- 1Department II of Infectious Diseases, Xixi Hospital of Hangzhou, Hangzhou, China
- 2Department of Infectious Diseases, State Key Laboratory for Diagnosis and Treatment of Infectious Diseases, Collaborative Innovation Center for Diagnosis and Treatment of Infectious Diseases, National Clinical Research Center for Infectious Diseases, The First Affiliated Hospital, College of Medicine, Zhejiang University, Hangzhou, Zhejiang, China
- 3Department of Clinical Laboratory, The First Affiliated Hospital, College of Medicine, Zhejiang University, Hangzhou, Zhejiang, China
β-glucan is the most abundant polysaccharide in the cell wall of Pneumocystis jirovecii, which has attracted extensive attention because of its unique immunobiological characteristics. β-glucan binds to various cell surface receptors, which produces an inflammatory response and accounts for its immune effects. A deeper comprehension of the processes by Pneumocystis β-glucan recognizes its receptors, activates related signaling pathways, and regulates immunity as required. Such understanding will provide a basis for developing new therapies against Pneumocystis. Herein, we briefly review the structural composition of β-glucans as a vital component of the Pneumocystis cell wall, the host immunity mediated by β-glucans after their recognition, and discuss opportunities for the development of new strategies to combat Pneumocystis.
1 Introduction
The ascomycetous fungus, Pneumocystis jirovecii, is the causative agent of serious fungal pneumonia, predominantly occurring in immunocompromised individuals, especially HIV-positive patients. The use of antiretrovirals and medications for prophylaxis in the developed world has led to a decline in the mortality of Pneumocystis pneumonia (PCP) in patients with HIV in recent years. However, Pneumocystis remains an important pathogen worldwide because of the continued epidemic of acquired immunodeficiency syndrome (AIDS). Pneumocystis is also a common pathogen in non-HIV individuals taking immunosuppressive medications. PCP has high mortality and morbidity, which are commonly higher in patients without AIDS than in those with AIDS (1). The mortality of PCP is 10%–30% in AIDS patients and 40%–70% in non-AIDS patients (2–5). This difference may be explained by the greater lung damage in non-AIDS patients caused by intense lung inflammation. Thus, research should focus on finding better therapies for PCP.
2 Cell wall structure
Pneumocystis has a high tropism for the lung and is usually only detected in the lungs of infected hosts, where it completes all of its life cycle stages (6). The cell wall of Pneumocystis comprises β-glucans, chitins, and other carbohydrate polymers as a dynamic carbohydrate backbone (7, 8). This dynamic carbohydrate backbone plays an important role in the integrity and growth of Pneumocystis and mediates the immune response of the host to Pneumocystis. The cell wall components of Pneumocystis vary at different stages of the life cycle, e.g., the cystic and trophic forms. Pneumocystis jirovecii has three distinct life cycle stages (trophic form, pre-cystic form, and mature cyst), which have different structures and sizes while growing in the lung (6). The pre-cystic and trophic forms lack β-glucans (9). A recent study considered that the Pneumocystis cell wall lacks chitin, outer chain N-mannans, and α-glucan, which are present in many other fungi (10).
β-glucans represent important components of the Pneumocystis cell wall. Fungal cell wall β-glucans comprise a β-1,3-glucan backbone with variable side chain β-1,6 linkages (11). Many of the enzymes located within the cell membrane participate in the formation of the glucose backbone of the Pneumocystis cell wall (Table 1). The enzyme PcGsc-1 (glucan synthetase) forms the essential β-1,3-glucan backbone by polymerizing uridine-5-diphosphoglucose (12). PcGsc-1 is activated by PcAce2, which is phosphorylated by the upstream PcCbk1, a cell wall biosynthesis kinase (18). A recent study proposed that Pckre6 encodes β-1,6-glucan synthase in Pneumocystis (13). Pneumocystis Pcphr1 is believed to encode a protein that binds to the β-1,6-glucans of the β-1,3 backbone (14), and it is also pH responsive, which helps the cell wall to adapt to changing environmental conditions. β-1,3 endoglucanase has the most important role of degrading β-glucans in fungal cell walls. The single copy gene, Pceng2, encodes an endo-β-1,3-glucanase (15), which probably functions to switch the expressed major surface glycoprotein (Msg) variant (16). Bgl2 is considered to have an endo-β-1,3-glucanase activity and a glucanosyltransferase activity in Pneumocystis, and it exerts this glucanosyltransferase activity by cleaving reduced laminaripentaose and transferring oligosaccharides, resulting in polymers of six and seven glucan residues (17). In addition, β-1,3-glucan in the host serum has a critical role in the diagnosis of PCP; however, novel systematic reviews and meta-analyses have been reported. They found that sensitivity was 91% and specificity was 79% for the diagnosis of PCP. The sensitivity was better in HIV patients (94%) than that in non-HIV patients (86%), and the specificity was equivalent (83% vs. 83%) (19). Here, we do not describe the diagnostic value of β-1,3-glucan in detail again. Pneumocystis glucan provides cell wall stability and mediates the host lung inflammatory and immune responses.
