- 1Centre for Ecological and Evolutionary Synthesis (CEES), Department of Biosciences, University of Oslo, Oslo, Norway
- 2Institute of Aquaculture, University of Stirling, Stirling, United Kingdom
- 3College of Oceanography, Hohai University, Nanjing, China
The absence of MHC class II antigen presentation and multiple pathogen recognition receptors in the Atlantic cod has not impaired its immune response however how underlying mechanisms have adapted remains largely unknown. In this study, ex vivo cod macrophages were challenged with various bacterial and viral microbe-associated molecular patterns (MAMP) to identify major response pathways. Cytosolic MAMP-PRR pathways based upon the NOD-like receptors (NLRs) and RIG-I-like receptors (RLRs) were identified as the critical response pathways. Our analyses suggest that internalization of exogenous ligands through scavenger receptors drives both pathways activating transcription factors like NF-kB (Nuclear factor-kappa B) and interferon regulatory factors (IRFs). Further, ligand-dependent differential expression of a unique TLR25 isoform and multiple NLR paralogues suggests (sub)neofunctionalization toward specific immune defensive strategies. Our results further demonstrate that the unique immune system of the Atlantic cod provides an unprecedented opportunity to explore the evolutionary history of PRR-based signaling in vertebrate immunity.
Introduction
In vertebrates, the genetic basis of immunity is considered highly conserved (1). With the increased use of high-throughput sequencing technologies, genomic resources from non-model species has become readily available and large efforts in comparative immunology has ensued (2–4). These studies have revealed several common denominators of vertebrate immunity, but also demonstrated considerable gene losses and expansions challenging our understanding of immune system organization and functional compartmentalization in vertebrates. The genome sequencing of the Atlantic cod (5), and later a set of ~30 Gadiform genomes (2), demonstrated that this group of teleosts display a very distinct immune gene repertoire affecting both innate and adaptive immunity, with the lack major histocompatibility complex (MHC) class II, invariant chain (CD74) and CD4. All hallmarks of the perceived classical vertebrate adaptive immune system (2, 5). In parallel, teleosts display a wide range of gene expansions/contractions related to major histocompatibility complex class I as well as innate immune gene families such as pattern recognition receptors (PRRs) (6–8). PRRs are a strongly conserved feature of the immune system indispensable from insects to mammals and in plants (R protein) (1, 9). Classically, these receptors are associated with detecting microbe-associated or danger-associated molecular patterns (MAMPS and DAMPS) with subsequent initiation of inflammation. However, they have also been implicated in regulation of development, antigen presentation and autophagy (10, 11).
Genomic investigations have provided detailed characterizations of all known PRR families including Toll-like receptors (TLRs), C-type lectin receptors (CLRs), retinoic acid-inducible (RIG)-I-like receptors (RLRs), and nucleotide-binding oligomerization (NOD)-like receptors (NLRs) in an array of vertebrates (9). The PRR repertoire in teleost fish has proven to be very diverse compared to other vertebrate groups, and more so within the Gadiformes (12, 13). All homologs of mammalian surface-located TLRs have been lost, whereas there are large expansions of teleost-specific TLRs with unknown cellular location and ligand type. Furthermore, loss of RIG-I and NOD2 has been reported in parallel to the overall NLR repertoire being greatly expanded (5, 7, 12–14). These significant gene losses and expansions result in a peculiar genetic basis for innate immunity that suggests the existence of an alternative MAMP-PRR activation system. The functional implication of the PRR repertoire observed in Gadiformes is poorly understood. Furthermore, there is a lack of functional investigations targeting PRR interaction and signaling. So far, complete insight into these gene losses and expansions, and the expression pattern of the various paralogs, have been hampered by a fragmented genome assembly and the use of short-read technology in, e.g., RNA-Seq analyses.
Certain PRR-encoding gene family groups such as the NLRs appear to contain species-specific expansions that present multiple modified forms (neo/sub-functionalization) and different genomic organizational patterns (tandem/dispersed). Atlantic cod and other gadiform species contain a high number of tandem-repeated gene families that cause assembly collapse, as a consequence PRR genes are often fragmented or collapsed (7, 12, 15). Additionally, some of the Gadiformes PRRs demonstrate significant gene expansions and neofunctionalization (3, 16). In the Atlantic cod genome, genes from both the TLR and NLR families were found to be highly expanded, accompanied by a loss of several other crucial PRRs including certain cell membrane-bound TLRs (TLR1, TLR2, TLR4, and TLR5), RIG-I, and NOD2 (5, 7, 12–14). This significant gene loss suggests that the intracellular mechanisms in mediating MAMP-PRR activation are likely of extra necessity and importance (5, 13). However, the underlying mechanisms of this process are not fully understood. In this sense, short-read deep sequencing like Illumina RNA-Seq have produced extensive atlases of transcriptomes. In addition, long-read sequencing such as PacBio (yielding reads with average > 15 kb, up to 100 kb or even longer), have proven able to generate high contiguous long reads spanning repeats, and is progressively used in genome assembly and full-length transcriptomics (7, 14, 17, 18). Therefore, integrating both the short- and long-read sequencing approaches would ensure a more precise point-to-point interpretation of the response to immune challenges, especially in organisms with highly complex expanded gene families like Atlantic cod.
Here, we have used Atlantic cod macrophages to further elucidate the functional outcome of the Gadiformes PRR repertoire. Macrophages play a pivotal role in host defense, both phagocytizing non-self-agents and orchestrating subsequent innate and adaptive immune responses (19–21). As a consequence, tissue macrophages perform a critical immune surveillance role with their befitting diversity of PRRs (21). Ex-vivo Atlantic cod macrophage cultures were established and challenged with a set of MAMPs mimicking bacterial and viral infections. In addition, a set of well-known pharmacological signaling pathway blockers were used to further unravel cod PRR signaling pathways. Finally, using long-read sequencing technology, a comparison between the predicted transcriptome and the actual macrophage transcriptome was performed. We find that Atlantic cod macrophages efficiently engulf microbes, produce inflammatory mediators and generate a MAMP-specific immune response that agrees with the overall vertebrate models. Sensing of bacterial ultra-pure peptidoglycan uPGN (containing MDP, muramyl dipeptide, and iE-DAP, γ-D-Glu-mDAP), and of the viral mimic dsRNA is mediated by the NLR and RLR pathways respectively. Comparative analyses demonstrate a role for the internalization for exogenous ligand through scavenger receptors for MAMP-PRR interactions. Intracellular pathways identified through inhibition highlights a central role of TBK1 (TANK Binding Kinase 1) in signal coordination for NOD-based signaling. Our findings demonstrate that Atlantic cod appears to rely more on cytosolic MAMP sensing using NLR and RLR-based signaling supported by neo-functionalized PRRs.
Material and Methods
Samples
One-year old Atlantic cod, Gadus morhua L., reared in land-based tanks supplied with filtered sea water were obtained from Ardtoe Marine Facility (UK) during 2014-2015. They were kept at environmental temperature and fed on commercial dry pellets once a day. All procedures were in accordance with UK Home Office welfare guidelines. The fish were sacrificed with UK Home Office approved methods, using an overdose of the anesthetic benzocaine (Sigma-Aldrich) followed by brain concussion. The head kidney and spleen of each animal were dissected out and kept at 4°C in high glucose Dulbecco’s Modified Eagle Medium (DMEM) (Sigma-Aldrich) and 0.2% Primocin™ (InvivoGen) until cell culture.
Macrophage Cell Culture
The sampled tissues were ground together through a 100 μm cell strainer (Fisher Scientific) in a proportion of 1:3 spleen to head kidney into a tube with fresh DMEM solution with 0.2% Primocin. Cells were precipitated by centrifugation at 1500 rpm for 5 min and resuspended in DMEM (0.2% Primocin and 10% chicken serum (Life Technologies)). The cell culture plates were coated with 0.5 ml of poly-D-lysine (Sigma-Aldrich) (0.1 mg/ml in no calcium, no magnesium Dulbecco’s Phosphate-Buffered Saline (DPBS) (Life Technologies)) per well for 30 min, rinsed with 1.5 ml of DPBS per well, and left to be air-dried prior seeding. Twelve-welled plates (Thermo Scientific) were filled with 1 ml per well of the resuspended cells (which contained approximately 2 million cells per ml). The cell cultures were kept in an incubator at 15°C with 4% CO2 supply. After one day and thereafter every two days (5 days in total), half of the medium per well was replaced with fresh DMEM (0.2% Primocin and 10% chicken serum from the same batch).
Phagocytosis Assay
Alexa fluor 488 labelled bacteria (E. coli, Sigma-Aldrich) and yeast (S. cerevisiae, Sigma-Aldrich) were used as phagocytosis targets. After 1 h and 3 h of incubation with bacteria and yeast respectively, macrophages from four individuals were analyzed using Guava® easyCyte™ 8HT Flow Cytometer (Merck Millipore) to measure phagocytosis. Flow cytometry assessment of the cell population demonstrated homogeneity (92%) and was gated for further functional assays (gate R1 in Figures 1A, B).
