merozoite once it has attached to an erythrocyte and is ready to commence the invasion process. At this moment, the erythrocyte will (partially) shield IgGs bound to microneme and rhoptry proteins from the Fc receptors of phagocytes. Thus, opsonic phagocytosis may not be an efficient effector function for antibodies against this class of proteins. (E) Both IgGs and IgMs (orange) efficiently initiate the classical complement cascade via C1q (dark blue) when targeting surface proteins to prevent merozoite invasion. (F) IgG is an effective mediator of the complement cascade when targeting microneme and rhoptry proteins. IgM may be less efficient against this class of proteins because large, pentameric IgM may not be able to reach between the erythrocyte and merozoite surfaces.
The implementation of novel functional assays has revealed the role of opsonic phagocytosis and complement fixation in antibody-mediated inhibition and has uncovered these effector functions as correlates of naturally acquired protection. As mentioned, opsonic phagocytosis by naturally acquired antibodies, mainly IgG1 and IgG3, has been reported to be strain-transcending (85) and correlates well with protection from disease (81, 88). Opsonic phagocytosis of whole merozoites increased with greater breadth of antibody responses (81, 88), confirming that targeting multiple antigens is beneficial for anti-merozoite immunity. Various antigens have been identified as targets for these opsonizing antibodies, including MSP1, MSP2, and MSP3 (86, 88, 89). To further delineate the role of individual antigen specificities, Kana et al. used beads coated with recombinant proteins to measure the ability of IgG antibodies against single merozoite antigens to induce opsonic phagocytosis (90). Antibody-induced phagocytosis in this assay showed an association with protection against malaria in a longitudinal cohort of 134 children in Ghana for MSP1-19, MSP2 (3D7 allele), MSP3 (N-terminal region of K1 allele), GLURP (region 2), and PfRH2-2030 (the fragment of PfRH2 covering amino acid residues 2030–2528), but not against 21 other antigens that have been implicated as targets of the protective immune response (90). Combinations of antibody responses against six antigens correlated better with protection than responses to any individual antigen, suggesting that antibodies against multiple antigens may be necessary to induce optimal merozoite phagocytosis.
Complement fixation and subsequent activation of the classical complement pathway is also strongly associated with protection for various merozoite antigens (74, 91). This complement cascade culminates in the formation of the membrane attack complex, which mediates merozoite lysis. Reiling et al. determined that fixation of C1q, the first step in the complement cascade, correlated strongly with membrane attack complex formation and therefore measured the ability of serum IgM and IgG antibodies against various merozoite antigens to bind C1q as a proxy of complement-activating potential (74). The prevalence of C1q-fixing antibodies was high for the majority of merozoite antigens studied and correlated strongly with protection against malaria. The strongest protective associations were observed for C1q-fixing antibodies against EBA-140 (region III–V), MSP7, rhoptry-associated leucine zipper-like protein 1 (RALP1), GPI-anchored micronemal antigen (GAMA), and PfRH2-2030 (74). Again, combinations of antibodies against three different antigens showed an increased protective effect over single antigens or combinations of two antigens, with RALP1 and MSP7 among the antigens most frequently found in protective combinations.
Interestingly, strong opsonic phagocytosis activity was mainly observed for antibodies targeting antigens expressed on the merozoite surface, while the strong complement-fixing activity was predominantly observed for antibodies directed against rhoptry and microneme proteins (74, 90). Of the antigens that were tested in both assays, antibodies that displayed strong opsonic phagocytosis activity against three out of four antigens in the bead-based assay performed by Kana et al. showed relatively weak or no complement-fixing activity and the reverse was true for IgG antibodies against EBA140RIII-V and RALP1 (74, 90). It is likely that multiple antibody effector mechanisms act in concert to provide immunity (92). Both complement fixation and opsonic phagocytosis are induced by the cytophilic IgG1 and IgG3 antibodies, underlining that a vaccine-induced response against merozoite antigens should be directed toward these isotypes. The results from Reiling et al. and Kana et al. suggest that cytophilic antibodies against different antigens preferentially act through one particular effector function, and it will be interesting to understand which antigenic properties influence this response. One explanation for the preferential opsonic phagocytosis activity of antibodies against merozoite surface proteins could be that many antigens located in rhoptries and micronemes only become exposed when the merozoite is in direct contact with an erythrocyte (93) [reviewed in (70, 94)] and may therefore be poorly accessible to phagocytes (Figure 3). However, the differences between results from Reiling et al. and Kana et al. could also be influenced by variation in antibody titers between individuals, the studied antigens, characteristics of the cohort, inclusion criteria for participants selected for the study, as well as other variables. To further evaluate the contribution of antibody specificities and effector mechanisms to inhibition of merozoite invasion, samples from the same individuals should be compared side-by-side in assays that measure these different effector functions against the same panel of merozoite antigens. Epitope mapping of antibodies with different levels of inhibitory activity against merozoites will also provide important insights into immune mechanisms. For example, binding of monoclonal IgGs to a non-neutralizing epitope of merozoite protein PfRH5 resulted in slower erythrocyte invasion, thereby increasing exposure times of other epitopes on PfRH5 to neutralizing antibodies (95). This resulted in a synergistic effect between the non-neutralizing and neutralizing antibodies (95). For MSP1, epitope mapping of non-inhibitory and inhibitory antibodies revealed MSP1 cleavage sites as the most vulnerable sites of this antigen (96). It would be highly informative to also perform such studies for other antigens to better understand how antibodies function and which epitopes should be targeted by vaccination to achieve an effective immune response.
Immunoglobulin M Responses Against Merozoite Proteins
Although the focus of studies on naturally acquired immunity has been on IgG, recent studies suggest that IgM may also play an important role in protection (97–99). IgM is an excellent mediator of complement activation and may function in this way to confer immunity (98). An interesting observation about the role of IgM in protection against malaria has come from a comparison between the Fulani and Dogon people, two genetically distinct ethnic groups living in West Africa with different susceptibility to malaria (100). The Fulani are more resistant to malaria but carry lower frequencies of classical malaria-resistance genes (101), which has prompted a search for immunological variables that can explain the observed differences in malaria susceptibility. Differences in the frequency of genetic variants in several immune-related genes have been identified between the Fulani and Dogon people, but the immunological consequences of these results are unclear (102). Transcriptional profiling showed that the Fulani have a strong inflammatory gene signature in the monocyte population during P. falciparum infection, leading to the hypothesis that monocytes in Fulani people exist in a “primed” state that will elicit a stronger immune response upon infection (103). The Fulani also had higher frequencies of activated memory B cells in the absence of infection and higher percentages of plasma cells during P. falciparum infection (104). These enhanced cellular immune responses most likely explain the increased IgM and IgG levels against many P. falciparum proteins in the Fulani as compared to the Dogon (97). Interestingly, the Fulani IgM responses recognized a broader repertoire of P. falciparum proteins than IgG responses, including a set of antigens to which antibody levels have been correlated with protection (97). These results suggest that IgM may play an underappreciated role in protection against malaria.
