- Jenner Institute, University of Oxford, Oxford, United Kingdom
Controlled Human Infection Models (CHIMs) involve deliberately exposing healthy human volunteers to a known pathogen, to allow the detailed study of disease processes and evaluate methods of treatment and prevention, including next generation vaccines. CHIMs are in development for both tuberculosis (TB) and Covid-19, but challenges remain in their ongoing optimisation and refinement. It would be unethical to deliberately infect humans with virulent Mycobacteria tuberculosis (M.tb), however surrogate models involving other mycobacteria, M.tb Purified Protein Derivative or genetically modified forms of M.tb either exist or are under development. These utilise varying routes of administration, including via aerosol, per bronchoscope or intradermal injection, each with their own advantages and disadvantages. Intranasal CHIMs with SARS-CoV-2 were developed against the backdrop of the evolving Covid-19 pandemic and are currently being utilised to both assess viral kinetics, interrogate the local and systemic immunological responses post exposure, and identify immune correlates of protection. In future it is hoped they can be used to assess new treatments and vaccines. The changing face of the pandemic, including the emergence of new virus variants and increasing levels of vaccination and natural immunity within populations, has provided a unique and complex environment within which to develop a SARS-CoV-2 CHIM. This article will discuss current progress and potential future developments in CHIMs for these two globally significant pathogens.
Introduction
Controlled human infection models (CHIMs) involve the deliberate inoculation of volunteers with a pathogen under carefully controlled conditions, facilitating detailed study of host-pathogen immunobiology. Validated models can then be used to expedite the development of novel vaccines and therapeutics by allowing efficacy testing in small scale clinical trials, prior to field efficacy studies. Dating back to Edward Jenner’s 18th century smallpox experiments, historically, the ethical conduct of CHIMs has been controversial. With the implementation of modern ethical frameworks and considered study design (Figure 1), they have proven to be a safe and efficacious tool, particularly in the field of vaccinology, contributing to the development of vaccines for malaria, influenza, typhoid and cholera (1–4).
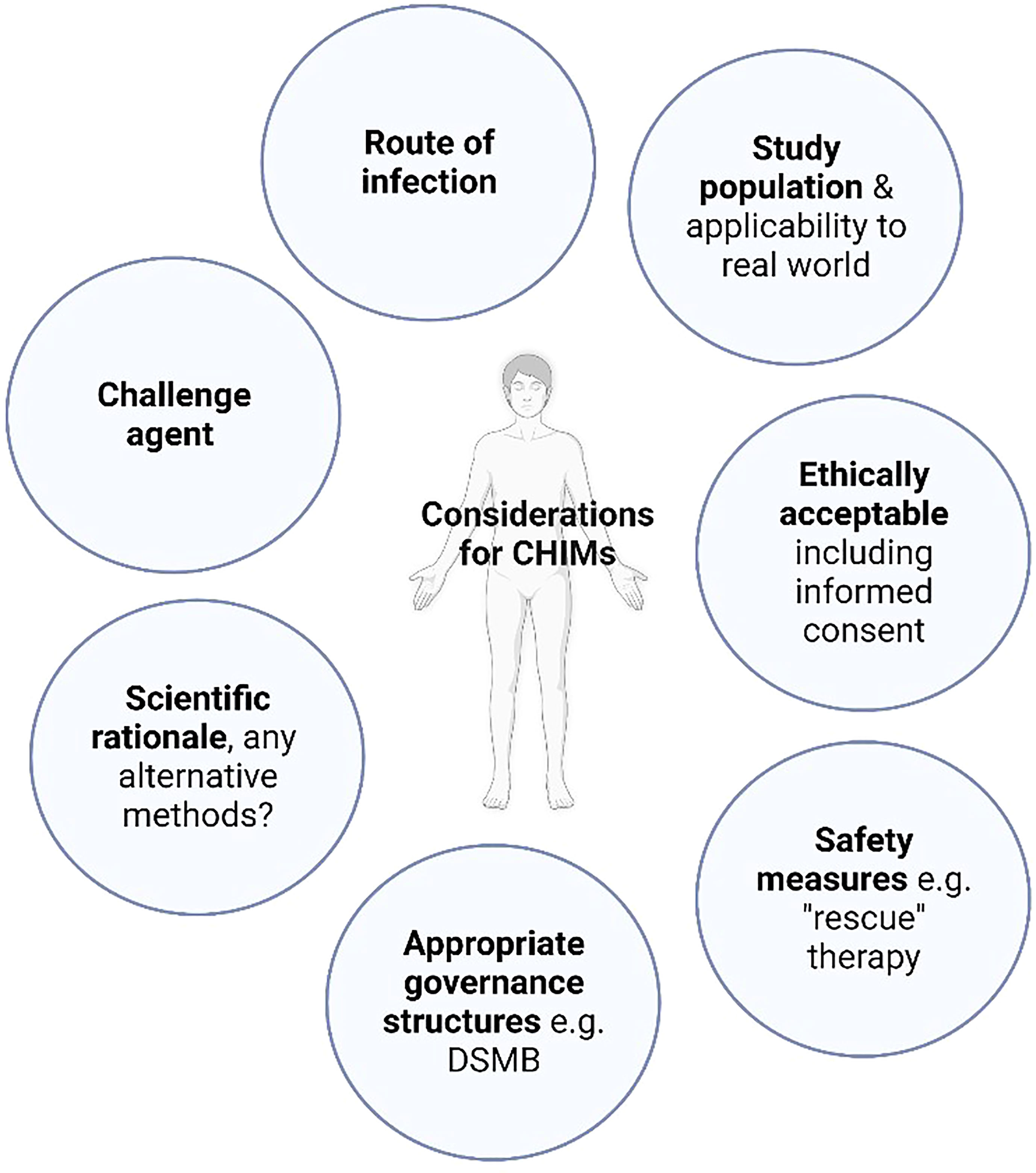
Figure 1 Controlled human infection model design. A common framework of considerations for CHIM design should be employed. “Rescue” therapy: treatment employed in CHIMs either to prevent the progression of volunteer symptoms experienced beyond mild disease or to abrogate infection, DSMB: Data safety monitoring board.
