- 1Department of Nephrology, The First Affiliated Hospital of Zhejiang Chinese Medical University (Zhejiang Provincial Hospital of Chinese Medicine), Hangzhou, China
- 2Department of Traditional Chinese Medicine, Jiande First People’s Hospital, Jiande, Hangzhou, China
- 3Department of Nephrology, The First People’s Hospital of Hangzhou Lin’an District, Hangzhou, China
- 4The First Clinical Medical College, Zhejiang Chinese Medical University, Hangzhou, China
- 5Department of Geriatric, Zhejiang Aged Care Hospital, Hangzhou, China
- 6Department of Geriatric, Tongde Hospital of Zhejiang Province, Hangzhou, China
In recent years, the role of intestinal homeostasis in health has received increasing interest, significantly improving our understanding of the complex pathophysiological interactions of the gut with other organs. Microbiota dysbiosis, impaired intestinal barrier, and aberrant intestinal immunity appear to contribute to the pathogenesis of immune-related chronic kidney diseases (CKD). Meanwhile, the relationship between the pathological changes in the respiratory tract (e.g., infection, fibrosis, granuloma) and immune-related CKD cannot be ignored. The present review aimed to elucidate the new underlying mechanism of immune-related CKD. The lungs may affect kidney function through intestinal mediation. Communication is believed to exist between the gut and lung microbiota across long physiological distances. Following the inhalation of various pathogenic factors (e.g., particulate matter 2.5 mum or less in diameter, pathogen) in the air through the mouth and nose, considering the anatomical connection between the nasopharynx and lungs, gut microbiome regulates oxidative stress and inflammatory states in the lungs and kidneys. Meanwhile, the intestine participates in the differentiation of T cells and promotes the migration of various immune cells to specific organs. This better explain the occurrence and progression of CKD caused by upper respiratory tract precursor infection and suggests the relationship between the lungs and kidney complications in some autoimmune diseases (e.g., anti-neutrophil cytoplasm antibodies -associated vasculitis, systemic lupus erythematosus). CKD can also affect the progression of lung diseases (e.g., acute respiratory distress syndrome and chronic obstructive pulmonary disease). We conclude that damage to the gut barrier appears to contribute to the development of immune-related CKD through gut-lung-kidney interplay, leading us to establish the gut-lung-kidney axis hypothesis. Further, we discuss possible therapeutic interventions and targets. For example, using prebiotics, probiotics, and laxatives (e.g., Rhubarb officinale) to regulate the gut ecology to alleviate oxidative stress, as well as improve the local immune system of the intestine and immune communication with the lungs and kidneys.
1 Introduction
Genetic and environmental factors play a role in the pathogenesis of immune-related chronic kidney diseases (CKD), such as immunoglobulin A (IgA) nephropathy (IgAN) (1, 2), anti-neutrophil cytoplasmic antibody (ANCA)-associated vasculitis (AAV) (3, 4), and systemic lupus erythematosus (SLE) (5, 6). Gastrointestinal symptoms are rare in the early stages of these diseases, although mild diarrhea occurs in patients with IgAN (7). The discovery of the gut-kidney axis establishes the relationship between disruption of gut homeostasis and CKD onset and progression.
The gastrointestinal tract, hosting the gut microbiome, is protected by a large number of immune cells and structures for intestinal homeostasis. These structures remain in direct contact with various microbial communities in the environment (8, 9). With increased permeability of the gut epithelium, pathogens and foreign antigens enter systemic circulation, leading to CKD progression. This process is regulated by the gut microbiota and immune cells (10).
Furthermore, respiratory tract infection is the most common exogenous factor in acute exacerbations of CKD (11, 12), resulting from immune and inflammatory responses induced by pathogen-associated molecular patterns (PAMPs). The proposal of the “gut-lung” axis suggested multidirectional interactions of the lung, kidney, and gut (13). Thus, further research was required to confirm the existence of interactions between the gut, lungs, and kidneys, contributing to the pathogenesis of immune-associated CKD.
This review aims to provide an up-to-date qualitative synthesis of recently published literature related to these organs and discusses the underlying pathophysiological mechanisms of the gut-lung-kidney axis in several diseases. Here, we focus on the connection between gut microbiota and innate and adaptive immunity, and propose possible treatment methods based on the improved understanding of the gut-lung-kidney axis. We made a graphical abstract of the gut-lung-kidney axis for clarity (Figure 1).
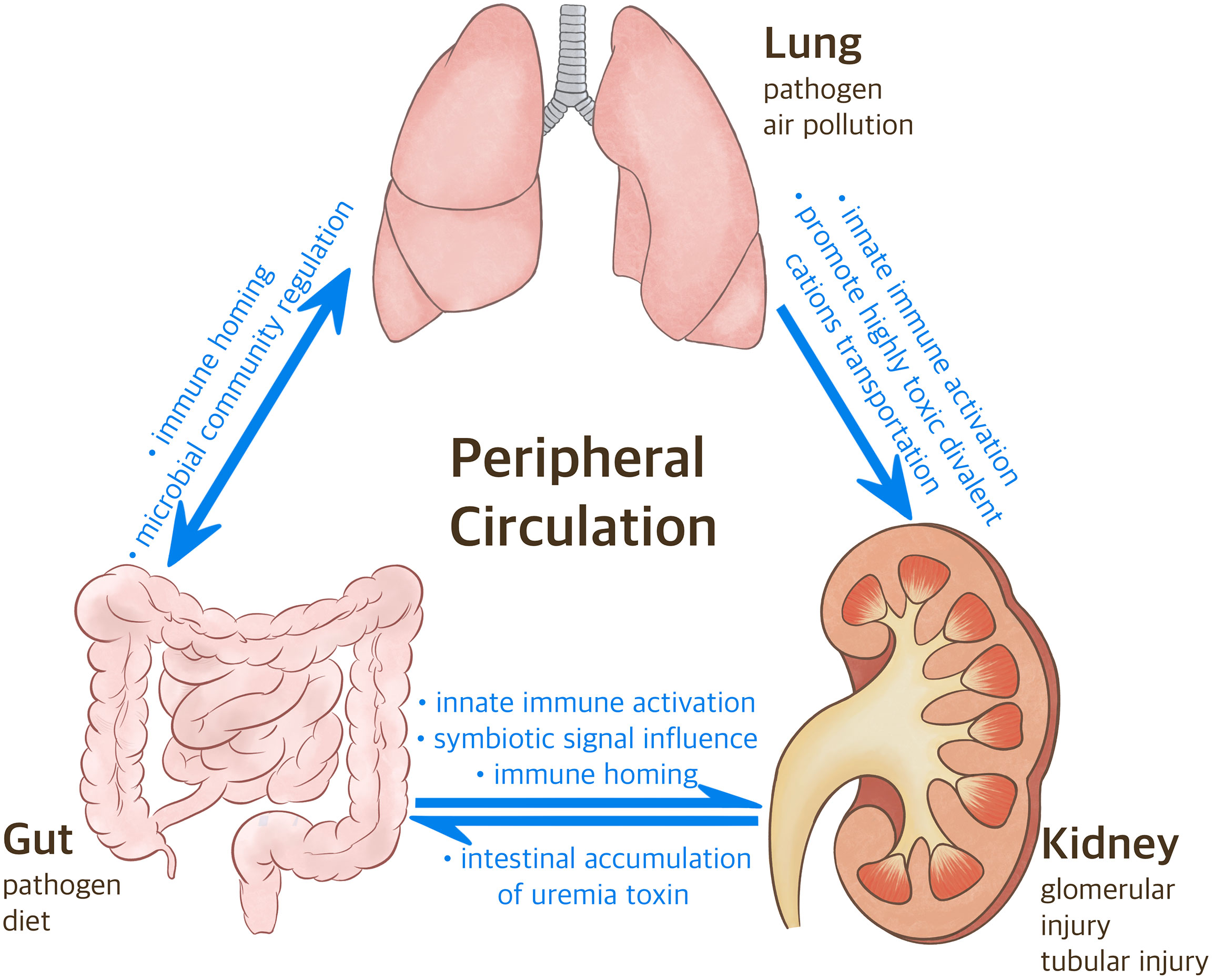
Figure 1 The Correlation between Gut-Lung-Kidney. Following the inhalation of various pathogenic factors (e.g., particulate matter 2.5 mum or less in diameter, pathogen) in the air through the mouth and nose, considering the anatomical connection between the nasopharynx and lungs, gut microbiome regulates oxidative stress and inflammatory states in the lungs and kidneys. Meanwhile, the intestine participates in the differentiation of T cells and promotes the migration of various immune cells to specific organs. CKD can also affect the progression of lung diseases via heavy metal.
2 Intestinal homeostasis and human health
2.1 Intestinal microbiota
The human gut microbiota, coexisting in complex interdependence, is an important ecosystem comprising bacteria, fungi, archaea, viruses, and protozoa (14, 15). The intestinal flora is dominated by Firmicutes and Bacteroides, with relatively few Proteobacteria, Actinobacteria, Fusobacteria, and Verrucomicrobia phyla (16). Bacteroidetes, Firmicutes, and Bifidobacteria help ferment soluble dietary fiber that cannot be degraded by human digestive enzymes into short-chain fatty acids (SCFAs) (17). SCFAs are a group of fatty acids with fewer than six carbons, with acetic acid (C2), propionic acid (C3), and butyric acid (C4) accounting for more than 95% of all SCFA in the gut (18). Functions performed by SCFAs include providing approximately 10% of the daily energy required by the human body, maintaining glucose homeostasis, and reducing cholesterol levels (19–23).
SCFAs participate widely in innate immune response and can activate orphan G-protein-coupled receptors (GPRs), GPR41, and GPR43 (24). GPR43 enhances production of interleukin-18 (IL-18) to promote colonic epithelial repair through the regulation of NACHT, LRR, and PYD domain-containing protein 3 (NLRP3) inflammasome (25). In addition, SCFAs facilitate neutrophil cell migration to enhance phagocytosis (26). Another study suggested that SCFAs inhibit intestinal macrophage production of pro-inflammatory mediators (e.g., nitric oxide (NO), IL-6, and IL-12) via histone deacetylase (HDAC) inhibition (27).
In addition, SCFAs are involved in adaptive immune response and can transform immature T cells into Th1 or Th17 cells based on different cytokine environments, and increase the production of IL-17, a key cytokine in inflammation (18, 28).
In summary, the gut microbiota maintains intestinal integrity through SCFAs, mainly by modulating immune responses.
2.2 Intestinal immunity
2.2.1 The small intestine
The small intestinal epithelium has a single layer mainly composed of columnar epithelial cells (29), expressing numerous pattern recognition receptors, including toll-like receptor 5 (TLR5) (30), TLR1, TLR2, TLR3, and TLR9 (31). Further, these cells promote the expression of chemotactic factors for myeloid cells, lymphoid cells, and neutrophil chemokines, following specific stimulation to initiate and coordinate inflammatory responses (32, 33). The intestinal epithelium contains intraepithelial lymphocytes (IELs) (34), which are almost all T cells. On stimulation, these cells express interferon-γ (IFN-γ) and keratinocyte growth factor to play protective and pathogenic roles during inflammation (35–37).
The lamina propria underlies the intestinal epithelium; within this layer, αβ T-cell receptor-positive T cells are the most commonly found lymphocytes (38). Subsets within this population have drastically different functions; CD4+ and CD25+ regulatory T cells in the lamina propria can inhibit T-cell proliferation, cytokine production, and development of colitis (39). In contrast, lamina propria CD4+ T cells secrete both IL-17 and IL-22, and are associated with intestinal inflammation (40, 41). Furthermore, lamina propria dendritic cells (LPDCs) play a significant role in determining whether a particular antigen receives an inflammatory or anti-inflammatory response. CD103− LPDCs promote inflammation and increase the expression of inflammatory mediators, such as tumor necrosis factor-α and IL-6, following stimulation with TLR ligands (42). Meanwhile, CD103 LPDCs prevent excessive inflammation (43).
Innate lymphoid cells (ILCs) can be classified into three broad groups (44). Group 3 ILCs are important intestinal sources of IL-17 and IL-22 and bidirectionally regulateintestinal damage (9).
2.2.2 The large intestine
B cells are the predominant lymphocytes in the lamina propria of the large intestine (38). Lamina propria B cells secrete dimeric IgA, which is transcytosed by epithelial cells into the gut lumen through the action of the polymeric immunoglobulin receptor (45, 46). Antigen-specific IgA can be generated during an intestinal infection (47). Intestinal IgA directly controls the composition of the intestinal microbiome (48). Goblet cells, another class of specialized epithelial cells, represent approximately 15% of cells found in the large intestinal epithelium (49, 50) and can produce the antimicrobial peptides Ang4, RegIIIγ, and RegIIIβ (51, 52). In addition, goblet cells may transfer antigens acquired from the intestinal lumen to dendritic cells in the lamina propria (53).
In summary, the intestinal local immune function and gut microbiota collaborate to maintain intestinal homeostasis.
2.2.3 Leaky gut
Researchers have found that the disruption of intestinal barrier due to various pathological factors, leads to increased permeability of the gastrointestinal epithelium. Initially, prohibited substances (e.g. microorganisms, microbial products, and food antigens) intrude into the systemic circulation and lead to intestinal inflammation; this process is also known as “gut leaky” (54).
Gut leaky may cause a series of immune-related diseases, such as systemic lupus erythematosus (SLE) (55) and ANCA-associated vasculitis (AAV) (56).
In addition, the long-term existence of the pathological condition can also affect the remodeling of other organ tissues, which leads to fibrosis and damage to organ function (e.g. liver and kidneys) (57, 58).
3 The gut-kidney axis
3.1 The microbial pathway
The main microbiota involved in the gut-kidney axis produce SCFAs and urinary toxin solutes.
In healthy individuals, amino acid metabolites are produced following diet protein metabolism and are then converted into urinary toxin solutes. Several reviews have summarized that gut microbiota is the potential source of uremic toxins, including p-Cresyl sulfate (PCS), indoxyl sulfonate (IS), indole-3-acetic acid, trimethylamine N-oxide (TMAO), and phenylacetylglutamine (PAGIn) (59, 60). The butyrate-producing bacteria, Roseburia, Faecalibacterium, Clostridium, Coprococcus, and Prevotella, are reduced in end-stage renal disease (ESRD) (61). Patients with ESRD have significantly altered gut microbiota compared with individuals with normal kidney function, with a larger number of bacterial families producing urease, uratase, indole, and p-cresol-forming enzymes (62). Li et al. showed that butyrate-derived fecal bacteria in the intestines of patients with CKD were significantly decreased and were not geographically related. Butyrate reduces renal inflammation and serum levels of uremic toxins, which are partially mediated by GPR-43 signaling (62, 63).
