- 1Animal Health Department, NEIKER-Basque Institute for Agricultural Research and Development-Basque Research and Technology Allianca (BRTA), Derio, Spain
- 2Food Quality and Safety Department, Universidad del País Vasco/Euskal Herriko Unibertsitatea (UPV/EHU), Vitoria-Gasteiz, Spain
- 3Molecular Immunology Laboratory, Department of Pathobiology and Population Sciences, Royal Veterinary College, Hatfield, United Kingdom
- 4Veterinary Practitioner, Gernika, Spain
- 5Inflammation and Macrophage Plasticity Laboratory, Centro de Investigaciones Cooperativas (CIC) bioGUNE-Basque Research and Technology Alliance (BRTA), Derio, Spain
- 6Basque Foundation for Science, Bilbao, Spain
Mycobacterium avium subsp. paratuberculosis (Map) is the underlying pathogen causing bovine paratuberculosis (PTB), an enteric granulomatous disease that mainly affects ruminants and for which an effective treatment is needed. Macrophages are the primary target cells for Map, which survives and replicates intracellularly by inhibiting phagosome maturation. Neutrophils are present at disease sites during the early stages of the infection, but seem to be absent in the late stage, in contrast to healthy tissue. Although neutrophil activity has been reported to be impaired following Map infection, their role in PTB pathogenesis has not been fully defined. Neutrophils are capable of releasing extracellular traps consisting of extruded DNA and proteins that immobilize and kill microorganisms, but this mechanism has not been evaluated against Map. Our main objective was to study the interaction of neutrophils with macrophages during an in vitro mycobacterial infection. For this purpose, neutrophils and macrophages from the same animal were cultured alone or together in the presence of Map or Mycobacterium bovis Bacillus-Calmette-Guérin (BCG). Extracellular trap release, mycobacteria killing as well as IL-1β and IL-8 release were assessed. Neutrophils released extracellular traps against mycobacteria when cultured alone and in the presence of macrophages without direct cell contact, but resulted inhibited in direct contact. Macrophages were extremely efficient at killing BCG, but ineffective at killing Map. In contrast, neutrophils showed similar killing rates for both mycobacteria. Co-cultures infected with Map showed the expected killing effect of combining both cell types, whereas co-cultures infected with BCG showed a potentiated killing effect beyond the expected one, indicating a potential synergistic cooperation. In both cases, IL-1β and IL-8 levels were lower in co-cultures, suggestive of a reduced inflammatory reaction. These data indicate that cooperation of both cell types can be beneficial in terms of decreasing the inflammatory reaction while the effective elimination of Map can be compromised. These results suggest that neutrophils are effective at Map killing and can exert protective mechanisms against Map that seem to fail during PTB disease after the arrival of macrophages at the infection site.
Introduction
Mycobacterium avium subsp. paratuberculosis (Map) is the etiological agent of paratuberculosis (PTB; Johne’s disease), a chronic granulomatous enteritis of ruminants characterized by cachexia and severe diarrhea. It results in production losses due to subclinical infection and animal losses due to clinically active disease (1). Infected animals in the subclinical stage shed Map intermittently to the environment, making its control difficult. Vaccination against PTB has been shown to reduce shedding and therefore in-herd transmission (2, 3). However, vaccination does not provide complete protection from infection (4) and due to the nature of Map antigens, it interferes with the official tests for bovine tuberculosis (bTB) eradication program.
Classically, PTB research has been focused on the action of macrophages (MΦ) as the main target cells. After infection, Map is able to survive and replicate intracellularly by inhibiting phagosome maturation. However, less attention has been paid to the role that polymorphonuclear neutrophils (PMN) may play in this process, although their presence is undeniable especially during the early stages of the infection (5). PMNs are short-lived effector cells capable of phagocytosing pathogens, killing microbes through the production of reactive oxygen species (ROS), lytic enzymes with potent antimicrobial activity (6) as well as the release of extracellular traps (ETs) (7, 8).
ET formation has been demonstrated as an important novel effector mechanism against pathogens in PMNs (8) and MΦs (9). ETs are extracellular structures composed of chromatin and granule proteins that trap and kill microorganisms (10). Their formation is triggered by pathogens, danger associated molecular patterns, exogenous compounds, platelets, antibodies and inflammatory cytokines such as IL-8 and TNF (11, 12). MΦ have been reported to produce ETs in response to Staphylococcus aureus (13), Mycobacterium tuberculosis (Mtb) (14) and the parasite Besnoitia besnoiti (15). However, this antimicrobial mechanism does not seem to be dominant in MΦs. It is more likely that the main function of MΦs in this context is to clear ETs produced by PMNs and the resulting apoptotic PMNs rather than to produce ETs themselves (16).
Indeed, several studies have described the cooperation of PMNs and MΦs to remove pathogens at the inflammation site (17, 18), with apoptotic PMNs being phagocytosed by MΦs in a process called efferocytosis, which can potentially result in cross-presentation. This mechanism provides MΦs with antimicrobial molecules that are lacking in mature MΦs and that aid the killing of intracellular pathogens (18, 19). PMNs also transfer ingested intracellular pathogens to MΦs during efferocytosis (18).
In addition to PMNs and MΦs, the pro-inflammatory cytokines IL-1β and IL-8 are also essential components in the formation of granulomas in mycobacterial infections. IL-1β is a key mediator of the innate inflammatory response that attracts further MΦ (20) and leads to a general activation of the immune system. Furthermore, IL-1β seems to play an important role in protecting mice against experimental Map infection (21). Although essential for resistance to infection, IL-1β also exacerbates damage during chronic diseases and acute injuries. Its induction by Map may result in attraction of MΦ to the site of infection, thus ensuring its survival and dissemination (22), and this process may further be aided by the release of IL-8 by PMN. IL-8 plays an important role in leukocyte recruitment, granuloma formation, and respiratory burst in response to Mycobacterium tuberculosis (Mtb), leading to enhanced phagocytosis and killing of Mtb (23), and it is considered as a major ET inducer for PMNs (24).
There is little research on the protective role of PMNs in Map infection in cattle. PMN isolated from cattle infected sub-clinically with Map showed diminished migratory properties when stimulated in vitro compared to PMNs of healthy cattle (25), suggesting that Map infection potentially undermines PMN functionality. Indeed, PMNs seem to be absent in tissues from cows with clinical PTB (26). In the last years, several transcriptome studies have supported the idea that Map infection causes an impairment of PMN recruitment (27, 28) and the downregulation of antimicrobial peptide production by PMNs, such as those of beta-defensins (29) and cathelicidins (28). A recent study has shown that cathelicidin LL-37 produced by human PMNs restricts Mtb growth (30) and facilitates Map clearance in murine MΦs by suppressing the production of tissue-damaging inflammatory cytokines such as IL-8, TNF and IFN-γ (31). Taken together, these studies suggest that PMNs may play a more important role than previously thought during the early stages of Map infection. Thus, studying this immune cell type during Map infection may help to identify novel intervention strategies.
In order to gain knowledge on PMNs and Map, we studied the interaction of bovine monocyte-derived MΦ (MDM) and PMNs, either alone or in co-cultures, infected with Map and BCG, by evaluating ET formation, bacterial killing and pro-inflammatory cytokine production. To our knowledge, this is the first study to investigate ET release in response to Map exposure.
Materials and Methods
Animals
Blood samples were drawn from healthy Holstein Friesian cows of a commercial dairy farm located in the Basque Country. The herd is enrolled in the national bTB eradication program. Animals on the farm tested negative for bTb by skin-test over the last five years. All animals used in this study (n=4) tested negative for Map shedding in feces, as confirmed by PCR, and were negative for Map-specific antibodies to Map, as determined by PPA-3 ELISA.
Animals used in this study were submitted only to procedures that according to European (Directive 2010/63/EU of the European Parliament and of the Council of 22 September 2010 on the protection of animals used for scientific purposes. Chapter 1, Article 1, Section 5, paragraphs b and f) and Spanish (Real Decreto 53/2013, de 1 de febrero, por el que se establecen las normas básicas aplicables para la protección de los animales utilizados en experimentación y otros fines científicos, incluyendo la docencia, Article 2, Section 5, Paragraphs b and f) legislation on experimental animals are exempt from its application. The animals, belonging to a registered commercial farm supervised by the local livestock authority (Servicio de Ganadería de la Diputación Foral de Bizkaia) were submitted only to the introduction of a needle in accordance with good veterinary practice and were not killed in relationship with this study.
Bovine Peripheral Blood Monocyte Isolation and Generation of Macrophages
Blood was taken from the jugular vein using a 16G x 11/2 hypodermic needle into a blood-collection bag containing 63 mL of citrate phosphate dextrose adenine (CPDA; TerumoBCT Teruflex) for a total capacity of 450 mL, which was used for monocyte isolation. One month later, 40 mL of blood from the same cows were collected with a 18G hypodermic needle into a 50 mL tube containing 7 mL of CPDA for PMN isolation. Blood samples were processed within two hours of the extraction.
Peripheral blood mononuclear cells (PBMCs) were separated using Histopaque 1077® and monocytes were selected using mouse anti-human CD14-coupled microbeads and MS columns (Miltenyi) following the manufacturer’s recommendations. Greater than 90% of purity of monocytes was achieved as determined by flow cytometry using a mouse anti-bovine CD14 FITC-labelled antibody (BioRad).
