- 1BC Children’s Hospital Research Institute, University of British Columbia, Vancouver, BC, Canada
- 2Department of Pediatrics, Faculty of Medicine, University of British Columbia, Vancouver, BC, Canada
- 3Experimental Medicine Program, Department of Medicine, Faculty of Medicine, University of British Columbia, Vancouver, BC, Canada
- 4Department of Pathology & Laboratory Medicine, School of Medicine, Emory University, Atlanta, GA, United States
- 5Department of Surgery, Faculty of Medicine, University of British Columbia, Vancouver, BC, Canada
- 6Faculty of Pharmaceutical Sciences, Faculty of Medicine, University of British Columbia, Vancouver, BC, Canada
Newborns are frequently affected by mucocutaneous candidiasis. Th17 cells essentially limit mucosal invasion by commensal Candida spp. Here, we sought to understand the molecular basis for the developmental lack of Th17 cell responses in circulating blood neonatal T cells. Naive cord blood CD4 T cells stimulated in Th17-differentiating conditions inherently produced high levels of the interleukin-22 immunoregulatory cytokine, particularly in the presence of neonatal antigen-presenting cells. A genome-wide transcriptome analysis comparing neonatal and adult naïve CD4 T cells ex vivo revealed major developmental differences in gene networks regulating Small Drosophila Mothers Against Decapentaplegic (SMAD) and Signal Transducer and Activator of Transcription 3 (STAT3) signaling. These changes were functionally validated by experiments showing that the requirement for TGF-β in human Th17 cell differentiation is age-dependent. Moreover, STAT3 activity was profoundly diminished while overexpression of the STAT3 gene restored Th17 cell differentiation capacity in neonatal T cells. These data reveal that Th17 cell responses are developmentally regulated at the gene expression level in human neonates. These developmental changes may protect newborns against pathological Th17 cell responses, at the same time increasing their susceptibility to mucocutaneous candidiasis.
Introduction
T helper 17 (Th17) cells are essential to limit Candida invasion at the skin and mucosal surfaces; this is evidenced by observations of loss-of-function genetic mutations in IL-17, and related receptor gene pathways, resulting in severe, recurrent mucocutaneous Candidiasis (MC) (1). Neonates are also susceptible to MC, which frequently manifests as oral thrush and diaper rashes, but the underlying mechanisms are not well understood (2). In full-term neonates, Candida species trigger robust in vitro innate immune responses (3). Neonatal T cells are also able to produce strong IL-17 responses in lymph nodes and the gut (4). However, according to other studies, peripheral blood naïve T cells produce only weak Th17 responses (5, 6), and IL-17-producing cells in the blood circulation are restricted to a subset of CD45RO+CCR7-CD25low CCR6+ effector memory (Tem) cells (7). These observations raise important questions about the mechanisms involved in these developmental changes in humans, and how they impact the ability of neonates to respond they to pathogens such as Candida species.
Functionally, neonatal T cells are biased towards Th2 or T regulatory cell differentiation (8). Earlier studies show that fetal CD4 T cells are transcriptionally distinct from their adult counterparts (9). To our knowledge there has been little studies directly comparing gene expression profiles in term neonatal and adult naïve T cells, at the genome-wide level. In mice, IL-6 and Transforming Growth Factor beta (TGF-β) are sufficient for Th17 cell differentiation (10). In humans, Th17 cells differentiation occurs in the presence of IL-1β and IL-23 alone (11). In light of these findings, Acosta-Rodriguez et al. proposed that TGF-β is not essential during human Th17 cell differentiation (12). As Zhang has pointed out (13), experiments showing that TGF-β is essential during human Th17 cell differentiation used cord blood, whereas experiments supporting that TGF-β is not required, used adult blood. This raises the possibility that previous observations reflect age-dependent TGF-β requirements during the Th17 differentiation of human naïve CD4 T cells.
In CD4 T cells, binding of IL-6 and IL-23 through their receptors results in phosphorylation of the Signal Transducer and Activator of Transcription 3 (STAT3), which in turn leads to activation of RORC, the gene encoding the master Th17 transcriptional regulator RAR-related Orphan Receptor gamma (RORγt) (14). Basic Leucine Zipper ATF-Like Transcription Factor (BATF) and Interferon Regulatory Factor 4 (IRF4) cooperate to increase accessibility of chromatin to STAT3 and RORγt leading to Th17 cell differentiation (10, 15). Compared to adults, RORC is poorly expressed in naive neonatal CD4 T cells, suggesting a transcriptional restriction in this pathway (5). Though these data were obtained in absence of stimulation, such differences may suggest that the mechanisms regulating Th17 cell responses during the neonatal period lie upstream of RORγt. During Th17 cell differentiation, STAT3 cooperates with other cell signals, including TGF-β signaling, resulting from activation of two serine/threonine kinase transmembrane receptors: type I and type II TGF-β receptors (16). TGF-β signaling is mediated through the Small Drosophila Mothers Against Decapentaplegic (SMAD) proteins. Notably, SMAD2 and SMAD3 cooperate oppositely to regulate expression of the RORC gene, but also expression of the IL17A gene through SMAD4 (17).
Here, we sought to better understand the transcriptional and molecular events regulating the lack of Th17 cell response in human neonatal CD4 T cells. To this end, we directly compare neonatal and adult naïve CD4 T cells in an unbiased genome-wide gene expression analysis. Our analyses uncover major developmental changes in gene networks between adult and neonatal Th17 cells, unexpected from cells of the same lineage. We show changes in basic cellular domains, but more notably, T cell-specific domains such as Th differentiation, IL-7-dependent homeostatic proliferation and TGF-β signaling. These observations were functionally validated, by experiments revealing that the requirement for TGF-β during the differentiation of human Th17 cells is age-dependent. These experiments imply developmental changes in the activity of STAT3 and SMADs, and T cell biasing towards an immunoregulatory Th22 phenotype. Our findings, in primary human newborn T cells, support developmentally regulated transcriptional networks programmed to limit systemic Th17 cell responses during the newborn period. We propose that these changes at the same time increase newborns’ susceptibility to MC.
Materials and Methods
Blood Sample Collection
Cord and peripheral blood samples were collected in sodium heparin anti-coagulated Vacutainers (Becton Dickinson, Canada) from healthy term (>38-41 weeks of gestation) neonates born by cesarean section without labor at the Children’s & Women’s Health Centre of British Columbia (C&W) and healthy adults (range 20 to 40 years old). Samples were processed within 2 hours of collection.
T Cell Purification, Stimulation and Cytokine Production
T cells were isolated by Fluorescent Activated Cell Sorting (FACS) or magnetic bead separation as detailed in Supplemental Methods (see also Supplemental Table S1 for list of samples). Isolated T cells were stimulated with a 1:1 ratio of anti-CD3/CD28 beads (Thermo Fisher Scientific, DE, USA), as specified in RPMI1640 medium supplemented with 10% fetal bovine serum and 1% Penicillin-Streptomycin. During stimulation, the following cytokine polarizing conditions were used: Th0 (no exogenous cytokines), Th1: IL-12 (10 ng ml−1) and Th17: IL-6 (10 ng ml−1), IL-23 (10 ng ml−1), IL-1β (10 ng ml−1) ± TGF-β (3 ng ml−1) (all purchased from Peprotech, NJ, USA). IL-10 inhibition was achieved in presence of an anti-IL-10 (5 µg ml−1) and anti-IL-10 receptor, alpha subunit (5 µg ml−1) blocking antibodies (both from R&D systems, Ontario, Canada). STAT3 inhibition was achieved using Stattic (10 µM, Cayman Chemical, MI, USA). For T cell stimulation in the presence of antigen-presenting cells, neonatal or adult T cells were stimulated with a 1:1 ratio of allogeneic CD3-depleted neonatal or adult carboxyfluorescein succinimidyl ester (CFSE, Thermo Fisher Scientific, cat# C34554)-labeled antigen-presenting cells in the presence of anti-CD3 (OKT3; 0.5 μg ml-1) with or without an anti-CD28 antibody (1 μg ml−1; Invitrogen, # 11131D) for 6 days. For measures of T cell STAT3 activity, mononuclear cells were rested overnight at 37°C after thawing in RPMI1640 medium without serum, and stimulated with or without recombinant IL-6 (Thermo Fisher Scientific, USA) at a final concentration of 100 ng ml-1 for 15 minutes at 37°C. Procedures for intracellular and secreted cytokine and STAT3 experiments are detailed in Supplemental Methods.
