- 1School of Medicine, Nankai University, Tianjin, China
- 2Center of Pulmonary & Critical Care Medicine, Chinese People’s Liberation Army (PLA) General Hospital, Beijing, China
- 3Medical School of Chinese People’s Liberation Army (PLA), Beijing, China
Acute lung injury/acute respiratory distress syndrome (ALI/ARDS) is a common condition with high mortality. ALI/ARDS is caused by multiple etiologies, and the main clinical manifestations are progressive dyspnea and intractable hypoxemia. Currently, supportive therapy is the main ALI/ARDS treatment, and there remains a lack of targeted and effective therapeutic strategies. Macrophages are important components of innate immunity. M1 macrophages are pro-inflammatory, while M2 macrophages are anti-inflammatory and promote tissue repair. Mesenchymal stem cells (MSCs) are stem cells with broad application prospects in tissue regeneration due to their multi-directional differentiation potential along with their anti-inflammatory and paracrine properties. MSCs can regulate the balance of M1/M2 macrophage polarization to improve the prognosis of ALI/ARDS. In this paper, we review the mechanisms by which MSCs regulate macrophage polarization and the signaling pathways associated with polarization. This review is expected to provide new targets for the treatment of ALI/ARDS.
1 Introduction
ALI/ARDS
Acute lung injury (ALI) and its more severe form, acute respiratory distress syndrome (ARDS), are critical illnesses caused by excessive and uncontrolled systemic inflammatory responses to direct or indirect lung injury. ALI is defined as the acute onset of diffuse bilateral pulmonary infiltrates by chest radiograph, and with a PaO2/FiO2 ≤ 300mmHg or without clinical evidence of left atrial hypertension (1). Those with more severe hypoxemia (PaO2/FiO2 ≤ 200mmHg) are considered to have ARDS. While according to the Berlin definition, ARDS is divided into three categories in terms of the degree of hypoxemia: mild (PaO2/FiO2 = 200–300 mmHg); moderate (PaO2/FiO2 = 100–200 mmHg); and severe (PaO2/FiO2 < 100 mmHg) (2). ALI and ARDS are characterized by severe intractable hypoxemia, hypoxic respiratory failure, alveolar and interstitial pulmonary edema, decreased pulmonary compliance, and increased pulmonary vascular permeability (3, 4). The main pathological changes associated with ALI/ARDS include damage to alveolar epithelial cells and alveolar capillary endothelial cell barriers, the exudation of protein-rich edema fluid from the alveolar lumen, and the infiltration of inflammatory cells such as neutrophils and macrophages (5).
ALI/ARDS may be caused by a variety of factors including shock, severe sepsis, pulmonary contusion, gastroesophageal reflux, aspiration of gastric contents, pneumonia, drug toxicity, blood transfusion, acute pancreatitis, ischemia-reperfusion, and drowning (6).
The pathogenesis of ALI/ARDS is complex, and the main pathogenic mechanisms are excessive inflammatory response caused by the massive release of pro-inflammatory cytokines and damage to alveolar epithelial cells resulting from the excessive activation of multiple immune cells. The lung tissue can be stimulated by multiple factors to trigger infiltration of various inflammatory cells such as macrophages and neutrophils, thereby releasing large amounts of pro-inflammatory cytokines and inflammatory mediators. This disrupts the integrity of alveolar epithelial cells and the pulmonary capillary endothelial cell barrier, leading to pulmonary edema and alveolar hemorrhage (6, 7). ARDS can be classified into two subtypes, hyperinflammatory and hypoinflammatory, based on clinical features such as oxygenation index and inflammation-related biomarkers such as the levels of IL-6, IL-8, IL-18, TNF-α, and ACE2. The hyperinflammatory subtype of ARDS has poor prognosis and high mortality (8).
Treatment of ALI/ARDS is supportive. The current clinical treatment of ALI/ARDS is based on lung-protective mechanical ventilation and fluid management therapy (9), supplemented by glucocorticoids (10, 11), surfactants (12, 13), N-acetylcysteine (14, 15), statins (16, 17), β2 agonists (18), neuromuscular blockade (3, 19), extracorporeal membrane pulmonary oxygenation (20) and prophylaxis for venous thromboembolism (21). In addition, for infections such as sepsis-induced ARDS, antimicrobial drugs can be used pertinently (22). Moreover, for ARDS caused by blood transfusion, the dose-response relationship between the amount of blood products transfused in patients and the risk of developing ARDS suggested that restrictive transfusion policies may reduce the incidence of ARDS in these patients (23, 24). Although these supportive treatments can improve patients’ symptoms to a certain extent, they do not significantly improve the prognosis, and the associated mortality remains high. An international multicenter prospective cohort study indicated that the intensive care unit (ICU) admission of ARDS patients was 10.4%, with an overall mortality rate of 35%–46% (25). Another large clinical trial found a 43% mortality rate at 90 days in patients with ARDS (26). Even patients in recovery may face long-term cognitive impairment and impaired quality of life (27). Moreover, although mechanical ventilation is the basic and important methods for the treatment of ALI/ARDS, long-term/excessive mechanical ventilation may lead to ventilator-related lung injury (VILI) and even pulmonary fibrosis (28–33). Therefore, there is an urgent need to explore more effective and safe therapeutic measures for ALI/ARDS.
Mesenchymal Stem Cells (MSCs)
MSCs are pluripotent, self-renewing stem cells with multi-directional differentiation potential and migration ability. MSCs originate from a variety of organs and tissues such as bone marrow, adipose, muscle, umbilical cord, and placenta tissues. Based on these characteristics, MSCs are widely used in the field of tissue regeneration (34, 35). MSCs can regulate immune homeostasis, reduce lung inflammation, repair tissue damage, and promote tissue regeneration, making them promising for the treatment of ALI/ARDS (36–39).
Macrophages
The presence of macrophages in lung tissue plays a critical role in the inflammatory response to ALI/ARDS. Macrophages in lung tissue mainly include two subpopulations: alveolar macrophages and interstitial macrophages. Alveolar macrophages, which are more abundant than interstitial macrophages, are the first line of defense against foreign invading factors and play an important role in host defense and the maintenance of immune homeostasis in the local microenvironment of lung tissue (40–42). In response to stimulation by foreign pathogens, alveolar macrophages can release a variety of inflammatory factors and chemokines to initiate a cascade of amplified inflammatory responses within lung tissue and mediate lung tissue injury.
Macrophages have good plasticity and can polarize into different phenotypes under different environmental conditions, including classically activated M1 macrophages and alternatively activated M2 macrophages (Figure 1). M1 macrophages produce a large amount of pro-inflammatory cytokines, which cause tissue damage while facilitating the host immune clearance of pathogens. M2 macrophages mainly secrete anti-inflammatory cytokines, which facilitate wound healing and the repair of tissue damage (43–45).
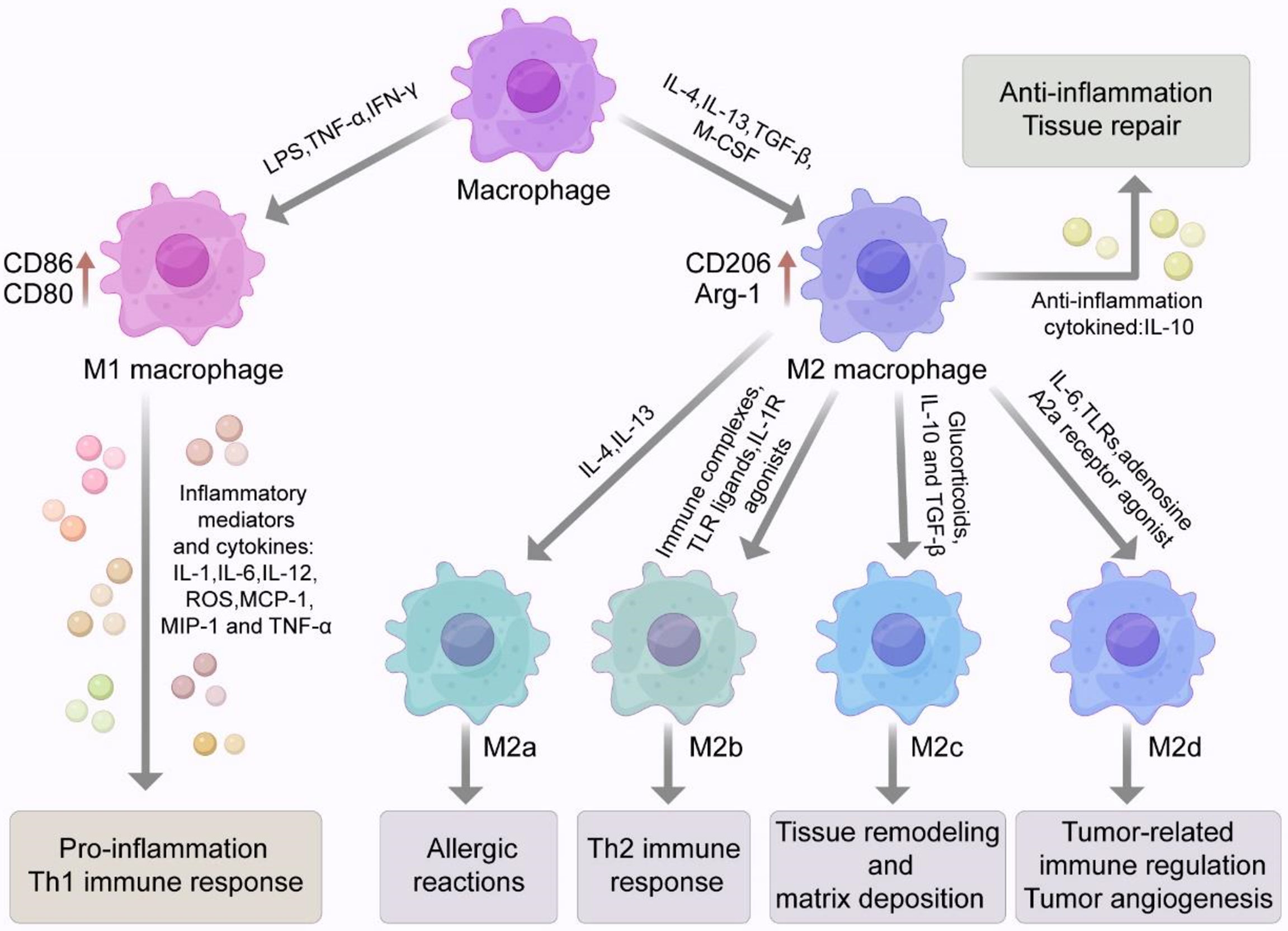
Figure 1 Macrophage phenotype and polarization. Macrophages have good plasticity and can differentiate into two phenotypes, classically activated M1 macrophages or alternatively activated M2 macrophages under different environmental conditions. Stimulated by LPS or Th1 related cytokines such as IFN-γ and TNF-α, macrophages can polarize into the M1 phenotype with high expression of surface markers such as CD86 and CD80. M1 macrophages can secrete a variety of pro-inflammatory cytokines and inflammatory mediators that can cause a strong inflammatory response and cause tissue damage. M2 macrophages are usually induced by IL-4, IL-13, TGF-β and M-CSF. M2 macrophages highly express specific surface markers such as CD206, CD163, Arg-1, Ym-1 and Fizz 1. M2 macrophage also secrete a large amount of the anti-inflammatory cytokine IL-10, which inhibits inflammatory responses and participates in tissue repair. M2 macrophages can be divided into four subtypes (M2a, M2b, M2c, M2d). Activation of M2a macrophages is induced by IL-4 and IL-13 and may be involved in allergic reactions. M2b macrophages are induced by immune complexes (ICs), Toll-like receptor (TLR) ligands and IL-1R agonists. Glucocorticoids, IL-10 and TGF-β are involved in the induction of M2c macrophages and may be involved in tissue remodeling and stromal deposition. M2d macrophages are induced synergistically by TLR and adenosine A2a receptor agonists or IL-6. With the characteristics of tumor-like macrophages, they can be involved in tumor-associated immune regulation and tumor angiogenesis.
