- 1Xiangya School of Medicine, Central South University, Changsha, China
- 2Department of Oncology, The Second Xiangya Hospital of Central South University, Changsha, China
- 3Department of Radiology, Johns Hopkins University School of Medicine, Baltimore, MD, United States
- 4Department Cardiovascular Surgery, The Second Xiangya Hospital of Central South University, Changsha, China
- 5Department of Thoracic Surgery, The Second Xiangya Hospital of Central South University, Changsha, China
- 6Hunan Key Laboratory of Early Diagnosis and Precise Treatment of Lung Cancer, The Second Xiangya Hospital of Central South University, Changsha, China
The unique environment of the lungs is protected by complex immune interactions. Human lung tissue-resident memory T cells (TRM) have been shown to position at the pathogen entry points and play an essential role in fighting against viral and bacterial pathogens at the frontline through direct mechanisms and also by orchestrating the adaptive immune system through crosstalk. Recent evidence suggests that TRM cells also play a vital part in slowing down carcinogenesis and preventing the spread of solid tumors. Less beneficially, lung TRM cells can promote pathologic inflammation, causing chronic airway inflammatory changes such as asthma and fibrosis. TRM cells from infiltrating recipient T cells may also mediate allograft immunopathology, hence lung damage in patients after lung transplantations. Several therapeutic strategies targeting TRM cells have been developed. This review will summarize recent advances in understanding the establishment and maintenance of TRM cells in the lung, describe their roles in different lung diseases, and discuss how the TRM cells may guide future immunotherapies targeting infectious diseases, cancers and pathologic immune responses.
Introduction
Tissue-resident memory T (TRM) cells comprise a recently identified lymphocyte lineage that occupies tissues without recirculating. They reside in epithelial barrier tissues, such as lung, gastrointestinal tract, reproductive tract, and skin, and in some non-barrier tissues, such as brain, kidney, and joint (1–3). TRM cells are transcriptionally, functionally and phenotypically distinct from circulating effector memory T cells (4).
The human lung is continuously exposed to environmental and microbial antigens (2). TRM cells in lung tissues play a crucial role in both innate and adaptive immune responses to lung infections, such as Respiratory Syncytial Virus (RSV), SARS-CoV-2, Brucella and Mycobacterium tuberculosis (5–7). Growing evidence has revealed that the TRM cells reside in tissues in the absence of antigens and may provide rapid on-site immune protection against previously exposed pathogens in peripheral tissues to accelerate pathogen clearance (5).
Recently, TRM cells have been found to participate in anti-tumor immunity as well. TRM cells can promote intra-tumoral cytotoxic T lymphocytes (CTLs) responses and are correlated with overall survival in lung cancer patients (8–10). Induction of TRM cells can enhance the efficacy of cancer vaccines (11) and increase the response rate when using anti-PD-1 antibodies to reverse tumor-induced T cell exhaustion in NSCLC patients (12, 13).
In addition to the protective roles against diseases, evidence suggests that TRM cells also become activated after sensitization to self-antigens. Aberrantly activated TRM cells can induce autoimmune disorders, such as autoimmune hepatitis and psoriasis (4, 14). In the respiratory system, TRM specifically activated by environmental allergens might underlie the development and worsening of allergic asthma and other airway diseases (15–18). Pathogenic TRM cells may contribute to chronic pulmonary inflammation and fibrosis (2). TRM cells are also recognized as the primary mediator of acute cellular rejection (ACR) after lung transplantation.
This review aims to comprehensively summarize the current understandings of the biology of TRM cells, including the distinguishing molecular markers, regulators, and functions of TRM cells, discuss the contributions of TRM cells to lung diseases, especially infectious diseases and tumors, and highlight potential TRM-related therapeutic strategies for respiratory diseases.
Defining Lung TRM Cells
Human memory T cells can be broadly categorized into three subsets: central memory T cells (TCM), effector memory T cells (TEM), and tissue-resident memory T cells (TRM). TCM cells are memory T cells that recirculate through secondary lymphoid organs, whereas TEM cells recirculate through nonlymphoid tissues. TRM cells, by contrast, typically reside in specific tissues, especially mucus organs, such as lungs and gastrointestinal tracts, defending against pathogens in peripheral nonlymphoid tissues. Compared with TCM and TEM cells, commitment to the tissue of residence is a defining characteristic of TRM cells, which has been described in almost all organs (19). Most memory T cells in non-lymphoid tissues are TRM cells, either CD4+ or CD8+ (9). TRM cells can be further fractionated by their functional characteristics into epithelial and stromal TRM cells (20, 21).
TRM cells are widely distributed throughout the body, including the skin, lungs, lymphoid organs, etc. However, TRM cells in different organs display distinct properties (21). In healthy human skin, most of the TRM cells are dermal CD4+CD69+CD103− cells, which express high levels of the cutaneous lymphocyte antigen (CLA) and specific chemokine receptors like CCR4 (22). With increased expression of T cell factor-1 (TCF-1) and lymphoid enhancer factor-1 (LEF-1), human lymph node CD8+ TRM cells exhibit a phenotype of tissue residency as well as an organ-specific signature (23). Human lymph node-specific profile of memory CD8+ T cells is defined by expression of CXCR5 and TCF-1 and high proliferative capacity, which accordingly indicates that human lymph node memory CD8+ T cells display higher proliferative capacity than their counterparts in other tissues (24). Similarly, TRM cells in non-small-cell lung cancer (NSCLC) may be identified by CD39 and CD103 (25). In mice, the formation and maintenance of skin TRM cells is mediated by chemokine receptors like CXCR3, CXCR6, and CCR10 (26). Genetic knockout studies of mice have shown that CD69 deficiency reduces the retention of CD4+ T cells in the bone marrow (27).
Eomes and T-bet are T-box transcription factors (TFs) that restrict the formation of CD103+TRM cells, indicating that downregulation of both transcription factors is crucial for the generation of CD103+ TRM cells. Eomes TF is significantly downregulated in CD8+CD103+TRM cells compared to circulating TEM or TCM cells. The residual T-bet expression upregulates interleukin-15 receptor (IL-15R) β-chain (CD122) expression, which is essential for long-term TRM cell survival. The coordinated downregulation of both T-box TFs optimizes cytokine transforming growth factor-beta (TGF-β) signaling, leading to the efficient development of CD8+CD103+TRM cells (28).
Both CD69 and CD103 are expressed in CD8+ TRM cells and less frequently, CD103 is expressed in CD4+TRM cells (29). CD69, which is an early activation marker involved in lymphocyte proliferation and retention, plays a key role in distinguishing TRM cells from those in circulation. Additionally, CD103 is a key to recognize most CD4+and CD8+TRM cells (30). The expression of CD103 helps TRM cells dock to the E-cadherin-expressed on epithelial cells and prevents them from re-circulating in the blood (31). It is generally accepted that TGF-β is an upstream regulator of TRM transformation. Increasing TGF-β in vivo appears to significantly increase the number of local TRM cells (32, 33). It has been revealed that the function and expression of CD103 greatly depends on the TGF-β, indicating that the TEM and other T cells might lack TGF-β cytokines and thus fail to upregulate CD103 (34). CD39 is also highly expressed in TRM cells and is associated with higher TRM cells activities and quantity. CD39 could protect cells from apoptosis induced by adenosine triphosphate (ATP). As a transmembrane glycoprotein and extracellular nucleosidase, CD39 is also present in many biological processes such as adenosine regulation, proliferation, and resident transduction signals (28). While most human CD4+T cells express CD69, a portion of them express CD103+at the same time, especially in the lung. The majority of lung CD4+ TEM phenotype cells express the canonical TRM marker CD69. Comparing the gene expression patterns of CD103+TRM cells in lung and TEM cells in the blood, human lung CD4+CD103+TRM cells express higher levels of ITGAE (which encodes CD103), CTLA4, KLRC1 (which encodes the inhibitory receptor NKG2A) (35, 36) and ICOS (31). CD4+CD103+TRM cells express deployment-ready mRNAs encoding effector molecules that rapidly respond to pathogens. Human lung TRM cells express lower levels of S1PR1 (which encodes the S1P receptor) (37), lymph node-homing molecules, SELL (which encodes the lymph node-homing receptor CD62L), KLRG1 (which encodes the activation marker KLRG1) (35), KLF2 (which drives expression of S1PR1) and CCR7 (31). Additionally, some genes have different expressions between CD103+TRM cells and peripheral TEM cells, including genes that encode heat-shock proteins (HSPA1A, HSPA7, HSPA2, and HSPD1), transcription factors (EGR2, FOSB, ATF3, RBPJ, EPAS1, and BATF) (31, 35), anti-apoptotic factors (PHLDA1 and BIRC3), the tumor necrosis factor (TNF) receptor signaling family (TRAF1 and TANK) (31), chemokine (XCL1), solute carrier family members mediating amino acid transport (SLC7A5 and SLC1A5), chemokine receptor (CXCR6), transforming growth factor (TGF-β1), interleukin (IL-21R), the ligand for the death receptor Fas (FASLG), adhesion G-protein-coupled receptor (CD97), interferon-γ receptors (IFNGR) (35), and fatty-acid-binding protein (FABP5) (38). All the genes mentioned above have higher expression levels in lung CD103+TRM cells. Characteristically, lung CD4+CD103+TRM cells exhibit high levels of various integrins and adhesion molecules (31, 39). Compared with blood-derived TEM cells, differences in the molecular expression of lung CD103+TRM cells are summarized in Table 1.
Several transcription factors play critical roles in the proliferation of TRM cells. The activation of the programmed cell death protein 1(PD-1) signal pathway downregulates the expression of Bhlhe40, which supports mitochondria and chromatin production in TRM cells and is thus essential to the proliferation and maintenance of TRM cells (40). Transcription factors such as BATF, NAB1, and NAB2 are also highly expressed in TRM cells. These factors can regulate T cell metabolisms to maintain their survival and reduce the expression of inhibitory phenotypes (41).