In a mouse model, IgM+ memory B cells were shown to be the first responders in secondary Plasmodium infections, giving rise to both IgM and IgG antibody-secreting cells (99). IgM+ memory B cells contained somatic hypermutations and recognized target antigens with high affinity, pointing to T-cell dependent affinity maturation within the germinal center. IgM+ memory B cells with specificity for merozoite antigens were also detected in malaria-experienced humans (99). Finally, Boyle et al. recently showed that IgM against merozoite antigens appears rapidly upon P. falciparum infection and reaches high levels in individuals with life-long exposure (98). IgM levels were more stable under low transmission conditions than IgG levels and were associated with protection (98). However, children with high IgM levels also had high levels of IgG against merozoite antigens, which were also associated with protection. The independent contribution of these antibody classes to immunity therefore remains to be determined.
The Acquisition and Maintenance of Humoral Immune Responses Against Plasmodium falciparum
Naturally acquired antibody responses against P. falciparum require repeated parasite exposure to attain protection, while probably never reaching sterilizing immunity. The rate of antibody acquisition against P. falciparum proteins is influenced by various factors, including age of the human host, transmission intensity, and the type of antigen (72, 73, 105, 106). In general, antibody levels increase with both age and higher transmission intensity (72, 73, 105). Antibodies against different antigens accumulate at varying rates, with stronger responses typically seen for antibodies targeting extracellular or plasma membrane proteins and proteins that are highly abundant, highly polymorphic, or lack a human ortholog (106).
It has been shown that IgGs against PfEMP1 are acquired during the first years of life (47, 107), and their breadth increases with age and exposure (38, 60). In a cohort of children from Papua New Guinea, levels of PfEMP1 IgG antibodies positively correlated with protection in both younger children (1–4 years) and older children (5–14 years) (107). Interestingly, antibodies against merozoite antigens were only associated with protection in older children in this cohort (107). These results suggest that antibodies against PfEMP1 may provide a first line of defense against severe malaria pathogenesis early in life, while antibodies to other parasite antigens may contribute to protection against uncomplicated malaria in older children. This may seem counterintuitive given the extremely high diversity among PfEMP1 variants, at the level of both amino acid sequences and domain composition, while polymorphisms in merozoite antigens provide relatively modest sequence variation in comparison. However, as detailed in Section 2.1, only a small fraction of PfEMP1 domains are pathogenic, with particularly strong evidence for the role of CIDRα1 in severe malaria. Targeting one or a small panel of such pathogenic PfEMP1 domains may be sufficient to achieve protection against severe disease. In contrast, a broader antibody response with higher antibody titers may be necessary to effectively inhibit erythrocyte invasion by merozoites. Indeed, it has been reported that antibodies against merozoite antigens must reach a threshold level before associating with protection (10, 11). While such a functional threshold level is also likely to exist for antibodies against PfEMP1 and antigens on other pathogens, the requirement for recognition of multiple merozoite antigens at high antibody concentrations may be exceptionally high for Plasmodium infections. This may explain why relatively low antibody levels against merozoites in young children are not sufficient to provide protection to malaria in these individuals, while similar levels of anti-PfEMP1 antibodies confer protection against severe disease. The contribution of immune responses against these two main groups of antigens to immunity remains to be fully untangled.
An additional complication to the generation of a protective antibody response against P. falciparum is the ability of the parasite to modulate immune responses via several mechanisms. Plasmodium infections induce a pro-inflammatory immune response that drives differentiation of CD4+ T cells into Th-1 polarized T follicular helper cells, resulting in impaired germinal center (GC) responses (108, 109). This pro-inflammatory environment also induces the expansion of a subset of B cells known as “atypical memory B cells” (110, 111) [reviewed in (112, 113)]. These cells express inhibitory receptors and have been described as functionally impaired (114, 115), although several studies have reported that these cells may in fact be functional and can contribute to anti-parasite immunity (116, 117). It has been postulated that naive B cells differentiate into atypical memory B cells in B cell follicles upon stimulation with interferon gamma produced by Th-1 polarized T follicular helper cells and Toll-like receptor agonists (118). In this process, atypical memory B cells may bypass the germinal center (GC) or exit the GC prematurely, thus limiting affinity maturation and the ability of these cells to produce high affinity antibodies. Finally, a recent study showed that in both human and mouse experimental Plasmodium infections, a large proportion of activated B cells differentiated into extrafollicular plasmablasts (119). The high demand of replicating plasmablasts for nutrients, in particular the amino acid L-glutamine, outcompeted that of GC B cells, thereby limiting the development of memory B cells and long-lived plasma cells. The disruption of GC formation during Plasmodium infections likely hampers the development of B cells with high affinity for parasite antigens. It is however conceivable that the chronic and repetitive nature of P. falciparum infections will ultimately allow for the acquisition of high levels of somatic hypermutations by consecutive rounds of memory B cell activation and affinity maturation, as observed in, for example, HIV-1-infected individuals (120–122). Alternatively, high affinity plasma cells could be derived from naive B cell precursors with intrinsic high binding affinity, which would limit the need for effective germinal center reactions (123). Longitudinal molecular studies of antibody evolution have not been performed in naturally P. falciparum-exposed individuals but would be useful to better understand how protective antibody responses develop over time.
Given the high levels of antibodies required for protection against malaria, protective antibody responses are generally not sustained over long periods of time without re-exposure. Antibody levels are maintained by long-lived antibody-secreting cells in the bone marrow. While these plasma cells are not easily accessible for study in humans, their half-life can be modeled based on serum antibody levels. Using this approach, Yman et al. found that antibody levels against merozoite antigens as well as the proportion of long-lived antibody-secreting cells after a malaria episode in individuals who were formerly exposed because of residency in an endemic area (a median of 14 years ago) were higher compared to previously malaria-naive travelers (12). These results suggest that repeated P. falciparum infections are necessary to drive the formation of long-lived antibody-secreting cells, providing an explanation for the observation that immunity requires prolonged exposure. In addition, the half-life of merozoite antigen-specific long-lived plasma cells was 2–4 years, which was shorter than that of long-lived antibody-secreting cells against tetanus toxoid (7 years) and explains why some degree of exposure is necessary to maintain immunity (12). However, this study also demonstrated that immunological memory is not completely lost after more than a decade of non-exposure. These results are in agreement with an earlier report that showed that in five Swedish residents who were previously treated for malaria following international travel, P. falciparum merozoite antigen-specific memory B cells were detected up to 16 years later in the absence of re-exposure (124). Findings from a recent study in a low transmission region in Zambia suggest that the level of exposure necessary to maintain antibody levels is probably very low (73). In this setting, parasite prevalence in the population was approximately 2.5%, most infections were asymptomatic, and about half of all individuals with parasitemia had parasite levels that were undetectable with rapid diagnostic tests. In the absence of significant recent transmission, adults showed stable antibody levels over a period of 2 years, suggesting that infrequent infections with low-level parasitemia are sufficient to maintain humoral immune responses. Among targets of the strongest antibody responses were PfEMP1 variants and merozoite antigens. Neither of the studies discussed above included a functional assessment of antibody responses, and it is thus unclear whether the antibodies measured in these studies contribute to protection. However, assuming that long-lived antibody-secreting cells and antibodies targeting protective epitopes follow similar kinetics, these results suggest that long-term protection by vaccination may be possible with frequent boosting of the humoral immune response.
