- Department of Medical Cell Biology, Uppsala University, Uppsala, Sweden
The incidence of Diabetes Mellitus is increasing globally. Individuals who have been burdened with diabetes for many years often develop complications as a result of hyperglycemia. More and more research is being conducted highlighting inflammation as an important factor in disease progression. In all kinds of diabetes, hyperglycemia leads to activation of alternative glucose metabolic pathways, resulting in problematic by-products including reactive oxygen species and advanced glycation end products. This review takes a look into the pathogenesis of three specific diabetic complications; retinopathy, nephropathy and neuropathy as well as their current treatment options. By considering recent research papers investigating the effects of immunotherapy on relevant conditions in animal models, multiple strategies are suggested for future treatment and prevention of diabetic complications with an emphasis on molecular targets associated with the inflammation.
Introduction
Diabetes Mellitus is a chronic metabolic disease causing those with the condition to be subjected to higher blood glucose ranges compared to healthy controls. According to the World Health Organization (WHO) an estimated 422 million people worldwide live with diabetes and 1.5 million global deaths are attributed to complications from the disease (1). Diabetic incidence worldwide has shown an increase over time, with a predicted 578 million cases by 2030 and a predicted total of 700 million by 2045 (2).
Hyperglycemia seen in diabetics stems from either the inability to produce insulin through beta cell loss (Type 1 Diabetes, T1D) or through an accumulated resistance to insulin (Type 2 Diabetes, T2D). Despite the difference in disease causation, chronic hyperglycemia remains problematic for all types of diabetes. Current recommendations for diabetics, in addition to increased control of their glycemic range via insulin injection or insulin sensitivity medication, is to eat a healthy balanced diet in addition to exercise in order to reduce the risk of long-term complications (3).
Complications from diabetes can generally be categorized into two main groups. Damage to large arteries can lead to problems with the heart, brain and legs and are often referred to as macrovascular complications. Microvascular complications, by contrast, affect the smaller blood vessels in many organs throughout the body, including the eyes, kidneys and nerves referred to as retinopathy, nephropathy and neuropathy, respectively (Figure 1).
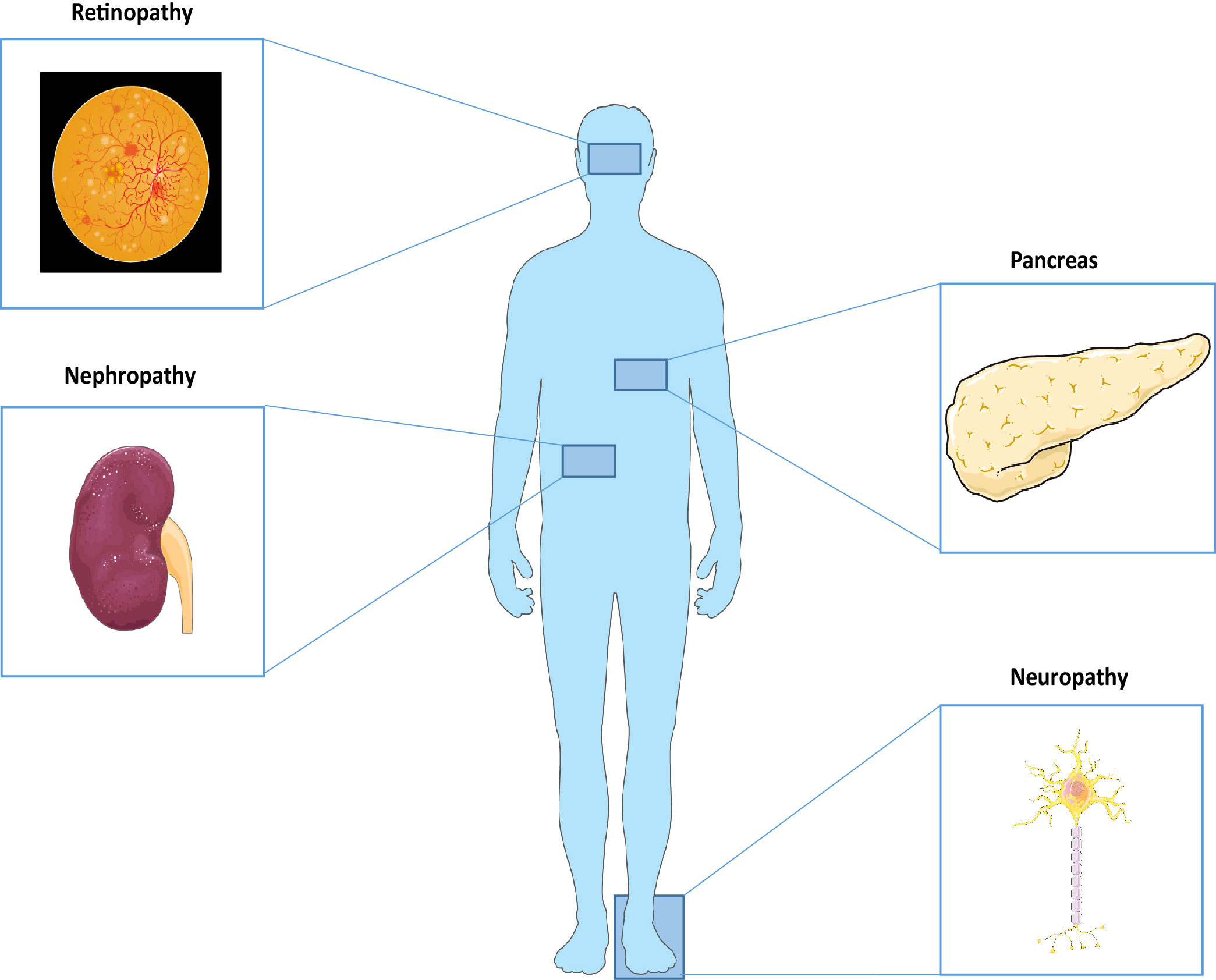
Figure 1 Microvascular damage affects the eyes, kidneys and nerves in diabetes. The three major organs affected by microvascular complications due to an extended exposure to hyperglycemic conditions. Parts of the figure were drawn by using pictures from Servier Medical Art. Servier Medical Art by Servier is licensed under a Creative Commons Attribution 3.0 Unported License (https://creativecommons.org/licenses/by/3.0/).
With a global increase in the number of diabetics each year, and a large enough percentage of diabetics statistically likely to develop complications as a result of diabetes, the quality of life and cost for individuals/health care institutions are sure to be negatively affected. With as many as 1 in 9 adults dying as a result of their diabetes, the prevention of diabetes and its complications is very important, especially in middle income countries (4). Currently medication is given to treat symptoms of diabetic complications without targeting the underlying cause of the disease progression. This analysis aims to review the current literary understanding of the role of the immune system in diabetic complications namely; retinopathy, nephropathy and neuropathy, and suggest treatment based on the advancements made in immunotherapy.
Microvascular complications of diabetes
Diabetic retinopathy
Diabetic Retinopathy (DR) is the most common complication to affect individuals with diabetes (5, 6). As of 2022, the number of diabetics with DR stands at around 93 million cases globally. Prevalence of DR is as much as 77.3% in T1D and 25.1% in T2D. Approximately 25-30% of these cases are thought to progress to vision threatening diabetic macular edema, 5-8% of which will require laser surgery to correct and another 5% requiring vitrectomy surgery (7–9). Although not life threatening, DR may lead to microvascular damage to the retina resulting in blindness (7, 9).
In response to hyperglycemia, alternative pathways of glucose metabolism are activated ultimately leading to cellular damage over a sustained amount of time (10–12). The polyol pathway is one such pathway in which glucose is reduced to sorbitol and then converted to fructose in the presence of sorbitol dehydrogenase (13). Sorbitol dehydrogenase is present in most tissues; however, it is absent in key tissues associated with diabetic complications, namely the eyes, kidneys and nerves (14, 15). Müller cells of the eyes as well as the retinal ganglia, vascular pericytes and endothelial cells do however contain the enzyme aldose reductase (16–19), responsible for the initial conversion of glucose to sorbitol. The excess of sorbitol leads to osmotic pressures in the retina due to its impermeability, leading to edema. The pathway further produces reactive oxygen species (ROS), advanced glycation end products (AGEs), as well as protein kinase C activation further contributing to disease progression (7, 20).
The presence or absence of neovascularization is used to categorize DR into one of two categories - proliferative or non-proliferative, respectively. Proliferative is the most advanced form of the disease, where hypoxia as a result of decreased vascular function by capillary occlusion drives neovascularization (21–26). Loss of vision caused by macular edema is common in both forms of DR, however, blindness caused by bleeding, hemorrhage and subsequent retinal detachment are benchmarks of proliferative DR (27, 28).
The presence of neovascularization and edema in DR provides evidence of a defining hallmark of inflammation (21, 29, 30). DR was not considered an inflammatory disease until recently, as the retina was originally considered an immune privileged tissue. This is true at default, where no inflammatory markers should be present. However, the increase in proinflammatory markers during diabetic retinopathy highlights the important inflammatory shift associated with the condition (31). In an experiment by Capozzi et al., concentrations of Interleukin-6 (IL-6), IL-8 and vascular endothelial growth factor (VEGF) were significantly increased by human Müller cells in the presence of both linoleic and oleic acid (32). When treated with D or L-glucose alone, an increase in the production of VEGF was observed in Müller cells. This was attributed by the group to the osmotic effects of increased blood glucose. Dietary levels of polyunsaturated fatty acids (like linoleic acid) have been shown to correlate with the incidence of retinopathy in T2D and has been suggested as a biomarker in T2 diabetes (33). These fatty acids collect in Müller cells, spanning the retina leading to inflammation from the increase of proinflammatory cytokines IL-6 and IL-8 among other factors (32, 34–38) (Figure 2).
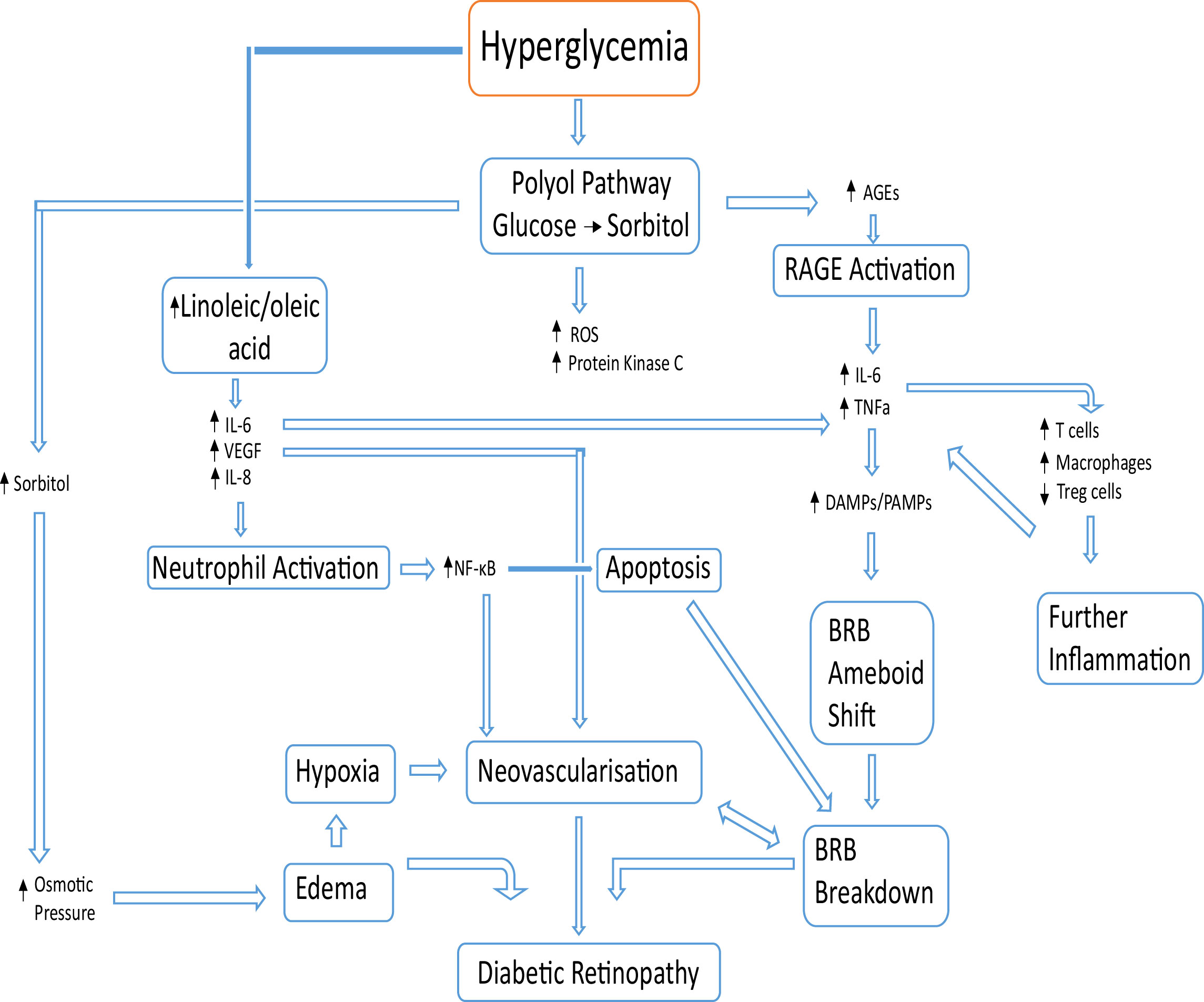
Figure 2 Basic overview of pathological events leading to DR. Beginning with hyperglycemia, alternative glucose metabolic pathways create excess sorbitol and cytotoxic end products. The increase in advanced glycation end products results in more binding with the corresponding receptor (RAGE) causing an increase in proinflammatory cytokines (IL-6, IL-8 and VEGF). An increase in linoleic and oleic acid increases the levels of IL-6, IL-8 and VEGF, which activate neutrophils. The blood retinal barrier breakdown is caused by apoptosis from neutrophil activation in conjunction with damage-associated molecular patterns (DAMPs) and pathogen-associated molecular patterns (PAMPs) activation causing a Blood Retinal Barrier (BRB) phenotype shift to a more ameboid state. DR results from BRB breakdown, local neovascularization and edema.
Increased levels of IL-8 result in neutrophil activation at the activated epithelium (32, 39). Activation of the neutrophil results in extracellular trap formation which further contributes to the pathogenesis of DR (39, 40). In addition to this, IL-8 is associated with the activation of the nuclear factor κB (NF-κB) pathway, which is present in many cell types and participates in cell apoptosis and neovascularization (41). Over time, an accumulation of NF-κB expression due to hypoxia, hyperglycemia, AGEs and some inflammatory cytokines, triggers an increase in VEGF expression and thus, neovascularization (41). The apoptotic breakdown of the blood-retinal barrier (BRB), either by endothelial cell death or supporting cell death (including Müller cells), damages the BRB microenvironment causing leakage of blood and negatively affects proper retinal barrier function (42) (Figure 2).
Before the degradation of the blood-retina barrier, the only immune sentinels are the microglia (43). However, upon the integrity loss of the blood-retina barrier, products of hyperglycemia may alter the physiology of microglia to initiate inflammation, for example AGEs binding with their RAGE receptor (receptor for advanced glycation end products) on microglia, resulting in secretion of proinflammatory IL-6 and TNFα (44). This BRB degradation is achieved by activation of the receptors of damage-associated molecular patterns (DAMPs) and pathogen-associated molecular patterns (PAMPs) located on the microglia, causing them to divide and shift morphology to a more amoeboid nature (31, 44) as seen in Figure 2.
Tumor necrosis factor alpha (TNFα) is a proinflammatory cytokine commonly produced by both macrophages and T cells which is responsible for inducing inflammation and apoptosis (45). In DR, TNFα appears to actively participate in the pathogenesis of inflammatory, edematous, neovascular, and neurodegenerative diseases (45, 46). In studies examining concentrations of TNFα in the vitreous, TNFα appears early and concentrations increase throughout disease progression (31, 47). TNFα and Interleukin-1 beta (IL-1β) have been shown by in vitro studies to cause microglia and endothelial cells to secrete VEGF (31, 47). IL-1β has also been noted to feature in cross-talk with IL-8, where IL-1β’s presence has initiated the release of IL-8, causing more inflammation in vivo in both human and rodent models (31, 48).
