- 1CAS Key Laboratory of Experimental Marine Biology, Institute of Oceanology, Center for Ocean Mega-Science, Chinese Academy of Sciences, Beijing, China
- 2Laboratory for Marine Biology and Biotechnology, Pilot National Laboratory for Marine Science and Technology (Qingdao), Qingdao, China
- 3College of Marine Science, University of Chinese Academy of Sciences, Beijing, China
MicroRNAs (miRNAs) are small non-coding RNAs that regulate diverse biological processes including immunity. In a previous high-throughput RNA sequencing study, a novel miRNA, pol-miR-novel_642, was identified from Japanese flounder (Paralichthys olivaceus), a farmed fish species with important economic value. In this study, we investigated the regulatory mechanism and the function of pol-miR-novel_642 and its target gene. We found that pol-miR-novel_642 targeted, in a sequence-specific manner, a flounder gene encoding an uncharacterized protein that is a structural homologue of murine granulocyte colony stimulating factor 3 (CSF3). The expression of pol-miR-novel_642 and its target gene (named PoCSF3-1) was regulated, in different manners, by the bacterial pathogen Edwardsiella tarda and the viral pathogen megalocytivirus. Overexpression of pol-miR-novel_642 or interference with PoCSF3-1 expression in flounder cells strongly potentiated E. tarda infection. Consistently, in vivo knockdown of PoCSF3-1 enhanced bacterial dissemination in flounder tissues but blocked viral replication, whereas in vivo overexpression of PoCSF3-1 inhibited bacterial dissemination and facilitated viral infection. Overexpression/knockdown of PoCSF3-1 and pol-miR-novel_642 also affected the activation of autophagy. Recombinant PoCSF3-1 (rPoCSF3-1) interacted with and inhibited the growth of Gram-negative bacteria in a manner relying on a PoCSF3-1-characteristic structural motif that is absent in mouse CSF3. rPoCSF3-1 also regulated the proliferation, inflammatory response, and immune defense of flounder head kidney leukocytes in a structure-dependent fashion. Together, these results reveal the function of a novel miRNA-CSF3 regulatory system of flounder, and add new insights into the role and mechanism of fish miRNA and CSF3 in antimicrobial immunity.
Introduction
MicroRNAs (miRNAs) are a type of small noncoding RNAs that negatively regulate the expression of protein-coding genes (1–3). MiRNAs are present widely in animals, plants, and viruses. In animals, miRNAs most often inhibit their target genes by blocking mRNA translation or decreasing mRNA stability via interaction with the 3’ untranslated regions (3’UTRs) of the target genes (4, 5). However, recent studies showed that some miRNAs interact with the coding regions of the target genes to modulate gene expression (1, 6–8). MiRNAs regulate diverse biological processes and signal pathways involved in infection and immunity (9–13). As a result, miRNAs play an important role in pathogen-induced immune response and are known to influence the outcome of host-pathogen interaction in mammals and teleost (14–18). In fish, miRNAs associated with bacterial and viral infection have been identified in various species, and some of the miRNAs were found to function by targeting specific immune genes such as IL-1 receptor-associated kinase 4 and signal transducer and activator of transcription (STAT) 3 (19–23).
Colony stimulating factor (CSF) superfamily proteins are hematopoietic growth factors that regulate proliferation, differentiation, and survival of lineage-specific myeloid cells (24). In mammals, the family had three canonical members, namely macrophage-colony stimulating factor (M-CSF or CSF1), granulocyte/macrophage-colony stimulating factor (GM-CSF or CSF2), and granulocyte-colony stimulating factor (G-CSF or CSF3) (25). CSF3 is a low molecular weight glycoprotein produced by monocytes, fibroblasts, and endothelial cells (26). It stimulates granulocyte production and regulates the migration and antimicrobial activity of neutrophils (27, 28). CSF3 also plays a role in regulating host immune response to pathogens such as Listeria monocytogenes and Candida albicans (28, 29). In teleost, CSF3-like genes have been identified in a number of species, including Japanese flounder (Paralichthys olivaceus), fugu (Takifugu rubipes), green-spotted pufferfish (Tetraodon nigroviridis), black rockfish (Sebastes schlegelii), zebrafish (Danio rerio), rock bream (Oplegnathus fasciatus), and large yellow croaker (Larimichthys crocea) (30–34). Two paralogs of CSF3 have been reported to exist in some fish, including common carp, rock bream, and zebrafish, likely as a result of genome duplication (33, 35–37). Several reports showed that some fish CSF3 members are involved in pathogen infection and able to promote leukocyte proliferation, phagocytosis, or migration (33, 34, 36–39),
Edwardsiella tarda is a Gram-negative bacterium and a severe pathogen to many farmed fish including Japanese flounder, a type of flat fish farmed worldwide. Flounder miRNAs involving in E. tarda infection have been reported previously (10, 11, 17, 19, 40–44). In a previous study, we identified 96 flounder miRNAs that exhibited differential expression during E. tarda infection (11). One of these miRNAs is Pol-miR-novel_642, a novel miRNA with unknown function. In the present study, we examined the function of pol-miR-novel_642 and its target gene, an uncharacterized paralog of CSF3 (named PoCSF3-1). Our results showed that pol-miR-novel_642 regulates host immune response and pathogen infection in a manner that involves PoCSF3-1, and that PoCSF3-1 possesses the properties of both a cytokine and a bacterial binding protein in a structure-dependent manner.
Materials and Methods
Animals
Healthy Japanese flounder (average weight 20.2 g) were purchased from a commercial fish farm in Shandong Province, China, and temporarily kept at 22°C in aerated seawater. Before tissue collection, the fish were euthanized with tricaine methanesulfonate solution (Sigma, USA) as reported previously (45).
Cell Lines
FG-9307 cells (46), a Japanese flounder gill cell line, were cultured at 24°C in L-15 medium (Thermo Scientific HyClone, USA) supplemented with 10% FBS, 100 units/mL penicillin, and 100 μg/mL streptomycin as reported previously (19). HEK293T cells were cultured at 37°C in DMEM medium (Invitrogen, Grand Island, USA) supplemented with 10% FBS, 100 units/mL penicillin, and 100 μg/mL streptomycin under humidified condition with 5% CO2 as previously reported (19). FG-9307 cells were used in this study because it is derived from flounder. HEK293T cells were used in luciferase reporter assay, because efficient transfection can be achieved with this cell line.
MiRNA Mimic, Inhibitor, and Small Interfering RNA (siRNA)
The mimics of pol-miR-novel_642, pol-miR-novel_642M (with the seed sequence of 5’-AGGCUGC-3’ mutated to 5’-UCCGACG-3’) and their negative control (miR-C) were synthesized by GenePharma (Shanghai, China). Pol-miR-novel_642 inhibitor and its negative control (miR inhibitor-C) were designed and synthesized by the same company. The PoCSF3-1 specific siRNA (siPoCSF3-1) and its negative control (siC) were designed and synthesized by Ribobio (Guangzhou, China).
Quantitative Real-Time Reverse Transcription-PCR (qRT-PCR)
To examine pol-miR-novel_642 expression in flounder during pathogen infection, E. tarda was cultured in LB medium at 28°C to mid-logarithmic phase and resuspended in PBS to 108 CFU/mL as reported previously (47). The fish viral pathogen megalocytivirus RBIV-C1 (48) was resuspended in PBS to 5×105 copies/mL. Flounder (as described above) were randomly divided into three groups and injected intraperitoneally with 100 μL suspension of E. tarda, megalocytivirus, or PBS. Kidney was taken aseptically from the fish at 12 h, 24 h, and 48 h post-bacterial infection and at 2 d, 4 d, 6 d, 8 d, and 10 d post-viral infection. To examine pol-miR-novel_642 expression, miRNA was isolated with miRNAiso plus kit (TaKaRa, China). The miRNA-specific reverse transcription was performed using miRNA first-strand cDNA synthesis kit (Vazyme, China) with specific stem-loop reverse transcription (RT) primer (5’-GTCGTATCCAGTGCAGGGTCCGAGGTATTCGCACTGGA TACGACGCTCAG-3’). MiRNA level was validated by qRT-PCR with SYBR ExScript qRT-PCR Kit (TaKaRa, Japan) and a QuantStudio 3 Real-time PCR system (Applied Biosystems, USA) as reported previously (19). 5s rRNA was used as an internal control. The primers used in the PCR are listed in Table S1.
To examine PoCSF3-1 expression in flounder during pathogen infection, the fish were injected intraperitoneally with E. tarda or megalocytivirus as mentioned above. Total RNA was isolated from kidney with TRIzol reagent (Invitrogen, USA). cDNA synthesis was performed with First Strand cDNA Synthesis Kit (ToYoBo, Japan) according to the manufacturer’s protocol. PoCSF3-1 expression was determined by qRT-PCR with primers PoCSF3-1-F and PoCSF3-1-R (Table S1). 18s rRNA and elongation factor-1-α (EF1A) were used as internal references for bacterial and viral infections, respectively, according previous reports (49, 50).
Plasmid Construction
To construct the plasmid pPoCSF3-1-Report, the coding sequence of PoCSF3-1 was amplified by PCR with primers PoCSF3-1-CDS-F1 and PoCSF3-1-CDS-R1 (Table S1). The PCR product was inserted into pmirGLO (Promega, USA) at between the Nhe I and Sal I sites as reported previously (41). To construct the plasmid pPoCSF3-1si, which expresses a PoCSF3-1 specific siRNA, a siRNA targeting PoCSF3-1 was chemically synthesized (TsingKe, China) and inserted into pRNAT-CMV3.1 (GenScript, USA) at between the BamH I and Alf II sites as reported previously (41). pPoCSF3-1siC, which expresses a nonspecific siRNA, was constructed similarly. To construct the plasmid pPoCSF3-1, which expresses PoCSF3-1, the coding sequence of PoCSF3-1 was amplified by PCR with the primers PoCSF3-1-CDS-F2 and PoCSF3-1-CDS-R2 (Table S1), and the PCR product was inserted into the EcoR V site of pCN3 as reported previously (51). To construct the plasmid pETPoCSF3-1, which expresses His-tagged PoCSF3-1, the coding sequence of PoCSF3-1 was amplified by PCR with the primers PoCSF3-1-CDS-F3 and PoCSF3-1-CDS-R3 (Table S1), and the PCR product was inserted into pET28a (Novagen, USA) at between the EcoR I and Hind III sites. To construct the plasmid pETPoCSF3-1M, which expresses the mutant PoCSF3-1 (PoCSF3-1M) with 36ILL39 to 36SLE39 substitution, the coding sequence of the mutant was amplified by PCR with the primers PoCSF3-1-Mut-F and PoCSF3-1-Mut-R (Table S1) using Fast Mutagenesis System (TransGen Biotech, China), and the product was inserted into pET28a as above. All plasmids were verified by DNA sequencing.