Tuberculosis (TB) remains a major global health issue, second only to COVID-19 as the leading cause of death from a single infectious pathogen (5). The COVID pandemic has itself reversed decades of progress towards meeting global TB reduction targets and new tools to combat TB are urgently needed (6, 7). Whilst astonishing research efforts worldwide have rapidly led to multiple licensed COVID-19 vaccines and therapeutics (8, 9), the ongoing potential of the virus to mutate, coupled with changing population immunity, means we cannot be complacent in our quest to develop new scientific tools and evaluate next generation vaccines and treatments. CHIMs against these two different, but both highly consequential, respiratory pathogens could be harnessed to help accelerate progress.
Tuberculosis controlled human infection models
Background and need for a TB CHIM
The only licenced vaccine against TB, Bacillus-Calmette Guérin (BCG), provides good protection against severe forms of infant TB, but highly variable efficacy against pulmonary TB and therefore limited impact on disease transmission. Ongoing challenges also exist in the accurate diagnosis of both TB infection and active disease, increasing drug resistance and treatment burden even for fully sensitive disease (10). Despite huge research efforts, developments in all of these areas are hampered by gaps in our understanding of intricate host-pathogen interactions, the complex spectrum of disease states that cannot be replicated fully in animal models and lack of defined immune correlates of protection (CoP). Judicious use of a mycobacterial CHIM could help facilitate advances in many of these domains, as a complement to animal and field studies (11). For example, a mycobacterial CHIM could enable the prioritisation of vaccine candidates that most effectively control mycobacterial growth, prior to larger, more costly field efficacy studies. Samples from such a CHIM could also be used to interrogate immune parameters that correlate with control after a defined timepoint infection, with any positive steps towards finding a validated TB immune CoP proving potentially transformative.
Current and future approaches to developing a TB CHIM
Intentionally infecting humans with virulent Mycobacterium tuberculosis (M.tb) would not be ethical, with the potential for significant morbidity and mortality. Even if these are avoided, long treatment duration with the risk of significant drug side effects, risk of M.tb transmission to others, inability to prove cure at the end of treatment and possibility of disease recurrence are all substantial arguments against a CHIM with wild-type M.tb. Therefore, researchers must pursue the use of alternative challenge agents (Summarised in Table 1), aiming to address key scientific questions with an acceptable risk profile to both volunteers and the wider community.
The tuberculin skin test (TST), where tuberculin Purified Protein Derivative (PPD) is injected intradermally, has traditionally been used as a diagnostic test for latent TB infection (LTBI). It has been employed as a challenge agent to investigate immunological responses to mycobacterial antigens at the site of skin challenge, for example identifying exaggerated Th17 responses in those with active TB disease as a potential target for host directed therapies (12, 13, 24). PPD has also been used to assess local respiratory mucosal responses following intrabronchial instillation (14, 15, 21). Whilst these methods may contribute to our knowledge of mycobacterial immunopathogenesis they cannot be utilised directly to assess efficacy of vaccines or therapeutics.
A CHIM that is to be used to evaluate vaccine efficacy requires a live replicating organism, for example an attenuated strain of mycobacteria. BCG itself is such a live attenuated mycobacteria, initially derived via passage from Mycobacterium bovis (M. Bovis), that does not cause disease or latency in healthy humans (25). The loss of key virulence genes encoded in the Region of Difference 1 (RD1) during this process confers the advantageous safety profile of BCG but means the full immunopathogenic pathways of M.tb are not entirely replicated and it could not be used to evaluate vaccines which incorporate RD1-encoded antigens, such as ESAT-6 and CFP-10. However, BCG has been shown to induce similar canonical CD4+ T cell-mediated immune responses to M. tb in humans (26) and assessment of vaccine efficacy using a BCG challenge in animal models are comparable to results obtained using M.tb as the challenge agent (27, 28). BCG manufactured under good manufacturing practice (GMP) conditions for human use is readily available and this therefore represents the only live replicating TB CHIM agent currently available (11).
CHIMs using intradermal (ID) BCG as a mycobacterial challenge agent have been developed and are able to detect a known BCG vaccine effect in animals and humans (17, 18, 27–29). The ID route allows straightforward quantification of mycobacteria from an easily accessible site, for example via minimally invasive punch skin biopsies (17). However, the natural route of M.tb infection is via the respiratory tract and initial pathogen interactions with the specialised host respiratory mucosal system cannot be evaluated using an ID CHIM.
Efforts are ongoing to develop pulmonary CHIMs that more closely mimic the natural route of M.tb infection. BCG delivered via aerosol (Clinicatrials.gov NCT02709278, NCT03912207, NCT04777721) or instilled directly into the lungs per bronchoscope (21) are both being evaluated and have been shown to be safe and well tolerated. A defined timepoint pulmonary mycobacterial infection would allow examination of localised mucosal immunology and the relationship to induced system responses, which are key areas of research interest. Vaccines or therapeutics tested using these CHIMs would have the advantage of accounting for the contribution of the specialised respiratory mucosa in conferring protective immunity. However, sampling of the respiratory mucosa for immunological interrogation and quantification of recoverable BCG in pulmonary models are both more complex and invasive than in skin models (30).
Following on from initial studies using BCG, live mycobacterial CHIMs could be enhanced by the use of rationally attenuated genetically modified organisms. BCG which has been modified, for example to include a fluorophore reporter gene or exhaled volatile compound detectable by mass spectrometry could reduce the need for invasive sampling for mycobacterial recovery and quantification (31, 32). Use of current live vaccine candidates such as MTBVAC, a rationally attenuated form of M.tb (33, 34) or VPM1002, a recombinant BCG (35, 36), could allow investigation of the antigens or immunological pathways missing from BCG.