Lung TRM Cells and Protection Against Respiratory Infection
CD8+TRM cells are considered as the first line of defense in peripheral tissues against pathogens. Many studies suggest that some risk factors may interfere with circulating memory CD8+T cell function (Table 2 and Figure 1). Reticular fibroblasts located near T cells around the infection site can transmit long-lasting activation signals to CD8+T cells by upregulating ICOS ligand (ICOSL), CD40, and interleukin-6 (IL-6), which promotes the preferential differentiation of T cells into TRM cells (55). CD8+TRM cells can produce chemokines after local tissue activation and recruit non-antigen-specific T cells, exerting natural effector functions (56, 57). TRM cells promote the production of IL-2 and some pro-inflammatory cytokines, effectively mobilizing inflammatory responses and exert immune defenses (58). At the same time, TRM cells produce IL-10 and express inhibitory receptors, thus inhibit excessive inflammatory response and limit tissue damage caused by inflammation (59). CD4+ TRM cells in non-lymphoid tissues, such as lung, skin, and genital mucosa, can influence the immune reaction of various pathogenic microorganisms (60–62).
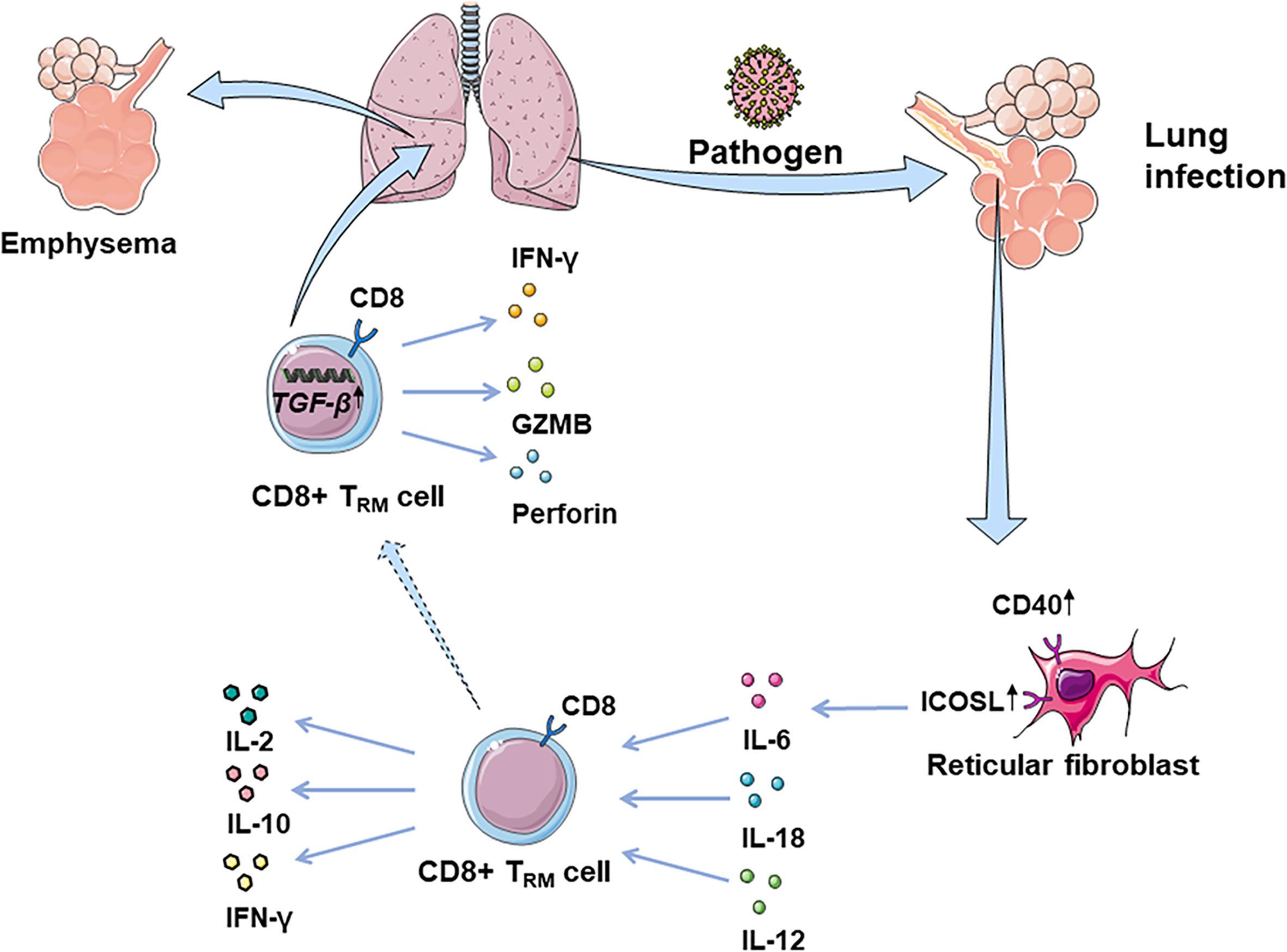
Figure 1 CD8+ TRM cells in lung infection and immunopathology. CD8+ TRM cells are considered as the first line of defense in peripheral tissues against earlier exposure to antigens. CD8+ TRM cells located in the lung parenchyma could rapidly synthesize IFN-γ following the inhalation of pathogens, driven by exposure to IL-12/IL-18. Fibroblast reticular cells located near T cells around the focus can transmit long-lasting activation signals to CD8+T cells, by upregulating ICOSL, CD40, and IL-6. Additionally, CD8+ TRM cells promote the production of IL-2, mobilizing inflammatory response. At the same time, TRM cells can produce IL-10, thus inhibiting the excessive inflammatory response and limiting tissue damage caused by inflammation. However, CD8+ TRM cells can be abnormally deposited in the lung due to overexpression of TGF-β-related genes, which may damage normal tissues by releasing IFN-γ, GZMB, and perforin, leading to lung emphysema or fibrosis.
Antivirus Effect
In lungs, follicular tissue-resident CD4+ T helper cells contribute to the defense against virus in conjunction with CD8+ T cells relying on IL-21 (63). These helper T cells can also induce antiviral B cell reactions in bronchus lymphoid tissue in flu virus infection, indicating that TRM cells could promote the protective response of B cells and CD8+T cells in lung infections (63). CD8+TRM cells in the lung act as protective agents against viruses through interferon-γ (IFN-γ) (64). Studies of Coronavirus disease 2019 (COVID-19) have suggested that the disease severity and lung injuries are related to the interaction of tissue-resident memory-like Th17 cells (TRM17 cells) with lung macrophages and cytotoxic CD8+T cells. High serum IL-17A and GM-CSF levels in COVID-19 patients are associated with more severe clinical courses (6). Overall, lung TRM17 cells are potential coordinators of excessive inflammation in severe COVID-19 (6). Patients recovering from COVID-19 acquire TRM cells with Th1 phenotype against COVID-19 (65). Neutrophils attracted to the site of infection secret IL-17A and stimulate lung epithelial cells to express CCL20. The expression of CCL20 recruits natural Th17 (nTh17) cells to the infected site. In the presence of IL-23, nTh17 are converted to TRM cells (66). COVID-19 prevents the TRM cells from remaining ex-Th17 cells and exerting Th1-like immunity effects (42). Previous studies showed in RSV-immune mice, TRM cells enhanced respiratory syncytial virus clearance, indicating CD8+TRM cells can enhance resistance against secondary RSV infection (43, 44). In RSV-immune mice, CD69 co-expressed heavily with CD38, consistent with its role as an early activation marker. Some CD4+CD69+T cells also expressed integrin CD103, and permanent memory CD4+T cells were enriched in airways. As the infection progressed, these TRM cells were enriched in infection site with increased CXCR3 expression (45). Similarly, TRM cells protect the human body from influenza viruses by producing large amounts of IFN-γ and TNF-α (50). When encountered with influenza A virus, dendritic cells with high expression of IRF4 can effectively promote the production of CD8+TRM cells, thus reducing infection severity (67). PPAR-γ expression accelerates the establishment of CD8+TRM cells, suggesting that PPAR-γ is a positive regulatory factor for TRM cells. Moreover, PPAR-γ deficiency reduces the number of alveolar macrophages residing in tissues during pulmonary infections, indicating that alveolar macrophages might be negative regulators of CD8+TRM cells and could limit the establishment of TRM cells (51).
Antibacterial Effect
Non-homologous bystander activation can trigger the sensory and alerting functions of lung CD8+TRM cells (68). Unlike memory CD8+T cells in circulating blood, CD8+TRM cells located in the lung parenchyma can rapidly synthesize IFN-γ after the inhalation of heat-killed bacteria or bacterial products, a process-driven by exposure to IL-12/IL-18 (69). Bacterial infection of respiratory tract leads to bystander activation of pulmonary TRM cells, enhancement of the recruitment of neutrophils to the airway and reduction of the severity of bacterial pneumonia (69). These activations suggest that TRM cells innately amplify inflammatory responses and participate in non-homologous responses to bacterial infections (68). Lung CD4+TRM cells remodel epithelial responses to accelerate neutrophil recruitment during pneumonia. During heterotypic immunity, CD4+T cells upregulate CXCL5 and drive neutrophil recruitment in the lung (70). In mice infected with Bordetella pertussis, TRM cells produced IL-17 and IFN-γ, recruiting neutrophils and preventing nasal colonization (46–49). In addition, uninfected mice acquired immunity after receiving adoptive transferred CD4 T cells isolated from either lungs or spleens of convalescent mice (71). Following mucosal znBAZ vaccination, lung CD8+ TRM cells exhibit superior protection against Brucella infections. Mucosal znBAZ immunization induces CD103+ and CD103- CD8+ TRM cells expressing CXCR3lo and CXCR3hi phenotypes in the lung parenchyma and airways, respectively. These CXCR3-expressing CD103+ and CD103-CD8+TRM cells are not depleted by anti-CD8 mAb treatment (52).