Apart from surgical options (including laser treatment), currently prescribed medication includes anti-VEGF agents (monoclonal antibodies, mABs) and steroid injections (49). VEGF secreting Müller cells have been shown to break down the blood-retina barrier and cause neovascularization in rodent models of DR (50). Anti-VEGF mABs (such as; bevacizumab, ranibizumab, and aflibercept (50)) may be administered as treatment, with Ranibizumab approved intravitreal use in Europe and the US. Intravitreal injection has seen some good results, however these injections must be taken continuously to maintain the therapeutic effect (51). An article by Nair and Modi proposed that anti-VEGF agents may in fact be masking DR’s true stage rather than treating the underlying pathology (52).
Medication targeting the influence of the immune system would in theory decrease the development of DR. TNFα blockers are already used in other disease treatments, however they are relatively new and long-term use still needs to be evaluated. Monoclonal antibodies also exert some positive effects on DR, where trials using combinations increase therapeutic effects (53). Microglia can form proinflammatory M1 phenotypes (CD26 and CD32 markers), as well as an anti-inflammatory M2 phenotype (CD163 and CD206 markers) where anti-inflammatory molecules such as IL-4, IL-10, IL-13 and transforming growth factor beta (TGFβ) are excreted (44, 54, 55). In the presence of chronic disease and danger signals (seen in chronic hyperglycemia) the phenotypic shift of microglia from balanced to proinflammatory reacts as a positive feedback mechanism, which can further disease progression (44). By taking advantage of the cellular communication environment, namely cytokines and receptors, research into the M1 to M2 phenotype shift is possible. By shifting the balance to an anti-inflammatory M2 phenotype, the homeostasis and protective effects should return in theory. Research has been conducted on M1 to M2 phenotype shift in neurodegenerative disorders (55), however more investigation is needed in DR.
Diabetic nephropathy
Diabetic kidney disease (DKD) can affect as many as 30% T1D patients and 40% of T2D patients, making diabetes accountable for 20-50% of those entering renal failure programs (56, 57). It is the leading contributor of End Stage Renal Disease (ESRD) in the US, prompting dialysis and kidney transplantation (58, 59). Of these ESRD cases, it is observed that 80% are due to diabetes or hypertension, with a 5-year survival rate of less than 40% (60). Diabetic Nephropathy (DN) is an umbrella term used for kidney diseases associated with the above conditions in diabetics. It presents as a chronic inflammation of the kidney(s) alongside proteinuria (albuminuria) and decline in renal functioning (61).
DN progresses in stages using different pathological benchmarks. In early stages of the disease, fibrosis is caused by glomerular and tubular hypertrophy ultimately caused by hyperglycemia, as well as a multitude of other hemodynamic factors (58, 62). This causes a thickening of the basement membrane and an expansion of the mesangium in later stages, leading to end-stage glomerular closure and tubulointerstitial fibrosis as well as cellular stress (63–68). The advanced stage of disease can be characterized by an infiltration of immune cells to the damaged kidney environment (61, 69). Disease progression usually occurs over 10-20 years, however, this can vary for different patients depending on glycemic control among other factors (58).
The pathobiology of the disease is a lot more complex in reality, with changes to kidney structure, function and metabolism that still remain to be better understood (70). Hyperglycemia leading to alternatively activated glucose pathways and their cytotoxic products are just one of many associated causes of disease onset, with other factors such as immune response, oxidative stress and oxidized lipids also playing distinct roles. All of these combined cause damage to the kidney cells, releasing DAMPs which trigger proinflammatory pathways (61) (Figure 3). The amount of proinflammatory cytokines both circulating and synthesized by the kidney increase during DKD (71–73).
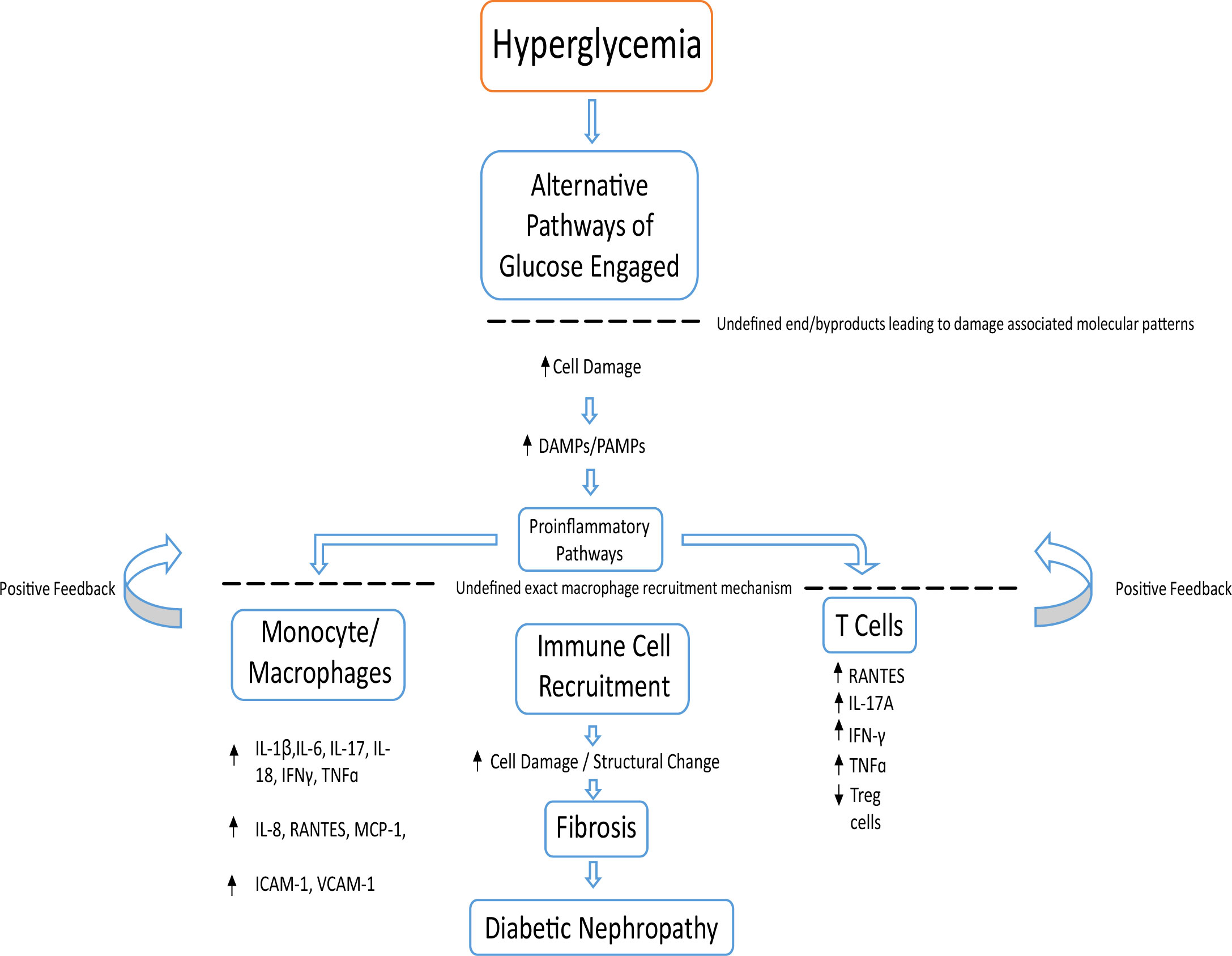
Figure 3 Basic overview of possible pathological events leading to DN. Chronic hyperglycemia leading to cellular damage increases DAMPs and PAMPs. This encourages inflammatory cells such as monocytes, macrophages, T cells and neutrophils to begin a positive feedback loop of immune cell recruitment and inflammation. This leads to kidney fibrosis and a decline in kidney function, leading to DN.
The recruitment of monocytes and macrophages (74, 75) responding to damaged cells of the renal mesangium, podocytes and endothelial cells causes surrounding cells to release various proinflammatory cytokines (IL-1β, IL-6, IL-8, IL-17, IL-18, interferon gamma (IFNγ), TNFɑ), chemokines (RANTES, and monocyte chemoattractant protein-1 (MCP-1); regulated on activation, normal T cell expressed and secreted (76) adhesion molecules; intracellular adhesion molecule-1 (ICAM-1) and vascular cell adhesion molecule-1 (VCAM-1), begetting a vicious cycle of chronic inflammation. This results in an accumulation of immune cells from both the innate and adaptive systems (77). Such inflammation drives structural changes in DN through fibrosis (63, 75) (Figure 3). The amount of proinflammatory cytokines both circulating and synthesized by the kidney increase during DKD (71–73). This is seen in urine samples and renal biopsies of patients with DKD, where an increase of IL-6 and IL-8 compared to healthy controls is observed (71, 78).
F4/80 or CD68 positive macrophages are often found in kidneys of DN patients via flow cytometry and immunohistochemical staining (61, 79). Depletion of macrophages conducted by You et al. showed that by removing macrophages in streptozotocin (STZ) model mice, one can better protect renal tissue. You et al. were the first to show evidence for direct interaction between podocytes and M1 macrophages, with impairment of podocyte integrity potentially due to the action of MCP-1 (80). As it stands, the precise nature of how macrophages are recruited to the kidneys remains to be clarified. Cell adhesion molecules and chemokines/chemokine receptors have been found to be involved in this process with the vascular endothelium overexpressing cell adhesion molecules on its surface, including ICAM-1 and VCAM-1, which recruit precursor macrophages (61). T cells accompany macrophages to the kidney and progress DN through the secretion of proinflammatory cytokines such as TNFɑ and INFɣ (61, 63, 75, 81, 82). A study published by Moon et al. also demonstrated that activated T cells were attributed to aberrant diabetic kidney injury in STZ mouse models in conjunction with hyperglycemia (83). As with macrophages, the mechanism of T cell recruitment to the kidneys remains unclarified, although as before it is known to involve proinflammatory cytokines, chemokines and adhesion molecules (75).
T cells, much like other immune cells, feature a great deal of plasticity (84–88). They can alter their phenotype in order to become the subset required in a given environment based on extracellular clues (85). It has been well documented that T cells have many subsets, including Th1, Th2, Th17 and Treg cells (89, 90). T cells which are CD4+ have been shown to interact with fibroblasts to induce fibrosis (91). In a study by Peng et al., the expression levels of IL-17A by renal T cell subsets was monitored in obstructed kidneys (91). It was found that Th17 T cells, γδ T lymphocytes and a population of CD3+CD4−CD8−γδTCR− cells produced IL-17A after obstructive injury in the kidney. They further demonstrated using IL-17A deficient mice that IL-17A enhanced the production of RANTES, a proinflammatory chemokine involved in the recruitment of T cells and monocytes leading to fibrosis. Th17 cells are known to secrete the most IL-17A (91). Their phenotype is induced by cytokines TGFβ and IL-6, levels of which increase during obstructive kidney injury (91–93).
In the past two decades, no new medication has been suggested in order to restore kidney function or prevent the further loss of kidney function (94–96). Currently, treatment of DN is blood glucose/blood pressure control, lipid lowering and renin-angiotensin system blockade. A major issue with these therapies however is that they are generally prescribed at the later stage of disease development, offering only some reno-protective element (70). Drug delivery to injured kidneys also requires high dosage, often associated with adverse effects (61). Given the above evidence for the role of the immune system in DN, consideration must be given to including immunotherapy as a means to treat DN and slow/stop disease progression.
Diabetic neuropathy
Diabetic Neuropathy is a term used to encapsulate the many clinical and sub-clinical issues affecting a broad range of tissues via different underlying molecular mechanisms (97). Clinical manifestations of diabetic neuropathy may be grouped under either diffuse or focal neuropathies (97), where diffuse tends to affect many nerves connected to distal parts of the body such as the feet. Diabetic Peripheral Neuropathy (DPN) is the most common subtype of the peripheral neuropathies (98). Approximately 50% of adults with diabetes will develop DPN at some stage in their life (99). Of these 50%, foot ulceration is common, with some patients even requiring foot amputation later on (100, 101). Diabetes can produce several types of peripheral nervous damage, the most common being bilateral and symmetric damage of the feet (102). Although the foot is the most common area affected by complications of the nervous system, diabetic neuropathies have the potential to affect many organs thereby lowering the quality of life and increasing morbidity (103).
The peripheral nervous system (PNS) is more exposed to environmental factors compared to the central nervous system. The types of nerves in the PNS range from highly myelinated motor nerves, less myelinated sensory nerves and lightly myelinated autonomic nerves. This is often dictated by length, where motor neurons tend to be longer and therefore more myelinated compared to sensory nerves for pain and temperature sensations (104). Symptoms of diabetic neuropathy usually start with a lack of sensation, over time developing further to more positive symptoms such as burning sensations, pins and needles, tingling and/or lightning pain (97). As DPN progresses, demyelination, beginning in the feet, moves up the legs and eventually reaches the hands in what is known as the “stocking-glove” distribution (105) as demonstrated in Figure 4.
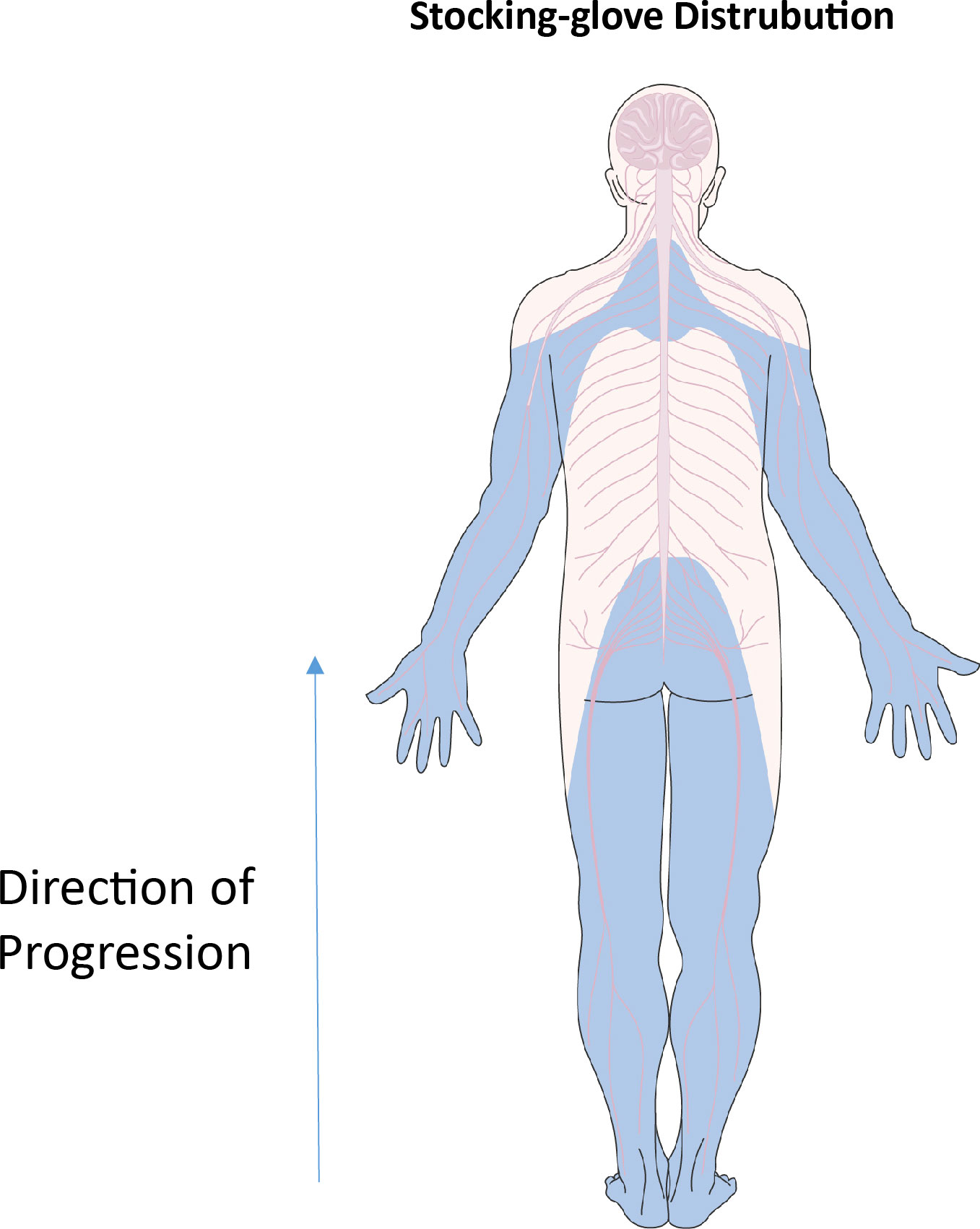
Figure 4 “Stocking-glove” distribution. Blue areas indicate regions affected by nerve damage in diabetic neuropathy. Beginning in the feet, nerve damage rises through the legs. Similar events happen in the arms, beginning in the hands and moving upwards in a distal to proximal direction. Parts of the figure were drawn by using pictures from Servier Medical Art. Servier Medical Art by Servier is licensed under a Creative Commons Attribution 3.0 Unported License (https://creativecommons.org/licenses/by/3.0/).