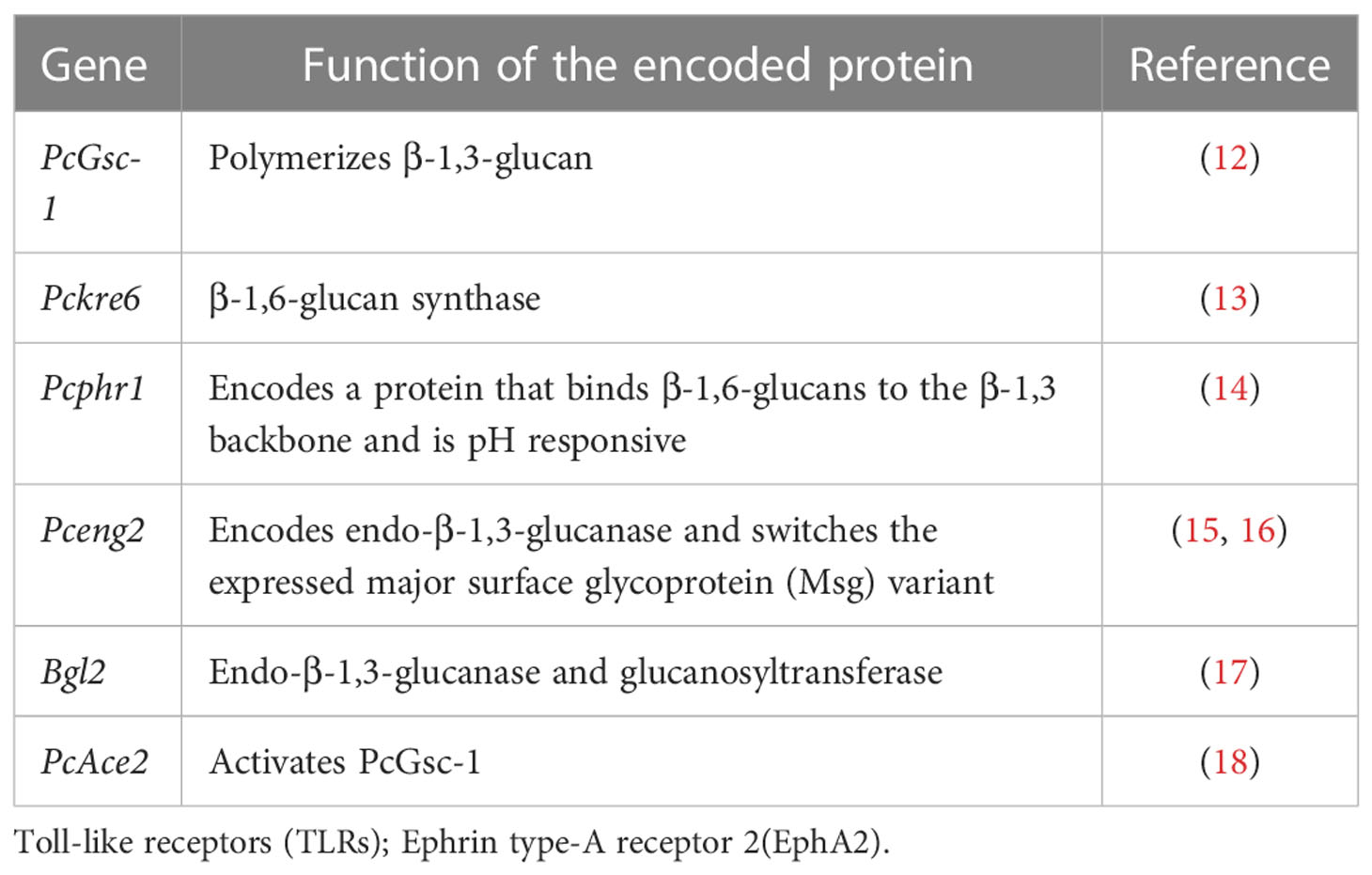
Table 1 Genes encode enzymes located within the cell membrane that participate in the formation of the glucose backbone in the Pneumocystis cell wall.
3 Immune recognition
The molecular immunogenic signatures of fungal pathogens in the cell wall are known as pathogen-associated molecular patterns (PAMPs). PAMPs usually comprise essential structural components that are lacking in the host and are recognized by host cells via binding to cellular or soluble pattern recognition receptors (PRRs). PRRs comprise four main types, RIG-I-like receptors (RLRs), NOD-like receptors (NLRs), C-type lectin-like receptors (CLRs), and Toll-like receptors (TLRs), which are expressed in most cell types (20). β-glucan, as a kind of PAMP, is important in the recognition of Pneumocystis by both alveolar macrophages (AMs) and alveolar epithelial cells (AECs). Glucans have a number of potential receptors, such as dectin-1 and TLRs (21). The major receptors of Pneumocystis β-glucans and the initial immunity mediated by β-glucan through these receptors are discussed below (Table 2; Figure 1).
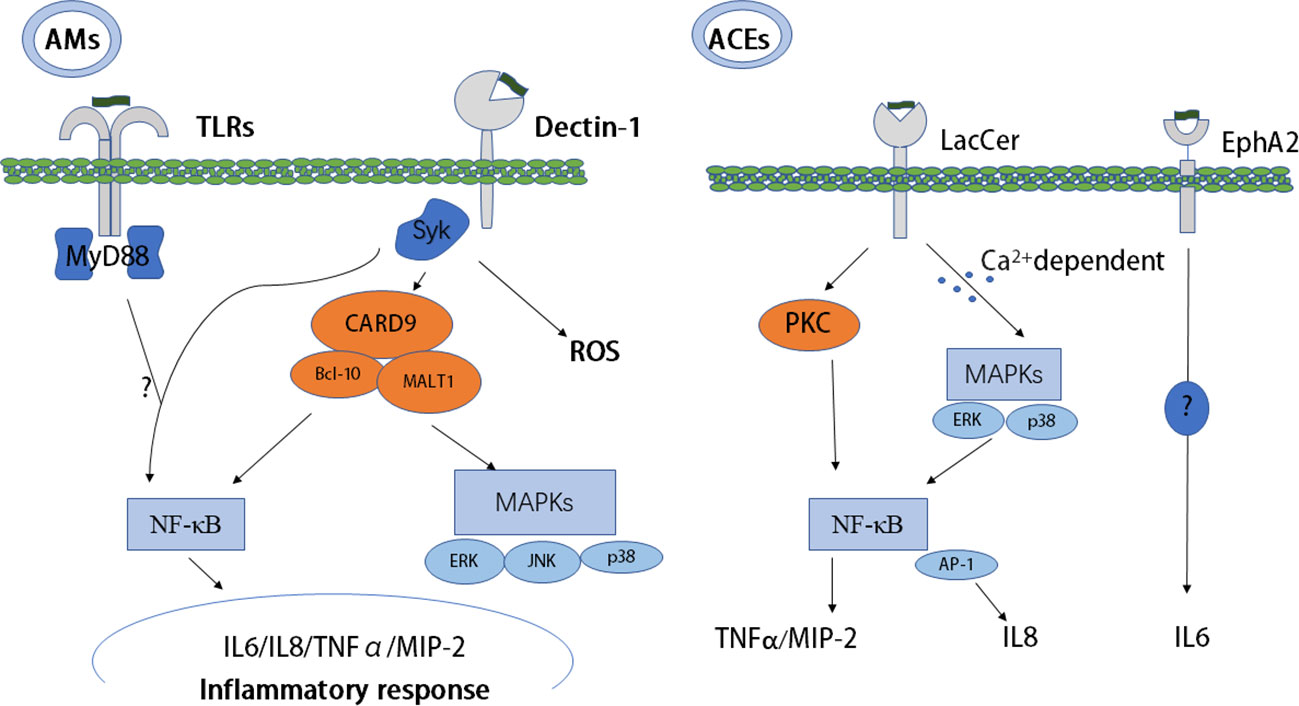
Figure 1 Host recognition of PCBG and PCBG-mediated initial immunity. Dectin-1, TLRs, EphA2, and LacCer as receptors recognize PCBG and mediate initial immunity resulting in an inflammatory response. Collaboration between dectin-1 and TLRs to mediate the NF-κB pathway is unclear, and the signaling pathway of EphA2 is also very unclear. AMs, alveolar macrophages; MyD88, myeloid differentiation primary response 88; NF-κB, nuclear factor kappa-light-chain-enhancer of activated B cells; PC, Pneumocystis; SYK, spleen tyrosine kinase; TLRs, Toll-like receptors; EphA2, ephrin type-A receptor 2; MIP-2, macrophage inflammatory protein 2, TNF-α, tumor necrosis factor-alpha; AECs, alveolar epithelial cells; PCBG, Pneumocystis carinii cell wall constituent β-1,3-glucan; LacCer, lactosylceramide.
3.1 Dectin-1
Studies have confirmed that the CLR dectin-1 is vital to recognize and kill Pneumocystis and is important in the production of proinflammatory mediators (32). The two main pathways of CLR signaling are the Ras-Raf pathway and the spleen tyrosine kinase (SYK) pathway. Dectin-1 recruits SYK directly or following binding with the Fc receptor c chain (FcRc) (33). After binding to β-glucan, dectin-1 activates SYK, leading to downstream inflammatory signaling (34). After recognizing Pneumocystis, dectin-1 induces internalization and killing, resulting in the production of reactive oxygen species (ROS) in AMs (22). In addition to AMs, dendritic cells (DCs) can also be activated by β-glucans, resulting in T-cell activation and polarization into a Type 1 T helper (Th1) patterned response, but with the absence of interleukin (IL)-12. Furthermore, DC activation is partially regulated by dectin-1 receptor-induced cytokine