For the production of monocyte-derived macrophages (MDMs), monocytes were plated at 1x105 cells/well for co-cultures and at 2x105 cells/well for MDM cultures in wells of 96-well plates for fluorimetric assays to quantify ET release. For immunofluorescence microscopy, monocytes were plated at 1x105 cells/well for co-cultures and at 2x105 cells/well for MDM cultures on 16-well chamber slides (178599PK Thermo Scientific). For contact independent cultures, monocytes were plated at 5x105 cells/well for co-cultures and at 1x106 cells/well in wells of 24-well plates. During maturation to MΦs, monocytes were cultured in RPMI 1640 media without phenol red (Gibco 11835030), supplemented with 2mM L-glutamine, 10% fetal bovine serum (FBS), 1% penicillin-streptomycin and 10 ng/mL recombinant bovine M-CSF (Kingfisher Biotech, Inc. RP1353B) at 37°C and 5% CO2. The media was replaced every three days and prior to the beginning of the mycobacterial infection assays. After 7 days of culture, MΦ morphology was confirmed by light microscopy (increased size, increased adherence, cytoplasmic granularity and presence of pseudopods).
Bovine Neutrophil Isolation
A week after PBMC isolation, 25 mL of blood were drawn from the same cows by venepuncture and mixed with 25 mL of PBS supplemented with 2% FBS. 25 mL of the mixture were layered on top of 15 mL of Histopaque® 1077 (Sigma) and centrifuged at 1200 x g for 45 min at RT with no brakes. PMNs were located at the lower end of the tube and were subjected to two flash hypotonic lysis using 25 mL of sterile distilled water during 20-30 sec, followed by 2.5 mL of a 10% NaCl solution and topped up with PBS-2% FBS, in order to eliminate remaining red blood cells. PMNs were then resuspended in RPMI 1640 with glutamine and without phenol red and counted. Over 90% purity was achieved as determined by FSC and SSC gating and visualization of characteristic nuclei after DAPI staining under a fluorescence microscope.
Bacterial Strains and Culture
Map strains K10, Map K10-GFP (kindly provided by Dr. Jeroen de Buck, University of Calgary (32) M.bovis BCG Danish strain 1331 and M. bovis BCG Danish strain 1331-GFP were used in the assays. All strains were grown to exponential phase at 37°C under aerobic conditions. Map-GFP and BCG-GFP were cultured for three weeks on Middlebrook 7H9 OADC with Kanamycin (25μg/mL) to select for GFP-plasmid carriers and supplementation with Mycobactin J was added for Map. Map strain K10 and BCG Danish strain 1331 were used for ET isolation and quantification and were cultured in the same medium without kanamycin. Cultures were adjusted after reaching an OD of 0.7 (3x108 bacteria/mL) and colony forming units (CFU) were confirmed on agar after using 10-fold serial dilutions of bacterial suspensions. In experiments comparing the response to live and killed bacteria, heat-inactivation was performed by heating bacteria for 30 min at 85°C. Inactivation of bacteria was confirmed by plating on agar plates.
Cell Cultures and In Vitro Infection
PMNs and MDMs isolated from the same cow were cultured separately or co-cultured together in the same well (CC; see Figures 1A and 3A for details). In order to study cellular changes following paracrine signaling in the absence of cell-to-cell contact between PMNs and MDMs as well as to determine ET formation, a transwell co-culture system was also employed. PMNs were seeded in the transwell insert (pore diameter 0.4 μm; Thermo Fisher Scientific 140620) to generate insert PMNs (TW-PMN), whereas MDMs were seeded into the lower well (TW-MDM). To assess the need for direct cell contact, conditioned MDM media (TW-CM) was produced by incubating MDMs with Map, BCG and Zymosan for 4h at 37°C and 5% CO2, which was subsequently added to the lower well underneath the insert containing PMNs (TW-PMN-CM). ET release quantification and mycobacteria killing were performed on 24 and 96 well plates. ET visualization was performed on 16-well chamber slides (Nunc Labtek).
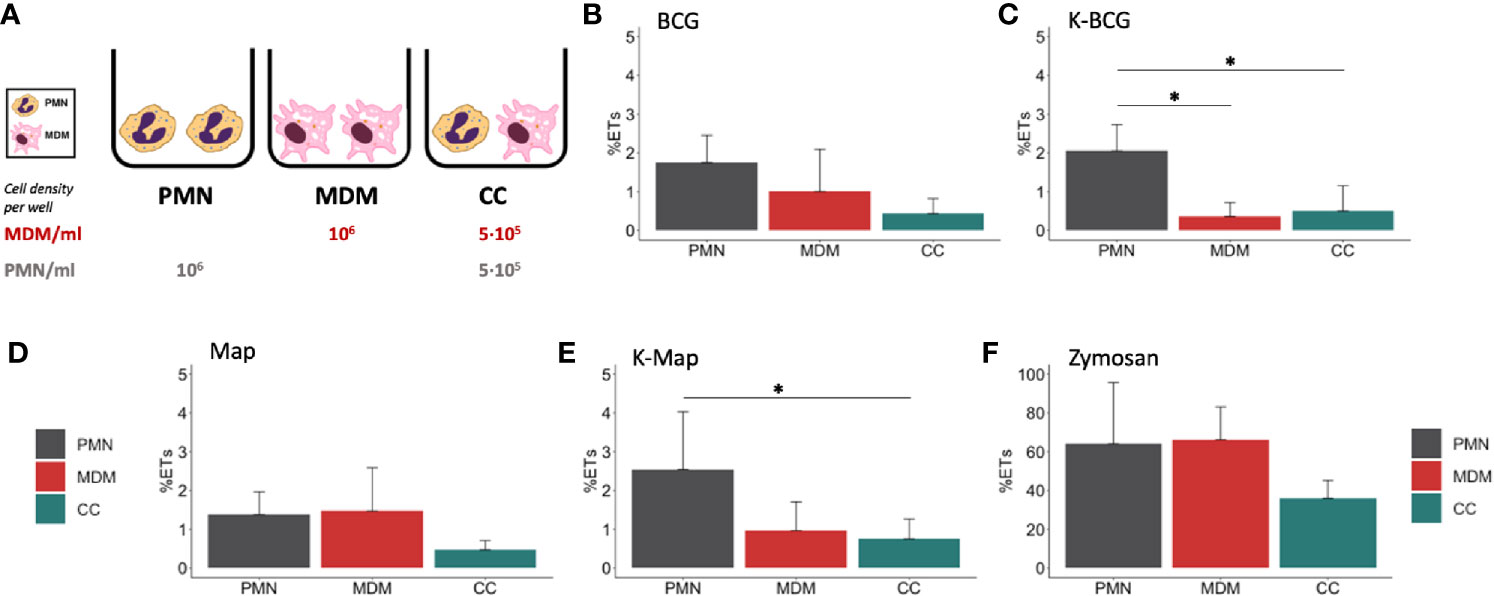
Figure 1 Fluorimetric ET release quantification of PMNs, MDMs and CCs. (A) Cell culture types and conditions in 96 well plates. ET release quantified by fluorimetry of cultures stimulated with (B) BCG, (C) K-BCG, killed BCG, (D) Map, (E) K-Map, killed Map and (F) Zymosan. Data are composed of combined values obtained of all cows (n=4), with samples run in triplicates. * depicts a p value of p < 0.05.
Once cell cultures were set, these were stimulated with live or heat-inactivated Map K10-GFP, M. bovis BCG -GFP, Map K10, M. bovis BCG at either a MOI of 1 (ET release) or a MOI of 5 (survival assay), zymosan (1mg/mL; Sigma Z4250), or left untreated (negative control). The original bacterial strains with no GFP were used for the isolation and quantification of NETs in the fluorometric assay to avoid GFP fluorescence interference.
Cultures were incubated at 37°C and 5% CO2 for 4h for ET release evaluation and for 24h for bacteria killing and cytokine level quantification.
Isolation and Quantification of ETs
ET release was assayed as described by Köckritz-Blickwede et al. (33) with some modifications. Briefly, ETs generated by cells were digested with 500 mU/mL of micrococcal nuclease (10107921001 Roche) for 10 mins at 37°C 5% CO2. The nuclease activity was stopped by the addition of 5 mM EDTA; thereafter, culture supernatants were collected and stored at 4°C overnight. Total DNA was extracted from naive cells with DNeasy® blood & tissue kit (Qiagen) following the manufacturer’s instructions. Extracted DNA was solubilized in TE buffer. Both ETs and genomic DNA was quantified using Quant-iT™ PicoGreen™ assay (Thermofisher) according to the manufacturer´s instructions. Plates were read in a fluorescence microplate reader (Synery HTX, Biotek) with filter settings at 488 nm excitation and 520 nm emission. The percentage of DNA released as ET-DNA was calculated by dividing the amount of quantified ET-DNA by the total amount of genomic DNA.
Visualization and Quantification of ET by Immunofluorescence Microscopy
To assess histone staining as a marker for ET formation, cells were seeded into 16-well chamber slides for subsequent antibody staining and microscopy according to Conejeros et al. (34). After 4 h of incubation with either Map-GFP, BCG-GFP or zymosan, cells were fixed for 15 min with 4% formaldehyde. Cells were washed twice with PBS and kept in PBS at 4°C until staining was performed. Subsequently, cells were permeabilized for 15 min with PBS 0.1% Triton X-100, blocked with 1% goat serum containing 0.05% Tween 20 and 3% BSA-PBS. The immunofluorescence staining was performed overnight at 4°C using a mouse anti-pan-histone antibody (Merk MAB3422; diluted 1:200). Thereafter, cells were washed three-times with PBS and incubated with anti-mouse Alexa Fluor 594 labelled antibody (Invitrogen A-1105, diluted 1:500) for 30 min at RT. Following further three washes with PBS, wells were detached from the slides and mounting medium containing DAPI was added before coverslips were added. Preparations were let dry and observed at 400x magnification on a DMi8 fluorescence inverted microscope (LEICA®). Pictures were taken using a DFC3000 G camera coupled to the microscope.