Gene Expression Analysis
The FACS isolation and data analysis strategies for gene expression experiments using naïve CD4 T cells data are depicted in Supplemental Figure S1 (see Supplemental Figure S2 for an example of the gating strategy that was used in FACS). RNA extraction, gene expression profiling, processing of whole-genome expression array data and Gene Set Enrichment analysis (GSEA) are detailed in Supplemental Methods. Thirteen genes (excluding STAT3) were chosen for confirmation by qPCR from separate neonatal and adult subjects (Supplemental Figure S3).
STAT3 Transfections
A plasmid containing the human STAT3 and green fluorescent protein (GFP) cDNAs (Supplemental Methods) was transfected into magnetic bead-purified neonatal naïve CD4 T cells using a Lonza Nucleofector 2b device (Basel, Switzerland). Prior to transfections, the purity of these naïve T cells (>99.5% CD45RA+ cells) was verified by flow cytometry staining for the CD3, CD4 and CD45RA surface markers. After overnight cell culture at 37°C, dead cells were removed using a Dead Cell Removal kit (Miltenyi Biotech, # 130-090-101). The efficiency of transfection was assessed by green fluorescence protein expression measured by flow cytometry comparing STAT3-transfected and empty-vector-transfected cells. Transfected cells were stimulated and polarized as described above for 3 days. Cytokine expression data was normalized to the percentage of GFP-expressing cells in each sample.
Statistics
Cytokine levels were compared using 2-tailed Mann-Whitney U (for independent groups) Wilcoxon test (for comparison of non-parametric data between stimulating conditions within same age groups) or paired t-tests (for comparison of parametric data between stimulating conditions within same age groups), or in some instances, 95% confidence intervals (for non-inferential, exploratory group comparisons where data was deemed to be normally distributed). Effects of age, stimulating cytokine conditions, and T cell or antigen-presenting cell age were assessed using 2-way ANOVAs as specified. Inferential group comparisons were adjusted for multiple testing using the Benjamini-Hochberg method and a 5% false-discovery rate, whenever indicated and as specified. P value of <0.05 was considered significant. All statistical analyses were conducted using GraphPad Prism 8 or 9 (San Diego, CA).
Results
Neonatal T Cells Are Inherently Biased Towards High IL-22 Cytokine Production
To understand age-specific T cell differentiation biases, cord blood (neonatal) and healthy adult peripheral blood naïve CD4 T cells (CD3+CD4+CD45RO-CCR7+CD25-) were isolated by Fluorescent Activated Cell Sorting (FACS) and stimulated using anti-CD3/CD28 beads for 6 days in the presence of either Th17-polarizing (IL-1β, IL-6 and IL-23), Th1-polarizing (IL-12), or no cytokines (Th0). As shown in Figure 1A, neonatal T cells stimulated in Th0 conditions produced low levels of Th1 (interferon-γ, IFN-γ) and Th17 (IL-17 and IL-21) cytokines compared to adult T cells. Notably, an abundance of IL-10, IL-13, Granulocyte-Macrophage Colony Stimulating Factor (GM-CSF), and also IL-22, was observed in Th1- or Th17-stimulated neonatal T cells (Figure 1A). These results confirm a Th2 bias, but further reveal another inherent neonatal T cell bias towards IL-22 rather than IL-17 production. Flow cytometry experiments by intracellular cytokine staining showed that IL-22-producing cells were distinct from IL-10-producing cells, and largely non-IL-17-producing (Supplemental Figure S4).
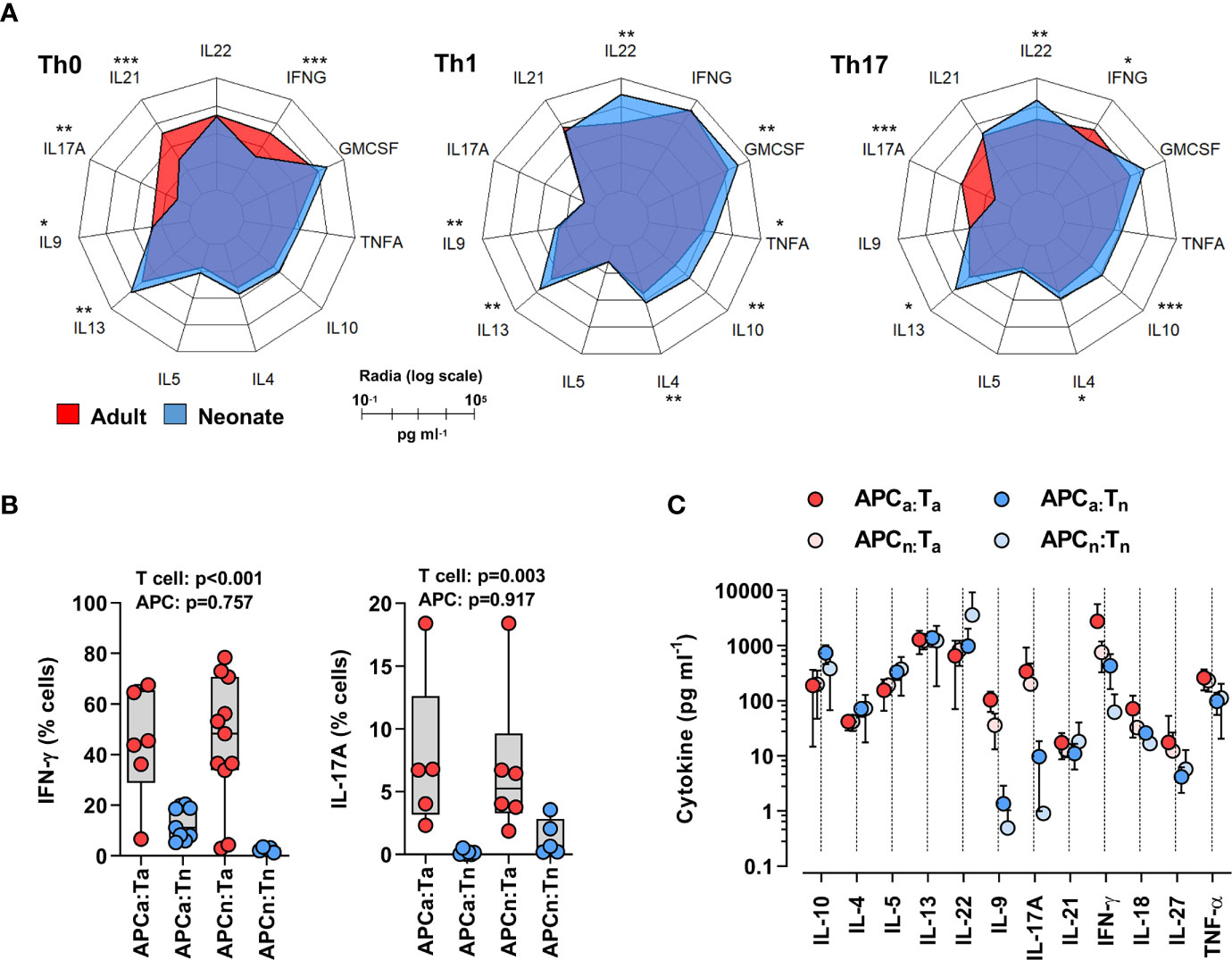
Figure 1 Neonatal T cells are inherently biased against Th17 responses independent of antigen-presenting cell signals. (A) Radar plots of cytokines produced by adult or neonatal naïve CD4 T cells 6 days post-stimulation (anti-CD3/CD28 beads) in Th0- (no exogenous cytokines), Th1- (IL-12) or Th17- (IL-1β, IL-6 and IL-23) polarizing conditions. Data are presented on a log-10 scale (pg ml−1) averaged from two separate sets of experiments that include a total of 6-12 subjects per cytokine polarizing condition per age group; P values for each cytokine were calculated by two-tailed Mann-Whitney U test using non-logarithmic values and correction was applied for multiple comparisons using the Benjamini-Hochberg method; *p < 0.05; **p < 0.01; ***p < 0.001; (B) Intracellular IL-17 and IFN-γ detection (by flow cytometry) in adult (red) or neonatal (blue) naïve CD4 T cells stimulated using soluble anti-CD3 antibody (OKT3) for 6 days in presence of carboxyfluorescein succinimidyl ester (CFSE)-labeled allogeneic neonatal (N) or adult (A) antigen-presenting cells (APCa = adult APCs, APCn = neonatal APCs, Ta = adult T cells, Tn = neonatal T cells). Data are aggregated from 11 independent experiments each using 2 freshly collected (same day) neonatal and adult samples, with p values for the effect of T cell or APC age on cytokine response, using a 2-way ANOVA; (C) Corresponding cytokine measurement in supernatants (ELISA); means with 95% CIs.