Despite many clinical variations of diabetic neuropathy, the same root cause can be attributed to the disease progression. Hyperglycemia leading to the activation of alternative pathways of glucose metabolism (Polyol, PARP, protein kinase C, hedgehog and more) leads to the formation of AGEs, ROS, lipooxygenase pathways (LOX) and an elevation of proinflammatory cytokines (IL-6 and TNFɑ) (97) (Figure 5). The biochemical result of this, leads to cellular damage of the associated nerves as well as oxidative stress, altering the cellular and metabolic function in diabetic neuropathy (97). The principal proinflammatory cytokine involved in DPN is TNFɑ, as opposed to IL-6 as seen in other microvascular complications such as DR and DN (106–108). Activated macrophages, CD4+ cells, natural killer cells, mast cells and eosinophils upregulate TNFɑ to promote inflammation, as discussed earlier in DR and DN. Both diabetics and diabetics with neuropathy express more plasma TNFɑ compared to healthy controls (97, 109–111). Hyperglycemia overtime may cause nerve myelin protein glycosylation, resulting in possible recognition by specific macrophages at a later date, subjugating the myelin to phagocytosis (112, 113). Overtime, phagocytosis leads to demyelination of the nerve, causing suboptimal conduction of nerve signalling. When exposed, myelin antigens activate T cells which respond by secreting proinflammatory cytokines (namely TNFɑ) initiating an inflammatory immune response (112, 114). The cytotoxic effects of elevated levels of TNFɑ on oligodendrocytes may also cause further demyelination (115). The action of TNFɑ on mononucleated cells further amplifies the inflammatory response via secretion of IL-1β and IL-6.
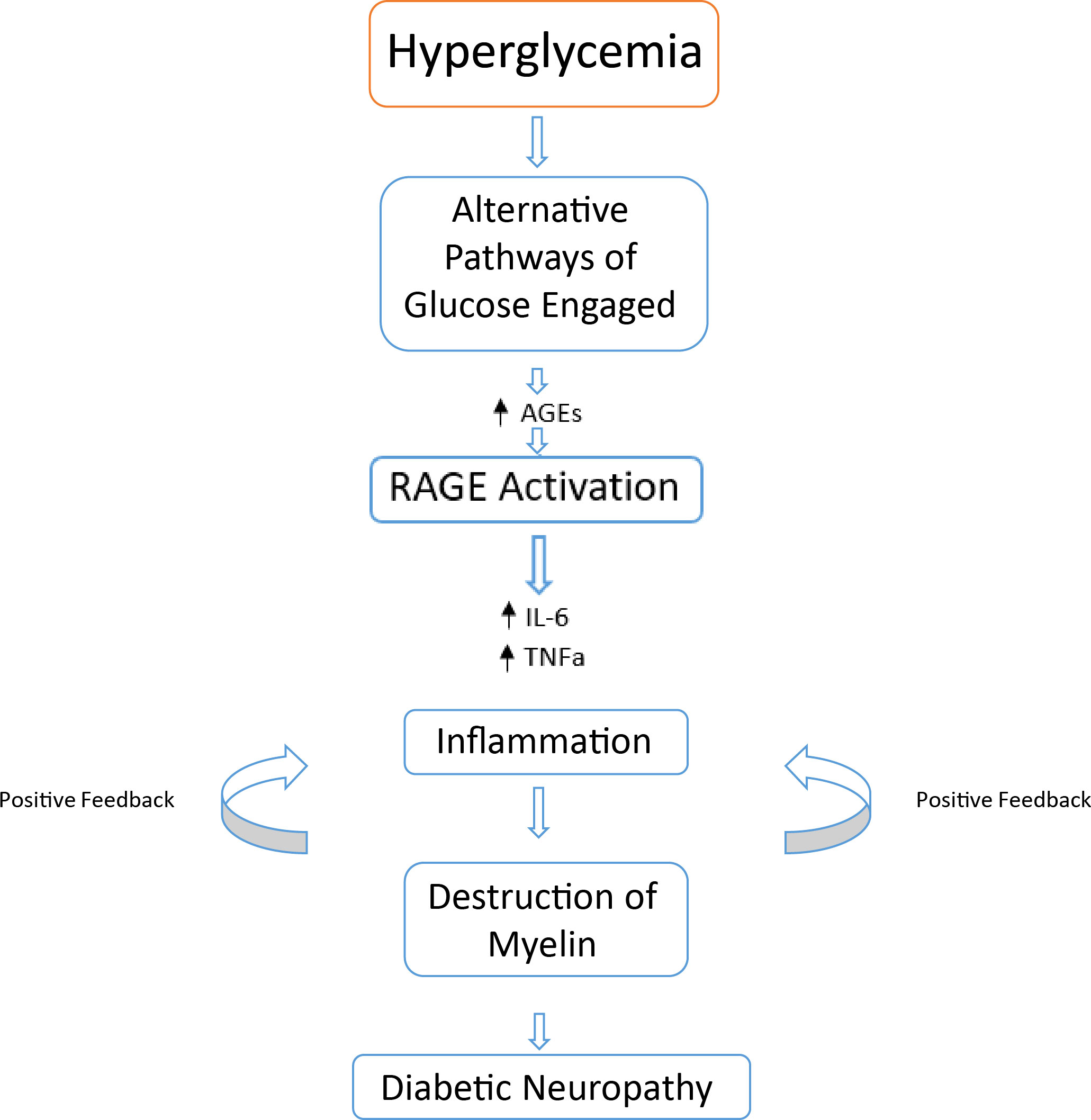
Figure 5 Basic overview of possible events leading to DPN. Chronic hyperglycemia alternatively activated glucose metabolism pathways result in increases of cytotoxic byproducts and end products. An increase in AGE - RAGE interaction results in an increase of proinflammatory cytokines leading to a positive feedback loop of immune cell activation. This leads to the destruction of nerve cell myelin, leading to a reduction in nerve functionality resulting in diabetic neuropathy.
Despite decades of research, progress in the medications combating diabetic neuropathy has not seen much progress, with clinicians primarily recommending higher standards of glycaemic control as well as exercise for prevention. There are no disease modifying therapies for those with DN, with most trials resulting in failure (116). As of the time of writing this review, most medication offered by clinicians is centred around the management of pain to improve quality of life. Anticonvulsants (e.g. pregabalin and lamotrigine), antidepressants (SNRIs and TCAs) and opioids (tramadol) are often used as therapies in diabetic neuropathy among others (100).
Inflammation in diabetic patients
Common to all three (DR, DN and DPN) aforementioned pathologies is the notion of hyperglycemia being ultimately responsible for complications with diabetes. Correct diet, exercise and good glycaemic control are the ultimate end goals of patient care and can prove to be greatly preventative, however, this is ultimately an incredibly hard practise to maintain living with diabetes. Chronic hyperglycemia then, although ultimately responsible, will not be considered as a therapeutic target for this review, given the incredible number of factors that may or may not affect an individual’s blood glucose levels.
Alternative pathways of glucose metabolism (such as the polyol pathway) are the second step in the procession of diabetic complications, with hyperglycemia activation ultimately resulting in cytotoxic by-products. In tissues lacking sorbitol dehydrogenase, such as those associated with diabetic complications, research has been conducted in animal models and human cell lines (117, 118), however more research is needed into the effect on humans in vivo. These studies target the initial enzyme in the pathway, aldolase reductase, for inhibition with some success (119). Matoba et al. have reviewed the literature with regards to redox imbalances in DKD and illustrated that Sodium Glucose Transporter 2 inhibitors and Glucagon Like Peptide-1 receptor agonists are potential pharmaceutical treatments (120–123). A general lack of consensus on the effectiveness of aldolase reductase inhibitors therefore merits further research (14, 124, 125).
Although inflammation in the short term is beneficial for the body, chronic levels of inflammation can be very damaging, resulting in a myriad of complications (126). By targeting the inflammation common to most diabetic complications, one may lessen the burden or even prevent complications in the first place. Targeting the immune response provides many viable targets including; inhibition of soluble proinflammatory mediators, inhibition of transcriptional factors as well as inhibition of other immune processes (61).
Macrophages, T cells and the complement system all play an important role during inflammation with regards to diabetic complications. This inflammation in turn begets a vicious cycle of immune cell activation and further organ damage. As hyperglycemia induces alternative metabolic pathways of glucose, ROS and AGEs are produced with often problematic outcomes (127).
AGEs bind to corresponding AGE receptors (RAGE) causing upregulation of two transcription factors connected with inflammation, NF-κB and early growth response-1 (Egr-1) (128). In diabetic tissues and during inflammation, RAGE is correlated with the continuous activation of NF-κB, contributing to cell stress and dysfunction over a sustained period (128). This in turn triggers the adaptive immune system, in which RAGE has been evidenced to be upregulated during T cell activation in addition to monocyte chemoattractant peptide-1 (MCP-1) (128).
Macrophages are attributed as the principal inflammatory cell involved in kidney damage and are also known as the ‘remodelers’ (72, 129). This is owed to the presence of both proinflammatory type 1 (M1) macrophages stimulated by Th1 cells producing interferon gamma (IFN-ɣ), or the anti-inflammatory/repair mediating type 2 (M2) phenotype differentiated by Th2 cells secreting IL-4 and/or IL-13 (129, 130). Polarisation of macrophages is dynamic and may be altered depending on the cytokine inputs from the surrounding environment. When RAGE receptors are stimulated, the upregulation of MCP-1 causes monocytes to transform into macrophages ultimately resulting in local IL-6 and Tumour Necrosis Factor Alpha (TNFɑ) increases (129).
T cells also play a large role in inflammation. Although the recruitment process of T cells to the kidney in diabetes is poorly understood, it is known that they accompany the macrophages (61). The released IL-6 (from the previous paragraph) is known to activate Th17 cells in conjunction with TGF-β, and may very well be the driving factor of T cell movement to the diabetic kidney environment (131–133). Activated T cells secrete proinflammatory cytokines such as TNFα and IFN-ɣ. Both cytokines can directly damage kidney tissues through cytotoxic effects indirectly promoting the migration to (and activation of) macrophages in DN. Aforementioned AGEs may also bind to the RAGE on T cells, in turn stimulating T cells to secrete IFN-ɣ, leading to further kidney inflammation in what may be comparable to a positive feedback loop (61, 129).
In addition to macrophages and T cells, the complement system has been shown to play a role in diabetic complication development (69, 134, 135). The complement system pertains to the numerous small proteins produced by the liver aiding in the clearance of pathogens and damaged cells from the body. However during hyperglycemia, the glycation of these complement proteins leads to dysregulation of the complement pathways as well as pattern recognition molecules of the complement system binding to glycated ficolins activating the leptin complement pathway in DN (134). Activation of the complement system in turn promotes inflammation ultimately causing further damage in diabetes. Clinical trials acknowledging the role of complement in DN development have targeted elements of the pathway for deletion, resulting in better disease outcomes (discussed later in the review).
Inflammation from macrophages and T cells may be hampered by the presence of regulatory T cells (Tregs) (136) among other methods. This review will now discuss the various possible methods of lessening inflammation in the aforementioned diabetic complications.
Therapeutic strategies
There are a number of proposed means to prevent inflammation culminating in diabetic complications. The following section includes several of these potential therapies to decrease the effect of inflammation.
Regulatory T cells
Tregs, (CD4+CD25+CD127-/lowFoxp3+) are part of the adaptive immune system that can decrease the immune response in order to maintain immune homeostasis. Initially, Tregs were thought to be a terminal phenotype, however, evidence has emerged of their capability in plasticity, as well as their dynamism (84, 88, 137, 138). In autoimmune patients, such as diabetics, the effectiveness of Tregs are often diminished due to a variety of possible reasons including; poor stability of Foxp3 expression (84, 139–141) or production of proinflammatory cytokine IFN-γ in aberrant processes (84, 88, 137, 142, 143). Our group has also reported that Treg cells switch their phenotype in T1D under lack of IL-35 (142–144).
Although it is possible to generate Tregs through the manipulation of cytokines and co-stimulation, the remaining issue is the functional efficiency of Tregs. Transcription factor Foxp3, a defining characteristic of a Treg cell, must be stable in order to function as a Treg cell (87). In vitro studies have documented that exposure to proinflammatory cytokines lead to loss of Treg cell stability, producing an “exTreg cell” (145). This same phenomenon is observed in vivo when Treg cells are transplanted into autoimmune mouse models, where the exTreg cells then become memory T cells and/or effector T cells (140, 146). Komatsu et al., investigating the plasticity of Treg cell populations, discovered that CD25+ cell populations exhibited a more stable lineage of Treg cells as opposed to CD25- (86). They had found that Foxp3 expression alone would not sustain a population of Tregs, finding that some Tregs (CD25-) would alter phenotype to Th17 T helper cells, confirmed by other studies (147, 148).
Approximately 0.5% of B cells belong to a subset known as regulatory B cells (Bregs) (149). Although the extracellular markers for this population are still being studied, what is known is that they release cytokines including IL-10, IL-35 and Transforming Growth Factor beta (TGF β) to transform Th1 and Th17 T helper cells into Treg cells (149–151). It has been shown in chimeric mice that by lowering IL-10 producing B cell numbers, one can lower Treg cell numbers and thus increase the amount of Th1 and Th17 T helper cells (142, 152). Hence by providing cytokines which encourage Breg cell differentiation, one would expect an increase in Tregs and thereafter a decrease in inflammation.
IL-35
IL-35 is an anti-inflammatory heterodimer cytokine from the IL-12 family composed of IL-12α (p35) and Epstein-Barr virus-induced gene 3 (Ebi3) protein chains which play a key role in the suppressive function of Tregs (153). IL-35 is secreted by a number of immune cells in vivo including both Treg and B regulatory cells as well as tolerogenic dendritic cells and pro metastatic cancer cells (153, 154). On binding with a corresponding cytokine receptor, IL-35 activates the Janus Kinase-signal transducer and activator of transcription pathway (JAK-STAT). The exact molecular pathways and function of IL-35 binding still remains to be totally illustrated in inflammatory autoimmune diseases (155). IL-35 is associated with many autoimmune conditions including: T1D, rheumatoid arthritis, multiple sclerosis, systemic lupus erythematosus, primary Sjögren syndrome and atherosclerosis in addition to cancer metastases (153). What is known however, is that IL-35 has been reported to have an anti-inflammatory effect by expanding Treg cell populations and preventing development of proinflammatory Th1 and Th17 cells (156).
Our group has found that administration of IL-35 reversed established T1D in NOD and MLD-STZ mice by maintaining the suppressive phenotype of Treg cells (143). In addition, we found that levels of IL-35 were lower in patients with recent onset and long standing T1D than in healthy controls. A decrease in IL-35 positive Treg and Breg cells has been reported by our group in patients with T1D compared to healthy controls (157). With similar results to our earlier studies, we also found that IL-35 maintains the suppressive phenotype of Breg cells in experimental T1D.
In a study performed by Jiang et al., the STZ rat model was used to simulate DN (158). In this study, Jiang et al. found that by administering IL-35 the progression of inflammation was prevented by the reduction of proinflammatory cytokines IL-1β, IL-6 and TNFɑ and by increasing IL-10 (an anti-inflammatory cytokine). Yan et al., using an STZ mouse model for diabetic retinopathy, also found very similar results in line with Jiang et al. (159). Patients with DR were found to have significant downregulation of IL-35 in vitreous tissues compared to controls (159). IL-35 administration had a suppressive effect on Th17 T helper cells, ultimately causing less inflammation and thus providing more evidence of the therapeutic effect of IL-35 in preventing experimental DR (160).
IL-35 treatment was also found to polarise classically activated proinflammatory M1 macrophages to an alternatively activated anti-inflammatory M2 phenotype (161). M1 macrophages, when stimulated by TNFɑ secreted by Th1 cells, release TNFɑ, IL-1β and ROS as part of a positive feedback mechanism of inflammation (162). A recent study by Luo et al. found that IL-35 treatment prevented the elevation of M1/M2 ratio in MLD-STZ mice, a model used for experimental T1D. In addition to this, the proportion of TNFɑ+ cells among macrophages in PDLN was decreased, with unaffected TNFɑ+ cell numbers in the spleen, maybe suggesting that effects are localised as opposed to systematic (162).