Other Effect
TRM cells also increase resistance to parasite invasions. Both the percentage and absolute numbers of lung CD4+ and CD8+ cells increase after Schistosoma. japonicum infection (72). CD103-expressing pulmonary CD4+ and CD8+ T cells play essential roles in mediating granulomatous inflammation induced by S. japonicum infection (72).
Lung TRM Cells in Anti-Tumor Immunity
Approximately 85% of lung cancers worldwide are non-small cell lung cancer (NSCLC), of which lung adenocarcinoma (LUAD) and lung squamous cell carcinoma (LUSC) are the most common (73). Growing evidence suggested that in human solid tumors, tumor-associated lymphocytes in NSCLC may comprise the function of TRM cells (25). CD8+TRM cells in the tumor microenvironment (TME) are a homogeneous CD103+CD49+CD69+ population expressing T-bet, porylated (p)STAT-3, and Aiolos transcription factors and a subset of these cells produces IFN-γ and IL-17. In patients with NSCLC, CD8+TRM cells overexpress several T cell inhibitory receptors and exhaustion surface markers and co-express PD-1 and CD39, implying that they are enriched in activated tumor-antigen reactive T cells (74). Cytotoxic CD8+T lymphocytes (CTLs) in NSCLC with a high level of CD103 display enhanced cytotoxicity and proliferation, suggesting a robust anti-tumor immune response in human lung cancer (41). Compared to T cells from adjacent and tumor-free lung tissues, these cells exhibit more significant heterogeneity in the expression of molecules associated with T cell antigen receptor (TCR) activation and immune checkpoints such as 4-1BB, PD-1, TIM-3. However, in human lung cancer, far from being exhausted, PD-1-expressing TRM cells in tumors are clonally expanded and enriched for transcripts linked to cell proliferation and cytotoxicity (12).
The origination, infiltration, and differentiation of TRM in NSCLC is still unclear. O’Brien and colleagues’ model speculated that in patients with early-stage NSCLC, TEM cells encountered antigens during tumor formation and were converted to CD103+TRM cells that exerted anti-tumor activity (75). However, due to a variety of factors associated with tumor growth in TME, the tumors might not be eliminated. These factors, combined with the chronic antigen stimulation, may trigger an exhaustion program characterized by increased Eomes and CD39 expression. The presence of B7-H4 in tumors or other TME stromal cells might upregulate Eomes expression in T cells. As a tumor grows, this exhaustion program may dominate TRM cells, causing increasing TILs hypofunctionality.
In addition, transcription factors may play a role. Patients suffering from advanced-stage NSCLC exhibit a progressive decrease of NFATc1 in tumor cells and TILs decrease progressively (76). Some CD103+TRM cells may lead to decreased function and cytotoxicity of CD8+T cells, a phenomenon observed in the lungs of tumor-bearing NFATc1ΔCD4 mice, likely promoted by decreased IL-2 in the absence of NFATc1 (77). Runx3 also plays an important part in the differentiation of TRM in NSCLC (78). The Runx3 is required for optimal TRM cell differentiation in the lung parenchyma and maximal expression of granzyme B in TRM cells. Several tissue-residency signature genes are upregulated in Runx3-overexpressing cells and downregulated in Runx3-deficient cells. Conversely, circulating memory cell signatures are enriched in Runx3-deficient cells and depleted from Runx3-overexpressing cells.
Other immune cells such as M1hot tumor-associated macrophages (TAM) can boost the infiltration and survival of TRM cells in patients with NSCLC (32). M1hot TAMs may recruit TRM cells via CXCL9 expression and sustain them by producing more essential fatty acids on which TRM cells depend. Monocytes acquire the ability to prime TRM cells via IL-10-mediated TGF-β release. IL-10 plays a negative regulatory role in the immune system per classical theory, but it has been found that CD103 was significantly upregulated, and T cells transform into TRM cells when influenced by IL-10 (33). Significantly upregulated CD103 leads to more T cells transforming into TRM cells. Therefore, IL-10-mediated TGF-β signaling may have a critical role in post-vaccination TRM generation (33).
Moreover, chemokine receptors and cytoskeleton proteins contribute to TRM cell infiltration. The focal adhesion-associated protein paxillin binding to the CD103 cytoplasmic tail triggers αEβ7 integrin outside-in signaling that promotes the migration and functions of CD8+T cells (79). This binding process explains the more favorable prognosis associated with more retention of TRM cells in TME (79). In both mice and human lungs, CXCR6 is expressed on the surface of TRM cells with the action of intrapulmonary antigens, aiding the migration of TRM cells from pulmonary interstitium to TME and maintaining the TRM cell pool (80), whereas memory CD8+T cells of the spleen do not express this receptor (81).
Duhen et al. proposed a model in human solid tumors associating TRM cells with tumor growth: CD8+ T cells are primed by dendritic cells presenting tumor antigens within the tumor-draining lymph nodes and then migrate to the tumor where they recognize the cognate antigens then clonally expand (25). The consequence of this TCR activation in a TGF-β-rich environment is the upregulation of CD39 and CD103 on CD8+ TILs. CD103 expressed on some TRM cells may promote immunologic synapse by binding to E-cadherin on tumor cells (82). Activation of these cells also leads to the downregulation of the proteins that are essential for T-cell recirculation, retaining CD8+ TILs within the tumor. In human lung cancer, there are many important anti-tumor costimulatory molecules such as SIRPG and KIR2DL4 on the surface of TRM cells, which help the CTLs kill tumor cells (41). In human, CD103+ TRM cells can also produce granzyme B (GZMB) and IFN-γ, which can restrict tumor cell growth and metastasis by inducing fibronectin production, make antigens available to prime for the priming of new tumor-specific T cells, and enhance recruitment of monocytes, NK cells, and XCR1+ cDC1 to the tumor site (9). However, repetitive TCR stimulations of the CD8+ TILs impair effector function, mediate immune escape, and ultimately tumor progression. CD103+CD39+CD8+TRM cells efficiently kill autologous tumor cells in an MHC-class I-dependent manner. Additionally, the content of cytokine and receptor function influence immune functions (Figure 2).
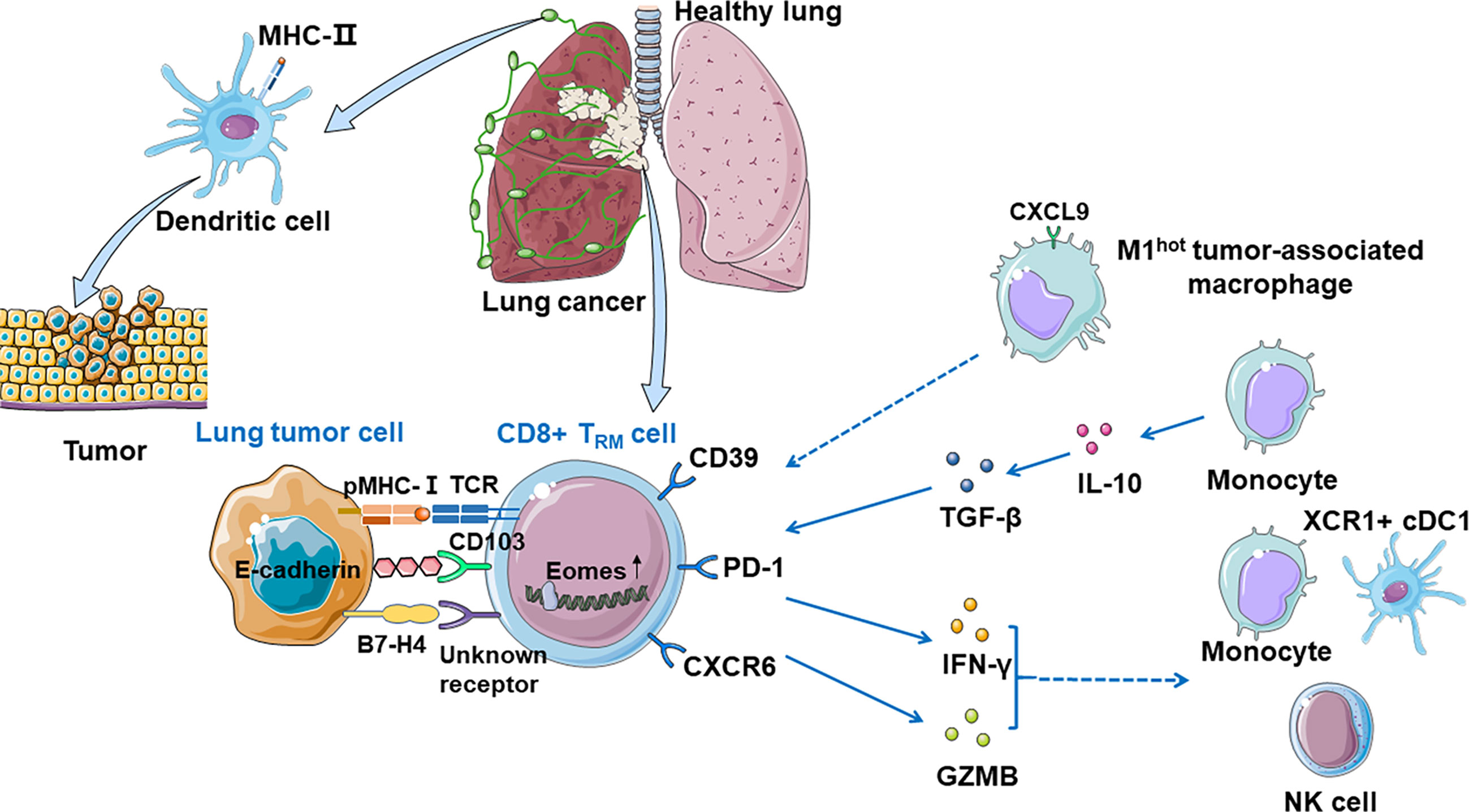
Figure 2 CD8+ TRM cells in anti-tumor immunity. Dendritic cells present tumor antigens in the tumor-draining lymph nodes and then migrate to the tumor where they recognize their cognate antigens and expand, priming CD8+ T cells significantly. Tumor-associated CD8+ TRM cells can be identified by CD39 and CD103, and CD103 promotes immunologic synapse by binding to E-cadherin on tumor cells. TRM cells which express PD-1 are expanded and enriched for transcripts linked to cell proliferation and cytotoxicity. CXCR6 is expressed on the surface of TRM cells when exposed to tumor antigens, transferring TRM cells from pulmonary interstitium to tumor microenvironment, and maintaining the TRM cell pool. M1hot TAMs recruit TRM cells via CXCL9 expression and sustain them by making more essential fatty acids on which TRM cells depend. Monocytes prime TRM cells via IL-10-mediated TGF-β release which increases the number of local TRM cells. CD8+ CD103+ TRM cells can also produce GZMB and IFN-γ, which recruits monocytes, NK cells, and XCR1+ cDC1 to the tumor site. B7-H4 on tumor cells might upregulate Eomes in T cells, which may cause growing TILs hypofunctionality.