Neonatal antigen-presenting cells, particularly dendritic cells, make copious amounts of IL-1β, IL-6, and IL-23 (18). To determine to what extent the poor Th17 cell differentiation of neonatal T cells might be reversed by signals from neonatal antigen-presenting cells, we stimulated neonatal or adult T cells (using anti-CD3) in the presence of allogeneic adult or neonatal (cord blood) CD3-depleted mononuclear cells (without exogenous cytokines). Of note, this crossover experimental design allowed the mitigation of effects due to expected age-related differences in the proportion of bona fide antigen-presenting cells among blood mononuclear cells (19, 20). In these conditions, neonatal T cells still produced substantially less IL-17 and IFN-γ than adult T cells, in addition to reduced levels of IL-9, regardless of whether neonatal or adult antigen-presenting cells were used (Figures 1B, C; Supplemental Figure S5). Notably, neonatal T cells showed greater IL-22 production in the presence of neonatal antigen-presenting cells (Figure 1C). Neonatal antigen-presenting cells generally express lower levels of the CD28 ligands B7.1/2, and therefore may generally provide poor CD28 co-stimulation (8). Co-stimulation through ICOS enhances IL-22 production (21). To determine whether the lack of antigen-presenting cell CD28 co-stimulation overall could play a role here, experiments were repeated in the presence or absence of an anti-CD28 antibody. Differentiation of neonatal T cells in the presence of an anti-CD28 antibody further decreased Th17 cell responses and alternatively promoted increased IFN-γ production in neonatal T cells, regardless of the antigen-presenting cell used (Supplemental Figure S6).
Unbiased Transcriptome Analyses Reveal a Th22 Bias and Altered TGF-β Signaling in Neonatal T Cells
To investigate the transcriptional basis underlying the poor Th17 cell differentiation of human neonatal T cells, we undertook a genome-wide gene expression analysis comparing FACS-isolated naïve CD4 T cells (CD3+CD4+CD45RO-CCR7+CD25-) ex vivo from 12 neonatal (cord blood) and 12 healthy adult subjects. Gene expression profiles showed strong separation between the two age groups (Figure 2A), exposing a large number of differentially expressed genes (n=5,976; FDR <5%), corresponding to ~46% of the 12,909 detectably expressed unique annotated transcripts (Supplemental Table S2). One third (n=2,282) of the differentially expressed genes showed greater than 1.2-fold expression change between neonatal and adult T cells. Gene Ontology analysis mapped differentially expressed genes to broad cellular domains regulating cell cycle, post-transcriptional events and purine nucleoside monophosphate metabolism (Supplemental Figure S7, Supplemental Table S3).
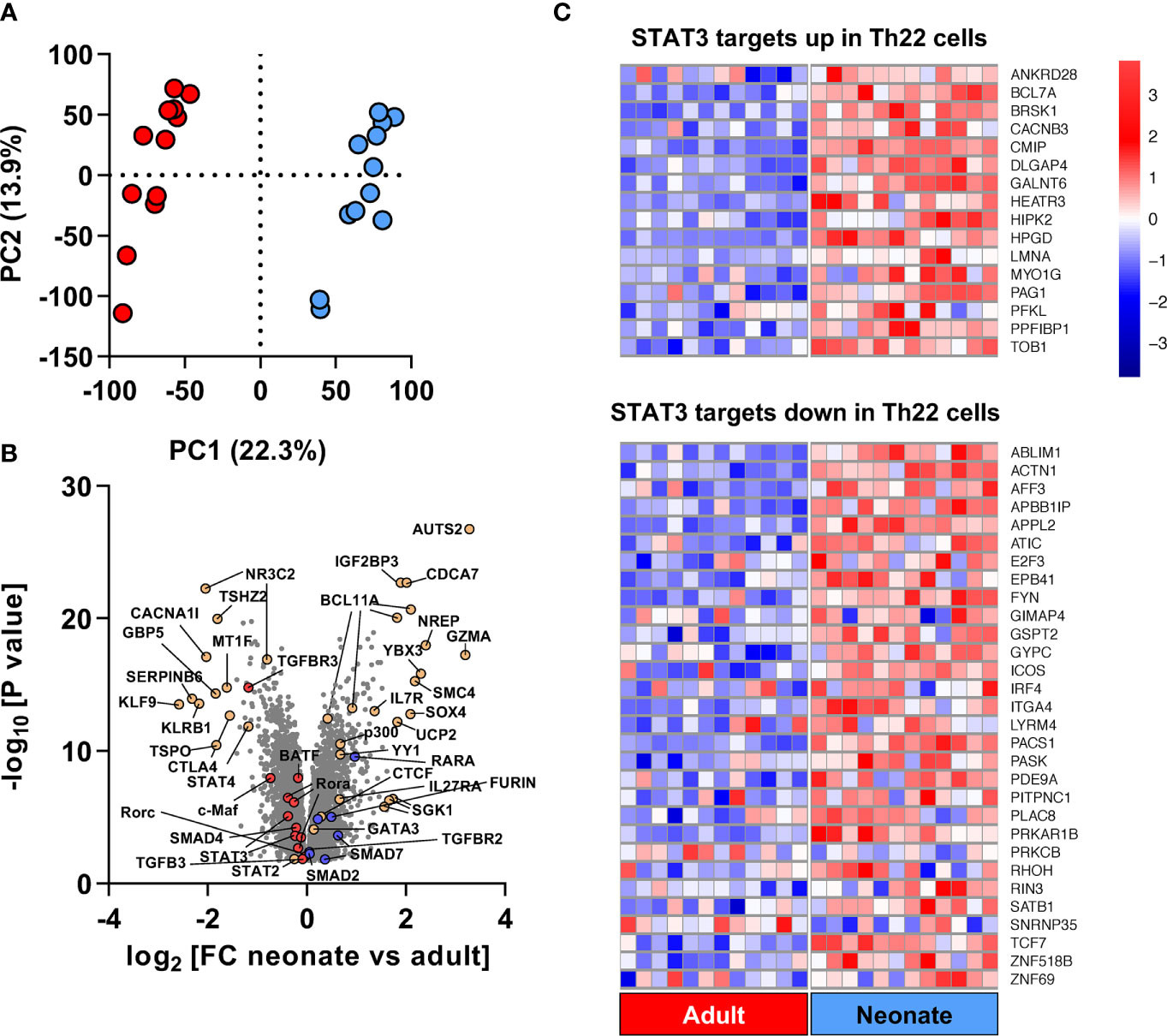
Figure 2 Genome-wide gene expression comparison identifies a unique developmental Th22 signature at baseline in naïve neonatal CD4 T cells. (A) Principal Component Analysis using above-intensity gene expression probes between unstimulated adult (red) and neonatal (blue) naïve CD4 T cells; (B) Volcano plot of fold-change and unadjusted p values of genes expressed differentially by neonatal and adult naïve CD4 T cells. TGF-β- and Th17-related genes with higher expression in adults are shown in red whereas genes with higher expression in neonates are shown in blue; (C) Heatmap of genes overlapping between STAT3 leading edge genes from the Chromatin Immunoprecipitation Enrichment Analysis (ChEA) database (Supplementary Table S5), and upregulated/down-regulated genes in Th22 cells compared to Th1, Th2 and Th17 cells based on (22). Red and blue colors in heat map represent Z-score for upregulated and downregulated genes in neonates compared to adults, respectively.