As observed in the aforementioned studies, the role of IL-35 in maintaining a population shift toward Foxp3 expressing Tregs and away from Th17 T cells was important, thereby reducing the amount of inflammation at target sites. IL-35 also holds the ability to shift proinflammatory M1 macrophages to anti-inflammatory M2 macrophages, reducing the secretion of TNFɑ among M1 macrophages. The value of IL-35 in patients with diabetic complications is of great importance and is therefore a hopeful choice for the future treatment of diabetes and its subsequent complications after safety testing in humans. As well as the use of cytokines, other techniques may be utilised to increase Treg quality and therefore lower inflammation.
CRISPR editing of Tregs for higher stability
An innovative method used to edit the genome in cells, CRISPR/Cas9 (clustered, regularly interspaced, short palindromic repeats/CRISPR associated protein 9) technology is being investigated to expand viable Treg numbers (163–166). By using CRISPR, one could target either disabling or enhancing pathways of Treg cell functionality.
One such pathway leading to the instability of Treg cells, moderated by IL-6, has been well documented and features in mAB treatment for autoimmune and inflammatory conditions such as SLE and rheumatoid arthritis (163, 167, 168). Genes associated with the IL-6 alpha (IL-6α) receptor (CD126) were knocked out in a study by Zeebroecket al. (163). The resulting Treg cells showed stable expression of Foxp3 and Helios, IL-2, IL-10, IFNγ and IL-17a compared to mock Tregs whilst showing significantly lower phosphorylation of STAT3 (a pathway activated by IL-6). By using CRISPR to target and delete the IL-6α gene, the ratio of Th17/Treg cell may shift in favour of Treg cells.
Hypoxia-inducible factor 1-alpha (HIF‐1α) is a subunit of HIF-1 encoded by the HIF-1α gene (169). Primarily, HIF-1α is activated in hypoxic conditions to upregulate genes associated with angiogenesis, erythropoiesis and glycolysis (170–175), however it is also induced by continuous TCR stimulation via mTOR in human T cells (165). In mice and human embryonic kidney cells, HIF-1α is capable of downregulating Foxp3 expression either by ubiquitination or proteasomal degradation even in non-hypoxic conditions (176). In HIF-1α deficient mouse models, the balance of Th17/Treg cells is disrupted to favour Treg cells (176).
More and more research is being conducted on potential genes involved in Foxp3 stability (177). As more gene functions are discovered, more targets emerge for CRISPR intervention leading to potential trials in vitro and in vivo. By increasing the stability of Tregs via CRISPR, the potential to effectively reduce the damage caused by inflammation also increases. This gives us a potential to treat or prevent diabetic complications, should trials be successful. In addition to the editing of Tregs through CRISPR, it is also possible to produce Tregs through stem cell technologies.
The effect of mesenchymal stromal cells on Tregs
NEPHSTROM is a newly proposed therapy funded by EU Horizon showing great promise for DKD treatment as well as a treatment for other micro- and macrovascular complications (96, 178, 179). Versatility is an attractive feature of mesenchymal stromal cells (MSCs), allowing them to differentiate into a number of cell types. This allows them to initiate tissue repair, restore lost function, migrate to sites of injury and target effector mechanisms associated with inflammation (180). Ex vivo-expanded MSCs and T-regs have been used successfully, and most importantly safely, in many animal models due to their immunomodulatory effects. This makes them an extremely attractive option in the treatment of autoimmune disease (180). The preclinical ORBCEL-M data provided a basis to further investigate the role of MSCs in slowing or stopping progressive DKD (181).
The specific mechanism of action is still to be clarified; however, it is commonly held that cell-to-cell contact dependent mechanisms alongside co-stimulation and soluble mediators play an important role (180). High affinity binding of MSCs with T-cells via upregulated expression of cell integrins, ICAM-1, VCAM-1, CD71 and CD58 play an important role in immune suppression. Notch receptors, expressed highly on MSCs, also stimulate iTreg cell differentiation, which better resist IL-6 conversion to Th17 phenotypes in inflammatory conditions compared to pTregs (182). Interaction with antigen presenting cells such as dendritic cells has also been identified as a mechanism of action of MSC therapy. Bone marrow-derived MSCs can reprogram mature dendritic cells into antigen presenting cells with distinct jagged-2-dependent regulatory properties. This in turn causes CD4+ T cells to differentiate into Tregs effectively repressing Th17 differentiation (183, 184).
The regenerative potential of MSCs can depend on other factors, since several exogenous (and other) factors may greatly impact the MSC’s biological properties and subsequent function (185, 186). Although the majority of MSC based therapy remains promising, the potential of some adverse effects is not zero, with some researchers highlighting that the effects of paracrine signalling and the secreteome have yet to be fully examined (185, 187).
Inhibitors of the complement system
As stated in the introduction, the complement system has also been identified to play a key role in diabetes related inflammation, particularly in cases of DKD (61, 134). Research in this area is still relatively new however, with only a small number of studies conducted on mice (188) and rats (189). In a study by Li et al, C3a was identified as a viable drug target in DN after rats were given a high-fat diet + repeated low dose STZ to induce diabetes in addition to a C3aRA versus a control group. After 8 weeks, biochemical analysis showed that mice treated with C3aRA had an improved renal function and morphology, compared to diabetic rat controls. Thus the complement system is a valid therapeutic target in DN.
Eculizumab is a humanised monoclonal antibody which binds to C5, blocking its ability to form the membrane attack complex and is already in clinical use in other diseases (61, 190). Although the question of whether these drugs will have any effect on the microvascular complications of diabetes remains to be answered (135).
The key issues remaining for complement inhibition pertains to the localisation of the effect to a target organ as well as dosing. Systemic effects of complement inhibition render the patient susceptible to infection, whilst the abundance and high turnover of complement proteins in the blood plasma present a challenge to dosing (191). Thrombomodulin has also been suggested as a target, as it is a regulator of both complement and coagulation activation as diabetic mice lacking thrombomodulin lectin-like domain had exasperated DN (135, 188).
In addition to the Tregs and the complement system, macrophages may also be targeted in order to alleviate the burden of inflammation on organs associated with diabetic complications.
Transcription factor EB
MSCs also have the ability to convert M1 macrophages to M2 macrophages via utilisation of Transcription Factor EB (TFEB). Classical M1 activation of macrophages begins with TLR activation on the macrophage surface alongside INFɣ stimulation. The activated M1 macrophage secretes proinflammatory cytokines, reactive nitrogen/oxygen species and promotes the Th1 response among other attributes (192). Alternative macrophage activation (M2) occurs in response to stimulation from IL-4/IL-13 and is known to induce tissue remodelling responses (192). M2 macrophages also reduce expression of MHC-II and co-stimulatory molecule CD80, consequentially reducing the activation of the T cell response.
These phenotypes are not terminal and may shift in vitro and in vivo. Although the exact mechanism responsible for M1 to M2 polarisation is still unclear, a study conducted by Fang et al. documented the effect of TFEB on macrophage polarisation in cancer. They found a reduction in TFEB promoted M2 polarisation via reduced SOCS3 expression and enhanced STAT3 signalling; whilst chemical over expression mediated via hydroxypropyl-β-cyclodextrin resulted in M2 macrophage polarisation inhibition (193). Tumours, when secreting TGF-β ultimately begin a cascade of intracellular reactions leading to M2 macrophage polarisation and a reduction in T cell response. Notably TGF-β is also secreted by Treg cells (194).
The therapeutic effect of TFEB has already been observed in conjunction with chemotherapy, where the presence of TFEB has increased metabolic pressure and therefore increased tumour sensitivity to chemotherapy treatment (195). The effect of TFEB is unclear in diabetic conditions, however an investigation by Song et al. showed that in diabetic mice, levels of activated TFEB (p-TFEB S142) increased in aortic endothelial cells whereas the total protein count of TFEB decreased (196). They found that diabetic factors such as IL-1β and insulin suppressed TFEB and suggest TFEB as a means of treating inflammation associated with diabetes, particularly aortic inflammation. By utilising RNA-seq, they identified key proinflammatory pathways regulated by TFEB, most importantly the effect TFEB has on NF-κB inhibition thereby suppressing the expression of proinflammatory genes such as VCAM1, ICAM1, SELE, and CCL2.
TNFɑ inhibition
At the time of writing, approximately five approved TNFα inhibitors exist on the market for treating inflammatory conditions such as rheumatoid arthritis, crohn’s and psoriasis as well as some off label alternatives (197, 198). Although largely successful as a therapeutic measure, there have been documented cases of adverse events, such as hypersensitivity reactions (46, 199). Further analysis conducted by post-market surveillance and clinical trials have detailed that some patients also run the risk of developing infections, autoantibodies, malignancies, paradoxical inflammation and demyelinating disorders (197, 199, 200).
A case study of a 35 year old woman who had been suffering from rheumatoid arthritis since the age of 15 was documented by Tack et al. (198). After beginning an anti-TNFα treatment (etanercept; dose 25mg 2x per week) in late 2000 she saw a beneficial effect, where her arthritis was reported to be in a much better condition using appropriate metric tests, however her joint inflammation persisted. At this juncture, her fasted blood sugars were within the homeostatic range of 5.7 and 6.1 mmol/l. The patient’s etanercept therapy continued, however, in 2003 the patient developed T1D. Although onset of the disease was not attributed to the treatment by the authors, it was also not ruled out. It was observed that anti-TNFα treatment did not prevent the development of diabetes. There has also been a further example of an arthritis sufferer developing T1D after beginning anti-TNFα therapy (201).
Anti-TNFα therapy on those already diagnosed with diabetes has seen mixed results. In one case study, a T1D patient receiving anti-TNFα treatment (etanercept) for arthritis lost stable control of her blood glucose levels, where they were described as “erratic” and lead to severe hypoglycemic attacks without warning, having previously had stable control (202). When the treatment was discontinued, her blood glucose control had returned to a stable condition. This was attributed to TNFα’s ability to cause insulin resistance in adipose tissue by down regulating glucose transporter mechanisms (203).
Treatment of anti-TNFα may also lead to demyelination of the central nervous system. A review conducted by Fromont et al. documented two patients who showed MS-like symptoms after beginning anti-TNFα therapy and a further who was diagnosed with MS (114). In a review of the post-marketing database of the US Food and Drug Administration, Shin et al. found a total of 15 patients were observed to develop Guillain-Barré syndrome (or variants of the syndrome) after taking different versions of anti-TNFα medication (204). It is therefore recommended that those with an underlying demyelinating disease (potentially such as those with a diabetic neuropathy) do not take anti-TNFα therapy (197).
Although anti-TNFα treatment may work for some, the risk of developing a complication is not insignificant. Until more research is conducted on the safety of other treatments, it is hard to comment on the effectiveness of TNFα as a target in diabetic complications. It is not evidently clear if diabetic neuropathy is a demyelination or axonal disease, or subsetted into either (205, 206), and therefore the treatment of diabetic neuropathy using anti-TNFα may potentially cause damage.
IL-6 inhibitors
IL-6, although proinflammatory, is also very important in other homeostatic functions. It is involved in the insulin-induced clearance of glucose in the liver and skeletal muscle, released by skeletal muscle during exercise and alters insulin secretion by increasing the secretion of glucagon-like peptide 1 (207). The duality of both the pro and anti-inflammatory potential of IL-6 is thought to be based on which pathway is involved in its activation. For example, IL-6 proinflammatory signals (notably in DR) are caused by trans-signalling whilst regenerative or anti-inflammatory activities of IL-6 are thought to be caused by classical activation (208).
Anti-IL-6 (sarilumab) has been seen to lower HbA1c in phase III clinical trials when used as a monotherapy (209). However, in an interesting contrast, a paper by Jörns et al. observed that anti-IL6 alone was insufficient at sustaining an anti-diabetic metabolic state. By studying the effect of various combinations of either anti-TCR, anti-IL-6, anti-IL17A treatments on LEW.1AR1-iddm (IDDM) rats, it was established that normo-glycemia was restored best in combination of all three treatments (210). Although appealing as a preventative measure of diabetic complications, anti-IL-6 treatment may also hold potential in related disorders or complications (207). However much more research into the anti-IL-6 is needed and if appropriate, which combination works most favourably at restoring blood glucose levels.
IL-17a inhibitors
IL-17a is an important inflammatory mediator in chronic inflammation, especially in examples of kidney disease and diabetic complications (211). As such, IL-17 inhibition has been a target for some clinical trials in other diseases relevant to chronic inflammation such as rheumatoid arthritis and psoriasis. Although well tolerated with good safety profiles, there have been some observations made of adverse events in IL-17 and IL-23 inhibitors compared to placebo controls (212). These include infections, nasopharyngitis and headaches as well as local injection site reactions in a trial for ixekizumab (an IL-17 inhibitor).
In their 2019 review of IL-17a as a novel target, Lavoz et al. considered ongoing clinical trials of anti-IL-17a drugs as well as FDA and EMA approved medication (for patients of psoriasis and arthritis) and generally found them to be safe and well tolerated (211, 213, 214). They further comment on the effectiveness of IL-17a inhibitors over TNFɑ inhibitors in the same conditions and ultimately suggested that IL-17a inhibitors be considered for investigation in diabetic complications.
Ceramide treatments
Ceramides, a molecule of the sphingolipid family, are crucial for the stability of mammalian cell membranes and are gaining more attention for their role in inflammation (215). Their metabolites sphingosine-1-phosphate (S1P), and ceramide-1-phosphate (C1P) are involved in a variety of cell survival traits, including growth and immune cell trafficking as well as inflammation (216, 217). The rate of ceramide generation is dependent on the availability of long-chain saturated fatty acids (218). Ceramide is generally proinflammatory, however S1P and C1P can be either proinflammatory or anti-inflammatory depending on the cell type (219).
Ceramides are implicit in T2D progression with some groups suggesting its use as a biomarker (220). They have been observed in high levels in tissues relevant to diabetic micro- and macrovascular complications (220). The novel use of anti-ceramide treatment could lead to a therapeutic effect in various diabetic complications. The effect of anti-ceramide scFv immunotherapy in retinopathy was recently investigated and found that one injection of scFv at hyperglycemia onset prevented an increase in retinal vascular permeability (221). Although the sample size was relatively low, the results suggest that scFv immunotherapy may be beneficial in early stages of diabetic retinopathy, however, further research would of course be necessary.
Klotho
Klotho is a transmembrane protein expressed predominantly in the kidneys and parts of the brain as well as other regions of the body in lower concentrations (222). The ecto domain, soluble klotho (sKL), acts as a hormone, activating many molecular pathways (223). It is often associated with anti-aging and has noted beneficial systemic effects, namely suppression of nuclear factor NF-κB activation and increasing lifespan (224). In db/db mice, renal Klotho (mRNA and protein) is significantly lower, further suggesting the role of Klotho as an agent of anti-inflammation (225). This is also true with regards to serum levels of soluble Klotho during T1D onset, where children have significantly lower levels compared to healthy controls (226). Furthermore, Klotho deficiency promotes β-cell apoptosis in T1D, where over-expression in turn improved β-cell function and offered a protective effect (224). By reducing the effect of the NF-κB pathway, adhesion molecules ICAM-1 and VCAM-1 are suppressed, preventing endothelial dysfunction.
The use of sKL for its anti-fibrotic effect in the kidney is of particular interest to the discussion of novel targets for DN. One mechanism suggested for nephroprotection is via the blockade of profibrotic TGFβ1 signalling (223). The problem with sKL however, is its relatively short half-life and large size, which is hard to reproduce in bioactive form. In their 2022 review, Isakova, Yanucil and Faul discussed research on one of two peptide domains on sKL, namely KL1 (223, 227). Although its role is largely unknown, it is thought to be involved in TGFβ1 signalling. It was further discussed in the same review that KP1 binds to TGFβ receptor 2, thus disrupting normal functioning of TGFβ signalling such as myofibroblast activation. In vivo studies show that KL1 has preference for accumulation in the kidney cells after intravenous injection and has antifibrotic properties (227).
With a length of 30 amino acids, KL1 is much smaller than sKL, and therefore easier and cheaper to replicate. However, a large volume of studies must still be conducted to assess various aspects of safety. Klotho appears to answer many of the issues seen in diabetic complications and onset, however much still remains to be learned regarding its potential as a therapeutic. Its promise as a protein that can offer protective effects in both early onset of diabetes as well as the subsequent complications cannot be understated.
DNAse I
In 1997, a landmark paper by Takei et al. documented the neutrophil’s ability to dispense decondensed chromatin into the extracellular space to form a neutrophil extracellular trap (NET), later interpreted and termed by Brinkman et al. (228, 229). Subsequent research has recorded disruptive behaviour as a result of NET formation in vivo such as clot formation as well as sterile inflammation in cancer (230, 231). NET formation has also been observed in diabetes and is thought to be involved in many complications from diabetes (230, 232, 233). Neutrophils follow chemokine gradients in order to find pathogens or damaged tissues and often, when forming NETs, release more proinflammatory cytokines (234). Although the exact mechanism behind NETosis is still unclear, NETs are known to form in hyper glycemic environments with lower pH and high ROS (40, 234).