secretion and by Fas-FasL, which further promotes inflammation, resulting in tumor necrosis factor-alpha (TNF-α) and IL-1β secretion in PCP (23). Interestingly, in response to Pneumocystis β-glucan, dectin-1 does not affect TNF-α and IL-12 secretion (22). However, dectin-1 expression can enhance β-glucan–containing particle activation of nuclear factor kappa-light-chain-enhancer of activated B cells (NF-κB) mediated by TLRs. Subsequently, TLRs and dectin-1 synergistically regulate the production of IL-12 and TNF-α through myeloid differentiation primary response 88 (MyD88) in macrophages and DCs (22, 24).
3.2 Toll-like receptors (TLRs)
TLRs activate two main signaling cascades: the The toll-interleukin-1 receptor (TIR) domain-containing adapter-inducing Interferon-B (TRIF) pathway and the MyD88 pathway (35). Our understanding of the functions of TLRs in the immune response induced by Pneumocystis is incomplete. Pneumocystis murina activates TLR2 in AMs, resulting in NF-κB nuclear translocation and the production of the proinflammatory cytokine TNF-α and chemokine macrophage inflammatory protein 2 (MIP-2) (25). Deficiency in TLR2 increases the susceptibility to P. murina, and it was proposed that TLR2-mediated inflammatory responses can help to clear Pneumocystis in mice (36). However, TLR2 is required by AMs, but not by epithelial cells, to trigger an inflammatory response (26). MyD88-deficient mice demonstrated a partially blunted response of AMs to Pneumocystis β-glucan, suggesting that macrophages are activated through NF-κB via cellular receptors and signaling pathways (27). MyD88-deficient mice also demonstrated the role of MyD88 signaling in both immunopathogenesis and the control of the fungal burden (37). However, Ripamonti et al. (38) found that MyD88 signaling is not required to control Pneumocystis infection. Deficiency of either SYK or TLRs (TLR4, TLR5, TLR7, and TLR9), or the adapter MyD88, abolished the production of TNF, MIP-1α, and MIP-2. Therefore, collaboration between SYK pathway signaling, activated by dectin-1, and TLRs/MyD88 signaling pathways is required to enhance NF-κB nuclear translocation (39). Furthermore, in human primary peripheral blood mononuclear cells (PBMCs) and in monocyte-derived macrophages, collaboration between dectin-1 and TLRs (TLR2, TLR4) to produce TNF-α has been demonstrated (40).
3.3 Lactosylceramide
AECs play an important role in promoting Pneumocystis carinii attachment and mediate lung inflammation via the production of cytokines and chemokines in PCP. Lactosylceramide is a prominent cell membrane glycosphingolipid stimulated by P. carinii cell wall constituent β-1,3-glucan (PCBG), subsequently causing the release of MIP-2 from isolated AECs (30). In addition, the release of IL-8 requires glycosphingolipid for optimal signaling after activation of PCBG by mitogen-activated protein kinases (MAPKs) (31).