Concluding Remarks
The main targets of naturally acquired immune responses against P. falciparum are variant surface antigens on the plasma membrane of the infected erythrocytes and antigens expressed by merozoites. Antibody responses against PfEMP1 variants develop early in human life and seem to be the main driver of protection against severe malaria in young children. Protection against malaria in older children is achieved once antibodies against merozoite antigens reach sufficiently high levels. A vaccine based on a combination of these two classes of antigens may elicit antibodies that have an additive effect, or possibly even act synergistically, toward the prevention of all clinical manifestations of malaria.
The main obstacle for the development of a vaccine targeting PfEMP1 is the identification of conserved epitopes, given the enormous sequence variation among this protein family. The CIDRα1 domain has the most potential as a vaccine candidate against severe malaria, given its role in the pathogenesis of severe malaria through binding to EPCR, and because of structural conservation of the EPCR binding site.
Correlations with protection have been observed for antibody responses against many different merozoite antigens. Broader antibody responses provide better protection, with near full protection for responses against combinations of three to four antigens. What remains unclear is which of these (combinations of) antigens should be moved forward for vaccine development. Since many of these antigens are polymorphic, the identification of conserved epitopes has high priority. In addition, several candidates that have been identified have unknown functions and further characterization of these proteins will provide more insight into the merozoite invasion process.
Finally, recent studies have revealed mechanisms of antibody-mediated parasite inhibition. Understanding how antibodies function against different target antigens is important to be able to induce the most effective immune response by vaccination and to establish correlates of protection. Nature has provided us with proof-of-concept that protection against blood stage infection is achievable. Our increased understanding of naturally acquired immunity therefore provides hope for the development of a malaria blood stage vaccine.
Author Contributions
The first draft of the manuscript was prepared by SG and RR, and revised by EB. AB, SB, and GB further edited the manuscript and provided intellectual contributions to the design of the figures. All authors contributed to the article and approved the submitted version.
Funding
This work was supported by the National Institutes of Health [grant numbers AI128466 and AI133274]. SG and AB are supported by Graduate Research in Immunology Program training grant NIH T32 AI138944. RR is supported by the National Center for Advancing Translational Sciences of the National Institutes of Health under Award Number TL1TR002647.
Conflict of Interest
The authors declare that the research was conducted in the absence of any commercial or financial relationships that could be construed as a potential conflict of interest.
References
1. World Health Organization. World Malaria Report 2019. Geneva: World Health Organization. (2019) Available at: https://www.who.int/publications/i/item/world-malaria-report-2019.
2. Draper SJ, Sack BK, King CR, Nielsen CM, Rayner JC, Higgins MK, et al. Malaria vaccines: recent advances and new horizons. Cell Host Microbe (2018) 24:43–56. doi: 10.1016/j.chom.2018.06.008
3. Cockburn IA, Seder RA. Malaria prevention: from immunological concepts to effective vaccines and protective antibodies. Nat Immunol (2018) 19:1199–211. doi: 10.1038/s41590-018-0228-6
4. Langhorne J, Ndungu FM, Sponaas AM, Marsh K. Immunity to malaria: more questions than answers. Nat Immunol (2008) 9:725–32. doi: 10.1038/ni.f.205
5. Camponovo F, Campo JJ, Le TQ, Oberai A, Hung C, Pablo JV, et al. Proteome-wide analysis of a malaria vaccine study reveals personalized humoral immune profiles in Tanzanian adults. Elife (2020) 9:e53080. doi: 10.7554/eLife.53080
6. Crompton PD, Kayala MA, Traore B, Kayentao K, Ongoiba A, Weiss GE, et al. A prospective analysis of the Ab response to Plasmodium falciparum before and after a malaria season by protein microarray. Proc Natl Acad Sci USA (2010) 107:6958–63. doi: 10.1073/pnas.1001323107
7. Dent AE, Nakajima R, Liang L, Baum E, Moormann AM, Sumba PO, et al. Plasmodium falciparum protein microarray antibody profiles correlate with protection from symptomatic malaria in Kenya. J Infect Dis (2015) 212:1429–38. doi: 10.1093/infdis/jiv224
8. Mu J, Awadalla P, Duan J, McGee KM, Keebler J, Seydel K, et al. Genome-wide variation and identification of vaccine targets in the Plasmodium falciparum genome. Nat Genet (2007) 39:126–30. doi: 10.1038/ng1924
9. Volkman SK, Sabeti PC, DeCaprio D, Neafsey DE, Schaffner SF, Milner DA Jr., et al. A genome-wide map of diversity in Plasmodium falciparum. Nat Genet (2007) 39:113–9. doi: 10.1038/ng1930
10. Murungi LM, Kamuyu G, Lowe B, Bejon P, Theisen M, Kinyanjui SM, et al. A threshold concentration of anti-merozoite antibodies is required for protection from clinical episodes of malaria. Vaccine (2013) 31:3936–42. doi: 10.1016/j.vaccine.2013.06.042
11. Stanisic DI, Fowkes FJ, Koinari M, Javati S, Lin E, Kiniboro B, et al. Acquisition of antibodies against Plasmodium falciparum merozoites and malaria immunity in young children and the influence of age, force of infection, and magnitude of response. Infect Immun (2015) 83:646–60. doi: 10.1128/IAI.02398-14