Overall, the gene expression profile of neonatal T cells was, expectedly, consistent with Th2 pre-commitment, as evidenced by increased baseline expression of GATA3 and decreased expression of Th1-regulating genes such as IL12RB1, IFNGR2 and STAT4 (Figure 2B; Supplemental Figure S8). Expression of other Th2-regulating genes, such as SGK1 (a positive regulator of Th2 cells) (23) and SATB1 (a negative Th17 regulator) (24) increased in neonatal T cells. The configuration of gene networks also supported a neonatal T cell bias against Th17 cell differentiation, as shown by a reduced expression of BATF and RORC, and the SGK1 genes that drive the ratio of Th17 and Treg cells towards pathogenic Th17 responses (25, 26). Gene Set Enrichment Analysis (GSEA) identified a Th22 gene signature within STAT3 targets in neonatal T cell, supporting Th22-biased neonatal T cell responses (Figure 2C).
GSEA also revealed enrichment in other major gene sets regulating T cell biology: namely, Th differentiation and IL-7-dependent homeostatic proliferation, and most notably, TGF-β signaling (Supplemental Figure S8; Supplemental Table S4). TGF-β signaling-regulating genes such as the TGF-β receptors TGFBR3, TGFBR2, Furin, and also the Small Drosophila Mothers Against Decapentaplegic 2 (SMAD2), SMAD4 and SMAD7 were among the key differentially expressed genes. Expression of the SMAD4 gene was decreased, whereas expression of the TGF-β-signaling inhibitor SMAD7 (16) was increased, in neonatal T cells (Figure 2B; Supplemental Table S2). Even though we were unable to detect a differential expression of the SMAD3 gene, the protein expression and activity of both SMAD2 and SMAD3 were increased in neonatal T cells (Figure 3). Additionally, GSEA showed enrichment in the expression of target genes for both SMAD2 and SMAD3 (Supplemental Figure S9; Supplemental Table S5). Altogether, these data support major developmental changes in key regulators of TGF-β signaling between neonatal and adult naive CD4 T cells.
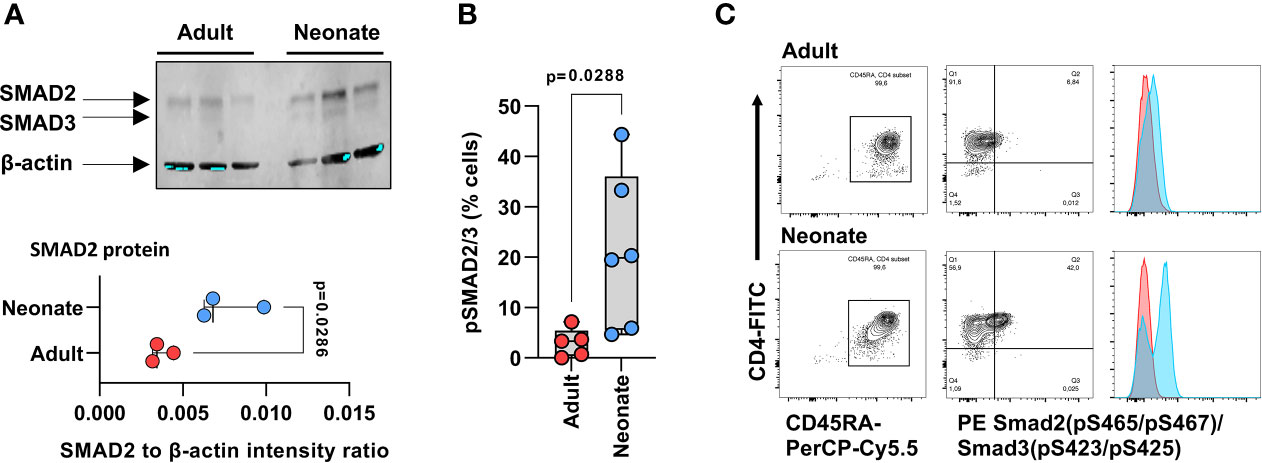
Figure 3 Expression and activity of SMAD2 and SMAD3 in naive neonatal and adult CD4 T cells. (A) Western blot detection of native SMAD2 and SMAD3 in magnetic bead-purified neonatal and adult naïve CD4 T cells (3 independent samples per age groups), and corresponding signal intensity for SMAD2 quantification relative to β-actin (lower panel). p value by two-tailed unpaired t-test; (B) SMAD2 and SMAD3 phosphorylation at serine residues 465 & 467, and serine residues 423 & 425 sites, respectively, by flow cytometry, in isolated naïve CD4 T cells (gated on CD4+CD45RA+ cells) after one hour incubation with TGF-β. p value also by two-tailed unpaired t-test; (C) Representative flow cytometry data in (B), with histograms for cells incubated with TGF-β in blue and histograms in absence of TGF-β in red.
The Requirement for TGF-β During the Differentiation of Human Th17 Cells Is Age-Dependent
The changes in TGF-β signaling between adult and neonatal T cells, at the transcriptome level, prompted further analyses to determine whether this could result in an age-dependent requirements for TGF-β signaling during human Th17 cell differentiation. To this end, magnetic bead-purified adult and neonatal naïve CD4 T cells were stimulated with anti-CD3/CD28 beads in the presence of combinations of IL-1β, IL-6, IL-23 and TGF-β cytokines, measuring IL-17A (Figure 4A) and IL-22 (Figure 4B) after 6 days. This experiment yielded three main observations: first, neonatal T cells produced much less IL-17, but substantially more IL-22 compared to adult T cells regardless of the cytokine stimulating conditions. This bias was confirmed by showing reduced RORγt expression in neonatal T cells (Figures 4C, D). Second, cytokine requirements somewhat differed between neonatal and adult naïve T cells during Th17 cell differentiation: IL-1β and IL-23 appeared sufficient in adult T cells, whereas IL-6 and TGF-β were additionally required to induce maximal IL-17 production in neonatal T cells. Exogenous TGF-β also enhanced IL-17 production in neonatal, but not in adult T cells. Third, adult T cells produced more IL-22 in the presence of IL-1β and IL-23, but IL-6 was essential for maximal IL-22 production by neonatal T cells; however, TGF-β suppressed this IL-22 production in both age groups (Figures 4A, B). This comparative experiment clearly identified age-related differences in the requirement for TGF-β during Th17 cell differentiation.