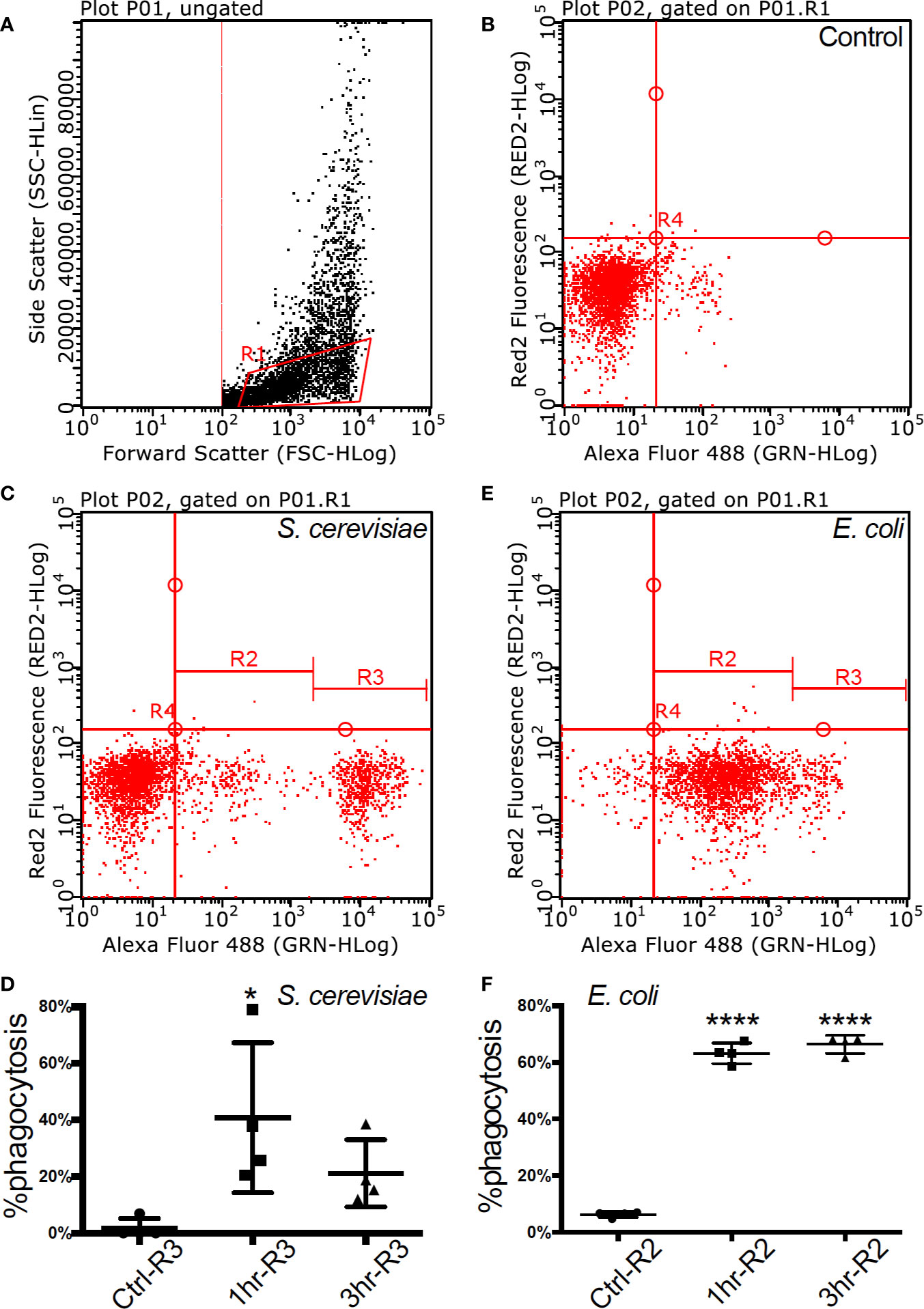
Figure 1 Flow cytometry of fully functional Atlantic cod macrophages during phagocytosis (A). Scatter plot of cell complexity (y-axis) and size (x-axis) that shows the presence of two cell subpopulations: one of morphologically similar cells (R1) and another one of heterogeneous cells with regards to both parameters (B–D). The first subpopulation was gated, and it is shown in an untreated resting state (B), during phagocytosis of yeast (C), and during phagocytosis of bacteria (D), (C, D). Macrophage abundance by size during an untreated resting state, after a 1-h and after a 3-h activation of yeast (C) and bacteria (D). Asterisks represent statistical significance: * < 0.05, **** < 0.0001.
Microbe-Associated Molecular Patterns Immune Challenge
One hour prior to stimulation, the cell culture medium was replaced with serum-free DMEM, eliminating the possible interference of serum with the assay. Ten µl of 1mg/ml stock solutions of MAMPs (i.e., Lipopolysaccharide (LPS; LPS-EK; E.coli), Peptidoglycan (PGN; PGN-EK; E.coli), ultra-pure peptidoglycan (uPGN; PGN-E.coli ndss ultrapure), dsRNA (High Molecular Weight, HMW) and CpG [E. coli ssDNA/LyoVec)] per ml was used in all cases to challenge the cells for 3h and 12h (Table S6). All MAMPs were purchased from Invivogen.
Inhibition of the Immune Response to Ultra-Pure Peptidoglycan
MAPK Kinase Inhibitors PD (MEK1 and MEK2 Inhibitor, Sigma-Aldrich), SB (p38/ERK MAP Kinase Inhibitor, Sigma-Aldrich), and NOD1/2 inhibitor GEF (Gefitinib, Invivogen) were added to the cell cultures along with either solution buffer (control) or uPGN (10 µg/ml). The working concentrations of the inhibitors were, respectively, 2, 0.5, and 10 µM (Table S6). The cultures were incubated for 12 h. All possible combinations of inhibitors were tested.
Prostaglandin E2 Measurement
Supernatants (serum-free DMEM with 0.2% primocin) of control and uPGN-challenged macrophages from 4 different individuals were preserved at −20°C prior testing. Prostaglandin E2 (PGE2) was quantified by a monoclonal EIA kit following the manufacturer instructions (Cayman, USA).
RNA Isolation and Quality Control
After the whole supernatant had been removed by pipetting, 250 µl of TriReagent® (Sigma-Aldrich, UK) was added to each well of a 12-welled plate. Total RNA of the macrophages was extracted by the phenol/1-bromo-3-chloropropane method (Sigma-Aldrich, UK) following the manufacturer’s instructions, with 1 μl of glycogen (Roche) per 1 ml TriReagent® added to enhance the RNA precipitation during the isopropanol step. The total RNA samples were then subjected to NanoDrop (Thermo Scientific) to determine the RNA concentration, and Bioanalyzer (Agilent Technologies) to estimate the integrity and purity of the RNA samples. Only the samples with RIN values greater than 8 were kept. Qubit® (Life Technology) was used to quantify the concentrations of the samples that were going to be sent for sequencing. All samples were stored at -80°C.
cDNA Synthesis
Different cDNA synthesis protocols were used depending on the purpose of the samples, following the manufacturer’s instructions of each kit. The Illumina RNA-seq cDNA library was prepared using 1 µg of RNA from each sample and TruSeq V2 kits (Illumina, CA, USA), with reduced RNA fragmentation time (3 min) to maximize obtention of longer reads. For PacBio IsoSeq, 1 µg of RNA per sample was used for first-strand synthesis by SMARTer PCR cDNA Synthesis Kit (Clontech). For quantitative RT-PCR (qPCR), 1 µg of total RNA per sample was used to synthesize cDNA with SuperScript III RNase Transcriptase (Invitrogen) and Oligo-dT primer (Invitrogen).
Library Preparation and Sequencing
Two rounds of Illumina RNA-seq were performed. For the first one, which consisted of a preliminary MAMP activation screening, RNA samples from macrophage cultures derived from 3 fish were pooled. In total, 9 pooled libraries were prepared: 3 control pooled samples (Ctl), 3 bacterial MAMP-activated pooled samples (Bac) and 3 dsRNA-activated pooled samples (Plc) (Table S7). They were loaded onto one lane of an eight-laned FlowCell Chip. The second round looked at the effects of exclusively uPGN on the cod macrophage transcriptome. For these, a total of 18 libraries were prepared, each individual fish had three aliquots of one control sample, one sample challenged with uPGN for 3 h and another one challenged with uPGN for 12 h. These were loaded onto two lanes of an eight-laned FlowCell Chip.
To prepare the libraries for IsoSeq, the Pacific Biosciences IsoSeq library preparation protocol was followed. Before library preparation, the cDNA was first size-selected using BluePippin (Sage Science) and then using AMPure® XP (Auto Q Biosciences) beads. Then they were sequenced on a Pacific Biosciences RS II instrument using P6v2/C4 chemistry (Pacific Biosciences of California Inc., Menlo Park, CA, USA). In total, 2 SMRT cells for 1–2kb, 3 SMRT cells for 2–3kb, and 4 SMRT cells for 3–6kb were used for sequencing.
Absolute Quantitative RT-PCR
All primers for qPCR were designed using BatchPrimer3 v1.0 based on the target genes annotated in the latest cod genome assembly gadMor2 (Table S8) (14). Target genes were validated using thermal gradient RT-PCR and the products that met the quality criteria were cloned into bacterial plasmids. Two micrograms of cDNA were used as a template for PCR with gene-specific primers. Target mRNAs were amplified using MyTaq HS DNA Polymerases (Bioline, UK), and amplicons were run on 1% agarose gels, stained with ethidium bromide and purified with NucleoSpin® Gel and PCR Clean-up (Macherey-Nagel, Germany). Purified PCR products were ligated in pGEM-T easy vectors (Promega, USA) and transformed into Escherichia coli (DH5a strain). One selected transformant of each construct was grown to obtain plasmid DNA (Miniprep kit, Macherey-Nagel). All constructs were verified by Sanger DNA sequencing with T7 and SP6 primer sets (GATC Biotech, Table S8). Pro-inflammatory cytokines mRNAs for il1b and il6 were selected on account of their high inducibility during PAMP responses (Figure S7). See details for PCR program and reaction systems in supplementary Information. One-way ANOVA was used to test the statistical differences between indicated experimental contrast. Significance was reported if P < 0.05. Graphs were plotted using Prism6® (Table S5).
Transcriptome Assembly and Transcript Abundance Estimation
Raw Illumina reads from each sample were trimmed using Cutadapt (v1.4.2) with cutoff as Phred < 20. For the downstream differential gene and transcript expression analysis two pipelines were used (1): reference genome based using the Tuxedo pipeline (Tophat2, cufflinks and cummeRbund) (22): Trimmed reads from macrophage samples (3x Ctl, 3x Bac, 3x Plc) were mapped to the gadMor2 cod genome assembly (14) with the short read aligner Tophat2 (v2.0.9) (see Supporting Information for details). The reads from all samples were assembled by Cufflinks (v2.2.1) and the results were visualized and plotted by R package “CummeRbund” (2). The updated Tuxedo pipeline consisting of Hisat2-Stringtie-Ballgown (23). The new tuxedo pipeline was used to analyze the uPGN activated samples),. The trimmed reads from uPGN activation with different time-intervals were mapped to the same genome assembly by Hisat2 (v2.0.5), assembled, merged and their expression abundance was estimated by Stringtie (v1.3.2-Pre) with default settings (23). The results were visualized and plotted with the R package “Ballgown”.
Transcriptomic Sequence Annotation
The nucleotide sequences of differentially expressed genes (DEGs) from the contrast between control and the various treatments were abstracted by Gffread from merged assemblies using Cufflinks and Stringtie (Table S9). The sequences were then annotated based on its similarities with human protein sequences and aligned to the human UniProt database (downloaded in Jan 2017) by Blastx (Blast+ v2.2.29). The non-redundant DEGs were obtained by collapsing the assembled “gene identifier” from both gene and transcript clusters and the unique ones were kept. Subsequently, the overlapping analysis of those DEGs were conducted by Venn Diagram with its annotated human gene names. Dammit (v2.0.1) was used to predict open reading frames and annotate from polished IsoSeq transcripts.