Quantification was performed by taking snapshots of five fields containing visible ETs with histone staining. Analysis was performed using the Image J software package. Snapshots in 8-bit format were analyzed using the following pipeline. Threshold was adjusted depending on the micrograph, being 4 for low level and 255 for high level. The percentage of picture area occupied by ETs plus nuclei (total DNA) was measured by adjusting the settings for fluorescent particle size: 0-infinity and circularity: 0-1. The percentage of picture area occupied by nuclei were analyzed adjusting the settings for particle size: 200-5000 and circularity: 0.3-1. ET percentage was calculated as total DNA percentage minus nuclei percentage. In the case of cultures that did not contain ETs, nuclei were measured, and ET release was considered zero.
Mycobacteria Killing Assay
PMNs, MDMs and co-cultures inoculated with BCG -GFP and Map-GFP were prepared. At time 0 (4°C control) and 24 h, the supernatants were removed and centrifuged to pellet non-internalized mycobacteria. Supernatants were stored for cytokine analysis. Wells were washed twice with PBS to remove the remaining non-internalized bacteria. Bacterial pellets and washes of remaining bacteria were centrifuged together, and the final pellets were resuspended in 100 μL of filtered PBS. Adherent cells were lysed by vigorous pipetting with 0.5 mL of 0.1% Triton X-100 (Sigma-Aldrich) in sterile water for 10 min at RT.
Two serial 10-fold dilutions of each lysed sample and supernatant were performed in a total volume of 1 mL using filtered PBS. 200 μl of each dilution was inoculated in duplicate in Middlebrook 7H9 OADC-Kanamycin (25μg/mL) agar plates, supplemented with mycobactin J in the case of Map cultures. Seeded agar plates were allowed to dry at RT until humidity was no longer visible. Plates were sealed with tape to avoid desiccation and incubated at 37 ± 1°C for 6 weeks. CFU were counted and 0h (4°C control) CFU were considered total inoculated bacteria, 24h CFU supernatant bacteria were considered non-internalized and non-killed and 24h CFU cell bacteria were considered internalized and non-killed bacteria. Killed bacteria were estimated as total inoculated bacteria minus (24h CFU supernatant bacteria plus 24h CFU cell bacteria). CFU counts were multiplied by the inverse dilution factor and by the seeded volume. The mean of duplicate plates was calculated and the group mean for each culture type was calculated. Supernatants’ fraction and cell-lysis’ fractions were divided by the 100% survival control to calculate the survival percentage of each portion and the killing rate.
Cytokine Detection
Commercially available direct ELISA kits were performed to determine bovine IL-1β (ESS0027 Invitrogen®) and IL-8 (3114-1A-6 MABTECH) release in 24h culture supernatants using the provided manufacturer’s instructions. IL-1β ELISA is based on streptavidin-HRP and IL-8 on streptavidin-ALP. Absorbances were measured using an automated ELISA plate reader (Multiskan EX®, Thermo Lab Systems, Finland) and in all cases standard curves were used to determine each cytokine amount in the supernatant samples.
Statistics
Data were assessed for normal distribution and homoscedasticity using Shapiro-Wilk and Bartlett´s test, respectively. All data are presented as the mean +/- standard deviation (SD), with samples being analyzed in triplicates (ET release by fluorimetry) or duplicates (bacterial killing and cytokine release). For ET release, percentage differences between values obtained for the mean of each culture type were calculated using pairwise comparisons (t-tests) with pooled SD for all the stimuli except for values obtained for unstimulated and zymosan stimulated cultures. Here, values were not normally distributed and thus comparisons were calculated using non-pooled SD test.
The number of bacteria (expressed as CFU) in the inoculum (0 h) was considered as 100% viable bacteria. The number of bacteria being alive after 24 h incubation was calculated as the sum of internalized mycobacteria and mycobacteria being present in the supernatant, with the killing rate being the difference between the bacteria at 0h and the survival at 24 h, expressed as percentage. Mean values obtained for killing rates under different conditions were compared by multiple pairwise comparisons using t tests with non-pooled SD with Benjamini-Hochberg correction. To obtain killing rates for co-cultures, values obtained for the killing rate of individual cultures (PMN, MDM) were divided by two and obtained values were added to each other.
Differences in IL-1β levels were compared by Kruskal-Wallis test with the post-hoc Dunn’s multiple comparison test. IL-8 level differences were calculated through multiple pairwise comparisons using t tests with pooled SD.
To identify correlations between variables, Pearson (ρx,y) and Spearman (ρ) coefficients were calculated to detect linear and non-linear correlations and in each case the best fitting coefficient value was chosen.
All statistical tests were performed using R studio desktop (version 1.2.5033; (RStudio Team, 2019. RStudio: Integrated Development for R. RStudio, Inc., Boston, MA URL http://www.rstudio.com/), and a p-value of < 0.05 was considered statistically significant.
Results
PMNs Release Extracellular Traps Against Both Mycobacteria Killed and Alive in Contrast to MDMs
In a first set of experiments, we assessed the induction of ET in PMNs and MDMs, either alone or in co-cultures, in response to Map and BCG. In order to assess whether this mechanism would rely on live or heat-killed bacteria, all culture types were incubated with both live and heat-inactivated Map and BCG. Fluorometric analysis revealed that both cell types cultured individually extruded similar detectable DNA levels in response to zymosan (Figure 1F), whereas lower ET levels were detected when cells were cultured together (Figure 1F). Levels of ET formation in response to live mycobacteria were similar for PMNs and MDMs and lower for CCs although no statistical differences were found (Figures 1B, D). However, in response to killed mycobacteria, PMNs showed higher ET release (Figures 1C, E) compared to MDMs (K-BCG, p=0.03; K-Map, p=0.065) and to CC (K-BCG, p=0.04; K-Map, p=0.043).
PMNs and MDMs Alone or in Direct Co-Cultures Exert Different Effector Mechanisms Against BCG and Map
Although fluorometric quantification analysis revealed significant differences in ET release among cell culture types, further analyses were performed to discriminate between DNA from ET release and DNA liberated by other means. Image analysis quantification of ETs showed that PMNs liberated significantly higher levels of ETs (6.52% - 7.23%) compared to MDMs (0%) and CCs (1.13% - 2.03%) and MDMs (0%) when stimulated with both mycobacteria, independent of whether the bacteria were alive or heat-killed (Figures 2A–D). As seen before, MDMs produced ET-like structures only when stimulated with zymosan (Figure 2E). MDMs and PMNs, cultured either alone or in co-cultures and stimulated with zymosan lost normal nuclei structure, ending in nuclear DNA extrusion (Figure 2E). However, co-cultures seemed to contain fewer ETs, which could be due to MDMs phagocytosing liberated ETs by PMNs or because direct contact of both cell types inhibits ET release.
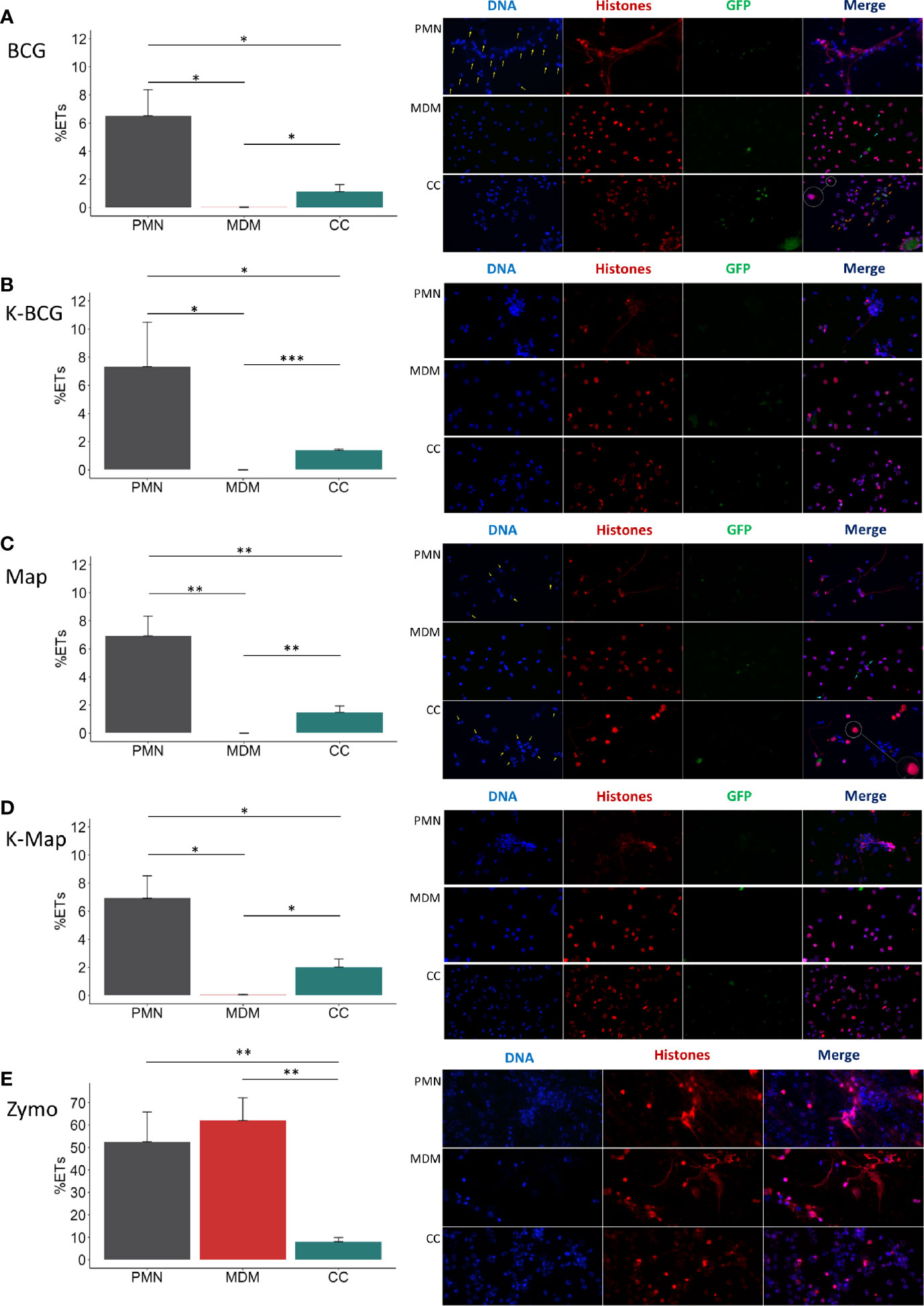
Figure 2 ET release analysis of microscope images. In the left of each section bar plots representing ET release quantified by immunofluorescence microscopy image analysis and in the right representative corresponding examples of micrographs (40x) of PMNs, MDMs and CCs stimulated with (A) BCG, (B) K-BCG: killed BCG, (C) Map, (D) K-Map: killed Map and (E) Zymosan. DAPI stained DNA in blue, histones in red and BCG-GFP or Map-GFP in green. Yellow arrows indicate nuclei stained with DAPI, which are absent in the red channel corresponding with anti-histone immunolabeling. Light-blue arrows indicate MDM phagocytosis. Orange arrows indicate PMN phagocytosis. A white circle has an augmented image showing a red punctate pattern in MDM cytoplasm. Data composed of combined values obtained of all cows (n=4), with samples run in duplicate and 5 fields quantified per micrograph. (*p < 0.05; ***p < 0.001).