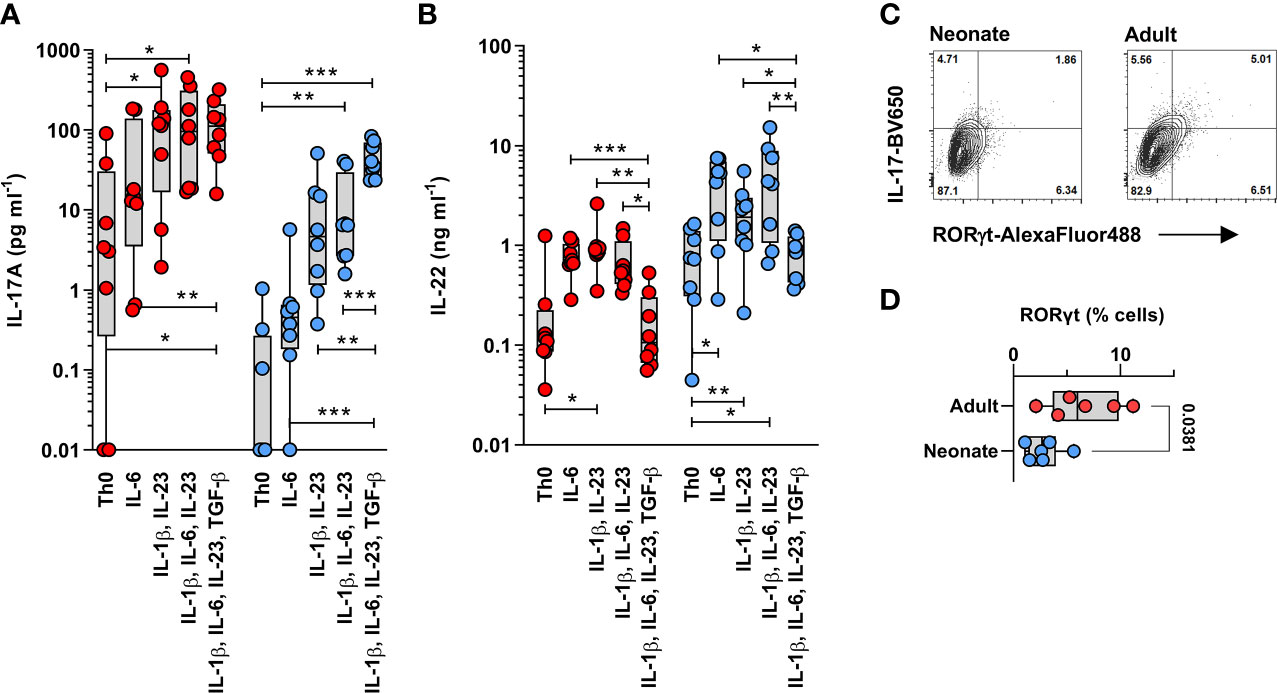
Figure 4 Neonatal T cells are skewed towards high IL-22 production and differ from adult T cells in their TGF-β during Th17 cell differentiation. IL-17 (A) and IL-22 (B) were measured in adult (red) and neonatal (blue) magnetic bead-isolated naïve CD4 T cells stimulated using anti-CD3/CD28 beads for 6 days in the presence of the indicated cytokine combinations. Data are combined from 8 separate experiments using cells from different subjects per age group, with cytokines measured in a single batch/assay; undetectable cytokines are shown as 0.01 pg ml-1; IL-17 (A) and IL-22 (B) production were significantly different between neonatal and adult T cells, as assessed using a 2-way ANOVA (age effects: p =0.023 for IL-17 and p =0.002 for IL-22); where statistical significance for the differences in cytokine stimulation conditions within age groups are shown after adjusting for multiple comparisons using a 5% false-discovery rate; *p < 0.05; **p < 0.01; ***p < 0.001; (C) Representative gating (on live cells) of RORγt expression in IL-17-producing cells from neonatal and adult naïve CD4 T cells differentiated for 6 days in the presence of IL-1β, IL-6, IL-23 and TGF-β, followed by a 5-hour PMA/Ionomycin stimulation (to bring up IL-17 expression), and (D) cumulative data from 6 independent neonatal and adult T cell samples.
Reduced STAT3 Limits the Th17 Cell Differentiation of Neonatal T Cells
Lastly, we aimed to determine whether STAT3 activity was reduced in neonatal T cells and whether this could limit their Th17 cell differentiation capacity. First, reduced STAT3 gene expression in neonatal T cells was confirmed by qPCR in another set of neonatal and adult naïve CD4 T cell samples (Figure 5A). We also confirmed that STAT3 activity was reduced in neonatal T cells, as evidenced by reduced STAT3 phosphorylation at tyrosine residue 705 (Y705) in the presence of IL-6 (Figure 5B, Supplemental Figure S10), despite adult-like expression levels of the IL-6 receptor (Supplemental Figure S11). However, production of IL-22 by human T cells being also largely STAT3-dependent (27), additional experiments confirmed that neonatal T cells show some functionally relevant residual STAT3 activity, as indicated by a decrease in IL-22 production in the presence of a STAT3 inhibitor (Figure 5C). Importantly, transient overexpression of STAT3 (Supplemental Figure S12) increased neonatal IL-17 production when cells were stimulated with anti-CD3/CD28 in the presence of IL-1β, IL-6, and IL-23 (Figure 5D). These data support an important role for altered STAT3 signaling in limiting neonatal Th17 cell differentiation. Notably, STAT3 overexpression also increased IL-22 production, which at the same time remained suppressible by TGF-β (Figure 5E). Finally, since phosphorylation of STAT3 can occur through the IL-10 receptor, and IL-10 inhibits Th17 cell differentiation and is abundantly expressed by neonatal T cells (Figure 1) (28), we wanted to exclude an inhibitory effect of this cytokine on IL-17 production. However, blocking of IL-10 and its high affinity receptor IL-10R1 did not detectably increase IL-17 production in neonatal T cells (Supplemental Figure S13). Thus, these experiments identified STAT3 as an important developmental modulator of Th17 cell differentiation in human neonatal T cells.
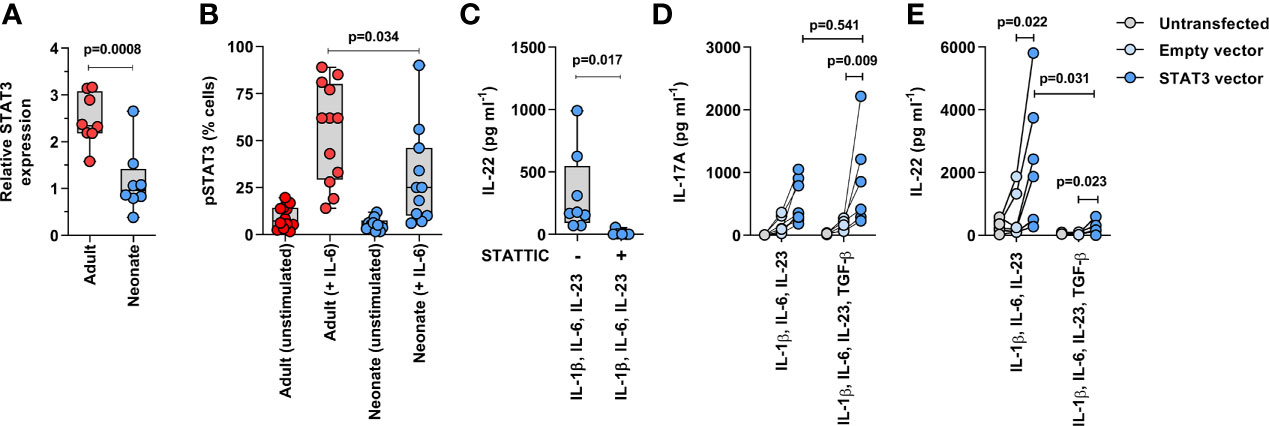
Figure 5 Reduced STAT3 expression limits neonatal Th17 cell differentiation. (A) qPCR confirmation of STAT3 gene expression in 8 neonatal and 8 adults naïve CD4 T cell samples (normalized on GAPDH; p value by unpaired T test); (B) STAT3 phosphorylation (intracellular flow cytometry) measured in neonatal and adult naïve CD4 T cells (gated on CD3+CD4+CD45RA+ cells) after 15 minutes stimulation with IL-6 (100 ng ml−1). Data are combined from 6 donors per age group in 2 separate experiments (p value by unpaired T test); (C) IL-22 production in neonatal T cells after 3 days of stimulation (anti-CD3/CD28 beads) in the presence of IL-1β, IL-6 and IL-23, with or without STAT3 inhibition (Stattic, 10μM); (D) IL-17 and (E) IL-22 production (normalized to %GFP+ live cells) in non-transfected, control (empty) GFP vector- and STAT3/GFP vector-transfected neonatal naïve CD4 T cells stimulated for 3 days (anti-CD3/CD28 beads) with IL-1β, IL-6 and IL-23 ± TGF-β; data are from 7 independent experiments, with lines connecting cells from the same blood donations; p value by paired t-test or Wilcoxon test.
Discussion
This study revealed surprisingly large differences in gene networks between neonatal and adult naïve CD4 T cells ex vivo, suggesting that these two cells represent distinct lineages (29). These results further show that the biology of human naïve T cells is fundamentally different at these two life stages, implying that the human adaptive immune system is programmed to complete its maturation during post-natal life when it is most exposed to foreign antigens. Neonatal CD4 T cells exhibited non-stereotypic Th17 cell responses characterized by a bias towards high production of the immunoregulatory IL-10 and IL-22 cytokines. Unbiased transcriptome and functional experiments implicate major developmental changes in gene networks regulating STAT3 and TGF-β signaling. To the best of our knowledge, this is the first study comparing the Th17 cell differentiation cytokine requirements between neonatal and adult CD4 T cells directly side-by-side. Results showed that the requirement for TGF-β during human Th17 cell differentiation is age-dependent, and manifested mainly with cord blood, but not adult peripheral blood. This important nuance mandates a re-interpretation of previous STAT3/TGF-β functional and gene network data, requiring that the age of the T cell donor be more carefully considered in the interpretation of these studies (15, 30).