Structure of Ultra-Pure Peptidoglycan-Responsive Pattern Recognition Receptors by Full-Length Transcriptome Sequencing
All the reads from three size-selected cDNA fractions were clustered and polished by IsoSeq_SA3nUP. After polishing by Quiver, 34,516 high-quality (hq) and 157,657 low-quality (lq) transcripts were obtained. All full-length transcript isoform sequences (with either high- or low-qualities) were merged, yielding 192,173 isoforms with average length of 2,351 bp. In total, 69.75% of the transcripts were uniquely mapped to the cod genome assembly (gadMor2) by aligner Star (2.5.3a) under instructions of IsoSeq_SA3nUP. The alignments of transcripts were sorted by Samtools (v0.1.19) and visualized with the R package “Gviz”.
Synteny of IFIH1 (Retinoic Acid-Inducible-II)
Full-length protein sequences of RIG-II for non-cod species were obtained from NCBI and used directly for phylogenetic analysis. Sequences were first aligned by ClustalW and a maximum likelihood tree was produced using Maximum Likelihood (ML) with 2,000 bootstraps by MEGA7. The evolutionary history was inferred by using the Maximum Likelihood method based on the JTT matrix-based model. Synteny analyses of IFIH1 among selected vertebrates are based on genome assemblies from NCBI (RefSeq Release 82), except cod, trout and grass carp whose genome resources are available elsewhere (Table S4).
Functional Gene Ontology Enrichment Analysis
Human gene identifiers were retrieved from UniProt-SwissProt for all DEGs. Gene clusters were further divided by different immune stimuli (dsRNA/uPGN) and expression patterns (up/down). Each of them was separately provided as input gene cluster and analyzed together. Cytoscape plugin ClueGO and CluePedia were to perform the GO enrichment analysis against KEGG (Figure 5) and comprehensive functional databases including KEGG, GO, WikiPathways, and REACTOME_Pathways (Figure S5 and Dataset S1). The following ClueGO parameters were selected: Go Term Fusion; display pathways that with adjusted-p-values less than 0.05; GO tree interval levels from 3 to 8; GO term minimum numbers of genes (#) and percentage of genes (%): #4+6%, #2+3%, #3+4%, #2+3% for dsRNA-Up, -Down, uPGN-Up, -Down respectively; kappa score 0.3; GO enrichment/depletion by two-sided hypergeometric test and corrected by Benjamini-Hocheberg; GO terms are presented as nodes and grouped together based on functional similarity. Node size is negatively proportional to the adjusted p-value for GO enrichment; interactome layout by Cytoscape plugin AllegroLayout with “Allegro Spring-Electric” option.
Results
Ex Vivo Cod Macrophage Culture
Adherent cells derived from cod head-kidney and spleen differentiated into a homogeneous macrophage phenotype, with filiform pseudopodia, after three days in culture. Flow cytometry assessment of the cell population demonstrated homogeneity (92%) and was gated for further functional assays (gate R1 in Figures 1A, B, Table S1). Phagocytic capacity was tested with fluorescence-labelled microbes (FITC Escherichia coli and Saccharomyces cerevisiae) demonstrating 40.77 ± 26.54% (mean ± std. deviation) internalization of yeast within 1 h of incubation at 12 °C (Figures 1C, D, Figure S1 and Table S1) and 66.42 ± 3.21% internalization of E.coli after 3 h of incubation (Figures 1E, F, Figure S1 and Table S1).
The Antiviral Response Is Conserved in the Absence of Retinoic Acid-Inducible -I
To elucidate the bacterial and viral transcriptional response of the ex vivo macrophage culture, a collection of MAMPs were used to mimic a bacterial (“Bac”, LPS, PGN, CpG DNA) and viral (“dsRNA”, HMW dsRNA) infection. In total, 101 up-regulated and 4 down-regulated genes were identified for group “Bac” (Figure 2A), and 688 up-regulated and 105 down-regulated genes for group “dsRNA” (Figure 2B). Analysis of annotated DEGs from both challenge groups revealed 46 up-regulated genes expressed in both treatments (Figure 2C, Table S2, Data S1). These were mostly related to membrane internalization and trafficking, activation of transcription and cellular signaling cascades including NF-KB and MAPKs pathways, inflammation-related cytokines, and feedback loop regulators (Figure 2D, Table S2 and Data S1). DEGs (492 total) specific to dsRNA were classified into eight major gene clusters conservatively associated to the antiviral immune response including: i) JAK/STAT pathway; ii) RLRs pathway; iii) transcriptional factors; iv) NF-kB and MAPK signaling; v) CAMs (Cell adhesion molecules); vi) antigen presentation by MHC class I; vii) inflammasome and apoptosis; viii) Cytokines and effector proteins (Figure 2D, Figure S2, Data S1).
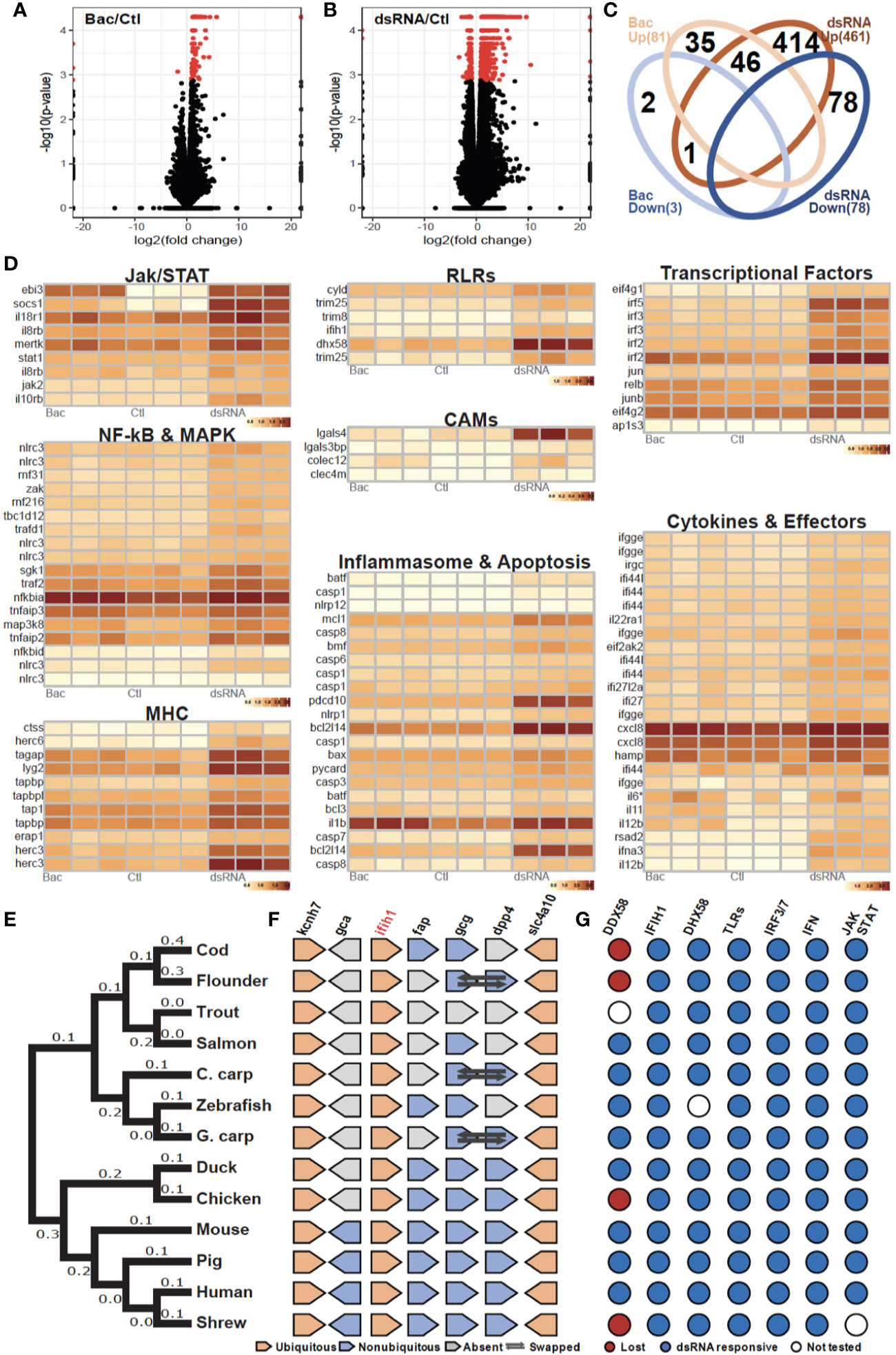
Figure 2 Evolutionarily conserved antibacterial and antiviral response of cod macrophages (A, B). Volcano plots of the expression patterns and statistical significance (shown in red, FDR < 0.05) of genes across three biological samples challenged with (A) bacteria-derived microbe-associated molecular patterns (MAMPs) (Bac) and (B) dsRNA contrasted with control samples (Ctl) (C). Venn diagram showing number of overlapped up-regulated (UP) and down-regulated (DOWN) genes that had been annotated among Bac and Plc (D). Transcript abundance (log10FPKM+1) of selected DEGs, grouped based on shared signaling pathways (E). Phylogenetic tree of ifih1 from 13 vertebrates. Number of nucleotide substitutions per site are shown next to each branch (F). Synteny analysis of ifih1. Pentagon shapes represent each gene, pointing in the direction of transcription. Ubiquitous genes are orange, absent genes are grey, and non-ubiquitous genes are blue. Dark arrows indicate swapped genes (G). Antiviral responsiveness of the “RLRs-TLRs-IRF3/7-type1IFN-JAK/STAT” axis in 13 vertebrates, as shown by published studies. Red circles indicate lost genes, white circles untested genes, and blue circles responsive genes.
Interestingly, cod (Gadus morhua) (24), flounder (Paralichthys olivaceus) (25), chicken (Gallus gallus) (26) and the tree shrew (Tupaia chinensis) (27) lack RIG-I (ddx58), once considered to be indispensable in jawed vertebrates, and yet can initiate robust antiviral responses. As we observed RLR pathway activation, we looked at other RLR genes in the Atlantic cod genome including ifih1 and slc4a10. Phylogenetic analysis placed the cod RIG-II (ifih1) into the teleost lineage as expected (Figure 2E). Further, synteny analysis revealed a conserved genomic gene array structure where ifih1 is located, with flanking genes kcnh7 and slc4a10 being ubiquitously presented across all tested vertebrate genomes (Figure 2F, Table S3). Synteny in the teleost lineage was less conserved for ifih1-adjacent genes including fap, gcg, and dpp4, which displayed significant variation. Notably, these flanking genes were absent from the trout genome which might be due to the incompleteness of the genome assembly and/or genomic rearrangement. Moreover, a survey of functional studies in these 13 vertebrates using dsRNA (or in some cases viruses) as stimuli highlights a conserved antiviral signaling axis, where key components RLRs, TLR3, IRF3/7, type I IFN, and JAK/STAT are involved (Figure 2G, Table S4).