Changes in the gut microbiota lead to poor prognoses in patients with CKD. Lack of dietary fiber or excessive protein intake can produce excessive cresol sulfate and indole sulfate (64). Decreased renal function causes the accumulation of large amounts of uremic solutes in the plasma (65, 66). Indole sulfate can cause systemic vascular inflammation, endothelial dysfunction, and vascular calcification (67). In addition, PCS induces insulin resistance in mice with normal renal function via activation of the ERK1/2 pathway (68). Indole sulfate and PCS increase expression of the angiotensin 1 (AT1) receptor and reduce expression of the AT2 receptor in semi-nephrectomized mice, thereby activating the renal renin-angiotensin-aldosterone system and significantly increasing transforming growth factor β (TGF-β) expression. This induces the transformation of renal tubular epithelial-mesenchymal tissue, which is a key factor in renal fibrosis (69), and ultimately exacerbates kidney damage (70). Plasma proteins have high binding rates to PCS and indole sulfate, making them difficult to identify via dialysis. They remain in circulation for a long time and are an important factor in the poor prognosis of patients with ESRD (71, 72). Unlike PCS and IS, TMAO and PAGIn are easily eliminated through hemodialysis; regardless, they are important uremic toxins. TMAO is produced via the products of intestinal bacterial metabolism and subsequent oxidation after dietary intake, and participates in the onset of atherosclerosis and cardiovascular disease (CVD) (73). PAGIn is the product of phenylalanine through gut microbiota metabolism, and it may increase the risk of thrombosis and CVD by stimulating platelets (74).
As renal function decreases, the colon replaces the kidneys as the main site for the excretion of urea and uric acid (75). In patients with CKD, the concentration of nitrogenous urea in the blood increases; subsequently, nitrogen moves freely from the blood to the digestive tract lumen, where it is converted into ammonia and carbon dioxide by urease. A significant positive correlation was found between blood urea concentration and total and free ammonia, thereby increasing the pH of intestinal fluid and disrupting intestinal homeostasis (76). Two studies by Waziri et al. suggested that continuous exposure of colonic epithelial cells to urea can disrupt epithelial barrier function via depletion of intestinal tight junction proteins, such as claudin 1, occludin, and zonal occludens 1 (77, 78). An increase in intestinal permeability leads to increased levels of endotoxins and bacterial products in the systemic and lymphatic circulations of patients with CKD, leading to chronic and systemic inflammation (79). This finding was confirmed in a study showing bacterial DNA in the extraintestinal region in the blood and mesenteric lymph nodes of uremic model rats, with increased levels of hsCRP and IL-6 in the plasma (80). Leaky gut causes translocation of lipopolysaccharide (LPS) and (1→3)-β-D-glucan (BG) into the circulation, activating pro-inflammatory and fibrotic pathways, leading to organ fibrosis, and exacerbating the progression of CKD (58).
In summary, the imbalance of intestinal microorganisms leads to the intestinal environment destruction and the urinary toxin solutes residual, ultimately cause the inflammation response, vascular damage and renal function damage.
3.2 The immune pathway
Another pathway connecting the intestines and kidneys is mediated by the immune system.
Soluble urokinase-type plasminogen activator receptor (suPAR) is an inflammatory signal with multiple biological effects expressed in various cells, including immunocompetent and endothelial cells and podocytes (81, 82). Earlier study suggested its concentration depends on the “activation” level of the immune system (81). Genua’s experiment in a mouse model of colitis found that suPAR increased irritability, prevented macrophage polarization to M1, and inhibited production of inflammatory factors in the intestinal inflammatory state (83).
In contrast, Ham et al. proposed that immature myeloid cells in the body’s bone marrow can cause a pathological increase in suPAR, and that transplantation of immature myeloid cells into healthy mice can induce proteinuria. These findings were indicative of a relationship between suPAR and CKD (84). Wei et al. believed that elevated levels of suPAR can activate the cell movement-promoting αvβ3 integrin, leading to the disappearance of foot processes and proteinuria (82).
Earlier studies suggested a specific association of high serum suPAR levels with primary focal segmental glomerulosclerosis (FSGS) (85). However, subsequent studies have confirmed that serum suPAR levels are associated with proteinuria levels and estimated glomerular filtration rate (eGFR) in primary non-FSGS glomerulonephritis, autoimmune diseases, and secondary glomerulonephritis (86), and with eGFR in patients with autosomal dominant polycystic kidney disease (87), especially those with FSGS and lupus nephritis (LN) (88). A follow-up study involving 3,683 patients suggested that higher plasma suPAR levels were independent risk factors for CKD (89). Furthermore, SuPAR is associated with glomerulonephritis and may serve as a common biomarker of glomerulonephritis (89).
In summary, the inflammatory state of the intestine may lead to renal inflammation by upregulating suPAR expression.
4 The gut-lung axis
The physiological distance between the intestine and lungs is relatively large, and researchers have focused on achieving long-distance communication.
4.1 The microbial pathway
Although the biomass of the lung microbiota is significantly lower than that of the intestinal microbiota in healthy individuals (90), the lungs and intestines have similar bacterial phyla, mainly Firmicutes and Bacteroides, followed by Proteus and Actinomyces (91). The main fungal phyla identified in the lungs are Ascomycetes and Microsporidia (92, 93).
Results of some preclinical trials suggest that changes in the pathophysiological state of the intestines or lungs can lead to changes in mutual microbiota, which closely related to inflammation. Rat fecal transplantation negatively regulates the abundance of the original intestinal microbiota and positively regulates that of the lung microbiota, thereby restoring them to a normal state (94). Conversely, acute pneumonia induced by LPS in mice affects microbiota in the blood and cecum (95).
Comparable results have been observed in both human and animal experiments. A high abundance of enterogenic Bacteroides, strongly associated with tumour necrosis factor alpha (TNF-α), was found in the alveolar lavage fluid of patients with acute respiratory distress syndrome (96). According to the above studies, gut microbiota had a transient response to lung damage, which may be related to the overload caused by the temporary translocation of bacteria to the intestine through circulation. However, this does not explain the improvement in pulmonary flora following fecal bacterial transplantation.
Another study on plasma metabolomics of patients with acute respiratory distress syndrome (ARDS) suggested that the increased mortality rate in ARDS was associated with high levels of plasma phenylalanine and its gut microbiota metabolite PAGIn. High levels of PAGIn are associated with thrombosis, which is one of the lethal pathological mechanisms of ARDS (74), indicating that the progress of ARDS involves gut ecology.
4.2 The immune pathway
Further research revealed the involvement of the local immune system in the lungs and intestines.
Local immunity in the lungs and intestines plays a vital role in organ protection. According to Samuelson’s hypothesis, the gut microbiota can be transported to gut-associated lymphoid tissue via dendritic cells (DC) and cross the intestinal barrier (97). Bacterial products can activate innate immune signaling events and trigger inflammatory reactions via the TLR pathways (97).
In addition, many T cells reside in the intestinal epithelium and lamina propria. On activation of the Peyer and mesentery lymph nodes potentiated by chemokine receptor 9 (CCR9) and integrin α (4) β (7), T cells are recruited into the intestinal mucosa (98).
Mikhak et al. suggested that DCs in the lungs activate antigen-specific CD4+ T cells and guide lung-specific homing via CCR4 to enhance immunity (99). Targeted immune responses help adjust the balance of the immune system.
Ruane et al. found cross-organ communication between the lungs and intestine. To guide the migration of T cells to the gastrointestinal (GI) tract, lung DCs activate TGF-β and retinoic acid receptor signaling pathways to upregulate the intestinal homing integrin α4β7 (100).
Furthermore, the gut microbiota is involved in phenotypic changes and migration of T cells. Intestinal segmented filamentous bacteria are symbiotic bacteria that colonize the ileum of most animals, including humans, and regulate polarization of CD4+ T cells into Th17 cells. If the lungs are in a highly inflammatory state, the level of CCL20 (a ligand of CCR6) increases, prioritizing the recruitment of Th17 cells into the lungs (101). Th17 cells aid in protection against pulmonary fungal and Staphylococcus aureus infections (102, 103).
In summary, the immune homing effects of the lungs and intestine partly via microbial community signal regulation contribute to mutual immune barriers.
5 Potential connections between the lungs and kidneys
The connection between the gut-kidney and gut-lung has been revealed gradually. Meanwhile, several studies suggest the potential physiological and pathological relationships between the lungs and kidneys.
As commonly known, CKD progression is closely associated with acute respiratory infection (11). Chronic opportunistic pathogens, such as Epstein–Barr virus (EBV), will lurk and and enter into the oropharynx, and indicate increased levels of renal involvement in patients with granulomatosis with polyangiitis (GPA) (104).
Large studies in multiple parts of the world also suggested that air pollutants (e.g., particulate matter 2.5 mum or less in diameter etc.) are significantly correlated with the decrease of eGFR and the incidence of CKD (105, 106).
Moreover, pulmonary disease, such as chronic obstructive pulmonary disease (COPD), can cause severe hypoxia and induce the expression of divalent metal transporter 1 (DMT-1) genes. DMT-1 is present in most organs, especially in the duodenum and kidneys. It transports divalent cationic cadmium 2+ (Cd 2+), which has significant osteotoxicity and nephrotoxicity. The harmful effects of Cd 2+ may worsen the microstructure of the bone and reduce renal function (107). A bidirectional Mendelian randomization study involving 321,047 Europeans suggested that renal dysfunction is a pathogenic factor for COPD (108).
Furthermore the intestinal metabolite PAGIn, also known as the urinary toxin solute, can increase the risk of thrombosis by stimulating platelets and simultaneously affect the progression of ARDS and CKD (59, 74).
The aforementioned studies strongly suggest a connection between the lungs and kidneys; however, the crosstalk mechanism of these two organs remains unclear.
6 The gut-lung-kidney axis and related diseases
Based on the studies cited above, we propose the hypothesis of the gut-lung-kidney axis, which suggests that the gut participates in the pathological mechanism between the lungs and kidneys, and that these organs interact with each other through microbiota and immune regulation. The possible underlying associations can be explained by observations from the following can be explained via the resulting diseases.
Studies on the topics regarding immune-related CKD are detailed in Table 1.
6.1 IgA nephropathy
The pathogenesis of IgAN is currently unclear. Its onset is usually accompanied by prodromic infections of the upper respiratory tract (URT) or intestines. Most people understand the importance of IgA1 with galactose deficiency (Gd-IgA1) in circulation (109, 110).
Under physiological conditions, serum IgA1 is a common human immunoglobulin with a half-life of approximately 5 days, widely present in organs (e.g., lungs and intestine) (111).
Recently, Suzuki et al. proposed a multiple-hit hypothesis, including the production of Gd-IgA1 (Hit 1), IgG, or IgA autoantibodies that recognize Gd-IgA1 (Hit 2), their subsequent immune complex formation (Hit 3), and glomerular deposition (Hit 4) (112).
6.1.1 Immune connection between prodromic infections of the URT and lungs
It is generally believed that this hypothesis is related to mucosal infections. IgAN rarely presents with obvious lower respiratory tract infection, but an emerging series of studies on mucosal vaccines has clarified the immune connection between upper respiratory tract (URT) infections and the lungs. Twenty days after primary influenza viral infection or intranasal vaccination, IgA PCs derived from resident memory B cells were observed in the submucosal areas of murine models below the bronchial epithelium (113, 114). Four days after the secondary infection, IgA PCs were detected next to the alveoli instead of the more proximal airways (114). This suggested an immune connection between URT infection and the lungs. Furthermore, IgA-producing B cells in murine models express C-X-C motif chemokine receptor 3 (CXCR3). This receptor is important for local IgA production, as CXCR3 mice have fewer IgA cells in the lungs (113–115). This suggests the possibility of URT infection participating in the course of IgAN through the lungs.
6.1.2 The connection between intestinal prodromic infections and lungs
Owing to the presence of the gut-kidney axis, increased intestinal permeability in patients with IgAN may promote absorption and circulation of LPS (116). The invasion of mucosal and systemic antigens, especially proteins, glycoproteins, and viral antigens (e.g., influenza and HIV), leads to a corresponding increase in serum IgA levels with abnormal O-glycosylation (117, 118). Moreover, some studies have proposed the possibility of bone marrow-derived abnormal IgA levels (119). After the intestinal mucosa is attacked, the lungs respond accordingly. After intranasal immunization with inactive cholera toxin (CT), lung dendritic cells stimulated retinoic acid-dependent upregulation of α4β7 and CCR9 gut-homing receptors on local IgA-expressing B cells. Migration of these cells to the gut facilitated IgA-mediated protection against an oral challenge with active CT (120). This process significantly regulated by the microbiota, suggesting that lungs may participate in local immune communication through immune homing as early as the stage of intestinal infection.
6.1.3 Intestinal involvement in the production of abnormal IgA1 complexes
Regarding the mechanism of abnormal IgA1 production, Coppo et al. reported that adults with IgAN have higher levels of TLR4 messenger RNA (mRNA) in peripheral hemolymph monocytes (121). Qin et al. found that LPS stimulated the activation of TLR4 in cultured peripheral B cells, inhibited cosmic mRNA expression, and resulted in galactose deficiency in IgA1 (122). When Gd-IgA1 combines with specific IgG or IgA1 antibodies to form a circulating immune complex (CIC), it remains undegraded by liver cells (123); this may be related to its excessive molecular weight and volume, which affect the normal clearance process (110). In addition, inflammation and immune activity are involved in accelerating IgA1 differentiation.
The formation of CIC also involves the intestine. A gluten diet exacerbates intestinal IgA1 secretion, inflammation, and villous atrophy, and may induce IgA1-sCD89 complex formation and mucosal immune response, ultimately potentiating IgAN development. CD89, an IgA receptor, has not been identified in human renal mesangial cells (124).
6.1.4 Intestine affect the kidneys via humoral immunity
However, the mechanism underlying IgA deposition from the circulation to the kidneys remains unexplored. Some studies have suggested the presence of the CD71 receptor in human renal mesangial cells (125), which may be one of the mechanisms.
The clue to another pathway may be obtained by focusing on the B cell activating factor (BAFF). BAFF is a peripheral B-cell survival factor. Immune response against viral antigens has a positive cycle: BAFF recruits neutrophils and active B cells and generates more BAFFs through the TLR9-interferon regulatory factor 5 (IRF5) signaling pathway (126).
McCarthy et al. showed that IgA levels increased in the intestinal lamina propria, circulation, and mesangium of BAFF-overexpressing transgenic mice (127). Subsequently, a follow-up study was conducted, confirming that, in addition to BAFF levels, IgA deposition in the kidney was strongly affected by persistent symbiotic-dependent signals in the intestine (128). Some studies have shown increased serum BAFF levels in patients with IgAN, which correlated positively with disease severity, mesangial IgA deposition density, and serum BAFF and IgA1 levels (129, 130). This means that BAFF may be an important factor in gut-induced IgAN development with the involvement of the gut microbiota.