The infiltration of CD8+ T cells in solid tumors is a favorable prognostic marker (83). In the tumors that exhibited a high level of infiltrated CD8+ T cells, the proliferation of CD103+ T cells was correlated with improved long-term survival, indicating that infiltration of CD8+CD103+ TRM cells is a favorable prognostic marker (84). Another study suggested that high intratumoral but not stromal CD103+ TILs were associated with better overall survival in patients with resected LUSC, another significant prognostic implication of CD103 expression in TILs in human LUSC (85). Moreover, CD103 and E-cadherin interaction play a vital role in granule polarization and exocytosis, enhancing recruitment and retention of tumor-antigen-specific TILs in human NSCLC. In human NSCLC, CD28H is mainly expressed in TRM cells and is thus associated with improved tumor prognosis (86). However, other studies conversely demonstrated opposite results that B7-H5 (the ligand of CD28H) was expressed in more than 60% of cases of NSCLC and was associated with worse prognosis. Hence, the expression patterns of CD103 in TILs of NSCLC and the associated prognostic implications are significant and merit further investigation.
In TRM cells in human NSCLC tissues, there are several dysfunctional subtypes, such as NKG2A+CD8+ T cells (87). NKG2A is an inhibitory receptor of both T cells and natural killer (NK) cells. Persistent activation causes T cells and NK cells to express NKG2A and may lead to chronic infection and cancer. Tumor-infiltrating NKG2A+CD8+T cells form the predominant subset of NKG2A+cells in human lung cancer (87). Blockading NKG2A may promote anti-tumor immunity by unleashing dysfunctional CD8+T cells in tumors, and targeting NKG2A+CD8+T cells is a promising approach for future anti-lung cancer immunotherapy.
While numerous studies have reported that the presence of TRM-like CD8+T cells in human NSCLC is a favorable prognosis (77), the role of CD4+TILs with a shared phenotype remains unclear. As CD4+TRM cells exhibit phenotypic and functional heterogeneity, different subsets are expected to play different and even opposite roles in TME. CD4+TRM cells are known to be essential for cytotoxic programming of CD8+T cells, and they can also suppress tumor growth through secretion of IFN-γ or direct killing tumor cells in human NSCLC (88).
Potential Therapeutic Strategies Based on TRM Cells
Given the remarkable roles of TRM cells in lung diseases, increasing TRM production or reactivating suppressed TRM cells may be a valuable therapeutic strategy (Table 3). It is believed that in lung diseases without medical intervention, TRM cells play a less critical role because their function is inhibited and disabled in the focal microenvironment (88). Therefore, current researches focus on reactivating TRM cells that have adapted to the disease microenvironment, increasing the load of TRM cells in the lesions, and mediating immune responses such as cytotoxicity and conditioning, to kill pathogens or slow down disease progression (Table 4).

Table 3 Strategies to improve the efficacy of vaccines and adoptive cell therapies by targeting TRM cells.
TRM Cells and Neoadjuvant Chemotherapy
TRM cells may be involved in varied NSCLC therapies. Neoadjuvant chemotherapy was one modality in the treatment of resectable NSCLC. The beneficial effects of neoadjuvant chemotherapy might be mediated partially by CD8+CD103+ mediated tumor cell killing (13). With neoadjuvant chemotherapy, more infiltration of CD4+CD103+PD-1 TRM cells at the time of surgery was associated with longer overall survival. Moreover, TRM cells could be of great importance in TME and in cancer immune checkpoint blockade immunotherapy. In both mice model and human, dual anti-PD-L1/anti-4-1BB immunotherapy increased the number of intratumoral CD103+CD8+ T cells and altered their distribution (90). Administration of PD-L1 mAb and 4-1BB mAb further increased the cytolytic capacity of CD103+CD8+T cells. Collectively, infiltrated CD103+CD8+T cells served as a potential effector T cell population. Combining 4-1BB agonism with PD-L1 blockade may increase tumor-infiltrated CD103+CD8+T cells, facilitating tumor regression. It is also reported that CD103+CD8+TRM cells could be considered potential biomarkers when selecting patients that may benefit from immune checkpoint blockade immunotherapy in patients with multiple primary lung adenocarcinoma after neoadjuvant immunotherapy (91). Yet more evidence is required to determine the clinical practice of potential the therapeutic strategies based on TRM cells, as a more favorable indicator of prognosis or a target of immune therapy.
Several treatments may potentially activate or increase the number of TRM cells. One possible treatment involves promoting the separation and trans-differentiation of T cells to TRM cells in TME, which could inhibit tumor progression. At the same time, in murine models, apoptosis induced by IR increases the number of newly infiltrated T cells and converts them into TRM cells, producing an inflammation-like effect that may assist immunotherapy (92).
TRM Cells and Radiotherapy
Since TRM cells have a unique survival advantage in radiotherapy, a RT-PD1-MerTK triple therapy based on radiotherapy may also be effective. Promoting TRM cell production from other sources such as traditional radiotherapy may be an equally valuable potential treatment. TRM cells have stronger radiation resistance than tumor cells, and efficient infrared irradiation (IR) makes pre-existing TRM cells survive and mediates the anti-tumor effect of TRM cells (93). In murine models, compared with traditional radiotherapy, anti-PD-1 therapy relieves the inhibitory effect on immune cells such as TRM cells, while anti-MerTK can transform apoptosis caused by radiation into cell necrosis and turn macrophages near tumors from M2 to M1 and reduce tumor load (94). This triple therapy could increase the content of CTLs and promote the differentiation of TRM cells, improving the anti-tumor effect. Adding anti-PD1 and anti-MerTK to radiation could significantly upregulate CD8+CD103+TRM at the abscopal tumors, suppress the abscopal tumor growth and extended the survival rate (95). As for epigenetic and metabolic regulation, a treatment scheme for TA/AC may be considered. TA, or microtubule inhibitor A, is a histone deacetylase inhibitor that can promote the production of IFN- γ in Bhlhe40+CD8+TRM cells (96). Acetic acid (AC) can be used as the substrate of acetyl-CoA synthesis, which is independent from the tricarboxylic acid (TCA) cycle and promotes histone acetylation and cytokine production in Bhlhe40+CD8+ TRM cells (97). The combination of TA and AC can promote tissue retention and functional differentiation of TRM cells (40). TA/Ac treatment not only enhances Bhlhe40−/− CD8+ T cell effector and resident gene expression but also promotes the expression of these genes in WT CD8+ T cells, indicating appropriate combinations of epigenetic modifiers with certain metabolites may represent promising approaches for maximally reinvigorating tissue or tumor-resident CD8+ T cell antiviral or antitumor activities.
TRM Cells and Vaccines
Another treatment approach involves the induction of persistent TRM cells by vaccines. Phosphatidylserine liposomes are excellent antigen carriers, which can be combined with polyconic adjuvants for the development of new BCG vaccines (71). Because anti-cytomegalovirus response is one of the most powerful and persistent cellular immune responses observed in human bodies, cytomegalovirus is a possible effective TRM-cell-inducing vaccine vector (98). Murine models show that other peptide nanofibers with strong immunogenicity may likewise improve the immune response (99), particularly with combined polypeptide antigen and adjuvant (33). Continuous stimulation with local homologous antigens can increase TRM cell population, and zymosan used as an adjuvant could transform CD8+T cells into TRM cells in the absence of antigens. Mice models indicate that adding zymosan adjuvant to a possible vaccine may moderate local inflammation as well as greatly enhance the production of TRM cells (100). Similarly, combining ovalbumin antigen and CpG DNA adjuvant hybridized pH-responsive substances can increase the TRM cells response range and lifespan. This combination can also activate antigen-presenting cells (APCs), and stimulate continuous TRM cell production in mice (101).
Intranasal vaccine administration induces TRM cells in the lung (11). Triggering an appropriate inflammatory response in the immune process may allow TRM cells to bypass antigen recognition. Lung TRM cells are most effectively induced at the memory stage of basic vaccines in murine models (99). In-depth analysis of the phenotypes of the locally induced CD8+T cells showed that after vaccination, TRM cells and CD8+T cells coexist as effector phenotypes and that TRM cells play an important role. Indeed, at the peak of the local immune response, concentrations of TRM are 10-fold higher than those of effector CD8+T cells, and only the TRM cells population persist locally after 30 days. Even when effector CD8+T cells are no longer detectable, tumor resistance is still observed (11).