Pathological increase in Th17 cell response during the newborn period has been linked to necrotizing enterocolitis - a serious gastrointestinal disease manifesting exclusively in newborns (31). In a neonatal mouse model, systemic blockade of IL-17 reduced mortality from polymicrobial sepsis, suggesting that a high IL-17 production is also disadvantageous during neonatal sepsis (32). During this period of life, naïve CD4 T cells may preferentially adopt an immunoregulatory Th22 phenotype, rather than a stereotypic Th17 phenotype, in order to help maintain local immune homeostasis (33). IL-17 and IL-22 are two cytokines that can be produced by bona fide Th17 cells. Similar to IL-17, IL-22 also acts on epithelial mucosal cells in the skin, liver, gut, and lung (34). IL-22 is a member of the IL-10 family of anti-inflammatory cytokines that target epithelial cells. However, in contrast to IL-17, IL-22 has protective effects in the gut, promoting intestinal epithelial regeneration, enhancing mucin production and the post-translational fucosylation of sugar moieties on mucin proteins, which promotes beneficial gut microbial colonization) (34, 35). Systemically, IL-22 also enhances antibody-mediated immunity and pathogenic bacterial clearance via the iron scavenger hemopexin (36, 37). In the light of these findings we postulate that the preferential bias of circulating neonatal T cells towards IL-22 is essential to limit the systemic consequences of mucosal inflammation during de novo gut colonization with commensal microbes.
Given the phenotype of humans with genetically impaired Th17 cell responses, it is tempting to establish a link between the developmental lack of neonatal Th17 cell differentiation and the increased susceptibility of neonates to MC. On the other hand, humans with mutations in the IL-17 pathway also exhibit increased susceptibility to Staphylococcus aureus folliculitis, which is not commonly seen in neonates. A possible explanation may lie in the ability of neonatal T cells to produce high levels of IL-10 regardless of their Th differentiation conditions (Figure 1A). Supporting this contention, we note that neonates who have a genetic defects in IL10RB also show an increased susceptibility to Staphylococcus aureus folliculitis (1, 38, 39). Thus, production of IL-10 by neonatal Th17 cells might be protective against this condition despite their developmental lack of IL-17 production. Thus, we also conclude that the phenotype of neonatal T cells resembles the immunoregulatory phenotype of IL-10-producing tissue-resident Th17 cells (40) more than the phenotype of T cells from humans with genetic IL-17 defects (38).
Data reveal age-related differences in STAT3 activity and in the requirements for the TGF-β cytokine during Th17 cell differentiation in humans. Gene expression data closely implicate differential activity in SMADs. Mechanistic studies are required to elucidate how these changes operate to alter TGF-β signaling in neonatal T cells. However, we can speculate on this matter here: on the one hand, SMAD2 phosphorylation enhances RORC gene expression (41). On the other hand, SMAD4 can indirectly suppress RORC expression by interacting with SKI, a transcriptional repressor that controls histone acetylation/de-acetylation at the RORC locus (16, 42). TGF-β signaling causes the degradation of SKI, to help SMAD4 suppress the RORC locus (16, 42). Therefore, our data support a model whereby increased SMAD2 activity in the context of reduced SMAD4 renders naïve neonatal CD4 T cells more dependent on TGF-β signaling during the Th17 cell differentiation, despite lower STAT3 activity. In addition, mechanisms common to both neonatal and adult T cells, such as the transcription factor c-Maf that suppresses IL-22 production by binding directly to its proximal gene promoter region (43), may explain how TGF-β suppresses IL-22 production in both of these cell types.
Despite limited STAT3 activity in neonatal T cells, STAT3 expression levels appear sufficient to produce a robust IL-22 response regardless of the IL-17 response. IL-21 also provides positive feedback to amplify the precursor frequency of Th17 cells along with TGF-β (44–46). Together with expression of the transcriptional regulator of Th22 cells, aryl hydrocarbon receptor (AhR), IL-21 can also activate STAT3 to induce IL-22, but not IL-17, production (47). However, the comparable IL-21 expression between neonatal and adult T cells in Th1 or Th17-polarizing conditions does not support a developmental effect through this cytokine. Intracellular flow cytometry cytokine staining experiments showed that the majority of cells between day 5 and day 21 produce either IL-17 or IL-22 rather than both cytokines. However, the interpretation of these preliminary experiments was limited by the differences in timing of intracellular detection for these two cytokine. Further studies are needed to determine whether a small subset of cells can express both cytokines, under specific T cell receptor/cytokine stimulatory conditions.
Studies are also needed to understand how human T cell gene network are regulated developmentally. Considering that the action of transcription-factor networks is context-dependent during the lineage differentiation of CD4 T cells (48), it is plausible that broad developmental-specific epigenetic changes underlie the changes in gene expression observed in this study. The implication of epigenetic changes regulating the network of active transcription factors, as well as the amount of TGF-β signaling forms the basis of the continuum of “classical” to “alternative” Th17 cells. This concept reinforces the notion that lineage-regulating transcription factors influence the final effector phenotype of Th17 cells, potentially through developmentally altered changes in chromatin accessibility (49).
Our study has limitations. First, it only examined cells from adult and cord blood, missing the developmental continuum between these two life stages. Other studies have shown that Th17 cell differentiation remained low up to 3 months of age in infants, so we expect similar mechanisms to operate at least from birth until then (50). Another limitation is that the data were focused on conventional CD4 T cells, while Th17 responses in newborns may also be carried out by other cells in peripheral organs (e.g. the gut), such as γδ T cells or type 3 innate lymphoid cells (31, 51), or other T cell subsets in peripheral tissues (52). Finally, others have shown that peripheral blood neonatal T cells can produce vigorous Th17 responses when challenged with Candida extracts (53), so it would be important to study neonatal T cell responses in the context of MC in order to fully understand their susceptibility to this micro-organism. Nonetheless, studies like the current one provides important mechanistic insights into the regulation and function of conventional T cells during the neonatal period.
Data Availability Statement
The datasets presented in this study can be found in online repositories. The names of the repository/repositories and accession number(s) can be found below: https://www.ncbi.nlm.nih.gov/geo/, GSE135467.
Ethics Statement
The studies involving human participants were reviewed and approved by University of British Columbia Children’s & Women’s Research Ethics Board. Written informed consent to participate in this study was provided by the participants’ legal guardian/next of kin.
Author Contributions
HR, ZS, AS, and PL designed the study. HR, ZS, AS, GB, KL, and RDS conducted the experiments. HR, ZS, AS, GB, and PL analyzed the data. PO and ZS designed and made the STAT3 construct. RPS provided reagents and resources. CR supervised the Illumina expression array experiments. HR, ZS, PO, and PL wrote the manuscript. All authors contributed to the article and approved the submitted version.
Funding
HR was funded by a fellowship from the MITACS national research organization. ZS was supported by Graduate Studentship Award from BC Children’s Hospital Research Institute. AS was supported by a Child & Family Research Institute (CFRI) Graduate Studentship. GB was supported by a University of British Columbia Faculty of Medicine Award. RDS was supported by a BC Children’s Hospital Research Institute Summer Studentship. PL was supported by an Investigator Award from the BC Children’s Hospital Research Institute and a Career Investigator Award from the Michael Smith Foundation for Health Research (MSFHR). This research was funded by Canadian Institutes of Health Research grants (MOP-110938 and MOP-123478, to PML).
Conflict of Interest
The authors declare that the research was conducted in the absence of any commercial or financial relationships that could be construed as a potential conflict of interest.
Acknowledgments
We thank Bruce Verchere, from the BC Children’s Hospital Research Institute, who provided salary support to PO while completing this study, through a Canadian Institutes of Health Research grant CIHR PJT-165943. We also thank all participants who donated cord and adult blood samples for this study, the Women’s Health Research Institute and BC Women’s hospital delivery room staff for their support, Lisa Xu for flow cytometer operation, and Mihoko Ladd for help with functional experiments.