Regulation of PoCSF3-1 by pol-miR-novel-642
Luciferase reporter assay was performed as reported previously (41). Briefly, HEK293T cells were distributed in 24-well plates (about 80% confluence) and transfected with pPoCSF3-1-Report (control), pPoCSF3-1-Report plus pol-miR-novel_642 mimic, pPoCSF3-1-Report plus miR-C, or pPoCSF3-1-Report plus pol-miR-novel_642M. The transfection was carried out with Lipofectamine 3000™ reagent (Invitrogen, USA) at a 1:1 ratio of plasmid: transfection reagent for 24 h. The cells were collected, and luciferase activity was assayed using the Dual Luciferase Reporter Assay Kit (Vazyme, Nanjing, China) according to the manufacturer’s protocol. To examine the regulation of PoCSF3-1 by pol-miR-novel-642 in flounder cells, FG-9307 cells were distributed in 6-well plates (about 80% confluence) and transfected with or without pol-miR-novel_642 mimic or miR-C for 24 h. qRT-PCR was performed to determine PoCSF3-1 mRNA expression with primers as described above. Western blot was performed to determine PoCSF3-1 protein expression as reported previously (41). Briefly, the transfected cells were lysed with RIPA lysis buffer (Beyotime Biotechnology, China) on ice for 30 min. The cell lysates were mixed with 5× sodiumdodecylsulfate-polyacrylamide gelelectrophoresis (SDS-PAGE) loading buffer, boiled for 10 min, and separated by SDS-PAGE. After electrophoresis, the proteins were transferred to a nitrocellulose blotting membrane (GE healthcare, Germany). The membrane was blocked with 5% skim milk, followed by incubation with anti-PoCSF3-1 antibody (Sangon Biotech, China) or anti-β-actin antibody (ABclonal, China) at 4°C for overnight. The membrane was incubated with HRP-conjuncted anti-rabbit antibody (Abcam, UK) for 1 h at room temperature. The membrane was then incubated with ECL substrate (Beyotime Biotechnology, China) and visualized with GelDoc XR System (Bio-Rad, USA).
Sequence Analysis
The amino acid sequence of PoCSF3-1 (accession no. XP_019938527) was analyzed with the BLAST program at the National Center for Biotechnology Information (NCBI). The amino acid sequence of Mus musculus CSF3 (accession no. EDL16160.1) is available from NCBI. Multiple sequence alignment was created with DNAMAN. The potential three-dimensional structure was created using the SWISS-MODEL prediction algorithm.
Overexpression and Knockdown of pol-miR-novel_642/PoCSF3-1
For pol-miR-novel_642 overexpression in FG-9307 cells, the cells were transfected with pol-miR-novel_642 mimic or miR-C as above. For PoCSF3-1 knockdown in FG-9307 cells, the cells were transfected with siPoCSF3-1 or siC as above. To examine the effect of pol-miR-novel_642 overexpression or PoCSF3-1 knockdown on E. tarda infection, the above transfected FG-9307 cells were infected with E. tarda at a MOI of 20. At 2 h, 4 h, and 8 h post infection, intracellular bacterial number was determined by plate count as reported previously (19). For PoCSF3-1 knockdown and overexpression in flounder, flounder were injected intramuscularly with pPoCSF3-1si, pPoCSF3-1siC, pPoCSF3-1 or pCN3 at the dose of 1 μg plasmid/1 g fish. The control group was similarly injected with the same volume of PBS. Kidney, spleen and liver were harvested from the fish at 7 d post-injection. PoCSF3-1 knockdown and overexpression were verified by qRT-PCR as described above (Figure S1). To examine the effect of PoCSF3-1 knockdown and overexpression on pathogen infection, flounder were administered with pPoCSF3-1si, pPoCSF3-1siC, pPoCSF3-1, pCN3 or PBS for 7 days. The fish were infected with E. tarda or megalocytivirus as above. At 12 h, 24 h, and 48 h post E. tarda infection, the numbers of E. tarda in kidney, spleen and liver were determined by plate count as previously reported (41). At 6 d and 8 d post-megalocytivirus infection, the viral loads in kidney were determined by absolute quantitative real time RT-PCR as reported previously (16).
Effects of PoCSF3-1 and pol-miR-novel_642 on Autophagy
To examine the effect of PoCSF3-1 knockdown and overexpression on autophagy gene expression in flounder, flounder were administered with pPoCSF3-1si, pPoCSF3-1siC, pPoCSF3-1, pCN3 or PBS. At 7 d post-plasmid administration, the expression of ATG5, AKT, mTOR, and beclin-1 in kidney, spleen and liver was examined by qRT-PCR. To examine the effect of pol-miR-novel_642 on autophagy in flounder cells, FG-9307 cells were transfected with pol-miR-novel_642 inhibitor or miR inhibitor-C for 24 h. Beclin-1 and LC3 proteins were detected by Western blot as described above.
Purification and Recombinant Proteins
Recombinant proteins were purified as reported previously (19). Briefly, Escherichia coli BL21 (DE3) (TransGen Biotech, China) was transformed with pETPoCSF3-1, pETPoCSF3-1M, or pET32a (which expresses His-tagged Trx). The transformants were cultured in LB medium at 37°C to OD600 of 0.5. Isopropyl-β-D-thiogalactopyranoside was added to the medium to a final concentration of 0.04 mM. The culture was continued at 16°C for 16 h, and the cells were harvested by centrifugation. rPoCSF3-1, rPoCSF3-1M, and rTrx were purified using Ni-NTA agarose (QIAGEN, USA).
Binding of rPoCSF3-1 to Bacteria and Its Effect on Bacterial Growth
The bacteria used in this study, i.e., E. tarda, Vibrio anguillarum, Vibrio harveyi, Pseudomonas fluorescens, E. coli, Bacillus subtilis, Micrococcus luteus, Streptococcus iniae, and Staphylococcus aureus, have been reported previously (52). Protein binding to bacteria was determined by enzyme-linked immunosorbent assay (ELISA) as reported previously (52). Briefly, the bacteria were resuspended in coating buffer (15 mM Na2CO3, 35 mM NaHCO3, pH 9.6) to 108 CFU/mL. Each of the bacterial suspension was added to 96-well plates (100 μL/well). The plates were incubated at 4°C for overnight and then blocked with 5% skim milk powder at 4°C for 12 h. The plates were washed extensively with PBS containing 0.1% Tween-20 (PBST). Different concentrations of rPoCSF3-1 and rTrx were added to the plates (100 μL/well). The plates were incubated at 22°C for 2 h, followed by extensive washing with PBST. Horseradish peroxidase (HRP)-conjugated mouse anti-His antibody (ABclonal, China) was added to the plates. The plates were incubated at 37°C for 1 h, followed by extensive washing with PBST. Color development was performed using the TMB Kit (Tiangen, China). The plates were read at 450 nm, and binding index was defined as the fold increase of the reading of rPoCSF3-1-treated bacteria compared to that of the PBS-treated bacteria. To determine the effect of rPoCSF3-1 on bacterial growth, E. tarda and V. harveyi were suspended in LB medium to 105 CFU/mL in 96-well plates (100 μL/well). rPoCSF3-1 or rTrx was added to each well to the final concentration of 5 μM. The same volume of PBS was added to the control sample. The plates were incubated at 28°C, and bacterial growth was determined by measuring OD600 at every hour. rPoCSF3-1 binding to lipid A was performed as above, except that the bacteria were replaced by E. coli lipid A (Sigma, USA). Binding of rPoCSF3-1M to lipid A and bacteria were performed as above.
Effects of rPoCSF3-1 and rPoCSF3-1M on Head Kidney Leukocytes (HKLs)
To examine the effect of rPoCSF3-1 and rPoCSF3-1M on HKLs proliferation, flounder HKLs were isolated using density Percoll gradient centrifugation (53). The cells were distributed in a 96-well plate (1×106 cells/well) and incubated with 5 μM rPoCSF3-1, rPoCSF3-1M, or rTrx at 22°C for 12 h, 24 h, and 48 h. The control cells were incubated with PBS. Cell proliferation was then determined using the cell counting kit-8 (Vazyme Biotech, Nanjing, China) according to the manufacturer’s instruction. To examine the effect of rPoCSF3-1 on gene expression, HKLs (1×106 cells/mL) were incubated with rPoCSF3-1 (5 μM), rPoCSF3-1M (5 μM), rTrx (5 μM), or PBS at 22°C for 12 h, 24 h, and 48 h. qRT-PCR was performed to examine the expression of TNF-α, IL-1β, IL-6, IL-8, janus kinase (JAK)2, signal transducer and activator of transcription (STAT)1, STAT3, STAT4, and STAT6. The primers used in the PCR are listed in Table S1. To examine the effect of rPoCSF3-1 and rPoCSF3-1M on bacterial infection, HKLs (1×106 cells/mL) were incubated with rPoCSF3-1 (5 μM), rPoCSF3-1M (5 μM), rTrx (5 μM), or PBS at 22°C for 2 h, followed by infection with E. tarda (1×106 CFU/mL). The cells were then incubated at 22°C for 2 h. Extracellular bacteria were killed by adding gentamicin (200 μg/mL) to the culture. The cells were then incubated at 22°C for 2 h. After incubation, the cells were washed two times with PBS and cultured in L-15 medium containing 30 μg/mL gentamicin for 2 h or 4 h. The cells were collected by centrifugation at 600 g for 10 min and washed two times with PBS. The cells were lysed with 1% Triton X-100, and the lysate was diluted and plated on LB agar plates. The plates were incubated at 28°C for 48 h, and the colonies emerged on the plates were counted.
Effects of rPoCSF3-1 and rPoCSF3-1M on Bacterial Survival in Host Serum
E. tarda (104 CFU) were incubated with rPoCSF3-1 (5 μM), rPoCSF3-1M (5 μM), rTrx (5 μM), or PBS at 22°C for 2 h. After incubation, the bacteria were mixed with flounder serum, heat-inactivated flounder serum, or PBS at 22°C for 1 h. The mixture was then diluted, and the dilutions were plated on LB agar plates. The plates were incubated at 28°C for 48 h, and the colonies on the plates were counted. The survival rate was calculated as follows: (number of bacteria surviving serum treatment/number of bacteria surviving PBS treatment) × 100%.
Statistical Analysis
All experiments were performed three times, and statistical analyses were carried out with GraphPad Prism 7 (GraphPad Software, USA) and SPSS 17.0 software (SPSS Inc., Chicago, USA). Data were analyzed with two-tailed student’s t-test and one-way analysis of variance (ANOVA), and a p value of less than 0.05 was considered statistically significant.
Results
PoCSF3-1 Is a Target Gene of pol-miR-novel_642
Pol-miR-novel_642 was identified in a previous RNA sequencing study (11) as a novel miRNA with unknown function. Based on its seed sequence, pol-miR-novel_642 was predicted to target a 7-base region in the CDS of a gene encoding a hypothetical protein annotated as “uncharacterized protein LOC109626803 isoform X2” in the flounder genome (accession no. XP_019938527). XP_019938527 contains the conserved domain of IL6/G-CSF family and shares 62.87%-85.79% identities with the putative CSF3 of a number of fish, but only 35.35% identity with the known G-CSF of flounder (30, 38) (Figure S2). Structure modeling showed that XP_019938527 resembles highly mouse CSF3 in the overall structure (see Recombinant PoCSF3-1 (rPoCSF3-1) Interacts With Bacteria in a Structure-Dependent Manner and Affects Bacterial Survival in Host Serum). Based on these sequence features, this uncharacterized protein was named Paralichthys olivaceus CSF3-1 (PoCSF3-1). Luciferase reporter assay showed that pol-miR-novel_642 mimic significantly reduced the expression of the luciferase gene fused to PoCSF3-1 (Figure 1A). In contrast, a pol-miR-novel_642 variant, pol-miR-novel_642M, which bears mutation at the seed sequence involved in interaction with PoCSF3-1, did not affect the luciferase activity, suggesting that pol-miR-novel_642 modulated PoCSF3-1 expression in a manner that depended on the specific interaction between pol-miR-novel_642 and PoCSF3-1. Concordantly, when flounder FG-9307 cells were transfected with pol-miR-novel_642 mimic, PoCSF3-1 expression was significantly suppressed at both mRNA and protein levels (Figures 1B, C). These results indicate that PoCSF3-1 is a target gene of pol-miR-novel_642.