The microscopic evaluations also revealed that ETs released by PMNs were the main source of histone-staining (shown in red), as this staining was mainly within the extruded ETs and not always associated with DAPI-stained nuclei (Figures 2A, C; PMNs and PMNs and CC). In agreement with data shown in Figure 1, MDMs showed internalized mycobacteria but did not show ET release (Figures 2A, C, MDMs), and anti-histone staining was always associated with the nuclei (as recognized by DAPI staining), independent of bacterial preparation used to stimulate MDM (Figures 2A–D; MDM). In addition, it seemed that MDMs showed less mycobacterial internalization when co-cultured with PMN, whereas the opposite was observed with PMNs (Figures 2A, C; CC). However, in Map stimulated CC, PMNs nuclei were not always labelled by the anti-histone antibody, and many PMNs showed condensed nuclei, potentially indicative of apoptosis (Figure 2C; CC). Furthermore, ETs were rarely detected and MDMs presented a red punctate pattern in the cytoplasm (Figures 2A, C; CCs) which could support the hypothesis that MDMs phagocytosed histones liberated by PMNs.
ET Release by PMNs in Response to Mycobacteria Is Not Affected by Stimulated MDMs Soluble Factors
Having established that both mycobacterial species induce ET formation in PMNs, but less so in MDMs or co-culture of both cells, we next assessed if ET release was influenced by secreted mediators or required direct cell-cell contact (Figure 3A). To further address the possible paracrine effect of MDMs, PMNs were also cultured in the transwell system (TW-PMN-CM) containing macrophage-conditioned media (CM) (Figure 3B). Additional controls included unstimulated and stimulated PMN kept in the transwell insert on their own (TW-CTRL and TW-PMN, respectively) to exclude an impact on ET release by the transwell culture condition compared to PMNs kept in the lower well (PMN) (see Figure 3A).
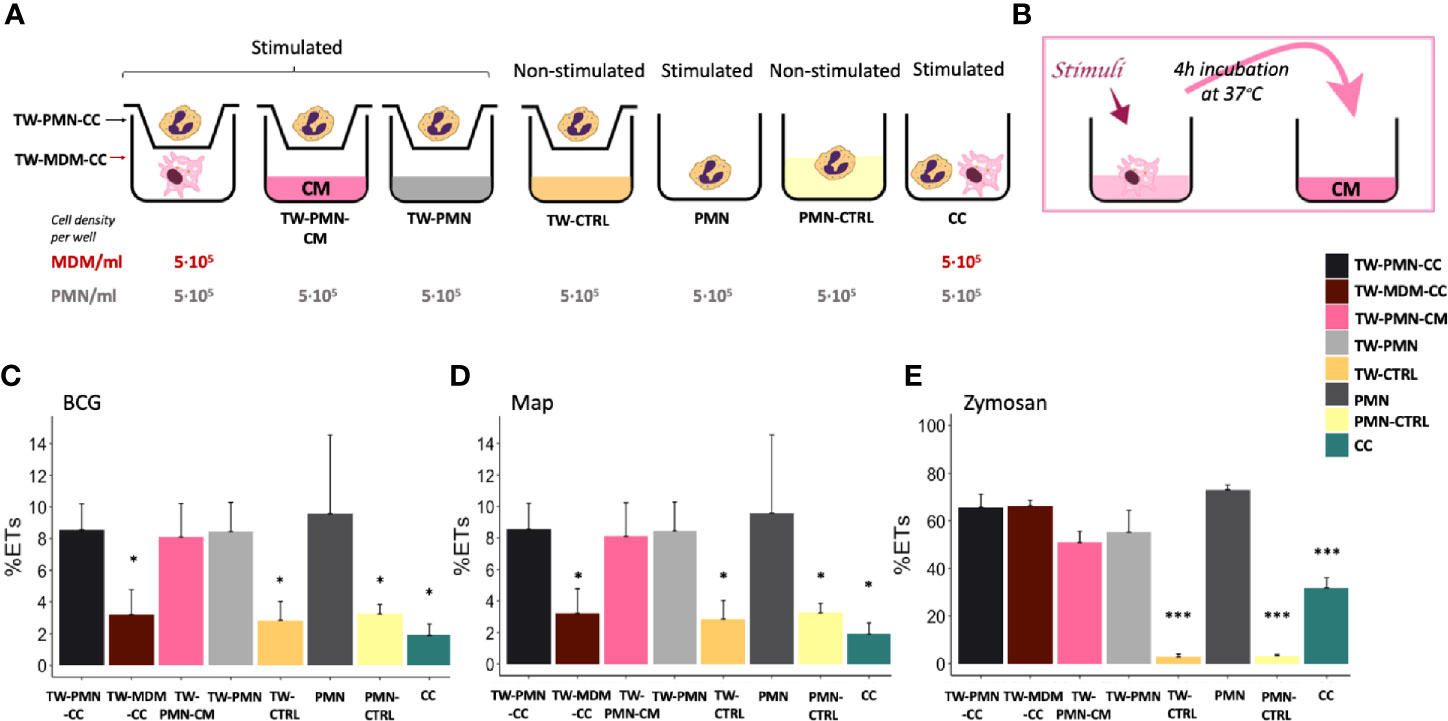
Figure 3 ET release quantified by fluorimetry of the transwell system experiment. (A) Culture types and conditions in 24 well plates. (B) Conditioned media preparation from stimulated macrophages for PMN stimulation. Fluorimetric quantification of ET release of (C) BCG, (D) Map and (E) Zymosan stimulated cultures. Data composed of combined values obtained of all cows (n=3), with samples run in duplicate (*p < 0.05, ***p < 0.001). TW, transwell, CC, co-culture; CM, conditioned media; CTRL, non-stimulated control.
PMNs incubated either in the transwell insert (TW-CTRL) or directly in the well (PMN-CTRL) showed no differences in baseline ET release, indicating that the culture conditions did not impact on ET release.
PMNs released equivalent levels of ETs in response to both mycobacteria when cultured either alone in the lower well (PMN) in the presence of MDM conditioned media (TW-PMN-CM), or cultured in the insert of the transwell (TW-PMN) (Figures 3C, D). In contrast, MDMs do not release ETs in transwell co-culture with PMNs when stimulated with mycobacteria (Figures 3C, D), confirming results obtained previously.
Direct cell contact induced the lowest ET release compared to the rest of the co-culture types independent whether cells were stimulated with either mycobacteria (p<0.05), and this effect was also present when cells were stimulated with zymosan (Figure 3E). Overall, the response to zymosan followed the response seen to either mycobacteria with the exemption of MDMs that showed a similar ET release compared to PMNs (60-70%) upon stimulation with zymosan.
PMNs Show Similar Killing Levels of Map and BCG, Whilst MDMs Are More Effective at Killing BCG
After demonstrating that ET release can be triggered by mycobacteria after 4 hours, we next assessed whether ET formation at an early time point may be beneficial in terms of bacterial clearance. For this purpose, the killing capacity of PMNs, MDMs and co-cultures against both mycobacteria after 24 h was quantified. Total inoculated CFU (0 h) and CFU recovered at 24h for all cultures are shown in Figures 4A, C. MDMs alone and CCs were more effective at killing BCG compared to PMNs alone, whereas PMNs were more effective at killing Map compared to MDMs.