Monteiro et al. reviewed the impact of ecological changes in the gut on individuals with IgAN and found that both the abundance of gut bacteria, as well as metabolites in the blood, urine, and feces of individuals with IgAN was altered. The level of representative SCFA with anti-inflammatory effects was reduced in the feces of patients with IgAN. Disease markers of IgAN, such as proteinuria and hematuria or Gd-IgA1, were positively or negatively correlated with different bacterial abundances (131).
After circulation to the kidney, CIC can induce the proliferation of human mesangial cells, cause high expression of TLR4 in renal mesangial cells, activate the TLR4-MyD88-NF- κ B signaling pathway, release humoral factors such as TNF α, IL-6, TGF β, MCP-1, injure podocytes, change glomerular permeability, and mediate the progression of inflammation and fibrosis (132–134).
The hypothesis regarding the gut-lung-kidney axis on IgAN is shown in Figure 2.
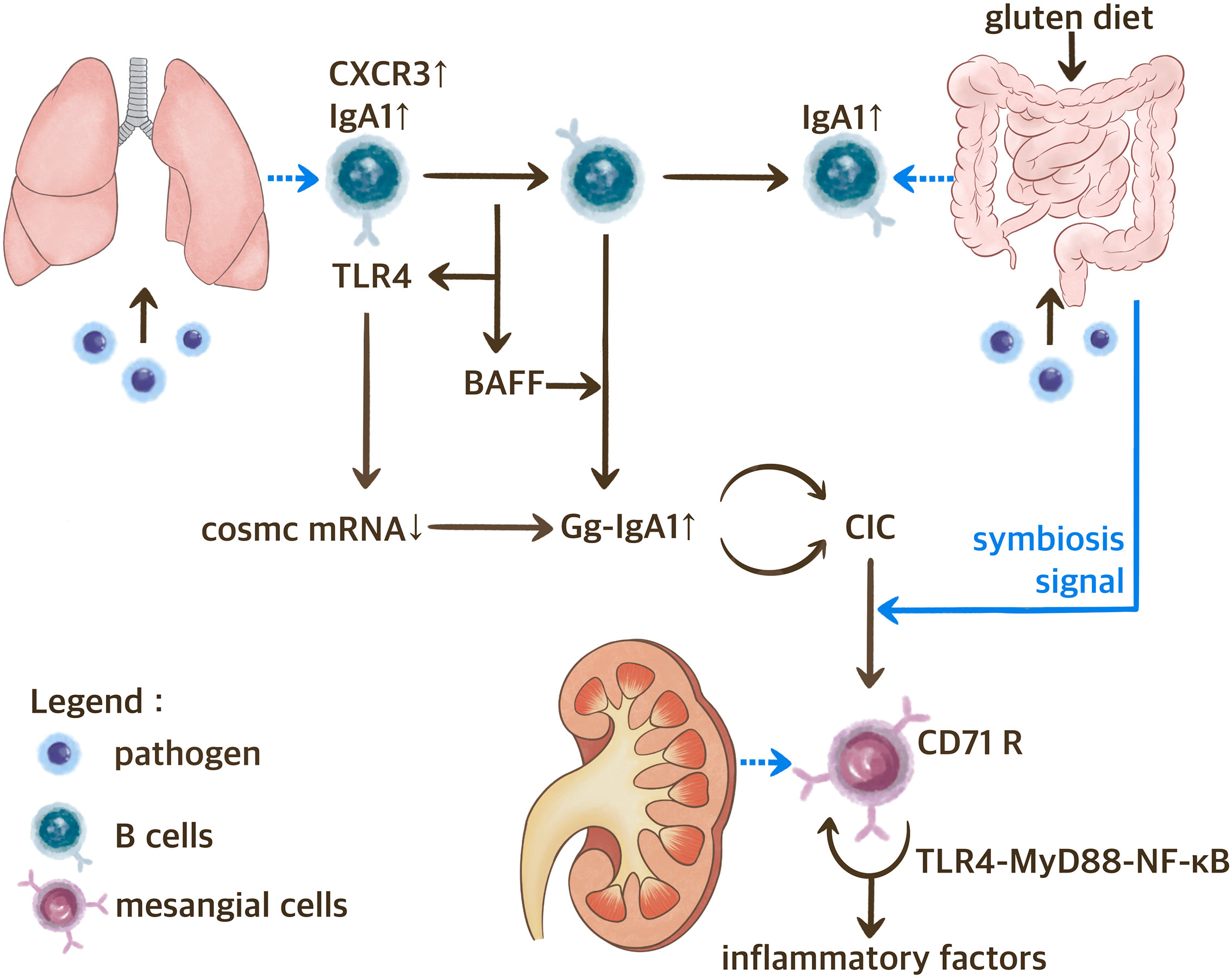
Figure 2 The Gut-Lung-Kidney Axis Hypothesis of IgAN. BAFF, B cell activating factor; CIC, circulating immune complex; CXCR3, C-X-C motif chemokine receptor 3; Gd-IgA1, IgA1 with galactose deficiency; TLR, toll-like receptor.
6.2 PR3-ANCA-associated vasculitis
ANCA-associated vasculitis (AAV) is a group of chronic inflammatory systemic autoimmune diseases characterized by high circulating ANCA levels. AAV pathogenesis occurs when ANCA levels exceed the limits of human self-tolerance. This section describes the pathological mechanisms underlying Wegener’s granulomatosis (WG), also known as GPA.
The levels of Th17/Treg cells is believed to be an important link in the pathogenesis of GPA. However, the role of B lymphocytes in the pathogenesis may be limited (135). An increase in PR3-specific Th17 cells and IL-17 levels was observed in the peripheral blood of patients with GPA (136). IL-17 is an important cytokine against bacterial infection that can promote granuloma formation, indirectly attract and stimulate neutrophils, and induce ANCA production (137, 138). Tadema et al. highlighted the importance of TLR-related pathways in IL-17 production (139). Given that these pathways are part of classic innate immune defense line and an important component in identifying PAMPs, it is consistent with a previous inference that GPA is closely related to infections (140, 141).
6.2.1 Respiratory infections and Th17 cells
Most studies indicate that specific pathogens are closely related to the occurrence and development of GPA. The most widely known bacteria are gram-positive bacteria (mainly Staphylococcus aureus), which are involved in IL-17 production in humans (142). Stegeman et al. reported a correlation between WG and Staphylococcus aureus mucosal infections; 95% of patients with GPA were infected with S. aureus, compared to only 28% of healthy people (143).
Further studies found S. aureus in the lungs of patients with GPA and demonstrated the benefits of antibacterial maintenance treatment. This elucidated the vital role of S. aureus infection in the pathology of WG (144, 145). The cell wall components of S. aureus include lipid teichoic acid and peptidoglycans (both TLR2 ligands), which may stimulate the release of IL-17 via TLR2-related pathways during respiratory tract infection (146–148).
In addition, gram-negative bacteria play important roles in pathogenesis. Many animal experiments have demonstrated the process of lung infection caused by gram-negative bacteria. TLR4 mediates CD4+ T cells producing IL-17 through IL-23, which is the dominant component of IL-17-inducing activity (149). The process of IL-23 participation appears to be very complex, and it involves the Jak2, PI3K/Akt, STAT3, and NF- κ B pathways (149, 150). Most studies suggest that TLR4 is upstream of IL-17; however, some studies suggest underlying interactions between IL-17 and TLR4 (151). Neutrophil extracellular traps (NETs) are released during IL-17- neutrophil recruitment, stimulating granuloma production. Histones, the main protein components of NETs, interact with TLR2 expressed on T cells, directly induce STAT3 phosphorylation, and ultimately activate Th17 cells (152). The interactions can lead to a vicious cycle of granuloma stimulation.
Excluding cell wall components, most bacteria contain CpG DNA (the TLR9 ligand), which is involved in the release of pro-inflammatory cytokines such as TNF-a and IL-1β (153). TLR4 may further interact with inflammatory cytokines to cause pulmonary fibrosis, which is a potential pathogenesis of AAV (154).
6.2.2 Gut-lung balance surrounding Th17 cells
Since clinical manifestations are significantly different in patients with GPA, particularly with regards to the respiratory system and kidneys, some patients may experience gastrointestinal symptoms such as diarrhea. Therefore, it is easy to assume the immune connection between these organs.
The aforementioned studies are limited to patients with respiratory infections. Some studies have demonstrated a relationship between GI pathogenic microorganisms, especially Helicobacter pylori (HP), and the incidence of granulomatosis, providing a theoretical basis for intestinal involvement in the pathogenesis of GPA (155). However, data on the relationship between HP and GPA is limited. Further research is needed to elucidate the potential mechanism.
In intestinal studies, NETs have been shown to promote inflammation and apoptosis by stimulating the TLR9-mediated endoplasmic reticulum stress pathway (156) or damaging the F-actin cytoskeleton of enterocyte-like cells. This leads to intestinal epithelial damage and intestinal barrier dysfunction (157), which can cause bacterial translocation and release of intestinal inflammatory factors into the circulation (158), further affecting the composition of the gut microbiota and abundance of probiotics (159). Destruction of the gut microbiota may affect IL-17 production in the intestine (101), TLR4/NF-kB signal pathways in the lungs (160), and lead to abnormal NETs production (161), ultimately damaging the immune function of the lungs.
A study on the influenza A virus suggested its possible mechanism, proposed that the lung and intestinal mucosal state of infected mice was regulated by the balance of Th17/Treg cells. After oral administration of Houttuynia cordata polysaccharides (HCP), the balance of Th17/Treg cells was first restored in the gut mucosal-associated lymphoid tissue (GALT), followed by the lungs. HCP regulates the expression of chemokine CCL20 and CCR6 (the CCL20 receptor), promoting the specific migration of Th17/Treg cells from the gut to the lungs (162).
6.2.3 Intestinal microbiota participates in gut-kidney axis migration of Th17 cells
The renal complications of GPA also involve the intestine. Krebs et al. found that mice infected with S. aureus had persistent Th17 cells in the kidney tissue and worsened crescentic glomerulonephritis (163). Th17 cells were amplified by Citrobacter rodentium infection in the intestine, migrating from the intestinal lamina propria to the kidney via the CCL20/CCR6 axis and exacerbating renal pathology in AAV mice. The cultivation of these mice under sterile conditions or antibiotic treatment significantly downregulates Th17 cells and alleviates kidney inflammation. This indicates a relationship between the gut microbiota, intestinal local immunity, and kidney involvement (164).
6.2.4 EBV infection and renal complications of GPA
EBV is a common human herpes virus. After the initial infection, most patients have no symptoms; however, EBV infects the B cells through oral epithelial cells and establishes a lifelong latent infection (165).
Patients with GPA exhibited more antibodies against EBV viral capsid antigen IgG and EBV early antigen IgG compared to healthy individuals. Patients with GPA presenting with increased titers of EBV viral capsid antigen IgG antibodies suffer from increased renal damage and have a higher Birmingham vasculitis activity score (104). Due to the interconnected anatomical structure of the URT and lungs, this may indicate an underlying connection between the renal system and lungs.
6.3 Systemic lupus erythematosus
SLE is an autoimmune disease characterized by abnormalities in adaptive immunity (166, 167) and accompanied by destruction of innate immunity (168).
6.3.1 Intestinal mucosal infection in SLE
Few studies exist on lupus-associated lung and GI injuries, especially on GI symptoms that are not clinically evident. However, in various human and animal experiments, serum PAMPs (e.g., LPS and BG) increased during the active period of lupus and were associated with leaky gut (169–171).
The close association of foreign pathogen infections with TLR-related pathways is detailed in the manuscript section on AAV. It has been suggested that, in TLR-2 and TLR-4 deficient mice, glomerular IgG deposition and mesangial cell proliferation are significantly reduced, and antinuclear, anti-dsDNA, and anti-cardiolipin autoantibody titers are decreased. This indicates the importance of pathogen infection in lupus (172).
In addition to damaging the intestinal barrier, some pathogens can expedite SLE onset through molecular simulations. Increase in anti-gut Ruminococcus gnavus (RG) was directly correlated with a high SLE disease activity index score (173). LPS cross-reacts with lupus anti-dsDNA antibodies (SLE-specific antibodies) (173). In addition, the latent viral protein Epstein–Barr virus nuclear antigen-1 (EBNA-1) cross-reacts with the Ro 60 kDa antibody, which is a common antibody in SLE (174).
6.3.2 Intestinal ecology regulates renal immunity
Furthermore, Mu et al. confirmed that activation of LN is regulated through intestinal ecology, not only through gut leakage. Supplementing female MRL/lpr mice with lactobacillus was found to reduce IL-6, increase IL-10, and shift the Treg/Th17 balance inside the kidney toward the Treg phenotype to protect renal function (175). However, contrasting results were observed in pregnant and lactating mice, in whom indoleamine 2,3-dioxygenase enzyme is inhibited upon supplementation with lactobacilli. This reduces Treg activation and increases the levels of serum INF γ (176), an important pro-inflammatory cytokine that promotes the development of lupus (177).
6.3.3 Air quality and LN
As shown by some studies, particulate matter 2.5 mum or less in diameter, which is related to the incidence and severity of lupus, leads to an increase in circulating neutrophils, proteinuria, and kidney weight; in addition, improving air quality can effectively alleviate the condition (178, 179). These findings demonstrate the relationship between the respiratory system and LN.
Some researchers believe that environmental molecules (e.g., LPS and BG) can serve as TLR-4 ligands and activate the Fc gamma receptor (FcγR) together, possibly through FcγR-TLR-4 crosstalk, thereby inducing synergistic pro-inflammatory responses (180, 181). The interaction of TLR4 and inflammatory cytokines may lead to pulmonary fibrosis in AAV and lupus patients (154). In addition, blocking spleen tyrosine kinases (the shared downstream signaling molecules of Fc gamma receptor (FcγR) and TLR-4) can alleviate lupus-induced inflammation (171).
6.3.4 Intestinal ecological imbalance and air pollution co-induce LN
In SLE, autoantibodies bind to cell-expressed antigens to form an immune complex (IC). These ICs pass through FcγR and bind to inflammatory cells, causing chronic inflammation and target cell destruction. This induces the formation of NET, which is degraded and impaired due to IFN (182, 183). Abnormal NETs are difficult to clear; therefore, the body must be exposed to dsDNA for a long time to produce antibodies (183). Yun et al. demonstrated that anti-dsDNA antibodies bind directly to mesangial and proximal renal tubular epithelial cells, triggering subsequent inflammatory responses through protein kinase C and mitogen-activated protein kinases pathways, eventually inducing fibrosis (184).