The M1 and M2 phenotypes of macrophages can be interconverted under certain conditions. M1 and M2 macrophages can effectively avoid excessive inflammatory responses that cause tissue damage by maintaining the immune homeostasis of the lung microenvironment. In the progression of ALI/ARDS, the balance of M1 and M2 macrophages can effectively remove harmful substances and over-produced pro-inflammatory cytokines from the body to promote the repair of lung tissue damage. However, the loss of balance may exacerbate lung injury and worsen ALI/ARDS. MSCs can modulate macrophage function by regulating the polarization of macrophages; thus, MSCs show promise for the treatment of ALI/ARDS and the improvement of ALI/ARDS patient prognosis (46–50).
2 Macrophage Phenotypes and ALI/ARDS
M1 Macrophages and ALI/ARDS
Macrophages are usually polarized to the M1 phenotype in response to microbial stimuli including lipopolysaccharide (LPS) and Th1-related cytokines such as IFN-γ and TNF-α (44) (Figure 1). M1 macrophages highly express CD86, CD80, and other surface markers. M1 macrophages also secrete numerous inflammatory mediators and cytokines such as TNF-α, IL-1, IL-6, IL-12, iNOS, reactive oxygen species (ROS), monocyte chemotactic protein 1 (MCP-1), and macrophage inflammatory protein 2 (MIP-2). These compounds lead to a strong inflammatory response and Th1 immune response and cause tissue damage while participating in pathogen clearance (51). In the early stage of ALI/ARDS, alveolar macrophages are M1-polarized and release various pro-inflammatory factors and harmful mediators while clearing pathogenic microorganisms and recruiting neutrophils and other inflammatory cells. Eventually, the excessive accumulation of pro-inflammatory cytokines and inflammatory cells lead to lung tissue injury (52).
M2 Macrophages and ALI/ARDS
M2 macrophages are usually induced by IL-4, IL-13, TGF-β, and macrophage colony-stimulating factor (M-CSF; Figure 1). Activated M2 macrophages highly express arginase 1 (Arg-1) and specific surface markers such as CD206, CD163, chitinase 3-like 3 (Ym-1) and found in inflammatory zone 1 (Fizz1). M2 macrophages also secrete large amounts of the anti-inflammatory cytokine IL-10, which inhibits inflammatory cell aggregation and negatively regulates the production of anti-inflammatory factors (53).
M2 macrophages can be further divided into four subtypes: M2a, M2b, M2c, and M2d (Figure 1). The activation of M2a macrophages is induced by IL-4 and IL-13, which can be involved in allergic reactions, wrapping and killing parasites. M2b macrophages are induced by immune complexes (ICs), Toll-like receptor (TLR) ligands, and IL-1R agonists. M2b macrophages, which secret high levels of IL-10 and low levels of IL-12, which is conductive to the Th2 immune responses. Glucocorticoids, IL-10, and TGF-β are involved in the induction of M2c macrophages. Activated M2c macrophages negatively regulate the production of anti-inflammatory cytokines involved in tissue remodeling and matrix deposition (43, 44, 54–56). M2d macrophages are synergistically induced by TLRs and adenosine A2a receptor agonists or by IL-6. M2d macrophages have the characteristics of tumor-like macrophages and can participate in tumor-related immune regulation, tumor growth, and tumor angiogenesis (57–59). In addition, some investigators also described a new subpopulation of pro-resolving macrophages, namely CD11blow macrophages that emerge during the resolution of zymosan-induced peritonitis in mice. These macrophages secrete pro-resolving mediators and are generated by M2-like macrophages in vivo and in vitro after phagocytosis of apoptotic leukocytes (60). Another study suggested that pro-resolving macrophages could produce antiangiogenic mediators such as endostatin, which could halt angiogenesis and restore tissue structure (61). In summary, macrophages are highly plastic cells. They may exert a vast “spectrum” of functions characterized by an array of different macrophage phenotypes/subtypes (62).
M2 macrophages are key regulators of tissue repair during ALI/ARDS recovery (63, 64). M2 macrophages can release anti-inflammatory cytokines, inhibit the production of pro-inflammatory mediators, and remove apoptotic neutrophils from inflammatory sites to promote lung injury repair. M2 macrophages can also suppress the expression of iNOS and prevent the production of ROS, thereby reducing damage to alveolar epithelial cells and promoting the recovery of host tissues (52, 65–67).
In summary, M2 macrophages can suppress inflammation, promote tissue remodeling, and participate in angiogenesis and immune regulation. M2 macrophages are also thought to promote fibrosis. The TGF-β1 secreted by M2 macrophages can trigger fibroblast activation and extracellular matrix-producing myofibroblast development while promoting the resolution of inflammatory response to drive fibrosis (68, 69). Maintaining the appropriate balance of M1 and M2 macrophages in vivo thus provides a target for the treatment of ALI/ARDS.
3 MSCs Modulate Macrophage Polarization to Attenuate ALI/ARDS
MSCs can regulate the transition of macrophages to play an important role in ALI/ARDS. In a model of ALI caused by sulfur mustard, treatment with adipose-derived MSCs decreased the expression of CD86 markers by macrophages, suggesting that MSCs inhibited macrophage polarization to the pro-inflammatory phenotype and promoted polarization to the anti-inflammatory phenotype (70). A study found that heat shock-pretreated umbilical cord-derived MSCs (UC-MSCs) exhibited enhanced immunomodulatory effects by inducing anti-inflammatory macrophage polarization (49). Moreover, the levels of M1 markers were significantly elevated after LPS precondition, while co-culturing with heat shock-pretreated UC-MSCs reversed this effect. This may be because heat shock pretreatment enhanced the protein levels of HSP70 in UC-MSCs and negatively regulated the activation of inflammasomes in macrophages (49). Another study found that a stress response protein with anti-oxidant capacity was highly expressed in MSCs; the knocking down of this protein decreased IL-10 secretion and diminished anti-inflammatory macrophage polarization (71).
Dental follicle stem cells, a unique population of MSCs, were found to attenuate histopathological damage and lung permeability, downregulate pro-inflammatory cytokines such as MCP-1, IL-6, and TNF-α, upregulate the anti-inflammatory cytokine IL-10, increase the expression of the anti-inflammatory macrophage marker Arg-1, and decrease the production of the pro-inflammatory macrophage polarization markers iNOS and CD86 (72). Morrison et al. found that co-culturing macrophages stimulated with bronchoalveolar lavage fluid (BALF) from ARDS patients with human bone marrow MSCs (BMSCs) increased the expressions of anti-inflammatory macrophage markers (73). The above studies demonstrate that MSCs can attenuate lung histopathological changes and the inflammatory response in the ARDS inflammatory microenvironment by regulating the balance between pro-inflammatory and anti-inflammatory macrophages.
4 How MSCs Regulate Macrophage Polarization
MSCs Regulate Macrophage Polarization Through the Paracrine Effects of Soluble Factors
MSCs can home to injured sites to reduce inflammatory response. MSCs differentiate toward type II alveolar epithelial cells, which participate in the tissue repair process (74). However, some studies suggested MSCs retained a low number and had a short duration in the injured tissue after transplantation (75–79). Therefore, MSCs homing and differentiation are not the key mechanisms of their effects in ALI/ARDS. Rather, paracrine action may be the primary mechanism of MSCs involvement in tissue injury and repair (Figure 2).
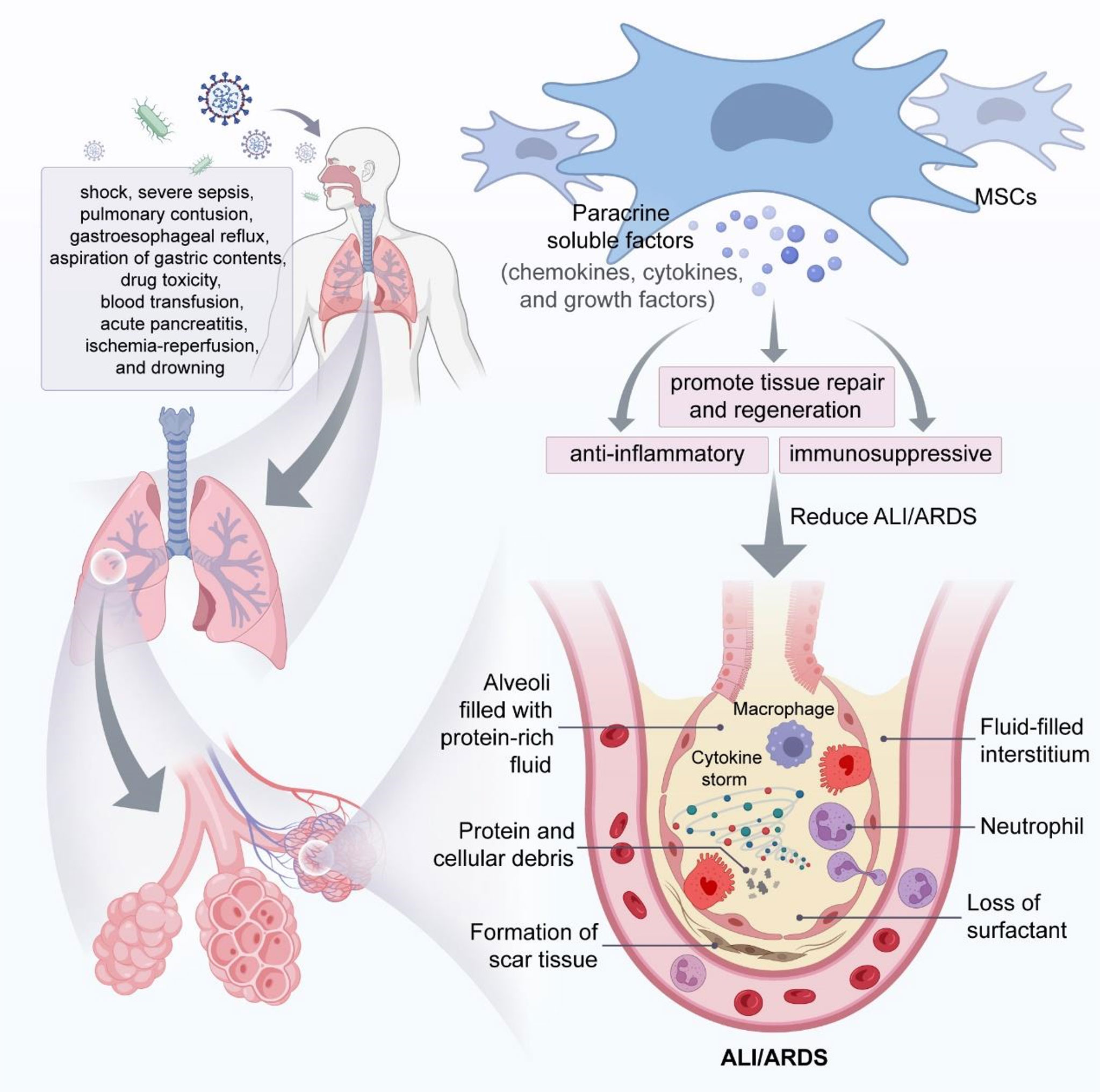
Figure 2 MSCs attenuate ALI/ARDS through paracrine soluble factors. In the phase of ALI/ARDS, immune cells (e.g., macrophages and neutrophils) accumulate in the alveolar space and produce large amounts of cytokines, leading to a cytokine storm that will eventually cause decreased surfactant from alveolar epithelial cells and fluid accumulation in the alveolar space and interstitium, resulting in alveolar and pulmonary interstitial edema, and MSCs can play a key role in mitigating ALI/ARDS through the immunomodulatory effects of paracrine soluble factors.
MSCs secret soluble factors such as chemokines, cytokines, and growth factors, which exert paracrine effects to promote tissue repair and regeneration (80, 81). The paracrine action of MSCs plays a crucial anti-inflammatory and immunosuppressive role in immune cells. Wakayama et al. reported that MSCs promoted macrophage polarization to the anti-inflammatory phenotype via a paracrine mechanism, expressed high levels of CD206 and Arg-1, attenuated pro-inflammatory responses, and reduced bleomyclin-induced ALI in mice (82).