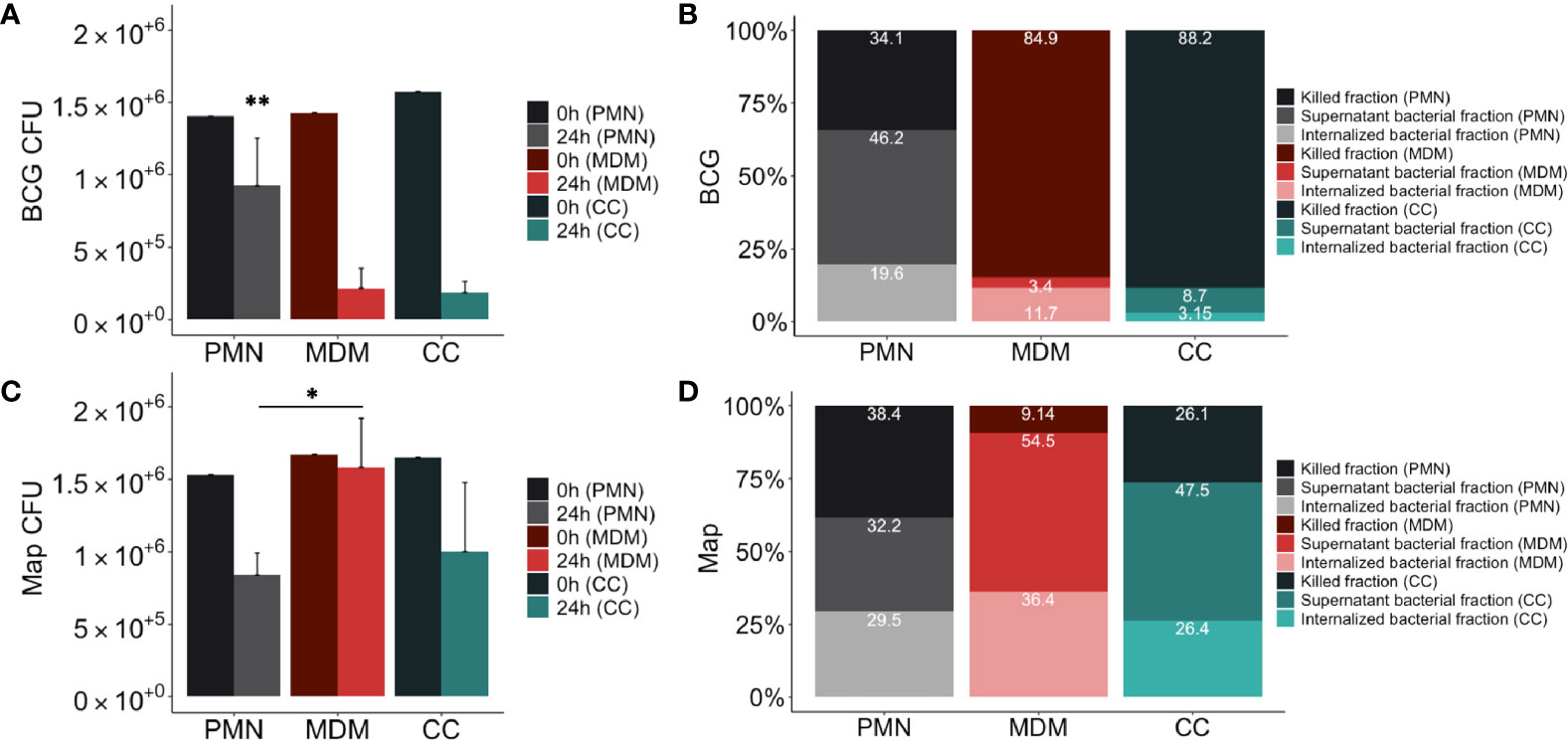
Figure 4 Mycobacterial killing assay results. Distribution of mycobacteria (A) total CFUs of BCG-GFP, (B) % BCG-GFP, (C) total CFUs Map-GFP and (D) % Map-GFP in 24h cultures of PMNs in grey, MDMs in red and in direct contact co-cultures (CC) in green. In (A, C) in the dark color the 0h control and in the light color the 24h assay of the corresponding culture type. In (B, D) in the darkest colors the fraction of killed bacteria, in the intermediate intensity colors the fraction of live bacteria non-internalized from the supernatant and in the lightest colors the proportion of internalized bacteria that survived inside the adherent cells of the corresponding culture type. Data composed of combined values obtained of all cows (n = 4), with samples run in duplicate (*p < 0.05, **p < 0.01).
The resulting distribution of live and killed BCG and Map in culture cells and supernatant is shown in Figures 4B, D. Statistical analysis revealed significant differences between MDMs, PMNs and CCs showing higher killing of BCG in MDM cultures compared to PMNs (84.85% vs 34.15%, p=0.0016), and higher Map killing in PMN cultures than in MDMs (38.36% vs 9.14%, p=0.045). PMN culture killing rate against BCG was lower than in co-cultures (34.14% vs 88.19%; p=0.001), whereas MDM culture killing rate against Map was lower compared to co-cultures (9.14% vs 26.10%; p=0.065), although it was not statistically significant. Considering that CCs include half of the amount of each cell type, and that each cell type shows a certain killing capacity in individual culture, a theorical killing rate for CCs against each mycobacterium was calculated by dividing each individual culture (PMN, MDM) killing rate by two and adding both obtained values. Results indicate that co-cultures infected with BCG showed improved killing results (expected killing: 59.49% vs observed killing: 88.19%) than individual cultures. In contrast, this synergic effect was not evident in co-cultures infected with Map, showing only 2.52% of improvement (expected killing: 23.58% vs observed killing: 26.1%).
When bacterial survival inside adherent cells of each cell-culture type was compared (Figures 4B, D), significant differences were not observed for either BCG or Map. However, non-internalized BCG survival in PMN cultures (46.23%) was higher than in MDM cultures (3.44%, p=0.021) and in co-cultures (8.65%, p=0.021), whereas non-internalized Map survival in PMN cultures was lower than in MDM cultures (54.46% vs 32.17%, p=0.03). Map-GFP survival inside adherent cells was higher than BCG-GFP as expected.
MDMs Alone Release Higher Cytokine Levels Compared to PMNs Independent of Culture Condition
Having assessed the killing capacity of both cell types to different mycobacterial strains, we next investigated whether their cytokine response follows a similar pattern. Considering that IL-8 is a major ET inducer in PMNs (23) and IL-1β is a key mediator of the inflammatory response that attracts MΦs to the infection site (22), we analysed the levels of both cytokines in the supernatant. IL-1β release after 24h showed significant differences between the cells involved as well as in response to both mycobacteria. MDM cultures released higher concentrations of IL-1β (Figure 5A) in response to BCG compared to PMN (p<0.001) and CC (p=0.009) and in response to Map compared to PMN (p=0.035). Furthermore, MDM released significantly more IL-1β in response to BCG compared to Map.
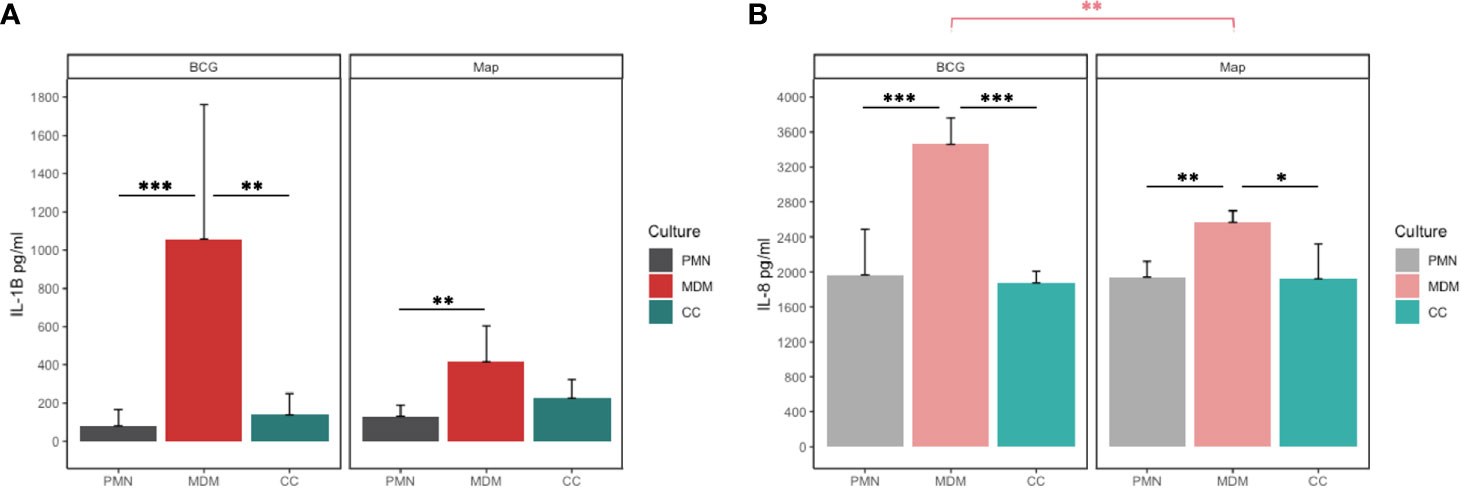
Figure 5 (A) IL-1β and (B) IL-8 release of PMN, MDM and CC stimulated with BCG and Map after 24h. Data composed of combined values obtained of all cows (n=4), with samples run in duplicate (*p < 0.05, **p < 0.01; ***p < 0.001).
Similar as seen for IL-1β, MDMs secreted more IL-8 into the supernatant in response to both mycobacteria (Figure 5B) compared to co-cultures (BCG; p< 0.001, Map; p=0.013) or PMNs alone (BCG; p=0.001, Map; p= 0.005). As before, MDMs challenged with BCG produced significantly more IL-8 than challenge with Map (p=0.011).