Supplementary Material
The Supplementary Material for this article can be found online at: https://www.frontiersin.org/articles/10.3389/fimmu.2021.655027/full#supplementary-material
References
1. Puel A, Cypowyj S, Bustamante J, Wright JF, Liu L, Lim HK, et al. Chronic Mucocutaneous Candidiasis in Humans With Inborn Errors of Interleukin-17 Immunity. Science (2011) 332(6025):65–8. doi: 10.1126/science.1200439
2. Michalski C, Kan B, Lavoie PM. Antifungal Immunological Defenses in Newborns. Front Immunol (2017) 8:281. doi: 10.3389/fimmu.2017.00281
3. Kan B, Michalski C, Fu H, Au HHT, Lee K, Marchant EA, et al. Cellular Metabolism Constrains Innate Immune Responses in Early Human Ontogeny. Nat Commun (2018) 9(1):4822. doi: 10.1038/s41467-018-07215-9
4. Kadekar D, Agerholm R, Rizk J, Neubauer HA, Suske T, Maurer B, et al. The Neonatal Microenvironment Programs Innate Gammadelta T Cells Through the Transcription Factor STAT5. J Clin Invest (2020) 130(5):2469–508. doi: 10.1172/JCI131241
5. de Roock S, Stoppelenburg AJ, Scholman R, Hoeks SBEA, Meerding J, Prakken BJ, et al. Defective TH17 Development in Human Neonatal T Cells Involves Reduced RORC2 mRNA Content. J Allergy Clin Immunol (2013) 132(3):754–6.e3. doi: 10.1016/j.jaci.2013.04.014
6. Caron JE, La Pine TR, Augustine NH, Martins TB, Kumanovics A, Hill HR. Severely Depressed Interleukin-17 Production by Human Neonatal Mononuclear Cells. Pediatr Res (2014) 76(6):522–7. doi: 10.1038/pr.2014.133
7. Zhang X, Mozeleski B, Lemoine S, Deriaud E, Lim A, Zhivaki D, et al. CD4 T Cells With Effector Memory Phenotype and Function Develop in the Sterile Environment of the Fetus. Sci Transl Med (2014) 6(238):238ra72. doi: 10.1126/scitranslmed.3008748
8. Kan B, Razzaghian HR, Lavoie PM. An Immunological Perspective on Neonatal Sepsis. Trends Mol Med (2016) 22(4):290–302. doi: 10.1016/j.molmed.2016.02.001
9. Mold JE, Venkatasubrahmanyam S, Burt TD, Michaelsson J, Rivera JM, Galkina SA, et al. Fetal and Adult Hematopoietic Stem Cells Give Rise to Distinct T Cell Lineages in Humans. Science (2010) 330(6011):1695–9. doi: 10.1126/science.1196509
10. Ciofani M, Madar A, Galan C, Sellars M, Mace K, Pauli F, et al. A Validated Regulatory Network for Th17 Cell Specification. Cell (2012) 151(2):289–303. doi: 10.1016/j.cell.2012.09.016
11. Wilson NJ, Boniface K, Chan JR, McKenzie BS, Blumenschein WM, Mattson JD, et al. Development, Cytokine Profile and Function of Human Interleukin 17-Producing Helper T Cells. Nat Immunol (2007) 8(9):950–7. doi: 10.1038/ni1497
12. Acosta-Rodriguez EV, Napolitani G, Lanzavecchia A, Sallusto F. Interleukins 1beta and 6 But Not Transforming Growth Factor-Beta are Essential for the Differentiation of Interleukin 17-Producing Human T Helper Cells. Nat Immunol (2007) 8(9):942–9. doi: 10.1038/ni1496
13. Zhang S. The Role of Transforming Growth Factor Beta in T Helper 17 Differentiation. Immunology (2018) 155(1):24–35. doi: 10.1111/imm.12938
14. Sallusto F. Heterogeneity of Human Cd4(+) T Cells Against Microbes. Annu Rev Immunol (2016) 34:317–34. doi: 10.1146/annurev-immunol-032414-112056
15. Tripathi SK, Chen Z, Larjo A, Kanduri K, Nousiainen K, Aijo T, et al. Genome-Wide Analysis of STAT3-Mediated Transcription During Early Human Th17 Cell Differentiation. Cell Rep (2017) 19(9):1888–901. doi: 10.1016/j.celrep.2017.05.013
16. Yoon JH, Sudo K, Kuroda M, Kato M, Lee IK, Han JS, et al. Phosphorylation Status Determines the Opposing Functions of Smad2/Smad3 as STAT3 Cofactors in TH17 Differentiation. Nat Commun (2015) 6:7600. doi: 10.1038/ncomms8600
17. Inman GJ, Nicolas FJ, Hill CS. Nucleocytoplasmic Shuttling of Smads 2, 3, and 4 Permits Sensing of TGF-beta Receptor Activity. Mol Cell (2002) 10(2):283–94. doi: 10.1016/S1097-2765(02)00585-3
18. Kollmann TR, Crabtree J, Rein-Weston A, Blimkie D, Thommai F, Wang XY, et al. Neonatal Innate TLR-mediated Responses are Distinct From Those of Adults. J Immunol (2009) 183(11):7150–60. doi: 10.4049/jimmunol.0901481
19. Marr N, Wang TI, Kam SH, Hu YS, Sharma AA, Lam A, et al. Attenuation of Respiratory Syncytial Virus-Induced and RIG-I-dependent Type I IFN Responses in Human Neonates and Very Young Children. J Immunol (2014) 192(3):948–57. doi: 10.4049/jimmunol.1302007
20. Sharma AA, Jen R, Kan B, Sharma A, Marchant E, Tang A, et al. Impaired NLRP3 Inflammasome Activity During Fetal Development Regulates IL-1beta Production in Human Monocytes. Eur J Immunol (2015) 45(1):238–49. doi: 10.1002/eji.201444707
21. Paulos CM, Carpenito C, Plesa G, Suhoski MM, Varela-Rohena A, Golovina TN, et al. The Inducible Costimulator (ICOS) is Critical for the Development of Human T(H)17 Cells. Sci Transl Med (2010) 2(55):55ra78. doi: 10.1126/scitranslmed.3000448
22. Hollbacher B, Duhen T, Motley S, Klicznik MM, Gratz IK, Campbell DJ. Transcriptomic Profiling of Human Effector and Regulatory T Cell Subsets Identifies Predictive Population Signatures. Immunohorizons (2020) 4(10):585–96. doi: 10.4049/immunohorizons.2000037
23. Heikamp EB, Patel CH, Collins S, Waickman A, Oh MH, Sun IH, et al. The AGC Kinase SGK1 Regulates TH1 and TH2 Differentiation Downstream of the mTORC2 Complex. Nat Immunol (2014) 15(5):457–64. doi: 10.1038/ni.2867
24. Tripathi SK, Valikangas T, Shetty A, Khan MM, Moulder R, Bhosale SD, et al. Quantitative Proteomics Reveals the Dynamic Protein Landscape During Initiation of Human Th17 Cell Polarization. iScience (2019) 11:334–55. doi: 10.1016/j.isci.2018.12.020
25. Wu C, Yosef N, Thalhamer T, Zhu C, Xiao S, Kishi Y, et al. Induction of Pathogenic TH17 Cells by Inducible Salt-Sensing Kinase SGK1. Nature (2013) 496(7446):513–7. doi: 10.1038/nature11984
26. Wu C, Chen Z, Xiao S, Thalhamer T, Madi A, Han T, et al. SGK1 Governs the Reciprocal Development of Th17 and Regulatory T Cells. Cell Rep (2018) 22(3):653–65. doi: 10.1016/j.celrep.2017.12.068
27. Rutz S, Eidenschenk C, Ouyang W. IL-22, Not Simply a Th17 Cytokine. Immunol Rev (2013) 252(1):116–32. doi: 10.1111/imr.12027
28. Huber S, Gagliani N, Esplugues E, O’Connor W Jr, Huber FJ, Chaudhry A, et al. Th17 Cells Express Interleukin-10 Receptor and are Controlled by Foxp3(-) and Foxp3+ Regulatory CD4+ T Cells in an Interleukin-10-Dependent Manner. Immunity (2011) 34(4):554–65. doi: 10.1016/j.immuni.2011.01.020
29. Rudd BD. Neonatal T Cells: A Reinterpretation. Annu Rev Immunol (2020) 38:229–47. doi: 10.1146/annurev-immunol-091319-083608