Whilst it is some way off from clinical evaluation, efforts are underway to develop a conditionally replicating M.tb strain with a genetically inserted suicide mechanism. This would aim to recapitulate the initial immunological mechanisms of M.tb, whilst ensuring complete eradication at a predefined timepoint and, if successful, could hugely advance the field of human TB study (22, 31).
Finally, for a TB CHIM to be truly useful, it should be safe, acceptable and deliverable in TB endemic populations and settings. Different environmental exposures, level of nutrition, microbiome composition, prior exposure to mycobacteria and prevalence of co-infections are just some of the known factors impacting vaccine efficacy. Utilising an ethically appropriate CHIM in endemic settings would ensure vaccines are tested in relevant populations (11, 37).
SARS-CoV-2 controlled human infection models
Background and need for a SARS-CoV-2 CHIM
Early in the COVID-19 pandemic, the World Health Organisation (WHO) acknowledged the potential benefits of a SARS-CoV-2 CHIM, for example to allow rapid prioritisation of vaccine candidates. A working group was promptly established to consider the practicalities, feasibility and ethics (38). Initial expert consensus was divided with concern about the lack of a suitable “rescue” therapy, potential for severe illness and high transmissibility, as well as the benefit and applicability of such a model over field studies (39).
Accruing data suggested that infection of young, healthy adults in whom disease was generally much milder could be justifiable. This prompted UK manufacture of a challenge virus under GMP conditions and development and rigorous ethical review of study protocols for both a UK SARS-CoV-2 naïve CHIM (NCT04865237) and one in previously infected volunteers (NCT04864548) (40). GMP manufacture of challenge viruses is a time-consuming process and enrolment did not commence in these studies until March (NCT04865237) and May, 2021 (NCT04864548) respectively, by which point several highly efficacious vaccine candidates were being deployed in the UK population (41, 42).
Despite the widespread availability of highly effective vaccines against SARS-CoV-2, there remains a justifiable role for SARS CoV-2 CHIMs. A clear advantage of a CHIM over natural infection field studies is the known-timepoint of infection; allowing the detailed characterisation of both viral kinetics and the host immune response post-exposure. The dose of virus can also be carefully controlled and adjusted, providing crucial information about how the infectious dose affects the clinical and immunological response to the virus. Importantly, CHIMs also allow the collection of pre-exposure samples. These baseline samples can be assessed against clinical outcomes to identify immune correlates of protection (CoP).
Whilst current literature clearly defines the role of neutralising antibodies (nABs) as a correlate for sterilising immunity against SARS-CoV-2 (43–46), emerging evidence, particularly with the evolution of Variants of Concern (VoC) that escape nABs, is that the immune response to SARS-CoV-2 is more complex. Cell-mediated immunity, memory B cells and non-neutralising Fc-mediated effector functions may all play a role (47–53). Local mucosal immune responses have demonstrably protected against infection from other respiratory pathogens (54, 55) but mucosal immunity against SARS-CoV-2 remains poorly described in the literature. A CHIM with infection at a controlled timepoint allows the detailed interrogation of all aspects of the protective immune response, particularly the early host mucosal responses that are often missed in natural field infection studies.
Furthermore, the ability to control confounders such as inoculum strain, route of exposure, viral load and patient heterogeneity in a CHIM allows direct comparison of vaccine and therapeutic candidates as well as dosing regimens. With the roll-out of successful vaccines, it is unfeasible and unethical to maintain an unvaccinated placebo group for the testing of new vaccine candidates. Non-inferiority trials require large sample sizes and sufficient naturally acquired infection which can be time consuming and expensive. A CHIM could be of particular use in assessing novel vaccines, including those developed to be mucosally-delivered, which may have differing end-points (such as prevention of infection or viral shedding) that would be extremely difficult to study without a defined timepoint of infection. Whilst field studies are considered gold standard for vaccine licensure, there are instances where CHIMs have been used directly as proof of efficacy (4).
Current and future approaches to developing a SARS-CoV-2 CHIM
To date there are three registered SARS-CoV-2 CHIMs (Summarised in Table 1). The wild-type (pre-Alpha) SARS-CoV-2 CHIM in healthy, seronegative, UK 18-29-year olds demonstrated infection in 53% (18/34) of volunteers using a low inoculum dose of 10TCID50 (50% tissue culture infectious dose). Challenge was safe and well-tolerated with no evidence of lower respiratory tract involvement, although smell disturbance was common and prolonged in a small number of volunteers (23). Killingley et al. were able to accurately delineate the viral kinetics of primary infection and identified differences in viral dynamics depending on swab site. Viable virus measured by focus forming assay (FFA) persisted for on average 10 (maximum of 12) days, consistent with pre-Alpha isolation guidance (23). FFA was shown to closely correlate with lateral flow antigen (LFA) tests performed on the same swab samples. This first in human SARS-CoV-2 CHIM has demonstrated the broad utility of CHIMs, strengthening confidence in the public health measures (such as isolation periods and use of LFA tests) employed in the UK. Exploration of immune correlates of protection in this seronegative cohort, such as cross-reactive responses from seasonal coronaviruses, is ongoing.
With increasing global seroprevalence to SARS-CoV-2 from vaccination and/or infection (56), a seropositive SARS-CoV-2 CHIM is needed in order to facilitate future vaccine and therapeutic development in volunteers that reflects real world immunity. Successfully establishing a re-infection model additionally allows the identification of both local and systemic immune markers attained via the infection or vaccination process that are protective against re-infection, which could inform future public health strategies as well as design of therapeutics and vaccines.
Ongoing use of a pre-Alpha strain for a seropositive CHIM has several potential issues. Field data suggests that acquired immunity (either by vaccination, natural infection or both – hybrid immunity) offers strong resistance to homologous re-infection (57, 58). Achieving consistent infection rates may therefore prove more difficult than in a study of naïve participants.