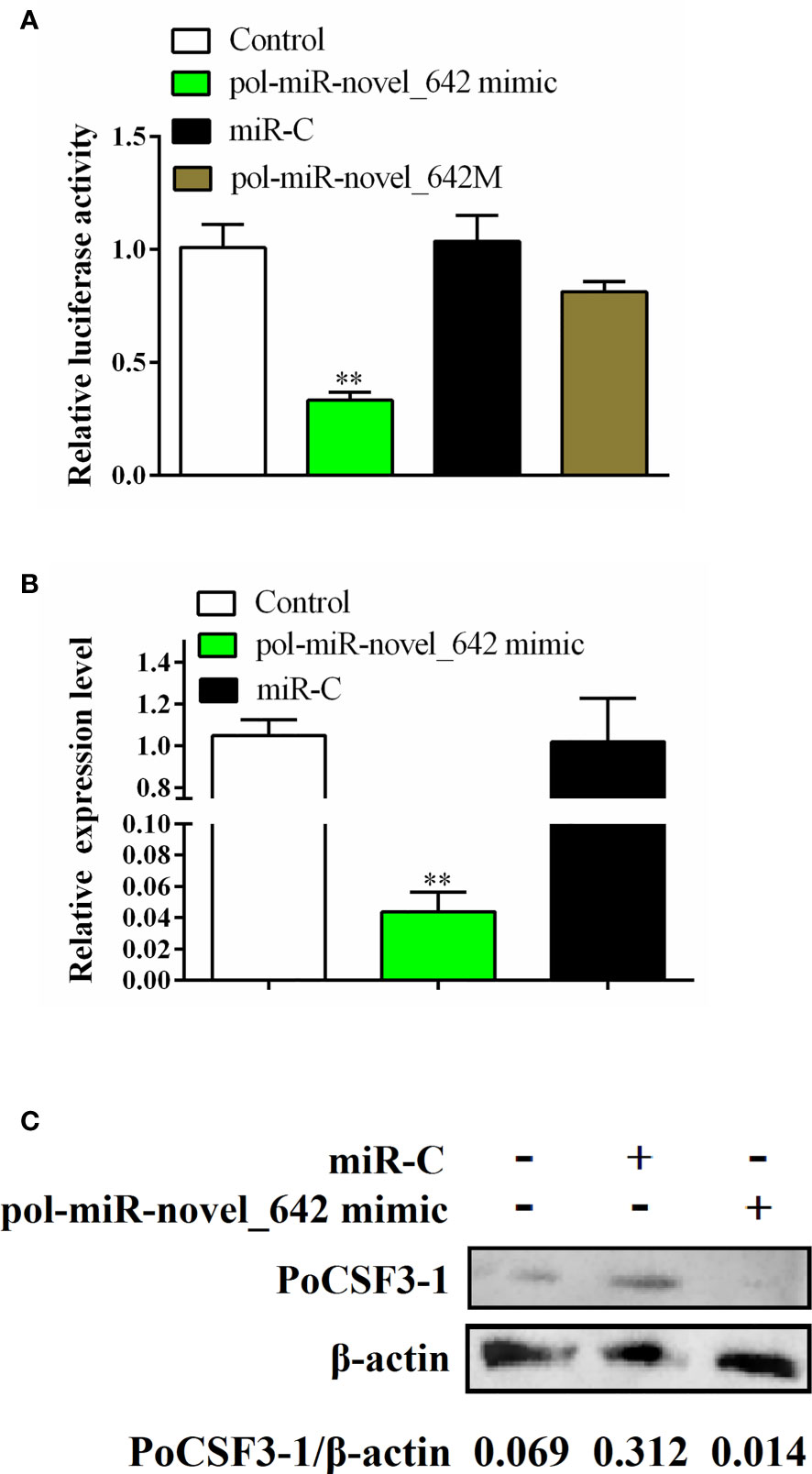
Figure 1 Regulation of PoCSF3-1 expression by pol-miR-novel_642. (A) HEK293T cells were transfected with pPoCSF3-1-Report in the absence (control) or presence of pol-miR-novel_642 mimic, miR-C (miRNA control), or pol-miR-novel_642M (mutant of pol-miR-novel_642) for 24 h, and the luciferase activity was then determined. (B, C) FG-9307 cells were transfected with or without (control) pol-miR-novel_642 mimic or miR-C, and PoCSF3-1 expression was determined by qRT-PCR (B) or Western blot with β-actin as a loading control (C). In (C), the relative densities of PoCSF3-1/β-actin are shown at the bottom of the figure. In (A, B), values are the means of triplicate experiments and shown as means ± SEM. **p < 0.01.
PoCSF3-1 and pol-miR-novel_642 Expressions Are Regulated by Bacterial and Viral Pathogens
In vivo study showed that the expressions of PoCSF3-1 and pol-miR-novel_642 in flounder were regulated by bacterial (E. tarda) and viral (megalocytivirus) pathogens (Figure 2). During E. tarda infection, pol-miR-novel_642 was significantly downregulated at 12, 24, and 48 hpi, whereas PoCSF3-1 expression was significantly upregulated at these time points (Figure 2). During megalocytivirus infection, pol-miR-novel_642 was significantly upregulated at 2, 4, 6, 8, and 10 dpi, whereas PoCSF3-1 expression was significantly downregulated at these time points (Figure 2).
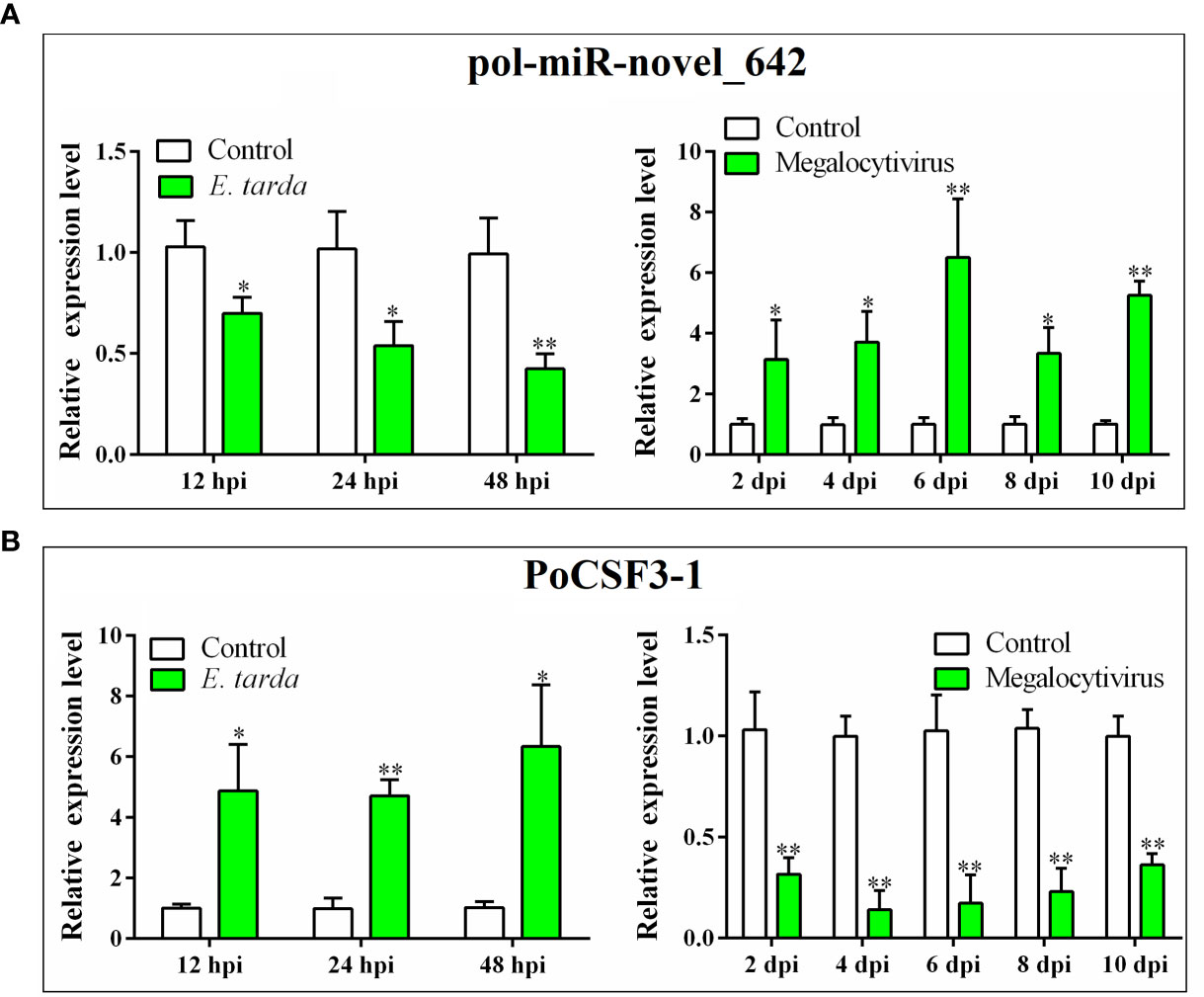
Figure 2 Expression of pol-miR-novel_642 (A) and PoCSF3-1 (B) in Japanese flounder in response to bacterial and viral infection. Flounder were infected with or without (control) Edwardsiella tarda or megalocytivirus. Pol-miR-novel_642 (A) and PoCSF3-1 (B) expression in kidney was determined by qRT-PCR at different hour post-infection (hpi) or day post-infection (dpi). Values are the means of triplicate experiments and shown as means ± SD. *p < 0.05; **p < 0.01.
PoCSF3-1 and pol-miR-novel_642 Affect Pathogen Infection
When flounder FG-9307 cells were transfected with siPoCSF3-1 (a siRNA targeting PoCSF3-1) that interferes with PoCSF3-1 expression, or when FG-9307 cells were transfected with pol-miR-novel_642 mimic, E. tarda infection in the cells was significantly enhanced (Figures 3A, B). Consistently, in vivo study showed that knockdown of PoCSF3-1 in flounder by administration of the plasmid pPoCSF3-1si (expressing a PoCSF3-1-targeting siRNA) significantly promoted E. tarda dissemination in fish tissues, whereas overexpression of PoCSF3-1 in flounder by administration of the plasmid pPoCSF3-1 (expressing PoCSF3-1) significantly inhibited E. tarda dissemination (Figures 3C, D). In addition to E. tarda, PoCSF3-1 also influenced megalocytivirus infection. PoCSF3-1 knockdown and overexpression significantly inhibited and promoted, respectively, megalocytivirus infection in flounder at 6 and 8 dpi (Figures 3E, F).