3.4 Ephrin type-A receptor 2
CLRs are critical in the myeloid cell response after recognizing Pneumocystis, as proven in vitro and in vivo (21). However, the inflammatory reaction has been proven to occur in epithelial cells, which release cytokines such as IL-6, IL-8, MIP-2, and TNF-α after recognizing fungal β-glucans (41). A previous study identified ephrin type-A receptor 2 (EphA2) as a receptor that binds to fungal β-glucans in lung epithelial cells (28). β-glucans isolated from exposed surfaces of Pneumocystis can also bind with EphA2. Furthermore, the EphA2 receptor can be phosphorylated after the binding, resulting in a downstream proinflammatory response and increased IL-6 cytokine production in lung epithelial cells (29).
4 β-glucan mediated initial immunity
The host’s innate immune system is activated greatly by Pneumocystis cell wall components, including β-glucans (42). However, Pneumocystis has evolved mechanisms to evade and adapt the host response.
AMs, as professional phagocytic cells, recognize microorganism surface PAMPs via PRRs. Attachment and activation of macrophages result in phagocytosis, followed by phagolysosomal fusion and degradation of the microorganisms. AMs are important because they can directly kill trophozoites and cysts, and the severity of PCP and the number of macrophages correlate inversely (43). PCBG from AMs activates NF-κB translocation, which stimulates the production of TNF-α and MIP-2, the murine homolog of IL-8 (27). Steele et al. (32) reported that after Pneumocystis–AM interaction, dectin-1 generates ROS, which mediates nonopsonic phagocytosis and subsequent killing of the pathogen. In Pneumocystis infection, knockout of dectin-1 in macrophages resulted in defective ROS production (22). The adapter molecule, caspase recruitment domain-containing protein 9 (CARD9), is initiated through dectin-1. Subsequent to SYK activation, CARD9 forms a trimolecular complex with B-cell lymphoma/leukemia 10 (BCL-10) and mucosa-associated lymphoid tissue lymphoma translocation protein 1 (MALT1), which activates NF-κB and MAPKs, such as p38, c-Jun N-terminal kinase (JNK), and extracellular signal-regulated kinase (ERK), resulting in the production of a proinflammatory response (44). Moreover, vitronectin and fibronectin binding to PCBG can augment macrophage inflammatory responses (45). Scott et al. proposed that in AECs, the cell surface lactosylceramide, rather than the dectin-1 receptor, mediated chemokine responses. In PCP, AECs are important in host responses, and the production of inflammatory cytokine is induced by β-glucan via NF-kB–dependent mechanisms, which are partly mediated by protein kinase C (PKC) signaling pathways (46). PKC localizes to AEC microdomains, which promote the expression of TNF-α and the rodent C-X-C chemokine MIP-2, in addition to identified inflammatory secondary signaling pathways (47). This might be a potential novel target for therapeutics in immunocompromised populations. Furthermore, PCBG stimulates DCs to interact with lymphocytes, resulting in the activation of the IL-23/IL-17 axis during infection, which functions via the accumulation of lactosylceramide in glycosphingolipid-rich microdomains of the plasma membrane (48). In addition, in a calcium-dependent manner, PCBG can also induce MAPK, ERK, and p38 phosphorylation, NF-κB activation, and subsequent IL-8 secretion through a possible receptor (glycosphingolipids) in human airway epithelial cells, resulting in neutrophil infiltration (31). Rapaka et al. (49) proposed that natural immunoglobulin M (IgM) modulates innate and adaptive immune responses by binding to Pneumocystis β-glucan. Furthermore, depletion of CD4+ T cells did not affect the amount of β-glucan cross-reactive IgG in the serum or mucosa but decreased lung mucosal levels of cross-reactive IgA at the same time as reducing active transforming growth factor β activation. Moreover, despite CD4+ T-cell depletion, IgM levels increased significantly. Thus, an immune response against Pneumocystis β-glucan might occur under conditions of CD4+ T cell-related immunodeficiency via differential CD4+ T cell-dependent regulation of mucosal antibody responses (50).
The specific PRR (dectin-1) for β-glucan has been discovered on the cell surface of phagocytes (51); therefore, β-glucan, as a key PAMP, has attracted increased attention in the study of the immune recognition of pathogenic fungi by the host. However, certain fungi have evolved surface structures that allow them to bypass this innate immune control mechanism. β-glucans of Candida and Aspergillus pathogens can be masked via a thick layer of mannoproteins, abrogating the activation of host innate immune responses (52, 53). Furthermore, Ballou et al. (54) showed that β-glucan on the Candida cell surface can be masked by L-lactate generated by host cells or bacteria from the host’s microbiota. This represents the immune escape of β-glucan masking in Candida, which reduces fungal visibility to the host immune system (54). In the Pneumocystis cyst stage, β-glucans are a major constituent of the cell wall, which can activate innate immune responses. Kutty et al. (55) demonstrated that Pneumocystis cyst cell wall β-1,3-glucans are largely masked by Msg and/or other surface proteins, which would likely block the activation of the innate immune response. Presumably, this mechanism evolved to adapt to immunocompetent hosts with reduced organism loads. Organism death and release of glucans might be important factors in deleterious host inflammatory responses in immunosuppressed hosts with a high organism burden (55). Pneumocystis Msg is a 120-kDa surface protein complex with important functions in adhesion and immune recognition. Kottom et al. (56) showed that the Pneumocystis Msg surface protein complex can suppress TNF-α secretion from macrophages induced by proinflammatory β-glucans.
5 Clinical therapeutic strategies and future perspectives
Currently, trimethoprim–sulfamethoxazole (TMP-SMX) is the first-line antibiotic to treat PCP (57). Pentamidine, clindamycin, primaquine, and atovaquone, as second-line agents, are reserved for mild to moderate disease (58). TMP-SMX can inhibit dihydropteroate synthase (DHPS) and impair the synthesis of folate in Pneumocystis. DHPS mutants in Pneumocystis have been reported, which might result in resistance to TMP-SMX therapy, and the reported increase in the frequency of DHPS mutations might be the result of selection pressure from using TMP-SMX for the prophylaxis and treatment of Pneumocystis globally (59). Echinocandin antibiotics, such as caspofungin, can be used to treat Pneumocystis infection, which disrupt the integrity of the cell wall by inhibiting β-1,3-glucan synthetases. Echinocandins are highly effective in eliminating the cyst forms in animal models but far less effective against the trophic forms (60). Our previous study proved the effectiveness of echinocandin treatment for mild to moderate AIDS-PCP disease (61). Therefore, it is crucial to develop novel therapies for Pneumocystis from different perspectives and to improve clinical outcomes and reduce the mortality of this deadly infection.