MSCs from dental capsules promoted anti-inflammatory polarization via the paracrine secretion of TGF-β3 and thrombospondin-1 to upregulate the level of anti-inflammatory factors, thereby reducing the pulmonary inflammatory response (72). Kwon et al. suggested that UC-MSCs could regulate the anti-inflammatory response by secreting decorin, a key regulator that polarizes inflammatory macrophages into anti-inflammatory macrophages (83). Kim et al. reported that the paracrine secretion of Pentraxin 3 from UC-MSCs enhanced the levels of anti-inflammatory macrophage markers and anti-inflammatory cytokines to improve hyperoxic lung injury (84). MSCs also attenuated lung inflammation by promoting the anti-inflammatory macrophage phenotype via the paracrine secretion of insulin-like growth factor (85). Prostaglandin (PGE2) secreted by MSCs induced an immunosuppressive anti-inflammatory phenotype in alveolar macrophages, increased the ability to produce IL-10, and inhibited the production of TNF-α (86). Other soluble factors such as Galectin-9 (87), tumor necrosis factor-stimulated gene 6 (TSG-6) (88), indoleamine 2,3-dioxygenase (89), and IL-6 (90) may also be involved in the regulation of macrophage polarization to combat inflammation.
MSCs Regulate Macrophage Polarization via Their Derived Exosomes
MSC-derived extracellular vesicles can be divided into three main subtypes based on their biological properties and diameters: exosomes (30–150 nm), microvesicles (100–1000 nm), and apoptotic vesicles (50–5000 nm) (91–94) (Figure 3). Exosomes are produced through the intracellular body pathway as follows. The cell membrane is invaginated to form early endosomes, which interact with vesicles formed by Golgi apparatus budding to form late endosomes. The late endosomes further develop into multivesicular bodies (MVBs) that contain intracellular vesicles. The MVBs fuse with the lysosomal membrane or cell membrane and degrade, releasing the contents (i.e., exosomes) into the extracellular environment through exocytosis (95, 96). The process of exosome formation is shown in Figure 3. The endosomal sorting complex required for transport plays a key role in exosome biogenesis (97). The density of exosomes is approximately 1.13–1.19 g/ml, and exosomes are widely found in plasma, serum, urine, BALF, breast milk, saliva, tears, ascites, and other body fluids (98–104). Exosomes express tetraspanins (CD9, CD81, CD63, and CD82), TSG10, heat-shock proteins, and apoptosis-linked gene 2-interacting protein X (Alix) (105–107). Exosomes carry proteins, lipids, and RNAs that mediate intercellular communication between different cell types, participate in the regulation of cellular activity, and perform a variety of biological functions (Figure 3) (108–111). Moreover, exosomes derived from MSCs can act as carriers of transported miRNA, enabling communication with recipient cells; thus, MSC-derived exosomes can affect disease pathogenesis along with tissue repair and regeneration (112, 113).
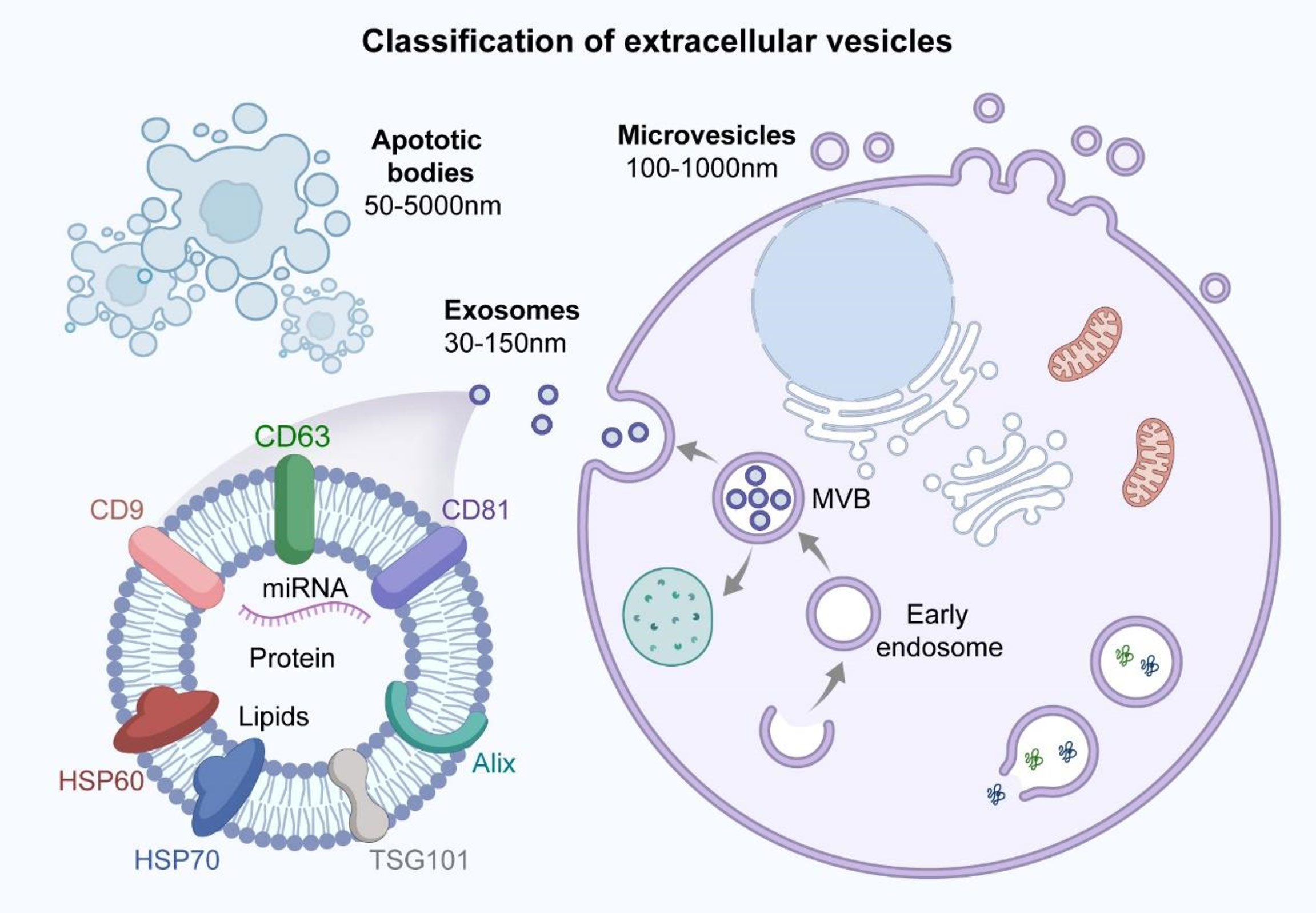
Figure 3 Classification of extracellular vesicles and structural characteristics of exosomes. Extracellular vesicles can be classified into three main subtypes based on biological properties and diameter size: exosomes (30-150nm), microvesicles (100-1000nm) and apoptotic bodies (50-5000nm). The surface of exosomes contains specific protein markers such as tetraspanins (CD9, CD81, CD63), HSP60, HSP70, TSG101 and Alix. Exosomes carry proteins, lipids and miRNA that are involved in mediating intercellular communication and regulating cellular activities, performing a variety of biological functions.
MSC-derived exosomes can attenuate ALI/ARDS by regulating macrophage polarization. Tian et al. found that adipose MSC-derived exosomes inhibited TLR4 expression to promote macrophage polarization and attenuate septic lung injury in mice (114). Song et al. reported that miR-146a expression was upregulated in MSC-derived exosomes after IL-1β stimulation, leading to macrophage polarization into the anti-inflammatory phenotype and increased survival in mice with sepsis-induced lung injury (115). Bao et al. demonstrated that MSC-derived exosomes can regulate alveolar macrophage pro-inflammatory polarization and block the pro-inflammatory pathway of macrophages, thereby reducing radiation-induced lung injury in mice (116). Lastly, Li et al. reported that the intratracheal administration of MSC-derived exosomes decreased pro-inflammatory polarization in alveolar macrophages while increasing anti-inflammatory polarization, leading to reduced pulmonary edema and pulmonary dysfunction along with decreased secretion of inflammatory factors such as IL-1β, IL-6, and TNF-α (117).
MSCs Regulate Macrophage Polarization Through Metabolic Reprogramming
Macrophage polarization is closely related to metabolic status. Pro-inflammatory macrophage polarization is characterized by enhanced glycolysis and pentose phosphate pathways and decreased oxidative phosphorylation. In contrast, anti-inflammatory macrophage polarization is characterized by enhanced oxidative phosphorylation, and anti-inflammatory macrophages rely on oxidative phosphorylation for energy production (118–121). MSCs can induce anti-inflammatory macrophage polarization and promote tissue repair through metabolic reprogramming (122). Deng et al. found that BMSC-derived exosomes inhibited pro-inflammatory polarization and promoted anti-inflammatory polarization in mice alveolar macrophages (63). The authors further demonstrated that BMSC-derived exosomes reduced LPS-induced lung pathological injury by inhibiting the expression of hypoxia-inducible factor 1-α and downregulating the expressions of key proteins of macrophage glycolysis, thereby regulating macrophage polarization and inflammatory response.
MSCs Regulate Macrophage Polarization Through Mitochondrial Transfer
In addition to increased lung epithelial and endothelial barrier permeability, the inflammatory response of ALI/ARDS can also cause mitochondrial dysfunction in lung tissue (123). MSCs may provide mitochondria to damaged cells, thereby enhancing cellular bioenergetics and improving organ dysfunction in ALI/ARDS and other inflammatory diseases. MSCs have been shown to restore mitochondrial function and alveolar bioenergetics through functional mitochondrial transfer, promote pulmonary epithelial injury repair, and improve alveolar capillary barrier permeability (124, 125).
Jackson et al. found that the extensive mitochondrial transfer of MSCs to macrophages enhanced macrophage phagocytosis and played an antibacterial role in a model of lung injury caused by E. coli-induced pneumonia (126). The mitochondrial transfer of MSCs plays a key role in regulating macrophage phenotype; Morrison et al. found that MSCs promoted macrophage oxidative phosphorylation through extracellular vesicle-mediated mitochondrial transfer and increased the expression of the anti-inflammatory macrophage marker CD206, thereby converting macrophages into the anti-inflammatory phenotype and ameliorating lung injury (73). Moreover, MSCs-derived exosomes can donate mitochondria components, improve macrophage mitochondrial integrity and oxidative phosphorylation levels, transform macrophages into an anti-inflammatory phenotype, and restore the metabolic and immune homeostasis of alveolar macrophages and reduce lung inflammation (127).
MSCs Regulate Macrophage Polarization Through Apoptotic and Efferocytosis Effects
MSCs undergo necessary apoptosis and releases apoptotic vesicles during therapeutic application, apoptotic MSCs have unique anti-inflammatory properties and immunomodulatory effects (128). Apoptotic umbilical cord MSCs were able to reduce inflammatory exudates and vascular permeability in ALI rat lungs more effectively than normal umbilical MSCs. It could also more effectively reduce the level of pro-inflammatory factors and could more effectively increase the expression of anti-inflammatory cytokine IL-4, which was able to reduce the degree of pathological damage in rats with ALI (129). In addition, apoptotic MSCs could also exert a beneficial role in sepsis and allergic airway inflammation (130, 131).
Macrophages and monocytes that phagocytose apoptotic cells are capable of producing anti-inflammatory mediators (132, 133). When graft-versus-host disease patients were infused with in vitro-generated apoptotic MSCs, it was observed that recipient phagocytes engulfed the apoptotic MSCs and produced indoleamine 2,3-dioxygenase, thereby achieving an immunosuppressive effect (134).
In an animal model of ovalibumin-induced allergic asthma, the investigators showed that apoptotic MSCs exerted immunosuppressive effects in the lungs and inhibited allergic asthma to a similar extent as administration with viable MSCs (135). Besides, apoptotic MSCs inhibited the inward flow of eosinophils in BALF and the production of specific IL-5 and IL-13 in ovalbumin-induced allergic asthma to a similar extent as surviving MSCs, which indicated that the immunosuppressive effects of MSCs in the lungs did not require MSCs to retain survival (135). Moreover, in myelin oligodendrocyte glycoprotein-induced experimental autoimmune encephalitis models, BKX-MSCs (depletion of the apoptotic effectors BAK and BAX) could not reduce circulating inflammatory monocytes that infiltrated the central nervous system to cause tissue injury, compared to untreated MSCs, which suggested that apoptotic MSCs were necessary for efficient in vivo immunosuppression and therapeutic effect (135).