Correlation Analysis of Cytokine Production and Bacteria Killing Rates Suggest Different Mechanism Interplay Between MDMs and PMNs Against BCG and Map
Correlation analyses were performed between cytokine levels, and mycobacteria killing rates to evaluate the strength of relationship between both parameters (Table 1). The correlation study was performed including the data from all the culture types together and then subdivided by culture type and stimuli. IL-1β and IL-8 levels were positively correlated at 24h including all culture types in the analysis indicating that both cytokines are secreted in the same culture conditions in our assay. This correlation improved when eliminating the CCs from the analysis suggesting that cytokine release follows a different pattern in CCs maybe due to the difference in cell number. BCG-stimulated cultures showed a positive correlation between IL-1β and killing rate, which increased when eliminating CCs from the analysis. On the other hand, Map-stimulated cultures showed a negative correlation between IL-1β and killing rates, and in this case, power was increased when analysing PMNs alone. IL-8 and killing capacity were positively correlated in MDM cultures. IL-8 and killing rates were negatively correlated in Map-stimulated cultures.
Discussion
In the present study, we compared aspects of the interaction of bovine PMNs and MDMs in vitro with Map and M. bovis BCG, a better studied mycobacterium. Specifically, we assessed whether a cooperation of both innate immune cell types would be advantageous for the host in eliminating these mycobacteria by analysing ET release, pro-inflammatory cytokine levels and killing of mycobacteria. To the best of our knowledge, this is the first study describing ET release against Map.
Bovine PMNs and MDMs were cultured separately or together. PMNs showed higher ET release against killed mycobacteria than against live bacteria as well as in comparison to MDMs (Figure 2). This is in line with other observation that live mycobacteria contains glycolipids that act as antagonist to TLR2, thus inhibiting activation of innate immune cells, whereas heat-inactivation allowed for recognition by TLR2 and Mincle (35). Interestingly, activation of Mincle expressed on PMNs leads to NET formation (36). In contrast, direct interaction of MDMs and PMNs resulted in less ET release compared to PMNs cultured on their own or PMN cultured in a transwell system (see Figure 3). Indeed, this was observed for all direct co-cultures tested, independent of the stimuli tested and independent whether these were alive or inactivated. This generalized lower ET detection in direct co-cultures could be due to ET removal by efferocytosis, a process in which apoptotic cells (PMNs and/or MDMs) are removed by phagocytic cells (MDMs), to the dampening of PMN response by MDMs, or to the activation of PMNs by MDMs resulting in other effector mechanisms than the release of ET.
Thus, we believe that in situations of direct cell-to-cell contact, ET release would not be the principal mechanism exerted by PMNs, and this would be in line with our observation that PMNs in co-culture situation (CC) present more internalized BCG bacilli and less detectable ETs. In contrast, in Map stimulated CCs, PMNs appear to have condensed nuclei indicative of apoptosis and no detectable histones, rarely detectable ETs and few internalized Map bacilli, whereas MDMs have histones in their cytoplasm. Whether this is a direct effect of Map on PMNs, or a result of the co-culture to enable macrophages to take up PMNs remains to be investigated. Indeed, histones can be actively secreted into the extracellular space by activated inflammatory cells (37) and by ET release (38) or are derived more passively by apoptotic and necrotic cells (37). Inhibition of efferocytosis by extracellular histones has been reported in both in vitro and in vivo conditions (39). In our experimental conditions, we believe that in Map stimulated CCs, activated or apoptotic PMNs liberate histones alone or with ETs that are engulfed subsequently by MDMs, inhibiting efferocytosis and for this reason apoptotic PMNs are still present but ETs are not detectable.
With regards to the differences seen for bacterial killing, these were most pronounced against both mycobacteria in MDMs alone. MDMs were highly efficient in killing the attenuated M. bovis BCG, but they seemed to be unable to kill Map under the tested conditions. In contrast, PMNs showed a similar killing capacity against both mycobacteria species tested. Interestingly though, when both immune cell types were co-cultured in a 1:1 ratio, the killing capacity was unaltered with regards to BCG, but was similar to that obtained in PMNs for Map. Nevertheless, taking into account that CCs are composed by half of each cell type compared to MDM or PMN cultures, the obtained CC results indicate that a synergistic effect (observed killing > expected killing) in terms of improved killing capacity in BCG-infected co-cultures is observed, whereas in Map-infected co-cultures the effect was additive (observed killing = expected killing). Thus, the presence of PMNs at the site of infection during the initial stages of Map may be beneficial for the resolution of the disease. Indeed, several studies (40, 41) demonstrated that human PMNs are capable of controlling Mtb infection in vitro and murine PMNs have been shown to kill Mycobacterium avium ex vivo when activated in vivo with G-CSF (42).
In contrast to our data regarding ET formation and killing, MDMs produced higher cytokine levels compared to PMNs and CCs in response to both mycobacteria. Cytokine levels of co-cultures were lower than expected, resulting equivalent to those obtained in cultures of PMNs alone. A study by Sawant and McMurray showed an additive effect of IL-8 and IL-1β release in co-cultures of guinea pig PMNs with alveolar MΦs challenged in vitro with Mtb (43). It is likely that those results were obtained because their co-cultures harboured PMNs and MΦs in a 1:1 ratio, but doubling the total amount of cells, whereas our study halves the amount of each cell type to preserve the total cell number. In addition, differences on host and mycobacteria species and MDM sources (lung isolated cells in their case and peripheral isolated cells in our case), make a comparison between both studies difficult.
However, it is also possible that anti-inflammatory mediators released by PMNs act in an autocrine/paracrine manner, suppressing the production of pro-inflammatory cytokines by MDM, similar as described before (44). Indeed, the high IL-1β and IL-1α levels described by others in PTB (45) could be a result of the activation and recruitment impairment of PMN (27–29, 46, 47) and the resulting low numbers of PMN associated to PTB granulomatous lesions (48, 49). Moreover, high levels of these cytokines are proposed as candidates responsible for PTB inflammation process and contributors to the development of the Th17 response during the final stages of the disease (50).
Similarly, IL-8 expression has also been described to increase in PTB and monocytes stimulated with Map (51, 52) compared to controls. In our study IL-8 secretion of the same individuals against BCG and Map was compared. The lower IL-8 release of Map versus BCG stimulated MDMs could be a reflection of higher pathogenicity of Map in MDMs. In line with this observation is the fact that multi-drug resistant Mtb strains decreased release of IL-8 by infected bronchial epithelial cells, thus limiting PMN recruitment to the site of infection (53).
When correlating cytokine release with killing, we observed a positive correlation with the attenuated M. bovis BCG strain, being higher in MDMs, whereas the response to Map infection was negatively correlated with killing, showing a stronger effect in PMNs. Low IL-8 levels have been associated to poor prognosis in hTB (23) and survival of Mtb inside MΦ by altering PMN effector functions (53). In the present experiments, IL-8 levels were high in PMN, MDM and co-cultures, indicating that despite the negative correlation with Map killing, more factors are highly likely to be involved in this interaction. As for IL-1β, previous studies in IL-1 knock-out mice showed an increased susceptibility to Mtb infection, developing a higher bacterial burden and mortality (54). With this in mind, we would have expected that the lowest mycobacterial survival rate would be seen in the cultures with the highest levels of IL-1β. Whereas this was the case for BCG, it was not the case of Map-stimulated MDM cultures, indicating once again that more factors are involved. In fact, in vitro studies indicate that Map infection promotes a self-destruction state in the epithelium caused by increased IL-1β levels that attract MΦ, thus providing Map with an escape route from destruction (22).
Based on the results obtained in our experiments, we hypothesize that bovine PMNs may have an important role against Map. PMNs trigger inflammatory responses through IL-1β release and start an effective response phagocytosing and immobilizing bacteria with their NETs. As a consequence, MΦs are activated and attracted by IL-1β production to the site of infection. Upon arrival, MΦs would phagocytose neutrophils’ ETs and apoptotic rests, which provides them with the antimicrobial compounds produced by neutrophils after first contact with Map. This efferocytosis activity, as part of the homeostasis process, would reduce the inflammatory response leaded by IL-1β, IL-23 and IL-17 expression, ending ultimately in Map destruction. The timing is probably important in this cooperation and if MΦs arrive at the infection site too early, PMNs might not have enough time to effectively expand their antimicrobial mechanisms, MΦs will be the dominant cell type attempting Map elimination but end-up being taken over by Map as a Trojan horse. PMNs have been postulated as innate effectors of TB resistance due to their highly effective antimicrobial effector mechanisms they can expand during the early stage of Mtb infection (55). Applied to Map, it could be that harboring a particular number of competent and tightly regulated PMNs capable of expanding the correct antimicrobial mechanism at the specific time may be the difference between being resistant to PTB or developing subclinical or clinical disease.
Conclusions
Taking all assayed parameters into consideration, we could conclude that cooperation of MDMs and PMNs may confer advantages through different mechanisms depending on the pathogen. As for BCG, co-cultures show good levels of elimination of the mycobacteria (high killing rates) and lower cytokine levels and ET release than the PMN and MDM cultures, which, could translate to a decreased tissue damage in the living organism, since an excessive inflammatory reaction would not take place. In Map stimulated cultures, co-cultures show slightly worse figures for mycobacteria killing compared to PMNs but improved rates compared to MDMs, also with lower levels of pro-inflammatory cytokines. In BCG, ET release is negatively correlated to bacterial killing, suggesting that killing could be due to phagocytosis, whereas as in Map ET release is positively correlated to bacterial killing, suggesting that probably most killing is due to PMNs ETosis and provision of macrophages with killing capacity molecules in the co-cultures. Further research should involve in vivo studies to confirm that PMNs are necessary in the defense against Map and studies clarifying which subsets of PMNs are involved in each effector mechanism in order to develop therapeutic agents targeted at improving PMN competence.