Respiratory failure associated with PCP resulting in intense lung inflammation is a major cause of death in immunocompromised patients. Moreover, β-glucan may be a potential target for the treatment of Pneumocystis infection from the aspect of destroying structures and inhibiting pathways, immunity, and inflammatory reactions. In steroid-treated infected rats, aculeacin A, a beta-1,3-glucan biosynthesis inhibitor, ameliorated Pneumocystis cell wall formation and cyst maturation, thereby preventing PCP (62). A recombinant protein, dectin-Fc, comprising dectin-1 fused to the Fc region of murine IgG1, could be used to specifically identify β-1,3 glucan linkages. Targeting Pneumocystis β-glucan with dectin-Fc enhanced host recognition and the clearance of Pneumocystis and might enhance resistance to PCP in immunodeficient hosts (63). Similarly, dectin immunoadhesins (dectin-1:mIgG1 and dectin-1:mIgG2a Fc), comprising dectin-1 fused to the Fc regions of the four subtypes of murine IgG (mIgG), could reduce cytokine production and hypoxemia, although they had less effect on the lung fungal burden (64). Furthermore, CARD9, as a downstream factor of dectin-1, is activated by recognition of β-glucans, resulting in damaging inflammation. A CARD9 inhibitor (BRD5529) was shown to reduce phospho-p38 and phospho-pERK1 signaling and TNF-α release during Pneumocystis β-glucan stimulation of macrophages (65). Macrophages can enhance effector functions toward subsequent heterologous stimuli after exposure to the fungal β-glucan as a kind of trained immunity. In addition, trained macrophages displayed increased glycolysis and oxidative phosphorylation when competing for limiting levels of nutrients (66). The mechanistic target of rapamycin (mTOR)/hypoxia-inducible factor 1 alpha (HIF-1α) axis regulates the functional and metabolic reprogramming of β-glucan-trained macrophages (67). Combining inhibitors of glucose uptake and glycolysis with antifungal agents, with the aim of decreasing inflammation and consequently diminishing the development of resistance to antifungals, might represent a potential therapeutic strategy.
6 Conclusion
Our understanding of Pneumocystis immunobiology has grown rapidly in recent decades. Vital components of the Pneumocystis cell wall and their synthetic mechanisms, as well as important interactions with the host, have been proposed. Comparisons with closely related fungi have revealed important cell signaling pathways in Pneumocystis. β-glucan has been demonstrated experimentally to have a vital function in the interaction between the host and the pathogen during infections. Research has revealed some molecular and cellular mechanisms by which β-glucan mediates immunity and immune escape in Pneumocystis infections. However, further studies to refine the immunomodulatory mechanisms and to develop therapeutic strategies are needed.
Author contributions
Conceptualization, MW, ZZ, and BZ; Data curation, MW and XD; Software analysis, MW and XD; Original draft preparation, MW, ZZ, and BZ; Review and Editing, MW and BZ; Supervision, BZ; Project administration, BZ; Funding acquisition, MW and BZ. All authors contributed to the article and approved the submitted version.
Funding
This work was supported by a grant from the Hangzhou Science and Technology Bureau Guided Project (China) (grant number 20220919Y035).
Conflict of interest
The authors declare that the research was conducted in the absence of any commercial or financial relationships that could be construed as a potential conflict of interest.
Publisher’s note
All claims expressed in this article are solely those of the authors and do not necessarily represent those of their affiliated organizations, or those of the publisher, the editors and the reviewers. Any product that may be evaluated in this article, or claim that may be made by its manufacturer, is not guaranteed or endorsed by the publisher.
References
1. Bienvenu AL, Traore K, Plekhanova I, Bouchrik M, Bossard C, Picot S. Pneumocystis pneumonia suspected cases in 604 non-HIV and HIV patients. Int J Infect Dis (2016) 46:11–7. doi: 10.1016/j.ijid.2016.03.018
2. Mansharamani NG, Garland R, Delaney D, Koziel H. Management and outcome patterns for adult pneumocystis carinii pneumonia, 1985 to 1995: Comparison of HIV-associated cases to other immunocompromised states. Chest. (2000) 118(3):704–11. doi: 10.1378/chest.118.3.704
3. Monnet X, Vidal-Petiot E, Osman D, Hamzaoui O, Durrbach A, Goujard C, et al. Critical care management and outcome of severe pneumocystis pneumonia in patients with and without HIV infection. Crit Care (2008) 12(1):R28. doi: 10.1186/cc6806
