- 1Institute of Cardiovascular Regeneration, Goethe University Frankfurt, Frankfurt, Germany
- 2Department of Cardiology and Angiology, Goethe University Frankfurt, University Hospital, Frankfurt, Germany
- 3German Center for Cardiovascular Research (DZHK), Partner Site Munich Heart Alliance, Munich, Germany
- 4Institute for Cardiovascular Prevention, Institut für Prophylaxe und Epidemiologie der Kreislaufkrankheiten (IPEK), Ludwig-Maximilians-Universität München, Munich, Germany
Blood clotting is a finely regulated process that is essential for hemostasis. However, when dysregulated or spontaneous, it promotes thrombotic disorders. The fact that these are triggered, accompanied and amplified by inflammation is reflected in the term thromboinflammation that includes chemokines. The role of chemokines in thrombosis is therefore illuminated from a cellular perspective, where endothelial cells, platelets, red blood cells, and leukocytes may be both the source and target of chemokines. Chemokine-dependent prothrombotic processes may thereby occur independently of chemokine receptors or be mediated by chemokine receptors, although the binding and activation of classical G protein-coupled receptors and their signaling pathways differ from those of atypical chemokine receptors, which do not function via cell activation and recruitment. Regardless of binding to their receptors, chemokines can induce thrombosis by forming platelet-activating immune complexes with heparin or other polyanions that are pathognomonic for HIT and VITT. In addition, chemokines can bind to NETs and alter their structure. They also change the electrical charge of the cell surface of platelets and interact with coagulation factors, thereby modulating the balance of fibrinolysis and coagulation. Moreover, CXCL12 activates CXCR4 on platelets independently of classical migratory chemokine activity and causes aggregation and thrombosis via the PI3Kβ and Btk signaling pathways. In contrast, typical chemokine-chemokine receptor interactions are involved in the processes that contribute to the adhesiveness of the endothelium in the initial phase of venous thrombosis, where neutrophils and monocytes subsequently accumulate in massive numbers. Later, the reorganization and resolution of a thrombus require coordinated cell migration and invasion of the thrombus, and, as such, indeed, chemokines recruit leukocytes to existing thrombi. Therefore, chemokines contribute in many independent ways to thrombosis.
1 Introduction
Inflammation and thrombosis are closely intertwined which is long known for atherosclerotic cardiovascular disease (1). Insight into this close interplay between the immune and hemostatic systems, coined the term immunothrombosis (2). This term refers to a primarily beneficial mechanism of how the organism can contain a noxious threat, for example, from a microbial invasion by preventing a further spread through the blood. Uncontrolled aberrant immunothrombosis wreaks havoc, causing thromboinflammation (3). In this way, immunothrombosis and thromboinflammation are two sides of the same coin. Among many relevant inflammatory effector molecules are chemokines that play an important role in inflammation, atherosclerosis and thrombosis and link in several aspects the immune and hemostatic system. They are a well-described group of chemotactic mediators of about 50 members. Despite a large sequence dissimilarity, chemokines display a typical molecular fold that resembles a Greek key. A structure held together by two cysteines that follow a flexible N-terminal tail and form bridges to loops around residue positions 30 (30s loop) and 50 (50s loop). The cysteine motif, the namesake of the CC and CXC family, is followed by the N-loop, which transitions into a β-sheet of three β-strands linked to the C-terminal helix. Exceptions are CX3CL1 which is the only chemokine that contains Nt cysteines separated by three amino acids, and like CXCL16, is a priori not soluble but integrated into the plasma membrane.
Most of the chemokines have one or more high-affinity receptors that belong to the 7TM receptor family that typically signal through G-proteins, mostly Gαi, GRKs or independent of G-proteins through arrestins or act as atypical receptors without signaling functioning as chemokine sink.
2 The endothelium and vascular wall are both a source and target of chemokines in thrombosis
The endothelial layer passively protects against thrombosis by separating the blood from the pro-thrombotic components of the underlying vascular wall, such as matrix proteins, or atherosclerotic plaque material including collagens. Denudation or dysfunction by injury, virus-induced endothelitis or plaque rupture exposes these structures (4). Healing requires migration and proliferation of endothelial cells (5). The endothelium can also play an active role in promoting thrombosis by supporting the extrinsic coagulation cascade and the rolling and adhesion of platelets. Although several chemokines and their receptors are expressed by endothelial cells, and their ability to control cell migration and induce cell adhesion is essential for thrombosis, the experimental knowledge about chemokine-driven endothelial-specific processes in thrombosis and healing is relatively limited.
Under steady-state conditions, single-cell RNAseq (tabula muris, tabula sapiens) reveals substantial transcripts of atypical chemokine receptors ACKR1/DARC >ACKR3/CXCR7 > ACKR2/D6 in endothelial cells from multiple organs and vascular beds, followed by classical chemokine receptors CXCR4, CCR10/GPR2 (Table 1). At lower levels, CCR1, CCR2, CCR5 and CX3CR1 are not detectable, which does not exclude a functional presence of other chemokine receptors in endothelial cells. Especially after endothelial activation, de novo transcription and a change in expression pattern will occur (7, 8). This renders endothelial cells susceptible to binding and activation by a large variety of chemokines.