Much of knowledge of re-infection rates was obtained prior to the emergence of variants such as Delta and subsequently Omicron, which are known to escape immunity. Both variants have antigenic divergence due to mutations in the spike protein and have been shown to demonstrate reduced neutralisation titres compared to pre-Alpha strains in vaccinated and hybrid cohorts (59–62). One approach which may circumvent any difficulty achieving infection in seropositive volunteers is to use variants more likely to cause breakthrough infections as the challenge agent, such as the Delta variant (isrctn.com ISRCTN94747181). Manufacture of an Omicron challenge agent is also being pursued (63).
There are pros and cons to the use of Delta or Omicron in a CHIM. Neutralisation against the Omicron variant is more markedly reduced than delta and associated with a higher rate of breakthrough infections (47, 61, 64) making it plausible that it would be easier to achieve infection in a CHIM. Omicron may also be a safer challenge agent demonstrating milder disease severity and reduced lower respiratory tract disease (65–68). However, the shorter infection course seen with the Omicron variant may also make it difficult to assess post-infection therapeutics (69).
Studies using currently prevalent variants are arguably more relevant both for the development pipeline of vaccines and therapeutics and understanding CoP. Limitations to this approach are that manufacturing a new challenge strain under GMP conditions takes at least 6 months (70). Furthermore, any specific clinical risks of that variant need to be understood from real world data prior to use in an ethically sound CHIM. The high incidence of SARS-CoV-2 and associated viral replication globally has resulted in the relatively rapid acquisition of mutations and development of new VoCs, meaning that by the time an inoculum strain is ready for use in a CHIM it may no longer be the dominant variant in the real world. However, developing several CHIMs that use variants derived from different lineages will enable broad assessment of different therapeutics and vaccines.
Discussion
Tuberculosis and Covid-19 represent two deadly, but distinct, respiratory diseases. Whilst highly efficacious vaccines against Covid-19 were developed at unprecedented speed against the backdrop of the evolving pandemic, progress in improving on the limited overall efficacy of the BCG vaccine against TB has been much slower. All possible research approaches that can be utilised to expedite progress should be harnessed to improve this situation. We must also remain vigilant against the potential for further SARS-CoV-2 mutations and need to have methods available to be able to rapidly assess new vaccines and therapeutics.
CHIMs may prove to be useful tools in our armoury against both of these pandemic pathogens, despite their unique situations and challenges. There are no validated CoP in TB and use of CHIMs to interrogate human immunological responses following a defined timepoint infection could increase our understanding in this area. Whilst validated CoP, for example in the form of nABs, do exist for Covid-19, these are clearly not the only factor contributing to immunity, particularly against initial infection and transmissibility. The early host mucosal immune response to M.tb and SARS-CoV-2 represent an important knowledge gap for both pathogens. The ability to abort infection at its point of entry could prevent LTBI and provide epidemic control by blocking onward transmission of SARS-CoV-2. These initial mucosal responses can only really be studied in an experimental setting with a known timepoint of infection.
Identification of the ideal challenge agent for a CHIM remains an issue for both of these diseases. Use of virulent M.tb is unethical and therefore any deployable TB CHIM will only provide partial information about the true protective efficacy of a tested vaccine or therapeutic against M.tb. Progress is underway to identify surrogate agents which could be utilised and, given the differing advantages and disadvantages of various agents and routes of challenge (see Table 1), it may be that a combination of the available options will need to be employed depending on the exact question to be answered or until new modified organisms are available (11). In Covid-19, viral mutations mean that manufacture of a challenge agent may lag behind currently circulating variants. Utilisation of a variant with optimal challenge properties (for example, high levels of infectivity with low potential to cause severe disease), such as those seen in the Omicron variant may be one approach. Or it may be that, similarly to TB, a range of challenge agents could be developed and utilised depending on the specific question to be answered.
A CHIM for the purpose of novel vaccine and therapeutic evaluation needs to be able to accurately quantify pathogen load. This is undertaken with quantitative PCR (qPCR) on minimally invasive samples from the oral or nasal mucosa for SARS-CoV-2. The sampling and quantification of mycobacteria, particularly from the respiratory tract, for a TB CHIM remains much less straightforward, for example due to the fastidious and slow growing nature of mycobacteria and colonisation of the respiratory tract with organisms including non-tuberculous mycobacteria. One potential entirely non-invasive solution under development is the use of specially adapted face masks, containing a collection matrix to sample exhaled pathogens, which are then detected via qPCR. Initially developed as a potential diagnostic tool for TB (71), these are currently being evaluated in both TB (Clinicaltrials.gov NCT03912207) and COVID (Clinicaltrials.gov NCT04864548), highlighting how solutions initially designed for one pathogen can be utilised in another.
Applicability of CHIMs utilised in young, healthy adults to real world populations of interest is an area of consideration for both pathogens. In TB, there is a drive to deliver CHIMs in TB endemic settings, to ensure information derived and interventions tested are relevant to eventual target populations (11). In Covid-19, applicability of results obtained in a CHIM to those most at risk of disease, including the elderly and immunocompromised, is not yet known. There may be fundamental differences in the way these populations respond to the virus that limit the generalisability of a CHIM conducted purely in young, immunocompetent adults. Interestingly, in more established respiratory pathogens, efforts are underway to develop safe CHIMs in older adults (72), but it is not at all clear that this would be ethical or feasible with SARS-CoV-2
With multiple studies ongoing to develop and optimise CHIMs within both TB and Covid-19, this is an area of considerable scientific interest and promise. Momentum gained in research during the Covid-19 pandemic should be harnessed to ensure CHIMs for these, and other, pathogens continue to be developed and to exploit their full potential, in particular the fields of vaccine development and to further our understanding of host-pathogen immunobiology.
Author contributions
HMo and SJ contirubuted equally to this work and share first authorship. HMo and SJ wrote the first draft of the manuscript. All authors contributed to the article and approved the submitted version.
Funding
The research was funded/supported by the National Institute for Health Research (NIHR) Oxford Biomedical Research Centre (BRC). The funder was not involved in the study design, collection, analysis, interpretation of data, the writing of this article or the decision to submit it for publication.