Ultra-Pure Peptidoglycan Drives Bacterial Microbe-Associated Molecular Patterns Recognition
In fish, the recognition of bacterial MAMPs and their respective roles in downstream signaling and activation remains under debate (28). In cod, the absence of homologs of the major cell surface mammalian TLRs allowed for further exploration of the downstream signaling pathway following LPS, PGN, and CpG DNA detection. Using il1b and il6 mRNA expression as markers of MAMP recognition, a subsequent pro-inflammatory response, although non-significant, was shown in macrophages to LPS (Figure 3A) and CpG DNA (Table S5). Ultra-pure PGN (MDP, & iE-DAP) treatment induced a significant activation both 3- and 12-h post challenge with MAMPs, while peptidoglycan did not (Figure 3A and Table S5).
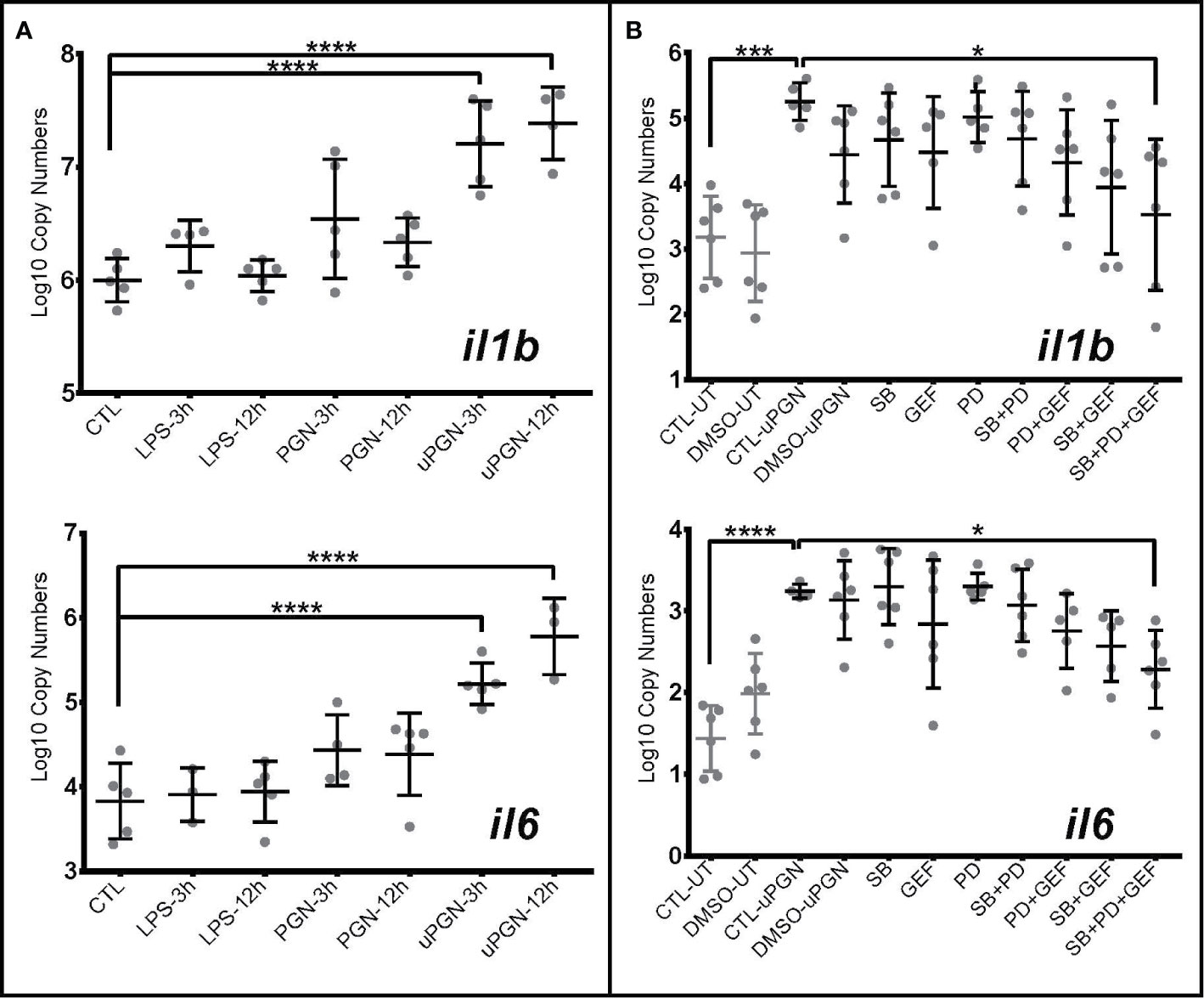
Figure 3 Ultra-pure peptidoglycan (uPGN) triggers the strongest antibacterial response in Atlantic cod macrophages, mediated by NOD1 and MAPKs (A, B). Copy number (log10) of interleukin il6 and il1b in response to (A) an activation by various individual MAMPS during 3 and 12 h and (B) inhibition of nucleotide-binding oligomerization (NOD) pathway-relevant genes in uPGN-activated samples. PD, MEK1 and MEK2 Inhibitor; GEF, gefitinib; SB, p38 MAP Kinase Inhibitor. Whiskers show the standard deviation. Asterisks represent statistical significance: *<0.05, ***<0.001,**** <0.0001. Abbreviations: CTL, control; LPS, lipopolysaccharide; PGN, peptidoglycan; uPGN, Muramyl dipeptide peptidoglycan; UT, untreated; DMSO, dimethyl sulfoxide.
To further delineate the signaling pathways involved, we employed a suite of kinase inhibitors targeting p38 MAPK and MAPKK (MEK1 and MEK2), including a NOD1/2 inhibitor acting through RIPK2. None of the inhibitors significantly blocked uPGN-driven il-1b and il-6 mRNA increases while used individually. However, when combined, a significant inhibition was observed (32.9% for il-1b and 29.5% for il-6) (Figure 3B and Table S5). Although sequence divergence may impact upon cod-specific MAPK inhibition, combinations of RIPK2 and MAPK inhibition produced a more pronounced effect in comparison to MAPKK and p38 inhibition.
uPGN-treated macrophage cultures that were positive for both il1b gene expression (Figure 4A) and extracellular secretion of the pro-inflammatory mediator prostaglandin E2 (PGE2) (Figure 4B) were analyzed by RNA-Seq. uPGN-activated macrophages showed higher activation (414 DEGs) than in response to the previously used combination of bacterial MAMPs (LPS, PGN and CpG DNA) (105 DEGs). Interestingly, at 3 h post uPGN treatment only 5 up-regulated DEGs and no down-regulated DEGs were observed (Figure 4C), while 286 up-regulated and 123 down-regulated DEGs were identified after 12 h of treatment (Figure 4D, Figure S3 and Data S1). The majority of DEGs (90%) were annotated, and functionally categorized based on six major pathways: i) G-protein-coupled receptors (GPCRs) and endocytosis; ii) PRRs sensing; iii) apoptosis; iv) NF-kB and MAPK; v) cytokines; and vi) transcription factors (Figure 4E, Data S1). Both il1b and ripk2 were significantly regulated consistently with previous gene expression results.
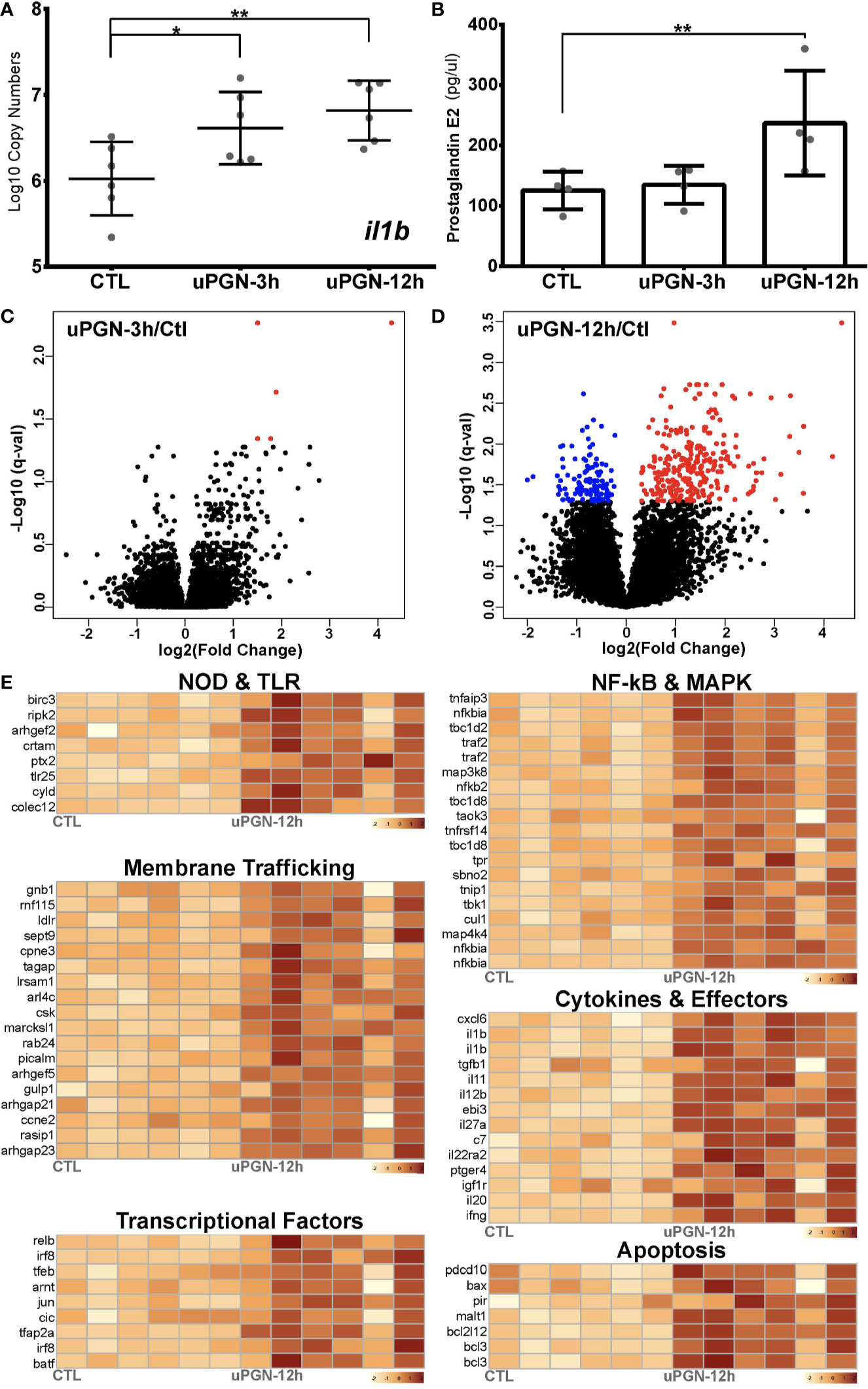
Figure 4 Antibacterial response to uPGN challenge (A, B). Immune response to uPGN measured by (A) copy number (log10) of il1b and (B) prostaglandin E2 secretion in ex-vivo cultured cod macrophages (C, D). Volcano plots of the expression patterns and statistical significance (red if significantly up-regulated, blue if significantly down-regulated, FDR < 0.05) of genes across six biological samples challenged with uPGN for (C) 3 h and (D) 12 h contrasted with control samples (E). Transcript abundance (log10FPKM+1) of selected DEGs challenged with uPGN for 12 h. Grouped based on shared signaling pathways.