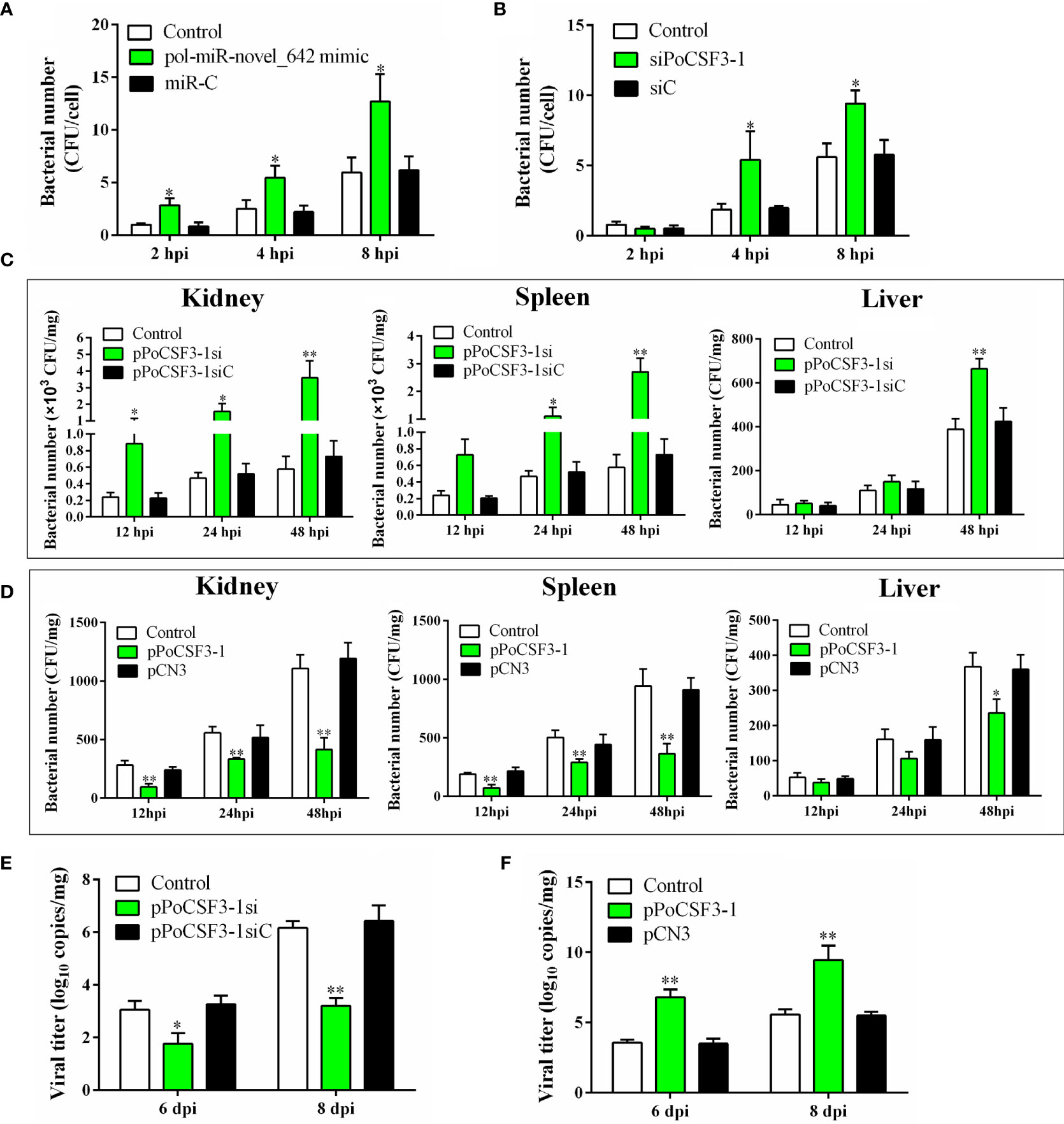
Figure 3 In vitro and in vivo effects of pol-miR-novel_642 and PoCSF3-1 on pathogen infection. (A) FG-9307 cells were transfected with or without (control) pol-miR-novel_642 mimic or miR-C (control miRNA) and then infected with Edwardsiella tarda. Intracellular bacterial number was determined at different hours post infection (hpi) and shown as CFU (colony forming unit). (B) FG-9307 cells were transfected with or without (control) siPoCSF3-1 or siC (control siRNA) and then infected with E. tarda. Intracellular bacterial number was determined as above. (C) Flounder were infected with E. tarda in the presence or absence (control) of pPoCSF3-1si or pPoCSF3-1siC (control plasmid). Bacterial loads in kidney, spleen, and liver were determined at different hpi. (D) Flounder were infected with E. tarda in the presence or absence (control) of pPoCSF3-1 or the control plasmid pCN3. Bacterial loads in tissues were determined as above. (E) Flounder were infected with megalocytivirus in the presence or absence (control) of pPoCSF3-1si or pPoCSF3-1siC, and viral copies in kidney were determined at 6 and 8 days post-injection (dpi). (F) Flounder were infected with megalocytivirus in the presence or absence (control) of pPoCSF3-1 or pCN3, and viral copies in kidney were determined as above. In all panels, values are the means of triplicate experiments and shown as means ± SD. *p < 0.05; **p < 0.01.
PoCSF3-1 and pol-miR-novel_642 Regulate Autophagy
The mechanism behind the obseved effect of PoCSF3-1 on pathogen infection was explored. qRT-PCR showed that in flounder with PoCSF3-1 overexpression, the expressions of AKT and mTOR, which are known to inhibit autophagy in mammals, were significantly repressed in kidney, spleen, and liver, while the expressions of two other autophagy related genes, ATG5 and beclin-1, were significantly induced (Figure 4A). The reverse expression patterns were detected in flounder with PoCSF3-1 knockdown (Figure 4B). Consistently, in FG-9307 cells transfected with pol-miR-novel_642 inhibitor, which inhibited pol–miR-novel_642 expression, augmented production of beclin-1 and LC3-II were detected (Figure 4C), suggesting activation of autophagy.
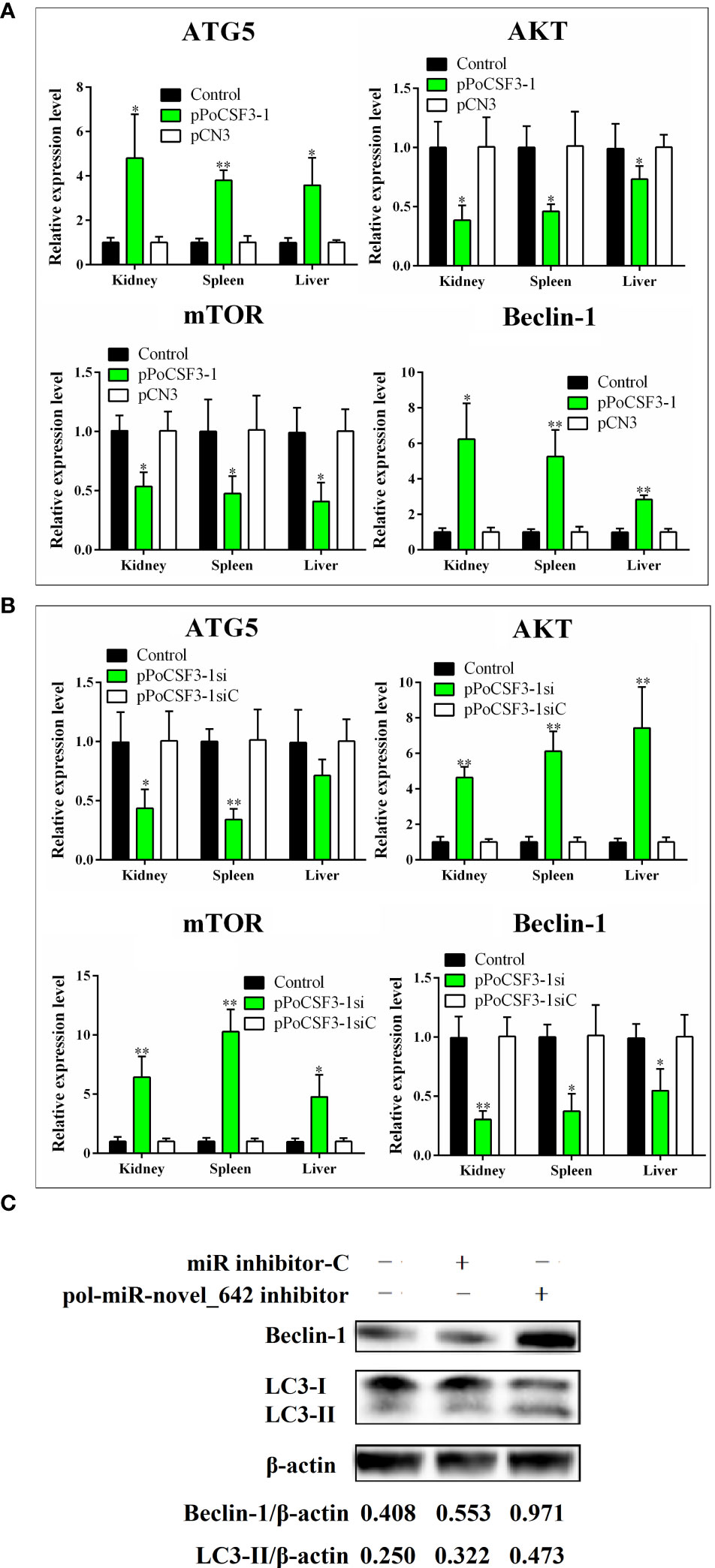
Figure 4 Effects of pol-miR-novel_642 and PoCSF3-1 on autophagy. (A) Flounder were administered with pPoCSF3-1, pCN3 (control plasmid), or PBS (control) for 7 days. The expressions of ATG5, AKT, mTOR, and beclin-1 in kidney, spleen and liver were determined by qRT-PCR. (B) Flounder were administered with pPoCSF3-1si, pPoCSF3-1siC (control plasmid), or PBS (control) for 7 days. Gene expression was determined as above. (C) FG-9307 cells were transfected with or without (control) pol-miR-novel_642 inhibitor or miR inhibitor-C (inhibitor control) for 24 h Beclin-1 and LC3-II were detected by Western blot. β-actin was used as a loading control. The relative densities of beclin-1/β-actin and LC3-II/β-actin are shown at the bottom of the figure. For panels (A, B), values are the means of triplicate experiments and shown as means ± SD. *p < 0.05; **p < 0.01.
Recombinant PoCSF3-1 (rPoCSF3-1) Interacts With Bacteria in a Structure-Dependent Manner and Affects Bacterial Survival in Host Serum
His-tagged rPoCSF3-1 was purified (Figure S3). ELISA showed that rPoCSF3-1 was able to bind Gram-negative bacteria, including E. tarda, Escherichia coli, Vibrio anguillarum, and Vibrio harveyi, but did not bind Gram-positive bacteria (Figure 5A). Consistently, rPoCSF3-1 exhibited apparent binding to lipid A (Figure 5B). In contrast, no bacteria binding activity was detected with mouse CSF3 (data not shown). rPoCSF3-1 did not kill the bound bacteria, but inhibited the growth of some of the bacteria, i.e., E. tarda and V. harveyi (Figure 5C). Structure modeling showed that both PoCSF3-1 and mouse CSF3 contain predominantly α–helixes; however, the H1 α–helix in mouse CSF3 is replaced largely by two smaller α–helixes (H1a and H1b) in PoCSF3-1 (Figure 6A). The difference is likely contributed primarily by the motif of 36ILL39 in PoCSF3-1, as mutation of this motif to 36SLE39 resulted in the disappearance of the two small α–helixes and the emergence of a large α–helix similar to that of mouse H1 (Figure 6A). Compared to the wild type rPoCSF3-1, the mutant rPoCSF3-1M that bears the 36SLE39 substitution exhibited significantly reduced binding to bacteria (Figure 6B). Serum survival analysis showed that the presence of rPoCSF3-1, but not rPoCSF3-1M, significantly enhanced the survival rate of E. tarda in flounder serum to the level of 100% (Figure S4). In contrast, rPoCSF3-1 had no effect on E. tarda survival in heat-inactivated serum (data not shown).