Meanwhile, Henault et al. reported that dsDNA-specific IgE antibodies activated plasmacytoid dendritic cells (pDCs) in SLE, resulting in the production of substantial amounts of IFN-α (a cytokine closely related to the degree of SLE activity), and TLR9 mediated dsDNA sensing (185, 186). pDCs accumulate in the glomeruli of patients with active LN (187).
Overall, the intestinal leakage of lupus enhances the level of serum pathogen molecules (e.g., LPS and BG) and cytokines (IFN- γ and IL-6), leading to extracellular DNA exposure and production of autoantibodies and cross-reactive antibodies. Environmental molecules (e.g., PM2.5) may participate in this process through the lungs. However, the TLR-4-related pathway actively promotes inflammation and induces subsequent pulmonary and renal fibrosis. On the other hand, dsDNA-specific IgE antibodies activate pDCs of the peripheral circulation and glomerulus, increase secretion of IFN- α, and enhance renal inflammation.
6.4 Coronavirus disease 2019
The oral cavity and conjunctiva are common infective routes of the coronavirus disease 2019 (COVID-19). Owing to the universality of angiotensin-converting enzyme 2 (ACE2) receptors in the human body, the virus can enter the body via these routes. Following this, the immune system responds quickly, activating immune cells and molecules (e.g., secretory IgA), which spread throughout the mucosa (188). High severe acute respiratory syndrome coronavirus 2 (SARS-CoV-2)-specific serum IgA levels were detected in the mucosal secretions; meanwhile, recirculating IgA-secreting plasmablasts with CCR10, which can efficiently home to and reside within the mucosa, were detected in infected individuals (189). Increased fecal calprotectin levels and severe ulcerative colitis in some patients are indicative of intestinal inflammatory response related to SARS-CoV-2 (190). Intestinal ecological imbalance can be observed in patients with COVID-19 infection, with decreased beneficial bacteria and increased opportunistic pathogens. Yeoh et al. reported a long-term lack of intestinal probiotics (e.g. Faecalibacterium prausnitzii, Eubacterium rectale, and bifidobacteria) following COVID-19 infection (191). In addition, Zuo et al. found an increase in the number of intestinal opportunistic pathogens (e.g. Coprobacillus, Clostridium ramosum, and Clostridium hathewayi) in patients with COVID-19 (192).
Similar to the above-mentioned diseases, gut leakage may exist in COVID-19 infection, causing the virus to directly attack the kidneys (193). High levels of ACE2 and cellular transmembrane serine proteases were successfully detected in renal tubular epithelial cells and podocytes, revealing the underlying mechanism of SARS-CoV-2-related renal injury (194). The autopsy results of 26 patients who died when infected with COVID-19 provided strong evidence for this hypothesis (195). Moreover, as an important factor in the renin-angiotensin-aldosterone system, abnormal activation of ACE2 may further accelerate pro-inflammatory and fibrotic processes in the kidneys (196).
6.5 Acute kidney injury-CKD in sepsis
Common complications of sepsis include acute lung injury (ALI) and acute kidney injury (AKI) among others. In some patients, AKI cannot be completely reversed and it develops into CKD, which involves complex immune responses.
6.5.1 Damage of intestinal barrier before the onset of sepsis
Research has found that the potential disruption of the intestinal barrier occurs prior to the onset of sepsis. A genome study on intestinal microorganisms and metabolites in patients with severe sepsis suggests that there is a substantial negative correlation between Coprococcus2 and the incidence rate of sepsis. Coprococcus2 is known as one of the butyrate-product bacteria. There is a significant correlation between α-hydroxybutyrate, a nascent biomarker for hypoxia and/or mitochondrial dysfunction, the incidence of sepsis, and the 28-day mortality rate (197).
The imbalance of intestinal homeostasis further exacerbates sepsis through gut leaky. The subsequent development of sepsis is closely related to IRF4.
6.5.2 IRF4 affects the lungs and kidneys by regulating macrophage polarization
IRF4 is a transcription factor in the IRF family which is expressed in various immune cells and can regulate immune response, cell growth, and metabolism. IRF4 is a multi-effect factor. Moreover, it can promote macrophage polarization toward the M2 phenotype and macrophage apoptosis, reduce macrophage migration and inflammatory factor expression, induce fibrosis, as well as negatively regulate TLR-related pathways. Furthermore, it promotes the maturation of Th17 cells and maintains an inflammatory state of the tissue by T cell-derived IL-17 (198).
An experiment on LPS-induced sepsis mice model suggests TLR-related pathways, as principle innate immune defense lines, are extensively activated in sepsis (199). he balance of Treg/Th17 immune cells is disrupted, and a large amount of IL-17 is secreted (200).
IRF4 expression is inhibited and thereby macrophages polarize towards M1. The maintenance of an inflammatory state leads to the occurrence of ALI. After the application of Dihydroquercetin or Dexmedetomidine, IRF4 is upregulated in sepsis mice models, and macrophages polarized towards M2, producing effective anti-inflammatory effects (201, 202).
The M2 state of macrophages also promotes fibrosis of renal tissue, which is one of the core mechanisms of AKI-CKD (198).
Sasaki et al. demonstrated that IRF4-deficient mice exhibited a decrease in renal interstitial fibrosis in ischemic kidney damage compared to normal mice, confirming the core role of IRF4 in this process (203).
6.5.3 Expression of intestinal IRF4 in sepsis: a blueprint yet to be elucidated
Interestingly, it has been observed in the mice models of inflammatory bowel disease, that the expression of IRF4 in CD3+T cells in the intestinal lamina propria is increased. Internal and exogenous IRF4 synergistically promotes the maturation of Th17 cells and maintains an inflammatory state in the intestine (204, 205).
This appears to be in contrast to the decrease in IRF4 levels measured in sepsis mice models. Hence, it is probable that depending on different organs/tissues, diseases, and compensatory states, the main regulatory direction of IRF4 may also be different.
Since the intestinal barrier disrupts before sepsis as mentioned above, we believe that changes in intestinal IRF4 expression and its impact on the internal environment may be important factors that have not yet been elucidated in our understanding of the mechanism of sepsis. Further experiments are needed in this regard.
6.6 Kidney allograft rejection
Kidney transplantation is an ideal treatment method for ESRD patients, but rejection reactions are common. Kidney allograft rejection can be classified into T-cell-mediated rejection and antibody-mediated rejection (ABMR) (206).
6.6.1 Influence of gut microbiota on the outcome of kidney transplantation
Immune tolerance is crucial for ensuring graft function and survival of transplant recipients. Peripheral tolerance of T lymphocytes is the main mechanism of organ transplantation tolerance. In this process, Tregs inhibit alloreactive T lymphocytes (207).
Researchers have found an immunological association between the gut microbiome and prognosis of kidney transplantation.
According to Wang et al., there is a significant difference in the gut microbiota between kidney transplant recipients (KTRs) with ABMR and KTRs without graft rejection. ABMR is associated with a lower microbial abundance of Clostridia, Paraprevotellaceae, and Faecalibacterium, as well as a higher abundance of Enterococcaceae, Coprobacillus, and Enterobacter (208).
Another study analyzed the rectal microbiota of four KTRs who experienced rejection and compared their observations with KTRs who did not experience rejection before and after transplantation. The decrease in the abundance of Anaerotruncatus, Coprobacillus and other bacteria was related to the development of future exclusion events (209).
Clostridia can regulate Tregs differentiation (207) and is related to a lower IL-6 level (210). In addition, lower IL-17 production is associated with a higher abundance of Faecalibacterium, while a higher abundance of Escherichia, Anaerotruncus, Coprobacillus, and Clostridium is linked to positive regulation in IL-17 production (210).
The gut microbial community affects cytokine levels and differentiation of immune cells, thereby affecting the prognosis of transplant hosts.
6.6.2 Oral microbiome and side effects of kidney transplantation
Rejection reactions could be acute or chronic. However, since they are not autoimmune diseases, kidney allograft rejection seems to have little connection with the entire respiratory tract when compared to other immune-related CKD.
According to reports, the prevalence of opportunistic pathogens, such as Enterobacteriaceae, Pseudomonas fluorescens, Actinetobacter spp., and Vibrio spp., is increased in the oral microbiome of transplant patients. Oral cancer is often observed in KTRs (211). Whether the higher incidence rate of oral cancer is related to the change in microbial activity in the lungs and intestines or is a result of the use of immunosuppressants after transplantation remains unclear.
7 New targets for treatment
At present, the mainstream treatment for pathogenic chain reactions caused by intestinal homeostasis imbalance is to restore abnormal gut microbiota to normal. Within the scope of this review, prebiotics and probiotics can be used as targeted interventions for the treatment of CKD. They can regulate intestinal microbiota, improve lipid profiles, reduce uremic toxins, maintain intestinal homeostasis, as well as reduce systemic inflammation and oxidative stress (17, 212). A survey involving 13271 Korean adults suggests that the prevalence of CKD is significantly lower in patients who take probiotic supplements than in those who do not (213).
Laxatives may be a new targeted intervention for gut microbiota in CKD. Sumida et al. demonstrated that patients with constipation have a significantly higher risk of incidental CKD and ESRD (214); the authors published a review on constipation and CKD in 2020, comparing the improving effects of various constipation drugs on CKD progression (215).
In China, Rhubarb officinale, a traditional Chinese herbal medicine with a laxative effect, is commonly used to treat CKD, and has a synergistic effect on angiotensin-converting enzyme inhibitors or angiotensin receptor blockers (216).
Another study conducted on 5/6 nephrectomy model rats showed that rhubarb enemas can regulate the abundance, and composition of the intestinal flora, increase SCFA levels, improve intestinal barrier damage, reduce inflammation levels, improve kidney pathology, reduce blood creatinine levels. However, the detailed mechanisms remain unclear. Further studies on the involvement of rhubarb in the gut-kidney axis are warranted (217).
The above two therapies mainly focus on the microbiota, considering the impact of local intestinal immunity on IgAN, several studies have been conducted on gut-targeted-release formulation of budesonide. Compared to systemic glucocorticoid therapy, its efficacy and safety have been effectively improved (218). The effectiveness of this drug on other immune-related CKD needs further confirmation.
8 Conclusions
In the field of immune-related CKD, a new concept of the gut-lung-kidney axis concept needs to be developed, which may be applied to clinical practice. Changes in intestinal homeostasis affect innate and adaptive immunities, as well as the gut-lung and gut-kidney axes, resulting in mutual interference between the intestine, lungs, and kidneys. In addition, existing data show that environmental molecules dominated by PAMPs respond to intestinal symbiotic communities through the lungs, which affect the progression of immune-related CKD.
Author contributions
XL: Writing – original draft. XW: Writing – original draft. PZ: Writing – review & editing. YF: Writing – review & editing. YD: Writing – review & editing. YL: Writing – review & editing. WZ: Writing – review & editing.
Funding
The author(s) declare financial support was received for the research, authorship, and/or publication of this article. This study was supported by research agreement funding sponsored by the Lin’an District Bureau of Science and Technology Scientific Research Project (2022Y13).
Conflict of interest
The authors declare that the research was conducted in the absence of any commercial or financial relationships that could be construed as a potential conflict of interest.
Publisher’s note
All claims expressed in this article are solely those of the authors and do not necessarily represent those of their affiliated organizations, or those of the publisher, the editors and the reviewers. Any product that may be evaluated in this article, or claim that may be made by its manufacturer, is not guaranteed or endorsed by the publisher.