Data Availability Statement
The raw data supporting the conclusions of this article will be made available by the authors, without undue reservation.
Ethics Statement
Ethical review and approval was not required for the animal study because Animals used in this study were submitted only to procedures that according to European (Directive 2010/63/EU of the European Parliament and of the Council of 22 September 2010 on the protection of animals used for scientific purposes. Chapter 1, Article 1, Section 5, paragraphs b and f) and Spanish (Real Decreto 53/2013, de 1 de febrero, por el que se establecen las normas básicas aplicables para la protección de los animales utilizados en experimentación y otros fines científicos, incluyendo la docencia, Article 2, Section 5, Paragraphs b and f) legislation on experimental animals are exempt from its application. The animals, belonging to a registered commercial farm supervised by the local livestock authority (Servicio de Ganadería de la Diputación Foral de Bizkaia) were submitted only to the introduction of a needle in accordance with good veterinary practice and were not killed in relationship with this study.
Author Contributions
DW and NE conceived the study. AU carried out the animal sampling. IL-A, EM, AH, JK, HH and NE performed the laboratory work. IL-A and NE compiled and analyzed the data. IL-A and NE collated the results. IL-A, NE, JA and DW drafted the preliminary manuscript. All authors contributed to the article and approved the submitted version.
Funding
Funding was provided by Spanish central government and Basque research project PROBAK (RTA 2017-00089-00-00) and by the Departamento de Economı́a e Infraestructuras of the Basque Government. IL-A held a predoctoral grant from Departamento de Economı́a e Infraestructuras of the Basque Government (2017) and was granted an EMBO short-term fellowship (8407) and a FEMS research and training grant (FEMS-GO-2019-507). The funders had no role in the study design, data collection and interpretation, or the decision to submit the work for publication.
Conflict of Interest
The authors declare that the research was conducted in the absence of any commercial or financial relationships that could be construed as a potential conflict of interest.
Acknowledgments
The authors want to thank Ainara Badiola and Maddi Oyanguren for technical support and Joseba Garrido for critical reading of the manuscript.
References
1. Hasonova L, Hasonova L, Pavlik I. Economic impact of paratuberculosis in dairy cattle herds: a review. Vet Med (Praha) (2006) 51:193–211. doi: 10.17221/5539-VETMED
2. Bastida F, Juste RA. Paratuberculosis control: A review with a focus on vaccination. J Immune Based Ther Vaccines (2011) 9:1–17. doi: 10.1186/1476-8518-9-8
3. Juste RA, Alonso-Hearn M, Molina E, Geijo M, Vazquez P, Sevilla IA, et al. Significant reduction in bacterial shedding and improvement in milk production in dairy farms after the use of a new inactivated paratuberculosis vaccine in a field trial. BMC Res Notes (2009) 2:233. doi: 10.1186/1756-0500-2-233
4. Reddacliff L, Eppleston J, Windsor P, Whittington R, Jones S. Efficacy of a killed vaccine for the control of paratuberculosis in Australian sheep flocks. Vet Microbiol (2006) 115:77–90. doi: 10.1016/j.vetmic.2005.12.021
5. Khare S, Nunes JS, Figueiredo JF, Lawhon SD, Rossetti CA, Gull T, et al. Early phase morphological lesions and transcriptional responses of bovine ileum infected with Mycobacterium avium subsp. paratuberculosis. Vet Pathol (2009) 46:717–28. doi: 10.1354/vp.08-VP-0187-G-FL
6. Jimbo S, Suleman M, Maina T, Prysliak T, Mulongo M, Perez-Casal J. Effect of Mycoplasma bovis on bovine neutrophils. Vet Immunol Immunopathol (2017) 188:27–33. doi: 10.1016/j.vetimm.2017.04.011
7. Cassatella MA, Locati M, Mantovani A. Never underestimate the power of a neutrophil. Immunity (2009) 31:698–700. doi: 10.1016/j.immuni.2009.10.003
8. Brinkmann V, Reichard U, Goosmann C, Fauler B, Uhlemann Y, Weiss DS, et al. Neutrophil Extracellular Traps Kill Bacteria. Science (2004) 303:1532–5. doi: 10.1126/science.1092385
9. Aulik NA, Hellenbrand KM, Czuprynski CJ. Mannheimia haemolytica and its leukotoxin cause macrophage extracellular trap formation by bovine macrophages. Infect Immun (2012) 80:1923–33. doi: 10.1128/IAI.06120-11
10. Fuchs TA, Abed U, Goosmann C, Hurwitz R, Schulze I, Wahn V, et al. Novel cell death program leads to neutrophil extracellular traps. J Cell Biol (2007) 176:231–41. doi: 10.1083/jcb.200606027
11. Skendros P, Mitroulis I, Ritis K. Autophagy in neutrophils: From granulopoiesis to neutrophil extracellular traps. Front Cell Dev Biol (2018) 6:109. doi: 10.3389/fcell.2018.00109
12. Shrestha S, Lee JM, Hong CW. Autophagy in neutrophils. Korean J Physiol Pharmacol (2020) 24:1–10. doi: 10.4196/kjpp.2020.24.1.1
13. Benati D, Ferro M, Savino MT, Ulivieri C, Schiavo E, Nuccitelli A, et al. Opposite effects of simvastatin on the bactericidal and inflammatory response of macrophages to opsonized S. aureus. J Leukoc Biol (2010) 87:433–42. doi: 10.1189/jlb.0409273
14. Wong KW, Jacobs WR. Mycobacterium tuberculosis exploits human interferon γ to stimulate macrophage extracellular trap formation and necrosis. J Infect Dis (2013) 208:109–19. doi: 10.1093/infdis/jit097
15. Muñoz-Caro T, Silva LMR, Ritter C, Taubert A, Hermosilla C. Besnoitia besnoiti tachyzoites induce monocyte extracellular trap formation. Parasitol Res (2014) 113:4189–97. doi: 10.1007/s00436-014-4094-3
16. Boe DM, Curtis BJ, Chen MM, Ippolito JA, Kovacs EJ. Extracellular traps and macrophages: new roles for the versatile phagocyte. J Leukoc Biol (2015) 97:1023–35. doi: 10.1189/jlb.4ri1014-521r
17. Silva MT. When two is better than one: macrophages and neutrophils work in concert in innate immunity as complementary and cooperative partners of a myeloid phagocyte system. J Leukoc Biol (2010) 87:93–106. doi: 10.1189/jlb.0809549
18. Silva MT, Silva MN, Appelberg R. Neutrophil-macrophage cooperation in the host defence against mycobacterial infections. Microb Pathog (1989) 6:369–80. doi: 10.1016/0882-4010(89)90079-X
19. Tan BH, Meinken C, Bastian M, Bruns H, Legaspi A, Ochoa MT, et al. Macrophages acquire neutrophil granules for antimicrobial activity against intracellular pathogens. J Immunol (2008) 180:664.2–664. doi: 10.4049/jimmunol.180.1.664-a
20. Lemon JK, Miller MR, Weiser JN. Sensing of interleukin-1 cytokines during Streptococcus pneumoniae colonization contributes to macrophage recruitment and bacterial clearance. Infect Immun (2015) 83:3204–12. doi: 10.1128/IAI.00224-15
21. Kenefick KB, Adams JL, Steinberg H, Czuprynski CJ. In vivo administration of a monoclonal antibody against the type I IL-1 receptor inhibits the ability of mice to eliminate Mycobacterium paratuberculosis. J Leukoc Biol (1994) 55:719–22. doi: 10.1002/jlb.55.6.719
22. Lamont EA, O’Grady SM, Davis WC, Eckstein T, Sreevatsan S. Infection with Mycobacterium avium subsp. paratuberculosis results in rapid interleukin-1ß release and Macrophage transepithelial migration. Infect Immun (2012) 80:3225–35. doi: 10.1128/IAI.06322-11
23. Krupa A, Fol M, Dziadek BR, Kepka E, Wojciechowska D, Brzostek A, et al. Binding of CXCL8/IL-8 to Mycobacterium tuberculosis Modulates the Innate Immune Response. Mediators Inflammation (2015) 2015:124762. doi: 10.1155/2015/124762
24. Gupta AK, Hasler P, Holzgreve W, Gebhardt S, Hahn S. Induction of neutrophil extracellular DNA lattices by placental microparticles and IL-8 and their presence in preeclampsia. Hum Immunol (2005) 66:1146–54. doi: 10.1016/j.humimm.2005.11.003
25. Dotta U, Guglielmino R, Cagnasso A, D’Angelo A, Prato S, Bosso M. Effects of subclinical bovine paratuberculosis on in-vitro polymorphonuclear neutrophil migration. J Comp Pathol (1999) 121:399–403. doi: 10.1053/jcpa.1999.0033
26. Coussens PM. Model for immune responses to Mycobacterium avium subspecies paratuberculosis in cattle. Infect Immun (2004) 72:3089–96. doi: 10.1128/IAI.72.6.3089-3096.2004
27. Park H-E, Park H-T, Jung YH, Yoo HS. Gene expression profiles of immune-regulatory genes in whole blood of cattle with a subclinical infection of Mycobacterium avium subsp. paratuberculosis. PLoS One (2018) 13:e0196502. doi: 10.1371/journal.pone.0196502