The apoptotic and efferocytosis effects of MSCs could regulate macrophage phenotype polarization. For example, a study showed that efferocytosis of adipose-derived MSCs could alter the macrophages phenotype toward regulatory and anti-inflammatory phenotype (136). Another study suggested that MSC‐derived apoptotic vesicles exerted extensive regulatory effects on macrophages at the transcription level, which contributed to macrophage polarization towards the anti‐inflammation phenotype in type 2 diabetes treatment (137). Moreover, the apoptosis and efferocytosis of MSCs could induce the alterations of metabolic and inflammatory pathway in alveolar macrophages, thus affecting immunosuppression and reducing disease severity (135). Therefore, the apoptotic and efferocytosis effects of MSCs may play a beneficial role in the treatment of ALI/ARDS by modulating the polarization of macrophage phenotype, and more preclinical and clinical studies are still needed to confirm this idea in the future.
In summary, MSCs may regulate macrophage polarization through mechanisms such as paracrine soluble factors, exosomes, modulation of metabolic reprogramming and mitochondrial transfer, apoptotic and efferocytosis effects (Table 1). The regulation of macrophage polarization by MSCs may be a potential and promising therapy for the treatment of ALI/ARDS.
5 Signaling Pathways Associated With the Regulation of Macrophage Polarization by MSCs
Nuclear Factor-Kappa B (NF-kB) Signaling Pathway
The NF-kB pathway is widely recognized as a pro-inflammatory signaling pathway that participates in host immune response. The activation of inflammatory stimuli such as LPSs leads to the release and nuclear translocation of NF-kB (138, 139). The NF-kB signaling pathway plays an important role in the regulation of macrophage polarization (140, 141).
MSCs can regulate macrophage polarization through NF-kB signaling. Gao et al. demonstrated that co-culturing LPS-induced mice macrophages with MSCs decreased TNF-α expression and enhanced IL-10 and Arg-1 expression; the authors also found that the activity of the NF-kB pathway was inhibited in MSC-treated mice macrophages (142). Wang et al. reported that MSC-derived extracellular vesicles regulated macrophage polarization, attenuated lung injury, and improved pulmonary function by inhibiting the activation of the NF-kB pathway (143). MSCs also attenuated burn-induced ALI by inhibiting the activation of the TLR4/NF-kB signaling pathway via paracrine TSG-6 and transforming macrophages from the pro-inflammatory phenotype to the anti-inflammatory phenotype (144). In a multidrug-resistant Pseudomonas aeruginosa-induced ALI mice model, MSC-derived extracellular vesicles modulated the balance between pro-inflammatory and anti-inflammatory macrophages by inhibiting the activity of the NF-kB signaling pathway, leading to the downregulation of the pro-inflammatory macrophage markers iNOS and IL-12 and the upregulation of the anti-inflammatory macrophage markers Arg-1 and IL-10; these effects reduced the bacterial load and inflammatory response, resulting in decreased mice mortality (145).
Notch Signaling Pathway
Notch signaling is a conserved and important signal transduction pathway that mediates intercellular communication and plays a role in many cell types and different stages of development (146). The Notch signaling pathway is closely associated with inflammation and immune function (147). The Notch signaling pathway also plays a key role in the activation of macrophage polarization. The activation of the Notch signaling pathway promotes pro-inflammatory macrophage polarization and increases the expressions of pro-inflammatory macrophage markers (148–150). Bai et al. found that adipose MSC-derived extracellular vesicles regulated LPS-induced pro-inflammatory polarization by inhibiting the Notch signaling pathway and attenuating sepsis-induced lung injury and inflammatory cell infiltration in lung tissue (151).
Nuclear Factor Erythroid 2-Related Factor 2 (Nrf2)/heme Oxygenase-1 (Ho-1) Signaling Pathway
Nrf2 is a transcription factor that regulates oxidation and inflammation to prevent oxidative damage and modulate apoptosis. Several anti-inflammatory factors can promote the nuclear translocation of Nrf2 and increase the expression of downstream HO-1 protein (152). The activation of the Nrf2/Ho-1 signaling pathway inhibits oxidative stress and inflammatory responses in sepsis-induced organ injury (153). The Nrf2/HO-1 signaling pathway plays a crucial role in regulating macrophage polarization (141, 154, 155). MSCs exert anti-inflammatory effects by activating the Nrf2/HO-1 signaling pathway, promoting anti-inflammatory macrophage polarization, inhibiting oxidative stress, and facilitating lung injury repair, which may be related to the expression of stanniocalcin-2, which has antioxidant properties, in MSCs (71).
JAK/STAT Signaling Pathway
The Janus family of kinases (JAK) is composed of four main members, JAK1, JAK2, JAK3 and Tyk2, which is non-receptor protein tyrosine kinase by nature (156, 157). JAK family kinases can phosphorylate signal transducers and activators of transcription (STATs) to regulate the expression of related genes; this pathway is called the JAK-STAT signaling pathway, The JAK-STAT signaling pathway can modulate cell proliferation, differentiation, apoptosis, and immune function (158). The STAT family includes seven members (STAT1, STAT2, STAT3, STAT4, STAT5A, STAT5B, and STAT6) that play crucial roles in many cellular functions (159, 160). STAT1 is the most important mediator of the IFN-γ-induced polarization of pro-inflammatory macrophages (44, 161, 162). The inhibition of JAK/STAT1 signaling can attenuate the activation of pro-inflammatory macrophages (163–165). STAT3 is an important transcription factor that activates macrophages and increases inflammation (166–168). STAT6 is a major transcription factor for IL-4- or IL-13-mediated signaling and plays an important role in anti-inflammatory macrophage polarization (169). MSCs can modulate macrophage polarization by regulating the JAK/STAT pathway to reduce ulcerative colitis and obesity-associated metabolic disorders (170, 171). MSCs also play an important role in improving ALI/ARDS by regulating macrophage polarization through STAT signaling. Xu et al. found that UC-MSCs promoted anti-inflammatory polarization and T-regulatory cell differentiation to suppress inflammatory responses and reduce LPS-stimulated lung injury (50). Meanwhile, the authors found that transfection with surfactant protein B enhanced the repair of ALI/ARDS by MSCs, which may be related to the regulation of STAT3 signaling (50).
6 Conclusions
In summary, MSCs can regulate macrophage polarization via the paracrine secretion of soluble factors, exosomes, metabolic reprogramming, and mitochondrial transfer to reduce lung inflammation and promote the repair of damaged lung tissue in ALI/ARDS. The regulation of macrophage polarization may become a therapeutic target for ALI/ARDS, thereby providing a new research direction for ALI/ARDS treatment. However, there are still some unresolved issues. For example, the signaling pathways involved in the modulation of macrophage polarization by MSCs are not fully understood, and it is not clear how to prevent lung fibrosis caused by excessive M2 macrophage polarization. Therefore, the treatment of lung injury via the regulation of macrophage polarization requires further study to lay the foundation for clinical application.
In addition, at present, severe acute respiratory syndrome coronavirus 2 (SARS-CoV-2) infection has led to a global pandemic, MSCs therapy seems to be an alternative option to improve inflammation, repair lung tissue damage and prevent long-term pulmonary dysfunction in patients with COVID-19, and cell therapy with reprogrammed macrophages for COVID-19-induced-ARDS may be successful. For example, shifting macrophage polarization from a pro-inflammatory phenotype to an anti-inflammatory phenotype could attenuate the production of pro-inflammatory cytokines, thereby preventing cytokine storm and reducing mortality in patients with COVID-19.
Author Contributions
The first draft of the manuscript was written by CL, and the manuscript was revised by KX and LX. All authors contributed to the article and approved the submitted version.
Funding
This work was supported by China PLA Scientific Key Grant (18CXZ026).
Conflict of Interest
The authors declare that the research was conducted in the absence of any commercial or financial relationships that could be construed as a potential conflict of interest.
Publisher’s Note
All claims expressed in this article are solely those of the authors and do not necessarily represent those of their affiliated organizations, or those of the publisher, the editors and the reviewers. Any product that may be evaluated in this article, or claim that may be made by its manufacturer, is not guaranteed or endorsed by the publisher.
Acknowledgment
The authors thank AiMi Academic Services (www.aimieditor.com) for the English language editing and review services. The authors also thanks Figuredraw (www.figdraw.com) and Biorender (https://biorender.com) for their help in drawing pictures.
References
1. Bernard GR, Artigas A, Brigham KL, Carlet J, Falke K, Hudson L, et al. The American-European Consensus Conference on Ards. Definitions, Mechanisms, Relevant Outcomes, and Clinical Trial Coordination. Am J Respir Crit Care Med (1994) 149(3 Pt 1):818–24. doi: 10.1164/ajrccm.149.3.7509706
2. Ranieri VM, Rubenfeld GD, Thompson BT, Ferguson ND, Caldwell E, Fan E, et al. Acute Respiratory Distress Syndrome: The Berlin Definition. JAMA (2012) 307(23):2526–33. doi: 10.1001/jama.2012.5669
3. Meyer NJ, Gattinoni L, Calfee CS. Acute Respiratory Distress Syndrome. Lancet (2021) 398(10300):622–37. doi: 10.1016/s0140-6736(21)00439-6