Divergent Microbe-Associated Molecular Pattern-Pattern Recognition Receptor Recognition Systems Converge
The extensive repertoire of MAMP sensing in animals is underpinned by a diverse set of adaptors and signaling proteins that form pathways that ultimately activate the inflammatory response. In cod, dependence upon intracellular MAMP sensors may be expected to impact upon the activation of critical signaling pathways. Using GO enrichment analysis with cod DEGs annotated to human SwissProt identifiers, significantly enriched KEGG pathways for dsRNA- and uPGN up-regulated DEGs were shown to be partially shared (Figure 5 and Data S1). Six major functional clusters with their associated DEGs highlighted extensive convergent signaling in cod macrophages upon distinct MAMP challenge (Figure 5 and Figure S5), consistent with shared DEGs between dsRNA and uPGN (Table S2). It is also noteworthy that the MAMP sensing pathways, for both dsRNA and uPGN, in cod demonstrate stimuli-specific DEGs that converge upon common thematic regulators, thus providing diversity in MAMP-specific regulators of signaling. For dsRNA-specific pathways, RLR- and NLR-receptors, JAK-STAT signaling, IRF3/7 signaling, type-I IFN response and MHC class I antigen presentation pathways were shown to be conserved across vertebrates, from mammals (29) to teleosts (30–32) (Data S1 and Figure S5). In contrast, the cod uPGN-specific pathway demonstrates a different MAMP sensing mechanism where NOD signaling, pro-inflammatory responses, type-II IFN responses and leukocyte differentiation mediated by IFNG, IRF8 and TGFB1, are enriched.
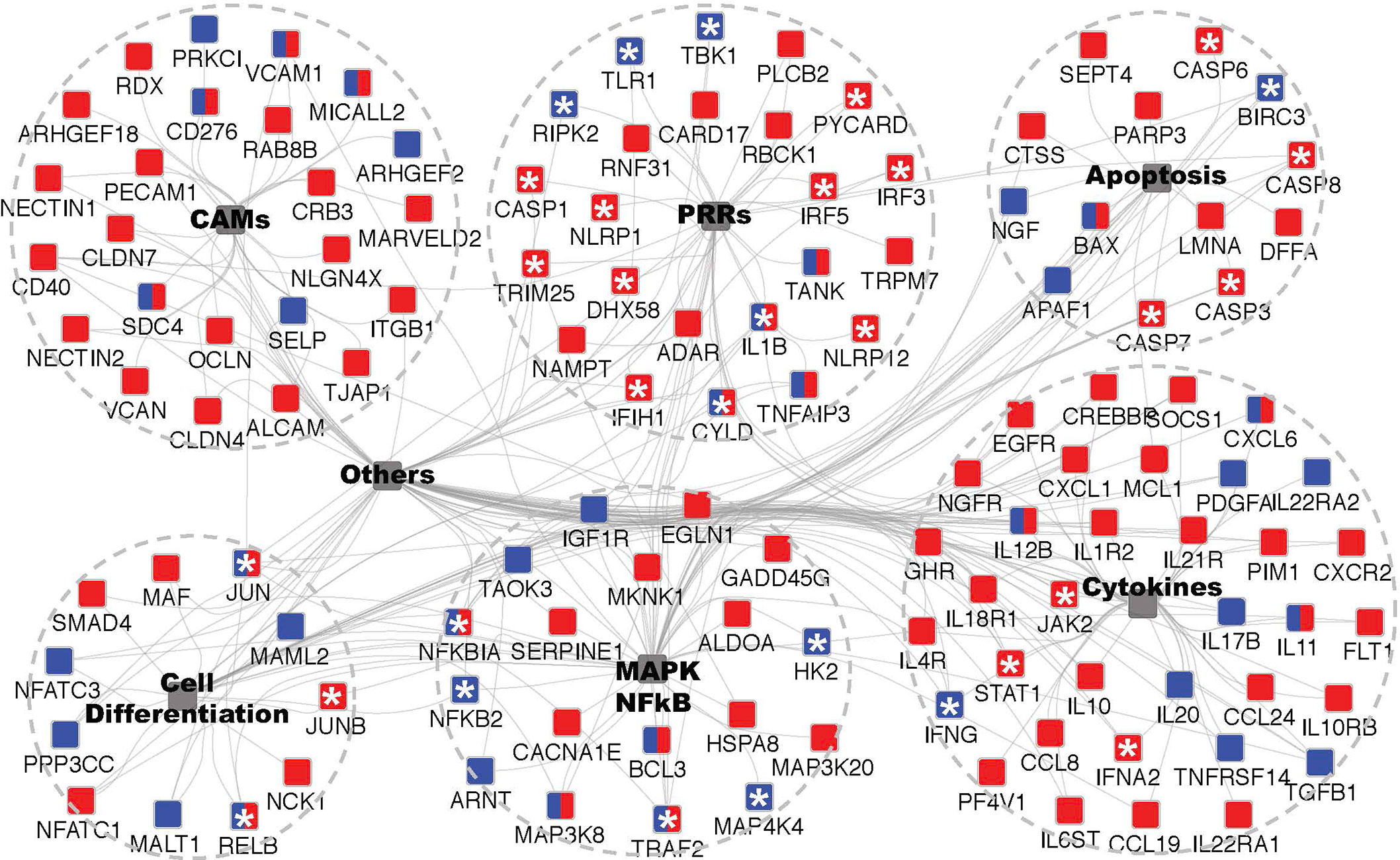
Figure 5 GO enrichment analysis of microbe-associated molecular pattern (MAMP) up-regulated DEGs to identify underpinned functional pathways in activating the cod macrophage inflammatory response. GO enrichment and network visualization was conducted by Cytoscape plugin ClueGO and CluePedia. Cod up-regulated DEGs annotated to human SwissProt identifiers were mapped to KEGG (Kyoto Encyclopedia of Genes and Genomes) pathways database. Enriched KEGG terms that are shared the same cluster were collapsed into one integrative node (shaded grey) labelled with brief descriptions respectively (See Figure S5 and Dataset S1 for the full network). Six major inflammation-related functional pathways were shown, and the remaining ones were labelled with “Others”. The associated DEGs with indicated KEGG pathways were color coded in a MAMP-dependent fashion, red and blue nodes denote dsRNA- and uPGN-responsive genes, respectively; whilst the dual-labelled nodes denote the common DEGs that are responsive to both MAMPs. Asterisks indicate genes that serve as key sensors, adaptors or regulators in mediating inflammatory response and had been intensively discussed in texts.
Evidence for (Sub) Neofunctionalization in Cod Pattern Recognition Receptor
To further capture the full-length mRNA isoforms, especially those of significance in MAMP sensing, long read PacBio transcriptome profiling (IsoSeq) was performed. After polishing by Quiver, 34,516 high-quality (hq) and 157,657 low-quality (lq) transcripts were obtained. Merging of the two, resulted in 192,173 isoforms with average length of 2,351 bp were obtained. In total, 69.75% of the transcripts were uniquely mapped to the cod genome assembly (gadMor2). For some of the low expressed genes detected using RNA-Seq (e.g., FPKM< 1), such as colec12, nlrc3 and ifih1 (RIG-II), long reads were not detected, probably due to low sequencing coverage. Most cod PRRs found in the Illumina sequencing data were captured at least once by IsoSeq. Inspection of the full-length transcripts of these PRRs obtained by IsoSeq validated the high sensitivity of short reads assembler Cufflinks and StringTie (Figure S5). For instance, three potential transcripts for RIG-III (dhx58) were assembled by Cufflinks, of which only one isoform “TCONS_00018715” was captured by IsoSeq (Figure S6). Accordingly, the most dominant (>75%) isoform “TCONS_00018715” had the highest abundance (FPKM=21.06) in untreated resting macrophages (Ctl). Additionally, two other isoforms “TCONS_00018716” (with an extra 5’-end exon), and “TCONS_00018717” (with exon skipping) were dsRNA-challenge specific. Multiple transcripts for tlr25 were identified by StringTie (after filtering out transcripts with low variance (see Supplementary Information for details), of which only transcript “MSTRG.16445.4” were significantly activated by uPGN (Fold Change=2.831, FDR=0.016). Sequence alignment of all these transcripts towards tlr25 isoforms (a~g) from Solbakken et al (12, 13)., showed that the isoform “MSTRG.16445.4” is identical to TLR25d (13) (Figure S6).
Discussion
Experimental studies, prior to the discovery of the alternative arrangement of the Atlantic cod immune system (5, 33, 34), described the Atlantic cod immune response, including survival (35), fitness (36), pathogen elimination (37), and antibody-production (38), as being broadly similar to other teleost fish (39, 40). Furthermore, the loss of most membrane-bound TLRs, MHC II, CD74 invariant chain and the CD4 receptor does not appear to have imposed restrictions on the Gadiformes, a highly successful family (>400 reported species) (2). Therefore, a more prominent role, due to increased gene dosage, for intracellular PRRs and MHC I has been proposed (5, 13).