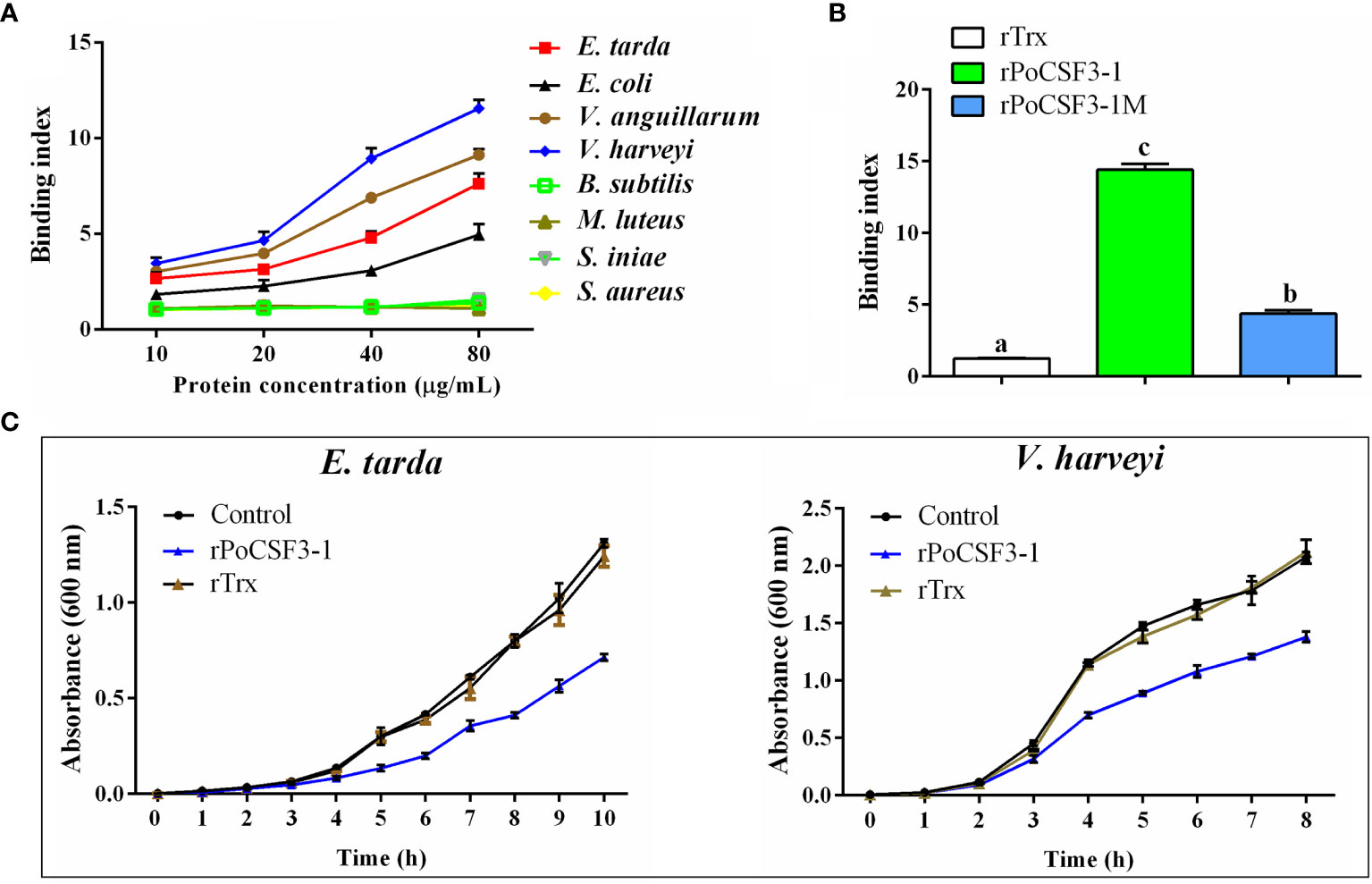
Figure 5 Interaction of rPoCSF3-1 variants with bacteria and its effect on bacterial growth. (A) Edwardsiella tarda, Escherichia coli, Vibrio anguillarum, Vibrio harveyi, Bacillus subtilis, Micrococcus luteus, Streptococcus iniae, and Staphylococcus aureus were incubated with or without (control) different concentrations of rPoCSF3-1 for 2 h The bound rPoCSF3-1 was determined by ELISA. (B) Lipid A was incubated with rPoCSF3-1, rPoCSF3-1M, rTrx, or PBS (control) for 2 h, and the protein-bound lipid A was determined by ELISA. Different letters indicate statistical significance (p < 0.05). (C) E. tarda and V. harveyi were incubated with or without (control) rPoCSF3-1 or rTrx, and bacterial growth was monitored at different hours. Values are the means of triplicate experiments and shown as means ± SEM. Different letters indicate statistical significance (p < 0.05).
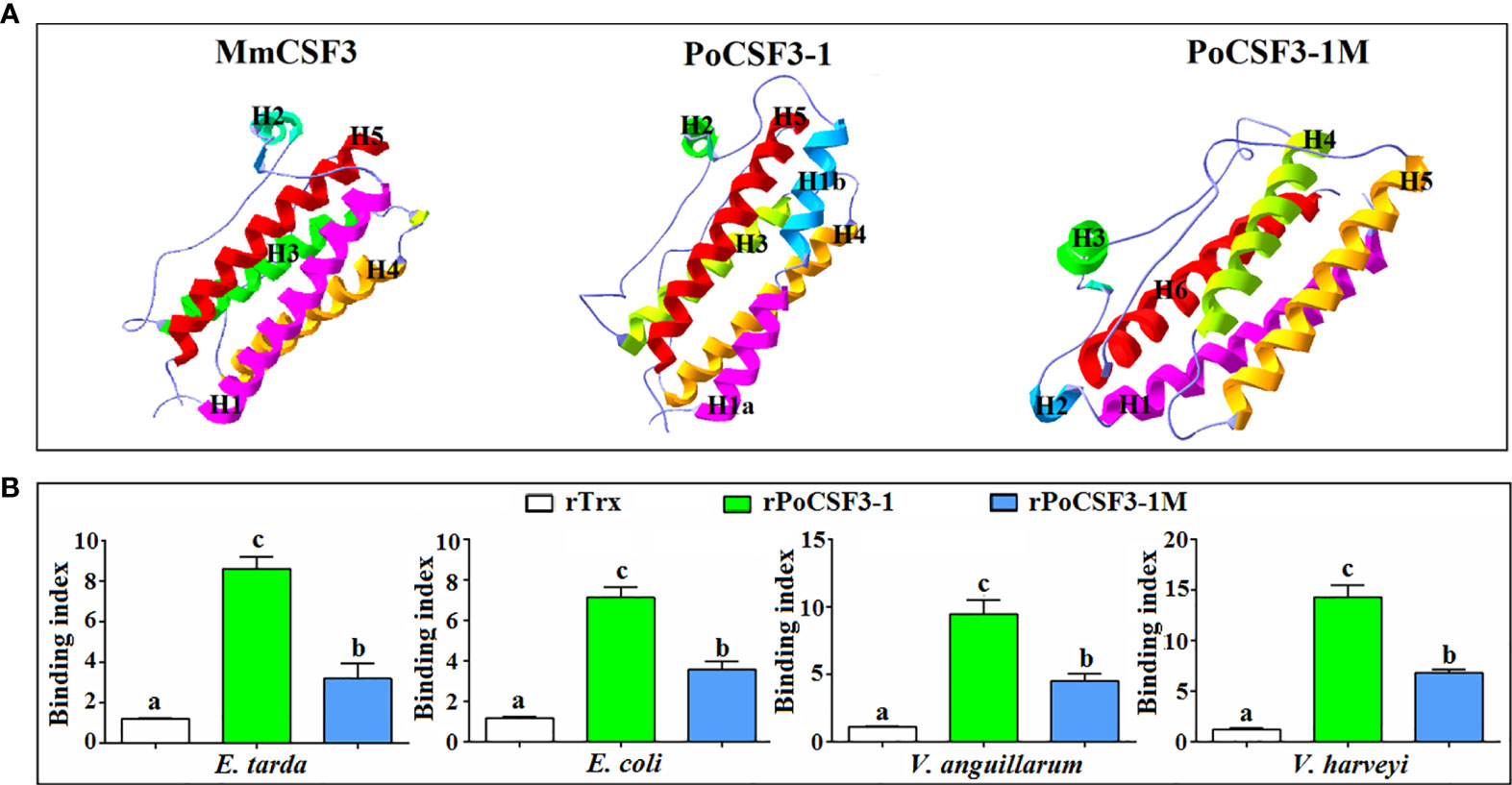
Figure 6 Structural modeling of PoCSF3-1 (A) and the structure-dependent binding of PoCSF3-1 to bacteria (B). (A) The overall three-dimensional structures of PoCSF3-1 and PoCSF3-1M were modeled using SWISS-MODEL based on the template protein of mouse CSF3 (PDB: 1PGR) and shown in ribbons with rainbow colors. (B) Bacteria were incubated with rPoCSF3-1, rPoCSF3-1M, rTrx, or PBS (control) for 2 h, and the bacteria-bound protein was determined by ELISA. Values are the means of triplicate experiments and shown as means ± SEM. Different letters indicate statistical significance (p < 0.05).
rPoCSF3-1 Regulates the Activation and Bacterial Resistance of HKLs
When HKLs were treated with rPoCSF3-1, the cells exhibited significantly enhanced proliferation (Figure S5). In these cells, the expression levels of the inflammatory cytokines of TNF-α, IL-1β, IL-6, and IL-8 significantly increased at 12 h and 24 h post-treatment (Figure 7A). The mutant rPoCSF3-1M also enhanced the expression of the cytokines, but to significantly lower degrees than that caused by rPoCSF3-1. Similarly, rPoCSF3-1 significantly upregulated the expression of the JAK/STAT pathway genes JAK2, STAT1, and STAT3 at 12 h and 24 h post-treatment, while rPoCSF3-1M only moderately, though significantly, upregulated the expression of JAK2 and STAT1, but not the expression of STAT3 (Figure 7B). Cellular infection showed that when HKLs were infected with E. tarda in the presence of rPoCSF3-1, the intracellular bacterial loads were significantly reduced compared to that in the control cells or in rPoCSF3-1M-treated cells (Figure 7C).