4. Swenson KE, Swenson ER. Pathophysiology of Acute Respiratory Distress Syndrome and Covid-19 Lung Injury. Crit Care Clin (2021) 37(4):749–76. doi: 10.1016/j.ccc.2021.05.003
5. Thompson BT, Chambers RC, Liu KD. Acute Respiratory Distress Syndrome. N Engl J Med (2017) 377(6):562–72. doi: 10.1056/NEJMra1608077
6. Kaku S, Nguyen CD, Htet NN, Tutera D, Barr J, Paintal HS, et al. Acute Respiratory Distress Syndrome: Etiology, Pathogenesis, and Summary on Management. J Intensive Care Med (2020) 35(8):723–37. doi: 10.1177/0885066619855021
7. Vassiliou AG, Kotanidou A, Dimopoulou I, Orfanos SE. Endothelial Damage in Acute Respiratory Distress Syndrome. Int J Mol Sci (2020) 21(22):8793. doi: 10.3390/ijms21228793
8. Wilson JG, Calfee CS. Ards Subphenotypes: Understanding a Heterogeneous Syndrome. Crit Care (2020) 24(1):102. doi: 10.1186/s13054-020-2778-x
9. Fan E, Brodie D, Slutsky AS. Acute Respiratory Distress Syndrome: Advances in Diagnosis and Treatment. JAMA (2018) 319(7):698–710. doi: 10.1001/jama.2017.21907
10. Tomazini BM, Maia IS, Cavalcanti AB, Berwanger O, Rosa RG, Veiga VC, et al. Effect of Dexamethasone on Days Alive and Ventilator-Free in Patients With Moderate or Severe Acute Respiratory Distress Syndrome and Covid-19: The Codex Randomized Clinical Trial. JAMA (2020) 324(13):1307–16. doi: 10.1001/jama.2020.17021
11. Vidal-Cortés P, Del Río-Carbajo L, Nieto-Del Olmo J, Prol-Silva E, Tizón-Varela AI, Rodríguez-Vázquez A, et al. Covid-19 and Acute Respiratory Distress Syndrome. Impact of Corticosteroid Treatment and Predictors of Poor Outcome. Rev Esp Quimioter (2021) 34(1):33–43. doi: 10.37201/req/091.2020
12. Spragg RG, Lewis JF, Wurst W, Häfner D, Baughman RP, Wewers MD, et al. Treatment of Acute Respiratory Distress Syndrome With Recombinant Surfactant Protein C Surfactant. Am J Respir Crit Care Med (2003) 167(11):1562–6. doi: 10.1164/rccm.200207-782OC
13. Basabe-Burgos O, Landreh M, Rising A, Curstedt T, Jan J. Treatment of Respiratory Distress Syndrome With Single Recombinant Polypeptides That Combine Features of Sp-B and Sp-C. ACS Chem Biol (2021) 16(12):2864–73. doi: 10.1021/acschembio.1c00816
14. Mohanty RR, Padhy BM, Das S, Meher BR. Therapeutic Potential of N-Acetyl Cysteine (Nac) in Preventing Cytokine Storm in Covid-19: Review of Current Evidence. Eur Rev Med Pharmacol Sci (2021) 25(6):2802–7. doi: 10.26355/eurrev_202103_25442
15. Ghorbi M, Rashidi M, Olapour A, Javaherforooshzadeh F, Akhondzadeh R. Effect of N-Acetylcysteine on the Treatment of Acute Respiratory Distress Syndrome in Mechanically Ventilated Patients Admitted to the Intensive Care Unit. Med J Islam Repub Iran (2021) 35:87. doi: 10.47176/mjiri.35.87
16. Shyamsundar M, McKeown ST, O’Kane CM, Craig TR, Brown V, Thickett DR, et al. Simvastatin Decreases Lipopolysaccharide-Induced Pulmonary Inflammation in Healthy Volunteers. Am J Respir Crit Care Med (2009) 179(12):1107–14. doi: 10.1164/rccm.200810-1584OC
17. Pawlos A, Niedzielski M, Gorzelak-Pabiś P, Broncel M, Woźniak E. Covid-19: Direct and Indirect Mechanisms of Statins. Int J Mol Sci (2021) 22(8):4177. doi: 10.3390/ijms22084177
18. Sriram K, Insel MB, Insel PA. Inhaled B2 Adrenergic Agonists and Other Camp-Elevating Agents: Therapeutics for Alveolar Injury and Acute Respiratory Disease Syndrome? Pharmacol Rev (2021) 73(4):488–526. doi: 10.1124/pharmrev.121.000356
19. Chaves-Cardona H, Hernandez-Torres V, Kiley S, Renew J. Neuromuscular Blockade Management in Patients With Covid-19. Korean J Anesthesiol (2021) 74(4):285–92. doi: 10.4097/kja.21106
20. Naddour M, Kalani M, Ashraf O, Patel K, Bajwa O, Cheema T. Extracorporeal Membrane Oxygenation in Ards. Crit Care Nurs Q (2019) 42(4):400–10. doi: 10.1097/cnq.0000000000000280
21. Lewis SR, Pritchard MW, Thomas CM, Smith AF. Pharmacological Agents for Adults With Acute Respiratory Distress Syndrome. Cochrane Database Syst Rev (2019) 7(7):Cd004477. doi: 10.1002/14651858.CD004477.pub3
22. Hu Q, Hao C, Tang S. From Sepsis to Acute Respiratory Distress Syndrome (Ards): Emerging Preventive Strategies Based on Molecular and Genetic Researches. Biosci Rep (2020) 40(5):BSR20200830. doi: 10.1042/bsr20200830
23. Khan H, Belsher J, Yilmaz M, Afessa B, Winters JL, Moore SB, et al. Fresh-Frozen Plasma and Platelet Transfusions Are Associated With Development of Acute Lung Injury in Critically Ill Medical Patients. Chest (2007) 131(5):1308–14. doi: 10.1378/chest.06-3048
24. Levitt JE, Calfee CS, Goldstein BA, Vojnik R, Matthay MA. Early Acute Lung Injury: Criteria for Identifying Lung Injury Prior to the Need for Positive Pressure Ventilation*. Crit Care Med (2013) 41(8):1929–37. doi: 10.1097/CCM.0b013e31828a3d99
25. Bellani G, Laffey JG, Pham T, Fan E, Brochard L, Esteban A, et al. Epidemiology, Patterns of Care, and Mortality for Patients With Acute Respiratory Distress Syndrome in Intensive Care Units in 50 Countries. JAMA (2016) 315(8):788–800. doi: 10.1001/jama.2016.0291
26. Moss M, Huang DT, Brower RG, Ferguson ND, Ginde AA, Gong MN, et al. Early Neuromuscular Blockade in the Acute Respiratory Distress Syndrome. N Engl J Med (2019) 380(21):1997–2008. doi: 10.1056/NEJMoa1901686
27. Sasannejad C, Ely EW, Lahiri S. Long-Term Cognitive Impairment After Acute Respiratory Distress Syndrome: A Review of Clinical Impact and Pathophysiological Mechanisms. Crit Care (2019) 23(1):352. doi: 10.1186/s13054-019-2626-z
28. Curley GF, Contreras M, Higgins B, O’Kane C, McAuley DF, O’Toole D, et al. Evolution of the Inflammatory and Fibroproliferative Responses During Resolution and Repair After Ventilator-Induced Lung Injury in the Rat. Anesthesiology (2011) 115(5):1022–32. doi: 10.1097/ALN.0b013e31823422c9
29. Cabrera-Benítez NE, Parotto M, Post M, Han B, Spieth PM, Cheng WE, et al. Mechanical Stress Induces Lung Fibrosis by Epithelial-Mesenchymal Transition. Crit Care Med (2012) 40(2):510–7. doi: 10.1097/CCM.0b013e31822f09d7
30. Cabrera-Benitez NE, Laffey JG, Parotto M, Spieth PM, Villar J, Zhang H, et al. Mechanical Ventilation-Associated Lung Fibrosis in Acute Respiratory Distress Syndrome: A Significant Contributor to Poor Outcome. Anesthesiology (2014) 121(1):189–98. doi: 10.1097/aln.0000000000000264
31. Zhang R, Pan Y, Fanelli V, Wu S, Luo AA, Islam D, et al. Mechanical Stress and the Induction of Lung Fibrosis Via the Midkine Signaling Pathway. Am J Respir Crit Care Med (2015) 192(3):315–23. doi: 10.1164/rccm.201412-2326OC
32. Klompas M. Ventilator-Associated Events: What They Are and What They Are Not. Respir Care (2019) 64(8):953–61. doi: 10.4187/respcare.07059
33. Li HH, Wang CW, Chang CH, Huang CC, Hsu HS, Chiu LC. Relationship Between Mechanical Ventilation and Histological Fibrosis in Patients With Acute Respiratory Distress Syndrome Undergoing Open Lung Biopsy. J Pers Med (2022) 12(3):474. doi: 10.3390/jpm12030474
34. Brown C, McKee C, Bakshi S, Walker K, Hakman E, Halassy S, et al. Mesenchymal Stem Cells: Cell Therapy and Regeneration Potential. J Tissue Eng Regener Med (2019) 13(9):1738–55. doi: 10.1002/term.2914
35. Maqsood M, Kang M, Wu X, Chen J, Teng L, Qiu L. Adult Mesenchymal Stem Cells and Their Exosomes: Sources, Characteristics, and Application in Regenerative Medicine. Life Sci (2020) 256:118002. doi: 10.1016/j.lfs.2020.118002
36. Laffey JG, Matthay MA. Fifty Years of Research in Ards. Cell-Based Therapy for Acute Respiratory Distress Syndrome. Biology and Potential Therapeutic Value. Am J Respir Crit Care Med (2017) 196(3):266–73. doi: 10.1164/rccm.201701-0107CP
37. Lu Z, Chang W, Meng S, Xu X, Xie J, Guo F, et al. Mesenchymal Stem Cells Induce Dendritic Cell Immune Tolerance Via Paracrine Hepatocyte Growth Factor to Alleviate Acute Lung Injury. Stem Cell Res Ther (2019) 10(1):372. doi: 10.1186/s13287-019-1488-2
38. Wick KD, Leligdowicz A, Zhuo H, Ware LB, Matthay MA. Mesenchymal Stromal Cells Reduce Evidence of Lung Injury in Patients With Ards. JCI Insight (2021) 6(12):e148983. doi: 10.1172/jci.insight.148983
39. Dos Santos CC, Amatullah H, Vaswani CM, Maron-Gutierrez T, Kim M, Mei SHJ, et al. Mesenchymal Stromal (Stem) Cell Therapy Modulates Mir-193b-5p Expression to Attenuate Sepsis-Induced Acute Lung Injury. Eur Respir J (2022) 59(1):2004216. doi: 10.1183/13993003.04216-2020
40. Byrne AJ, Mathie SA, Gregory LG, Lloyd CM. Pulmonary Macrophages: Key Players in the Innate Defence of the Airways. Thorax (2015) 70(12):1189–96. doi: 10.1136/thoraxjnl-2015-207020
41. Allard B, Panariti A, Martin JG. Alveolar Macrophages in the Resolution of Inflammation, Tissue Repair, and Tolerance to Infection. Front Immunol (2018) 9:1777. doi: 10.3389/fimmu.2018.01777
42. Woo YD, Jeong D, Chung DH. Development and Functions of Alveolar Macrophages. Mol Cells (2021) 44(5):292–300. doi: 10.14348/molcells.2021.0058
43. Martinez FO, Gordon S. The M1 and M2 Paradigm of Macrophage Activation: Time for Reassessment. F1000Prime Rep (2014) 6:13. doi: 10.12703/p6-13
44. Murray PJ, Allen JE, Biswas SK, Fisher EA, Gilroy DW, Goerdt S, et al. Macrophage Activation and Polarization: Nomenclature and Experimental Guidelines. Immunity (2014) 41(1):14–20. doi: 10.1016/j.immuni.2014.06.008
45. Sica A, Mantovani A. Macrophage Plasticity and Polarization: In Vivo Veritas. J Clin Invest (2012) 122(3):787–95. doi: 10.1172/jci59643
46. Zhao J, Li X, Hu J, Chen F, Qiao S, Sun X, et al. Mesenchymal Stromal Cell-Derived Exosomes Attenuate Myocardial Ischaemia-Reperfusion Injury Through Mir-182-Regulated Macrophage Polarization. Cardiovasc Res (2019) 115(7):1205–16. doi: 10.1093/cvr/cvz040
47. Liu W, Yu M, Xie D, Wang L, Ye C, Zhu Q, et al. Melatonin-Stimulated Msc-Derived Exosomes Improve Diabetic Wound Healing Through Regulating Macrophage M1 and M2 Polarization by Targeting the Pten/Akt Pathway. Stem Cell Res Ther (2020) 11(1):259. doi: 10.1186/s13287-020-01756-x
48. Porzionato A, Zaramella P, Dedja A, Guidolin D, Bonadies L, Macchi V, et al. Intratracheal Administration of Mesenchymal Stem Cell-Derived Extracellular Vesicles Reduces Lung Injuries in a Chronic Rat Model of Bronchopulmonary Dysplasia. Am J Physiol Lung Cell Mol Physiol (2021) 320(5):L688–l704. doi: 10.1152/ajplung.00148.2020