Conflict of interest
HMcS is a Wellcome Trust Investigator grant code WT 206331/Z/17/Z.
The remaining authors declare that the research was conducted in the absence of any commercial or financial relationships that could be construed as a potential conflict of interest.
Publisher’s note
All claims expressed in this article are solely those of the authors and do not necessarily represent those of their affiliated organizations, or those of the publisher, the editors and the reviewers. Any product that may be evaluated in this article, or claim that may be made by its manufacturer, is not guaranteed or endorsed by the publisher.
Author disclaimer
The views expressed are those of the author(s) and not necessarily those of the NHS, the NIHR, or the Department of Health.
References
1. Kester KE, Cummings JF, Ofori-Anyinam O, Ockenhouse CF, Krzych U, Moris P, et al. Randomized, double-blind, phase 2a trial of falciparum malaria vaccines RTS,S/AS01B and RTS,S/AS02A in malaria-naive adults: safety, efficacy, and immunologic associates of protection. J Infect Dis (2009) 200(3):337–46. doi: 10.1086/600120
2. Clements ML, Betts RF, Murphy BR. Advantage of live attenuated cold-adapted influenza a virus over inactivated vaccine for A/Washington/80 (H3N2) wild-type virus infection. Lancet (1984) 1(8379):705–8. doi: 10.1016/S0140-6736(84)92222-0
3. Jin C, Gibani MM, Moore M, Juel HB, Jones E, Meiring J, et al. Efficacy and immunogenicity of a vi-tetanus toxoid conjugate vaccine in the prevention of typhoid fever using a controlled human infection model of salmonella typhi: a randomised controlled, phase 2b trial. Lancet (2017) 390(10111):2472–80. doi: 10.1016/S0140-6736(17)32149-9
4. Chen WH, Cohen MB, Kirkpatrick BD, Brady RC, Galloway D, Gurwith M, et al. Single-dose live oral cholera vaccine CVD 103-HgR protects against human experimental infection with vibrio cholerae O1 El tor. Clin Infect Dis (2016) 62(11):1329–35. doi: 10.1093/cid/ciw145
5. WHO. World health organisation: global tuberculosis report 2022 (2022). Available at: https://www.who.int/teams/global-tuberculosis-programme/tb-reports/global-tuberculosis-report-2022.
6. Kirby T. Global tuberculosis progress reversed by COVID-19 pandemic. Lancet Respir Med (2021) 9(12):e118–9. doi: 10.1016/S2213-2600(21)00496-3
7. Giovanni Battista M, Pei Min T, Jan-Willem A, Justin D, Marina T, Fatma A, et al. Gauging the impact of the COVID-19 pandemic on tuberculosis services: a global study. Eur Respir J (2021) 58(5):2101786. doi: 10.1136/bmj.m3379
8. Lamontagne F, Agarwal A, Rochwerg B, Siemieniuk RA, Agoritsas T, Askie L, et al. A living WHO guideline on drugs for covid-19. Bmj (2020) 370:m3379. doi: 10.1136/bmj.m3379
9. Ramchandani R, Kazatchkine M, Liu J, Sudan P, Dybul M, Matsoso P, et al. Vaccines, therapeutics, and diagnostics for covid-19: redesigning systems to improve pandemic response. Bmj (2021) 375:e067488. doi: 10.1136/bmj-2021-067488
10. Sakamoto H, Lee S, Ishizuka A, Hinoshita E, Hori H, Ishibashi N, et al. Challenges and opportunities for eliminating tuberculosis – leveraging political momentum of the UN high-level meeting on tuberculosis. BMC Public Health (2019) 19(1):76. doi: 10.1186/s12889-019-6399-8
11. Gordon S, Sichone S, Chirwa A, Hazenberg P, Kafuko Z, Ferreira D, et al. Practical considerations for a TB controlled human infection model (TB-CHIM); the case for TB-CHIM in Africa, a systematic review of the literature and report of 2 workshop discussions in UK and Malawi [version 1; peer review: 2 approved, 1 approved with reservations]. Wellcome Open Res (2023) 8(71). doi: 10.12688/wellcomeopenres.18767.1
12. Tomlinson GS, Cashmore TJ, Elkington PT, Yates J, Lehloenya RJ, Tsang J, et al. Transcriptional profiling of innate and adaptive human immune responses to mycobacteria in the tuberculin skin test. Eur J Immunol (2011) 41(11):3253–60. doi: 10.1002/eji.201141841
13. Pollara G, Turner CT, Rosenheim J, Chandran A, Bell LCK, Khan A, et al. Exaggerated IL-17A activity in human in vivo recall responses discriminates active tuberculosis from latent infection and cured disease. Sci Transl Med (2021) 13(592). doi: 10.1126/scitranslmed.abg7673
14. Silver RF, Zukowski L, Kotake S, Li Q, Pozuelo F, Krywiak A, et al. Recruitment of antigen-specific Th1-like responses to the human lung following bronchoscopic segmental challenge with purified protein derivative of mycobacterium tuberculosis. Am J Respir Cell Mol Biol (2003) 29(1):117–23. doi: 10.1165/rcmb.4840
15. Walrath J, Zukowski Krywiak L, Silver A RF. Resident Th1-like effector memory cells in pulmonary recall responses to mycobacterium tuberculosis. Am J Respir Cell Mol Biol (2005) 33(1):48–55. doi: 10.1165/rcmb.2005-0060OC
16. Schreiber F, Huo Z, Giemza R, Woodrow M, Fenner N, Stephens Z, et al. An investigation of clinical and immunological events following repeated aerodigestive tract challenge infections with live mycobacterium bovis bacille calmette guérin. Vaccine (2010) 28(33):5427–31. doi: 10.1016/j.vaccine.2010.06.005
17. Minassian AM, Satti I, Poulton ID, Meyer J, Hill AV, McShane H. A human challenge model for mycobacterium tuberculosis using mycobacterium bovis bacille calmette-guerin. J Infect Dis (2012) 205(7):1035–42. doi: 10.1093/infdis/jis012
18. Harris SA, Meyer J, Satti I, Marsay L, Poulton ID, Tanner R, et al. Evaluation of a human BCG challenge model to assess antimycobacterial immunity induced by BCG and a candidate tuberculosis vaccine, MVA85A, alone and in combination. J Infect Dis (2014) 209(8):1259–68. doi: 10.1093/infdis/jit647
19. Minhinnick A, Harris S, Wilkie M, Peter J, Stockdale L, Manjaly-Thomas ZR, et al. Optimization of a human bacille calmette-guérin challenge model: a tool to evaluate antimycobacterial immunity. J Infect Dis (2016) 213(5):824–30. doi: 10.1093/infdis/jiv482
20. Blazevic A, Xia M, Turan A, Tennant J, Hoft DF. Pilot studies of a human BCG challenge model. Tuberculosis (Edinb) (2017) 105:108–12. doi: 10.1016/j.tube.2017.05.001
21. Davids M, Pooran A, Hermann C, Mottay L, Thompson F, Cardenas J, et al. A human lung challenge model to evaluate the safety and immunogenicity of PPD and live bacillus calmette-guérin. Am J Respir Crit Care Med (2020) 201(10):1277–91. doi: 10.1164/rccm.201908-1580OC
22. Jackson S, McShane H. Challenges in developing a controlled human tuberculosis challenge model. (2022) Springer Berlin Heidelberg: Berlin, Heidelberg p. 1–27.