Glossary
References
1. Luo C, Ouyang Y, Shi S, Li G, Zhao Z, Luo H, et al. Particulate matter of air pollution may increase risk of kidney failure in iga nephropathy. Kidney Int (2022) 102(6):1382–91. doi: 10.1016/j.kint.2022.08.020
2. Magistroni R, D’Agati VD, Appel GB, Kiryluk K. New developments in the genetics, pathogenesis, and therapy of iga nephropathy. Kidney Int (2015) 88(5):974–89. doi: 10.1038/ki.2015.252
3. Zhao W-M, Wang Z-J, Shi R, Zhu Y-Y, Zhang S, Wang R-F, et al. Environmental factors influencing the risk of anca-associated vasculitis. Front Immunol (2022) 5154:991256. doi: 10.3389/fimmu.2022.991256
4. Ciavatta D, Falk R. Epigenetics and complementary proteins. Clin Exp Immunol (2011) 164(Supplement_1):17–9. doi: 10.1111/j.1365-2249.2011.04360.x
5. Barbhaiya M, Costenbader KH. Environmental exposures and the development of systemic lupus erythematosus. Curr Opin Rheumatol (2016) 28(5):497. doi: 10.1097/BOR.0000000000000318
6. Wu H, Chang C, Lu Q. The epigenetics of lupus erythematosus. Epigenet Allergy Autoimmun (2020) 1253:185–207. doi: 10.1007/978-981-15-3449-2_7
7. Pohjonen JT, Kaukinen KM, Metso MJ, Nurmi RK, Huhtala HS, Pörsti IH, et al. Presence of gastrointestinal symptoms in iga nephropathy: A cross-sectional study. BMC Nephrol (2022) 23(1):395. doi: 10.1186/s12882-022-03019-8
8. Vereecke L, Beyaert R, van Loo G. Enterocyte death and intestinal barrier maintenance in homeostasis and disease. Trends Mol Med (2011) 17(10):584–93. doi: 10.1016/j.molmed.2011.05.011
9. McDermott AJ, Huffnagle GB. The microbiome and regulation of mucosal immunity. Immunology (2014) 142(1):24–31. doi: 10.1111/imm.12231
10. Yang T, Richards EM, Pepine CJ, Raizada MK. The gut microbiota and the brain–gut–kidney axis in hypertension and chronic kidney disease. Nat Rev Nephrol (2018) 14(7):442–56. doi: 10.1038/s41581-018-0018-2
11. Xu H, Gasparini A, Ishigami J, Mzayen K, Su G, Barany P, et al. Egfr and the risk of community-acquired infections. Clin J Am Soc Nephrol (2017) 12(9):1399–408. doi: 10.2215/CJN.00250117
12. Su G, Trevisan M, Ishigami J, Matsushita K, Stålsby Lundborg C, Carrero JJ. Short-and long-term outcomes after incident pneumonia in adults with chronic kidney disease: A time-dependent analysis from the stockholm creatinine measurement project. Nephrol Dial Transplant (2020) 35(11):1894–900. doi: 10.1093/ndt/gfz119
13. Enaud R, Prevel R, Ciarlo E, Beaufils F, Wieërs G, Guery B, et al. The gut-lung axis in health and respiratory diseases: A place for inter-organ and inter-kingdom crosstalks. Front Cell infection Microbiol (2020) 10:9. doi: 10.3389/fcimb.2020.00009
14. Qin J, Li R, Raes J, Arumugam M, Burgdorf KS, Manichanh C, et al. A human gut microbial gene catalogue established by metagenomic sequencing. Nature (2010) 464(7285):59–65. doi: 10.1038/nature08821
15. Fan Y, Pedersen O. Gut microbiota in human metabolic health and disease. Nat Rev Microbiol (2021) 19(1):55–71. doi: 10.1038/s41579-020-0433-9
16. Eckburg PB, Bik EM, Bernstein CN, Purdom E, Dethlefsen L, Sargent M, et al. Diversity of the human intestinal microbial flora. science (2005) 308(5728):1635–8. doi: 10.1126/science.1110591
17. Guan Z-W, Yu E-Z, Feng Q. Soluble dietary fiber, one of the most important nutrients for the gut microbiota. Molecules (2021) 26(22):6802. doi: 10.3390/molecules26226802
18. Sun M, Wu W, Liu Z, Cong Y. Microbiota metabolite short chain fatty acids, gpcr, and inflammatory bowel diseases. J Gastroenterol (2017) 52:1–8. doi: 10.1007/s00535-016-1242-9
19. Blaak E, Canfora E, Theis S, Frost G, Groen A, Mithieux G, et al. Short chain fatty acids in human gut and metabolic health. Beneficial Microbes (2020) 11(5):411–55. doi: 10.3920/BM2020.0057
20. De Vadder F, Kovatcheva-Datchary P, Zitoun C, Duchampt A, Bäckhed F, Mithieux G. Microbiota-produced succinate improves glucose homeostasis via intestinal gluconeogenesis. Cell Metab (2016) 24(1):151–7. doi: 10.1016/j.cmet.2016.06.013
21. Brown L, Rosner B, Willett WW, Sacks FM. Cholesterol-lowering effects of dietary fiber: A meta-analysis. Am J Clin Nutr (1999) 69(1):30–42. doi: 10.1093/ajcn/69.1.30
22. Canfora EE, Jocken JW, Blaak EE. Short-chain fatty acids in control of body weight and insulin sensitivity. Nat Rev Endocrinol (2015) 11(10):577–91. doi: 10.1038/nrendo.2015.128
23. Brown EM, Sadarangani M, Finlay BB. The role of the immune system in governing host-microbe interactions in the intestine. Nat Immunol (2013) 14(7):660–7. doi: 10.1038/ni.2611
24. Brown AJ, Goldsworthy SM, Barnes AA, Eilert MM, Tcheang L, Daniels D, et al. The orphan g protein-coupled receptors gpr41 and gpr43 are activated by propionate and other short chain carboxylic acids. J Biol Chem (2003) 278(13):11312–9. doi: 10.1074/jbc.M211609200
25. Macia L, Tan J, Vieira AT, Leach K, Stanley D, Luong S, et al. Metabolite-sensing receptors gpr43 and gpr109a facilitate dietary fibre-induced gut homeostasis through regulation of the inflammasome. Nat Commun (2015) 6(1):1–15. doi: 10.1038/ncomms7734
26. Vinolo MA, Hatanaka E, Lambertucci RH, Newsholme P, Curi R. Effects of short chain fatty acids on effector mechanisms of neutrophils. Cell Biochem Funct (2009) 27(1):48–55. doi: 10.1002/cbf.1533
27. Chang PV, Hao L, Offermanns S, Medzhitov R. The microbial metabolite butyrate regulates intestinal macrophage function via histone deacetylase inhibition. Proc Natl Acad Sci (2014) 111(6):2247–52. doi: 10.1073/pnas.1322269111
28. Park J, Kim M, Kang SG, Jannasch AH, Cooper B, Patterson J, et al. Short-chain fatty acids induce both effector and regulatory t cells by suppression of histone deacetylases and regulation of the mtor–s6k pathway. Mucosal Immunol (2015) 8(1):80–93. doi: 10.1038/mi.2014.44
29. Yen T-H, Wright NA. The gastrointestinal tract stem cell niche. Stem Cell Rev (2006) 2:203–12. doi: 10.1007/s12015-006-0048-1
30. Gewirtz AT, Navas TA, Lyons S, Godowski PJ, Madara JL. Cutting edge: Bacterial flagellin activates basolaterally expressed tlr5 to induce epithelial proinflammatory gene expression. J Immunol (2001) 167(4):1882–5. doi: 10.4049/jimmunol.167.4.1882
31. Abreu MT. Toll-like receptor signalling in the intestinal epithelium: How bacterial recognition shapes intestinal function. Nat Rev Immunol (2010) 10(2):131–44. doi: 10.1038/nri2707
32. Yang S, Eckmann L, Panja A, Kagnoff MF. Differential and regulated expression of cxc, cc, and c-chemokines by human colon epithelial cells. Gastroenterology (1997) 113(4):1214–23. doi: 10.1053/gast.1997.v113.pm9322516
33. Awane M, Andres PG, Li DJ, Reinecker H-C. Nf-κb-inducing kinase is a common mediator of il-17-, tnf-α-, and il-1β-induced chemokine promoter activation in intestinal epithelial cells. J Immunol (1999) 162(9):5337–44. doi: 10.4049/jimmunol.162.9.5337
34. Cheroutre H, Lambolez F, Mucida D. The light and dark sides of intestinal intraepithelial lymphocytes. Nat Rev Immunol (2011) 11(7):445–56. doi: 10.1038/nri3007
35. Wang H-C, Zhou Q, Dragoo J, Klein JR. Most murine cd8+ intestinal intraepithelial lymphocytes are partially but not fully activated t cells. J Immunol (2002) 169(9):4717–22. doi: 10.4049/jimmunol.169.9.4717
36. Moretto M, Weiss LM, Khan IA. Induction of a rapid and strong antigen-specific intraepithelial lymphocyte response during oral encephalitozoon cuniculi infection. J Immunol (2004) 172(7):4402–9. doi: 10.4049/jimmunol.172.7.4402
37. Boismenu R, Havran WL. Modulation of epithelial cell growth by intraepithelial γδ t cells. Science (1994) 266(5188):1253–5. doi: 10.1126/science.7973709
38. Reséndiz-Albor AA, Esquivel R, López-Revilla R, Verdín L, Moreno-Fierros L. Striking phenotypic and functional differences in lamina propria lymphocytes from the large and small intestine of mice. Life Sci (2005) 76(24):2783–803. doi: 10.1016/j.lfs.2004.08.042
39. Makita S, Kanai T, Oshima S, Uraushihara K, Totsuka T, Sawada T, et al. Cd4+ cd25bright t cells in human intestinal lamina propria as regulatory cells. J Immunol (2004) 173(5):3119–30. doi: 10.4049/jimmunol.173.5.3119
40. Kleinschek MA, Boniface K, Sadekova S, Grein J, Murphy EE, Turner SP, et al. Circulating and gut-resident human th17 cells express cd161 and promote intestinal inflammation. J Exp Med (2009) 206(3):525–34. doi: 10.1084/jem.20081712
41. Muñoz M, Heimesaat MM, Danker K, Struck D, Lohmann U, Plickert R, et al. Interleukin (il)-23 mediates toxoplasma gondii–induced immunopathology in the gut via matrixmetalloproteinase-2 and il-22 but independent of il-17. J Exp Med (2009) 206(13):3047–59. doi: 10.1084/jem.20090900
42. del Rio M-L, Rodriguez-Barbosa J-I, Boülter J, Ballmaier M, Dittrich-Breiholz O, Kracht M, et al. Cx3cr1+ c-kit+ bone marrow cells give rise to cd103+ and cd103– dendritic cells with distinct functional properties. J Immunol (2008) 181(9):6178–88. doi: 10.4049/jimmunol.181.9.6178
43. Varol C, Vallon-Eberhard A, Elinav E, Aychek T, Shapira Y, Luche H, et al. Intestinal lamina propria dendritic cell subsets have different origin and functions. Immunity (2009) 31(3):502–12. doi: 10.1016/j.immuni.2009.06.025
44. Spits H, Artis D, Colonna M, Diefenbach A, Di Santo JP, Eberl G, et al. Innate lymphoid cells—a proposal for uniform nomenclature. Nat Rev Immunol (2013) 13(2):145–9. doi: 10.1038/nri3365
45. Macpherson AJ, Slack E. The functional interactions of commensal bacteria with intestinal secretory iga. Curr Opin Gastroenterol (2007) 23(6):673–8. doi: 10.1097/MOG.0b013e3282f0d012
46. Cerutti A. Location, location, location: B-cell differentiation in the gut lamina propria. Mucosal Immunol (2008) 1(1):8–10. doi: 10.1038/mi.2007.8
47. Frankel G, Phillips AD, Novakova M, Field H, Candy D, Schauer DB, et al. Intimin from enteropathogenic escherichia coli restores murine virulence to a citrobacter rodentium eaea mutant: Induction of an immunoglobulin a response to intimin and espb. Infect Immun (1996) 64(12):5315–25. doi: 10.1128/iai.64.12.5315-5325.1996
48. Suzuki K, Meek B, Doi Y, Muramatsu M, Chiba T, Honjo T, et al. Aberrant expansion of segmented filamentous bacteria in iga-deficient gut. Proc Natl Acad Sci (2004) 101(7):1981–6. doi: 10.1073/pnas.0307317101
49. Chang W, Leblond C. Renewal of the epithelium in the descending colon of the mouse. I. Presence of three cell populations: Vacuolated-columnar, mucous and argentaffin. Am J Anat (1971) 131(1):73–99. doi: 10.1002/aja.1001310105
50. Karam SM. Lineage commitment and maturation of epithelial cells in the gut. Front Bioscience-Landmark (1999) 4(4):286–98. doi: 10.2741/Karam
51. Forman RA, deSchoolmeester ML, Hurst RJ, Wright SH, Pemberton AD, Else KJ. The goblet cell is the cellular source of the anti-microbial angiogenin 4 in the large intestine post trichuris muris infection. Plos One(2012) 7(9):e42248. doi: 10.1371/journal.pone.0042248
52. Burger-van Paassen N, Loonen LM, Witte-Bouma J, Korteland-van Male AM, De Bruijn AC, van der Sluis M, et al. Mucin muc2 deficiency and weaning influences the expression of the innate defense genes reg3β, reg3γ and angiogenin-4. PloS One (2012) 7(6):e38798. doi: 10.1371/journal.pone.0038798
53. McDole JR, Wheeler LW, McDonald KG, Wang B, Konjufca V, Knoop KA, et al. Goblet cells deliver luminal antigen to cd103+ dendritic cells in the small intestine. Nature (2012) 483(7389):345–9. doi: 10.1038/nature10863
54. Paray BA, Albeshr MF, Jan AT, Rather IA. Leaky gut and autoimmunity: An intricate balance in individuals health and the diseased state. Int J Mol Sci (2020) 21(24):9770. doi: 10.3390/ijms21249770
55. Kalayci F, Ozen S. Possible role of dysbiosis of the gut microbiome in sle. Curr Rheumatol Rep (2023), 1–12. doi: 10.1007/s11926-023-01115-8
56. Yu M, Li L, Ren Q, Feng H, Tao S, Cheng L, et al. Understanding the gut-kidney axis in antineutrophil cytoplasmic antibody-associated vasculitis: An analysis of gut microbiota composition. Front Pharmacol (2022) 13:783679. doi: 10.3389/fphar.2022.783679
57. Jee JJ, Lee YS, Bang JY, Lee HW, Koh H, Bae SH. Fecal microbiota transplantation improves hepatic fibro-inflammation via regulating oxidative stress in experimental nash. Dig Liver Dis (2023) S1590-8658(23)00712-0. doi: 10.1016/j.dld.2023.06.015
58. Tungsanga S, Udompornpitak K, Worasilchai J, Ratana-Aneckchai T, Wannigama DL, Katavetin P, et al. Candida administration in 5/6 nephrectomized mice enhanced fibrosis in internal organs: An impact of lipopolysaccharide and (1→ 3)-β-d-glucan from leaky gut. Int J Mol Sci (2022) 23(24):15987. doi: 10.3390/ijms232415987
59. Khiabani SA, Asgharzadeh M, Kafil HS. Chronic kidney disease and gut microbiota. Heliyon (2023) 9(8):e18991. doi: 10.1016/j.heliyon.2023.e18991