49. Lv H, Yuan X, Zhang J, Lu T, Yao J, Zheng J, et al. Heat Shock Preconditioning Mesenchymal Stem Cells Attenuate Acute Lung Injury Via Reducing Nlrp3 Inflammasome Activation in Macrophages. Stem Cell Res Ther (2021) 12(1):290. doi: 10.1186/s13287-021-02328-3
50. Xu H, Nie G, Yin T, Shao C, Ding D, Zou M. Umbilical Cord-Derived Mesenchymal Stem Cells With Surfactant Protein B Alleviates Inflammatory Response in Acute Respiratory Distress Syndrome by Regulating Macrophage Polarization. Balkan Med J (2022) 39(1):130–9. doi: 10.4274/balkanmedj.galenos.2021.2021-9-8
51. Aggarwal NR, King LS, D’Alessio FR. Diverse Macrophage Populations Mediate Acute Lung Inflammation and Resolution. Am J Physiol Lung Cell Mol Physiol (2014) 306(8):L709–25. doi: 10.1152/ajplung.00341.2013
52. Huang X, Xiu H, Zhang S, Zhang G. The Role of Macrophages in the Pathogenesis of Ali/Ards. Mediators Inflammation (2018) 2018:1264913. doi: 10.1155/2018/1264913
53. Shapouri-Moghaddam A, Mohammadian S, Vazini H, Taghadosi M, Esmaeili SA, Mardani F, et al. Macrophage Plasticity, Polarization, and Function in Health and Disease. J Cell Physiol (2018) 233(9):6425–40. doi: 10.1002/jcp.26429
54. Mantovani A, Sica A, Sozzani S, Allavena P, Vecchi A, Locati M. The Chemokine System in Diverse Forms of Macrophage Activation and Polarization. Trends Immunol (2004) 25(12):677–86. doi: 10.1016/j.it.2004.09.015
55. Rőszer T. Understanding the Mysterious M2 Macrophage Through Activation Markers and Effector Mechanisms. Mediators Inflammation (2015) 2015:816460. doi: 10.1155/2015/816460
56. Anders CB, Lawton TMW, Smith HL, Garret J, Doucette MM, Ammons MCB. Use of Integrated Metabolomics, Transcriptomics, and Signal Protein Profile to Characterize the Effector Function and Associated Metabotype of Polarized Macrophage Phenotypes. J Leukoc Biol (2022) 111(3):667–93. doi: 10.1002/jlb.6a1120-744r
57. Duluc D, Delneste Y, Tan F, Moles MP, Grimaud L, Lenoir J, et al. Tumor-Associated Leukemia Inhibitory Factor and Il-6 Skew Monocyte Differentiation Into Tumor-Associated Macrophage-Like Cells. Blood (2007) 110(13):4319–30. doi: 10.1182/blood-2007-02-072587
58. Ferrante CJ, Pinhal-Enfield G, Elson G, Cronstein BN, Hasko G, Outram S, et al. The Adenosine-Dependent Angiogenic Switch of Macrophages to an M2-Like Phenotype Is Independent of Interleukin-4 Receptor Alpha (Il-4rα) Signaling. Inflammation (2013) 36(4):921–31. doi: 10.1007/s10753-013-9621-3
59. Wang Q, Ni H, Lan L, Wei X, Xiang R, Wang Y. Fra-1 Protooncogene Regulates Il-6 Expression in Macrophages and Promotes the Generation of M2d Macrophages. Cell Res (2010) 20(6):701–12. doi: 10.1038/cr.2010.52
60. Schif-Zuck S, Gross N, Assi S, Rostoker R, Serhan CN, Ariel A. Saturated-Efferocytosis Generates Pro-Resolving Cd11b Low Macrophages: Modulation by Resolvins and Glucocorticoids. Eur J Immunol (2011) 41(2):366–79. doi: 10.1002/eji.201040801
61. Michaeli S, Dakwar V, Weidenfeld K, Granski O, Gilon O, Schif-Zuck S, et al. Soluble Mediators Produced by Pro-Resolving Macrophages Inhibit Angiogenesis. Front Immunol (2018) 9:768. doi: 10.3389/fimmu.2018.00768
62. Xue J, Schmidt SV, Sander J, Draffehn A, Krebs W, Quester I, et al. Transcriptome-Based Network Analysis Reveals a Spectrum Model of Human Macrophage Activation. Immunity (2014) 40(2):274–88. doi: 10.1016/j.immuni.2014.01.006
63. Deng H, Wu L, Liu M, Zhu L, Chen Y, Zhou H, et al. Bone Marrow Mesenchymal Stem Cell-Derived Exosomes Attenuate Lps-Induced Ards by Modulating Macrophage Polarization Through Inhibiting Glycolysis in Macrophages. Shock (2020) 54(6):828–43. doi: 10.1097/shk.0000000000001549
64. Khan MJ, Singh P, Dohare R, Jha R, Rahmani AH, Almatroodi SA, et al. Inhibition of Mirna-34a Promotes M2 Macrophage Polarization and Improves Lps-Induced Lung Injury by Targeting Klf4. Genes (Basel) (2020) 11(9):966. doi: 10.3390/genes11090966
65. Chen X, Tang J, Shuai W, Meng J, Feng J, Han Z. Macrophage Polarization and Its Role in the Pathogenesis of Acute Lung Injury/Acute Respiratory Distress Syndrome. Inflammation Res (2020) 69(9):883–95. doi: 10.1007/s00011-020-01378-2
66. Lee JW, Chun W, Lee HJ, Min JH, Kim SM, Seo JY, et al. The Role of Macrophages in the Development of Acute and Chronic Inflammatory Lung Diseases. Cells (2021) 10(4):897. doi: 10.3390/cells10040897
67. Li S, Qi D, Li JN, Deng XY, Wang DX. Vagus Nerve Stimulation Enhances the Cholinergic Anti-Inflammatory Pathway to Reduce Lung Injury in Acute Respiratory Distress Syndrome Via Stat3. Cell Death Discovery (2021) 7(1):63. doi: 10.1038/s41420-021-00431-1
68. Cheng P, Li S, Chen H. Macrophages in Lung Injury, Repair, and Fibrosis. Cells (2021) 10(2):436. doi: 10.3390/cells10020436
69. Wynn TA, Vannella KM. Macrophages in Tissue Repair, Regeneration, and Fibrosis. Immunity (2016) 44(3):450–62. doi: 10.1016/j.immuni.2016.02.015
70. Sadeghi S, Mosaffa N, Hashemi SM, Mehdi Naghizadeh M, Ghazanfari T. The Immunomodulatory Effects of Mesenchymal Stem Cells on Long Term Pulmonary Complications in an Animal Model Exposed to a Sulfur Mustard Analog. Int Immunopharmacol (2020) 80:105879. doi: 10.1016/j.intimp.2019.105879
71. Lv H, Liu Q, Sun Y, Yi X, Wei X, Liu W, et al. Mesenchymal Stromal Cells Ameliorate Acute Lung Injury Induced by Lps Mainly Through Stanniocalcin-2 Mediating Macrophage Polarization. Ann Transl Med (2020) 8(6):334. doi: 10.21037/atm.2020.02.105
72. Chen X, Yang B, Tian J, Hong H, Du Y, Li K, et al. Dental Follicle Stem Cells Ameliorate Lipopolysaccharide-Induced Inflammation by Secreting Tgf-B3 and Tsp-1 to Elicit Macrophage M2 Polarization. Cell Physiol Biochem (2018) 51(5):2290–308. doi: 10.1159/000495873
73. Morrison TJ, Jackson MV, Cunningham EK, Kissenpfennig A, McAuley DF, O’Kane CM, et al. Mesenchymal Stromal Cells Modulate Macrophages in Clinically Relevant Lung Injury Models by Extracellular Vesicle Mitochondrial Transfer. Am J Respir Crit Care Med (2017) 196(10):1275–86. doi: 10.1164/rccm.201701-0170OC
74. Shao Y, Zhou F, He D, Zhang L, Shen J. Overexpression of Cxcr7 Promotes Mesenchymal Stem Cells to Repair Phosgene-Induced Acute Lung Injury in Rats. BioMed Pharmacother (2019) 109:1233–9. doi: 10.1016/j.biopha.2018.10.108
75. Eggenhofer E, Luk F, Dahlke MH, Hoogduijn MJ. The Life and Fate of Mesenchymal Stem Cells. Front Immunol (2014) 5:148. doi: 10.3389/fimmu.2014.00148
76. De Becker A, Riet IV. Homing and Migration of Mesenchymal Stromal Cells: How to Improve the Efficacy of Cell Therapy? World J Stem Cells (2016) 8(3):73–87. doi: 10.4252/wjsc.v8.i3.73
77. Li L, Chen X, Wang WE, Zeng C. How to Improve the Survival of Transplanted Mesenchymal Stem Cell in Ischemic Heart? Stem Cells Int (2016) 2016:9682757. doi: 10.1155/2016/9682757
78. Li D, Liu Q, Qi L, Dai X, Liu H, Wang Y. Low Levels of Tgf-B1 Enhance Human Umbilical Cord-Derived Mesenchymal Stem Cell Fibronectin Production and Extend Survival Time in a Rat Model of Lipopolysaccharide-Induced Acute Lung Injury. Mol Med Rep (2016) 14(2):1681–92. doi: 10.3892/mmr.2016.5416
79. Zhao L, Hu C, Zhang P, Jiang H, Chen J. Preconditioning Strategies for Improving the Survival Rate and Paracrine Ability of Mesenchymal Stem Cells in Acute Kidney Injury. J Cell Mol Med (2019) 23(2):720–30. doi: 10.1111/jcmm.14035
80. Alvites R, Branquinho M, Sousa AC, Lopes B, Sousa P, Maurício AC. Mesenchymal Stem/Stromal Cells and Their Paracrine Activity-Immunomodulation Mechanisms and How to Influence the Therapeutic Potential. Pharmaceutics (2022) 14(2):381. doi: 10.3390/pharmaceutics14020381
81. Fu X, Liu G, Halim A, Ju Y, Luo Q, Song AG. Mesenchymal Stem Cell Migration and Tissue Repair. Cells (2019) 8(8):784. doi: 10.3390/cells8080784
82. Wakayama H, Hashimoto N, Matsushita Y, Matsubara K, Yamamoto N, Hasegawa Y, et al. Factors Secreted From Dental Pulp Stem Cells Show Multifaceted Benefits for Treating Acute Lung Injury in Mice. Cytotherapy (2015) 17(8):1119–29. doi: 10.1016/j.jcyt.2015.04.009
83. Kwon JH, Kim M, Bae YK, Kim GH, Choi SJ, Oh W, et al. Decorin Secreted by Human Umbilical Cord Blood-Derived Mesenchymal Stem Cells Induces Macrophage Polarization Via Cd44 to Repair Hyperoxic Lung Injury. Int J Mol Sci (2019) 20(19):4815. doi: 10.3390/ijms20194815
84. Kim M, Kwon JH, Bae YK, Kim GH, Um S, Ha J, et al. Soluble Ptx3 of Human Umbilical Cord Blood-Derived Mesenchymal Stem Cells Attenuates Hyperoxic Lung Injury by Activating Macrophage Polarization in Neonatal Rat Model. Stem Cells Int (2020) 2020:1802976. doi: 10.1155/2020/1802976
85. Ionescu L, Byrne RN, van Haaften T, Vadivel A, Alphonse RS, Rey-Parra GJ, et al. Stem Cell Conditioned Medium Improves Acute Lung Injury in Mice: In Vivo Evidence for Stem Cell Paracrine Action. Am J Physiol Lung Cell Mol Physiol (2012) 303(11):L967–77. doi: 10.1152/ajplung.00144.2011
86. Németh K, Leelahavanichkul A, Yuen PS, Mayer B, Parmelee A, Doi K, et al. Bone Marrow Stromal Cells Attenuate Sepsis Via Prostaglandin E(2)-Dependent Reprogramming of Host Macrophages to Increase Their Interleukin-10 Production. Nat Med (2009) 15(1):42–9. doi: 10.1038/nm.1905
87. Zhao Y, Yu D, Wang H, Jin W, Li X, Hu Y, et al. Galectin-9 Mediates the Therapeutic Effect of Mesenchymal Stem Cells on Experimental Endotoxemia. Front Cell Dev Biol (2022) 10:700702. doi: 10.3389/fcell.2022.700702
88. Huang Q, Cheng X, Luo C, Yang S, Li S, Wang B, et al. Placental Chorionic Plate-Derived Mesenchymal Stem Cells Ameliorate Severe Acute Pancreatitis by Regulating Macrophage Polarization Via Secreting Tsg-6. Stem Cell Res Ther (2021) 12(1):337. doi: 10.1186/s13287-021-02411-9
89. Kang JY, Oh MK, Joo H, Park HS, Chae DH, Kim J, et al. Xeno-Free Condition Enhances Therapeutic Functions of Human Wharton’s Jelly-Derived Mesenchymal Stem Cells Against Experimental Colitis by Upregulated Indoleamine 2,3-Dioxygenase Activity. J Clin Med (2020) 9(9):2913. doi: 10.3390/jcm9092913