12. Yman V, White MT, Asghar M, Sundling C, Sonden K, Draper SJ, et al. Antibody responses to merozoite antigens after natural Plasmodium falciparum infection: kinetics and longevity in absence of re-exposure. BMC Med (2019) 17:22. doi: 10.1186/s12916-019-1255-3
13. Kinyanjui SM, Conway DJ, Lanar DE, Marsh K. IgG antibody responses to Plasmodium falciparum merozoite antigens in Kenyan children have a short half-life. Malar J (2007) 6:82. doi: 10.1186/1475-2875-6-82
14. Fowkes FJ, McGready R, Cross NJ, Hommel M, Simpson JA, Elliott SR, et al. New insights into acquisition, boosting, and longevity of immunity to malaria in pregnant women. J Infect Dis (2012) 206:1612–21. doi: 10.1093/infdis/jis566
15. White MT, Griffin JT, Akpogheneta O, Conway DJ, Koram KA, Riley EM, et al. Dynamics of the antibody response to Plasmodium falciparum infection in African children. J Infect Dis (2014) 210:1115–22. doi: 10.1093/infdis/jiu219
16. Baruch DI, Pasloske BL, Singh HB, Bi X, Ma XC, Feldman M, et al. Cloning the P. falciparum gene encoding PfEMP1, a malarial variant antigen and adherence receptor on the surface of parasitized human erythrocytes. Cell (1995) 82:77–87. doi: 10.1016/0092-8674(95)90054-3
17. Su XZ, Heatwole VM, Wertheimer SP, Guinet F, Herrfeldt JA, Peterson DS, et al. The large diverse gene family var encodes proteins involved in cytoadherence and antigenic variation of Plasmodium falciparum-infected erythrocytes. Cell (1995) 82:89–100. doi: 10.1016/0092-8674(95)90055-1
18. Smith JD, Chitnis CE, Craig AG, Roberts DJ, Hudson-Taylor DE, Peterson DS, et al. Switches in expression of Plasmodium falciparum var genes correlate with changes in antigenic and cytoadherent phenotypes of infected erythrocytes. Cell (1995) 82:101–10. doi: 10.1016/0092-8674(95)90056-X
19. Goel S, Palmkvist M, Moll K, Joannin N, Lara P, Akhouri RR, et al. RIFINs are adhesins implicated in severe Plasmodium falciparum malaria. Nat Med (2015) 21:314–7. doi: 10.1038/nm.3812
20. Niang M, Bei AK, Madnani KG, Pelly S, Dankwa S, Kanjee U, et al. STEVOR is a Plasmodium falciparum erythrocyte binding protein that mediates merozoite invasion and rosetting. Cell Host Microbe (2014) 16:81–93. doi: 10.1016/j.chom.2014.06.004
21. Rowe JA, Moulds JM, Newbold CI, Miller LH. P. falciparum rosetting mediated by a parasite-variant erythrocyte membrane protein and complement-receptor 1. Nature (1997) 388:292–5. doi: 10.1038/40888
22. Semblat JP, Ghumra A, Czajkowsky DM, Wallis R, Mitchell DA, Raza A, et al. Identification of the minimal binding region of a Plasmodium falciparum IgM binding PfEMP1 domain. Mol Biochem Parasitol (2015) 201:76–82. doi: 10.1016/j.molbiopara.2015.06.001
23. Wahlgren M, Goel S, Akhouri RR. Variant surface antigens of Plasmodium falciparum and their roles in severe malaria. Nat Rev Microbiol (2017) 15:479–91. doi: 10.1038/nrmicro.2017.47
24. David PH, Hommel M, Miller LH, Udeinya IJ, Oligino LD. Parasite sequestration in Plasmodium falciparum malaria: spleen and antibody modulation of cytoadherence of infected erythrocytes. Proc Natl Acad Sci USA (1983) 80:5075–9. doi: 10.1073/pnas.80.16.5075
25. Ghumra A, Khunrae P, Ataide R, Raza A, Rogerson SJ, Higgins MK, et al. Immunisation with recombinant PfEMP1 domains elicits functional rosette-inhibiting and phagocytosis-inducing antibodies to Plasmodium falciparum. PloS One (2011) 6:e16414. doi: 10.1371/journal.pone.0016414
26. Guillotte M, Nato F, Juillerat A, Hessel A, Marchand F, Lewit-Bentley A, et al. Functional analysis of monoclonal antibodies against the Plasmodium falciparum PfEMP1-VarO adhesin. Malar J (2016) 15:28. doi: 10.1186/s12936-015-1016-5
27. Chan JA, Boyle MJ, Moore KA, Reiling L, Lin Z, Hasang W, et al. Antibody targets on the surface of Plasmodium falciparum-infected erythrocytes that are associated with immunity to severe malaria in young children. J Infect Dis (2019) 219:819–28. doi: 10.1093/infdis/jiy580
28. Arora G, Hart GT, Manzella-Lapeira J, Doritchamou JY, Narum DL, Thomas LM, et al. NK cells inhibit Plasmodium falciparum growth in red blood cells via antibody-dependent cellular cytotoxicity. Elife (2018) 7:e53080. doi: 10.7554/eLife.36806
29. Deitsch KW, Dzikowski R. Variant gene expression and antigenic variation by malaria parasites. Annu Rev Microbiol (2017) 71:625–41. doi: 10.1146/annurev-micro-090816-093841
30. Larremore DB, Sundararaman SA, Liu W, Proto WR, Clauset A, Loy DE, et al. Ape parasite origins of human malaria virulence genes. Nat Commun (2015) 6:8368. doi: 10.1038/ncomms9368
31. Olsen RW, Ecklu-Mensah G, Bengtsson A, Ofori MF, Kusi KA, Koram KA, et al. Acquisition of IgG to ICAM-1-binding DBLbeta comains in the Plasmodium falciparum erythrocyte membrane protein 1 antigen family varies between groups A, B, and C. Infect Immun (2019) 87:e00224-19. doi: 10.1128/IAI.00224-19
32. Rask TS, Hansen DA, Theander TG, Gorm Pedersen A, Lavstsen T. Plasmodium falciparum erythrocyte membrane protein 1 diversity in seven genomes–divide and conquer. PloS Comput Biol (2010) 6:e1000933. doi: 10.1371/journal.pcbi.1000933
33. Avril M, Benjamin M, Dols MM, Smith JD. Interplay of Plasmodium falciparum and thrombin in brain endothelial barrier disruption. Sci Rep (2019) 9:13142. doi: 10.1038/s41598-019-49530-1
34. Turner GD, Morrison H, Jones M, Davis TM, Looareesuwan S, Buley ID, et al. An immunohistochemical study of the pathology of fatal malaria. Evidence for widespread endothelial activation and a potential role for intercellular adhesion molecule-1 in cerebral sequestration. Am J Pathol (1994) 145:1057–69.