60. Chen Y-Y, Chen D-Q, Chen L, Liu J-R, Vaziri ND, Guo Y, et al. Microbiome–metabolome reveals the contribution of gut–kidney axis on kidney disease. J Transl Med (2019) 17(1):1–11. doi: 10.1186/s12967-018-1756-4
61. Jiang S, Xie S, Lv D, Wang P, He H, Zhang T, et al. Alteration of the gut microbiota in chinese population with chronic kidney disease. Sci Rep (2017) 7(1):2870. doi: 10.1038/s41598-017-02989-2
62. Wong J, Piceno YM, DeSantis TZ, Pahl M, Andersen GL, Vaziri ND. Expansion of urease-and uricase-containing, indole-and p-cresol-forming and contraction of short-chain fatty acid-producing intestinal microbiota in esrd. Am J Nephrol (2014) 39(3):230–7. doi: 10.1159/000360010
63. Li H-B, Xu M-L, Xu X-D, Tang Y-Y, Jiang H-L, Li L, et al. Faecalibacterium prausnitzii attenuates ckd via butyrate-renal gpr43 axis. Circ Res (2022) 131(9):e120–e34. doi: 10.1161/CIRCRESAHA.122.320184
64. Sirich TL, Plummer NS, Gardner CD, Hostetter TH, Meyer TW. Effect of increasing dietary fiber on plasma levels of colon-derived solutes in hemodialysis patients. Clin J Am Soc Nephrol (2014) 9(9):1603–10. doi: 10.2215/CJN.00490114
65. Mair RD, Sirich TL, Plummer NS, Meyer TW. Characteristics of colon-derived uremic solutes. Clin J Am Soc Nephrol (2018) 13(9):1398–404. doi: 10.2215/CJN.03150318
66. Wu I-W, Hsu K-H, Lee C-C, Sun C-Y, Hsu H-J, Tsai C-J, et al. P-cresyl sulphate and indoxyl sulphate predict progression of chronic kidney disease. Nephrol Dial Transplant (2011) 26(3):938–47. doi: 10.1093/ndt/gfq580
67. Ito S, Yoshida M. Protein-bound uremic toxins: New culprits of cardiovascular events in chronic kidney disease patients. Toxins (2014) 6(2):665–78. doi: 10.3390/toxins6020665
68. Koppe L, Pillon NJ, Vella RE, Croze ML, Pelletier CC, Chambert S, et al. P-cresyl sulfate promotes insulin resistance associated with ckd. J Am Soc Nephrol (2013) 24(1):88–99. doi: 10.1681/ASN.2012050503
69. Sun C-Y, Chang S-C, Wu M-S. Uremic toxins induce kidney fibrosis by activating intrarenal renin–angiotensin–aldosterone system associated epithelial-to-mesenchymal transition. PloS One (2012) 7(3):e34026. doi: 10.1371/journal.pone.0034026
70. Cosola C, Rocchetti MT, Cupisti A, Gesualdo L. Microbiota metabolites: Pivotal players of cardiovascular damage in chronic kidney disease. Pharmacol Res (2018) 130:132–42. doi: 10.1016/j.phrs.2018.03.003
71. Jourde-Chiche N, Dou L, Cerini C, Dignat-George F, Brunet P. Vascular Incompetence in Dialysis Patients—Protein-Bound Uremic Toxins and Endothelial Dysfunction. Semin Dial (2011) 24(3):327–37. doi: 10.1111/j.1525-139X.2011.00925.x
72. Viaene L, Annaert P, de Loor H, Poesen R, Evenepoel P, Meijers B. Albumin is the main plasma binding protein for indoxyl sulfate and p-cresyl sulfate. Biopharm Drug Dispos (2013) 34(3):165–75. doi: 10.1002/bdd.1834
73. Manor O, Zubair N, Conomos MP, Xu X, Rohwer JE, Krafft CE, et al. A multi-omic association study of trimethylamine n-oxide. Cell Rep (2018) 24(4):935–46. doi: 10.1016/j.celrep.2018.06.096
74. Xu J, Pan T, Qi X, Tan R, Wang X, Liu Z, et al. Increased mortality of acute respiratory distress syndrome was associated with high levels of plasma phenylalanine. Respir Res (2020) 21(1):1–13. doi: 10.1186/s12931-020-01364-6
75. Hatch M, Vaziri N. Enhanced enteric excretion of urate in rats with chronic renal failure. Clin Sci (1994) 86(5):511–6. doi: 10.1042/cs0860511
76. Bourke E, Milne M, Stokes G. Caecal ph and ammonia in experimental uraemia. Gut (1966) 7(5):558. doi: 10.1136/gut.7.5.558
77. Vaziri ND, Yuan J, Rahimi A, Ni Z, Said H, Subramanian VS. Disintegration of colonic epithelial tight junction in uremia: A likely cause of ckd-associated inflammation. Nephrol Dial Transplant (2012) 27(7):2686–93. doi: 10.1093/ndt/gfr624
78. Vaziri ND, Yuan J, Norris K. Role of urea in intestinal barrier dysfunction and disruption of epithelial tight junction in chronic kidney disease. Am J Nephrol (2013) 37(1):1–6. doi: 10.1159/000345969
79. Shi K, Wang F, Jiang H, Liu H, Wei M, Wang Z, et al. Gut bacterial translocation may aggravate microinflammation in hemodialysis patients. Dig Dis Sci (2014) 59:2109–17. doi: 10.1007/s10620-014-3202-7
80. Wang F, Zhang P, Jiang H, Cheng S. Gut bacterial translocation contributes to microinflammation in experimental uremia. Dig Dis Sci (2012) 57:2856–62. doi: 10.1007/s10620-012-2242-0
81. Thunø M, Macho B, Eugen-Olsen J. Supar: The molecular crystal ball. Dis Markers (2009) 27(3-4):157–72. doi: 10.1155/2009/504294
82. Wei C, Möller CC, Altintas MM, Li J, Schwarz K, Zacchigna S, et al. Modification of kidney barrier function by the urokinase receptor. Nat Med (2008) 14(1):55–63. doi: 10.1038/nm1696
83. Genua M, D’Alessio S, Cibella J, Gandelli A, Sala E, Correale C, et al. The urokinase plasminogen activator receptor (upar) controls macrophage phagocytosis in intestinal inflammation. Gut (2015) 64(4):589–600. doi: 10.1136/gutjnl-2013-305933
84. Hahm E, Wei C, Fernandez I, Li J, Tardi NJ, Tracy M, et al. Bone marrow-derived immature myeloid cells are a main source of circulating supar contributing to proteinuric kidney disease. Nat Med (2017) 23(1):100–6. doi: 10.1038/nm.4242
85. Wei C, El Hindi S, Li J, Fornoni A, Goes N, Sageshima J, et al. Circulating urokinase receptor as a cause of focal segmental glomerulosclerosis. Nat Med (2011) 17(8):952–60. doi: 10.1038/nm.2411
86. Musetti C, Quaglia M, Cena T, Chiocchetti A, Monti S, Clemente N, et al. Circulating supar levels are affected by glomerular filtration rate and proteinuria in primary and secondary glomerulonephritis. J Nephrol (2015) 28:299–305. doi: 10.1007/s40620-014-0137-1
87. Hayek SS, Landsittel DP, Wei C, Zeier M, Alan S, Torres VE, et al. Soluble urokinase plasminogen activator receptor and decline in kidney function in autosomal dominant polycystic kidney disease. J Am Soc Nephrol (2019) 30(7):1305–13. doi: 10.1681/ASN.2018121227
88. Sołtysiak J, Zachwieja J, Benedyk A, Lewandowska-Stachowiak M, Nowicki M, Ostalska-Nowicka D. Circulating supar as a biomarker of disease severity in children with proteinuric glomerulonephritis. Minerva Pediatr (2016) 71(1):4–11. doi: 10.23736/S0026-4946.16.04461-3
89. Hayek SS, Sever S, Ko Y-A, Trachtman H, Awad M, Wadhwani S, et al. Soluble urokinase receptor and chronic kidney disease. N Engl J Med (2015) 373(20):1916–25. doi: 10.1056/NEJMoa1506362
90. Sze MA, Dimitriu PA, Hayashi S, Elliott WM, McDonough JE, Gosselink JV, et al. The lung tissue microbiome in chronic obstructive pulmonary disease. Am J Respir Crit Care Med (2012) 185(10):1073–80. doi: 10.1164/rccm.201111-2075OC
91. Charlson ES, Bittinger K, Haas AR, Fitzgerald AS, Frank I, Yadav A, et al. Topographical continuity of bacterial populations in the healthy human respiratory tract. Am J Respir Crit Care Med (2011) 184(8):957–63. doi: 10.1164/rccm.201104-0655OC
92. Nguyen LD, Viscogliosi E, Delhaes L. The lung mycobiome: An emerging field of the human respiratory microbiome. Front Microbiol (2015) 6:89. doi: 10.3389/fmicb.2015.00089
93. Vandenborght L, Enaud R, Coron N, Denning D, Delhaes L. From culturomics to metagenomics: The mycobiome in chronic respiratory diseases. Lung Microbiome (Norwich: Eur Respir Society) (2019), 88–118. doi: 10.1183/2312508X.10015918
94. Liu T, Yang Z, Zhang X, Han N, Yuan J, Cheng Y. 16s rdna analysis of the effect of fecal microbiota transplantation on pulmonary and intestinal flora. 3 Biotech (2017) 7:1–9. doi: 10.1007/s13205-017-0997-x
95. Sze MA, Tsuruta M, Yang S-WJ, Oh Y, Man SP, Hogg JC, et al. Changes in the bacterial microbiota in gut, blood, and lungs following acute lps instillation into mice lungs. PloS One (2014) 9(10):e111228. doi: 10.1371/journal.pone.0111228
96. Dickson RP, Singer BH, Newstead MW, Falkowski NR, Erb-Downward JR, Standiford TJ, et al. Enrichment of the lung microbiome with gut bacteria in sepsis and the acute respiratory distress syndrome. Nat Microbiol (2016) 1(10):1–9. doi: 10.1038/nmicrobiol.2016.113
97. Samuelson DR, Welsh DA, Shellito JE. Regulation of lung immunity and host defense by the intestinal microbiota. Front Microbiol (2015) 6:1085. doi: 10.3389/fmicb.2015.01085
98. Johansson-Lindbom B, Agace WW. Generation of gut-homing t cells and their localization to the small intestinal mucosa. Immunol Rev (2007) 215(1):226–42. doi: 10.1111/j.1600-065X.2006.00482.x
99. Mikhak Z, Strassner JP, Luster AD. Lung dendritic cells imprint t cell lung homing and promote lung immunity through the chemokine receptor ccr4. J Exp Med (2013) 210(9):1855–69. doi: 10.1084/jem.20130091
100. Ruane D, Brane L, Reis BS, Cheong C, Poles J, Do Y, et al. Lung dendritic cells induce migration of protective t cells to the gastrointestinal tract. J Exp Med (2013) 210(9):1871–88. doi: 10.1084/jem.20122762
101. Bradley CP, Teng F, Felix KM, Sano T, Naskar D, Block KE, et al. Segmented filamentous bacteria provoke lung autoimmunity by inducing gut-lung axis th17 cells expressing dual tcrs. Cell Host Microbe (2017) 22(5):697–704.e4. doi: 10.1016/j.chom.2017.10.007
102. McAleer JP, Nguyen NL, Chen K, Kumar P, Ricks DM, Binnie M, et al. Pulmonary th17 antifungal immunity is regulated by the gut microbiome. J Immunol (2016) 197(1):97–107. doi: 10.4049/jimmunol.1502566
103. Gauguet S, D’Ortona S, Ahnger-Pier K, Duan B, Surana NK, Lu R, et al. Intestinal microbiota of mice influences resistance to staphylococcus aureus pneumonia. Infect Immun (2015) 83(10):4003–14. doi: 10.1128/IAI.00037-15
104. Lidar M, Lipschitz N, Langevitz P, Barzilai O, Ram M, Porat-Katz BS, et al. Infectious serologies and autoantibodies in wegener’s granulomatosis and other vasculitides: Novel associations disclosed using the rad bioplex 2200. Ann N Y Acad Sci (2009) 1173(1):649–57. doi: 10.1111/j.1749-6632.2009.04641.x
105. Su W-Y, Wu D-W, Tu H-P, Chen S-C, Hung C-H, Kuo C-H. Association between ambient air pollutant interaction with kidney function in a large Taiwanese population study. Environ Sci pollut Res Int. (2023) 30(34):82341–52. doi: 10.1007/s11356-023-28042-6
106. Duan J-W, Li Y-L, Li S-X, Yang Y-P, Li F, Li Y, et al. Association of long-term ambient fine particulate matter (pm2. 5) and incident ckd: A prospective cohort study in China. Am J Kidney Dis (2022) 80(5):638–47.e1. doi: 10.1053/j.ajkd.2022.03.009
107. Cirovic A, Denic A, Clarke BL, Vassallo R, Cirovic A, Landry GM. A hypoxia-driven occurrence of chronic kidney disease and osteoporosis in copd individuals: New insights into environmental cadmium exposure. Toxicology (2022) 482:153355. doi: 10.1016/j.tox.2022.153355
108. Park S, Lee S, Kim Y, Cho S, Kim K, Kim YC, et al. Kidney function and obstructive lung disease: A bidirectional mendelian randomisation study. Eur Respir J (2021) 58(6):2100848. doi: 10.1183/13993003.00848-2021
109. Wyatt RJ, Julian BA. Iga nephropathy. N Engl J Med (2013) 368(25):2402–14. doi: 10.1056/NEJMra1206793
110. Knoppova B, Reily C, Maillard N, Rizk DV, Moldoveanu Z, Mestecky J, et al. The origin and activities of iga1-containing immune complexes in iga nephropathy. Front Immunol (2016) 7:117. doi: 10.3389/fimmu.2016.00117
111. Moldoveanu Z, Moro I, Radl J, Thorpe S, Komiyama K, Mestecky J. Site of catabolism of autologous and heterologous iga in non-human primates. Scand J Immunol (1990) 32(6):577–83. doi: 10.1111/j.1365-3083.1990.tb03199.x
112. Suzuki H, Kiryluk K, Novak J, Moldoveanu Z, Herr AB, Renfrow MB, et al. The pathophysiology of iga nephropathy. J Am Soc Nephrol (2011) 22(10):1795–803. doi: 10.1681/ASN.2011050464
113. Oh JE, Song E, Moriyama M, Wong P, Zhang S, Jiang R, et al. Intranasal priming induces local lung-resident b cell populations that secrete protective mucosal antiviral iga. Sci Immunol (2021) 6(66):eabj5129. doi: 10.1126/sciimmunol.abj5129
114. MacLean AJ, Richmond N, Koneva L, Attar M, Medina CA, Thornton EE, et al. Secondary influenza challenge triggers resident memory b cell migration and rapid relocation to boost antibody secretion at infected sites. Immunity (2022) 55(4):718–33. doi: 10.1016/j.immuni.2022.03.003
115. Onodera T, Takahashi Y, Yokoi Y, Ato M, Kodama Y, Hachimura S, et al. Memory b cells in the lung participate in protective humoral immune responses to pulmonary influenza virus reinfection. Proc Natl Acad Sci (2012) 109(7):2485–90. doi: 10.1073/pnas.1115369109
116. Baenziger JU, Fiete D. Galactose and n-acetylgalactosamine-specific endocytosis of glycopeptides by isolated rat hepatocytes. Cell (1980) 22(2):611–20. doi: 10.1016/0092-8674(80)90371-2
117. Smith AC, Molyneux K, Feehally J, Barratt J. O-glycosylation of serum iga1 antibodies against mucosal and systemic antigens in iga nephropathy. J Am Soc Nephrol (2006) 17(12):3520–8. doi: 10.1681/ASN.2006060658