23. Killingley B, Mann AJ, Kalinova M, Boyers A, Goonawardane N, Zhou J, et al. Safety, tolerability and viral kinetics during SARS-CoV-2 human challenge in young adults. Nat Med (2022) 28(5):1031–41. doi: 10.1038/s41591-022-01780-9
24. Bell LC, Pollara G, Pascoe M, Tomlinson GS, Lehloenya RJ, Roe J, et al. In vivo molecular dissection of the effects of HIV-1 in active tuberculosis. PloS Pathog (2016) 12(3):e1005469. doi: 10.1371/journal.ppat.1005469
25. Calmette. A. L’infection bacillaire et la tuberculose chez l’homme et chez les animaux (1920). Paris: Masson.
26. Hanekom WA. The immune response to BCG vaccination of newborns. Ann N Y Acad Sci 2005 (1062) p:69–78. doi: 10.1196/annals.1358.010
27. Minassian AM, Ronan EO, Poyntz H, Hill AV, McShane H. Preclinical development of an in vivo BCG challenge model for testing candidate TB vaccine efficacy. PloS One (2011) 6(5):e19840. doi: 10.1371/journal.pone.0019840
28. Harris SA, White A, Stockdale L, Tanner R, Sibley L, Sarfas C, et al. Development of a non-human primate BCG infection model for the evaluation of candidate tuberculosis vaccines. Tuberculosis (2018) 108:99–105. doi: 10.1016/j.tube.2017.11.006
29. Villarreal-Ramos B, Berg S, Chamberlain L, McShane H, Hewinson RG, Clifford D, et al. Development of a BCG challenge model for the testing of vaccine candidates against tuberculosis in cattle. Vaccine (2014) 32(43):5645–9. doi: 10.1016/j.vaccine.2014.08.009
30. Morrison H, McShane H. Local pulmonary immunological biomarkers in tuberculosis. Front Immunol (2021) 12:640916. doi: 10.3389/fimmu.2021.640916
31. Bekeredjian-Ding I, Van Molle W, Baay M, Neels P, Berthels N, Conrad C, et al. Human challenge trial workshop: focus on quality requirements for challenge agents, langen, Germany, October 22, 2019. Biologicals (2020) 66:53–61. doi: 10.1016/j.biologicals.2020.04.005
32. Basu Roy R, Sambou B, Uhía I, Roetynck S, Robertson BD, Kampmann B, et al. An auto-luminescent fluorescent BCG whole blood assay to enable evaluation of paediatric mycobacterial responses using minimal blood volumes. Front Pediatr (2019) 7:151. doi: 10.3389/fped.2019.00151
33. Dijkman K, Aguilo N, Boot C, Hofman SO, Sombroek CC, Vervenne RAW, et al. Pulmonary MTBVAC vaccination induces immune signatures previously correlated with prevention of tuberculosis infection. Cell Rep Med (2021) 2(1):100187. doi: 10.1016/j.xcrm.2020.100187
34. Tameris M, Mearns H, Penn-Nicholson A, Gregg Y, Bilek N, Mabwe S, et al. Live-attenuated mycobacterium tuberculosis vaccine MTBVAC versus BCG in adults and neonates: a randomised controlled, double-blind dose-escalation trial. Lancet Respir Med (2019) 7(9):757–70. doi: 10.1016/S2213-2600(19)30251-6
35. Loxton AG, Knaul JK, Grode L, Gutschmidt A, Meller C, Eisele B, et al. Safety and immunogenicity of the recombinant mycobacterium bovis BCG vaccine VPM1002 in HIV-unexposed newborn infants in south Africa. Clin Vaccine Immunol (2017) 24(2). doi: 10.1128/CVI.00439-16
36. Nieuwenhuizen NE, Kulkarni PS, Shaligram U, Cotton MF, Rentsch CA, Eisele B, et al. The recombinant bacille calmette-guérin vaccine VPM1002: ready for clinical efficacy testing. Front Immunol (2017) 8:1147. doi: 10.3389/fimmu.2017.01147
37. Gordon SB, Rylance J, Luck A, Jambo K, Ferreira DM, Manda-Taylor L, et al. A framework for controlled human infection model (CHIM) studies in Malawi: report of a wellcome trust workshop on CHIM in low income countries held in Blantyre, Malawi. Wellcome Open Res (2017) 2:70. doi: 10.12688/wellcomeopenres.12256.1
38. Jamrozik E, Littler K, Bull S, Emerson C, Kang G, Kapulu M, et al. Key criteria for the ethical acceptability of COVID-19 human challenge studies: report of a WHO working group. Vaccine (2021) 39(4):633–40. doi: 10.1016/j.vaccine.2020.10.075
39. Levine MM, Abdullah S, Arabi YM, Darko DM, Durbin AP, Estrada V, et al. Viewpoint of a WHO advisory group tasked to consider establishing a closely-monitored challenge model of coronavirus disease 2019 (COVID-19) in healthy volunteers. Clin Infect Dis (2021) 72(11):2035–41. doi: 10.1093/cid/ciaa1290
40. Rapeport G, Smith E, Gilbert A, Catchpole A, McShane H, Chiu C. SARS-CoV-2 human challenge studies - establishing the model during an evolving pandemic. N Engl J Med (2021) 385(11):961–4. doi: 10.1056/NEJMp2106970