Deoxyribose nuclease I (DNase I) is an in vivo serum endonuclease excreted by non hematopoietic cells. Among other purposes, DNase I acts to remove NETs by targeting the phosphodiester bonds of the exposed DNA. A study by Jiménez-Alcázar et al. showed that in vivo, two forms of DNase I (DNase I and DNase 1 like 3) work to degrade NETs in circulation (235). A genetically engineered form of recombinant human DNase I (called Dornase Alfa) is already used for human patients with cystic fibrosis (236, 237). After inhaling recombinant human DNase I (rhDNase), patients showed an increase in their ability to clear airway obstructions compared to healthy control groups (238). RhDNA is safe, with no immune reactions, local irritation or major adverse events. A study conducted by Davis et al. evaluated an IV injection of rhDNase in patients with SLE. They found no immune response or other adverse effects pre or post treatment to rhDNase following IV administration. It was further observed by the group that serum rhDNase concentrations were still sufficient for the hydrolysis of NETs after a few hours post administration (239).
More research into the effects of NETosis with regards to diabetic complications provides interesting results. Circulating biomarkers for Netosis include circulating DNA–histone complex and polymorphonuclear neutrophil elastase levels are elevated in diabetics with DR compared to diabetics without DR (240). A multivariable logistic regression model, accounting for fasting blood glucose and HbA1c levels, highlighted that NET formation biomarkers are significant independent risk factors for those with DR (240, 241).
These studies among others are beginning to highlight the importance of considering the role of NETs in diabetic complications. By developing further forms of DNase I with an increased half-life, such as the IV study conducted by Davis et al., investigations into the effects of NET formation on diabetic complications could provide insight into novel target therapies.
Conclusion
As the global incidence of diabetes is increasing, more people are proportionally likely to develop diabetic complications as a result. Current medication mainly provides pain relief in order to better the patient’s quality of life. With increasing evidence validating chronic inflammation and the immune system’s impact on the development of diabetic complications, immunotherapy may play a vital role in the development of new medications for such conditions.
The suggested therapies of increasing Treg cell numbers via IL-35, CRISPR, MSCs or administration of their secretions (TGF-β) has been shown in diabetic animal models to restore immune homeostasis and return glycemic values to appropriate levels. By targeting other pro-inflammation mediators such as IL-6 or IL-17A one may further support the development of Treg cells. Other anti-inflammation therapies such as anti-ceramide treatment or klotho may take some more time for development, but hold great potential as novel targets. More investigations into DNase I in diabetics may prevent NET formation and have a beneficial effect overall.
Although prevention is the best means to avoid diabetic complications, great progress has been made by research teams in investigating novel targets of the immune system with the aim to prevent disease development. Consideration of the above therapies with a good glycemic control could potentially improve the quality of life for those living with diabetes.
Author contributions
Conception: KS; research: LR, KS and ZL; LR and KS wrote the manuscript. KS arranged research fundings and supervised the study, which was revised by ZL; diagrams: LR. All authors contributed to the article and approved the submitted version.
Funding
This study was supported by the Swedish Research Council (2018-06563), the Swedish Diabetes Foundation, ExoDiab, Barndiabetesfonden, O.E. och Edla Johanssons, Sederholms fund, Magnus Bergvalls Stiftelse, the Ernfors Fund, Nils Erik Holmstens and SEB-Diabetesfonden. KS was supported from Swedish Research Council (2018-06563), Olga Jonsson and EFIS-IL fellowship.
Conflict of interest
The authors declare that the research was conducted in the absence of any commercial or financial relationships that could be construed as a potential conflict of interest.
Publisher’s note
All claims expressed in this article are solely those of the authors and do not necessarily represent those of their affiliated organizations, or those of the publisher, the editors and the reviewers. Any product that may be evaluated in this article, or claim that may be made by its manufacturer, is not guaranteed or endorsed by the publisher.
References
1. Diabetes. Available at: https://www.who.int/health-topics/diabetes (Accessed 9 November 2022).
2. Saeedi P, Petersohn I, Salpea P, Malanda B, Karuranga S, Unwin N, et al. Global and regional diabetes prevalence estimates for 2019 and projections for 2030 and 2045: results from the international diabetes federation diabetes atlas, 9th edition. Diabetes Res Clin Pract (2019) 157:107843. doi: 10.1016/j.diabres.2019.107843
3. Appuhamy J.A.D.R.N., Kebreab E, Simon M, Yada R, Milligan LP, France J. Effects of diet and exercise interventions on diabetes risk factors in adults without diabetes: meta-analyses of controlled trials. Diabetol Metab Syndr (2014) 6:127. doi: 10.1186/1758-5996-6-127
4. Saeedi P, Salpea P, Karuranga S, Petersohn I, Malanda B, Gregg EW, et al. Mortality attributable to diabetes in 20–79 years old adults, 2019 estimates: results from the international diabetes federation diabetes atlas, 9th edition. Diabetes Res Clin Pract (2020) 162:108086. doi: 10.1016/j.diabres.2020.108086
5. Wang W, Lo ACY. Diabetic retinopathy: pathophysiology and treatments. Int J Mol Sci (2018) 19:1816. doi: 10.3390/ijms19061816
6. Teo ZL, Tham Y-C, Yu M, Chee ML, Rim TH, Cheung N, et al. Global prevalence of diabetic retinopathy and projection of burden through 2045: systematic review and meta-analysis. Ophthalmology (2021) 128:1580–91. doi: 10.1016/j.ophtha.2021.04.027
7. Shukla UV, Tripathy K. Diabetic retinopathy. Treasure Island (FL: StatPearls; StatPearls Publishing (2022).
8. Wilkinson-Berka JL, Miller AG. Update on the treatment of diabetic retinopathy. Sci World J (2008) 8:98–120. doi: 10.1100/tsw.2008.25
9. Sabanayagam C, Banu R, Chee ML, Lee R, Wang YX, Tan G, et al. Incidence and progression of diabetic retinopathy: a systematic review. Lancet Diabetes Endocrinol (2019) 7:140–9. doi: 10.1016/S2213-8587(18)30128-1
10. Yan L-J. Pathogenesis of chronic hyperglycemia: from reductive stress to oxidative stress. J Diabetes Res (2014) 2014:137919. doi: 10.1155/2014/137919
11. Urbaniak SK, Boguszewska K, Szewczuk M. Kaźmierczak-barańska, j.; karwowski, B.T. 8-Oxo-7,8-Dihydro-2’-Deoxyguanosine (8-OxodG) and 8-Hydroxy-2’-Deoxyguanosine (8-OHdG) as a potential biomarker for gestational diabetes mellitus (GDM) development. Mol Basel Switz (2020) 25:202. doi: 10.3390/molecules25010202
12. Draznin B, Gilden J, Golden SH, Inzucchi SE. Pathways to quality inpatient management of hyperglycemia and diabetes: a call to action. Diabetes Care (2013) 36:1807–14. doi: 10.2337/dc12-2508
13. Lorenzi M. The polyol pathway as a mechanism for diabetic retinopathy: attractive, elusive, and resilient. Exp Diabetes Res (2007) 2007:61038. doi: 10.1155/2007/61038
14. Srikanth KK, Orrick JA. Biochemistry, polyol or sorbitol pathways. Treasure Island (FL: StatPearls; StatPearls Publishing (2022).
15. Naeser P, Brolin SE, Eriksson UJ. Sorbitol metabolism in the retina, optic nerve, and sural nerve of diabetic rats treated with an aldose reductase inhibitor. Metab - Clin Exp (1988) 37:1143–5. doi: 10.1016/0026-0495(88)90191-6
16. Dagher Z, Park YS, Asnaghi V, Hoehn T, Gerhardinger C, Lorenzi M. Studies of rat and human retinas predict a role for the polyol pathway in human diabetic retinopathy. Diabetes (2004) 53:2404–11. doi: 10.2337/diabetes.53.9.2404
17. Stribling D, Mirrlees DJ, Harrison HE, Earl DCN. Properties of ICI 128,436, a novel aldose reductase inhibitor, and its effects on diabetic complications in the rat. Metab - Clin Exp (1985) 34:336–44. doi: 10.1016/0026-0495(85)90223-9
18. Poulsom R, Mirrlees DJ, Earl DC, Heath H. The effects of an aldose reductase inhibitor upon the sorbitol pathway, fructose-1-Phosphate and lactate in the retina and nerve of streptozotocin-diabetic rats. Exp Eye Res (1983) 36:751–60. doi: 10.1016/0014-4835(83)90112-4
19. Gillon KR, King RH, Thomas PK. The pathology of diabetic neuropathy and the effects of aldose reductase inhibitors. Clin Endocrinol Metab (1986) 15:837–53. doi: 10.1016/s0300-595x(86)80077-9
20. Naeser P, Brolin SE, Berggren PO. The sorbitol shunt in the retina and the optic nerve of mice with inherited and STZ-induced diabetes. Acta Ophthalmol (Copenh.) (1987) 65:693–8. doi: 10.1111/j.1755-3768.1987.tb07065.x
21. Zhang W, Liu H, Rojas M, Caldwell RW, Caldwell RB. Anti-inflammatory therapy for diabetic retinopathy. Immunotherapy (2011) 3:609–28. doi: 10.2217/imt.11.24
22. Arden GB, Sivaprasad S. Hypoxia and oxidative stress in the causation of diabetic retinopathy. Curr Diabetes Rev (2011) 7:291–304. doi: 10.2174/157339911797415620
23. Cai S, Yang Q, Hou M, Han Q, Zhang H, Wang J, et al. A-Melanocyte-Stimulating hormone protects early diabetic retina from blood-retinal barrier breakdown and vascular leakage via MC4R. Cell Physiol Biochem Int J Exp Cell Physiol Biochem Pharmacol (2018) 45:505–22. doi: 10.1159/000487029
24. Lin M, Chen Y, Jin J, Hu Y, Zhou KK, Zhu M, et al. Ischaemia-induced retinal neovascularisation and diabetic retinopathy in mice with conditional knockout of hypoxia-inducible factor-1 in retinal müller cells. Diabetologia (2011) 54:1554–66. doi: 10.1007/s00125-011-2081-0
25. Anitha RE, Janani R, Peethambaran D, Baskaran V. Lactucaxanthin protects retinal pigment epithelium from hyperglycemia-regulated Hypoxia/ER Stress/VEGF pathway mediated angiogenesis in ARPE-19 cell and rat model. Eur J Pharmacol (2021) 899:174014. doi: 10.1016/j.ejphar.2021.174014
26. Lazzara F, Trotta MC, Platania CBM, D’Amico M, Petrillo F, Galdiero M, et al. Stabilization of HIF-1α in human retinal endothelial cells modulates expression of MiRNAs and proangiogenic growth factors. Front Pharmacol (2020) 11:1063. doi: 10.3389/fphar.2020.01063
27. Davidson JA, Ciulla TA, McGill JB, Kles KA, Anderson PW. How the diabetic eye loses vision. Endocrine (2007) 32:107–16. doi: 10.1007/s12020-007-0040-9
28. Wan T-T, Li X-F, Sun Y-M, Li Y-B, Su Y. Recent advances in understanding the biochemical and molecular mechanism of diabetic retinopathy. Biomed Pharmacother (2015) 74:145–7. doi: 10.1016/j.biopha.2015.08.002
29. Forrester JV, Kuffova L, Delibegovic M. The role of inflammation in diabetic retinopathy. Front Immunol (2020) 11. doi: 10.3389/fimmu.2020.583687
30. Rübsam A, Parikh S, Fort PE. Role of inflammation in diabetic retinopathy. Int J Mol Sci (2018) 19:942. doi: 10.3390/ijms19040942
31. Pan WW, Lin F, Fort PE. The innate immune system in diabetic retinopathy. Prog Retin Eye Res (2021) 84:100940. doi: 10.1016/j.preteyeres.2021.100940
32. Capozzi ME, McCollum GW, Cousins DB, Penn JS. Linoleic acid is a diabetes-relevant stimulator of retinal inflammation in human retinal Muller cells and microvascular endothelial cells. J Diabetes Metab (2016) 7:718. doi: 10.4172/2155-6156.1000718
33. Howard-Williams J, Patel P, Jelfs R, Carter RD, Awdry P, Bron A, et al. Polyunsaturated fatty acids and diabetic retinopathy. Br J Ophthalmol (1985) 69:15–8. doi: 10.1136/bjo.69.1.15
34. Dong L, Bai J, Jiang X, Yang M-M, Zheng Y, Zhang H, et al. The gene polymorphisms of IL-8(-251T/A) and IP-10(-1596C/T) are associated with susceptibility and progression of type 2 diabetic retinopathy in northern Chinese population. Eye Lond Engl (2017) 31:601–7. doi: 10.1038/eye.2016.287
35. Feng S, Yu H, Yu Y, Geng Y, Li D, Yang C, et al. Levels of inflammatory cytokines IL-1β, IL-6, IL-8, IL-17A, and TNF-α in aqueous humour of patients with diabetic retinopathy. J Diabetes Res (2018) 2018:8546423. doi: 10.1155/2018/8546423
36. Yenihayat F, Özkan B, Kasap M, Karabaş VL, Güzel N, Akpınar G, et al. Vitreous IL-8 and VEGF levels in diabetic macular edema with or without subretinal fluid. Int Ophthalmol (2019) 39:821–8. doi: 10.1007/s10792-018-0874-6
37. Koskela UE, Kuusisto SM, Nissinen AE, Savolainen MJ, Liinamaa MJ. High vitreous concentration of IL-6 and IL-8, but not of adhesion molecules in relation to plasma concentrations in proliferative diabetic retinopathy. Ophthalmic Res (2013) 49:108–14. doi: 10.1159/000342977
38. Hernández C, Segura RM, Fonollosa A, Carrasco E, Francisco G, Simó R. Interleukin-8, monocyte chemoattractant protein-1 and IL-10 in the vitreous fluid of patients with proliferative diabetic retinopathy. Diabet Med (2005) 22:719–22. doi: 10.1111/j.1464-5491.2005.01538.x
39. Liu H, Lessieur EM, Saadane A, Lindstrom SI, Taylor PR, Kern TS. Neutrophil elastase contributes to the pathological vascular permeability characteristic of diabetic retinopathy. Diabetologia (2019) 62:2365–74. doi: 10.1007/s00125-019-04998-4
40. Wang L, Zhou X, Yin Y, Mai Y, Wang D, Zhang X. Hyperglycemia induces neutrophil extracellular traps formation through an NADPH oxidase-dependent pathway in diabetic retinopathy. Front Immunol (2019) 9:3076. doi: 10.3389/fimmu.2018.03076
41. Jiang N, Chen X-L, Yang H-W, Ma Y-R. Effects of nuclear factor KB expression on retinal neovascularization and apoptosis in a diabetic retinopathy rat model. Int J Ophthalmol (2015) 8:448–52. doi: 10.3980/j.issn.2222-3959.2015.03.03
42. Feenstra DJ, Yego EC, Mohr S. Modes of retinal cell death in diabetic retinopathy. J Clin Exp Ophthalmol (2013) 4:298. doi: 10.4172/2155-9570.1000298
43. Yang I, Han SJ, Kaur G, Crane C, Parsa AT. The role of microglia in central nervous system immunity and glioma immunology. J Clin Neurosci Off J Neurosurg Soc Australas (2010) 17:6–10. doi: 10.1016/j.jocn.2009.05.006
44. Kinuthia UM, Wolf A, Langmann T. Microglia and inflammatory responses in diabetic retinopathy. Front Immunol (2020) 11. doi: 10.3389/fimmu.2020.564077
45. Mirshahi A, Hoehn R, Lorenz K, Kramann C, Baatz H. Anti-tumor necrosis factor alpha for retinal diseases: current knowledge and future concepts. J Ophthalmic Vis Res (2012) 7:39–44.
46. Yao Y, Li R, Du J, Li X, Zhao L, Long L, et al. Tumor necrosis factor-α and diabetic retinopathy: review and meta-analysis. Clin Chim Acta (2018) 485:210–7. doi: 10.1016/j.cca.2018.06.028
47. Demircan N, Safran BG, Soylu M, Ozcan AA, Sizmaz S. Determination of vitreous interleukin-1 (IL-1) and tumour necrosis factor (TNF) levels in proliferative diabetic retinopathy. Eye (2006) 20:1366–9. doi: 10.1038/sj.eye.6702138
48. Kim G-Y, Lee J-W, Ryu H-C, Wei J-D, Seong C-M, Kim J-H. Proinflammatory cytokine IL-1beta stimulates IL-8 synthesis in mast cells via a leukotriene B4 receptor 2-linked pathway, contributing to angiogenesis. J Immunol Baltim Md 1950 (2010) 184:3946–54. doi: 10.4049/jimmunol.0901735
49. Diabetic retinopathy - treatment. Available at: https://www.nhs.uk/conditions/diabetic-retinopathy/treatment/ (Accessed 10 October 2022).