In order to assess the MAMP-PRR response in cod, an ex-vivo macrophage cell culture model was developed. As one of the major professional antigen-presenting cells (APCs), highly phagocytic macrophages are responsible for innate-adaptive immunity cross-talk (21). Differentiated cod macrophages efficiently engulf microbes, produce several inflammatory mediators and generate a canonical time-dependent MAMP-specific immune response in agreement with vertebrate macrophage models (19, 20, 29) (multidimensional scaling; Figures S2 and S3, functional clustering; Figure 5). The MAMP-activated cod macrophage transcriptome is characterized by profound changes in the expression of cytokines, chemokines, antimicrobial peptides and transcriptional factors (Data S1), driven by a set of stimuli-specific gene-clusters tailored to cope with different MAMP-PRR signals. Consistently, functional interactomes for dsRNA and uPGN responsive DEGs are all convergent into functional pathways such as NF-kB and IRFs (Figures 2 and 4). Similar results were reported in in-vivo studies on cod using the facultative intracellular bacterium Francisella noatunensis (41). Our data demonstrates that in cod, macrophage immune response specificity is generated in a ligand-dependent manner in the absence of certain membrane-bound TLR interactions (Figures 2 and 4).
The co-evolution of both diversity and restriction across the different components of the immune system is an intriguing puzzle, but hampered by the challenge of developing suitable experimental models (42). Strong evolutionary constraints imposed upon metazoan regulatory molecular circuits ensure robust regulatory mechanisms whereas pressure to diversify recognition structures toward pathogens is critical (9). Interestingly, the deletions observed in the cod genome appear contradictory in that specific cell-surface TLR are absent thus reducing recognition capacity (5), although functionality in cod macrophages is not impaired. Studies in Atlantic cod suggest that endocytosis of lipoprotein and polyribonucleotides (dsRNA) is mediated by scavenger receptors (43). For exogenous dsRNA sensing, variations of membrane-trafficking delivery can trigger distinct immune responses (44, 45). Lipid-based dsRNA transfection induces a highly efficient and scavenger receptor Class-A (SR-As) independent endocytosis triggering TLR3-mediated apoptosis (44, 45). In contrast, directly adding dsRNA to the culture medium, as in this study, induces a SR-As-dependent endocytosis, mediated by the RLR family of cytosolic receptors as seen in mammalian cells (44, 45). In this study, cod macrophages increased the transcription of the Scavenger Receptor Class-A Member (SR-As) colec12 (aka SCARA4) with both dsRNA and uPGN indicating activation of SR-As-dependent endocytosis. Moreover, many other vesicle-trafficking related pathways were significantly activated indicating a common response (Figure 5 and Data 1). In accordance, elevated transcription of all cod RLRs, including ifih1 and dhx58, post dsRNA challenge was observed. In contrast, no activation was observed for tlr3 or its relevant adaptors including myd88 and trif. PGN does not induce any inflammatory response via direct microinjection into cytosol in mammals, unless delivered by membrane encapsulated-vesicles (46). In fact, recent studies highlight the endosome as a crucial platform for NOD1/2-dependent ligand sensing and inflammatory signaling (47–49). Despite nod1 not being significantly induced by uPGN (Fold-change: 1.7; p-val: 0.01; FDR: 0.13, Figure S4), transcription of its adaptor (arhgef2), enzymes (ripk2, hk2), and ubiquitination regulators (birc3, cyld, tnfiap3) were all consistently elevated in our study which is consistent with observations from studies in mammals (48, 50). Additionally, results from our blockade assays using a cocktail of inhibitors against RIPK2 and MAPKs further validated the role of NOD-pathways in sensing uPGN in cod macrophages. Therefore, despite the absence of nod2, the NOD pathway in cod remains functional, in line with observations of NOD1-mediated antibacterial responses in birds lacking NOD2 including both duck (51) and chicken (52).
In addition to the loss of specific TLRs and the classical MHC II pathway, some intracellular PRRs including RIG-I and NOD2 were also found to be absent in the Atlantic cod (13). Similarly, RIG-I is lost in the coelacanth, spotted gar, cave fish, lamprey and tree shrew and NOD2 is absent in the spotted gar, frog, reptiles and birds (Ensembl Gene gain/loss tree). We found that the remaining MDA5/LGP2 and NOD1 initiate the RLR- and NOD-pathways specific to dsRNA and uPGN sensing, respectively, in Atlantic cod. In the tree shrew, MDA5 and LGP2 synergistically mediate dsRNA sensing regardless of molecular-weight differences in compensation for the loss of RIG-I (27). This is achieved by the RIG-I-specific adaptor, STING (Stimulator of interferon genes protein, encoded by tmem173), that acquired a novel MDA5 interacting function (27). In this study, the high-molecular-weight dsRNA was used as a general ligand for MDA5/LGP2. Further studies concerning the differences in ligand types (e.g., HMW v.s. LMW) and delivery routes (e.g., extracellularly or via liposome transfection) would provide additional information in partitioning the specificities of receptor profiles. In zebrafish, NOD1 knockout mediated by gene-editing dramatically affected many other NLRs members by inducing expression whilst conventional NF-κB and MAPK immune signaling pathways were unimpaired (53). Interestingly, in line with above mentioned PRRs, in dsRNA or uPGN challenged cod macrophages, other receptors such as nlrc3s, nlrp1, nlrp12 and tlr25 were also significantly up-regulated (Figure 5 and Data 1). In contrast to mammals, the dramatically expanded NLR and TLR gene repertoire in the cod genome suggest a potential rapid functional shift (7, 14). TLR25, one of the teleost-specific TLRs, belongs to the TLR1 family and shares significant sequence similarity with mammalian TLR1 (12, 13). In this study, one of the TLR25 isoforms, tlr25d, was uniquely regulated by uPGN challenge, however no changes were observed in any Myddosome or Triffosome related factors leaving its downstream signaling in cod unclear. On the other hand, several NLR family members’ associated adaptors including pycard and casp1 were induced suggesting that these mediators of inflammasome formation are active in Atlantic cod as reported in mammals (54). Recent studies, in zebrafish, described a conserved functional role for the NLRP1 inflammasome in teleosts (55) and our observations further support a central role for NLRs in innate immunity in the Atlantic cod and across the Teleost fish.
The underlying molecular patterns of activation in cod macrophages point toward a conserved cytosolic MAMP-PRR recognition that link to supra molecular organizing centers which themselves appear to be conserved across vertebrate innate immune signaling (9, 31). RLRs, NLRs and TLRs engage in convergent signaling cascades to regulate the expression and degradation of pro-inflammatory mediators upon ligand sensing, that are highly conserved owing to strong functional and regulatory constraints (56, 57). Interestingly, in fish canonical LPS-TLR4 sensing is seemingly absent (58), with the exception of zebrafish, as a negative regulator of inflammation (28). In this study, no significant response to a standard LPS preparation was detected. However, a robust uPGN-NOD driven activation of inflammation coupled to an enrichment of the IRF8 pathway was observed (Figure S5 and Dataset S1). In mice, IRF8-impairment led to reduced secretion of IL-12, an essential inducer of T helper (Th1) cell polarization, causing a dramatic increase in susceptibility to intracellular infections highlighting a critical defense function (59). Furthermore, a regulatory role for IRF8 has been suggested in MHC II antigen presentation (60, 61). Recently, MHC class I based cross-presentation through the acquisition of functional sorting motifs has been suggested as a mechanism in cod for MHC II loss (62). The cod macrophage model and further exploration of the IRF8 pathway provides a platform for further functional studies. Multiple genome-based studies across a wide range of fishes highlight an exceptional diversity at the level of cytosolic PRR families (63–65). It is tempting to speculate that the environment, both aquatic and internal, coupled to restricted adaptive immunity has driven innovation in cytosolic MAMP detection.
Available evidence, including this study, suggests that functionality including intracellular PRR systems are conserved throughout vertebrates, and thus, also provide an efficient host defense in the Gadiformes lineage contributing to their evolutionary success (31, 41, 66–68). In light of the loss of the majority of extracellular PRR capability and lack of MHC class II-based antigen presentation it suggests, at least in the Gadiformes, these functions may not be ‘core’ to the immune response and possibly bony fishes in general. Notably, evidence for sub- or neofunctionalization of PRR, in a ligand-dependent manner, is demonstrated via several NLRs paralogues and one of the TLR25 isoforms. Sub- or neofunctionalization following gene duplication of multigene gene families driven by selection is a recognized mechanism of genome innovation and adaptation (4). The dramatically diversified NLR and TLR paralogues, coupled with their complex protein-protein interactions via ligand-sensing and signal transduction domains, presumably allow the cod immune system to adapt to fast-evolving pathogens.
In summary, our results, based upon a novel ex vivo macrophage cell culture, demonstrate that intracellular PRR systems based upon RLR and NOD signaling, in the absence of RIG-I and NOD2, are critical drivers of the response to bacterial and viral pathogens in Atlantic cod. Our analysis highlighted the inflammatory response to be intimately linked to scavenger receptor-based internalization of MAMPs coupled to highly conserved intracellular signaling platforms. Cytosolic PRR capabilities in the Atlantic cod are functional, in line with most vertebrate studies, and are based upon canonical NF-kB and IRFs activation pathways. Additionally, we uncover that the multiple NLR paralogues and the unique TLR25 isoform identified in Atlantic cod display ligand-dependent differential expression suggesting (sub)neofunctionalisation toward specific immune defensive strategies. Our results further demonstrate that the extreme remodeling of the Atlantic cod immune system provides an unprecedented opportunity to explore the evolutionary history of PRR-based signaling in vertebrate immunity.
Author’s Note
This manuscript has been released as a pre-print at bioRxiv 2020.08.07.241067; doi: https://doi.org/10.1101/2020.08.07.24106765.
Data Availability Statement
The datasets presented in this study can be found in online repositories. The names of the repository/repositories and accession number(s) can be found in the article/Supplementary Material.
Ethics Statement
The animal study was reviewed and approved by Animal Welfare and Ethical Review Body (AWERB), University of Stirling. Application number: AWERB/1718/132/New Non ASPA.
Author Contributions
SM, KJ, and SJ designed the study and obtained financial support. XJ, BM, VM, and SM participated in the experimental design, macrophage cell culture development, and sampling. XJ, BM, OT, and MS carried out bioinformatic analyses and posterior statistical analysis. All authors participated in drafting the final manuscript. All authors contributed to the article and approved the submitted version.
Conflict of Interest
The authors declare that the research was conducted in the absence of any commercial or financial relationships that could be construed as a potential conflict of interest.