28. Alonso-Hearn M, Canive M, Blanco-Vazquez C, Torremocha R, Balseiro A, Amado J, et al. RNA-Seq analysis of ileocecal valve and peripheral blood from Holstein cattle infected with Mycobacterium avium subsp. paratuberculosis revealed dysregulation of the CXCL8/IL8 signaling pathway. Sci Rep (2019) 9:14845. doi: 10.1038/s41598-019-51328-0
29. David J, Barkema HW, Guan LL, De Buck J. Gene-expression profiling of calves 6 and 9 months after inoculation with Mycobacterium avium subspecies paratuberculosis. Vet Res (2014) 45:96. doi: 10.1186/s13567-014-0096-5
30. Martineau AR, Newton SM, Wilkinson KA, Kampmann B, Hall BM, Nawroly N, et al. Neutrophil-mediated innate immune resistance to mycobacteria. J Clin Invest (2007) 117:1988–94. doi: 10.1172/JCI31097
31. Cirone KM, Lahiri P, Holani R, Tan YL, Arrazuria R, De Buck J, et al. Synthetic cathelicidin LL-37 reduces Mycobacterium avium subsp. paratuberculosis internalization and pro-inflammatory cytokines in macrophages. Cell Tissue Res (2020) 379:207–17. doi: 10.1007/s00441-019-03098-4
32. Parker AE, Bermudez LE. Expression of the green fluorescent protein (GFP) in Mycobacterium avium as a tool to study the interaction between Mycobacteria and host cells. Microb Pathog (1997) 22:193–8. doi: 10.1006/mpat.1996.0106
33. Von Köckritz-Blickwede M, Chow O, Ghochani M, Nizet V. Visualization and functional evaluation of phagocyte extracellular. Methods Microbiol (2010). 37:139–60. doi: 10.1016/S0580-9517(10)37007-3
34. Conejeros I, Velásquez ZD, Grob D, Zhou E, Salecker H, Hermosilla C, et al. Histone h2a and bovine neutrophil extracellular traps induce damage of Besnoitia besnoiti-infected host endothelial cells but fail to affect total parasite proliferation. Biol (Basel) (2019) 8:78. doi: 10.3390/biology8040078
35. Ishikawa E, Ishikawa T, Morita YS, Toyonaga K, Yamada H, Takeuchi O, et al. Direct recognition of the mycobacterial glycolipid, trehalose dimycolate, by C-type lectin Mincle. J Exp Med (2009) 206:2879–88. doi: 10.1084/jem.20091750
36. Sharma A, Simonson TJ, Jondle CN, Mishra BB, Sharma J. Mincle-Mediated Neutrophil Extracellular Trap Formation by Regulation of Autophagy. J Infect Dis (2017) 215:1040–8. doi: 10.1093/infdis/jix072
37. Xu J, Zhang X, Monestier M, Esmon NL, Esmon CT. Extracellular Histones Are Mediators of Death through TLR2 and TLR4 in Mouse Fatal Liver Injury. J Immunol (2011) 187:2626–31. doi: 10.4049/jimmunol.1003930
38. Papayannopoulos V, Zychlinsky A. NETs: a new strategy for using old weapons. Trends Immunol (2009) 30:513–21. doi: 10.1016/j.it.2009.07.011
39. Friggeri A, Banerjee S, Xie N, Cui H, de Freitas A, Zerfaoui M, et al. Extracellular histones inhibit efferocytosis. Mol Med (2012) 18:825–33. doi: 10.2119/molmed.2012.00005
40. Brown AE, . Holzer TJ, . Andersen BR. Capacity of Human Neutrophils to Kill Mycobacterium tuberculosis. J Infect Dis (1987) 156:985–9. doi: 10.1093/infdis/156.6.985
41. Jones GS, Amirault HJ, Andersen BR. Killing of Mycobacterium tuberculosis by neutrophils: A nonoxidative process. J Infect Dis (1990) 162:700–4. doi: 10.1093/infdis/162.3.700
42. Bermudez LE, Petrofsky M, Stevens P. Treatment with recombinant granulocyte colony-stimulating factor (Filgrastin™) stimulates neutrophils and tissue macrophages and induces an effective non-specific response against Mycobacterium avium in mice. Immunology (1998) 94:297–303. doi: 10.1046/j.1365-2567.1998.00529.x
43. Sawant KV, McMurray DN. Guinea pig neutrophils infected with Mycobacterium tuberculosis produce cytokines which activate alveolar macrophages in noncontact cultures. Infect Immun (2007) 75:1870–7. doi: 10.1128/IAI.00858-06
44. Vandivier RW, Henson PM, Douglas IS. Burying the dead: The Impact of Failed Apoptotic Cell Removal (Efferocytosis) on Chronic Inflammatory Lung Disease - ProQuest . Available at: https://search-proquest-com.ehu.idm.oclc.org/docview/200486527 (Accessed April 24, 2020).
45. Chiang SK, Sommer S, Aho AD, Kiupel M, Colvin C, Tooker B, et al. Relationship between Mycobacterium avium subspecies paratuberculosis, IL-1a, and TRAF1 in primary bovine monocyte-derived macrophages. Vet Immunol Immunopathol (2007) 116:131–44. doi: 10.1016/j.vetimm.2007.01.005
46. Ibeagha-Awemu E, Do D, Dudemaine P, Bissonnette N. PSXIV-17 Gene co-expression network analysis identifies important modules and genes for cow’s response to Mycobacterium avium ssp. paratuberculosis infection in the small intestine. J Anim Sci (2018) 96:39. doi: 10.1093/jas/sky404.088
47. Gossner A, Watkins C, Chianini F, Hopkins J. Pathways and Genes Associated with Immune Dysfunction in Sheep Paratuberculosis. Sci Rep (2017) 7:1–12. doi: 10.1038/srep46695
48. Krüger C, Köhler H, Liebler-Tenorio EM. Cellular composition of granulomatous lesions in gut-associated lymphoid tissues of goats during the first year after experimental infection with Mycobacterium avium subsp. paratuberculosis. Vet Immunol Immunopathol (2015) 163:33–45. doi: 10.1016/j.vetimm.2014.11.002
49. Lee H, Stabel JR, Kehrli ME. Cytokine gene expression in ileal tissues of cattle infected with Mycobacterium paratuberculosis. Vet Immunol Immunopathol (2001) 82:73–85. doi: 10.1016/S0165-2427(01)00340-3
50. DeKuiper JL. Inflammatory Th17 responses to infection with Mycobacterium avium subspecies paratuberculosis (Map) in cattle and their potential role in the development of Johne’s Disease. Vet Immunol Immunopathol (2019) 218:109954. doi: 10.25335/7F47-KM75
51. Weiss DJ, Evanson OA, Moritz A, Deng MQ, Abrahamsen MS. Differential responses of bovine macrophages to Mycobacterium avium subsp. paratuberculosis and Mycobacterium avium subsp. avium. Infect Immun (2002) 70:5556–61. doi: 10.1128/IAI.70.10.5556-5561.2002
52. Coussens PM, Verman N, Coussens MA, Elftman MD, McNulty AM. Cytokine Gene Expression in Peripheral Blood Mononuclear Cells and Tissues of Cattle Infected with Mycobacterium avium subsp. paratuberculosis: Evidence for An Inherent Proinflammatory Gene Expression Pattern. Infect Immun (2004) 72:1409–22. doi: 10.1128/IAI.72.3.1409-1422.2004
53. Kviatcovsky D, Rivadeneyra L, Balboa L, Yokobori N, López B, Ritacco V, et al. Mycobacterium tuberculosis Multidrug-Resistant Strain M Induces Low IL-8 and Inhibits TNF- α Secretion by Bronchial Epithelial Cells Altering Neutrophil Effector Functions. Mediators Inflamm (2017) 2017:2810606. doi: 10.1155/2017/2810606
54. Mayer-Barber KD, Barber DL, Shenderov K, White SD, Wilson MS, Cheever A, et al. Cutting Edge: Caspase-1 Independent IL-1β Production Is Critical for Host Resistance to Mycobacterium tuberculosis and Does Not Require TLR Signaling In Vivo. J Immunol (2010) 184:3326–30. doi: 10.4049/jimmunol.0904189
Keywords: neutrophils (PMNs), macrophages, IL-1β, IL-8, extracellular trap, Mycobacterium avium subsp. paratuberculosis, Mycobacterium bovis BCG
Citation: Ladero-Auñon I, Molina E, Holder A, Kolakowski J, Harris H, Urkitza A, Anguita J, Werling D and Elguezabal N (2021) Bovine Neutrophils Release Extracellular Traps and Cooperate With Macrophages in Mycobacterium avium subsp. paratuberculosis clearance In Vitro. Front. Immunol. 12:645304. doi: 10.3389/fimmu.2021.645304
Received: 22 December 2020; Accepted: 25 February 2021;
Published: 17 March 2021.
Edited by:
Javier Dominguez, Instituto Nacional de Investigación y Tecnología Agroalimentaria (INIA), SpainReviewed by:
Victor P.M.G. Rutten, Utrecht University, NetherlandsJodi L. McGill, Iowa State University, United States
Copyright © 2021 Ladero-Auñon, Molina, Holder, Kolakowski, Harris, Urkitza, Anguita, Werling and Elguezabal. This is an open-access article distributed under the terms of the Creative Commons Attribution License (CC BY). The use, distribution or reproduction in other forums is permitted, provided the original author(s) and the copyright owner(s) are credited and that the original publication in this journal is cited, in accordance with accepted academic practice. No use, distribution or reproduction is permitted which does not comply with these terms.
*Correspondence: Natalia Elguezabal, bmVsZ3VlemFiYWxAbmVpa2VyLmV1cw==