4. Festic E, Gajic O, Limper AH, Aksamit TR. Acute respiratory failure due to pneumocystis pneumonia in patients without human immunodeficiency virus infection: Outcome and associated features. Chest. (2005) 128(2):573–9. doi: 10.1378/chest.128.2.573
5. Fei MW, Kim EJ, Sant CA, Jarlsberg LG, Davis JL, Swartzman A, et al. Predicting mortality from HIV-associated pneumocystis pneumonia at illness presentation: An observational cohort study. Thorax. (2009) 64(12):1070–6. doi: 10.1136/thx.2009.117846
6. Skalski JH, Kottom TJ, Limper AH. Pathobiology of pneumocystis pneumonia: Life cycle, cell wall and cell signal transduction. FEMS Yeast Res (2015) 15(6). doi: 10.1093/femsyr/fov046
7. Roth A, Wecke J, Karsten V, Janitschke K. Light and electron microscopy study of carbohydrate antigens found in the electron-lucent layer of pneumocystis carinii cysts. Parasitol Res (1997) 83(2):177–84. doi: 10.1007/s004360050229
8. De Stefano JA, Myers JD, Du Pont D, Foy JM, Theus SA, Walzer PD. Cell wall antigens of pneumocystis carinii trophozoites and cysts: Purification and carbohydrate analysis of these glycoproteins. J Eukaryot Microbiol (1998) 45(3):334–43. doi: 10.1111/j.1550-7408.1998.tb04545.x
9. Chabé M, Aliouat-Denis CM, Delhaes L, Aliouat el M, Viscogliosi E, Dei-Cas E. Pneumocystis: from a doubtful unique entity to a group of highly diversified fungal species. FEMS Yeast Res (2011) 11(1):2–17. doi: 10.1111/j.1567-1364.2010.00698.x
10. Ma L, Chen Z, Huang da W, Kutty G, Ishihara M, Wang H, et al. Genome analysis of three pneumocystis species reveals adaptation mechanisms to life exclusively in mammalian hosts. Nat Commun (2016) 7:10740. doi: 10.1038/ncomms10740
11. Douglas CM. Fungal beta(1,3)-d-glucan synthesis. Med Mycol (2001) 39 Suppl 1:55–66. doi: 10.1080/mmy.39.1.55.66
12. Kottom TJ, Limper AH. Cell wall assembly by pneumocystis carinii. evidence for a unique gsc-1 subunit mediating beta -1,3-glucan deposition. J Biol Chem (2000) 275(51):40628–34. doi: 10.1074/jbc.M002103200
13. Kottom TJ, Hebrink DM, Jenson PE, Gudmundsson G, Limper AH. Evidence for proinflammatory β-1,6 glucans in the pneumocystis carinii cell wall. Infect Immun (2015) 83(7):2816–26. doi: 10.1128/iai.00196-15
14. Kottom TJ, Thomas CF Jr., Limper AH. Characterization of pneumocystis carinii PHR1, a pH-regulated gene important for cell wall integrity. J Bacteriol (2001) 183(23):6740–5. doi: 10.1128/jb.183.23.6740-6745.2001
15. Villegas LR, Kottom TJ, Limper AH. Characterization of PCEng2, a {beta}-1,3-endoglucanase homolog in pneumocystis carinii with activity in cell wall regulation. Am J Respir Cell Mol Biol (2010) 43(2):192–200. doi: 10.1165/rcmb.2009-0131OC
16. Kutty G, Davis AS, Ma L, Taubenberger JK, Kovacs JA. Pneumocystis encodes a functional endo-β-1,3-glucanase that is expressed exclusively in cysts. J Infect Dis (2015) 211(5):719–28. doi: 10.1093/infdis/jiu517
17. Kutty G, Davis AS, Schuck K, Masterson M, Wang H, Liu Y, et al. Characterization of pneumocystis murina Bgl2, an endo-β-1,3-Glucanase and glucanosyltransferase. J Infect Dis (2019) 220(4):657–65. doi: 10.1093/infdis/jiz172
18. Kottom TJ, Limper AH. The pneumocystis Ace2 transcription factor regulates cell wall-remodeling genes and organism virulence. J Biol Chem (2013) 288(33):23893–902. doi: 10.1074/jbc.M113.471243
19. Del Corpo O, Butler-Laporte G, Sheppard DC, Cheng MP, McDonald EG, Lee TC. Diagnostic accuracy of serum (1-3)-β-D-glucan for pneumocystis jirovecii pneumonia: A systematic review and meta-analysis. Clin Microbiol Infect (2020) 26(9):1137–43. doi: 10.1016/j.cmi.2020.05.024
20. Brubaker SW, Bonham KS, Zanoni I, Kagan JC. Innate immune pattern recognition: a cell biological perspective. Annu Rev Immunol (2015) 33:257–90. doi: 10.1146/annurev-immunol-032414-112240
21. Hoving JC. Pneumocystis and interactions with host immune receptors. PloS Pathog (2018) 14(2):e1006807. doi: 10.1371/journal.ppat.1006807
22. Saijo S, Fujikado N, Furuta T, Chung SH, Kotaki H, Seki K, et al. Dectin-1 is required for host defense against pneumocystis carinii but not against candida albicans. Nat Immunol (2007) 8(1):39–46. doi: 10.1038/ni1425
23. Carmona EM, Vassallo R, Vuk-Pavlovic Z, Standing JE, Kottom TJ, Limper AH. Pneumocystis cell wall beta-glucans induce dendritic cell costimulatory molecule expression and inflammatory activation through a fas-fas ligand mechanism. J Immunol (2006) 177(1):459–67. doi: 10.4049/jimmunol.177.1.459