The CXCR6-CXCL16 axis demonstrably governs the growth of NSCLC in the migration of CD8+ resident memory T cells in lung mucosa after vaccination. CXCR6 deficiency impairs cancer vaccine efficacy and CD8+ resident memory T-cell recruitment in lung tumors (80). Interestingly, intranasal vaccination induces higher and more sustained concentrations of CXCL16 than intramuscular vaccination, particularly compared with other chemokines in the bronchoalveolar lavage fluid and pulmonary parenchyma in both mice and human (81).
Despite it is observed that vaccines can promote the TRM cells population, retention and function and then enhance the anti-tumor immunity both in mice and human, the efficiency and safety of tumor-related vaccines remains unclear thus require further investigations.
Lung TRM and Immunopathology
In certain conditions, lung TRM cells may cause excessive inflammatory responses and tissue fibrosis (Table 4). After acute influenza infection, abnormal reactivation of TRM cells in the lung may likewise cause lung tissue changes and fibrosis (102). In elderly mice, CD8+ TRM cells can be abnormally deposited in the lung due to overexpression of TGF-β-related genes (103). Low responsive TRM cells not only fail to perform an immune function but may also lead to chronic inflammation and the sequelae of fibrosis (89). CD69hiCD103loCD4+ TRM cells produce effector cytokines and promoted the inflammation and fibrotic responses induced by chronic exposure to Aspergillus fumigatus (15). Studies have shown that pathogenic immune cells like CD69hiCD103loCD4+TRM cells enhance the secretion of IL-5 and IL-13, which can cause excessive pulmonary inflammation and fibrosis. By contrast, CD69hiCD103hiCD4+TRM cells can improve the fibrosis reaction caused by pulmonary inflammation and reduce lung injury, indicating that lung CD4+ TRM cells play crucial roles in the pathology of chronic lung inflammation, and CD103 expression defines pathogenic effector and immunosuppressive TRM cell subpopulations in the the lung (15).
Association With Asthma
Th2-TRM cells are associated with asthma. These cells release cytokines that recruit eosinophils and sustain mast cells in the airway, leading to an inflammatory response. Th2-TRM cells expressed with high levels of CD44 and ST2 have been observed in lung tissues and can retain allergen memory throughout the life of a host organism (53). Re-exposure to a known allergen causes Th2-TRM cells to proliferate near the airway, producing type 2 cytokines that enhance eosinophil activation and promote peribronchial inflammation (104). Together with circulating memory Th2 cells, they perform non-redundant functions in asthma induction (18, 53, 54). Although these TRM cells eliminate invasive pathogens, the release of pro-inflammatory factors (such as IFN-γ, perforin, and granulose) may damage normal cells, leading to lung damage, emphysema, or fibrosis.
Participation in Acute Cellular Rejection (ACR) After Lung Transplantation
T cells are mediators of acute cellular rejection (ACR) after lung transplantation (105). The role of pulmonary TRM cells in ACR in lung transplantation remains uncertain. Longitudinal analysis of lung transplant recipients has indicated that TRM cells from recipients gradually formed TRM phenotypes approximating healthy people after 6 months allograft, while donor T cells persisted in the form of TRM. The increase in the proportion of recipient-derived TRM cells was associated with ACR, suggesting that TRM cells may influence the inflammatory environment of lung allograft after transplantation (2).
Conclusion
Human lung TRM cells, whether CD8+ or CD4+, persist in lung tissues for decades of human life. The essential role of lung TRM cells is maintaining tissue homeostasis when facing viruses, antigens, and pathogens encountered through respiration, and may also be important in tumor surveillance. Lung TRM cells can also promote pathologic inflammation, inducing chronic airway inflammatory changes leading to asthma and fibrosis. Similarly, lung TRM cells from infiltrating recipient T cells in transplantation may mediate allograft immunopathology and promote lung damage. More comprehensive understanding of the induction and maintenance of TRM cells by cancer vaccines or other immunotherapeutic approaches may provide insights into the innovation of immunotherapies of lung diseases.
Author Contributions
CC provided the concepts and ideas of the article. RY, JY, ZJ, and JL performed literature search and wrote the manuscript’s first draft. CC, FW, XH, and RY performed a critical revision of the first draft and the final editing of the manuscript. All authors contributed to the article and approved the submitted version.
Funding
This study was funded by the China National Natural Science Foundation No. 81902351, the Hunan Provincial Natural Science Foundation (No. 2020SK53419, 2021JJ30926 and No. 2019JJ50953), Hunan Provincial Key Area R&D Program NO. 2019SK2253, CSCO Cancer Research Foundation (CSCO-Y-young2019-034 and CSCO-2019Roche-073), and the Changsha Municipal Natural Science Foundation NO. kq2014246.
Conflict of Interest
The authors declare that the research was conducted in the absence of any commercial or financial relationships that could be construed as a potential conflict of interest.
Publisher’s Note
All claims expressed in this article are solely those of the authors and do not necessarily represent those of their affiliated organizations, or those of the publisher, the editors and the reviewers. Any product that may be evaluated in this article, or claim that may be made by its manufacturer, is not guaranteed or endorsed by the publisher.
Acknowledgments
The authors greatly appreciate Mr. Jameson Goodman for critical review of the manuscript.
References
1. Clark RA. Resident Memory T Cells in Human Health and Disease. Sci Transl Med (2015) 7(269):269rv1. doi: 10.1126/scitranslmed.3010641
2. Snyder ME, Farber DL. Human Lung Tissue Resident Memory T Cells in Health and Disease. Curr Opin Immunol (2019) 59:101–8. doi: 10.1016/j.coi.2019.05.011
3. Schenkel JM, Masopust D. Tissue-Resident Memory T Cells. Immunity (2014) 41(6):886–97. doi: 10.1016/j.immuni.2014.12.007
4. Wu H, Liao W, Li Q, Long H, Yin H, Zhao M, et al. Pathogenic Role of Tissue-Resident Memory T Cells in Autoimmune Diseases. Autoimmun Rev (2018) 17(9):906–11. doi: 10.1016/j.autrev.2018.03.014
5. Gebhardt T, Palendira U, Tscharke DC, Bedoui S. Tissue-Resident Memory T Cells in Tissue Homeostasis, Persistent Infection, and Cancer Surveillance. Immunol Rev (2018) 283(1):54–76. doi: 10.1111/imr.12650
6. Zhao Y, Kilian C, Turner JE, Bosurgi L, Roedl K, Bartsch P, et al. Clonal Expansion and Activation of Tissue-Resident Memory-Like Th17 Cells Expressing GM-CSF in the Lungs of Severe COVID-19 Patients. Sci Immunol (2021) 6(56):eabf6692. doi: 10.1126/sciimmunol.abf6692
7. Ogongo P, Porterfield JZ, Leslie A. Lung Tissue Resident Memory T-Cells in the Immune Response to Mycobacterium Tuberculosis. Front Immunol (2019) 10:992. doi: 10.3389/fimmu.2019.00992
8. Park SL, Gebhardt T, Mackay LK. Tissue-Resident Memory T Cells in Cancer Immunosurveillance. Trends Immunol (2019) 40(8):735–47. doi: 10.1016/j.it.2019.06.002
9. Amsen D, van Gisbergen K, Hombrink P, van Lier RAW. Tissue-Resident Memory T Cells at the Center of Immunity to Solid Tumors. Nat Immunol (2018) 19(6):538–46. doi: 10.1038/s41590-018-0114-2
10. Djenidi F, Adam J, Goubar A, Durgeau A, Meurice G, de Montpreville V, et al. CD8+CD103+ Tumor-Infiltrating Lymphocytes Are Tumor-Specific Tissue-Resident Memory T Cells and a Prognostic Factor for Survival in Lung Cancer Patients. J Immunol (2015) 194(7):3475–86. doi: 10.4049/jimmunol.1402711