35. Kessler A, Dankwa S, Bernabeu M, Harawa V, Danziger SA, Duffy F, et al. Linking EPCR-binding PfEMP1 to brain swelling in pediatric cerebral malaria. Cell Host Microbe (2017) 22:601–614 e5. doi: 10.1016/j.chom.2017.09.009
36. Lavstsen T, Turner L, Saguti F, Magistrado P, Rask TS, Jespersen JS, et al. Plasmodium falciparum erythrocyte membrane protein 1 domain cassettes 8 and 13 are associated with severe malaria in children. Proc Natl Acad Sci USA (2012) 109:E1791–800. doi: 10.1073/pnas.1120455109
37. Gupta S, Snow RW, Donnelly CA, Marsh K, Newbold C. Immunity to non-cerebral severe malaria is acquired after one or two infections. Nat Med (1999) 5:340–3. doi: 10.1038/6560
38. Kanoi BN, Nagaoka H, Morita M, White MT, Palacpac NMQ, Ntege EH, et al. Comprehensive analysis of antibody responses to Plasmodium falciparum erythrocyte membrane protein 1 domains. Vaccine (2018) 36:6826–33. doi: 10.1016/j.vaccine.2018.08.058
39. Duffy MF, Noviyanti R, Tsuboi T, Feng ZP, Trianty L, Sebayang BF, et al. Differences in PfEMP1s recognized by antibodies from patients with uncomplicated or severe malaria. Malar J (2016) 15:258. doi: 10.1186/s12936-016-1296-4
40. Tessema SK, Nakajima R, Jasinskas A, Monk SL, Lekieffre L, Lin E, et al. Protective immunity against severe malaria in children is associated with a limited repertoire of antibodies to conserved PfEMP1 variants. Cell Host Microbe (2019) 26:579–590 e5. doi: 10.1016/j.chom.2019.10.012
41. Travassos MA, Niangaly A, Bailey JA, Ouattara A, Coulibaly D, Lyke KE, et al. Children with cerebral malaria or severe malarial anaemia lack immunity to distinct variant surface antigen subsets. Sci Rep (2018) 8:6281. doi: 10.1038/s41598-018-24462-4
42. Tuju J, Mackinnon MJ, Abdi AI, Karanja H, Musyoki JN, Warimwe GM, et al. Antigenic cartography of immune responses to Plasmodium falciparum erythrocyte membrane protein 1 (PfEMP1). PloS Pathog (2019) 15:e1007870. doi: 10.1371/journal.ppat.1007870
43. Jespersen JS, Wang CW, Mkumbaye SI, Minja DT, Petersen B, Turner L, et al. Plasmodium falciparum var genes expressed in children with severe malaria encode CIDRalpha1 domains. EMBO Mol Med (2016) 8:839–50. doi: 10.15252/emmm.201606188
44. Mkumbaye SI, Wang CW, Lyimo E, Jespersen JS, Manjurano A, Mosha J, et al. The severity of Plasmodium falciparum infection is associated with transcript levels of var genes encoding endothelial protein C receptor-binding P. falciparum erythrocyte membrane protein 1. Infect Immun (2017) 85:e00841-16. doi: 10.1128/IAI.00841-16
45. Rambhatla JS, Turner L, Manning L, Laman M, Davis TME, Beeson JG, et al. Acquisition of antibodies against endothelial protein C receptor-binding domains of Plasmodium falciparum erythrocyte membrane protein 1 in children with severe malaria. J Infect Dis (2019) 219:808–18. doi: 10.1093/infdis/jiy564
46. Obeng-Adjei N, Larremore DB, Turner L, Ongoiba A, Li S, Doumbo S, et al. Longitudinal analysis of naturally acquired PfEMP1 CIDR domain variant antibodies identifies associations with malaria protection. JCI Insight (2020) 5:e137262. doi: 10.1172/jci.insight.137262
47. Turner L, Lavstsen T, Mmbando BP, Wang CW, Magistrado PA, Vestergaard LS, et al. IgG antibodies to endothelial protein C receptor-binding cysteine-rich interdomain region domains of Plasmodium falciparum erythrocyte membrane protein 1 are acquired early in life in individuals exposed to malaria. Infect Immun (2015) 83:3096–103. doi: 10.1128/IAI.00271-15
48. Lau CK, Turner L, Jespersen JS, Lowe ED, Petersen B, Wang CW, et al. Structural conservation despite huge sequence diversity allows EPCR binding by the PfEMP1 family implicated in severe childhood malaria. Cell Host Microbe (2015) 17:118–29. doi: 10.1016/j.chom.2014.11.007
49. Fernandez V, Hommel M, Chen Q, Hagblom P, Wahlgren M. Small, clonally variant antigens expressed on the surface of the Plasmodium falciparum-infected erythrocyte are encoded by the rif gene family and are the target of human immune responses. J Exp Med (1999) 190:1393–404. doi: 10.1084/jem.190.10.1393
50. Saito F, Hirayasu K, Satoh T, Wang CW, Lusingu J, Arimori T, et al. Corrigendum: Immune evasion of Plasmodium falciparum by RIFIN via inhibitory receptors. Nature (2018) 554:554. doi: 10.1038/nature25498
51. Meyaard L, Adema GJ, Chang C, Woollatt E, Sutherland GR, Lanier LL, et al. LAIR-1, a novel inhibitory receptor expressed on human mononuclear leukocytes. Immunity (1997) 7:283–90. doi: 10.1016/S1074-7613(00)80530-0
52. Naji A, Menier C, Morandi F, Agaugue S, Maki G, Ferretti E, et al. Binding of HLA-G to ITIM-bearing Ig-like transcript 2 receptor suppresses B cell responses. J Immunol (2014) 192:1536–46. doi: 10.4049/jimmunol.1300438
53. van der Vuurst de Vries AR, Clevers H, Logtenberg T, Meyaard L. Leukocyte-associated immunoglobulin-like receptor-1 (LAIR-1) is differentially expressed during human B cell differentiation and inhibits B cell receptor-mediated signaling. Eur J Immunol (1999) 29:3160–7. doi: 10.1002/(SICI)1521-4141(199910)29:10<3160::AID-IMMU3160>3.0.CO;2-S
54. Harrison TE, Morch AM, Felce JH, Sakoguchi A, Reid AJ, Arase H, et al. Structural basis for RIFIN-mediated activation of LILRB1 in malaria. Nature (2020). doi: 10.1038/s41586-020-2530-3
55. Saito F, Hirayasu K, Satoh T, Wang CW, Lusingu J, Arimori T, et al. Immune evasion of Plasmodium falciparum by RIFIN via inhibitory receptors. Nature (2017) 552:101–5. doi: 10.1038/nature24994
56. Pieper K, Tan J, Piccoli L, Foglierini M, Barbieri S, Chen Y, et al. Public antibodies to malaria antigens generated by two LAIR1 insertion modalities. Nature (2017) 548:597–601. doi: 10.1038/nature23670
57. Tan J, Pieper K, Piccoli L, Abdi A, Perez MF, Geiger R, et al. A LAIR1 insertion generates broadly reactive antibodies against malaria variant antigens. Nature (2016) 529:105–9. doi: 10.1038/nature16450