24. Gantner BN, Simmons RM, Canavera SJ, Akira S, Underhill DM. Collaborative induction of inflammatory responses by dectin-1 and toll-like receptor 2. J Exp Med (2003) 197(9):1107–17. doi: 10.1084/jem.20021787
25. Zhang C, Wang SH, Lasbury ME, Tschang D, Liao CP, Durant PJ, et al. Toll-like receptor 2 mediates alveolar macrophage response to pneumocystis murina. Infect Immun (2006) 74(3):1857–64. doi: 10.1128/iai.74.3.1857-1864.2006
26. Bello-Irizarry SN, Wang J, Olsen K, Gigliotti F, Wright TW. The alveolar epithelial cell chemokine response to pneumocystis requires adaptor molecule MyD88 and interleukin-1 receptor but not toll-like receptor 2 or 4. Infect Immun (2012) 80(11):3912–20. doi: 10.1128/iai.00708-12
27. Lebron F, Vassallo R, Puri V, Limper AH. Pneumocystis carinii cell wall beta-glucans initiate macrophage inflammatory responses through NF-kappaB activation. J Biol Chem (2003) 278(27):25001–8. doi: 10.1074/jbc.M301426200
28. Swidergall M, Solis NV, Lionakis MS, Filler SG. EphA2 is an epithelial cell pattern recognition receptor for fungal β-glucans. Nat Microbiol (2018) 3(1):53–61. doi: 10.1038/s41564-017-0059-5
29. Kottom TJ, Schaefbauer K, Carmona EM, Limper AH. EphA2 is a lung epithelial cell receptor for pneumocystis β-glucans. J Infect Dis (2022) 225(3):525–30. doi: 10.1093/infdis/jiab384
30. Hahn PY, Evans SE, Kottom TJ, Standing JE, Pagano RE, Limper AH. Pneumocystis carinii cell wall beta-glucan induces release of macrophage inflammatory protein-2 from alveolar epithelial cells via a lactosylceramide-mediated mechanism. J Biol Chem (2003) 278(3):2043–50. doi: 10.1074/jbc.M209715200
31. Carmona EM, Lamont JD, Xue A, Wylam M, Limper AH. Pneumocystis cell wall beta-glucan stimulates calcium-dependent signaling of IL-8 secretion by human airway epithelial cells. Respir Res (2010) 11(1):95. doi: 10.1186/1465-9921-11-95
32. Steele C, Marrero L, Swain S, Harmsen AG, Zheng M, Brown GD, et al. Alveolar macrophage-mediated killing of pneumocystis carinii f. sp. muris involves molecular recognition by the dectin-1 beta-glucan receptor. J Exp Med (2003) 198(11):1677–88. doi: 10.1084/jem.20030932
33. Sancho D, Reis e Sousa C. Signaling by myeloid c-type lectin receptors in immunity and homeostasis. Annu Rev Immunol (2012) 30:491–529. doi: 10.1146/annurev-immunol-031210-101352
34. Kottom TJ, Hebrink DM, Jenson PE, Nandakumar V, Wüthrich M, Wang H, et al. The interaction of pneumocystis with the c-type lectin receptor mincle exerts a significant role in host defense against infection. J Immunol (2017) 198(9):3515–25. doi: 10.4049/jimmunol.1600744
35. Kawai T, Akira S. The role of pattern-recognition receptors in innate immunity: Update on toll-like receptors. Nat Immunol (2010) 11(5):373–84. doi: 10.1038/ni.1863
36. Wang SH, Zhang C, Lasbury ME, Liao CP, Durant PJ, Tschang D, et al. Decreased inflammatory response in toll-like receptor 2 knockout mice is associated with exacerbated pneumocystis pneumonia. Microbes Infect (2008) 10(4):334–41. doi: 10.1016/j.micinf.2007.12.014
37. Bello-Irizarry SN, Wang J, Johnston CJ, Gigliotti F, Wright TW. MyD88 signaling regulates both host defense and immunopathogenesis during pneumocystis infection. J Immunol (2014) 192(1):282–92. doi: 10.4049/jimmunol.1301431
38. Ripamonti C, Bishop LR, Yang J, Lempicki RA, Kovacs JA. Clearance of pneumocystis murina infection is not dependent on MyD88. Microbes Infect (2014) 16(6):522–7. doi: 10.1016/j.micinf.2014.03.005
39. Dennehy KM, Ferwerda G, Faro-Trindade I, Pyz E, Willment JA, Taylor PR, et al. Syk kinase is required for collaborative cytokine production induced through dectin-1 and toll-like receptors. Eur J Immunol (2008) 38(2):500–6. doi: 10.1002/eji.200737741
40. Ferwerda G, Meyer-Wentrup F, Kullberg BJ, Netea MG, Adema GJ. Dectin-1 synergizes with TLR2 and TLR4 for cytokine production in human primary monocytes and macrophages. Cell Microbiol (2008) 10(10):2058–66. doi: 10.1111/j.1462-5822.2008.01188.x
41. Kottom TJ, Hebrink DM, Limper AH. Binding of pneumocystis carinii to the lung epithelial cell receptor HSPA5 (GRP78). J Med Microbiol (2018) 67(12):1772–7. doi: 10.1099/jmm.0.000864
42. Kottom TJ, Carmona EM, Limper AH. Current state of carbohydrate recognition and c-type lectin receptors in pneumocystis innate immunity. Front Immunol (2021) 12:798214. doi: 10.3389/fimmu.2021.798214
43. Fleury J, Escudier E, Pocholle MJ, Carre C, Bernaudin JF. Cell population obtained by bronchoalveolar lavage in pneumocystis carinii pneumonitis. Acta Cytol. (1985) 29(5):721–6.