11. Nizard M, Roussel H, Diniz MO, Karaki S, Tran T, Voron T, et al. Induction of Resident Memory T Cells Enhances the Efficacy of Cancer Vaccine. Nat Commun (2017) 8:15221. doi: 10.1038/ncomms15221
12. Clarke J, Panwar B, Madrigal A, Singh D, Gujar R, Wood O, et al. Single-Cell Transcriptomic Analysis of Tissue-Resident Memory T Cells in Human Lung Cancer. J Exp Med (2019) 216(9):2128–49. doi: 10.1084/jem.20190249
13. Gaudreau PO, Negrao MV, Mitchell KG, Reuben A, Corsini EM, Li J, et al. Neoadjuvant Chemotherapy Increases Cytotoxic T Cell, Tissue Resident Memory T Cell, and B Cell Infiltration in Resectable NSCLC. J Thorac Oncol (2021) 16(1):127–39. doi: 10.1016/j.jtho.2020.09.027
14. You Z, Li Y, Wang Q, Zhao Z, Li Y, Qian Q, et al. The Clinical Significance of Hepatic CD69(+) CD103(+) CD8(+) Resident Memory T Cells in Autoimmune Hepatitis. Hepatology (2021) 74(2):847–63. doi: 10.1002/hep.31739
15. Ichikawa T, Hirahara K, Kokubo K, Kiuchi M, Aoki A, Morimoto Y, et al. CD103(hi) Treg Cells Constrain Lung Fibrosis Induced by CD103(lo) Tissue-Resident Pathogenic CD4 T Cells. Nat Immunol (2019) 20(11):1469–80. doi: 10.1038/s41590-019-0494-y
16. Goplen NP, Wu Y, Son YM, Li C, Wang Z, Cheon IS, et al. Tissue-Resident CD8(+) T Cells Drive Age-Associated Chronic Lung Sequelae After Viral Pneumonia. Sci Immunol (2020) 5(53):eabc4557. doi: 10.1126/sciimmunol.abc4557
17. Hondowicz BD, An D, Schenkel JM, Kim KS, Steach HR, Krishnamurty AT, et al. Interleukin-2-Dependent Allergen-Specific Tissue-Resident Memory Cells Drive Asthma. Immunity (2016) 44(1):155–66. doi: 10.1016/j.immuni.2015.11.004
18. Turner DL, Goldklang M, Cvetkovski F, Paik D, Trischler J, Barahona J, et al. Biased Generation and In Situ Activation of Lung Tissue-Resident Memory CD4 T Cells in the Pathogenesis of Allergic Asthma. J Immunol (2018) 200(5):1561–9. doi: 10.4049/jimmunol.1700257
19. Wang X, Tian Z, Peng H. Tissue-Resident Memory-Like ILCs: Innate Counterparts of TRM Cells. Protein Cell (2020) 11(2):85–96. doi: 10.1007/s13238-019-0647-7
20. Takamura S. Divergence of Tissue-Memory T Cells: Distribution and Function-Based Classification. Cold Spring Harb Perspect Biol (2020) 12(10):a037762. doi: 10.1101/cshperspect.a037762
21. Szabo PA, Miron M, Farber DL. Location, Location, Location: Tissue Resident Memory T Cells in Mice and Humans. Sci Immunol (2019) 4(34):eaas9673. doi: 10.1126/sciimmunol.aas9673
22. Clark RA, Chong B, Mirchandani N, Brinster NK, Yamanaka K, Dowgiert RK, et al. The Vast Majority of CLA+ T Cells Are Resident in Normal Skin. J Immunol (2006) 176(7):4431–9. doi: 10.4049/jimmunol.176.7.4431
23. Miron M, Kumar BV, Meng W, Granot T, Carpenter DJ, Senda T, et al. Human Lymph Nodes Maintain TCF-1(Hi) Memory T Cells With High Functional Potential and Clonal Diversity Throughout Life. J Immunol (2018) 201(7):2132–40. doi: 10.4049/jimmunol.1800716
24. Im SJ, Hashimoto M, Gerner MY, Lee J, Kissick HT, Burger MC, et al. Defining CD8+ T Cells That Provide the Proliferative Burst After PD-1 Therapy. Nature (2016) 537(7620):417–21. doi: 10.1038/nature19330
25. Duhen T, Duhen R, Montler R, Moses J, Moudgil T, de Miranda NF, et al. Co-Expression of CD39 and CD103 Identifies Tumor-Reactive CD8 T Cells in Human Solid Tumors. Nat Commun (2018) 9(1):2724. doi: 10.1038/s41467-018-05072-0
26. Mackay LK, Rahimpour A, Ma JZ, Collins N, Stock AT, Hafon ML, et al. The Developmental Pathway for CD103(+)CD8+ Tissue-Resident Memory T Cells of Skin. Nat Immunol (2013) 14(12):1294–301. doi: 10.1038/ni.2744
27. Shinoda K, Tokoyoda K, Hanazawa A, Hayashizaki K, Zehentmeier S, Hosokawa H, et al. Type II Membrane Protein CD69 Regulates the Formation of Resting T-Helper Memory. Proc Natl Acad Sci USA (2012) 109(19):7409–14. doi: 10.1073/pnas.1118539109
28. Milner JJ, Goldrath AW. Transcriptional Programming of Tissue-Resident Memory CD8(+) T Cells. Curr Opin Immunol (2018) 51:162–9. doi: 10.1016/j.coi.2018.03.017
29. Steel KJA, Srenathan U, Ridley M, Durham LE, Wu SY, Ryan SE, et al. Polyfunctional, Proinflammatory, Tissue-Resident Memory Phenotype and Function of Synovial Interleukin-17a+CD8+ T Cells in Psoriatic Arthritis. Arthritis Rheumatol (2020) 72(3):435–47. doi: 10.1002/art.41156
30. Topham DJ, Reilly EC. Tissue-Resident Memory CD8(+) T Cells: From Phenotype to Function. Front Immunol (2018) 9:515. doi: 10.3389/fimmu.2018.00515
31. Oja AE, Piet B, Helbig C, Stark R, van der Zwan D, Blaauwgeers H, et al. Trigger-Happy Resident Memory CD4(+) T Cells Inhabit the Human Lungs. Mucosal Immunol (2018) 11(3):654–67. doi: 10.1038/mi.2017.94
32. Garrido-Martin EM, Mellows TWP, Clarke J, Ganesan AP, Wood O, Cazaly A, et al. M1(hot) Tumor-Associated Macrophages Boost Tissue-Resident Memory T Cells Infiltration and Survival in Human Lung Cancer. J Immunother Cancer (2020) 8(2):e000778. doi: 10.1136/jitc-2020-000778
33. Thompson EA, Darrah PA, Foulds KE, Hoffer E, Caffrey-Carr A, Norenstedt S, et al. Monocytes Acquire the Ability to Prime Tissue-Resident T Cells via IL-10-Mediated TGF-Beta Release. Cell Rep (2019) 28(5):1127–35.e4. doi: 10.1016/j.celrep.2019.06.087
34. Pizzolla A, Nguyen TH, Sant S, Jaffar J, Loudovaris T, Mannering SI, et al. Influenza-Specific Lung-Resident Memory T Cells Are Proliferative and Polyfunctional and Maintain Diverse TCR Profiles. J Clin Invest (2018) 128(2):721–33. doi: 10.1172/JCI96957
35. Hombrink P, Helbig C, Backer RA, Piet B, Oja AE, Stark R, et al. Programs for the Persistence, Vigilance and Control of Human CD8(+) Lung-Resident Memory T Cells. Nat Immunol (2016) 17(12):1467–78. doi: 10.1038/ni.3589
36. Snyder ME, Finlayson MO, Connors TJ, Dogra P, Senda T, Bush E, et al. Generation and Persistence of Human Tissue-Resident Memory T Cells in Lung Transplantation. Sci Immunol (2019) 4(33):eaav5581. doi: 10.1126/sciimmunol.aav5581
37. Yoshizawa A, Bi K, Keskin DB, Zhang G, Reinhold B, Reinherz EL. TCR-pMHC Encounter Differentially Regulates Transcriptomes of Tissue-Resident CD8 T Cells. Eur J Immunol (2018) 48(1):128–50. doi: 10.1002/eji.201747174
38. Pan Y, Tian T, Park CO, Lofftus SY, Mei S, Liu X, et al. Survival of Tissue-Resident Memory T Cells Requires Exogenous Lipid Uptake and Metabolism. Nature (2017) 543(7644):252–6. doi: 10.1038/nature21379
39. Hombrink P, Helbig C, Backer RA, Piet B, Oja AE, Stark R, et al. Erratum: Programs for the Persistence, Vigilance and Control of Human CD8(+) Lung-Resident Memory T Cells. Nat Immunol (2017) 18(2):246. doi: 10.1038/ni0217-246d
40. Li C, Zhu B, Son YM, Wang Z, Jiang L, Xiang M, et al. The Transcription Factor Bhlhe40 Programs Mitochondrial Regulation of Resident CD8(+) T Cell Fitness and Functionality. Immunity (2019) 51(3):491–507.e7. doi: 10.1016/j.immuni.2019.08.013
41. Ganesan AP, Clarke J, Wood O, Garrido-Martin EM, Chee SJ, Mellows T, et al. Tissue-Resident Memory Features Are Linked to the Magnitude of Cytotoxic T Cell Responses in Human Lung Cancer. Nat Immunol (2017) 18(8):940–50. doi: 10.1038/ni.3775
42. Katayama H. Can Immunological Manipulation Defeat SARS-CoV-2? Why G-CSF Induced Neutrophil Expansion Is Worth a Clinical Trial: G-CSF Treatment Against COVID-19. Bioessays (2021) 43(2):e2000232. doi: 10.1002/bies.202000232
43. Luangrath MA, Schmidt ME, Hartwig SM, Varga SM. Tissue-Resident Memory T Cells in the Lungs Protect Against Acute Respiratory Syncytial Virus Infection. Immunohorizons (2021) 5(2):59–69. doi: 10.4049/immunohorizons.2000067
44. Matyushenko V, Kotomina T, Kudryavtsev I, Mezhenskaya D, Prokopenko P, Matushkina A, et al. Conserved T-Cell Epitopes of Respiratory Syncytial Virus (RSV) Delivered by Recombinant Live Attenuated Influenza Vaccine Viruses Efficiently Induce RSV-Specific Lung-Localized Memory T Cells and Augment Influenza-Specific Resident Memory T-Cell Responses. Antiviral Res (2020) 182:104864. doi: 10.1016/j.antiviral.2020.104864