118. Eijgenraam J, Oortwijn B, Kamerling S, De Fijter J, Van Den Wall Bake A, Daha M, et al. Secretory immunoglobulin a (iga) responses in iga nephropathy patients after mucosal immunization, as part of a polymeric iga response. Clin Exp Immunol (2008) 152(2):227–32. doi: 10.1111/j.1365-2249.2008.03616.x
119. Suzuki Y, Suzuki H, Sato D, Kajiyama T, Okazaki K, Hashimoto A, et al. Reevaluation of the mucosa-bone marrow axis in iga nephropathy with animal models. Recent Adv tonsils Mucosal barriers upper airways (2011) 72:64–7. doi: 10.1159/000324608
120. Ruane D, Chorny A, Lee H, Faith J, Pandey G, Shan M, et al. Microbiota regulate the ability of lung dendritic cells to induce iga class-switch recombination and generate protective gastrointestinal immune responses. J Exp Med (2016) 213(1):53–73. doi: 10.1084/jem.20150567
121. Coppo R, Camilla R, Amore A, Peruzzi L, Daprà V, Loiacono E, et al. Toll-like receptor 4 expression is increased in circulating mononuclear cells of patients with immunoglobulin a nephropathy. Clin Exp Immunol (2010) 159(1):73–81. doi: 10.1111/j.1365-2249.2009.04045.x
122. Qin W, Zhong X, Fan JM, Zhang YJ, Liu XR, Ma XY. External suppression causes the low expression of the cosmc gene in iga nephropathy. Nephrol Dial Transplant (2008) 23(5):1608–14. doi: 10.1093/ndt/gfm781
123. Novak J, Vu HL, Novak L, Julian BA, Mestecky J, Tomana M. Interactions of human mesangial cells with iga and iga-containing immune complexes1. Kidney Int (2002) 62(2):465–75. doi: 10.1046/j.1523-1755.2002.00477.x
124. Papista C, Lechner S, Mkaddem SB, LeStang M-B, Abbad L, Bex-Coudrat J, et al. Gluten exacerbates iga nephropathy in humanized mice through gliadin–cd89 interaction. Kidney Int (2015) 88(2):276–85. doi: 10.1038/ki.2015.94
125. Moura IC, Centelles MN, Arcos-Fajardo M, Malheiros DM, Collawn JF, Cooper MD, et al. Identification of the transferrin receptor as a novel immunoglobulin (ig) a1 receptor and its enhanced expression on mesangial cells in iga nephropathy. J Exp Med (2001) 194(4):417–26. doi: 10.1084/jem.194.4.417
126. Wang Y, Qu K, Lu W, Zhao P, Wang Z, Cui C, et al. Neutrophils recruited to immunization sites initiating vaccine-induced antibody responses by locally expressing baff. iScience (2022) 25(6):104453. doi: 10.1016/j.isci.2022.104453
127. McCarthy DD, Chiu S, Gao Y, Summers-deLuca LE, Gommerman JL. Baff induces a hyper-iga syndrome in the intestinal lamina propria concomitant with iga deposition in the kidney independent of light. Cell Immunol (2006) 241(2):85–94. doi: 10.1016/j.cellimm.2006.08.002
128. McCarthy DD, Kujawa J, Wilson C, Papandile A, Poreci U, Porfilio EA, et al. Mice overexpressing baff develop a commensal flora–dependent, iga-associated nephropathy. J Clin Invest (2011) 121(10):3991–4002. doi: 10.1172/JCI45563
129. Xin G, Shi W, Xu L-X, Su Y, Yan L-J, Li K-S. Serum baff is elevated in patients with iga nephropathy and associated with clinical and histopathological features. J Nephrol (2012) 26(4):683–90. doi: 10.5301/jn.5000218
130. Li W, Peng X, Liu Y, Liu H, Liu F, He L, et al. Tlr9 and baff: Their expression in patients with iga nephropathy. Mol Med Rep (2014) 10(3):1469–74. doi: 10.3892/mmr.2014.2359
131. Monteiro RC, Berthelot L. Role of gut–kidney axis in renal diseases and iga nephropathy. Curr Opin Gastroenterol (2021) 37(6):565–71. doi: 10.1097/MOG.0000000000000789
132. Lai KN, Leung JC, Chan LY, Saleem MA, Mathieson PW, Lai FM, et al. Activation of podocytes by mesangial-derived tnf-α: Glomerulo-podocytic communication in iga nephropathy. Am J Physiology-Renal Physiol (2008) 294(4):F945–F55. doi: 10.1152/ajprenal.00423.2007
133. Lai KN, Leung JC, Chan LY, Saleem MA, Mathieson PW, Tam KY, et al. Podocyte injury induced by mesangial-derived cytokines in iga nephropathy. Nephrol Dial Transplant (2009) 24(1):62–72. doi: 10.1093/ndt/gfn441
134. Zhang J, Mi Y, Zhou R, Liu Z, Huang B, Guo R, et al. The tlr4-myd88-nf-κb pathway is involved in siga-mediated iga nephropathy. J Nephrol (2020) 33:1251–61. doi: 10.1007/s40620-020-00722-3
135. Zhao Y, Odell E, Choong LM, Barone F, Fields P, Wilkins B, et al. Granulomatosis with polyangiitis involves sustained mucosal inflammation that is rich in b-cell survival factors and autoantigen. Rheumatology (2012) 51(9):1580–6. doi: 10.1093/rheumatology/kes123
136. Abdulahad WH, Stegeman CA, Limburg PC, Kallenberg CG. Skewed distribution of th17 lymphocytes in patients with wegener’s granulomatosis in remission. Arthritis Rheumatism (2008) 58(7):2196–205. doi: 10.1002/art.23557
137. Abdulahad WH, Stegeman CA, Kallenberg CG. The role of cd4+ t cells in anca-associated systemic vasculitis. Nephrology (2009) 14(1):26–32. doi: 10.1111/j.1440-1797.2008.01069.x
138. Okamoto Yoshida Y, Umemura M, Yahagi A, O’Brien RL, Ikuta K, Kishihara K, et al. Essential role of il-17a in the formation of a mycobacterial infection-induced granuloma in the lung. J Immunol (2010) 184(8):4414–22. doi: 10.4049/jimmunol.0903332
139. Tadema H, Heeringa P, Kallenberg CG. Bacterial infections in wegener’s granulomatosis: Mechanisms potentially involved in autoimmune pathogenesis. Curr Opin Rheumatol (2011) 23(4):366–71. doi: 10.1097/BOR.0b013e328346c332
140. Wegener F. Uber eine eigenartige rhinogene granulomatose mit besonderer beteiligung des arteriensystems und der nieren. Beitr Path Anat (1993) 102:36.
141. Pinching A, Rees A, Pussell B, Lockwood C, Mitchison R, Peters D. Relapses in wegener’s granulomatosis: The role of infection. Br Med J (1980) 281(6244):836–8. doi: 10.1136/bmj.281.6244.836
142. Niebuhr M, Gathmann M, Scharonow H, Mamerow D, Mommert S, Balaji H, et al. Staphylococcal alpha-toxin is a strong inducer of interleukin-17 in humans. Infect Immun (2011) 79(4):1615–22. doi: 10.1128/IAI.00958-10
143. Laudien M, Gadola SD, Podschun R, Hedderich J, Paulsen J, Reinhold-Keller E, et al. Nasal carriage of staphylococcus aureus and endonasal activity in wegener’s granulomatosis as compared to rheumatoid arthritis and chronic rhinosinusitis with nasal polyps. Clin Exp Rheumatol (2010) 28(1):S51.
144. Zycinska K, Wardyn K, Zielonka T, Krupa R, Lukas W. Co-trimoxazole and prevention of relapses of pr3-anca positive vasculitis with pulmonary involvement. Eur J Med Res (2009) 14(4):1–3. doi: 10.1186/2047-783X-14-S4-265
145. Richter AG, Stockley RA, Harper L, Thickett DR. Pulmonary infection in wegener granulomatosis and idiopathic pulmonary fibrosis. Thorax (2009) 64(8):692–7. doi: 10.1136/thx.2008.110445
146. Gillrie MR, Zbytnuik L, McAvoy E, Kapadia R, Lee K, Waterhouse CC, et al. Divergent roles of toll-like receptor 2 in response to lipoteichoic acid and staphylococcus aureus. vivo. Eur J Immunol (2010) 40(6):1639–50. doi: 10.1002/eji.200939929
147. Bitto NJ, Cheng L, Johnston EL, Pathirana R, Phan TK, Poon IK, et al. Staphylococcus aureus membrane vesicles contain immunostimulatory DNA, rna and peptidoglycan that activate innate immune receptors and induce autophagy. J Extracellular Vesicles (2021) 10(6):e12080. doi: 10.1002/jev2.12080
148. Banerjee S, Ninkovic J, Meng J, Sharma U, Ma J, Charboneau R, et al. Morphine compromises bronchial epithelial tlr2/il17r signaling crosstalk, necessary for lung il17 homeostasis. Sci Rep (2015) 5(1):1–17. doi: 10.1038/srep11384
149. Happel KI, Zheng M, Young E, Quinton LJ, Lockhart E, Ramsay AJ, et al. Cutting edge: Roles of toll-like receptor 4 and il-23 in il-17 expression in response to klebsiella pneumoniae infection. J Immunol (2003) 170(9):4432–6. doi: 10.4049/jimmunol.170.9.4432
150. Cho M-L, Kang J-W, Moon Y-M, Nam H-J, Jhun J-Y, Heo S-B, et al. Stat3 and nf-κb signal pathway is required for il-23-mediated il-17 production in spontaneous arthritis animal model il-1 receptor antagonist-deficient mice. J Immunol (2006) 176(9):5652–61. doi: 10.4049/jimmunol.176.9.5652
151. Tang H, Pang S, Wang M, Xiao X, Rong Y, Wang H, et al. Tlr4 activation is required for il-17–induced multiple tissue inflammation and wasting in mice. J Immunol (2010) 185(4):2563–9. doi: 10.4049/jimmunol.0903664
152. Wilson AS, Randall KL, Pettitt JA, Ellyard JI, Blumenthal A, Enders A, et al. Neutrophil extracellular traps and their histones promote th17 cell differentiation directly via tlr2. Nat Commun (2022) 13(1):528. doi: 10.1038/s41467-022-28172-4
153. Hurtado PR, Jeffs L, Nitschke J, Patel M, Sarvestani G, Cassidy J, et al. Cpg oligodeoxynucleotide stimulates production of anti-neutrophil cytoplasmic antibodies in anca associated vasculitis. BMC Immunol (2008) 9(1):1–9. doi: 10.1186/1471-2172-9-34
154. Bolourani S, Brenner M, Wang P. The interplay of damps, tlr4, and proinflammatory cytokines in pulmonary fibrosis. J Mol Med (2021) 99(10):1373–84. doi: 10.1007/s00109-021-02113-y
155. Zycinska K, Wardyn K, Zycinski Z, Smolarczyk R. Correlation between helicobacter pylori infection and pulmonary wegener’s granulomatosis activity. J Physiol Pharmacol (2008) 59(Suppl 6):845–51.
156. Sun S, Duan Z, Wang X, Chu C, Yang C, Chen F, et al. Neutrophil extracellular traps impair intestinal barrier functions in sepsis by regulating tlr9-mediated endoplasmic reticulum stress pathway. Cell Death Dis (2021) 12(6):606. doi: 10.1038/s41419-021-03896-1
157. Marin-Esteban V, Turbica I, Dufour G, Semiramoth N, Gleizes A, Gorges R, et al. Afa/dr diffusely adhering escherichia coli strain c1845 induces neutrophil extracellular traps that kill bacteria and damage human enterocyte-like cells. Infect Immun (2012) 80(5):1891–9. doi: 10.1128/IAI.00050-12
158. Deitch EA. Gut-origin sepsis: Evolution of a concept. Surgeon (2012) 10(6):350–6. doi: 10.1016/j.surge.2012.03.003
159. Liang Y, Wang X, He D, You Q, Zhang T, Dong W, et al. Ameliorating gut microenvironment through staphylococcal nuclease-mediated intestinal nets degradation for prevention of type 1 diabetes in nod mice. Life Sci (2019) 221:301–10. doi: 10.1016/j.lfs.2019.02.034
160. Tang J, Xu L, Zeng Y, Gong F. Effect of gut microbiota on lps-induced acute lung injury by regulating the tlr4/nf-kb signaling pathway. Int Immunopharmacol (2021) 91:107272. doi: 10.1016/j.intimp.2020.107272
161. Vong L, Yeung CW, Pinnell LJ, Sherman PM. Adherent-invasive escherichia coli exacerbates antibiotic-associated intestinal dysbiosis and neutrophil extracellular trap activation. Inflammation Bowel Dis (2016) 22(1):42–54. doi: 10.1097/MIB.0000000000000591
162. Shi C-C, Zhu H-Y, Li H, Zeng D-L, Shi X-L, Zhang Y-Y, et al. Regulating the balance of th17/treg cells in gut-lung axis contributed to the therapeutic effect of houttuynia cordata polysaccharides on h1n1-induced acute lung injury. Int J Biol Macromol (2020) 158:52–66. doi: 10.1016/j.ijbiomac.2020.04.211
163. Krebs CF, Reimers D, Zhao Y, Paust H-J, Bartsch P, Nuñez S, et al. Pathogen-induced tissue-resident memory th17 (trm17) cells amplify autoimmune kidney disease. Sci Immunol (2020) 5(50):eaba4163. doi: 10.1126/sciimmunol.aba4163
164. Krebs CF, Paust H-J, Krohn S, Koyro T, Brix SR, Riedel J-H, et al. Autoimmune renal disease is exacerbated by s1p-receptor-1-dependent intestinal th17 cell migration to the kidney. Immunity (2016) 45(5):1078–92. doi: 10.1016/j.immuni.2016.10.020
165. Hayman IR, Temple RM, Burgess CK, Ferguson M, Liao J, Meyers C, et al. New insight into epstein-barr virus infection using models of stratified epithelium. PloS Pathog (2023) 19(1):e1011040. doi: 10.1371/journal.ppat.1011040
166. Ohl K, Tenbrock K. Inflammatory cytokines in systemic lupus erythematosus. J biotechnol biomed (2011) 2011:432595. doi: 10.1155/2011/432595
167. Liu Y-J. Ipc: Professional type 1 interferon-producing cells and plasmacytoid dendritic cell precursors. Annu Rev Immunol (2005) 23:275–306. doi: 10.1146/annurev.immunol.23.021704.115633
168. Herrada AA, Escobedo N, Iruretagoyena M, Valenzuela RA, Burgos PI, Cuitino L, et al. Innate immune cells’ contribution to systemic lupus erythematosus. Front Immunol (2019) 10:772. doi: 10.3389/fimmu.2019.00772
169. Thim-Uam A, Surawut S, Issara-Amphorn J, Jaroonwitchawan T, Hiengrach P, Chatthanathon P, et al. Leaky-gut enhanced lupus progression in the fc gamma receptor-iib deficient and pristane-induced mouse models of lupus. Sci Rep (2020) 10(1):777. doi: 10.1038/s41598-019-57275-0
170. Ogunrinde E, Zhou Z, Luo Z, Alekseyenko A, Li QZ, Macedo D, et al. A link between plasma microbial translocation, microbiome, and autoantibody development in first-degree relatives of systemic lupus erythematosus patients. Arthritis Rheumatol (2019) 71(11):1858–68. doi: 10.1002/art.40935