50. Simó R, Sundstrom JM, Antonetti DA. Ocular anti-VEGF therapy for diabetic retinopathy: the role of VEGF in the pathogenesis of diabetic retinopathy. Diabetes Care (2014) 37:893–9. doi: 10.2337/dc13-2002
51. Titchenell PM, Antonetti DA. Using the past to inform the future: anti-VEGF therapy as a road map to develop novel therapies for diabetic retinopathy. Diabetes (2013) 62:1808–15. doi: 10.2337/db12-1744
53. Papadopoulos N, Martin J, Ruan Q, Rafique A, Rosconi MP, Shi E, et al. Binding and neutralization of vascular endothelial growth factor (VEGF) and related ligands by VEGF trap, ranibizumab and bevacizumab. Angiogenesis (2012) 15:171–85. doi: 10.1007/s10456-011-9249-6
54. Da Pozzo E, Tremolanti C, Costa B, Giacomelli C, Milenkovic VM, Bader S, et al. Microglial pro-inflammatory and anti-inflammatory phenotypes are modulated by translocator protein activation. Int J Mol Sci (2019) 20:4467. doi: 10.3390/ijms20184467
55. Guo S, Wang H, Yin Y. Microglia polarization from M1 to M2 in neurodegenerative diseases. Front Aging Neurosci (2022) 14. doi: 10.3389/fnagi.2022.815347
56. Cameron JS. The discovery of diabetic nephropathy: from small print to centre stage. J Nephrol (2006) 19 Suppl 10:S75–87.
57. Hussain S, Chand Jamali M, Habib A, Hussain MS, Akhtar M, Najmi AK. Diabetic kidney disease: an overview of prevalence, risk factors, and biomarkers. Clin Epidemiol Glob Health (2021) 9:2–6. doi: 10.1016/j.cegh.2020.05.016
58. Forbes JM, Cooper ME. Mechanisms of diabetic complications. Physiol Rev (2013) 93:137–88. doi: 10.1152/physrev.00045.2011
59. Sapra A, Bhandari P. Diabetes mellitus. Treasure Island (FL: StatPearls; StatPearls Publishing (2022).
60. Ling W, Huang Y, Huang Y-M, Fan R-R, Sui Y, Zhao H-L. Global trend of diabetes mortality attributed to vascular complications, 2000–2016. Cardiovasc Diabetol (2020) 19:182. doi: 10.1186/s12933-020-01159-5
61. Chen J, Liu Q, He J, Li Y. Immune responses in diabetic nephropathy: pathogenic mechanisms and therapeutic target. Front Immunol (2022) 13:958790. doi: 10.3389/fimmu.2022.958790
62. Thomas MC, Brownlee M, Susztak K, Sharma K, Jandeleit-Dahm KAM, Zoungas S, et al. Diabetic kidney disease. Nat Rev Dis Primer (2015) 1:15018. doi: 10.1038/nrdp.2015.18
63. Zheng Z, Zheng F. Immune cells and inflammation in diabetic nephropathy. J Diabetes Res (2016) 2016:10. doi: 10.1155/2016/1841690
64. Goto M, Wakai K, Kawamura T, Ando M, Endoh M, Tomino YA. Scoring system to predict renal outcome in IgA nephropathy: a nationwide 10-year prospective cohort study. Nephrol Dial Transplant (2009) 24:3068–74. doi: 10.1093/ndt/gfp273
65. Zeisberg M, Neilson EG. Mechanisms of tubulointerstitial fibrosis. J Am Soc Nephrol (2010) 21:1819–34. doi: 10.1681/ASN.2010080793
66. Cao Z, Cooper ME. Pathogenesis of diabetic nephropathy. J Diabetes Investig (2011) 2:243–7. doi: 10.1111/j.2040-1124.2011.00131.x
67. Sulaiman MK. Diabetic nephropathy: recent advances in pathophysiology and challenges in dietary management. Diabetol Metab Syndr (2019) 11:7. doi: 10.1186/s13098-019-0403-4
68. Selby NM, Taal MW. An updated overview of diabetic nephropathy: diagnosis, prognosis, treatment goals and latest guidelines. Diabetes Obes Metab (2020) 22:3–15. doi: 10.1111/dom.14007
69. Tang SCW, Yiu WH. Innate immunity in diabetic kidney disease. Nat Rev Nephrol (2020) 16:206–22. doi: 10.1038/s41581-019-0234-4
70. Zoja C, Xinaris C, Macconi D. Diabetic nephropathy: novel molecular mechanisms and therapeutic targets. Front Pharmacol (2020) 11. doi: 10.3389/fphar.2020.586892
71. Donate-Correa J, Ferri CM, Sánchez-Quintana F, Pérez-Castro A, González-Luis A, Martín-Núñez E, et al. Inflammatory cytokines in diabetic kidney disease: pathophysiologic and therapeutic implications. Front Med (2021) 7. doi: 10.3389/fmed.2020.628289
72. Chow F, Ozols E, Nikolic-Paterson DJ, Atkins RC, Tesch GH. Macrophages in mouse type 2 diabetic nephropathy: correlation with diabetic state and progressive renal injury. Kidney Int (2004) 65:116–28. doi: 10.1111/j.1523-1755.2004.00367.x
73. Navarro-González JF, Mora-Fernández C. The role of inflammatory cytokines in diabetic nephropathy. J Am Soc Nephrol (2008) 19:433–42. doi: 10.1681/ASN.2007091048
74. Sassy-Prigent C, Heudes D, Mandet C, Bélair MF, Michel O, Perdereau B, et al. Early glomerular macrophage recruitment in streptozotocin-induced diabetic rats. Diabetes (2000) 49:466–75. doi: 10.2337/diabetes.49.3.466
75. Hickey FB, Martin F. Diabetic kidney disease and immune modulation. Curr Opin Pharmacol (2013) 13:602–12. doi: 10.1016/j.coph.2013.05.002
76. Turner MD, Nedjai B, Hurst T, Pennington DJ. Cytokines and chemokines: At the crossroads of cell signalling and inflammatory disease. Biochim Biophys Acta BBA - Mol Cell Res (2014) 1843:2563–82. doi: 10.1016/j.bbamcr.2014.05.014
77. Kong L, Andrikopoulos S, MacIsaac RJ, Mackay LK, Nikolic-Paterson DJ, Torkamani N, et al. Role of the adaptive immune system in diabetic kidney disease. J Diabetes Investig (2022) 13:213–26. doi: 10.1111/jdi.13725
78. Araújo LS, Torquato BGS, da Silva CA, dos Reis Monteiro MLG, dos Santos Martins ALM, da Silva MV, et al. Renal expression of cytokines and chemokines in diabetic nephropathy. BMC Nephrol (2020) 21:308. doi: 10.1186/s12882-020-01960-0
79. Klessens CQF, Zandbergen M, Wolterbeek R, Bruijn JA, Rabelink TJ, Bajema IM, et al. Macrophages in diabetic nephropathy in patients with type 2 diabetes. Nephrol Dial Transplant Off Publ Eur Dial Transpl Assoc - Eur Ren Assoc (2017) 32:1322–9. doi: 10.1093/ndt/gfw260
80. You H, Gao T, Cooper TK, Brian Reeves W, Awad AS. Macrophages directly mediate diabetic renal injury. Am J Physiol - Ren Physiol (2013) 305:F1719–27. doi: 10.1152/ajprenal.00141.2013
81. Mehta AK, Gracias DT, Croft M. TNF activity and T cells. Cytokine (2018) 101:14–8. doi: 10.1016/j.cyto.2016.08.003
82. Schoenborn JR, Wilson CB. Regulation of interferon-γ during innate and adaptive immune responses. In: Advances in immunology, vol. 96. Academic Press (2007). p. 41–101.
83. Moon J-Y, Jeong K-H, Lee T-W, Ihm C-G, Lim SJ, Lee S-H. Aberrant recruitment and activation of T cells in diabetic nephropathy. Am J Nephrol (2012) 35:164–74. doi: 10.1159/000334928
84. Piconese S, Walker LSK, Dominguez-Villar M. Editorial: control of regulatory T cell stability, plasticity, and function in health and disease. Front Immunol (2021) 11. doi: 10.3389/fimmu.2020.611591
85. DuPage M, Bluestone JA. Harnessing the plasticity of CD4+ T cells to treat immune-mediated disease. Nat Rev Immunol (2016) 16:149–63. doi: 10.1038/nri.2015.18
86. Komatsu N, Mariotti-Ferrandiz ME, Wang Y, Malissen B, Waldmann H, Hori S. Heterogeneity of natural Foxp3+ T cells: a committed regulatory T-cell lineage and an uncommitted minor population retaining plasticity. Proc Natl Acad Sci (2009) 106:1903–8. doi: 10.1073/pnas.0811556106
87. Shi H, Chi H. Metabolic control of treg cell stability, plasticity, and tissue-specific heterogeneity. Front Immunol (2019) 10. doi: 10.3389/fimmu.2019.02716
88. McClymont SA, Putnam AL, Lee MR, Esensten JH, Liu W, Hulme MA, et al. Plasticity of human regulatory T cells in healthy subjects and patients with type 1 diabetes. J Immunol (2011) 186:3918–26. doi: 10.4049/jimmunol.1003099
89. Crotty S. Follicular helper CD4 T cells (TFH). Annu Rev Immunol (2011) 29:621–63. doi: 10.1146/annurev-immunol-031210-101400
90. Golubovskaya V, Wu L. Different subsets of T cells, memory, effector functions, and CAR-T immunotherapy. Cancers (2016) 8:36. doi: 10.3390/cancers8030036
91. Peng X, Xiao Z, Zhang J, Li Y, Dong Y, Du J. IL-17A produced by both Γδ T and Th17 cells promotes renal fibrosis via RANTES-mediated leukocyte infiltration after renal obstruction. J Pathol (2015) 235:79–89. doi: 10.1002/path.4430
92. Gaffen SL. Recent advances in the IL-17 cytokine family. Curr Opin Immunol (2011) 23:613–9. doi: 10.1016/j.coi.2011.07.006
93. Sato M, Muragaki Y, Saika S, Roberts AB, Ooshima A. Targeted disruption of TGF-B1/Smad3 signaling protects against renal tubulointerstitial fibrosis induced by unilateral ureteral obstruction. J Clin Invest (2003) 112:1486–94. doi: 10.1172/JCI200319270
94. Breyer MD, Susztak K. THE NEXT GENERATION OF THERAPEUTICS FOR CHRONIC KIDNEY DISEASE. Nat Rev Drug Discov (2016) 15:568–88. doi: 10.1038/nrd.2016.67
95. ZHOU W, LIU Y, Hu Q, ZHOU J, LIN H. The landscape of immune cell infiltration in the glomerulus of diabetic nephropathy: evidence based on bioinformatics. BMC Nephrol (2022) 23:303. doi: 10.1186/s12882-022-02906-4
97. Dewanjee S, Das S, Das AK, Bhattacharjee N, Dihingia A, Dua TK, et al. Molecular mechanism of diabetic neuropathy and its pharmacotherapeutic targets. Eur J Pharmacol (2018) 833:472–523. doi: 10.1016/j.ejphar.2018.06.034
98. Bodman MA, Varacallo M. Peripheral diabetic neuropathy. Treasure Island (FL: StatPearls; StatPearls Publishing (2022).
99. Hicks CW, Selvin E. Epidemiology of peripheral neuropathy and lower extremity disease in diabetes. Curr Diab Rep (2019) 19:86. doi: 10.1007/s11892-019-1212-8
100. Callaghan BC, Cheng H, Stables CL, Smith AL, Feldman EL. Diabetic neuropathy: clinical manifestations and current treatments. Lancet Neurol (2012) 11:521–34. doi: 10.1016/S1474-4422(12)70065-0
101. Yu Y. Gold standard for diagnosis of DPN. Front Endocrinol (2021) 12:719356. doi: 10.3389/fendo.2021.719356
102. Feldman EL, Bennett DLH, Nave K-A, Jensen TS. New horizons in diabetic neuropathy: mechanisms, bioenergetics, and pain. Neuron (2017) 93:1296–313. doi: 10.1016/j.neuron.2017.02.005
103. Román-Pintos LM, Villegas-Rivera G, Rodríguez-Carrizalez AD, Miranda-Díaz AG, Cardona-Muñoz EG. Diabetic polyneuropathy in type 2 diabetes mellitus: inflammation, oxidative stress, and mitochondrial function. J Diabetes Res (2016) 2016:3425617. doi: 10.1155/2016/3425617
104. Akinrodoye MA, Lui F. Neuroanatomy, somatic nervous system. Treasure Island (FL: StatPearls; StatPearls Publishing (2022).
105. Cascio MA, Mukhdomi T. Small fiber neuropathy. Treasure Island (FL: StatPearls; StatPearls Publishing (2022).
106. Ristikj-Stomnaroska D, Risteska-Nejashmikj V, Papazova M. Role of inflammation in the pathogenesis of diabetic peripheral neuropathy. Open Access Maced J Med Sci (2019) 7:2267–70. doi: 10.3889/oamjms.2019.646
107. Mu Z-P, Wang Y-G, Li C-Q, Lv W-S, Wang B, Jing Z-H, et al. Association between tumor necrosis factor-α and diabetic peripheral neuropathy in patients with type 2 diabetes: a meta-analysis. Mol Neurobiol (2017) 54:983–96. doi: 10.1007/s12035-016-9702-z
108. Li X, Wu T-T, Chen J, Qiu W. Elevated expression levels of serum insulin-like growth factor-1, tumor necrosis factor-α and vascular endothelial growth factor 165 might exacerbate type 2 diabetic nephropathy. J Diabetes Investig (2017) 8:108–14. doi: 10.1111/jdi.12542
109. Empl M, Renaud S, Erne B, Fuhr P, Straube A, Schaeren-Wiemers N, et al. TNF-alpha expression in painful and nonpainful neuropathies. Neurology (2001) 56:1371–7. doi: 10.1212/wnl.56.10.1371
110. Shi X, Chen Y, Nadeem L, Xu G. Beneficial effect of TNF-α inhibition on diabetic peripheral neuropathy. J Neuroinflamm (2013) 10:69. doi: 10.1186/1742-2094-10-69
111. Navarro JF, Mora C, Muros M, García J. Urinary tumour necrosis factor-α excretion independently correlates with clinical markers of glomerular and tubulointerstitial injury in type 2 diabetic patients. Nephrol Dial Transplant (2006) 21:3428–34. doi: 10.1093/ndt/gfl469
112. Li X, Zhu J, Liu N, Liu J, Zhang Z. TNF-alpha in peripheral neuropathy patients with impaired glucose regulation. J Diabetes Res (2017) 2017:7024024. doi: 10.1155/2017/7024024
113. Cermenati G, Abbiati F, Cermenati S, Brioschi E, Volonterio A, Cavaletti G, et al. Diabetes-induced myelin abnormalities are associated with an altered lipid pattern: protective effects of LXR activation. J Lipid Res (2012) 53:300–10. doi: 10.1194/jlr.M021188
114. Fromont A, De Seze J, Fleury MC, Maillefert JF, Moreau T. Inflammatory demyelinating events following treatment with anti-tumor necrosis factor. Cytokine (2009) 45:55–7. doi: 10.1016/j.cyto.2008.11.002
115. Jurewicz A, Matysiak M, Tybor K, Selmaj K. TNF-induced death of adult human oligodendrocytes is mediated by c-jun NH2-terminal kinase-3. Brain (2003) 126:1358–70. doi: 10.1093/brain/awg146
116. Pop-Busui R, Boulton AJM, Feldman EL, Bril V, Freeman R, Malik RA, et al. Diabetic neuropathy: a position statement by the American diabetes association. Diabetes Care (2017) 40:136–54. doi: 10.2337/dc16-2042
117. Trammell SAJ, Weidemann BJ, Chadda A, Yorek MS, Holmes A, Coppey LJ, et al. Nicotinamide riboside opposes type 2 diabetes and neuropathy in mice. Sci Rep (2016) 6:26933. doi: 10.1038/srep26933
118. Cantó C, Houtkooper RH, Pirinen E, Youn DY, Oosterveer MH, Cen Y, et al. The NAD+ precursor nicotinamide riboside enhances oxidative metabolism and protects against high-fat diet induced obesity. Cell Metab (2012) 15:838–47. doi: 10.1016/j.cmet.2012.04.022
119. Narayanan S. Aldose reductase and its inhibition in the control of diabetic complications. Ann Clin Lab Sci (1993) 23:148–58.