90. Ortiz-Virumbrales M, Menta R, Pérez LM, Lucchesi O, Mancheño-Corvo P, Avivar-Valderas Á, et al. Human Adipose Mesenchymal Stem Cells Modulate Myeloid Cells Toward an Anti-Inflammatory and Reparative Phenotype: Role of Il-6 and Pge2. Stem Cell Res Ther (2020) 11(1):462. doi: 10.1186/s13287-020-01975-2
91. Raposo G, Stoorvogel W. Extracellular Vesicles: Exosomes, Microvesicles, and Friends. J Cell Biol (2013) 200(4):373–83. doi: 10.1083/jcb.201211138
92. Yáñez-Mó M, Siljander PR, Andreu Z, Zavec AB, Borràs FE, Buzas EI, et al. Biological Properties of Extracellular Vesicles and Their Physiological Functions. J Extracell Vesicles (2015) 4:27066. doi: 10.3402/jev.v4.27066
93. Iraci N, Leonardi T, Gessler F, Vega B, Pluchino S. Focus on Extracellular Vesicles: Physiological Role and Signalling Properties of Extracellular Membrane Vesicles. Int J Mol Sci (2016) 17(2):171. doi: 10.3390/ijms17020171
94. van Niel G, D’Angelo G, Raposo G. Shedding Light on the Cell Biology of Extracellular Vesicles. Nat Rev Mol Cell Biol (2018) 19(4):213–28. doi: 10.1038/nrm.2017.125
95. Huotari J, Helenius A. Endosome Maturation. EMBO J (2011) 30(17):3481–500. doi: 10.1038/emboj.2011.286
96. Lai RC, Yeo RW, Lim SK. Mesenchymal Stem Cell Exosomes. Semin Cell Dev Biol (2015) 40:82–8. doi: 10.1016/j.semcdb.2015.03.001
97. Hanson PI, Cashikar A. Multivesicular Body Morphogenesis. Annu Rev Cell Dev Biol (2012) 28:337–62. doi: 10.1146/annurev-cellbio-092910-154152
98. Diaz G, Bridges C, Lucas M, Cheng Y, Schorey JS, Dobos KM, et al. Protein Digestion, Ultrafiltration, and Size Exclusion Chromatography to Optimize the Isolation of Exosomes From Human Blood Plasma and Serum. J Vis Exp (2018) (134):5767. doi: 10.3791/57467
99. Elsharkawi F, Elsabah M, Shabayek M, Khaled H. Urine and Serum Exosomes as Novel Biomarkers in Detection of Bladder Cancer. Asian Pac J Cancer Prev (2019) 20(7):2219–24. doi: 10.31557/apjcp.2019.20.7.2219
100. Zhu L, Chen Y, Chen M, Wang W. Mechanism of Mir-204-5p in Exosomes Derived From Bronchoalveolar Lavage Fluid on the Progression of Pulmonary Fibrosis Via Ap1s2. Ann Transl Med (2021) 9(13):1068. doi: 10.21037/atm-20-8033
101. Miyake H, Lee C, Chusilp S, Bhalla M, Li B, Pitino M, et al. Human Breast Milk Exosomes Attenuate Intestinal Damage. Pediatr Surg Int (2020) 36(2):155–63. doi: 10.1007/s00383-019-04599-7
102. Michael A, Bajracharya SD, Yuen PS, Zhou H, Star RA, Illei GG, et al. Exosomes From Human Saliva as a Source of Microrna Biomarkers. Oral Dis (2010) 16(1):34–8. doi: 10.1111/j.1601-0825.2009.01604.x
103. Inubushi S, Kawaguchi H, Mizumoto S, Kunihisa T, Baba M, Kitayama Y, et al. Oncogenic Mirnas Identified in Tear Exosomes From Metastatic Breast Cancer Patients. Anticancer Res (2020) 40(6):3091–6. doi: 10.21873/anticanres.14290
104. Menay F, Herschlik L, De Toro J, Cocozza F, Tsacalian R, Gravisaco MJ, et al. Exosomes Isolated From Ascites of T-Cell Lymphoma-Bearing Mice Expressing Surface Cd24 and Hsp-90 Induce a Tumor-Specific Immune Response. Front Immunol (2017) 8:286. doi: 10.3389/fimmu.2017.00286
105. Kourembanas S. Exosomes: Vehicles of Intercellular Signaling, Biomarkers, and Vectors of Cell Therapy. Annu Rev Physiol (2015) 77:13–27. doi: 10.1146/annurev-physiol-021014-071641
106. Poliakov A, Spilman M, Dokland T, Amling CL, Mobley JA. Structural Heterogeneity and Protein Composition of Exosome-Like Vesicles (Prostasomes) in Human Semen. Prostate (2009) 69(2):159–67. doi: 10.1002/pros.20860
107. Simpson RJ, Jensen SS, Lim JW. Proteomic Profiling of Exosomes: Current Perspectives. Proteomics (2008) 8(19):4083–99. doi: 10.1002/pmic.200800109
108. Huang-Doran I, Zhang CY, Vidal-Puig A. Extracellular Vesicles: Novel Mediators of Cell Communication in Metabolic Disease. Trends Endocrinol Metab (2017) 28(1):3–18. doi: 10.1016/j.tem.2016.10.003
109. Valadi H, Ekström K, Bossios A, Sjöstrand M, Lee JJ, Lötvall JO. Exosome-Mediated Transfer of Mrnas and Micrornas Is a Novel Mechanism of Genetic Exchange Between Cells. Nat Cell Biol (2007) 9(6):654–9. doi: 10.1038/ncb1596
110. van den Boorn JG, Dassler J, Coch C, Schlee M, Hartmann G. Exosomes as Nucleic Acid Nanocarriers. Adv Drug Delivery Rev (2013) 65(3):331–5. doi: 10.1016/j.addr.2012.06.011
111. Alexander M, Hu R, Runtsch MC, Kagele DA, Mosbruger TL, Tolmachova T, et al. Exosome-Delivered Micrornas Modulate the Inflammatory Response to Endotoxin. Nat Commun (2015) 6:7321. doi: 10.1038/ncomms8321
112. Cheng C, Chen X, Wang Y, Cheng W, Zuo X, Tang W, et al. Mscs−Derived Exosomes Attenuate Ischemia-Reperfusion Brain Injury and Inhibit Microglia Apoptosis Might Via Exosomal Mir-26a-5p Mediated Suppression of Cdk6. Mol Med (2021) 27(1):67. doi: 10.1186/s10020-021-00324-0
113. Chen S, Zhou H, Zhang B, Hu Q. Exosomal Mir-512-3p Derived From Mesenchymal Stem Cells Inhibits Oxidized Low-Density Lipoprotein-Induced Vascular Endothelial Cells Dysfunction Via Regulating Keap1. J Biochem Mol Toxicol (2021) 35(6):1–11. doi: 10.1002/jbt.22767
114. Tian J, Cui X, Sun J, Zhang J. Exosomal Microrna-16-5p From Adipose Mesenchymal Stem Cells Promotes Tlr4-Mediated M2 Macrophage Polarization in Septic Lung Injury. Int Immunopharmacol (2021) 98:107835. doi: 10.1016/j.intimp.2021.107835
115. Song Y, Dou H, Li X, Zhao X, Li Y, Liu D, et al. Exosomal Mir-146a Contributes to the Enhanced Therapeutic Efficacy of Interleukin-1β-Primed Mesenchymal Stem Cells Against Sepsis. Stem Cells (2017) 35(5):1208–21. doi: 10.1002/stem.2564
116. Bao P, Zhao W, Mou M, Liu X. Microrna-21 Mediates Bone Marrow Mesenchymal Stem Cells Protection of Radiation-Induced Lung Injury During the Acute Phase by Regulating Polarization of Alveolar Macrophages. Transl Cancer Res (2020) 9(1):231–9. doi: 10.21037/tcr.2019.12.77
117. Li JW, Wei L, Han Z, Chen Z. Mesenchymal Stromal Cells-Derived Exosomes Alleviate Ischemia/Reperfusion Injury in Mouse Lung by Transporting Anti-Apoptotic Mir-21-5p. Eur J Pharmacol (2019) 852:68–76. doi: 10.1016/j.ejphar.2019.01.022
118. Zhu L, Zhao Q, Yang T, Ding W, Zhao Y. Cellular Metabolism and Macrophage Functional Polarization. Int Rev Immunol (2015) 34(1):82–100. doi: 10.3109/08830185.2014.969421
119. Boscá L, González-Ramos S, Prieto P, Fernández-Velasco M, Mojena M, Martín-Sanz P, et al. Metabolic Signatures Linked to Macrophage Polarization: From Glucose Metabolism to Oxidative Phosphorylation. Biochem Soc Trans (2015) 43(4):740–4. doi: 10.1042/bst20150107
120. Mouton AJ, Li X, Hall ME, Hall JE. Obesity, Hypertension, and Cardiac Dysfunction: Novel Roles of Immunometabolism in Macrophage Activation and Inflammation. Circ Res (2020) 126(6):789–806. doi: 10.1161/circresaha.119.312321
121. Luque-Campos N, Bustamante-Barrientos FA, Pradenas C, García C, Araya MJ, Bohaud C, et al. The Macrophage Response Is Driven by Mesenchymal Stem Cell-Mediated Metabolic Reprogramming. Front Immunol (2021) 12:624746. doi: 10.3389/fimmu.2021.624746
122. Selleri S, Bifsha P, Civini S, Pacelli C, Dieng MM, Lemieux W, et al. Human Mesenchymal Stromal Cell-Secreted Lactate Induces M2-Macrophage Differentiation by Metabolic Reprogramming. Oncotarget (2016) 7(21):30193–210. doi: 10.18632/oncotarget.8623
123. Liu X, Gao C, Wang Y, Niu L, Jiang S, Pan S. Bmsc-Derived Exosomes Ameliorate Lps-Induced Acute Lung Injury by Mir-384-5p-Controlled Alveolar Macrophage Autophagy. Oxid Med Cell Longev (2021) 2021:9973457. doi: 10.1155/2021/9973457
124. Dutra Silva J, Su Y, Calfee CS, Delucchi KL, Weiss D, McAuley DF, et al. Mesenchymal Stromal Cell Extracellular Vesicles Rescue Mitochondrial Dysfunction and Improve Barrier Integrity in Clinically Relevant Models of Ards. Eur Respir J (2021) 58(1):2002978. doi: 10.1183/13993003.02978-2020
125. Islam MN, Das SR, Emin MT, Wei M, Sun L, Westphalen K, et al. Mitochondrial Transfer From Bone-Marrow-Derived Stromal Cells to Pulmonary Alveoli Protects Against Acute Lung Injury. Nat Med (2012) 18(5):759–65. doi: 10.1038/nm.2736
126. Jackson MV, Morrison TJ, Doherty DF, McAuley DF, Matthay MA, Kissenpfennig A, et al. Mitochondrial Transfer Via Tunneling Nanotubes Is an Important Mechanism by Which Mesenchymal Stem Cells Enhance Macrophage Phagocytosis in the in Vitro and in Vivo Models of Ards. Stem Cells (2016) 34(8):2210–23. doi: 10.1002/stem.2372
127. Xia L, Zhang C, Lv N, Liang Z, Ma T, Cheng H, et al. Admsc-Derived Exosomes Alleviate Acute Lung Injury Via Transferring Mitochondrial Component to Improve Homeostasis of Alveolar Macrophages. Theranostics (2022) 12(6):2928–47. doi: 10.7150/thno.69533
128. Thum T, Bauersachs J, Poole-Wilson PA, Volk HD, Anker SD. The Dying Stem Cell Hypothesis: Immune Modulation as a Novel Mechanism for Progenitor Cell Therapy in Cardiac Muscle. J Am Coll Cardiol (2005) 46(10):1799–802. doi: 10.1016/j.jacc.2005.07.053
129. Liu FB, Lin Q, Liu ZW. A Study on the Role of Apoptotic Human Umbilical Cord Mesenchymal Stem Cells in Bleomycin-Induced Acute Lung Injury in Rat Models. Eur Rev Med Pharmacol Sci (2016) 20(5):969–82. doi: 10.26355/eurrev_201605_12438
130. Sung PH, Chang CL, Tsai TH, Chang LT, Leu S, Chen YL, et al. Apoptotic Adipose-Derived Mesenchymal Stem Cell Therapy Protects Against Lung and Kidney Injury in Sepsis Syndrome Caused by Cecal Ligation Puncture in Rats. Stem Cell Res Ther (2013) 4(6):155. doi: 10.1186/scrt385
131. Laing AG, Riffo-Vasquez Y, Sharif-Paghaleh E, Lombardi G, Sharpe PT. Immune Modulation by Apoptotic Dental Pulp Stem Cells in Vivo. Immunotherapy (2018) 10(3):201–11. doi: 10.2217/imt-2017-0117
132. Cheung TS, Galleu A, von Bonin M, Bornhäuser M, Dazzi F. Apoptotic Mesenchymal Stromal Cells Induce Prostaglandin E2 in Monocytes: Implications for the Monitoring of Mesenchymal Stromal Cell Activity. Haematologica (2019) 104(10):e438–e41. doi: 10.3324/haematol.2018.214767