30. Tuomela S, Salo V, Tripathi SK, Chen Z, Laurila K, Gupta B, et al. Identification of Early Gene Expression Changes During Human Th17 Cell Differentiation. Blood (2012) 119(23):e151–60. doi: 10.1182/blood-2012-01-407528
31. Egan CE, Sodhi CP, Good M, Lin J, Jia H, Yamaguchi Y, et al. Toll-Like Receptor 4-Mediated Lymphocyte Influx Induces Neonatal Necrotizing Enterocolitis. J Clin Invest (2016) 126(2):495–508. doi: 10.1172/JCI83356
32. Wynn JL, Wilson CS, Hawiger J, Scumpia PO, Marshall AF, Liu JH, et al. Targeting IL-17A Attenuates Neonatal Sepsis Mortality Induced by IL-18. Proc Natl Acad Sci U S A (2016) 113(19):E2627–35. doi: 10.1073/pnas.1515793113
33. Godfrey WR, Spoden DJ, Ge YG, Baker SR, Liu B, Levine BL, et al. Cord Blood CD4(+)CD25(+)-Derived T Regulatory Cell Lines Express FoxP3 Protein and Manifest Potent Suppressor Function. Blood (2005) 105(2):750–8. doi: 10.1182/blood-2004-06-2467
34. Dudakov JA, Hanash AM, van den Brink MR. Interleukin-22: Immunobiology and Pathology. Annu Rev Immunol (2015) 33:747–85. doi: 10.1146/annurev-immunol-032414-112123
35. Schreiber F, Arasteh JM, Lawley TD. Pathogen Resistance Mediated by IL-22 Signaling At the Epithelial-Microbiota Interface. J Mol Biol (2015) 427(23):3676–82. doi: 10.1016/j.jmb.2015.10.013
36. Sakamoto K, Kim YG, Hara H, Kamada N, Caballero-Flores G, Tolosano E, et al. IL-22 Controls Iron-Dependent Nutritional Immunity Against Systemic Bacterial Infections. Sci Immunol (2017) 2(8). doi: 10.1126/sciimmunol.aai8371
37. Fung TC, Bessman NJ, Hepworth MR, Kumar N, Shibata N, Kobuley D, et al. Lymphoid-Tissue-Resident Commensal Bacteria Promote Members of the IL-10 Cytokine Family to Establish Mutualism. Immunity (2016) 44(3):634–46. doi: 10.1016/j.immuni.2016.02.019
38. Levy R, Okada S, Beziat V, Moriya K, Liu C, Chai LY, et al. Genetic, Immunological, and Clinical Features of Patients With Bacterial and Fungal Infections Due to Inherited IL-17RA Deficiency. Proc Natl Acad Sci U S A (2016) 113(51):E8277–E85. doi: 10.1073/pnas.1618300114
39. Kotlarz D, Beier R, Murugan D, Diestelhorst J, Jensen O, Boztug K, et al. Loss of Interleukin-10 Signaling and Infantile Inflammatory Bowel Disease: Implications for Diagnosis and Therapy. Gastroenterology (2012) 143(2):347–55. doi: 10.1053/j.gastro.2012.04.045
40. Aschenbrenner D, Foglierini M, Jarrossay D, Hu D, Weiner HL, Kuchroo VK, et al. An Immunoregulatory and Tissue-Residency Program Modulated by c-MAF in Human TH17 Cells. Nat Immunol (2018) 19(10):1126–36. doi: 10.1038/s41590-018-0200-5
41. Malhotra N, Robertson E, Kang J. SMAD2 is Essential for TGF Beta-Mediated Th17 Cell Generation. J Biol Chem (2010) 285(38):29044–8. doi: 10.1074/jbc.C110.156745
42. Zhang S, Takaku M, Zou L, Gu AD, Chou WC, Zhang G, et al. Reversing SKI-SMAD4-Mediated Suppression is Essential for TH17 Cell Differentiation. Nature (2017) 551(7678):105–9. doi: 10.1038/nature24283
43. Rutz S, Noubade R, Eidenschenk C, Ota N, Zeng W, Zheng Y, et al. Transcription Factor c-Maf Mediates the TGF-beta-Dependent Suppression of IL-22 Production in T(H)17 Cells. Nat Immunol (2011) 12(12):1238–45. doi: 10.1038/ni.2134
44. Nurieva R, Yang XO, Martinez G, Zhang Y, Panopoulos AD, Ma L, et al. Essential Autocrine Regulation by IL-21 in the Generation of Inflammatory T Cells. Nature (2007) 448(7152):480–3. doi: 10.1038/nature05969
45. Korn T, Bettelli E, Gao W, Awasthi A, Jager A, Strom TB, et al. IL-21 Initiates an Alternative Pathway to Induce Proinflammatory T(H)17 Cells. Nature (2007) 448(7152):484–7. doi: 10.1038/nature05970
46. Zhou L, Ivanov II, Spolski R, Min R, Shenderov K, Egawa T, et al. IL-6 Programs T(H)-17 Cell Differentiation by Promoting Sequential Engagement of the IL-21 and IL-23 Pathways. Nat Immunol (2007) 8(9):967–74. doi: 10.1038/ni1488
47. Yeste A, Mascanfroni ID, Nadeau M, Burns EJ, Tukpah AM, Santiago A, et al. IL-21 Induces IL-22 Production in CD4+ T Cells. Nat Commun (2014) 5:3753. doi: 10.1038/ncomms4753
48. Haining WN, Weiss SA. c-Maf in CD4(+) T Cells: It’s All About Context. Nat Immunol (2018) 19(5):429–31. doi: 10.1038/s41590-018-0087-1
49. Peters A, Lee Y, Kuchroo VK. The Many Faces of Th17 Cells. Curr Opin Immunol (2011) 23(6):702–6. doi: 10.1016/j.coi.2011.08.007
50. Dijkstra KK, Hoeks SBEA, Prakken BJ, de Roock S. TH17 Differentiation Capacity Develops Within the First 3 Months of Life. J Allergy Clin Immunol (2014) 133(3):891–4.e5. doi: 10.1016/j.jaci.2013.09.022
51. Haas Jan D, Ravens S, Düber S, Sandrock I, Oberdörfer L, Kashani E, et al. Development of Interleukin-17-Producing γδ T Cells Is Restricted to a Functional Embryonic Wave. Immunity (2012) 37(1):48–59. doi: 10.1016/j.immuni.2012.06.003
52. Aggor FEY, Break TJ, Trevejo-Nunez G, Whibley N, Coleman BM, Bailey RD, et al. Oral Epithelial IL-22/STAT3 Signaling Licenses IL-17-mediated Immunity to Oral Mucosal Candidiasis. Sci Immunol (2020) 5(48). doi: 10.1126/sciimmunol.aba0570
Keywords: Stat3, gene regulation, T helper (Th) 17 cells, TGF-β signaling, neonatal, T cells
Citation: Razzaghian HR, Sharafian Z, Sharma AA, Boyce GK, Lee K, Da Silva R, Orban PC, Sekaly R-P, Ross CJ and Lavoie PM (2021) Neonatal T Helper 17 Responses Are Skewed Towards an Immunoregulatory Interleukin-22 Phenotype. Front. Immunol. 12:655027. doi: 10.3389/fimmu.2021.655027
Received: 18 January 2021; Accepted: 13 April 2021;
Published: 03 May 2021.
Edited by:
Monika C. Brunner-Weinzierl, University Hospital Magdeburg, GermanyReviewed by:
Takashi MaruYama, National Institutes of Health (NIH), United StatesValerie Dardalhon, UMR5535 Institut de Génétique Moléculaire de Montpellier (IGMM), France
Copyright © 2021 Razzaghian, Sharafian, Sharma, Boyce, Lee, Da Silva, Orban, Sekaly, Ross and Lavoie. This is an open-access article distributed under the terms of the Creative Commons Attribution License (CC BY). The use, distribution or reproduction in other forums is permitted, provided the original author(s) and the copyright owner(s) are credited and that the original publication in this journal is cited, in accordance with accepted academic practice. No use, distribution or reproduction is permitted which does not comply with these terms.
*Correspondence: Pascal M. Lavoie, cGxhdm9pZUBiY2Noci5jYQ==
†These authors have contributed equally to this work