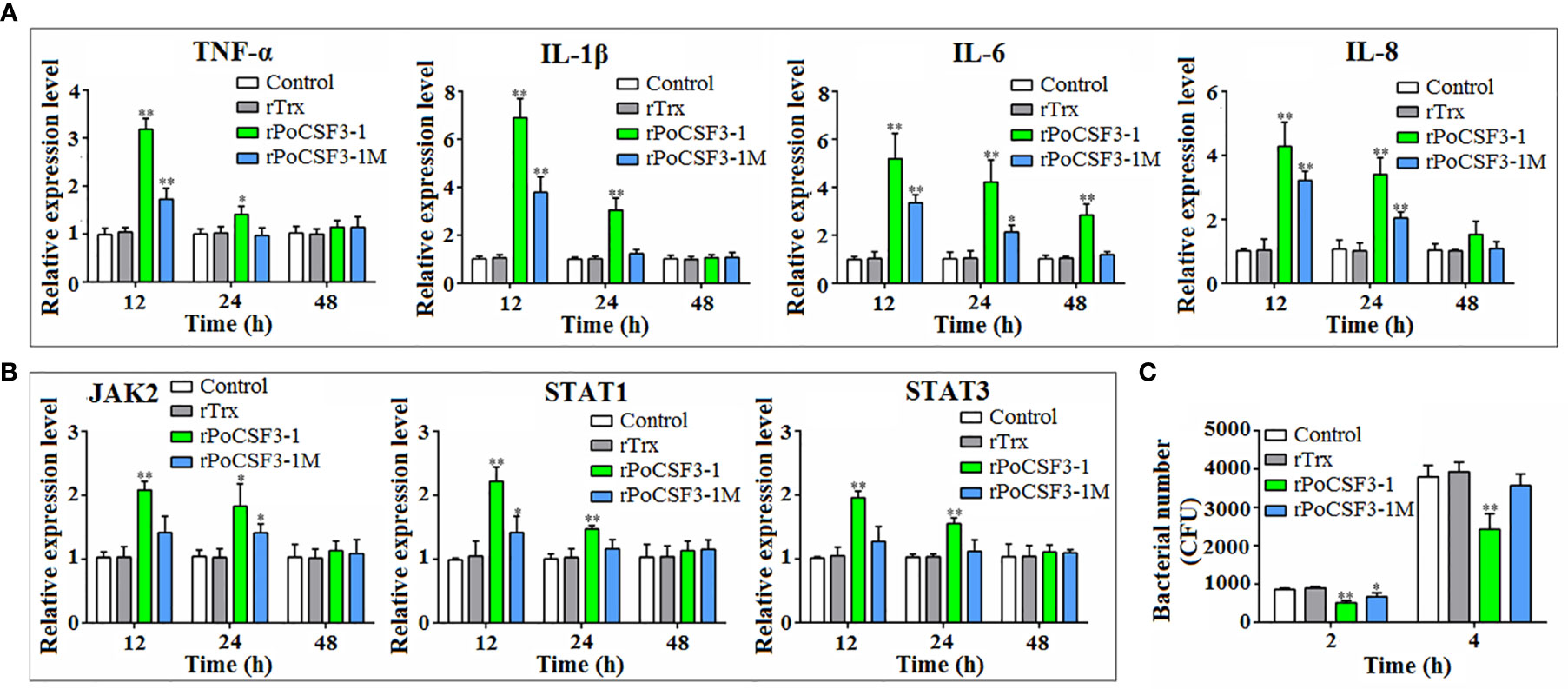
Figure 7 Effects of rPoCSF3-1 on immune gene expression and bacterial infection in head kidney leukocytes (HKLs). (A, B) Flounder HKLs were incubated with or without (control) rPoCSF3-1, rPoCSF3-1M, or rTrx for different hours. The expressions of the immune genes were determined by qRT-PCR. (C) Flounder HKLs were infected with Edwardsiella tarda in the presence or absence (control) of rPoCSF3-1, rPoCSF3-1M, or rTrx. Intracellular bacterial numbers (shown as colony forming unit, CFU) were determined at 2 and 4 hpi. Values are the means of triplicate experiments and shown as means ± SEM. *p < 0.05; **p < 0.01.
Discussion
Accumulating evidences have demonstrated that pathogen infection can induce extensive host miRNA response, which provides a potential mechanism for host antibacterial defense and pathogen manipulation of the host system (54–56). In this study, we found that flounder pol-miR-novel_642, a novel miRNA with unknown function, exhibited differential expression during E. tarda and megalocytivirus infection, suggesting an involvement of pol-miR-novel_642 in flounder immunity against bacterial and viral pathogens. In animals, miRNAs decrease mRNA stability or inhibit mRNA translation mostly through binding the 3’UTR of the target genes (4, 5, 57), while in plants, miRNA targets are often present in the coding regions of the target genes (58, 59). However, recent studies demonstrated that the coding regions of animal genes, such as zebrafish let-7 and human DNA methyltransferase 3b, can be targeted by miRNAs (1, 6, 8). In our study, luciferase reporter assay showed that pol-miR-novel_642 negatively regulated a hypothetical gene encoding an uncharacterized protein, which was named PoCSF3-1 in this study based on its structural similarity with mammalian CSF3, by specific interaction with the coding region of PoCSF3-1. Consistently, overexpression of pol-miR-novel_642 in flounder cells effectively decreased the mRNA and protein levels of PoCSF3-1. These results indicate that PoCSF3-1 is a target gene of pol-miR-novel-642.
The antimicrobial effect of CSF3/G-CSF has been widely reported. In humans, G-CSF was induced by human respiratory syncytial virus (hRSV) and constituted an important modulator of neutrophil infiltration during hRSV infection (60). In mice, G-CSF expression was elevated upon uropathogenic E. coli infection, and G-CSF-induced emigration of neutrophils was thought to be critical for bacterial clearance (61). G-CSF was required for the induction of circulating neutrophils elicited by L. monocytogenes and suppression of bacterial invasion in mouse tissues (28). In fish, the G-CSF of rock bream was highly expressed after S. iniae and E. tarda challenge (33), and the G-CSF of Japanese flounder, which shares 35.35% identity with the PoCSF3-1 identified in this study, showed altered expression during pathogen infection and regulated immune gene expression and bacterial infection (30, 38). In this study, PoCSF3-1 expression was modulated by E. tarda and megalocytivirus in a way opposite to that of pol-miR-novel_642 expression, which supported the negative regulatory relationship between pol-miR-novel_642 and PoCSF3-1. PoCSF3-1 knockdown or pol-miR-novel_642 overexpression significantly augmented E. tarda infection, whereas PoCSF3-1 overexpression significantly attenuated E. tarda infection. These observations indicate a negative effect of PoCSF3-1 on E. tarda infection. Since PoCSF3-1 expression was upregulated by E. tarda, it is likely that during the infection process, flounder activates PoCSF3-1 expression as a defense mechanism to eliminate the invading E. tarda. In contrast, PoCSF3-1 overexpression promoted megalocytivirus infection, whereas PoCSF3-1 knockdown suppressed megalocytivirus infection, suggesting that PoCSF3-1 facilitated megalocytivirus infection. Since PoCSF3-1 expression was downregulated from the early to late stages of megalocytivirus infection, it is likely that during the infection, flounder reduces the expression level of PoCSF3-1 as a strategy to block the viral infection. Together, these results indicate that PoCSF3-1 is involved in flounder immune defense against microbial pathogens, and that PoCSF3-1 plays different roles in E. tarda and megalocytivirus infection, which may be due to the different infection mechanisms of the bacterial and viral pathogens. qRT-PCR showed that PoCSF3-1 overexpression upregulated the expression of ATG5 and beclin-1, which promote autophagy (62, 63), and downregulated the expression of AKT and mTOR, which inhibited autophagy (64, 65). Consistently, pol-miR-novel_642 knockdown enhanced the production of beclin 1 and LC3 II. These results indicate that, like human CFS3 that is known to induce autophagy in an acute spinal cord injury model (66), PoCSF3-1 is able to activate the process of autophagy, which probably contributes to the observed effects of PoCSF3-1 on pathogen infection.
G-CSF has long been known as a cytokine, and no reports on direct interaction between G-CSF and bacteria have been documented. In our study, rPoCSF3-1 was found to bind Gram-negative bacteria, probably via interaction with lipid A. Binding of rPoCSF3-1 markedly inhibited the growth of E. tarda, which may explain, at least in part, the in vivo observation that PoCSF3-1 attenuated E. tarda infection. Unlike PoCSF3-1, the mutant protein rPoCSF3-1M, which bears the 36ILL39 to 36SLE39 substitution, displayed a significantly impaired ability to bind bacteria and lipid A. Structural modeling showed that mutation of 36ILL39 to 36SLE39 resulted in the loss of the PoCSF3-1-characteristic α–helixes and enabled the mutant resembling more the mouse CSF3. These results suggest that the α–helixes involving 36ILL39 are essential to bacterial interaction, and the lack of this structural feature in mouse CSF3 may possibly account for the inability of mouse CSF3 to bind bacteria.
In mammals, G-CSF is a key modulator responsible for the proliferation of neutrophils, endothelial cells, and macrophages (24, 67). Mammalian G-CSF is known to activate the JAK/STAT pathway (68, 69). For example, human G-CSF promoted phosphorylation of JAK1, JAK2, STAT3 and STAT5 in placenta or neutrophils (70, 71). In teleost, large yellow croaker GCSFa could promote the proliferation of primary head kidney leucocytes (PKL) and elevate the expression of STAT3 and STAT5 (39). In this study, we found that rPoCSF3-1 stimulated the proliferation of HKLs and enhanced the expression of inflammatory cytokines and JAK2, STAT1, and STAT3, indicating an immune regulatory function of rPoCSF3-1 involving specific JAK/STAT pathways. Consistently, rPoCSF3-1 increased the ability of HKLs to resist E. tarda infection, which is likely due to the augmented immune response induced by rPoCSF3-1. It is interesting that compared to rPoCSF3-1, rPoCSF3-1M exhibited much weaker effects on immune gene expression and bacterial infection, suggesting further the importance of the 36ILL39 motif in the activity of PoCSF3-1.
In conclusion, in this study we unraveled the functions of the novel miRNA pol-miR-novel_642 and its target gene PoCSF3-1. Our results indicate that pol-miR-novel_642 and PoCSF3-1 play a critical role in pathogen infection by affecting host immune response including autophagy, inflammation, and cellular proliferation. Importantly, we demonstrated that rPoCSF3-1 possesses the ability to directly interact with and inhibit the growth of some bacteria, and both the bacterial interaction and the immune effect of rPoCSF3-1 depend on a characteristic structural motif that is absent in murine CSF3. These results add new insights into the function of flounder CSF3 and suggest a diversified role of fish CSF3 as both a cytokine and a bacterial binding protein.
Data Availability Statement
The datasets presented in this study can be found in online repositories. The names of the repository/repositories and accession number(s) can be found in the article/Supplementary Material.
Ethics Statement
The animal study was reviewed and approved by The Ethics Committee of the Institute of Oceanology, Chinese Academy of Sciences.
Author Contributions
LS conceived the study. WL conducted the experiments. BS prepared the HKLs. WL, BS, and XG analyzed the data. WL wrote the first draft of the manuscript. LS edited the manuscript. All authors contributed to the article and approved the submitted version.
Funding
This work was supported by the grants of the National Natural Science Foundation of China (31730100), the Marine S&T Fund of Shandong Province for Pilot National Laboratory for Marine Science and Technology (Qingdao) (2018SDKJ0302-2), and the Taishan Scholar Program of Shandong Province.
Conflict of Interest
The authors declare that the research was conducted in the absence of any commercial or financial relationships that could be construed as a potential conflict of interest.