133. de Witte SFH, Luk F, Sierra Parraga JM, Gargesha M, Merino A, Korevaar SS, et al. Immunomodulation by Therapeutic Mesenchymal Stromal Cells (Msc) Is Triggered Through Phagocytosis of Msc by Monocytic Cells. Stem Cells (2018) 36(4):602–15. doi: 10.1002/stem.2779
134. Galleu A, Riffo-Vasquez Y, Trento C, Lomas C, Dolcetti L, Cheung TS, et al. Apoptosis in Mesenchymal Stromal Cells Induces in Vivo Recipient-Mediated Immunomodulation. Sci Transl Med (2017) 9(416):eaam7828. doi: 10.1126/scitranslmed.aam7828
135. Pang SHM, D’Rozario J, Mendonca S, Bhuvan T, Payne NL, Zheng D, et al. Mesenchymal Stromal Cell Apoptosis Is Required for Their Therapeutic Function. Nat Commun (2021) 12(1):6495. doi: 10.1038/s41467-021-26834-3
136. Ghahremani Piraghaj M, Soudi S, Ghanbarian H, Bolandi Z, Namaki S, Hashemi SM. Effect of Efferocytosis of Apoptotic Mesenchymal Stem Cells (Mscs) on C57bl/6 Peritoneal Macrophages Function. Life Sci (2018) 212:203–12. doi: 10.1016/j.lfs.2018.09.052
137. Zheng C, Sui B, Zhang X, Hu J, Chen J, Liu J, et al. Apoptotic Vesicles Restore Liver Macrophage Homeostasis to Counteract Type 2 Diabetes. J Extracell Vesicles (2021) 10(7):e12109. doi: 10.1002/jev2.12109
138. Lawrence T. The Nuclear Factor Nf-Kappab Pathway in Inflammation. Cold Spring Harb Perspect Biol (2009) 1(6):a001651. doi: 10.1101/cshperspect.a001651
139. Alharbi KS, Fuloria NK, Fuloria S, Rahman SB, Al-Malki WH, Javed Shaikh MA, et al. Nuclear Factor-Kappa B and Its Role in Inflammatory Lung Disease. Chem Biol Interact (2021) 345:109568. doi: 10.1016/j.cbi.2021.109568
140. Zhang J, Zheng Y, Luo Y, Du Y, Zhang X, Fu J. Curcumin Inhibits Lps-Induced Neuroinflammation by Promoting Microglial M2 Polarization Via Trem2/Tlr4/Nf-Kb Pathways in Bv2 Cells. Mol Immunol (2019) 116:29–37. doi: 10.1016/j.molimm.2019.09.020
141. Ning H, Chen H, Deng J, Xiao C, Xu M, Shan L, et al. Exosomes Secreted by Fndc5-Bmmscs Protect Myocardial Infarction by Anti-Inflammation and Macrophage Polarization Via Nf-Kb Signaling Pathway and Nrf2/Ho-1 Axis. Stem Cell Res Ther (2021) 12(1):519. doi: 10.1186/s13287-021-02591-4
142. Gao S, Mao F, Zhang B, Zhang L, Zhang X, Wang M, et al. Mouse Bone Marrow-Derived Mesenchymal Stem Cells Induce Macrophage M2 Polarization Through the Nuclear Factor-Kb and Signal Transducer and Activator of Transcription 3 Pathways. Exp Biol Med (Maywood) (2014) 239(3):366–75. doi: 10.1177/1535370213518169
143. Wang J, Huang R, Xu Q, Zheng G, Qiu G, Ge M, et al. Mesenchymal Stem Cell-Derived Extracellular Vesicles Alleviate Acute Lung Injury Via Transfer of Mir-27a-3p. Crit Care Med (2020) 48(7):e599–610. doi: 10.1097/ccm.0000000000004315
144. Hu X, Liu L, Wang Y, Yu Y, Li Z, Liu Y, et al. Human Umbilical Cord-Derived Mesenchymal Stem Cells Alleviate Acute Lung Injury Caused by Severe Burn Via Secreting Tsg-6 and Inhibiting Inflammatory Response. Stem Cells Int (2022) 2022:8661689. doi: 10.1155/2022/8661689
145. Shi MM, Zhu YG, Yan JY, Rouby JJ, Summah H, Monsel A, et al. Role of Mir-466 in Mesenchymal Stromal Cell Derived Extracellular Vesicles Treating Inoculation Pneumonia Caused by Multidrug-Resistant Pseudomonas Aeruginosa. Clin Transl Med (2021) 11(1):e287. doi: 10.1002/ctm2.287
146. Artavanis-Tsakonas S, Rand MD, Lake RJ. Notch Signaling: Cell Fate Control and Signal Integration in Development. Science (1999) 284(5415):770–6. doi: 10.1126/science.284.5415.770
147. Shang Y, Smith S, Hu X. Role of Notch Signaling in Regulating Innate Immunity and Inflammation in Health and Disease. Protein Cell (2016) 7(3):159–74. doi: 10.1007/s13238-016-0250-0
148. Huang F, Zhao JL, Wang L, Gao CC, Liang SQ, An DJ, et al. Mir-148a-3p Mediates Notch Signaling to Promote the Differentiation and M1 Activation of Macrophages. Front Immunol (2017) 8:1327. doi: 10.3389/fimmu.2017.01327
149. Wei W, Li ZP, Bian ZX, Han QB. Astragalus Polysaccharide Rap Induces Macrophage Phenotype Polarization to M1 Via the Notch Signaling Pathway. Molecules (2019) 24(10):2016. doi: 10.3390/molecules24102016
150. Ma T, Li X, Zhu Y, Yu S, Liu T, Zhang X, et al. Excessive Activation of Notch Signaling in Macrophages Promote Kidney Inflammation, Fibrosis, and Necroptosis. Front Immunol (2022) 13:835879. doi: 10.3389/fimmu.2022.835879
151. Bai X, Li J, Li L, Liu M, Liu Y, Cao M, et al. Extracellular Vesicles From Adipose Tissue-Derived Stem Cells Affect Notch-Mir148a-3p Axis to Regulate Polarization of Macrophages and Alleviate Sepsis in Mice. Front Immunol (2020) 11:1391. doi: 10.3389/fimmu.2020.01391
152. Loboda A, Damulewicz M, Pyza E, Jozkowicz A, Dulak J. Role of Nrf2/Ho-1 System in Development, Oxidative Stress Response and Diseases: An Evolutionarily Conserved Mechanism. Cell Mol Life Sci (2016) 73(17):3221–47. doi: 10.1007/s00018-016-2223-0
153. Shen K, Jia Y, Wang X, Zhang J, Liu K, Wang J, et al. Exosomes From Adipose-Derived Stem Cells Alleviate the Inflammation and Oxidative Stress Via Regulating Nrf2/Ho-1 Axis in Macrophages. Free Radic Biol Med (2021) 165:54–66. doi: 10.1016/j.freeradbiomed.2021.01.023
154. Feng J, Liu Z, Chen H, Zhang M, Ma X, Han Q, et al. Protective Effect of Cynaroside on Sepsis-Induced Multiple Organ Injury Through Nrf2/Ho-1-Dependent Macrophage Polarization. Eur J Pharmacol (2021) 911:174522. doi: 10.1016/j.ejphar.2021.174522
155. Wu Q, Ma Y, Liu Y, Wang N, Zhao X, Wen D. Cb2r Agonist Jwh-133 Attenuates Chronic Inflammation by Restraining M1 Macrophage Polarization Via Nrf2/Ho-1 Pathway in Diet-Induced Obese Mice. Life Sci (2020) 260:118424. doi: 10.1016/j.lfs.2020.118424
156. Galien R. Janus Kinases in Inflammatory Bowel Disease: Four Kinases for Multiple Purposes. Pharmacol Rep (2016) 68(4):789–96. doi: 10.1016/j.pharep.2016.04.001
157. Xu P, Shen P, Yu B, Xu X, Ge R, Cheng X, et al. Janus Kinases (Jaks): The Efficient Therapeutic Targets for Autoimmune Diseases and Myeloproliferative Disorders. Eur J Med Chem (2020) 192:112155. doi: 10.1016/j.ejmech.2020.112155
158. Murray PJ. The Jak-Stat Signaling Pathway: Input and Output Integration. J Immunol (2007) 178(5):2623–9. doi: 10.4049/jimmunol.178.5.2623
159. Akira S. Functional Roles of Stat Family Proteins: Lessons From Knockout Mice. Stem Cells (1999) 17(3):138–46. doi: 10.1002/stem.170138
160. Burrell JA, Boudreau A, Stephens JM. Latest Advances in Stat Signaling and Function in Adipocytes. Clin Sci (Lond) (2020) 134(6):629–39. doi: 10.1042/cs20190522
161. Juhas U, Ryba-Stanisławowska M, Szargiej P, Myśliwska J. Different Pathways of Macrophage Activation and Polarization. Postepy Hig Med Dosw (Online) (2015) 69:496–502. doi: 10.5604/17322693.1150133
162. Ji L, Zhao X, Zhang B, Kang L, Song W, Zhao B, et al. Slc6a8-Mediated Creatine Uptake and Accumulation Reprogram Macrophage Polarization Via Regulating Cytokine Responses. Immunity (2019) 51(2):272–84.e7. doi: 10.1016/j.immuni.2019.06.007
163. Wu BM, Liu JD, Li YH, Li J. Margatoxin Mitigates Ccl4−Induced Hepatic Fibrosis in Mice Via Macrophage Polarization, Cytokine Secretion and Stat Signaling. Int J Mol Med (2020) 45(1):103–14. doi: 10.3892/ijmm.2019.4395
164. Xie C, Ye F, Zhang N, Huang Y, Pan Y, Xie X. Ccl7 Contributes to Angiotensin Ii-Induced Abdominal Aortic Aneurysm by Promoting Macrophage Infiltration and Pro-Inflammatory Phenotype. J Cell Mol Med (2021) 25(15):7280–93. doi: 10.1111/jcmm.16757
165. Lv Y, Li Y, Wang J, Li M, Zhang W, Zhang H, et al. Mir-382-5p Suppresses M1 Macrophage Polarization and Inflammatory Response in Response to Bronchopulmonary Dysplasia Through Targeting Cdk8: Involving Inhibition of Stat1 Pathway. Genes Cells (2021) 26(10):772–81. doi: 10.1111/gtc.12883
166. Bode JG, Ehlting C, Häussinger D. The Macrophage Response Towards Lps and Its Control Through the P38(Mapk)-Stat3 Axis. Cell Signal (2012) 24(6):1185–94. doi: 10.1016/j.cellsig.2012.01.018
167. Zhao J, Yu H, Liu Y, Gibson SA, Yan Z, Xu X, et al. Protective Effect of Suppressing Stat3 Activity in Lps-Induced Acute Lung Injury. Am J Physiol Lung Cell Mol Physiol (2016) 311(5):L868–l80. doi: 10.1152/ajplung.00281.2016
168. Liang Y, Yang N, Pan G, Jin B, Wang S, Ji W. Elevated Il-33 Promotes Expression of Mmp2 and Mmp9 Via Activating Stat3 in Alveolar Macrophages During Lps-Induced Acute Lung Injury. Cell Mol Biol Lett (2018) 23:52. doi: 10.1186/s11658-018-0117-x
169. Ishii M, Wen H, Corsa CA, Liu T, Coelho AL, Allen RM, et al. Epigenetic Regulation of the Alternatively Activated Macrophage Phenotype. Blood (2009) 114(15):3244–54. doi: 10.1182/blood-2009-04-217620
170. Cao L, Xu H, Wang G, Liu M, Tian D, Yuan Z. Extracellular Vesicles Derived From Bone Marrow Mesenchymal Stem Cells Attenuate Dextran Sodium Sulfate-Induced Ulcerative Colitis by Promoting M2 Macrophage Polarization. Int Immunopharmacol (2019) 72:264–74. doi: 10.1016/j.intimp.2019.04.020
Keywords: macrophage polarization, mesenchymal stem cells, acute lung injury, acute respiratory distress syndrome, treatment
Citation: Liu C, Xiao K and Xie L (2022) Advances in the Regulation of Macrophage Polarization by Mesenchymal Stem Cells and Implications for ALI/ARDS Treatment. Front. Immunol. 13:928134. doi: 10.3389/fimmu.2022.928134
Received: 25 April 2022; Accepted: 16 June 2022;
Published: 08 July 2022.
Edited by:
Philippe Saas, INSERM U1098 Interactions Hôte-Greffon-Tumeur & Ingénierie Cellulaire et Génique, FranceReviewed by:
Rita Barcia, SIRPant Immunotherapeutics, United StatesArturo Briva, Universidad de la República, Uruguay
Bruno L. Diaz, Federal University of Rio de Janeiro, Brazil
Copyright © 2022 Liu, Xiao and Xie. This is an open-access article distributed under the terms of the Creative Commons Attribution License (CC BY). The use, distribution or reproduction in other forums is permitted, provided the original author(s) and the copyright owner(s) are credited and that the original publication in this journal is cited, in accordance with accepted academic practice. No use, distribution or reproduction is permitted which does not comply with these terms.
*Correspondence: Kun Xiao, xiaok301@foxmail.com; Lixin Xie, xielx301@126.com