Acknowledgments
The sequencing service was provided by the Norwegian Sequencing Centre (www.sequencing.uio.no), a national technology platform hosted by the University of Oslo and supported by the “Functional Genomics” and “Infrastructure” programs of the Research Council of Norway and the Southeastern Regional Health Authorities. All bioinformatics analysis was performed using Abel high performance computer cluster (under project Nos n9244kk), owned by the University of Oslo and the Norwegian metacenter for High Performance Computing (NOTUR), and operated by the Department for Research Computing at USIT, the University of Oslo IT-department. The project was supported by the Research Council of Norway (grant number 222378/F20 to KJ).
Supplementary Material
The Supplementary Material for this article can be found online at: https://www.frontiersin.org/articles/10.3389/fimmu.2020.609456/full#supplementary-material
Supplementary Data Sheet 1 | Differential expression analysis and GOs/KEGGs enrichment output.
References
1. Netea MG, Schlitzer A, Placek K, Joosten LAB, Schultze JL. Innate and Adaptive Immune Memory: an Evolutionary Continuum in the Host’s Response to Pathogens. Cell Host Microbe (2019) 25(1):13–26. doi: 10.1016/j.chom.2018.12.006
2. Malmstrom M, Matschiner M, Torresen OK, Star B, Snipen LG, Hansen TF, et al. Evolution of the immune system influences speciation rates in teleost fishes. Nat Genet (2016) 48(10):1204–10. doi: 10.1038/ng.3645
3. Palti Y. Toll-like receptors in bony fish: From genomics to function. Dev Comp Immunol (2011) 35(12):1263–72. doi: 10.1016/j.dci.2011.03.006
4. Wang B, Wangkahart E, Secombes CJ, Wang T. Insights into the Evolution of the Suppressors of Cytokine Signaling (SOCS) Gene Family in Vertebrates. Mol Biol Evol (2019) 36(2):393–411. doi: 10.1093/molbev/msy230
5. Star B, Nederbragt AJ, Jentoft S, Grimholt U, Malmstrom M, Gregers TF, et al. The genome sequence of Atlantic cod reveals a unique immune system. Nature (2011) 477(7363):207–10. doi: 10.1038/nature10342
6. Roth O, Solbakken MH, Tørresen OK, Bayer T, Matschiner M, Baalsrud HT, et al. Evolution of male pregnancy associated with remodeling of canonical vertebrate immunity in seahorses and pipefishes. Proc Natl Acad Sci (2020) 117(17):9431. doi: 10.1073/pnas.1916251117
7. Tørresen OK, Brieuc MSO, Solbakken MH, Sørhus E, Nederbragt AJ, Jakobsen KS, et al. Genomic architecture of haddock (Melanogrammus aeglefinus) shows expansions of innate immune genes and short tandem repeats. BMC Genomics (2018) 19(1):240. doi: 10.1186/s12864-018-4616-y
8. Kang S, Kim J-H, Jo E, Lee SJ, Jung J, Kim B-M, et al. Chromosomal-level assembly of Takifugu obscurus (Abe, 1949) genome using third-generation DNA sequencing and Hi-C analysis. Mol Ecol Resour (2020) 20(2):520–30. doi: 10.1111/1755-0998.13132
9. Kieser KJ, Kagan JC. Multi-receptor detection of individual bacterial products by the innate immune system. Nat Rev Immunol (2017) 17(6):376–90. doi: 10.1038/nri.2017.25
10. Meunier E, Broz P. Evolutionary Convergence and Divergence in NLR Function and Structure. Trends Immunol (2017) 38(10):744–57. doi: 10.1016/j.it.2017.04.005
11. Stocks CJ, Schembri MA, Sweet MJ, Kapetanovic R. For when bacterial infections persist: Toll-like receptor-inducible direct antimicrobial pathways in macrophages. J Leukoc Biol (2018) 103(1):35–51. doi: 10.1002/JLB.4RI0917-358R
12. Solbakken MH, Voje KL, Jakobsen KS, Jentoft S. Linking species habitat and past palaeoclimatic events to evolution of the teleost innate immune system. Proc Biol Sci (2017) 284(1853):20162810. doi: 10.1098/rspb.2016.2810
13. Solbakken MH, Tørresen OK, Nederbragt AJ, Seppola M, Gregers TF, Jakobsen KS, et al. Evolutionary redesign of the Atlantic cod (Gadus morhua L.) Toll-like receptor repertoire by gene losses and expansions. Sci Rep (2016) 6:25211. doi: 10.1038/srep25211
14. Tørresen OK, Star B, Jentoft S, Reinar WB, Grove H, Miller JR, et al. An improved genome assembly uncovers prolific tandem repeats in Atlantic cod. BMC Genomics (2017) 18(1):95. doi: 10.1186/s12864-016-3448-x
15. Torresen OK, Star B, Mier P, Andrade-Navarro MA, Bateman A, Jarnot P, et al. Tandem repeats lead to sequence assembly errors and impose multi-level challenges for genome and protein databases. Nucleic Acids Res (2019) 47(21):10994–1006. doi: 10.1093/nar/gkz841
16. Franchi L, Warner N, Viani K, Nuñez G. Function of Nod-like receptors in microbial recognition and host defense. Immunol Rev (2009) 227(1):106–28. doi: 10.1111/j.1600-065X.2008.00734.x
17. Giolai M, Paajanen P, Verweij W, Witek K, Jones JDG, Clark MD. Comparative analysis of targeted long read sequencing approaches for characterization of a plant’s immune receptor repertoire. BMC Genomics (2017) 18(1):564. doi: 10.1186/s12864-017-3936-7
18. Conesa A, Madrigal P, Tarazona S, Gomez-Cabrero D, Cervera A, McPherson A, et al. A survey of best practices for RNA-seq data analysis. Genome Biol (2016) 17:13. doi: 10.1186/s13059-016-0881-8
19. MacKenzie SA, Roher N, Boltaña S, Goetz FW. Peptidoglycan, not endotoxin, is the key mediator of cytokine gene expression induced in rainbow trout macrophages by crude LPS. Mol Immunol (2010) 47(7–8):1450–7. doi: 10.1016/j.molimm.2010.02.009
20. Callol A, Roher N, Amaro C, MacKenzie S. Characterization of PAMP/PRR interactions in European eel (Anguilla anguilla) macrophage-like primary cell cultures. Fish Shellfish Immunol (2013) 35(4):1216–23. doi: 10.1016/j.fsi.2013.07.037
21. Nakagaki BN, Vieira AT, Rezende RM, David BA, Menezes GB. Tissue macrophages as mediators of a healthy relationship with gut commensal microbiota. Cell Immunol (2018) 330:16–26. doi: 10.1016/j.cellimm.2018.01.017
22. Trapnell C, Roberts A, Goff L, Pertea G, Kim D, Kelley DR, et al. Differential gene and transcript expression analysis of RNA-seq experiments with TopHat and Cufflinks. Nat Protoc (2012) 7(3):562–78. doi: 10.1038/nprot.2012.016
23. Pertea M, Kim D, Pertea GM, Leek JT, Salzberg SL. Transcript-level expression analysis of RNA-seq experiments with HISAT, StringTie and Ballgown. Nat Protoc (2016) 11(9):1650–67. doi: 10.1038/nprot.2016.095
24. Eslamloo K, Xue X, Booman M, Smith NC, Rise ML. Transcriptome profiling of the antiviral immune response in Atlantic cod macrophages. Dev Comp Immunol (2016) 63:187–205. doi: 10.1016/j.dci.2016.05.021
25. Hikima J-I, Yi M-K, Ohtani M, Jung CY, Kim YK, Mun JY, et al. LGP2 Expression is Enhanced by Interferon Regulatory Factor 3 in Olive Flounder, Paralichthys olivaceus. PloS One (2012) 7(12):e51522. doi: 10.1371/journal.pone.0051522
26. Barber MRW, Aldridge JR, Webster RG, Magor KE. Association of RIG-I with innate immunity of ducks to influenza. Proc Natl Acad Sci (2010) 107(13):5913. doi: 10.1073/pnas.1001755107
27. Xu L, Yu D, Fan Y, Peng L, Wu Y, Yao Y-G. Loss of RIG-I leads to a functional replacement with MDA5 in the Chinese tree shrew. Proc Natl Acad Sci (2016) 113(39):10950–5. doi: 10.1073/pnas.1604939113
28. Sepulcre MP, Alcaraz-Perez F, Lopez-Munoz A, Roca FJ, Meseguer J, Cayuela ML, et al. Evolution of lipopolysaccharide (LPS) recognition and signaling: fish TLR4 does not recognize LPS and negatively regulates NF-kappaB activation. J Immunol (Baltimore Md 1950) (2009) 182(4):1836–45. doi: 10.4049/jimmunol.0801755
29. Jia X, Yuan S, Wang Y, Fu Y, Ge Y, Ge Y, et al. The role of alternative polyadenylation in the antiviral innate immune response. Nat Commun (2017) 8:14605. doi: 10.1038/ncomms14605
30. Nie L, Zhang Y-S, Dong W-R, Xiang L-X, Shao J-Z. Involvement of zebrafish RIG-I in NF-κB and IFN signaling pathways: Insights into functional conservation of RIG-I in antiviral innate immunity. Dev Comp Immunol (2015) 48(1):95–101. doi: 10.1016/j.dci.2014.09.008
31. Inkpen SM, Solbakken MH, Jentoft S, Eslamloo K, Rise ML. Full characterization and transcript expression profiling of the interferon regulatory factor (IRF) gene family in Atlantic cod (Gadus morhua). Dev Comp Immunol (2019) 98:166–80. doi: 10.1016/j.dci.2019.03.015
32. Eslamloo K, Inkpen SM, Rise ML, Andreassen R. Discovery of microRNAs associated with the antiviral immune response of Atlantic cod macrophages. Mol Immunol (2018) 93:152–61. doi: 10.1016/j.molimm.2017.11.015
33. Magnadottir B, Jonsdottir H, Helgason S, Bjornsson B, Jorgensen TO, Pilstrom L. Humoral immune parameters in Atlantic cod (Gadus morhua L.): I. The effects of environmental temperature. Comp Biochem Physiol B Biochem Mol Biol (1999) 122:173–80 doi: 10.1016/s0305-0491(98)10157-8
34. Magnadóttir B. Innate immunity of fish (overview). Fish Shellfish Immunol (2006) 20(2):137–51. doi: 10.1016/j.fsi.2004.09.006
35. Jensen I, Seppola M, Steiro K, Sandaker E, Mennen S, Sommer A-I. Susceptibility of Atlantic cod Gadus morhua juveniles to different routes of experimental challenge with infectious pancreatic necrosis virus (IPNV). Dis Aquat Organisms (2009) 85(2):105–13. doi: 10.3354/dao02066
36. Lange S, Bambir S, Dodds AW, Magnadóttir B. The ontogeny of complement component C3 in Atlantic cod (Gadus morhua L.)—an immunohistochemical study. Fish Shellfish Immunol (2004) 16(3):359–67. doi: 10.1016/j.fsi.2003.06.001
37. Gudmundsdottir S, Magnadottir B, Bjornsdottir B, Arnadottir H, Gudmundsdottir BK. Specific and natural antibody response of cod juveniles vaccinated against Vibrio anguillarum. Fish Shellfish Immunol (2009) 26(4):619–24. doi: 10.1016/j.fsi.2008.09.017
38. Pilström L, Petersson A. Isolation and partial characterization of immunoglobulin from cod (Gadus morhua L.). Dev Comp Immunol (1991) 15(3):143–52. doi: 10.1016/0145-305X(91)90005-J
39. Grayfer L, Kerimoglu B, Yaparla A, Hodgkinson JW, Xie J, Belosevic M. Mechanisms of Fish Macrophage Antimicrobial Immunity. Front Immunol (2018) 9:(1105):173–80. doi: 10.3389/fimmu.2018.01105
40. Pereiro P, Figueras A, Novoa B. Insights into teleost interferon-gamma biology: An update. Fish Shellfish Immunol (2019) 90:150–64. doi: 10.1016/j.fsi.2019.04.002
41. Solbakken MH, Jentoft S, Reitan T, Mikkelsen H, Gregers TF, Bakke O, et al. Disentangling the immune response and host-pathogen interactions in Francisella noatunensis infected Atlantic cod. Comp Biochem Physiol Part D: Genomics Proteomics (2019) 30:333–46. doi: 10.1016/j.cbd.2019.04.004
42. Pasquier LD. Germline and somatic diversification of immune recognition elements in Metazoa. Immunol Lett (2006) 104(1):2–17. doi: 10.1016/j.imlet.2005.11.022
43. Sorensen KK, Melkko J, Smedsrod B. Scavenger-receptor-mediated endocytosis in endocardial endothelial cells of Atlantic cod Gadus morhua. J Exp Biol (1998) 201(Pt 11):1707–18.