41. Voysey M, Clemens SAC, Madhi SA, Weckx LY, Folegatti PM, Aley PK, et al. Safety and efficacy of the ChAdOx1 nCoV-19 vaccine (AZD1222) against SARS-CoV-2: an interim analysis of four randomised controlled trials in Brazil, south Africa, and the UK. Lancet (2021) 397(10269):99–111. doi: 10.1016/S0140-6736(20)32661-1
42. Kleinwaks G, Schmit V, Morrison J. Considering human challenge trials for tuberculosis vaccine development. Vaccine (2021) 2:173–4. doi: 10.1016/j.vaccine.2021.11.024
43. Garcia-Beltran WF, Lam EC, Astudillo MG, Yang D, Miller TE, Feldman J, et al. COVID-19-neutralizing antibodies predict disease severity and survival. Cell (2021) 184(2):476–488.e11. doi: 10.1016/j.cell.2020.12.015
44. Addetia A, Crawford KHD, Dingens A, Zhu H, Roychoudhury P, Huang ML, et al. Neutralizing antibodies correlate with protection from SARS-CoV-2 in humans during a fishery vessel outbreak with a high attack rate. J Clin Microbiol (2020) 58(11). doi: 10.1128/JCM.02107-20
45. Bergwerk M, Gonen T, Lustig Y, Amit S, Lipsitch M, Cohen C, et al. Covid-19 breakthrough infections in vaccinated health care workers. N Engl J Med (2021) 385(16):1474–84. doi: 10.1056/NEJMoa2109072
46. Khoury DS, Cromer D, Reynaldi A, Schlub TE, Wheatley AK, Juno JA, et al. Neutralizing antibody levels are highly predictive of immune protection from symptomatic SARS-CoV-2 infection. Nat Med (2021) 27(7):1205–11. doi: 10.1038/s41591-021-01377-8
47. Garcia-Beltran WF, St Denis KJ, Hoelzemer A, Lam EC, Nitido AD, Sheehan ML, et al. mRNA-based COVID-19 vaccine boosters induce neutralizing immunity against SARS-CoV-2 omicron variant. Cell (2022) 185(3):457–466.e4. doi: 10.1016/j.cell.2021.12.033
48. Tarke A, Coelho CH, Zhang Z, Dan JM, Yu ED, Methot N, et al. SARS-CoV-2 vaccination induces immunological T cell memory able to cross-recognize variants from alpha to omicron. Cell (2022) 185:847–59.e11. doi: 10.1101/2021.12.28.474333
49. Keeton R, Tincho MB, Ngomti A, Baguma R, Benede N, Suzuki A, et al. T Cell responses to SARS-CoV-2 spike cross-recognize omicron. Nature (2022) 603(7901):488–92. doi: 10.1038/s41586-022-04460-3
50. Liu J, Chandrashekar A, Sellers D, Barrett J, Jacob-Dolan C, Lifton M, et al. Vaccines elicit highly conserved cellular immunity to SARS-CoV-2 omicron. Nature (2022) 603(7901):493–6. doi: 10.1038/s41586-022-04465-y
51. Kaplonek P, Fischinger S, Cizmeci D, Bartsch YC, Kang J, Burke JS, et al. mRNA-1273 vaccine-induced antibodies maintain fc effector functions across SARS-CoV-2 variants of concern. Immunity (2022) 55(2):355–365.e4. doi: 10.1016/j.immuni.2022.01.001
52. Wang Z, Muecksch F, Schaefer-Babajew D, Finkin S, Viant C, Gaebler C, et al. Naturally enhanced neutralizing breadth against SARS-CoV-2 one year after infection. Nature (2021) 595(7867):426–31. doi: 10.1038/s41586-021-03696-9
53. Muecksch F, Wang Z, Cho A, Gaebler C, Ben Tanfous T, DaSilva J, et al. Increased memory b cell potency and breadth after a SARS-CoV-2 mRNA boost. Nature (2022) 607(7917):128–34. doi: 10.1038/s41586-022-04778-y
54. Bagga B, Cehelsky JE, Vaishnaw A, Wilkinson T, Meyers R, Harrison LM, et al. Effect of preexisting serum and mucosal antibody on experimental respiratory syncytial virus (RSV) challenge and infection of adults. J Infect Dis (2015) 212(11):1719–25. doi: 10.1093/infdis/jiv281
55. Renegar KB, Small PA Jr, Boykins LG, Wright PF. Role of IgA versus IgG in the control of influenza viral infection in the murine respiratory tract. J Immunol (2004) 173(3):1978–86. doi: 10.4049/jimmunol.173.3.1978
56. Bergeri I, Whelan MG, Ware H, Subissi L, Nardone A, Lewis HC, et al. Global SARS-CoV-2 seroprevalence from January 2020 to April 2022: a systematic review and meta-analysis of standardized population-based studies. PloS Med (2022) 19(11):e1004107. doi: 10.1371/journal.pmed.1004107
57. Hall V, Foulkes S, Insalata F, Kirwan P, Saei A, Atti A, et al. Protection against SARS-CoV-2 after covid-19 vaccination and previous infection. New Engl J Med (2022) 386(13):1207–20. doi: 10.1056/NEJMoa2118691
58. Abu-Raddad LJ, Chemaitelly H, Coyle P, Malek JA, Ahmed AA, Mohamoud YA, et al. SARS-CoV-2 antibody-positivity protects against reinfection for at least seven months with 95% efficacy. EClinicalMedicine (2021) 35:100861. doi: 10.1016/j.eclinm.2021.100861
59. Mlcochova P, Kemp SA, Dhar MS, Papa G, Meng B, Ferreira I, et al. SARS-CoV-2 B.1.617.2 delta variant replication and immune evasion. Nature (2021) 599(7883):114–9. doi: 10.1038/s41586-021-03944-y