45. Guvenel A, Jozwik A, Ascough S, Ung SK, Paterson S, Kalyan M, et al. Epitope-Specific Airway-Resident CD4+ T Cell Dynamics During Experimental Human RSV Infection. J Clin Invest (2020) 130(1):523–38. doi: 10.1172/JCI131696
46. Wilk MM, Borkner L, Misiak A, Curham L, Allen AC, Mills KHG. Immunization With Whole Cell But Not Acellular Pertussis Vaccines Primes CD4 TRM Cells That Sustain Protective Immunity Against Nasal Colonization With Bordetella Pertussis. Emerg Microbes Infect (2019) 8(1):169–85. doi: 10.1080/22221751.2018.1564630
47. Dubois V, Chatagnon J, Thiriard A, Bauderlique-Le Roy H, Debrie AS, Coutte L, et al. Suppression of Mucosal Th17 Memory Responses by Acellular Pertussis Vaccines Enhances Nasal Bordetella Pertussis Carriage. NPJ Vaccines (2021) 6(1):6. doi: 10.1038/s41541-020-00270-8
48. Zurita ME, Wilk MM, Carriquiriborde F, Bartel E, Moreno G, Misiak A, et al. A Pertussis Outer Membrane Vesicle-Based Vaccine Induces Lung-Resident Memory CD4 T Cells and Protection Against Bordetella Pertussis, Including Pertactin Deficient Strains. Front Cell Infect Microbiol (2019) 9:125. doi: 10.3389/fcimb.2019.00125
49. Raeven RHM, Rockx-Brouwer D, Kanojia G, van der Maas L, Bindels THE, Ten Have R, et al. Intranasal Immunization With Outer Membrane Vesicle Pertussis Vaccine Confers Broad Protection Through Mucosal IgA and Th17 Responses. Sci Rep (2020) 10(1):7396. doi: 10.1038/s41598-020-63998-2
50. Paik DH, Farber DL. Influenza Infection Fortifies Local Lymph Nodes to Promote Lung-Resident Heterosubtypic Immunity. J Exp Med (2021) 218(1):e20200218. doi: 10.1084/jem.20200218
51. Goplen NP, Huang S, Zhu B, Cheon IS, Son YM, Wang Z, et al. Tissue-Resident Macrophages Limit Pulmonary CD8 Resident Memory T Cell Establishment. Front Immunol (2019) 10:2332. doi: 10.3389/fimmu.2019.02332
52. Wang H, Hoffman C, Yang X, Clapp B, Pascual DW. Targeting Resident Memory T Cell Immunity Culminates in Pulmonary and Systemic Protection Against Brucella Infection. PloS Pathog (2020) 16(1):e1008176. doi: 10.1371/journal.ppat.1008176
53. Rahimi RA, Nepal K, Cetinbas M, Sadreyev RI, Luster AD. Distinct Functions of Tissue-Resident and Circulating Memory Th2 Cells in Allergic Airway Disease. J Exp Med (2020) 217(9):e20190865. doi: 10.1084/jem.20190865
54. Bosnjak B, Kazemi S, Altenburger LM, Mokrovic G, Epstein MM. Th2-TRMs Maintain Life-Long Allergic Memory in Experimental Asthma in Mice. Front Immunol (2019) 10:840. doi: 10.3389/fimmu.2019.00840
55. Brown FD, Sen DR, LaFleur MW, Godec J, Lukacs-Kornek V, Schildberg FA, et al. Fibroblastic Reticular Cells Enhance T Cell Metabolism and Survival via Epigenetic Remodeling. Nat Immunol (2019) 20(12):1668–80. doi: 10.1038/s41590-019-0515-x
56. Schenkel JM, Fraser KA, Vezys V, Masopust D. Sensing and Alarm Function of Resident Memory CD8(+) T Cells. Nat Immunol (2013) 14(5):509–13. doi: 10.1038/ni.2568
57. Ariotti S, Hogenbirk MA, Dijkgraaf FE, Visser LL, Hoekstra ME, Song JY, et al. T Cell Memory. Skin-Resident Memory CD8(+) T Cells Trigger a State of Tissue-Wide Pathogen Alert. Science (2014) 346(6205):101–5. doi: 10.1126/science.1254803
58. Haynes L, Eaton SM, Burns EM, Rincon M, Swain SL. Inflammatory Cytokines Overcome Age-Related Defects in CD4 T Cell Responses In Vivo. J Immunol (2004) 172(9):5194–9. doi: 10.4049/jimmunol.172.9.5194
59. Kumar BV, Ma W, Miron M, Granot T, Guyer RS, Carpenter DJ, et al. Human Tissue-Resident Memory T Cells Are Defined by Core Transcriptional and Functional Signatures in Lymphoid and Mucosal Sites. Cell Rep (2017) 20(12):2921–34. doi: 10.1016/j.celrep.2017.08.078
60. Iijima N, Iwasaki A. T Cell Memory. A Local Macrophage Chemokine Network Sustains Protective Tissue-Resident Memory CD4 T Cells. Science (2014) 346(6205):93–8. doi: 10.1126/science.1257530
61. Teijaro JR, Turner D, Pham Q, Wherry EJ, Lefrancois L, Farber DL. Cutting Edge: Tissue-Retentive Lung Memory CD4 T Cells Mediate Optimal Protection to Respiratory Virus Infection. J Immunol (2011) 187(11):5510–4. doi: 10.4049/jimmunol.1102243
62. Glennie ND, Volk SW, Scott P. Skin-Resident CD4+ T Cells Protect Against Leishmania Major by Recruiting and Activating Inflammatory Monocytes. PloS Pathog (2017) 13(4):e1006349. doi: 10.1371/journal.ppat.1006349
63. Son YM, Cheon IS, Wu Y, Li C, Wang Z, Gao X, et al. Tissue-Resident CD4(+) T Helper Cells Assist the Development of Protective Respiratory B and CD8(+) T Cell Memory Responses. Sci Immunol (2021) 6(55):eabb6852. doi: 10.1126/sciimmunol.abb6852
64. McMaster SR, Gabbard JD, Koutsonanos DG, Compans RW, Tripp RA, Tompkins SM, et al. Memory T Cells Generated by Prior Exposure to Influenza Cross React With the Novel H7N9 Influenza Virus and Confer Protective Heterosubtypic Immunity. PloS One (2015) 10(2):e0115725. doi: 10.1371/journal.pone.0115725
65. Grau-Exposito J, Sanchez-Gaona N, Massana N, Suppi M, Astorga-Gamaza A, Perea D, et al. Peripheral and Lung Resident Memory T Cell Responses Against SARS-CoV-2. Nat Commun (2021) 12(1):3010. doi: 10.1038/s41467-021-23333-3
66. Getschman AE, Imai Y, Larsen O, Peterson FC, Wu X, Rosenkilde MM, et al. Protein Engineering of the Chemokine CCL20 Prevents Psoriasiform Dermatitis in an IL-23-Dependent Murine Model. Proc Natl Acad Sci USA (2017) 114(47):12460–5. doi: 10.1073/pnas.1704958114
67. Ainsua-Enrich E, Hatipoglu I, Kadel S, Turner S, Paul J, Singh S, et al. IRF4-Dependent Dendritic Cells Regulate CD8(+) T-Cell Differentiation and Memory Responses in Influenza Infection. Mucosal Immunol (2019) 12(4):1025–37. doi: 10.1038/s41385-019-0173-1
68. Ge C, Monk IR, Pizzolla A, Wang N, Bedford JG, Stinear TP, et al. Bystander Activation of Pulmonary Trm Cells Attenuates the Severity of Bacterial Pneumonia by Enhancing Neutrophil Recruitment. Cell Rep (2019) 29(13):4236–44 e3. doi: 10.1016/j.celrep.2019.11.103
69. Wu T, Hu Y, Lee YT, Bouchard KR, Benechet A, Khanna K, et al. Lung-Resident Memory CD8 T Cells (TRM) are Indispensable for Optimal Cross-Protection Against Pulmonary Virus Infection. J Leukoc Biol (2014) 95(2):215–24. doi: 10.1189/jlb.0313180
70. Shenoy AT, Wasserman GA, Arafa EI, Wooten AK, Smith NMS, Martin IMC, et al. Lung CD4(+) Resident Memory T Cells Remodel Epithelial Responses to Accelerate Neutrophil Recruitment During Pneumonia. Mucosal Immunol (2020) 13(2):334–43. doi: 10.1038/s41385-019-0229-2
71. Wilk MM, Misiak A, McManus RM, Allen AC, Lynch MA, Mills KHG. Lung CD4 Tissue-Resident Memory T Cells Mediate Adaptive Immunity Induced by Previous Infection of Mice With Bordetella Pertussis. J Immunol (2017) 199(1):233–43. doi: 10.4049/jimmunol.1602051
72. Zhao Y, Yang Q, Jin C, Feng Y, Xie S, Xie H, et al. Changes of CD103-Expressing Pulmonary CD4(+) and CD8(+) T Cells in S. Japonicum Infected C57BL/6 Mice. BMC Infect Dis (2019) 19(1):999. doi: 10.1186/s12879-019-4633-8
73. Herbst RS, Morgensztern D, Boshoff C. The Biology and Management of Non-Small Cell Lung Cancer. Nature (2018) 553(7689):446–54. doi: 10.1038/nature25183
74. Corgnac S, Malenica I, Mezquita L, Auclin E, Voilin E, Kacher J, et al. CD103(+)CD8(+) TRM Cells Accumulate in Tumors of Anti-PD-1-Responder Lung Cancer Patients and Are Tumor-Reactive Lymphocytes Enriched With Tc17. Cell Rep Med (2020) 1(7):100127. doi: 10.1016/j.xcrm.2020.100127
75. O’Brien SM, Klampatsa A, Thompson JC, Martinez MC, Hwang WT, Rao AS, et al. Function of Human Tumor-Infiltrating Lymphocytes in Early-Stage Non-Small Cell Lung Cancer. Cancer Immunol Res (2019) 7(6):896–909. doi: 10.1158/2326-6066.CIR-18-0713
76. Heim L, Friedrich J, Engelhardt M, Trufa DI, Geppert CI, Rieker RJ, et al. NFATc1 Promotes Antitumoral Effector Functions and Memory CD8(+) T-Cell Differentiation During Non-Small Cell Lung Cancer Development. Cancer Res (2018) 78(13):3619–33. doi: 10.1158/0008-5472.CAN-17-3297