44. Nellimarla S, Baid K, Loo Y-M, Gale M, Bowdish DM, Mossman KL. Class A scavenger receptor-mediated dsRNA internalization is independent of innate antiviral signaling and does not require PI3K activity(). J Immunol (Baltimore Md 1950) (2015) 195(8):3858–65. doi: 10.4049/jimmunol.1501028
45. Palchetti S, Starace D, De Cesaris P, Filippini A, Ziparo E, Riccioli A. Transfected Poly(I:C) Activates Different dsRNA Receptors, Leading to Apoptosis or Immunoadjuvant Response in Androgen-independent Prostate Cancer Cells. J Biol Chem (2015) 290(9):5470–83. doi: 10.1074/jbc.M114.601625
46. Kaparakis M, Turnbull L, Carneiro L, Firth S, Coleman HA, Parkington HC, et al. Bacterial membrane vesicles deliver peptidoglycan to NOD1 in epithelial cells. Cell Microbiol (2010) 12(3):372–85. doi: 10.1111/j.1462-5822.2009.01404.x
47. Nakamura N, Lill JR, Phung Q, Jiang Z, Bakalarski C, de Maziere A, et al. Endosomes are specialized platforms for bacterial sensing and NOD2 signalling. Nature (2014) 509(7499):240–4. doi: 10.1038/nature13133
48. Irving AT, Mimuro H, Kufer TA, Lo C, Wheeler R, Turner LJ, et al. The immune receptor NOD1 and kinase RIP2 interact with bacterial peptidoglycan on early endosomes to promote autophagy and inflammatory signaling. Cell Host Microbe (2014) 15(5):623–35. doi: 10.1016/j.chom.2014.04.001
49. Bonham KS, Kagan JC. Endosomes as Platforms for NOD-like Receptor Signaling. Cell Host Microbe (2014) 15(5):523–5. doi: 10.1016/j.chom.2014.05.001
50. Caruso R, Warner N, Inohara N, Nunez G. NOD1 and NOD2: signaling, host defense, and inflammatory disease. Immunity (2014) 41(6):898–908. doi: 10.1016/j.immuni.2014.12.010
51. Li H, Jin H, Li Y, Liu D, Foda MF, Jiang Y, et al. Molecular cloning and functional characterization of duck nucleotide-binding oligomerization domain 1 (NOD1). Dev Comp Immunol (2017) 74:82–9. doi: 10.1016/j.dci.2017.04.012
52. Tao Z, Zhu C, Song W, Xu W, Zhang S, Liu H, et al. Inductive expression of the NOD1 signalling pathway in chickens infected with Salmonella pullorum. Br Poultry Sci (2017) 58(3):242–50. doi: 10.1080/00071668.2017.1280771
53. Hu YW, Wu XM, Ren SS, Cao L, Nie P, Chang MX. NOD1 deficiency impairs CD44a/Lck as well as PI3K/Akt pathway. Sci Rep (2017) 7(1):2979. doi: 10.1038/s41598-017-03258-y
54. Lamkanfi M, Dixit VM. Inflammasomes: guardians of cytosolic sanctity. Immunol Rev (2009) 227(1):95–105. doi: 10.1111/j.1600-065X.2008.00730.x
55. Li J-Y, Gao K, Shao T, Fan D-D, Hu C-B, Sun C-C, et al. Characterization of an NLRP1 Inflammasome from Zebrafish Reveals a Unique Sequential Activation Mechanism Underlying Inflammatory Caspases in Ancient Vertebrates. J Immunol (2018) 201(7):1946. doi: 10.4049/jimmunol.1800498
56. Takeuchi O, Akira S. Pattern Recognition Receptors and Inflammation. Cell (2010) 140(6):805–20. doi: 10.1016/j.cell.2010.01.022
57. Newton K, Dixit VM. Signaling in innate immunity and inflammation. Cold Spring Harbor Perspect Biol (2012) 4(3):a006049. doi: 10.1101/cshperspect.a006049
58. Iliev DB RJ, Mackenzie S, Planas JV, Goetz FW. Endotoxin recognition: in fish or not in fish? FEBS Lett (2005) 579(29):6519–28. doi: 10.1016/j.febslet.2005.10.061
59. Turcotte K, Gauthier S, Malo D, Tam M, Stevenson MM, Gros P. Icsbp1/IRF-8 Is Required for Innate and Adaptive Immune Responses against Intracellular Pathogens. J Immunol (2007) 179(4):2467–76. doi: 10.4049/jimmunol.179.4.2467
60. Marquis J-F, Kapoustina O, Langlais D, Ruddy R, Dufour CR, Kim B-H, et al. Interferon Regulatory Factor 8 Regulates Pathways for Antigen Presentation in Myeloid Cells and during Tuberculosis. PloS Genet (2011) 7(6):e1002097. doi: 10.1371/journal.pgen.1002097
61. Berghout J, Langlais D, Radovanovic I, Tam M, MacMicking JD, Stevenson MM, et al. Irf8-Regulated Genomic Responses Drive Pathological Inflammation during Cerebral Malaria. PloS Pathog (2013) 9(7):e1003491. doi: 10.1371/journal.ppat.1003491
62. Malmstrøm M, Jentoft S, Gregers TF, Jakobsen KS. Unraveling the Evolution of the Atlantic Cod’s (Gadus morhua L.) Alternative Immune Strategy. PloS One (2013) 8(9):e74004. doi: 10.1371/journal.pone.0074004
63. Howe K, Schiffer PH, Zielinski J, Wiehe T, Laird GK, Marioni JC, et al. Structure and evolutionary history of a large family of NLR proteins in the zebrafish. Open Biol (2016) 6(4):160009. doi: 10.1098/rsob.160009
64. Tørresen OK, Rise ML, Jin X, Star B, MacKenzie S, Jakobsen KS, et al. 3 - An improved version of the Atlantic cod genome and advancements in functional genomics: implications for the future of cod farming. In: MacKenzie S, Jentoft S, editors. Genomics in Aquaculture. San Diego: Academic Press (2016). p. 45–72. doi: 10.1016/B978-0-12-801418-9.00003-2
65. Motta V, Soares F, Sun T, Philpott DJ. NOD-like receptors: versatile cytosolic sentinels. Physiol Rev (2015) 95(1):149–78. doi: 10.1152/physrev.00009.2014
66. Eslamloo K, Ghorbani A, Xue X, Inkpen SM, Larijani M, Rise ML. Characterization and Transcript Expression Analyses of Atlantic Cod Viperin. Front Immunol (2019) 10(311):311. doi: 10.3389/fimmu.2019.00311
67. Solbakken MH, Jentoft S, Reitan T, Mikkelsen H, Jakobsen KS, Seppola M. Whole transcriptome analysis of the Atlantic cod vaccine response reveals subtle changes in adaptive immunity. Comp Biochem Physiol Part D: Genomics Proteomics (2019) 31:100597. doi: 10.1016/j.cbd.2019.100597
Keywords: cod, immune response, nucleotide-binding oligomerization-like receptor (NLR), macrophage, Toll-like receptor (TLR)
Citation: Jin X, Morro B, Tørresen OK, Moiche V, Solbakken MH, Jakobsen KS, Jentoft S and MacKenzie S (2020) Innovation in Nucleotide-Binding Oligomerization-Like Receptor and Toll-Like Receptor Sensing Drives the Major Histocompatibility Complex-II Free Atlantic Cod Immune System. Front. Immunol. 11:609456. doi: 10.3389/fimmu.2020.609456
Received: 23 September 2020; Accepted: 09 November 2020;
Published: 11 December 2020.
Edited by:
Jun-ichi Hikima, University of Miyazaki, JapanReviewed by:
Jorge Galindo-Villegas, Nord University, NorwaySarah J. Poynter, University of Waterloo, Canada
Copyright © 2020 Jin, Morro, Tørresen, Moiche, Solbakken, Jakobsen, Jentoft and MacKenzie. This is an open-access article distributed under the terms of the Creative Commons Attribution License (CC BY). The use, distribution or reproduction in other forums is permitted, provided the original author(s) and the copyright owner(s) are credited and that the original publication in this journal is cited, in accordance with accepted academic practice. No use, distribution or reproduction is permitted which does not comply with these terms.
*Correspondence: Sissel Jentoft, c2lzc2VsLmplbnRvZnRAaWJ2LnVpby5ubw==; Simon MacKenzie, c2ltb24ubWFja2VuemllQHN0aXIuYWMudWs=