60. Planas D, Veyer D, Baidaliuk A, Staropoli I, Guivel-Benhassine F, Rajah MM, et al. Reduced sensitivity of SARS-CoV-2 variant delta to antibody neutralization. Nature (2021) 596(7871):276–80. doi: 10.1038/s41586-021-03777-9
61. Rössler A, Riepler L, Bante D, von Laer D, Kimpel J. SARS-CoV-2 omicron variant neutralization in serum from vaccinated and convalescent persons. New Engl J Med (2022) 386(7):698–700. doi: 10.1056/NEJMc2119236
62. Cele S, Jackson L, Khoury DS, Khan K, Moyo-Gwete T, Tegally H, et al. Omicron extensively but incompletely escapes pfizer BNT162b2 neutralization. Nature (2022) 602(7898):654–6. doi: 10.1038/s41586-021-04387-1
63. hVIVO. Covid-19 challenge models (2023). Available at: https://hvivo.com/human-challenge-models/covid-19/.
64. Goga A, Bekker L-G, Garrett N, Reddy T, Yende-Zuma N, Fairall L, et al. Breakthrough SARS-CoV-2 infections during periods of delta and omicron predominance, south Africa. Lancet (2022) 400(10348):269–71. doi: 10.1016/S0140-6736(22)01190-4
65. Wolter N, Jassat W, Walaza S, Welch R, Moultrie H, Groome M, et al. Early assessment of the clinical severity of the SARS-CoV-2 omicron variant in south Africa: a data linkage study. Lancet (2022) 399(10323):437–46. doi: 10.1016/S0140-6736(22)00017-4
66. Christensen PA, Olsen RJ, Long SW, Snehal R, Davis JJ, Ojeda Saavedra M, et al. Signals of significantly increased vaccine breakthrough, decreased hospitalization rates, and less severe disease in patients with coronavirus disease 2019 caused by the omicron variant of severe acute respiratory syndrome coronavirus 2 in Houston, Texas. Am J Pathol (2022) 192(4):642–52. doi: 10.1016/j.ajpath.2022.01.007
67. Hui KPY, Ho JCW, Cheung MC, Ng KC, Ching RHH, Lai KL, et al. SARS-CoV-2 omicron variant replication in human bronchus and lung ex vivo. Nature (2022) 603:715–20. doi: 10.1038/s41586-022-04479-6
68. Tsakok MT, Watson RA, Saujani SJ, Kong M, Xie C, Peschl H, et al. Reduction in chest CT severity and improved hospital outcomes in SARS-CoV-2 omicron compared with delta variant infection. Radiology (2023) 306(1):261–9. doi: 10.1148/radiol.220533
69. Wu Y, Guo Z, Yuan J, Cao G, Wang Y, Gao P, et al. Duration of viable virus shedding and polymerase chain reaction positivity of the SARS-CoV-2 omicron variant in the upper respiratory tract: a systematic review and meta-analysis. Int J Infect Dis (2023) 129:228–35. doi: 10.1016/j.ijid.2023.02.011
70. Catchpole AP, Fullen DJ, Noulin N, Mann A, Gilbert AS, Lambkin-Williams R. The manufacturing of human viral challenge agents for use in clinical studies to accelerate the drug development process. BMC Res Notes (2018) 11(1):620. doi: 10.1186/s13104-018-3636-7
71. Williams CM, Abdulwhhab M, Birring SS, De Kock E, Garton NJ, Townsend E, et al. Exhaled mycobacterium tuberculosis output and detection of subclinical disease by face-mask sampling: prospective observational studies. Lancet Infect Dis (2020) 20(5):607–17. doi: 10.1016/S1473-3099(19)30707-8
72. Ascough S, Dayananda P, Kalyan M, Kuong SU, Gardener Z, Bergstrom E, et al. Divergent age-related humoral correlates of protection against respiratory syncytial virus infection in older and young adults: a pilot, controlled, human infection challenge model. Lancet Healthy Longev (2022) 3(6):e405–16. doi: 10.1016/S2666-7568(22)00103-9
Keywords: tuberculosis, COVID-19, CHIM, controlled human infection, challenge models
Citation: Morrison H, Jackson S and McShane H (2023) Controlled human infection models in COVID-19 and tuberculosis: current progress and future challenges. Front. Immunol. 14:1211388. doi: 10.3389/fimmu.2023.1211388
Received: 24 April 2023; Accepted: 09 May 2023;
Published: 25 May 2023.
Edited by:
Alex Sigal, Africa Health Research Institute (AHRI), South AfricaReviewed by:
Zhou Xing, McMaster University, CanadaCopyright © 2023 Morrison, Jackson and McShane. This is an open-access article distributed under the terms of the Creative Commons Attribution License (CC BY). The use, distribution or reproduction in other forums is permitted, provided the original author(s) and the copyright owner(s) are credited and that the original publication in this journal is cited, in accordance with accepted academic practice. No use, distribution or reproduction is permitted which does not comply with these terms.
*Correspondence: Helen McShane, aGVsZW4ubWNzaGFuZUBuZG0ub3guYWMudWs=
†These authors have contributed equally to this work and share first authorship