120. Matoba K, Takeda Y, Nagai Y, Yokota T, Utsunomiya K, Nishimura R. Targeting redox imbalance as an approach for diabetic kidney disease. Biomedicines (2020) 8:40. doi: 10.3390/biomedicines8020040
121. Kawanami D, Matoba K, Takeda Y, Nagai Y, Akamine T, Yokota T, et al. SGLT2 inhibitors as a therapeutic option for diabetic nephropathy. Int J Mol Sci (2017) 18:1083. doi: 10.3390/ijms18051083
122. Fujita H, Morii T, Fujishima H, Sato T, Shimizu T, Hosoba M, et al. The protective roles of GLP-1R signaling in diabetic nephropathy: possible mechanism and therapeutic potential. Kidney Int (2014) 85:579–89. doi: 10.1038/ki.2013.427
123. Kawai Y, Uneda K, Yamada T, Kinguchi S, Kobayashi K, Azushima K, et al. Comparison of effects of SGLT-2 inhibitors and GLP-1 receptor agonists on cardiovascular and renal outcomes in type 2 diabetes mellitus patients with/without albuminuria: a systematic review and network meta-analysis. Diabetes Res Clin Pract (2022) 183:109146. doi: 10.1016/j.diabres.2021.109146
124. Chalk C, Benstead TJ, Moore F. Aldose reductase inhibitors for the treatment of diabetic polyneuropathy. Cochrane Database Syst Rev (2007) 2007:CD004572. doi: 10.1002/14651858.CD004572.pub2
125. Jannapureddy S, Sharma M, Yepuri G, Schmidt AM, Ramasamy R. Aldose reductase: an emerging target for development of interventions for diabetic cardiovascular complications. Front Endocrinol (2021) 12:636267. doi: 10.3389/fendo.2021.636267
126. Pahwa R, Goyal A, Jialal I. Chronic inflammation. Treasure Island (FL: StatPearls; StatPearls Publishing (2022).
127. Robertson RP. Chronic oxidative stress as a central mechanism for glucose toxicity in pancreatic islet beta cells in diabetes*. J Biol Chem (2004) 279:42351–4. doi: 10.1074/jbc.R400019200
128. Ramasamy R, Yan SF, Schmidt AM. Receptor for AGE (RAGE): signaling mechanisms in the pathogenesis of diabetes and its complications. Ann N Y Acad Sci (2011) 1243:88–102. doi: 10.1111/j.1749-6632.2011.06320.x
129. Duran-Salgado MB, Rubio-Guerra AF. Diabetic nephropathy and inflammation. World J Diabetes (2014) 5:393–8. doi: 10.4239/wjd.v5.i3.393
130. Zhang X, Yang Y, Zhao Y. Macrophage phenotype and its relationship with renal function in human diabetic nephropathy. PloS One (2019) 14:e0221991. doi: 10.1371/journal.pone.0221991
131. Harbour SN, DiToro DF, Witte SJ, Zindl CL, Gao M, Schoeb TR, et al. Th17 cells require ongoing classic IL-6 receptor signaling to retain transcriptional and functional identity. Sci Immunol (2020) 5:eaaw2262. doi: 10.1126/sciimmunol.aaw2262
132. Bettelli E, Carrier Y, Gao W, Korn T, Strom TB, Oukka M, et al. Reciprocal developmental pathways for the generation of pathogenic effector TH17 and regulatory T cells. Nature (2006) 441:235–8. doi: 10.1038/nature04753
133. Veldhoen M, Hocking RJ, Atkins CJ, Locksley RM, Stockinger B. TGFβ in the context of an inflammatory cytokine milieu supports De novo differentiation of IL-17-Producing T cells. Immunity (2006) 24:179–89. doi: 10.1016/j.immuni.2006.01.001
134. Flyvbjerg A. The role of the complement system in diabetic nephropathy. Nat Rev Nephrol (2017) 13:311–8. doi: 10.1038/nrneph.2017.31
135. Ajjan RA, Schroeder V. Role of complement in diabetes. Mol Immunol (2019) 114:270–7. doi: 10.1016/j.molimm.2019.07.031
136. Shevyrev D, Tereshchenko V. Treg heterogeneity, function, and homeostasis. Front Immunol (2020) 10. doi: 10.3389/fimmu.2019.03100
137. Dominguez-Villar M, Baecher-Allan CM, Hafler DA. Identification of T helper type 1–like, Foxp3+ regulatory T cells in human autoimmune disease. Nat Med (2011) 17:673–5. doi: 10.1038/nm.2389
138. Hulme MA, Wasserfall CH, Atkinson MA, Brusko TM. Central role for interleukin-2 in type 1 diabetes. Diabetes (2012) 61:14–22. doi: 10.2337/db11-1213
139. Du J, Wang Q, Yang S, Chen S, Fu Y, Spath S, et al. FOXP3 exon 2 controls treg stability and autoimmunity. Sci Immunol (2022) 7:eabo5407. doi: 10.1126/sciimmunol.abo5407
140. Zhou X, Bailey-Bucktrout SL, Jeker LT, Penaranda C, Martínez-Llordella M, Ashby M, et al. Instability of the transcription factor Foxp3 leads to the generation of pathogenic memory T cells in vivo. Nat Immunol (2009) 10:1000–7. doi: 10.1038/ni.1774
141. Miyao T, Floess S, Setoguchi R, Luche H, Fehling HJ, Waldmann H, et al. Plasticity of Foxp3(+) T cells reflects promiscuous Foxp3 expression in conventional T cells but not reprogramming of regulatory T cells. Immunity (2012) 36:262–75. doi: 10.1016/j.immuni.2011.12.012
142. Singh K. Regulatory T cells in type 1 diabetes: the role of IL-35 in counteracting the disease. (2017).
143. Singh K, Kadesjö E, Lindroos J, Hjort M, Lundberg M, Espes D, et al. Interleukin-35 administration counteracts established murine type 1 diabetes – possible involvement of regulatory T cells. Sci Rep (2015) 5:12633. doi: 10.1038/srep12633
144. Espes D, Singh K, Sandler S, Carlsson P-O. Increased interleukin-35 levels in patients with type 1 diabetes with remaining c-peptide. Diabetes Care (2017) 40:1090–5. doi: 10.2337/dc16-2121
145. Saxena V, Lakhan R, Iyyathurai J, Bromberg JS. Mechanisms of ExTreg induction. Eur J Immunol (2021) 51:1956–67. doi: 10.1002/eji.202049123
146. Junius S, Mavrogiannis AV, Lemaitre P, Gerbaux M, Staels F, Malviya V, et al. Unstable regulatory T cells, enriched for naïve and Nrp1neg cells, are purged after fate challenge. Sci Immunol (2021) 6:eabe4723. doi: 10.1126/sciimmunol.abe4723
147. Xu L, Kitani A, Fuss I, Strober W. Cutting edge: regulatory T cells induce CD4+CD25–Foxp3– T cells or are self-induced to become Th17 cells in the absence of exogenous TGF-β. J Immunol (2007) 178:6725–9. doi: 10.4049/jimmunol.178.11.6725
148. Yang XO, Nurieva R, Martinez GJ, Kang HS, Chung Y, Pappu BP, et al. Molecular antagonism and plasticity of regulatory and inflammatory T cell programs. Immunity (2008) 29:44–56. doi: 10.1016/j.immuni.2008.05.007
149. Chekol Abebe E, Asmamaw Dejenie T, Mengie Ayele T, Dagnew Baye N, Agegnehu Teshome A, Tilahun Muche Z. The role of regulatory b cells in health and diseases: a systemic review. J Inflamm Res (2021) 14:75–84. doi: 10.2147/JIR.S286426
150. Mauri C. Novel frontiers in regulatory b cells. Immunol Rev (2021) 299:5–9. doi: 10.1111/imr.12964
151. Mauri C, Menon M. The expanding family of regulatory b cells. Int Immunol (2015) 27:479–86. doi: 10.1093/intimm/dxv038
152. Carter NA, Vasconcellos R, Rosser EC, Tulone C, Muñoz-Suano A, Kamanaka M, et al. Mice lacking endogenous IL-10–producing regulatory b cells develop exacerbated disease and present with an increased frequency of Th1/Th17 but a decrease in regulatory T cells. J Immunol (2011) 186:5569–79. doi: 10.4049/jimmunol.1100284
153. Ye C, Yano H, Workman CJ, Vignali DAA. Interleukin-35: structure, function and its impact on immune-related diseases. J Interferon Cytokine Res (2021) 41:391–406. doi: 10.1089/jir.2021.0147
154. Liu K, Huang A, Nie J, Tan J, Xing S, Qu Y, et al. IL-35 regulates the function of immune cells in tumor microenvironment. Front Immunol (2021) 12. doi: 10.3389/fimmu.2021.683332
155. Cai Z, Zhang S, Wu P, Ren Q, Wei P, Hong M, et al. Novel potential target of IL-35-regulated JAK/STAT signaling pathway in lupus nephritis. Clin Transl Med (2021) 11:e309. doi: 10.1002/ctm2.309
156. Vignali DAA, Kuchroo VK. IL-12 family cytokines: immunological playmakers. Nat Immunol (2012) 13:722–8. doi: 10.1038/ni.2366
157. Singh K, Luo Z, Carlsson P-O, Hansell P, Sandler S. IL-35+ regulatory immune cells in diabetic nephropathy. J Immunol (2019) 202:182.47–7. doi: 10.4049/jimmunol.202.Supp.182.47
158. Jiang Y, Wang J, Li H, Xia L. IL-35 alleviates inflammation progression in a rat model of diabetic neuropathic pain via inhibition of JNK signaling. J Inflamm (2019) 16:19. doi: 10.1186/s12950-019-0217-z
159. Yan A, You H, Zhang X. Levels of interleukin 27 and interleukin 35 in the serum and vitreous of patients with proliferative diabetic retinopathy. Ocul Immunol Inflamm (2018) 26:273–9. doi: 10.1080/09273948.2016.1203959
160. Yan A, Zhang Y, Wang X, Cui Y, Tan W. Interleukin 35 regulates interleukin 17 expression and T helper 17 in patients with proliferative diabetic retinopathy. Bioengineered (2022) 13:13293–9. doi: 10.1080/21655979.2022.2080367
161. Zhang J, Lin Y, Li C, Zhang X, Cheng L, Dai L, et al. IL-35 decelerates the inflammatory process by regulating inflammatory cytokine secretion and M1/M2 macrophage ratio in psoriasis. J Immunol Baltim Md 1950 (2016) 197:2131–44. doi: 10.4049/jimmunol.1600446
162. Luo Z, Soläng C, Larsson R, Singh K. Interleukin-35 prevents the elevation of the M1/M2 ratio of macrophages in experimental type 1 diabetes. Int J Mol Sci (2022) 23:7970. doi: 10.3390/ijms23147970
163. Van Zeebroeck L, Arroyo Hornero R, Côrte-Real BF, Hamad I, Meissner TB, Kleinewietfeld M. Fast and efficient genome editing of human FOXP3+ regulatory T cells. Front Immunol (2021) 12. doi: 10.3389/fimmu.2021.655122
164. Lam AJ, Lin DTS, Gillies JK, Uday P, Pesenacker AM, Kobor MS, et al. Optimized CRISPR-mediated gene knockin reveals FOXP3-independent maintenance of human treg identity. Cell Rep (2021) 36:109494. doi: 10.1016/j.celrep.2021.109494
165. Amini L, Greig J, Schmueck-Henneresse M, Volk H-D, Bézie S, Reinke P, et al. Super-treg: toward a new era of adoptive treg therapy enabled by genetic modifications. Front Immunol (2021) 11. doi: 10.3389/fimmu.2020.611638
166. Ferreira LMR, Muller YD, Bluestone JA, Tang Q. Next-generation regulatory T cell therapy. Nat Rev Drug Discovery (2019) 18:749–69. doi: 10.1038/s41573-019-0041-4
167. Lin G, Wang J, Lao X, Wang J, Li L, Li S, et al. Interleukin-6 inhibits regulatory T cells and improves the proliferation and cytotoxic activity of cytokine-induced killer cells. J Immunother Hagerstown Md 1997 (2012) 35:337–43. doi: 10.1097/CJI.0b013e318255ada3
168. Kimura A, Kishimoto T. IL-6: regulator of Treg/Th17 balance. Eur J Immunol (2010) 40:1830–5. doi: 10.1002/eji.201040391
169. Semenza GL, Rue EA, Iyer NV, Pang MG, Kearns WG. Assignment of the hypoxia-inducible factor 1α gene to a region of conserved synteny on mouse chromosome 12 and human chromosome 14q. Genomics (1996) 34:437–9. doi: 10.1006/geno.1996.0311
170. Cavadas MA, Nguyen LK, Cheong A. Hypoxia-inducible factor (HIF) network: insights from mathematical models. Cell Commun Signal (2013) 11:42. doi: 10.1186/1478-811X-11-42
171. Kierans SJ, Taylor CT. Regulation of glycolysis by the hypoxia-inducible factor (HIF): implications for cellular physiology. J Physiol (2021) 599:23–37. doi: 10.1113/JP280572
172. Lum JJ, Bui T, Gruber M, Gordan JD, DeBerardinis RJ, Covello KL, et al. The transcription factor HIF-1alpha plays a critical role in the growth factor-dependent regulation of both aerobic and anaerobic glycolysis. Genes Dev (2007) 21:1037–49. doi: 10.1101/gad.1529107
173. Robey IF, Lien AD, Welsh SJ, Baggett BK, Gillies RJ. Hypoxia-inducible factor-1alpha and the glycolytic phenotype in tumors. Neoplasia N Y N (2005) 7:324–30. doi: 10.1593/neo.04430
174. Del Rey MJ, Valín Á., Usategui A, García-Herrero CM, Sánchez-Aragó M, Cuezva JM, et al. Hif-1α knockdown reduces glycolytic metabolism and induces cell death of human synovial fibroblasts under normoxic conditions. Sci Rep (2017) 7:3644. doi: 10.1038/s41598-017-03921-4
175. Miska J, Lee-Chang C, Rashidi A, Muroski ME, Chang AL, Lopez-Rosas A, et al. HIF-1α is a metabolic switch between glycolytic-driven migration and oxidative phosphorylation-driven immunosuppression of tregs in glioblastoma. Cell Rep (2019) 27:226–237.e4. doi: 10.1016/j.celrep.2019.03.029
176. Dang EV, Barbi J, Yang H-Y, Jinasena D, Yu H, Zheng Y, et al. Control of TH17/Treg balance by hypoxia-inducible factor 1. Cell (2011) 146:772–84. doi: 10.1016/j.cell.2011.07.033
177. Schumann K, Raju SS, Lauber M, Kolb S, Shifrut E, Cortez JT, et al. Functional CRISPR dissection of gene networks controlling human regulatory T cell identity. Nat Immunol (2020) 21:1456–66. doi: 10.1038/s41590-020-0784-4
178. Fazekas B, Griffin MD. Mesenchymal stromal cell–based therapies for acute kidney injury: progress in the last decade. Kidney Int (2020) 97:1130–40. doi: 10.1016/j.kint.2019.12.019
179. Lin W, Li H-Y, Yang Q, Chen G, Lin S, Liao C, et al. Administration of mesenchymal stem cells in diabetic kidney disease: a systematic review and meta-analysis. Stem Cell Res Ther (2021) 12:43. doi: 10.1186/s13287-020-02108-5
180. Negi N, Griffin MD. Effects of mesenchymal stromal cells on regulatory T cells: current understanding and clinical relevance. Stem Cells (2020) 38:596–605. doi: 10.1002/stem.3151
181. Novel therapy for diabetic kidney disease | news | CORDIS | European commission. Available at: https://cordis.europa.eu/article/id/124495-novel-therapy-for-diabetic-kidney-disease (Accessed 18 October 2022).