58. Zhou AE, Berry AA, Bailey JA, Pike A, Dara A, Agrawal S, et al. Antibodies to peptides in semiconserved domains of RIFINs and STEVORs correlate with malaria exposure. mSphere (2019) 4:e00097-19. doi: 10.1128/mSphere.00097-19
59. Petter M, Haeggstrom M, Khattab A, Fernandez V, Klinkert MQ, Wahlgren M. Variant proteins of the Plasmodium falciparum RIFIN family show distinct subcellular localization and developmental expression patterns. Mol Biochem Parasitol (2007) 156:51–61. doi: 10.1016/j.molbiopara.2007.07.011
60. Chan JA, Howell KB, Reiling L, Ataide R, Mackintosh CL, Fowkes FJ, et al. Targets of antibodies against Plasmodium falciparum-infected erythrocytes in malaria immunity. J Clin Invest (2012) 122:3227–38. doi: 10.1172/JCI62182
61. Limpaiboon T, Shirley MW, Kemp DJ, Saul A. 7H8/6, a multicopy DNA probe for distinguishing isolates of Plasmodium falciparum. Mol Biochem Parasitol (1991) 47:197–206. doi: 10.1016/0166-6851(91)90179-A
62. Wichers JS, Scholz JAM, Strauss J, Witt S, Lill A, Ehnold LI, et al. Dissecting the gene expression, localization, membrane topology, and function of the Plasmodium falciparum STEVOR protein family. mBio (2019) 10:e01500-19. doi: 10.1128/mBio.01500-19
63. McRobert L, Preiser P, Sharp S, Jarra W, Kaviratne M, Taylor MC, et al. Distinct trafficking and localization of STEVOR proteins in three stages of the Plasmodium falciparum life cycle. Infect Immun (2004) 72:6597–602. doi: 10.1128/IAI.72.11.6597-6602.2004
64. Lee WC, Russell B, Renia L. Sticking for a cause: the falciparum malaria parasites cytoadherence paradigm. Front Immunol (2019) 10:1444. doi: 10.3389/fimmu.2019.01444
65. Nilsson SK, Childs LM, Buckee C, Marti M. Targeting human transmission biology for malaria elimination. PloS Pathog (2015) 11:e1004871. doi: 10.1371/journal.ppat.1004871
66. Blythe JE, Yam XY, Kuss C, Bozdech Z, Holder AA, Marsh K, et al. Plasmodium falciparum STEVOR proteins are highly expressed in patient isolates and located in the surface membranes of infected red blood cells and the apical tips of merozoites. Infect Immun (2008) 76:3329–36. doi: 10.1128/IAI.01460-07
67. Singh H, Madnani K, Lim YB, Cao J, Preiser PR, Lim CT. Expression dynamics and physiologically relevant functional study of STEVOR in asexual stages of Plasmodium falciparum infection. Cell Microbiol (2017) 19:e12715. doi: 10.1111/cmi.12715
68. Kanoi BN, Nagaoka H, White MT, Morita M, Palacpac NMQ, Ntege EH, et al. Global repertoire of human antibodies against Plasmodium falciparum RIFINs, SURFINs, and STEVORs in a malaria exposed population. Front Immunol (2020) 11:893. doi: 10.3389/fimmu.2020.00893
69. Lavazec C, Sanyal S, Templeton TJ. Hypervariability within the Rifin, Stevor and Pfmc-2TM superfamilies in Plasmodium falciparum. Nucleic Acids Res (2006) 34:6696–707. doi: 10.1093/nar/gkl942
70. Cowman AF, Tonkin CJ, Tham WH, Duraisingh MT. The molecular basis of erythrocyte invasion by malaria parasites. Cell Host Microbe (2017) 22:232–45. doi: 10.1016/j.chom.2017.07.003
71. Beeson JG, Kurtovic L, Dobano C, Opi DH, Chan JA, Feng G, et al. Challenges and strategies for developing efficacious and long-lasting malaria vaccines. Sci Transl Med (2019) 11:eaau1458. doi: 10.1126/scitranslmed.aau1458
72. King CL, Davies DH, Felgner P, Baum E, Jain A, Randall A, et al. Biosignatures of Exposure/Transmission and Immunity. Am J Trop Med Hyg (2015) 93:16–27. doi: 10.4269/ajtmh.15-0037
73. Kobayashi T, Jain A, Liang L, Obiero JM, Hamapumbu H, Stevenson JC, et al. Distinct antibody signatures associated with different malaria transmission intensities in Zambia and Zimbabwe. mSphere (2019) 4:e00061-19. doi: 10.1128/mSphereDirect.00061-19
74. Reiling L, Boyle MJ, White MT, Wilson DW, Feng G, Weaver R, et al. Targets of complement-fixing antibodies in protective immunity against malaria in children. Nat Commun (2019) 10:610. doi: 10.1038/s41467-019-08528-z
75. Richards JS, Arumugam TU, Reiling L, Healer J, Hodder AN, Fowkes FJ, et al. Identification and prioritization of merozoite antigens as targets of protective human immunity to Plasmodium falciparum malaria for vaccine and biomarker development. J Immunol (2013) 191:795–809. doi: 10.4049/jimmunol.1300778
76. Proietti C, Krause L, Trieu A, Dodoo D, Gyan B, Koram KA, et al. Immune signature against Plasmodium falciparum antigens predicts clinical immunity in distinct malaria endemic communities. Mol Cell Proteomics (2020) 19:101–13. doi: 10.1074/mcp.RA118.001256
77. Valletta JJ, Recker M. Identification of immune signatures predictive of clinical protection from malaria. PloS Comput Biol (2017) 13:e1005812. doi: 10.1371/journal.pcbi.1005812
78. Guevara Patino JA, Holder AA, McBride JS, Blackman MJ. Antibodies that inhibit malaria merozoite surface protein-1 processing and erythrocyte invasion are blocked by naturally acquired human antibodies. J Exp Med (1997) 186:1689–99. doi: 10.1084/jem.186.10.1689
79. Bustamante LY, Powell GT, Lin YC, Macklin MD, Cross N, Kemp A, et al. Synergistic malaria vaccine combinations identified by systematic antigen screening. Proc Natl Acad Sci USA (2017) 114:12045–50. doi: 10.1073/pnas.1702944114
80. Garcia-Senosiain A, Kana IH, Singh SK, Chourasia BK, Das MK, Dodoo D, et al. Peripheral merozoite surface proteins are targets of naturally acquired immunity against malaria in both India and Ghana. Infect Immun (2020) 88:e00778-19. doi: 10.1128/IAI.00778-19
81. Kana IH, Garcia-Senosiain A, Singh SK, Tiendrebeogo RW, Chourasia BK, Malhotra P, et al. Cytophilic antibodies against key Plasmodium falciparum blood stage antigens contribute to protection against clinical malaria in a high transmission region of eastern India. J Infect Dis (2018) 218:956–65. doi: 10.1093/infdis/jiy258