Publisher’s Note
All claims expressed in this article are solely those of the authors and do not necessarily represent those of their affiliated organizations, or those of the publisher, the editors and the reviewers. Any product that may be evaluated in this article, or claim that may be made by its manufacturer, is not guaranteed or endorsed by the publisher.
Supplementary Material
The Supplementary Material for this article can be found online at: https://www.frontiersin.org/articles/10.3389/fimmu.2021.723401/full#supplementary-material
References
1. Lewis BP, Burge CB, Bartel DP. Conserved Seed Pairing, Often Flanked by Adenosines, Indicates That Thousands of Human Genes Are microRNA Targets. Cell (2005) 120:15–20. doi: 10.1016/j.cell.2004.12.035
2. Stark A, Brennecke J, Bushati N, Russell RB, Cohen SM. Animal microRNAs Confer Robustness to Gene Expression and Have a Significant Impact on 3’UTR Evolution. Cell (2005) 123:1133–46. doi: 10.1016/j.cell.2005.11.023
3. Bartel DP. MicroRNAs: Genomics, Biogenesis, Mechanism, and Function. Cell (2004) 116:281–97. doi: 10.1016/S0092-8674(04)00045-5
4. Wu L, Fan J, Belasco JG. MicroRNAs Direct Rapid Deadenylation of mRNA. Proc Natl Acad Sci (2006) 103:4034–39. doi: 10.1073/pnas.0510928103
5. Didiano D, Hobert O. Molecular Architecture of a miRNA-Regulated 3’utr. RNA (2008) 14:1297–317. doi: 10.1261/rna.1082708
6. Duursma AM, Kedde M, Schrier M, le Sage C, Agami R. miR-148 Targets Human DNMT3b Protein Coding Region. RNA (2008) 14:872–77. doi: 10.1261/rna.972008
7. Grimson A, Farh KK, Johnston WK, Garrett-Engele P, Lim LP, Bartel DP. MicroRNA Targeting Specificity in Mammals: Determinants Beyond Seed Pairing. Mol Cell (2007) 27:91–105. doi: 10.1016/j.molcel.2007.06.017
8. Kloosterman WP, Wienholds E, Ketting RF, Plasterk RH. Substrate Requirements for Let-7 Function in the Developing Zebrafish Embryo. Nucleic Acids Res (2004) 32:6284–91. doi: 10.1093/nar/gkh968
9. O’Neill LA, Sheedy FJ, McCoy CE. MicroRNAs: The Fine-Tuners of Toll-Like Receptor Signalling. Nat Rev Immunol (2011) 11:163–75. doi: 10.1038/nri2957
10. Liu YZ, Liu YX, Han M, Du XX, Liu XM, Zhang QQ, et al. Edwardsiella Tarda-Induced miR-7a Functions as a Suppressor in PI3K/AKT/GSK3beta Signaling Pathway by Targeting Insulin Receptor Substrate-2 (IRS2a and IRS2b) in Paralichthys Olivaceus. Fish Shellfish Immunol (2019) 89:477–85. doi: 10.1016/j.fsi.2019.03.076
11. Li WR, Hu YH, Jiang S, Sun L. Global Profiling and Characterization of Japanese Flounder (Paralichthys Olivaceus) Kidney microRNAs Regulated by Edwardsiella Tarda Infection in a Time-Dependent Fashion. Fish Shellfish Immunol (2019) 93:766–80. doi: 10.1016/j.fsi.2019.07.078
12. Hu YH, Zhang BC, Zhou HZ, Guan XL, Sun L. Edwardsiella Tarda-Induced miRNAs in a Teleost Host: Global Profile and Role in Bacterial Infection as Revealed by Integrative miRNA-mRNA Analysis. Virulence (2017) 8:1457–64. doi: 10.1080/21505594.2017.1356541
13. Xu JZ, Wang YF, Tan XR, Jing HJ. MicroRNAs in Autophagy and Their Emerging Roles in Crosstalk With Apoptosis. Autophagy (2012) 8:873–82. doi: 10.4161/auto.19629
14. O’Connell RM, Rao DS, Baltimore D. MicroRNA Regulation of Inflammatory Responses. Annu Rev Immunol (2012) 30:295–312. doi: 10.1146/annurev-immunol-020711-075013
15. Nahid MA, Satoh M, Chan EK. Mechanistic Role of microRNA-146a in Endotoxin-Induced Differential Cross-Regulation of TLR Signaling. J Immunol (2011) 186:1723–34. doi: 10.4049/jimmunol.1002311
16. Zhang BC, Zhou ZJ, Sun L. Pol-miR-731, A Teleost miRNA Upregulated by Megalocytivirus, Negatively Regulates Virus-Induced Type I Interferon Response, Apoptosis, and Cell Cycle Arrest. Sci Rep (2016) 6:28354. doi: 10.1038/srep28354
17. Guan XL, Zhang BC, Sun L. Pol-miR-194a of Japanese Flounder (Paralichthys Olivaceus) Suppresses Type I Interferon Response and Facilitates Edwardsiella Tarda Infection. Fish Shellfish Immunol (2019) 87:220–25. doi: 10.1016/j.fsi.2019.01.017
18. Chu Q, Gao YH, Bi DK, Xu TJ. MicroRNA-148 as a Negative Regulator of the Common TLR Adaptor Mediates Inflammatory Response in Teleost Fish. Sci Rep (2017) 7:4124. doi: 10.1038/s41598-017-04354-9
19. Li WR, Guan XL, Jiang S, Sun L. The Novel Fish miRNA pol-miR-Novel_171 and its Target Gene FAM49B Play a Critical Role in Apoptosis and Bacterial Infection. Dev Comp Immunol (2020) 106:103616. doi: 10.1016/j.dci.2020.103616
20. Xu TJ, Chu Q, Cui JX, Zhao XY. The Inducible microRNA-203 in Fish Represses the Inflammatory Responses to Gram-Negative Bacteria by Targeting IL-1 Receptor-Associated Kinase 4. J Biol Chem (2018) 293:1386–96. doi: 10.1074/jbc.RA117.000158
21. Kumar R, Sahu SK, Kumar M, Jana K, Gupta P, Gupta UD, et al. MicroRNA 17-5p Regulates Autophagy in Mycobacterium Tuberculosis-Infected Macrophages by Targeting Mcl-1 and STAT3. Cell Microbial (2016) 18:679–91. doi: 10.1111/cmi.12540
22. Zhou XK, Li XF, Ye Y, Zhao KL, Zhuang Y, Li Y, et al. MicroRNA-302b Augments Host Defense to Bacteria by Regulating Inflammatory Responses via Feedback to TLR/IRAK4 Circuits. Nat Commun (2014) 10:3619. doi: 10.1038/ncomms4619
23. Nie L, Cai SY, Sun J, Chen J. MicroRNA-155 Promotes Pro-Inflammatory Functions and Augments Apoptosis of Monocytes/Macrophages During Vibrio Anguillarum Infection in Ayu, Plecoglossus Altivelis. Fish Shellfish Immunol (2019) 86:70–81. doi: 10.1016/j.fsi.2018.11.030
24. Barreda DR, Hanington PC, Belosevic M. Regulation of Myeloid Development and Function by Colony Stimulating Factors. Dev Comp Immunol (2004) 28:509–54. doi: 10.1016/j.dci.2003.09.010
25. Becher B, Tugues S, Greter M. GM-CSF: From Growth Factor to Central Mediator of Tissue Inflammation. Immunity (2016) 45:963–73. doi: 10.1016/j.immuni.2016.10.026
26. Welte K, Platzer E, Lu L, Gabrilove JL, Levi E, Mertelsmann R, et al. Purification and Biochemical Characterization of Human Pluripotent Hematopoietic Colony-Stimulating Factor. Proc Natl Acad Sci (1985) 82:1526–30. doi: 10.1073/pnas.82.5.1526
27. Demetri GD, Griffin JD. Granulocyte Colony-Stimulating Factor and its Receptor. Blood (1991) 78:2791–808. doi: 10.1182/blood.V78.11.2791.2791
28. Lieschke GJ, Grail D, Hodgson G, Metcalf D, Stanley E, Cheers C, et al. Mice Lacking Granulocyte Colony-Stimulating Factor Have Chronic Neutropenia, Granulocyte and Macrophage Progenitor Cell Deficiency, and Impaired Neutrophil Mobilization. Blood (1994) 84:1737–46. doi: 10.1182/blood.V84.6.1737.bloodjournal8461737
29. Basu S, Hodgson G, Zhang HH, Katz M, Quilici C, Dunn AR. “Emergency” Granulopoiesis in GCSF-Deficient Mice in Response to Candida Albicans Infection. Blood (2000) 95:3725–33. doi: 10.1182/blood.V95.12.3725.012k06_3725_3733
30. Santos MD, Yasuike M, Hirono I, Aoki T. The Granulocyte Colony-Stimulating Factors (CSF3s) of Fish and Chicken. Immunogenetics (2006) 58:422–32. doi: 10.1007/s00251-006-0106-5
31. Nam BH, An GH, Baeck GW, Kim MC, Kim JW, Park HJ, et al. Molecular Cloning and Expression of cDNAs for Two Distinct Granulocyte Colony Stimulating Factor Genes From Black Rockfish Sebastes Schlegeli. Fish Shellfish Immunol (2009) 27:360–4. doi: 10.1016/j.fsi.2009.06.005
32. Liongue C, Hall CJ, O’Connell BA, Crosier P, Ward AC. Zebrafish Granulocyte Colony-Stimulating Factor Receptor Signaling Promotes Myelopoiesis and Myeloid Cell Migration. Blood (2009) 113:2535–46. doi: 10.1182/blood-2008-07-171967
33. Jeswin J, Jeong JM, Shim JD, Bae JS, Park CI. Protective Responses of Two Paralogs of Granulocyte Colony Stimulating Factor (GCSF) in Rock Bream, Oplegnathus Fasciatus During Bacterial and Viral Infection. Fish Shellfish Immunol (2017) 65:206–12. doi: 10.1016/j.fsi.2017.04.006
34. Li QH, Xu LB, Ao JQ, Ai CX, Chen XH. Identification and Bioactivity of a Granulocyte Colony-Stimulating Factor B Homologue From Large Yellow Croaker (Larimichthys Crocea). Fish Shellfish Immunol (2019) 90:20–9. doi: 10.1016/j.fsi.2019.04.048
35. Amores AA, Force A, Yan YL, Joly L, Amemiya C, Fritz A, et al. Zebrafish Hox Clusters and Vertebrate Genome Evolution. Science (1998) 282:1711–14. doi: 10.1126/science.282.5394.1711
36. Katakura F, Nishiya K, Wentzel AS, Hino E, Miyamae J, Okano M, et al. Paralogs of Common Carp Granulocyte Colony-Stimulating Factor (G-CSF) Have Different Functions Regarding Development, Trafficking and Activation of Neutrophils. Front Immunol (2019) 19:255. doi: 10.3389/fimmu.2019.00255
37. Stachura DL, Svoboda O, Campbell CA, Espin-Palazon R, Lau RP, Zon LI, et al. The Zebrafish Granulocyte Colony-Stimulating Factors (Gcsfs): 2 Paralogous Cytokines and Their Roles in Hematopoietic Development and Maintenance. Blood (2013) 122:3918–28. doi: 10.1182/blood-2012-12-475392
38. Du HH, Huang HQ, Si KW, Dai HF, Hu YH. Granulocyte Colony Stimulating Factor (GCSF) of Japanese Flounder (Paralichthys Olivaceus): Immunoregulatory Property and Anti-Infectious Function. Fish Shellfish Immunol (2019) 89:27–34. doi: 10.1016/j.fsi.2019.03.015