182. Mota C, Nunes-Silva V, Pires AR, Matoso P, Victorino RMM, Sousa AE, et al. Delta-like 1–mediated notch signaling enhances the In vitro conversion of human memory CD4 T cells into FOXP3-expressing regulatory T cells. J Immunol (2014) 193:5854–62. doi: 10.4049/jimmunol.1400198
183. Babu GS. Immunomodulatory actions of mesenchymal stromal cells (MSCs) in osteoarthritis of the knee. Osteology (2021) 1:209–24. doi: 10.3390/osteology1040020
184. Zhang B, Liu R, Shi D, Liu X, Chen Y, Dou X, et al. Mesenchymal stem cells induce mature dendritic cells into a novel Jagged-2–dependent regulatory dendritic cell population. Blood (2009) 113:46–57. doi: 10.1182/blood-2008-04-154138
185. Lukomska B, Stanaszek L, Zuba-Surma E, Legosz P, Sarzynska S, Drela K. Challenges and controversies in human mesenchymal stem cell therapy. Stem Cells Int (2019) 2019:e9628536. doi: 10.1155/2019/9628536
186. Reynolds L. The success of stem cell transplantations and the potential post-transplantation complications may be dependent, among other factors, on the capacity of the recipient and the transplanted cells to repair DNA damage. BioDiscovery (2016) 19:e9076. doi: 10.3897/BioDiscovery.19.e9076
187. Crivelli B, Chlapanidas T, Perteghella S, Lucarelli E, Pascucci L, Brini AT, et al. Mesenchymal Stem/Stromal cell extracellular vesicles: from active principle to next generation drug delivery system. J Control Release (2017) 262:104–17. doi: 10.1016/j.jconrel.2017.07.023
188. Wang H, Vinnikov I, Shahzad K, Bock F, Ranjan S, Wolter J, et al. The lectin-like domain of thrombomodulin ameliorates diabetic glomerulopathy via complement inhibition. Thromb Haemost (2012) 108:1141–53. doi: 10.1160/TH12-07-0460
189. Li L, Yin Q, Tang X, Bai L, Zhang J, Gou S, et al. C3a receptor antagonist ameliorates inflammatory and fibrotic signals in type 2 diabetic nephropathy by suppressing the activation of TGF-β/Smad3 and IKBα pathway. PloS One (2014) 9:e113639. doi: 10.1371/journal.pone.0113639
190. Eliesen GAM, van Drongelen J, van den Broek PHH, Sarlea A, van der Heijden OWH, Langemeijer S, et al. Placental disposition of eculizumab, C5 and C5-eculizumab in two pregnancies of a woman with paroxysmal nocturnal haemoglobinuria. Br J Clin Pharmacol (2021) 87:2128–31. doi: 10.1111/bcp.14565
191. Morgan BP, Harris CL. Complement, a target for therapy in inflammatory and degenerative diseases. Nat Rev Drug Discov (2015) 14:857–77. doi: 10.1038/nrd4657
192. Sica A, Mantovani A. Macrophage plasticity and polarization: In vivo veritas. J Clin Invest (2012) 122:787–95. doi: 10.1172/JCI59643
193. Fang L, Hodge J, Saaoud F, Wang J, Iwanowycz S, Wang Y, et al. Transcriptional factor EB regulates macrophage polarization in the tumor microenvironment. Oncoimmunology (2017) 6:e1312042. doi: 10.1080/2162402X.2017.1312042
194. Wan YY, Flavell RA. Regulatory T cells, transforming growth factor–β, and immune suppression. Proc Am Thorac Soc (2007) 4:271–6. doi: 10.1513/pats.200701-020AW
195. Zhu S, Yao R, Li Y, Zhao P, Ren C, Du X, et al. The role and regulatory mechanism of transcription factor EB in health and diseases. Front Cell Dev Biol (2021) 9. doi: 10.3389/fcell.2021.667750
196. Song W, Zhang C-L, Gou L, He L, Gong Y-Y, Qu D, et al. Endothelial TFEB (Transcription factor EB) restrains IKK (IκB kinase)-P65 pathway to attenuate vascular inflammation in diabetic Db/Db mice. Arterioscler Thromb Vasc Biol (2019) 39:719–30. doi: 10.1161/ATVBAHA.119.312316
197. Gerriets V, Goyal A, Khaddour K. Tumor necrosis factor inhibitors. Treasure Island (FL: StatPearls; StatPearls Publishing (2022).
198. Tack CJ, Kleijwegt FS, Van Riel PLCM, Roep BO. Development of type 1 diabetes in a patient treated with anti-TNF-α therapy for active rheumatoid arthritis. Diabetologia (2009) 52:1442–4. doi: 10.1007/s00125-009-1381-0
199. Nicolela Susanna F, Pavesio CA. Review of ocular adverse events of biological anti-TNF drugs. J Ophthalmic Inflamm Infect (2020) 10:11. doi: 10.1186/s12348-020-00202-6
200. Kucharz EJ, Kotulska-Kucharz A. Tumor necrosis factor alpha inhibitors and demyelinating disease: what is behind it? Reumatologia (2021) 59:65–7. doi: 10.5114/reum.2021.105438
201. Bloom BJ. Development of diabetes mellitus during etanercept therapy in a child with systemic-onset juvenile rheumatoid arthritis. Arthritis Rheumatol (2000) 43:2606–8. doi: 10.1002/1529-0131(200011)43:11<2606::AID-ANR31>3.0.CO;2-X
202. Boulton JG, Bourne JT. Unstable diabetes in a patient receiving anti-TNF-α for rheumatoid arthritis. Rheumatology (2007) 46:178–9. doi: 10.1093/rheumatology/kel322
203. Kern PA, Ranganathan S, Li C, Wood L, Ranganathan G. Adipose tissue tumor necrosis factor and interleukin-6 expression in human obesity and insulin resistance. Am J Physiol-Endocrinol Metab (2001) 280:E745–51. doi: 10.1152/ajpendo.2001.280.5.E745
204. Shin I-SJ, Baer AN, Kwon HJ, Papadopoulos EJ, Siegel JN. Guillain-Barré And miller Fisher syndromes occurring with tumor necrosis factor alpha antagonist therapy. Arthritis Rheumatol (2006) 54:1429–34. doi: 10.1002/art.21814
205. Valls-Canals J, Povedano M, Montero J, Pradas J. Diabetic polyneuropathy. axonal or demyelinating? Electromyogr Clin Neurophysiol (2002) 42:3–6.
206. Abraham A, Alabdali M, Qrimli M, Breiner A, Barnett C, Katzberg H, et al. Chronic inflammatory demyelinating polyneuropathy in diabetes patients. US Neurol (2015) 11(1):47–52. doi: 10.17925/USN.2015.11.01.47
207. Kreiner FF, Kraaijenhof JM, von Herrath M, Hovingh GKK, von Scholten BJ. Interleukin 6 in diabetes, chronic kidney disease, and cardiovascular disease: mechanisms and therapeutic perspectives. Expert Rev Clin Immunol (2022) 18:377–89. doi: 10.1080/1744666X.2022.2045952
208. Sharma S. Interleukin-6 trans-signaling: a pathway with therapeutic potential for diabetic retinopathy. Front Physiol (2021) 12. doi: 10.3389/fphys.2021.689429
209. Genovese MC, Burmester GR, Hagino O, Thangavelu K, Iglesias-Rodriguez M, John GS, et al. Interleukin-6 receptor blockade or TNFα inhibition for reducing glycaemia in patients with RA and diabetes: Post hoc analyses of three randomised, controlled trials. Arthritis Res Ther (2020) 22:206. doi: 10.1186/s13075-020-02229-5
210. Jörns A, Ishikawa D, Teraoku H, Yoshimoto T, Wedekind D, Lenzen S. Remission of autoimmune diabetes by anti-TCR combination therapies with anti-IL-17A or/and anti-IL-6 in the IDDM rat model of type 1 diabetes. BMC Med (2020) 18:33. doi: 10.1186/s12916-020-1503-6
211. Lavoz C, Rayego-Mateos S, Orejudo M, Opazo-Ríos L, Marchant V, Marquez-Exposito L, et al. Could IL-17A be a novel therapeutic target in diabetic nephropathy? J Clin Med (2020) 9:272. doi: 10.3390/jcm9010272
212. Loft N. d., Vaengebjerg S, Halling A-S, Skov L, Egeberg A. Adverse events with IL-17 and IL-23 inhibitors for psoriasis and psoriatic arthritis: a systematic review and meta-analysis of phase III studies. J Eur Acad Dermatol Venereol (2020) 34:1151–60. doi: 10.1111/jdv.16073
213. Mease P, van der Heijde D, Landewé R, Mpofu S, Rahman P, Tahir H, et al. Secukinumab improves active psoriatic arthritis symptoms and inhibits radiographic progression: primary results from the randomised, double-blind, phase III FUTURE 5 study. Ann Rheumatol Dis (2018) 77:890–7. doi: 10.1136/annrheumdis-2017-212687
214. Sbidian E, Chaimani A, Garcia-Doval I, Do G, Hua C, Mazaud C, et al. Systemic pharmacological treatments for chronic plaque psoriasis: a network meta-analysis. Cochrane Database Syst Rev (2017) (12). doi: 10.1002/14651858.CD011535.pub2
215. Obinata H, Hla T. Sphingosine 1-phosphate and inflammation. Int Immunol (2019) 31:617–25. doi: 10.1093/intimm/dxz037
216. Hait NC, Maiti A. The role of sphingosine-1-Phosphate and ceramide-1-Phosphate in inflammation and cancer. Mediators Inflamm (2017) 2017:4806541. doi: 10.1155/2017/4806541
217. Gomez-Muñoz A, Gangoiti P, Arana L, Ouro A, Rivera I-G, Ordoñez M, et al. New insights on the role of ceramide 1-phosphate in inflammation. Biochim Biophys Acta BBA - Mol Cell Biol Lipids (2013) 1831:1060–6. doi: 10.1016/j.bbalip.2013.02.001
218. Sokolowska E, Blachnio-Zabielska A. The role of ceramides in insulin resistance. Front Endocrinol (2019) 10:577. doi: 10.3389/fendo.2019.00577
219. Gomez-Muñoz A, Presa N, Gomez-Larrauri A, Rivera I-G, Trueba M, Ordoñez M. Control of inflammatory responses by ceramide, sphingosine 1-phosphate and ceramide 1-phosphate. Prog Lipid Res (2016) 61:51–62. doi: 10.1016/j.plipres.2015.09.002
220. Mandal N, Grambergs R, Mondal K, Basu SK, Tahia F, Dagogo-Jack S. Role of ceramides in the pathogenesis of diabetes mellitus and its complications. J Diabetes Complications (2021) 35:107734. doi: 10.1016/j.jdiacomp.2020.107734
221. Dorweiler TF, Nolan P, Kolesnick RN, Busik JV. Anti-ceramide immunotherapy for diabetic retinopathy. Invest Ophthalmol Vis Sci (2022) 63:459.
222. Typiak M, Piwkowska A. Antiinflammatory actions of klotho: implications for therapy of diabetic nephropathy. Int J Mol Sci (2021) 22:956. doi: 10.3390/ijms22020956
223. Isakova T, Yanucil C, Faul CA. Klotho-derived peptide as a possible novel drug to prevent kidney fibrosis. Am J Kidney Dis (2022) 80:285–8. doi: 10.1053/j.ajkd.2022.03.006
224. Buendía P, Ramírez R, Aljama P, Carracedo J. Chapter five - klotho prevents translocation of NFκB. In: Litwack G, editor. Vitamins & hormones, vol. 101 . Klotho: Academic Press (2016). p. 119–50.
225. Zhao Y, Banerjee S, Dey N, LeJeune WS, Sarkar PS, Brobey R, et al. Klotho depletion contributes to increased inflammation in kidney of the Db/Db mouse model of diabetes via RelA (Serine)536 phosphorylation. Diabetes (2011) 60:1907–16. doi: 10.2337/db10-1262
226. Zubkiewicz-Kucharska A, Wikiera B, Noczyńska A. Soluble klotho is decreased in children with type 1 diabetes and correlated with metabolic control. Front Endocrinol (2021) 12:709564. doi: 10.3389/fendo.2021.709564
227. Yuan Q, Ren Q, Li L, Tan H, Lu M, Tian Y, et al. A klotho-derived peptide protects against kidney fibrosis by targeting TGF-β signaling. Nat Commun (2022) 13:438. doi: 10.1038/s41467-022-28096-z
228. Takei H, Araki A, Watanabe H, Ichinose A, Sendo F. Rapid killing of human neutrophils by the potent activator phorbol 12-myristate 13-acetate (PMA) accompanied by changes different from typical apoptosis or necrosis. J Leukoc Biol (1996) 59:229–40. doi: 10.1002/jlb.59.2.229
229. Brinkmann V, Reichard U, Goosmann C, Fauler B, Uhlemann Y, Weiss DS, et al. Neutrophil extracellular traps kill bacteria. Science (2004) 303:1532–5. doi: 10.1126/science.1092385
230. de Vries JJ, Hoppenbrouwers T, Martinez-Torres C, Majied R, Özcan B, van Hoek M, et al. Effects of diabetes mellitus on fibrin clot structure and mechanics in a model of acute neutrophil extracellular traps (NETs) formation. Int J Mol Sci (2020) 21:7107. doi: 10.3390/ijms21197107
231. Cedervall J, Herre M, Dragomir A, Melo F, Svensson A, Thålin C, et al. Neutrophil extracellular traps promote cancer-associated inflammation and myocardial stress. OncoImmunology (2022) 11. doi: 10.1080/2162402X.2022.2049487
232. Sørensen OE, Borregaard N. Neutrophil extracellular traps [[/amp]]mdash; the dark side of neutrophils. J Clin Invest (2016) 126:1612–20. doi: 10.1172/JCI84538
233. Shafqat A, Abdul Rab S, Ammar O, Al Salameh S, Alkhudairi A, Kashir J, et al. Emerging role of neutrophil extracellular traps in the complications of diabetes mellitus. Front Med (2022) 9. doi: 10.3389/fmed.2022.995993
234. Rosazza T, Warner J, Sollberger G. NET formation – mechanisms and how they relate to other cell death pathways. FEBS J (2021) 288:3334–50. doi: 10.1111/febs.15589
235. Jiménez-Alcázar M, Rangaswamy C, Panda R, Bitterling J, Simsek YJ, Long AT, et al. Host DNases prevent vascular occlusion by neutrophil extracellular traps. Science (2017) 358:1202–6. doi: 10.1126/science.aam8897
236. Law SM, Gray RD. Neutrophil extracellular traps and the dysfunctional innate immune response of cystic fibrosis lung disease: a review. J Inflamm Lond Engl (2017) 14:29. doi: 10.1186/s12950-017-0176-1
237. Law JL, McFerran TAMA. DNAse. In: Law J, editor. A dictionary of nursing. Oxford University Press (2021).
238. Laube BL, Auci RM, Shields DE, Christiansen DH, Lucas MK, Fuchs HJ, et al. Effect of RhDNase on airflow obstruction and mucociliary clearance in cystic fibrosis. Am J Respir Crit Care Med (1996) 153:752–60. doi: 10.1164/ajrccm.153.2.8564129
239. Davis JC, Manzi S, Yarboro C, Rairie J, Mcinnes I, Averthelyi D, et al. Recombinant human dnase I (RhDNase) in patients with lupus nephritis. Lupus (1999) 8:68–76. doi: 10.1191/096120399678847380
240. Njeim R, Azar WS, Fares AH, Azar ST, Kassouf HK, Eid AA. NETosis contributes to the pathogenesis of diabetes and its complications. J Mol Endocrinol (2020) 65:R65–76. doi: 10.1530/JME-20-0128
Keywords: diabetes mellitus, type 1 diabetes (T1D), type 2 diabetes (T2D), diabetes complications, immunotherapy, inflammation, regulatory B (Breg) cells, regulatory T (Treg) cells
Citation: Reynolds L, Luo Z and Singh K (2023) Diabetic complications and prospective immunotherapy. Front. Immunol. 14:1219598. doi: 10.3389/fimmu.2023.1219598
Received: 09 May 2023; Accepted: 22 June 2023;
Published: 07 July 2023.
Edited by:
Silvia Gregori, San Raffaele Telethon Institute for Gene Therapy (SR-Tiget), ItalyReviewed by:
Maciej Zieliński, Medical University of Gdansk, PolandNikolina Papac-Milicevic, Medical University of Vienna, Austria
Copyright © 2023 Reynolds, Luo and Singh. This is an open-access article distributed under the terms of the Creative Commons Attribution License (CC BY). The use, distribution or reproduction in other forums is permitted, provided the original author(s) and the copyright owner(s) are credited and that the original publication in this journal is cited, in accordance with accepted academic practice. No use, distribution or reproduction is permitted which does not comply with these terms.
*Correspondence: Lewis Reynolds, bGV3aXMtcmV5bm9sZHNAaG90bWFpbC5jby51aw==; Kailash Singh, a2FpbGFzaC5zaW5naEBtY2IudXUuc2U=