82. Osier FH, Mackinnon MJ, Crosnier C, Fegan G, Kamuyu G, Wanaguru M, et al. New antigens for a multicomponent blood-stage malaria vaccine. Sci Transl Med (2014) 6:247ra102. doi: 10.1126/scitranslmed.3008705
83. Cohen S, Mc GI, Carrington S. Gamma-globulin and acquired immunity to human malaria. Nature (1961) 192:733–7. doi: 10.1038/192733a0
84. Sabchareon A, Burnouf T, Ouattara D, Attanath P, Bouharoun-Tayoun H, Chantavanich P, et al. Parasitologic and clinical human response to immunoglobulin administration in falciparum malaria. Am J Trop Med Hyg (1991) 45:297–308. doi: 10.4269/ajtmh.1991.45.297
85. Hill DL, Wilson DW, Sampaio NG, Eriksson EM, Ryg-Cornejo V, Harrison GLA, et al. Merozoite antigens of Plasmodium falciparum elicit strain-transcending opsonizing immunity. Infect Immun (2016) 84:2175–84. doi: 10.1128/IAI.00145-16
86. Irani V, Ramsland PA, Guy AJ, Siba PM, Mueller I, Richards JS, et al. Acquisition of functional antibodies that block the binding of Erythrocyte-Binding Antigen 175 and protection against Plasmodium falciparum malaria in children. Clin Infect Dis (2015) 61:1244–52. doi: 10.1093/cid/civ525
87. Douglas AD, Baldeviano GC, Jin J, Miura K, Diouf A, Zenonos ZA, et al. A defined mechanistic correlate of protection against Plasmodium falciparum malaria in non-human primates. Nat Commun (2019) 10:1953. doi: 10.1038/s41467-019-09894-4
88. Osier FH, Feng G, Boyle MJ, Langer C, Zhou J, Richards JS, et al. Opsonic phagocytosis of Plasmodium falciparum merozoites: mechanism in human immunity and a correlate of protection against malaria. BMC Med (2014) 12:108. doi: 10.1186/1741-7015-12-108
89. Jaschke A, Coulibaly B, Remarque EJ, Bujard H, Epp C. Merozoite Surface Protein 1 from Plasmodium falciparum is a major target of opsonizing antibodies in individuals with acquired immunity against malaria. Clin Vaccine Immunol (2017) 24:e00155-17. doi: 10.1128/CVI.00155-17
90. Kana IH, Singh SK, Garcia-Senosiain A, Dodoo D, Singh S, Adu B, et al. Breadth of functional antibodies is associated with Plasmodium falciparum merozoite phagocytosis and protection against febrile malaria. J Infect Dis (2019) 220:275–84. doi: 10.1093/infdis/jiz088
91. Boyle MJ, Reiling L, Feng G, Langer C, Osier FH, Aspeling-Jones H, et al. Human antibodies fix complement to inhibit Plasmodium falciparum invasion of erythrocytes and are associated with protection against malaria. Immunity (2015) 42:580–90. doi: 10.1016/j.immuni.2015.02.012
92. Murungi LM, Sonden K, Llewellyn D, Rono J, Guleid F, Williams AR, et al. Targets and mechanisms associated with protection from severe Plasmodium falciparum malaria in Kenyan children. Infect Immun (2016) 84:950–63. doi: 10.1128/IAI.01120-15
93. Zuccala ES, Gout AM, Dekiwadia C, Marapana DS, Angrisano F, Turnbull L, et al. Subcompartmentalisation of proteins in the rhoptries correlates with ordered events of erythrocyte invasion by the blood stage malaria parasite. PloS One (2012) 7:e46160. doi: 10.1371/journal.pone.0046160
94. Kats LM, Black CG, Proellocks NI, Coppel RL. Plasmodium rhoptries: how things went pear-shaped. Trends Parasitol (2006) 22:269–76. doi: 10.1016/j.pt.2006.04.001
95. Alanine DGW, Quinkert D, Kumarasingha R, Mehmood S, Donnellan FR, Minkah NK, et al. Human antibodies that slow erythrocyte invasion potentiate malaria-neutralizing antibodies. Cell (2019) 178:216–228 e21. doi: 10.1016/j.cell.2019.05.025
96. Blank A, Furle K, Jaschke A, Mikus G, Lehmann M, Husing J, et al. Immunization with full-length Plasmodium falciparum merozoite surface protein 1 is safe and elicits functional cytophilic antibodies in a randomized first-in-human trial. NPJ Vaccines (2020) 5:10. doi: 10.1038/s41541-020-0160-2
97. Arama C, Skinner J, Doumtabe D, Portugal S, Tran TM, Jain A, et al. Genetic resistance to malaria is associated with greater enhancement of Immunoglobulin (Ig)M than IgG responses to a broad array of Plasmodium falciparum antigens. Open Forum Infect Dis (2015) 2:ofv118. doi: 10.1093/ofid/ofv118
98. Boyle MJ, Chan JA, Handayuni I, Reiling L, Feng G, Hilton A, et al. IgM in human immunity to Plasmodium falciparum malaria. Sci Adv (2019) 5:eaax4489. doi: 10.1126/sciadv.aax4489
99. Krishnamurty AT, Thouvenel CD, Portugal S, Keitany GJ, Kim KS, Holder A, et al. Somatically hypermutated Plasmodium-specific IgM(+) memory B cells are rapid, plastic, early responders upon malaria rechallenge. Immunity (2016) 45:402–14. doi: 10.1016/j.immuni.2016.06.014
100. Dolo A, Modiano D, Maiga B, Daou M, Dolo G, Guindo H, et al. Difference in susceptibility to malaria between two sympatric ethnic groups in Mali. Am J Trop Med Hyg (2005) 72:243–8. doi: 10.4269/ajtmh.2005.72.243
101. Modiano D, Luoni G, Sirima BS, Lanfrancotti A, Petrarca V, Cruciani F, et al. The lower susceptibility to Plasmodium falciparum malaria of Fulani of Burkina Faso (west Africa) is associated with low frequencies of classic malaria-resistance genes. Trans R Soc Trop Med Hyg (2001) 95:149–52. doi: 10.1016/S0035-9203(01)90141-5
102. Maiga B, Dolo A, Toure O, Dara V, Tapily A, Campino S, et al. Human candidate polymorphisms in sympatric ethnic groups differing in malaria susceptibility in Mali. PloS One (2013) 8:e75675. doi: 10.1371/journal.pone.0075675
103. Quin JE, Bujila I, Cherif M, Sanou GS, Qu Y, Vafa Homann M, et al. Major transcriptional changes observed in the Fulani, an ethnic group less susceptible to malaria. Elife (2017) 6:e29156. doi: 10.7554/eLife.29156
104. Portugal S, Doumtabe D, Traore B, Miller LH, Troye-Blomberg M, Doumbo OK, et al. B cell analysis of ethnic groups in Mali with differential susceptibility to malaria. Malar J (2012) 11:162. doi: 10.1186/1475-2875-11-162