39. Li QH, Chen YH, Xu LB, Yang YS, Wen Q, Gu L, et al. Identification and Bioactivity of a Granulocyte Colony-Stimulating Factor a Homologue From Large Yellow Croaker (Larimichthys Crocea). Fish Shellfish Immunol (2020) 98:168–75. doi: 10.1016/j.fsi.2020.01.003
40. Guan XL, Zhang BC, Sun L. Japanese Flounder pol-miR-3p-2 Suppresses Edwardsiella Tarda Infection by Regulation of Autophagy via P53. Dev Comp Immunol (2020) 103:103531. doi: 10.1016/j.dci.2019.103531
41. Li WR, Guan XL, Sun L. Phosphatase and Tensin Homolog (PTEN) of Japanese Flounder-its Regulation by miRNA and Role in Autophagy, Apoptosis and Pathogen Infection. Int J Mol Sci (2020) 21:7725. doi: 10.3390/ijms21207725
42. Xiu YJ, Jiang GP, Zhou S, Diao J, Liu HJ, Su BF, et al. Identification of Potential Immune-Related circRNA-miRNA-mRNA Regulatory Network in Intestine of Paralichthys Olivaceus During Edwardsiella Tarda Infection. Front Genet (2019) 10:731. doi: 10.3389/fgene.2019.00731
43. Xiu YJ, Li YR, Liu XF, Su L, Zhou S, Li C. Identification and Characterization of Long Non-Coding RNAs in the Intestine of Olive Flounder (Paralichthys Olivaceus) During Edwardsiella Tarda Infection. Front Immunol (2021) 12:623764. doi: 10.3389/fimmu.2021.623764
44. Li WR, Guan XL. PUF60 of Japanese Flounder Is Regulated by pol-miR-Novel_395 and Involved in Pathogen Infection, Autophagy, and Apoptosis. Dev Comp Immunol (2021) 123:104170. doi: 10.1016/j.dci.2021.104170
45. Wang HR, Hu YH, Zhang WW, Sun L. Construction of an Attenuated Pseudomonas Fluorescens Strain and Evaluation of Its Potential as a Cross-Protective Vaccine. Vaccine (2009) 27:4047–55. doi: 10.1016/j.vaccine.2009.04.023
46. Tong SL, Li H, Miao HZ. The Establishment and Partial Characterization of a Continuous Fish Cell Line FG-9307 From the Gill of Flounder Paralichthys Olivaceus. Aquaculture (1997) 156:327–33. doi: 10.1016/S0044-8486(97)00070-7
47. Sun K, Wang HL, Zhang M, Xiao ZZ, Sun L. Genetic Mechanisms of Multi-Antimicrobial Resistance in a Pathogenic Edwardsiella Tarda Strain. Aquaculture (2009) 289:134–39. doi: 10.1016/j.aquaculture.2008.12.021
48. Zhang BC, Zhang M, Sun BG, Fang Y, Xiao Z, Sun L. Complete Genome Sequence and Transcription Profiles of the Rock Bream Iridovirus RBIV-C1. Dis Aquat Organ (2013) 104:203–14. doi: 10.3354/dao02587
49. Zheng WJ, Sun L. Evaluation of Housekeeping Genes as References for Quantitative Real Time RT-PCR Analysis of Gene Expression in Japanese Flounder (Paralichthys Olivaceus). Fish Shellfish Immunol (2011) 30:638–45. doi: 10.1016/j.fsi.2010.12.014
50. Zhang J, Hu YH, Sun BG, Xiao ZZ, Sun L. Selection of Normalization Factors for Quantitative Real Time RT-PCR Studies in Japanese Flounder (Paralichthys Olivaceus) and Turbot (Scophthalmus Maximus) Under Conditions of Viral Infection. Vet Immunol Immunopathol (2013) 152:303–16. doi: 10.1016/j.vetimm.2012.12.018
51. Sun Y, Sun L. CsBAFF, A Teleost B Cell Activating Factor, Promotes Pathogen-Induced Innate Immunity and Vaccine-Induced Adaptive Immunity. PloS One (2015) 10:e0136015. doi: 10.1371/journal.pone.0136015
52. Wang T, Sun L. CsSAP, A Teleost Serum Amyloid P Component, Interacts With Bacteria, Promotes Phagocytosis, and Enhances Host Resistance Against Bacterial and Viral Infection. Dev Comp Immunol (2016) 55:12–20. doi: 10.1016/j.dci.2015.10.002
53. Delamare-Deboutteville J, Kawasaki M, Zoccola E, Heath CM, Bowater RO, Barnes AC. Interactions of Head-Kidney Leucocytes From Giant Grouper, Epinephelus Lanceolatus, With Pathogenic Streptococcus Agalactiae Strains From Marine and Terrestrial Origins. Fish Shellfish Immunol (2019) 90:250–63. doi: 10.1016/j.fsi.2019.04.058
54. Han JJ, Sun YN, Song WH, Xu TJ. MicroRNA-145 Regulates the RLR Signaling Pathway in Miiuy Croaker After Poly (I:C) Stimulation via Targeting MDA5. Dev Comp Immunol (2017) 68:79–86. doi: 10.1016/j.dci.2016.11.021
55. Ma F, Xu S, Liu XG, Zhang Q, Xu XF, Liu MF, et al. The microRNA miR-29 Controls Innate and Adaptive Immune Responses to Intracellular Bacterial Infection by Targeting Interferon-γ. Nat Immunol (2011) 12:861–69. doi: 10.1038/ni.2073
56. Eulalio A, Schulte LN, Vogel J. The Mammalian microRNA Response to Bacterial Infections. RNA Biol (2012) 9:742–50. doi: 10.4161/rna.20018
57. Standart N, Jackson RJ. MicroRNAs Repress Translation of m7Gppp-Capped Target mRNAs In Vitro by Inhibiting Initiation and Promoting Deadenylation. Genes Dev (2007) 21:1975–82. doi: 10.1101/gad.1591507
58. Llave C, Xie Z, Kasschau KD, Carrington JC. Cleavage of Scarecrow-Like mRNA Targets Directed by a Class of Arabidopsis miRNA. Science (2002) 297:2053–6. doi: 10.1126/science.1076311
59. Chen X. A microRNA as a Translational Repressor of APE-TALA2 in Arabidopsis Flower Development. Science (2004) 303:2022–5. doi: 10.1126/science.1088060
60. Touzelet O, Broadbent L, Armstrong SD, Aljabr W, Cloutman-Green E, Power UF, et al. The Secretome Profiling of a Pediatric Airway Epithelium Infected With hRSV Identified Aberrant Apical/Basolateral Trafficking and Novel Immune Modulating (CXCL6, CXCL16, CSF3) and Antiviral (CEACAM1) Proteins. Mol Cell Proteomics (2020) 19:793–807. doi: 10.1074/mcp.RA119.001546
61. Ingersoll MA, Kline KA, Nielsen HV. Hultgren SJ. G-CSF Induction Early in Uropathogenic Escherichia Coli Infection of the Urinary Tract Modulates Host Immunity. Cell Microbiol (2008) 10:2568–78. doi: 10.1111/j.1462-5822.2008.01230.x
62. Yao Z, Delorme-Axford E, Backues SK, Klionsky DJ. Atg41/Icy2 Regulates Autophagosome Formation. Autophagy (2015) 11:2288–99. doi: 10.1080/15548627.2015.1107692
63. McKnight NC, Yue Z. Beclin 1, an Essential Component and Master Regulator of PI3K-III in Health and Disease. Curr Pathobiol Rep (2013) 1:231–38. doi: 10.1007/s40139-013-0028-5
64. Shi BH, Ma MQ, Zheng YT, Pan YY, Lin XH. mTOR and Beclin1: Two Key Autophagy-Related Molecules and Their Roles in Myocardial Ischemia/Reperfusion Injury. J Cell Physiol (2019) 234:12562–68. doi: 10.1002/jcp.28125
65. Jafari M, Ghadami E, Dadkhah T, Akhavan-Niaki H. PI3k/AKT Signaling Pathway: Erythropoiesis and Beyond. J Cell Physiol (2018) 234:2373–85. doi: 10.1002/jcp.27262
66. Guo YJ, Liu SM, Zhang XH, Wang LY, Gao JG, Han AQ, et al. G-CSF Promotes Autophagy and Reduces Neural Tissue Damage After Spinal Cord Injury in Mice. Lab Invest (2015) 95:1439–49. doi: 10.1038/labinvest.2015.120
67. Roberts AW. G-CSF, a Key Regulator of Neutrophil Production, But That’s Not All! Growth Factors (2005) 23:33–41. doi: 10.1080/08977190500055836
68. de Koning JP, Soede-Bobok AA, Ward AC, Schelen AM, Antonissen C, van Leeuwen D, et al. STAT3-Mediated Dierentiation and Survival of Myeloid Cells in Response to Granulocyte Colony-Stimulating Factor: Role for the Cyclin-Dependent Kinase Inhibitor p27Kip1. Oncogene (2000) 19:3290–98. doi: 10.1038/sj.onc.1203627
69. Harada M, Qin Y, Takano H, Minamino T, Zou Y, Toko H, et al. G-CSF Prevents Cardiac Remodeling After Myocardial Infarction by Activating the Jak-Stat Pathway in Cardiomyocytes. Nat Med (2005) 11:305–11. doi: 10.1038/nm1199
70. Marino VJ, Roguin LP. The Granulocyte Colony Stimulating Factor (G-CSF) Activates Jak/STAT and MAPK Pathways in a Trophoblastic Cell Line. J Cell Biochem (2008) 103:1512–23. doi: 10.1002/jcb.21542
71. Sakamoto C, Suzuki K, Hato F, Akahori M, Hasegawa T, Hino M, et al. Anti-Apoptotic Effect of Granulocyte Colony-Stimulating Factor, Granulocyte-Macrophage Colony-Stimulating Factor and Cyclic AMP on Human Neutrophils: Protein Synthesis-Dependent and Protein Synthesis-Independent Mechanisms and Role of Janus Kinase-STAT Pathway. Int J Hematol (2003) 77:60–70. doi: 10.1007/BF02982604
Keywords: Paralichthys olivaceus, fish microRNA, granulocyte colony stimulating factor 3, bacterial and viral pathogens, antimicrobial immune defense
Citation: Li W, Guan X, Sun B and Sun L (2021) A Novel microRNA of Japanese Flounder Regulates Antimicrobial Immunity Involving a Bacteria-Binding CSF3. Front. Immunol. 12:723401. doi: 10.3389/fimmu.2021.723401
Received: 10 June 2021; Accepted: 04 August 2021;
Published: 19 August 2021.
Edited by:
Yong-hua Hu, Chinese Academy of Tropical Agricultural Sciences, ChinaCopyright © 2021 Li, Guan, Sun and Sun. This is an open-access article distributed under the terms of the Creative Commons Attribution License (CC BY). The use, distribution or reproduction in other forums is permitted, provided the original author(s) and the copyright owner(s) are credited and that the original publication in this journal is cited, in accordance with accepted academic practice. No use, distribution or reproduction is permitted which does not comply with these terms.
*Correspondence: Li Sun, bHN1bkBxZGlvLmFjLmNu
†These authors have contributed equally to this work