171. Issara-Amphorn J, Somboonna N, Pisitkun P, Hirankarn N, Leelahavanichkul A. Syk inhibitor attenuates inflammation in lupus mice from fcgriib deficiency but not in pristane induction: The influence of lupus pathogenesis on the therapeutic effect. Lupus (2020) 29(10):1248–62. doi: 10.1177/0961203320941106
172. Lartigue A, Colliou N, Calbo S, François A, Jacquot S, Arnoult C, et al. Critical role of tlr2 and tlr4 in autoantibody production and glomerulonephritis in lpr mutation-induced mouse lupus. J Immunol (2009) 183(10):6207–16. doi: 10.4049/jimmunol.0803219
173. Azzouz D, Omarbekova A, Heguy A, Schwudke D, Gisch N, Rovin BH, et al. Lupus nephritis is linked to disease-activity associated expansions and immunity to a gut commensal. Ann rheumatic Dis (2019) 78(7):947–56. doi: 10.1136/annrheumdis-2018-214856
174. McClain MT, Heinlen LD, Dennis GJ, Roebuck J, Harley JB, James JA. Early events in lupus humoral autoimmunity suggest initiation through molecular mimicry. Nat Med (2005) 11(1):85–9. doi: 10.1038/nm1167
175. Mu Q, Zhang H, Liao X, Lin K, Liu H, Edwards MR, et al. Control of lupus nephritis by changes of gut microbiota. Microbiome (2017) 5(1):1–12. doi: 10.1186/s40168-017-0300-8
176. Mu Q, Cabana-Puig X, Mao J, Swartwout B, Abdelhamid L, Cecere TE, et al. Pregnancy and lactation interfere with the response of autoimmunity to modulation of gut microbiota. Microbiome (2019) 7:1–13. doi: 10.1186/s40168-019-0720-8
177. Pollard KM, Cauvi DM, Toomey CB, Morris KV, Kono DH. Interferon-γ and systemic autoimmunity. Discovery Med (2013) 16(87):123. doi: 10.1186/s12989-021-00407-0
178. Yariwake VY, Torres JI, Dos Santos ARP, Freitas SCF, De Angelis K, Farhat SCL, et al. Chronic exposure to pm2. 5 aggravates sle manifestations in lupus-prone mice. Particle fibre Toxicol (2021) 18(1):1–16. doi: 10.1016/j.envres.2021.110875
179. Bai H, Jiang L, Li T, Liu C, Zuo X, Liu Y, et al. Acute effects of air pollution on lupus nephritis in patients with systemic lupus erythematosus: A multicenter panel study in China. Environ Res (2021) 195:110875. doi: 10.1016/j.envres.2021.110875
180. Molteni M, Gemma S, Rossetti C. The role of toll-like receptor 4 in infectious and noninfectious inflammation. Mediat Inflamm (2016) 2016:6978936. doi: 10.1155/2016/6978936
181. Vogelpoel LT, Hansen IS, Rispens T, Muller FJ, Van Capel TM, Turina MC, et al. Fc gamma receptor-tlr cross-talk elicits pro-inflammatory cytokine production by human m2 macrophages. Nat Commun (2014) 5(1):5444. doi: 10.1038/ncomms6444
182. Shashidharamurthy R, Machiah D, Bozeman EN, Srivatsan S, Patel J, Cho A, et al. Hydrodynamic delivery of plasmid DNA encoding human fcγr-ig dimers blocks immune-complex mediated inflammation in mice. Gene Ther (2012) 19(9):877–85. doi: 10.1038/gt.2011.175
183. van Dam LS, Kraaij T, Kamerling SW, Bakker JA, Scherer UH, Rabelink TJ, et al. Intrinsically distinct role of neutrophil extracellular trap formation in antineutrophil cytoplasmic antibody–associated vasculitis compared to systemic lupus erythematosus. Arthritis Rheumatol (2019) 71(12):2047–58. doi: 10.1002/art.41047
184. Yung S, Chan TM. Anti-dsdna antibodies and resident renal cells—their putative roles in pathogenesis of renal lesions in lupus nephritis. Clin Immunol (2017) 185:40–50. doi: 10.1016/j.clim.2016.09.002
185. Henault J, Riggs JM, Karnell JL, Liarski VM, Li J, Shirinian L, et al. Self-reactive ige exacerbates interferon responses associated with autoimmunity. Nat Immunol (2016) 17(2):196–203. doi: 10.1038/ni.3326
186. Infante B, Mercuri S, Dello Strologo A, Franzin R, Catalano V, Troise D, et al. Unraveling the link between interferon-α and systemic lupus erythematosus: From the molecular mechanisms to target therapies. Int J Mol Sci (2022) 23(24):15998. doi: 10.3390/ijms232415998
187. Peterson KS, Huang J-F, Zhu J, D’Agati V, Liu X, Miller N, et al. Characterization of heterogeneity in the molecular pathogenesis of lupus nephritis from transcriptional profiles of laser-captured glomeruli. J Clin Invest (2004) 113(12):1722–33. doi: 10.1172/JCI200419139
188. Russell MW, Moldoveanu Z, Ogra PL, Mestecky J. Mucosal immunity in covid-19: A neglected but critical aspect of sars-cov-2 infection. Front Immunol (2020) 11:3221. doi: 10.3389/fimmu.2020.611337
189. Sterlin D, Mathian A, Miyara M, Mohr A, Anna F, Claër L, et al. Iga dominates the early neutralizing antibody response to sars-cov-2. Sci Transl Med (2021) 13(577):eabd2223. doi: 10.1126/scitranslmed.abd2223
190. Mazza S, Sorce A, Peyvandi F, Vecchi M, Caprioli F. A fatal case of covid-19 pneumonia occurring in a patient with severe acute ulcerative colitis. Gut (2020) 69(6):1148–9. doi: 10.1136/gutjnl-2020-321183
191. Yeoh YK, Zuo T, Lui GC-Y, Zhang F, Liu Q, Li AY, et al. Gut microbiota composition reflects disease severity and dysfunctional immune responses in patients with covid-19. Gut (2021) 70(4):698–706. doi: 10.1136/gutjnl-2020-323020
192. Zuo T, Zhang F, Lui GC, Yeoh YK, Li AY, Zhan H, et al. Alterations in gut microbiota of patients with covid-19 during time of hospitalization. Gastroenterology (2020) 159(3):944–55.e8. doi: 10.1053/j.gastro.2020.05.048
193. Abbate M, Rottoli D, Gianatti A. Covid-19 attacks the kidney: Ultrastructural evidence for the presence of virus in the glomerular epithelium. Nephron (2020) 144(7):341–2. doi: 10.1159/000508430
194. Pan X-W, Xu D, Zhang H, Zhou W, Wang L-H, Cui X-G. Identification of a potential mechanism of acute kidney injury during the covid-19 outbreak: A study based on single-cell transcriptome analysis. Intensive Care Med (2020) 46:1114–6. doi: 10.1007/s00134-020-06026-1
195. Su H, Yang M, Wan C, Yi L-X, Tang F, Zhu H-Y, et al. Renal histopathological analysis of 26 postmortem findings of patients with covid-19 in China. Kidney Int (2020) 98(1):219–27. doi: 10.1016/j.kint.2020.04.003
196. Perico L, Benigni A, Remuzzi G. Should covid-19 concern nephrologists? Why and to what extent? The emerging impasse of angiotensin blockade. Nephron (2020) 144(5):213–21. doi: 10.1159/000507305
197. Zhao J, Pan X, Hao D, Zhao Y, Chen Y, Zhou S, et al. Causal associations of gut microbiota and metabolites on sepsis: A two-sample mendelian randomization study. Front Immunol (2023) 14. doi: 10.3389/fimmu.2023.1190230
198. Song J, Ke B, Tu W, Fang X. Roles of interferon regulatory factor 4 in the aki-ckd transition, glomerular diseases and kidney allograft rejection. Ren Fail (2023) 45(2):2259228. doi: 10.1080/0886022X.2023.2259228
199. Zhang S-Y, Xu Q-P, Shi L-N, Li S-W, Wang W-H, Wang Q-Q, et al. Soluble cd4 effectively prevents excessive tlr activation of resident macrophages in the onset of sepsis. Signal Transduction Targeted Ther (2023) 8(1):236. doi: 10.1038/s41392-023-01438-z
200. Chen X, Chen X, Yang Y, Luo N, Yang J, Zhong L, et al. Protective role of the novel cytokine metrnl/interleukin-41 in host immunity defense during sepsis by promoting macrophage recruitment and modulating treg/th17 immune cell balance. Clin Immunol (2023) 254:109690. doi: 10.1016/j.clim.2023.109690
201. Li C, Liu J, Zhang C, Cao L, Zou F, Zhang Z. Dihydroquercetin (dhq) ameliorates lps-induced acute lung injury by regulating macrophage m2 polarization through irf4/mir-132-3p/fbxw7 axis. Pulm Pharmacol Ther (2023) 83:102249. doi: 10.1016/j.pupt.2023.102249
202. Chen Q, Qin Z, Sun Y, Liu X, Pac Soo A, Chang E, et al. Dexmedetomidine activates akt, stat6 and irf4 modulating cytoprotection and macrophage anti-inflammatory phenotype against acute lung injury in vivo and in vitro. J Inflamm Res (2022) 15:2707–20. doi: 10.2147/JIR.S357012
203. Sasaki K, Terker AS, Pan Y, Li Z, Cao S, Wang Y, et al. Deletion of myeloid interferon regulatory factor 4 (irf4) in mouse model protects against kidney fibrosis after ischemic injury by decreased macrophage recruitment and activation. J Am Soc Nephrology: JASN (2021) 32(5):1037. doi: 10.1681/ASN.2020071010
204. Buchele V, Konein P, Vogler T, Kunert T, Enderle K, Khan H, et al. Th17 cell-mediated colitis is positively regulated by interferon regulatory factor 4 in a t cell-extrinsic manner. Front Immunol (2021) 11:590893. doi: 10.3389/fimmu.2020.590893
205. Mudter J, Amoussina L, Schenk M, Yu J, Brüstle A, Weigmann B, et al. The transcription factor ifn regulatory factor–4 controls experimental colitis in mice via t cell–derived il-6. J Clin Invest (2008) 118(7):2415–26. doi: 10.1172/JCI33227
206. Cornell LD, Smith RN, Colvin RB. Kidney transplantation: Mechanisms of rejection and acceptance. Annu Rev Pathol Mech Dis (2008) 3:189–220. doi: 10.1146/annurev.pathmechdis.3.121806.151508
207. García-Martínez Y, Borriello M, Capolongo G, Ingrosso D, Perna AF. The gut microbiota in kidney transplantation: A target for personalized therapy? Biology (2023) 12(2):163. doi: 10.3390/biology12020163
208. Wang J, Li X, Wu X, Wang Z, Zhang C, Cao G, et al. Gut microbiota alterations associated with antibody-mediated rejection after kidney transplantation. Appl Microbiol Biotechnol (2021) 105:2473–84. doi: 10.1007/s00253-020-11069-x
209. Fricke W, Maddox C, Song Y, Bromberg J. Human microbiota characterization in the course of renal transplantation. Am J Transplant (2014) 14(2):416–27. doi: 10.1111/ajt.12588
210. Schirmer M, Smeekens SP, Vlamakis H, Jaeger M, Oosting M, Franzosa EA, et al. Linking the human gut microbiome to inflammatory cytokine production capacity. Cell (2016) 167(4):1125–36. doi: 10.1016/j.cell.2016.10.020
211. Campbell PM, Humphreys GJ, Summers AM, Konkel JE, Knight CG, Augustine T, et al. Does the microbiome affect the outcome of renal transplantation? Front Cell infect Microbiol (2020) 10:794. doi: 10.3389/fcimb.2020.558644
212. Pereira-Balbino K, de-Paula-Jorge M, Queiroz-Ribeiro A, Stampini-Duarte-Martino H. Modulation of intestinal microbiota, control of nitrogen products and inflammation by pre/probiotics in chronic kidney disease: A systematic review. Nutr Hosp (2018) 35(3):722–30. doi: 10.20960/nh.1642
213. Fang Y, Lee H, Son S, Oh S, Jo S-K, Cho W, et al. Association between consumption of dietary supplements and chronic kidney disease prevalence: Results of the korean nationwide population-based survey. Nutrients (2023) 15(4):822. doi: 10.3390/nu15040822
214. Sumida K, Molnar MZ, Potukuchi PK, Thomas F, Lu JL, Matsushita K, et al. Constipation and incident ckd. J Am Soc Nephrol (2017) 28(4):1248–58. doi: 10.1681/ASN.2016060656
215. Sumida K, Yamagata K, Kovesdy CP. Constipation in ckd. Kidney Int Rep (2020) 5(2):121–34. doi: 10.1016/j.ekir.2019.11.002
216. Yang Y, Ye-ping M, Zhang Z, Pei-lin D, Li P, Wen-ge L. Effects of adding rheum officinale to angiotensin-converting enzyme inhibitors or angiotensin receptor blockers on renal function in patients with chronic renal failure: A meta-analysis of randomized controlled trials. Clin Nephrol (2018) 89(6):445. doi: 10.5414/CN109193
217. Ji C, Lu F, Wu Y, Lu Z, Mo Y, Han L, et al. Rhubarb enema increasing short-chain fatty acids that improves the intestinal barrier disruption in ckd may be related to the regulation of gut dysbiosis. BioMed Res Int (2022) 2022:1896781. doi: 10.1155/2022/1896781
218. Barratt J, Lafayette R, Kristensen J, Stone A, Cattran D, Floege J, et al. Results from part a of the multi-center, double-blind, randomized, placebo-controlled nefigard trial, which evaluated targeted-release formulation of budesonide for the treatment of primary immunoglobulin a nephropathy. Kidney Int (2023) 103(2):391–402. doi: 10.1016/j.kint.2022.09.017
Keywords: chronic kidney disease, gut-lung-kidney axis, intestinal homeostasis, microbiome, immunity
Citation: Liu X, Wang X, Zhang P, Fang Y, Liu Y, Ding Y and Zhang W (2023) Intestinal homeostasis in the gut-lung-kidney axis: a prospective therapeutic target in immune-related chronic kidney diseases. Front. Immunol. 14:1266792. doi: 10.3389/fimmu.2023.1266792
Received: 25 July 2023; Accepted: 17 October 2023;
Published: 01 November 2023.
Edited by:
Laureline Berthelot, Institut National de la Santé et de la Recherche Médicale (INSERM), FranceReviewed by:
Wiwat Chancharoenthana, Mahidol University, ThailandMagdiel Pérez-Cruz, Stanford University, United States
Copyright © 2023 Liu, Wang, Zhang, Fang, Liu, Ding and Zhang. This is an open-access article distributed under the terms of the Creative Commons Attribution License (CC BY). The use, distribution or reproduction in other forums is permitted, provided the original author(s) and the copyright owner(s) are credited and that the original publication in this journal is cited, in accordance with accepted academic practice. No use, distribution or reproduction is permitted which does not comply with these terms.
*Correspondence: Wen Zhang, MzA2NzYyODI3QHFxLmNvbQ==
†These authors have contributed equally to this work and share first authorship