105. McCallum FJ, Persson KE, Fowkes FJ, Reiling L, Mugyenyi CK, Richards JS, et al. Differing rates of antibody acquisition to merozoite antigens in malaria: implications for immunity and surveillance. J Leukoc Biol (2017) 101:913–25. doi: 10.1189/jlb.5MA0716-294R
106. Liu EW, Skinner J, Tran TM, Kumar K, Narum DL, Jain A, et al. Protein-specific features associated with variability in human antibody responses to Plasmodium falciparum malaria antigens. Am J Trop Med Hyg (2018) 98:57–66. doi: 10.4269/ajtmh.17-0437
107. Chan JA, Stanisic DI, Duffy MF, Robinson LJ, Lin E, Kazura JW, et al. Patterns of protective associations differ for antibodies to P. falciparum-infected erythrocytes and merozoites in immunity against malaria in children. Eur J Immunol (2017) 47:2124–36. doi: 10.1002/eji.201747032
108. Obeng-Adjei N, Portugal S, Tran TM, Yazew TB, Skinner J, Li S, et al. Circulating Th1-cell-type Tfh cells that exhibit impaired B cell help are preferentially activated during acute malaria in children. Cell Rep (2015) 13:425–39. doi: 10.1016/j.celrep.2015.09.004
109. Ryg-Cornejo V, Ioannidis LJ, Ly A, Chiu CY, Tellier J, Hill DL, et al. Severe malaria infections impair germinal center responses by inhibiting T follicular helper cell differentiation. Cell Rep (2016) 14:68–81. doi: 10.1016/j.celrep.2015.12.006
110. Weiss GE, Crompton PD, Li S, Walsh LA, Moir S, Traore B, et al. Atypical memory B cells are greatly expanded in individuals living in a malaria-endemic area. J Immunol (2009) 183:2176–82. doi: 10.4049/jimmunol.0901297
111. Obeng-Adjei N, Portugal S, Holla P, Li S, Sohn H, Ambegaonkar A, et al. Malaria-induced interferon-gamma drives the expansion of Tbethi atypical memory B cells. PloS Pathog (2017) 13:e1006576. doi: 10.1371/journal.ppat.1006576
112. Portugal S, Obeng-Adjei N, Moir S, Crompton PD, Pierce SK. Atypical memory B cells in human chronic infectious diseases: An interim report. Cell Immunol (2017) 321:18–25. doi: 10.1016/j.cellimm.2017.07.003
113. Braddom A, Batugedara G, Bol S, Bunnik E. Potential functions of atypical memory B cells in Plasmodiumexposed individuals. Int J Parasitol (2020). (In press). doi: 10.1016/j.ijpara.2020.08.003
114. Portugal S, Tipton CM, Sohn H, Kone Y, Wang J, Li S, et al. Malaria-associated atypical memory B cells exhibit markedly reduced B cell receptor signaling and effector function. Elife (2015) 4:e07218. doi: 10.7554/eLife.07218
115. Sullivan RT, Kim CC, Fontana MF, Feeney ME, Jagannathan P, Boyle MJ, et al. FCRL5 delineates functionally impaired memory B cells associated with Plasmodium falciparum exposure. PloS Pathog (2015) 11:e1004894. doi: 10.1371/journal.ppat.1004894
116. Muellenbeck MF, Ueberheide B, Amulic B, Epp A, Fenyo D, Busse CE, et al. Atypical and classical memory B cells produce Plasmodium falciparum neutralizing antibodies. J Exp Med (2013) 210:389–99. doi: 10.1084/jem.20121970
117. Ambegaonkar AA, Kwak K, Sohn H, Manzella-Lapeira J, Brzostowski J, Pierce SK. Expression of inhibitory receptors by B cells in chronic human infectious diseases restricts responses to membrane-associated antigens. Sci Adv (2020) 6:eaba6493. doi: 10.1126/sciadv.aba6493
118. Holla P, Ambegaonkar A, Sohn H, Pierce SK. Exhaustion may not be in the human B cell vocabulary, at least not in malaria. Immunol Rev (2019) 292:139–48. doi: 10.1111/imr.12809
119. Vijay R, Guthmiller JJ, Sturtz AJ, Surette FA, Rogers KJ, Sompallae RR, et al. Infection-induced plasmablasts are a nutrient sink that impairs humoral immunity to malaria. Nat Immunol (2020) 21:790–801. doi: 10.1038/s41590-020-0678-5
120. Wu X, Zhou T, Zhu J, Zhang B, Georgiev I, Wang C, et al. Focused evolution of HIV-1 neutralizing antibodies revealed by structures and deep sequencing. Science (2011) 333:1593–602. doi: 10.1126/science.1207532
121. Klein F, Diskin R, Scheid JF, Gaebler C, Mouquet H, Georgiev IS, et al. Somatic mutations of the immunoglobulin framework are generally required for broad and potent HIV-1 neutralization. Cell (2013) 153:126–38. doi: 10.1016/j.cell.2013.03.018
122. Liao HX, Lynch R, Zhou T, Gao F, Alam SM, Boyd SD, et al. Co-evolution of a broadly neutralizing HIV-1 antibody and founder virus. Nature (2013) 496:469–76. doi: 10.1038/nature12053
123. Murugan R, Buchauer L, Triller G, Kreschel C, Costa G, Pidelaserra Marti G, et al. Clonal selection drives protective memory B cell responses in controlled human malaria infection. Sci Immunol (2018) 3:eaap8029. doi: 10.1126/sciimmunol.aap8029
Keywords: antibody, protection, variant surface antigens, PfEMP1, merozoite, vaccine
Citation: Gonzales SJ, Reyes RA, Braddom AE, Batugedara G, Bol S and Bunnik EM (2020) Naturally Acquired Humoral Immunity Against Plasmodium falciparum Malaria. Front. Immunol. 11:594653. doi: 10.3389/fimmu.2020.594653
Received: 14 August 2020; Accepted: 07 October 2020;
Published: 29 October 2020.
Edited by:
Mark Robin Boothby, Vanderbilt University Medical Center, United StatesReviewed by:
Christopher Sundling, Karolinska Institutet (KI), SwedenJulius Lautenbach, in collaboration with reviewer CS
Wanli Liu, Tsinghua University, China
Copyright © 2020 Gonzales, Reyes, Braddom, Batugedara, Bol and Bunnik. This is an open-access article distributed under the terms of the Creative Commons Attribution License (CC BY). The use, distribution or reproduction in other forums is permitted, provided the original author(s) and the copyright owner(s) are credited and that the original publication in this journal is cited, in accordance with accepted academic practice. No use, distribution or reproduction is permitted which does not comply with these terms.
*Correspondence: Evelien M. Bunnik, YnVubmlrQHV0aHNjc2EuZWR1
†These authors have contributed equally to this work