77. Corgnac S, Boutet M, Kfoury M, Naltet C, Mami-Chouaib F. The Emerging Role of CD8(+) Tissue Resident Memory T (TRM) Cells in Antitumor Immunity: A Unique Functional Contribution of the CD103 Integrin. Front Immunol (2018) 9:1904. doi: 10.3389/fimmu.2018.01904
78. Milner JJ, Toma C, Yu B, Zhang K, Omilusik K, Phan AT, et al. Runx3 Programs CD8(+) T Cell Residency in non-Lymphoid Tissues and Tumours. Nature (2017) 552(7684):253–7. doi: 10.1038/nature24993
79. Gauthier L, Corgnac S, Boutet M, Gros G, Validire P, Bismuth G, et al. Paxillin Binding to the Cytoplasmic Domain of CD103 Promotes Cell Adhesion and Effector Functions for CD8(+) Resident Memory T Cells in Tumors. Cancer Res (2017) 77(24):7072–82. doi: 10.1158/0008-5472.CAN-17-1487
80. Karaki S, Blanc C, Tran T, Galy-Fauroux I, Mougel A, Dransart E, et al. CXCR6 Deficiency Impairs Cancer Vaccine Efficacy and CD8(+) Resident Memory T-Cell Recruitment in Head and Neck and Lung Tumors. J Immunother Cancer (2021) 9(3):e001948. doi: 10.1136/jitc-2020-001948
81. Wein AN, McMaster SR, Takamura S, Dunbar PR, Cartwright EK, Hayward SL, et al. CXCR6 Regulates Localization of Tissue-Resident Memory CD8 T Cells to the Airways. J Exp Med (2019) 216(12):2748–62. doi: 10.1084/jem.20181308
82. Dhodapkar MV, Dhodapkar KM. Tissue-Resident Memory-Like T Cells in Tumor Immunity: Clinical Implications. Semin Immunol (2020) 49:101415. doi: 10.1016/j.smim.2020.101415
83. Lalos A, Tulek A, Tosti N, Mechera R, Wilhelm A, Soysal S, et al. Prognostic Significance of CD8+ T-Cells Density in Stage III Colorectal Cancer Depends on SDF-1 Expression. Sci Rep (2021) 11(1):775. doi: 10.1038/s41598-020-80382-2
84. Amsen D, Hombrink P, van Lier RAW. Tumor Immunity Requires Border Patrol to Fight the Enemy Within. Nat Immunol (2017) 18(8):870–2. doi: 10.1038/ni.3792
85. Koh J, Kim S, Kim MY, Go H, Jeon YK, Chung DH. Prognostic Implications of Intratumoral CD103+ Tumor-Infiltrating Lymphocytes in Pulmonary Squamous Cell Carcinoma. Oncotarget (2017) 8(8):13762–9. doi: 10.18632/oncotarget.14632
86. Zhong C, Lang Q, Yu J, Wu S, Xu F, Tian Y. Phenotypical and Potential Functional Characteristics of Different Immune Cells Expressing CD28H/B7-H5 and Their Relationship With Cancer Prognosis. Clin Exp Immunol (2020) 200(1):12–21. doi: 10.1111/cei.13413
87. Chen Y, Xin Z, Huang L, Zhao L, Wang S, Cheng J, et al. CD8(+) T Cells Form the Predominant Subset of NKG2A(+) Cells in Human Lung Cancer. Front Immunol (2019) 10:3002. doi: 10.3389/fimmu.2019.03002
88. Oja AE, Piet B, van der Zwan D, Blaauwgeers H, Mensink M, de Kivit S, et al. Functional Heterogeneity of CD4(+) Tumor-Infiltrating Lymphocytes With a Resident Memory Phenotype in NSCLC. Front Immunol (2018) 9:2654. doi: 10.3389/fimmu.2018.02654
89. Wang Z, Wang S, Goplen NP, Li C, Cheon IS, Dai Q, et al. PD-1(Hi) CD8(+) Resident Memory T Cells Balance Immunity and Fibrotic Sequelae. Sci Immunol (2019) 4(36):eaaw1217. doi: 10.1126/sciimmunol.aaw1217
90. Qu QX, Zhu XY, Du WW, Wang HB, Shen Y, Zhu YB, et al. 4-1bb Agonism Combined With PD-L1 Blockade Increases the Number of Tissue-Resident CD8+ T Cells and Facilitates Tumor Abrogation. Front Immunol (2020) 11:577. doi: 10.3389/fimmu.2020.00577
91. Zhang C, Yin K, Liu SY, Yan LX, Su J, Wu YL, et al. Multiomics Analysis Reveals a Distinct Response Mechanism in Multiple Primary Lung Adenocarcinoma After Neoadjuvant Immunotherapy. J Immunother Cancer (2021) 9(4):e002312. doi: 10.1136/jitc-2020-002312
92. Arina A, Beckett M, Fernandez C, Zheng W, Pitroda S, Chmura SJ, et al. Tumor-Reprogrammed Resident T Cells Resist Radiation to Control Tumors. Nat Commun (2019) 10(1):3959. doi: 10.1038/s41467-019-11906-2
93. Muroyama Y, Nirschl TR, Kochel CM, Lopez-Bujanda Z, Theodros D, Mao W, et al. Stereotactic Radiotherapy Increases Functionally Suppressive Regulatory T Cells in the Tumor Microenvironment. Cancer Immunol Res (2017) 5(11):992–1004. doi: 10.1158/2326-6066.CIR-17-0040
94. Zhuang Y, Li S, Wang H, Pi J, Xing Y, Li G. PD-1 Blockade Enhances Radio-Immunotherapy Efficacy in Murine Tumor Models. J Cancer Res Clin Oncol (2018) 144(10):1909–20. doi: 10.1007/s00432-018-2723-4
95. Caetano MS, Younes AI, Barsoumian HB, Quigley M, Menon H, Gao C, et al. Triple Therapy With MerTK and PD1 Inhibition Plus Radiotherapy Promotes Abscopal Antitumor Immune Responses. Clin Cancer Res (2019) 25(24):7576–84. doi: 10.1158/1078-0432.CCR-19-0795
96. Williams RJ, Trichostatin A. An Inhibitor of Histone Deacetylase, Inhibits Hypoxia-Induced Angiogenesis. Expert Opin Investig Drugs (2001) 10(8):1571–3. doi: 10.1517/13543784.10.8.1571
97. Li C, Zhu B, Son YM, Wang Z, Jiang L, Xiang M, et al. The Transcription Factor Bhlhe40 Programs Mitochondrial Regulation of Resident CD8(+) T Cell Fitness and Functionality. Immunity (2020) 52(1):201–2. doi: 10.1016/j.immuni.2019.12.008
98. Zheng X, Oduro JD, Boehme JD, Borkner L, Ebensen T, Heise U, et al. Mucosal CD8+ T Cell Responses Induced by an MCMV Based Vaccine Vector Confer Protection Against Influenza Challenge. PloS Pathog (2019) 15(9):e1008036. doi: 10.1371/journal.ppat.1008036
99. Haddadi S, Vaseghi-Shanjani M, Yao Y, Afkhami S, D’Agostino MR, Zganiacz A, et al. Mucosal-Pull Induction of Lung-Resident Memory CD8 T Cells in Parenteral TB Vaccine-Primed Hosts Requires Cognate Antigens and CD4 T Cells. Front Immunol (2019) 10:2075. doi: 10.3389/fimmu.2019.02075
100. Caminschi I, Lahoud MH, Pizzolla A, Wakim LM. Zymosan by-Passes the Requirement for Pulmonary Antigen Encounter in Lung Tissue-Resident Memory CD8(+) T Cell Development. Mucosal Immunol (2019) 12(2):403–12. doi: 10.1038/s41385-018-0124-2
101. Knight FC, Gilchuk P, Kumar A, Becker KW, Sevimli S, Jacobson ME, et al. Mucosal Immunization With a pH-Responsive Nanoparticle Vaccine Induces Protective CD8(+) Lung-Resident Memory T Cells. ACS Nano (2019) 13(10):10939–60. doi: 10.1021/acsnano.9b00326
102. Wilk MM, Mills KHG. CD4 TRM Cells Following Infection and Immunization: Implications for More Effective Vaccine Design. Front Immunol (2018) 9:1860. doi: 10.3389/fimmu.2018.01860
103. Ferguson KT, McQuattie-Pimentel AC, Malsin ES, Sporn PHS. Dynamics of Influenza-Induced Lung-Resident Memory T Cells, Anatomically and Functionally Distinct Lung Mesenchymal Populations, and Dampening of Acute Lung Injury by Neutrophil Transfer of Micro-RNA-223 to Lung Epithelial Cells. Am J Respir Cell Mol Biol (2018) 59(3):397–9. doi: 10.1165/rcmb.2018-0047RO
104. Hamelmann E, Gelfand EW. IL-5-Induced Airway Eosinophilia–the Key to Asthma? Immunol Rev (2001) 179:182–91. doi: 10.1034/j.1600-065X.2001.790118.x
Keywords: tissue-resident memory T cells, non-small-cell lung cancer, lung infection, immunotherapy, vaccine
Citation: Yuan R, Yu J, Jiao Z, Li J, Wu F, Yan R, Huang X and Chen C (2021) The Roles of Tissue-Resident Memory T Cells in Lung Diseases. Front. Immunol. 12:710375. doi: 10.3389/fimmu.2021.710375
Received: 16 May 2021; Accepted: 27 September 2021;
Published: 11 October 2021.
Edited by:
Vandana Kalia, University of Washington, United StatesReviewed by:
Aki Hoji, Indiana University, Purdue University Indianapolis, United StatesHaina Shin, Washington University in St. Louis, United States
Copyright © 2021 Yuan, Yu, Jiao, Li, Wu, Yan, Huang and Chen. This is an open-access article distributed under the terms of the Creative Commons Attribution License (CC BY). The use, distribution or reproduction in other forums is permitted, provided the original author(s) and the copyright owner(s) are credited and that the original publication in this journal is cited, in accordance with accepted academic practice. No use, distribution or reproduction is permitted which does not comply with these terms.
*Correspondence: Chen Chen, Y2hlbmNoZW4xOTgxNDEyQGNzdS5lZHUuY24=
†These authors have contributed equally to this work and share first authorship