44. Kottom TJ, Nandakumar V, Hebrink DM, Carmona EM, Limper AH. A critical role for CARD9 in pneumocystis pneumonia host defence. Cell Microbiol (2020) 22(10):e13235. doi: 10.1111/cmi.13235
45. Vassallo R, Kottom TJ, Standing JE, Limper AH. Vitronectin and fibronectin function as glucan binding proteins augmenting macrophage responses to pneumocystis carinii. Am J Respir Cell Mol Biol (2001) 25(2):203–11. doi: 10.1165/ajrcmb.25.2.4427
46. Evans SE, Hahn PY, McCann F, Kottom TJ, Pavlovic ZV, Limper AH. Pneumocystis cell wall beta-glucans stimulate alveolar epithelial cell chemokine generation through nuclear factor-kappaB-dependent mechanisms. Am J Respir Cell Mol Biol (2005) 32(6):490–7. doi: 10.1165/rcmb.2004-0300OC
47. Evans SE, Kottom TJ, Pagano RE, Limper AH. Primary alveolar epithelial cell surface membrane microdomain function is required for pneumocystis β-glucan-induced inflammatory responses. Innate Immun (2012) 18(5):709–16. doi: 10.1177/1753425912436763
48. Carmona EM, Kottom TJ, Hebrink DM, Moua T, Singh RD, Pagano RE, et al. Glycosphingolipids mediate pneumocystis cell wall β-glucan activation of the IL-23/IL-17 axis in human dendritic cells. Am J Respir Cell Mol Biol (2012) 47(1):50–9. doi: 10.1165/rcmb.2011-0159OC
49. Rapaka RR, Ricks DM, Alcorn JF, Chen K, Khader SA, Zheng M, et al. Conserved natural IgM antibodies mediate innate and adaptive immunity against the opportunistic fungus pneumocystis murina. J Exp Med (2010) 207(13):2907–19. doi: 10.1084/jem.20100034
50. Rapaka RR, Dai G, Zheng M, Kolls JK. CD4(+) T cell regulation of antibodies cross-reactive with fungal cell wall-associated carbohydrates after pneumocystis murina infection. Infect Immun (2019) 87(7). doi: 10.1128/iai.00158-19
51. Brown GD, Gordon S. Immune recognition. A New receptor beta-glucans. Nature. (2001) 413(6851):36–7. doi: 10.1038/35092620
52. Hohl TM, Feldmesser M, Perlin DS, Pamer EG. Caspofungin modulates inflammatory responses to aspergillus fumigatus through stage-specific effects on fungal beta-glucan exposure. J Infect Dis (2008) 198(2):176–85. doi: 10.1086/589304
53. Wheeler RT, Fink GR. A drug-sensitive genetic network masks fungi from the immune system. PloS Pathog (2006) 2(4):e35. doi: 10.1371/journal.ppat.0020035
54. Ballou ER, Avelar GM, Childers DS, Mackie J, Bain JM, Wagener J, et al. Lactate signalling regulates fungal β-glucan masking and immune evasion. Nat Microbiol (2016) 2:16238. doi: 10.1038/nmicrobiol.2016.238
55. Kutty G, Davis AS, Ferreyra GA, Qiu J, Huang da W, Sassi M, et al. β-glucans are masked but contribute to pulmonary inflammation during pneumocystis pneumonia. J Infect Dis (2016) 214(5):782–91. doi: 10.1093/infdis/jiw249
56. Kottom TJ, Hebrink DM, Carmona EM, Limper AH. Pneumocystis carinii major surface glycoprotein dampens macrophage inflammatory responses to fungal β-glucan. J Infect Dis (2020) 222(7):1213–21. doi: 10.1093/infdis/jiaa218
57. Salzer HJF, Schäfer G, Hoenigl M, Günther G, Hoffmann C, Kalsdorf B, et al. Clinical, diagnostic, and treatment disparities between HIV-infected and non-HIV-Infected immunocompromised patients with pneumocystis jirovecii pneumonia. Respiration. (2018) 96(1):52–65. doi: 10.1159/000487713
58. Limper AH, Knox KS, Sarosi GA, Ampel NM, Bennett JE, Catanzaro A, et al. An official American thoracic society statement: Treatment of fungal infections in adult pulmonary and critical care patients. Am J Respir Crit Care Med (2011) 183(1):96–128. doi: 10.1164/rccm.2008-740ST
59. Sheikholeslami MF, Sadraei J, Farnia P, Forozandeh Moghadam M, Emadikochak H. Dihydropteroate synthase gene mutation rates in pneumocystis jirovecii strains obtained from Iranian HIV-positive and non-HIV-positive patients. Med Mycol (2015) 53(4):361–8. doi: 10.1093/mmy/myu095
60. Sun P, Tong Z. Efficacy of caspofungin, a 1,3-β-D-glucan synthase inhibitor, on pneumocystis carinii pneumonia in rats. Med Mycol (2014) 52(8):798–803. doi: 10.1093/mmy/myu060
61. Wang M, Lang G, Chen Y, Hu C, Guo Y, Tao R, et al. A pilot study of echinocandin combination with Trimethoprim/Sulfamethoxazole and clindamycin for the treatment of AIDS patients with pneumocystis pneumonia. J Immunol Res (2019) 2019:8105075. doi: 10.1155/2019/8105075
62. Matsumoto Y, Yamada M, Amagai T. Yeast glucan of pneumocystis carinii cyst wall: an excellent target for chemotherapy. J Protozool. (1991) 38(6):6s–7s.
63. Rapaka RR, Goetzman ES, Zheng M, Vockley J, McKinley L, Kolls JK, et al. Enhanced defense against pneumocystis carinii mediated by a novel dectin-1 receptor fc fusion protein. J Immunol (2007) 178(6):3702–12. doi: 10.4049/jimmunol.178.6.3702
64. Ricks DM, Chen K, Zheng M, Steele C, Kolls JK. Dectin immunoadhesins and pneumocystis pneumonia. Infect Immun (2013) 81(9):3451–62. doi: 10.1128/iai.00136-13
65. Kottom TJ, Carmona EM, Limper AH. Targeting CARD9 with small-molecule therapeutics inhibits innate immune signaling and inflammatory response to pneumocystis carinii β-glucans. Antimicrob Agents Chemother (2020) 64(11). doi: 10.1128/aac.01210-20
66. Arts RJW, Carvalho A, La Rocca C, Palma C, Rodrigues F, Silvestre R, et al. Immunometabolic pathways in BCG-induced trained immunity. Cell Rep (2016) 17(10):2562–71. doi: 10.1016/j.celrep.2016.11.011
Keywords: Pneumocystis, β-glucan, inflammatory, initial immunity, therapy
Citation: Wang M, Zhang Z, Dong X and Zhu B (2023) Targeting β-glucans, vital components of the Pneumocystis cell wall. Front. Immunol. 14:1094464. doi: 10.3389/fimmu.2023.1094464
Received: 10 November 2022; Accepted: 16 January 2023;
Published: 09 February 2023.
Edited by:
Sha Lu, Sun Yat-sen Memorial Hospital, ChinaReviewed by:
Flavio Vieira Loures, Federal University of São Paulo, BrazilDaniel Alford Powell, University of Arizona, United States
Copyright © 2023 Wang, Zhang, Dong and Zhu. This is an open-access article distributed under the terms of the Creative Commons Attribution License (CC BY). The use, distribution or reproduction in other forums is permitted, provided the original author(s) and the copyright owner(s) are credited and that the original publication in this journal is cited, in accordance with accepted academic practice. No use, distribution or reproduction is permitted which does not comply with these terms.
*Correspondence: Biao Zhu, emh1YmlhbzEyMDdAemp1LmVkdS5jbg==
†These authors have contributed equally to this work and share first authorship