ACKR1/DARC is preferentially expressed under steady-state conditions by vein and capillary endothelial cells (Table 1) but is upregulated under inflammatory conditions and may play a role for arteries through its expression in the vasa vasorum (9, 10). ACKR1 mainly binds inflammatory chemokines of the CC and CXC families, such as CCL2, CXCL1, and CXCL8, and actively transports them from the tissue through the body of an endothelial cell (transcytosis) to present them at the surface (Figure 1) (11, 12). ACKR1 binds also CXCL12 which is regarded as a homeostatic chemokine (13). Recent evidence indicates that ACKR1/DARC preferentially binds dimeric CXCL12 over monomeric CXCL12, presumably modulating CXCL12 activity by scavenging at higher CXCL12 concentrations when dimers dominate (14). Chemokine presentation be endothelial cells could be an important step in initiating thrombosis, in which the accumulation of myeloid cells is a major factor. Conversely, chemokines can be transported from the vascular lumen into the endothelial cell. This is true for CCL5 and CXCL4 (platelet factor 4, PF4), which are rapidly transported intracellularly to the nucleus of venous endothelial cells by active endocytosis that requires GPCR signaling (15). The effects of this process on cell recruitment and endothelial cell function could also influence thrombotic processes, which remains to be shown (15).
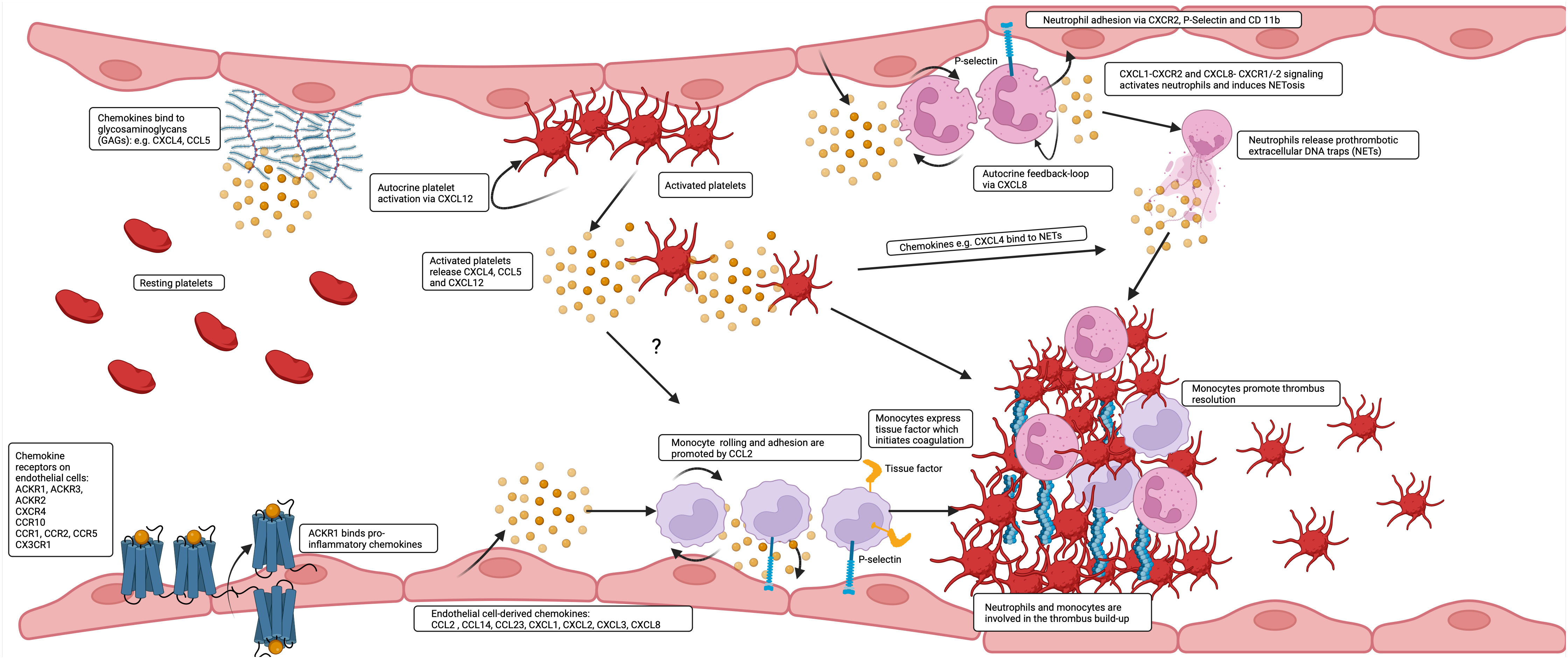
Figure 1 Chemokines are produced by and bind to endothelial cells to affect thrombus build up and resolution. A great variety of chemokines is presented by the endothelium. Inflammatory chemokines are transported and presented by ACKR1 to the luminal surface or are released and deposited by platelets and leukocytes or directly released by endothelial cells and additionally presented by GAGs. CCL2 and CXCL8 contribute in interaction with tissue factor (TF) and P-selectin to the prothrombotic accumulation of monocytes and neutrophils on the endothelium and are at later stages also involved in thrombus resolution.
CXCL1/CXCL2 and CCL2 activate CXCR1/CXCR2 and CCR2, respectively. These chemokines are important for thrombus resolution by organizing monocyte infiltration into the venous thrombus, which results in a reduction in thrombus size and collagen content over the course of three weeks (16, 17). CCL2 is expressed by arterial, venous and capillary endothelial cells (6). The physiological relevance of this expression in the human system is supported by the finding that tissue factor (TF) drives endothelial CCL2 expression, leading to smooth muscle cell migration toward endothelial cells and thus facilitating the maturation of newly formed microvessels (18). In the time course of venous thrombosis simulated in a mouse model, CCL2 levels increase in the vein wall and, to a lesser extent, in the thrombus itself by day seven. Direct injection of CCL2 into a developing ligature-induced thrombus increases the number of monocytes in the vein and decreases the size of the thrombus after one week (19). It is conceivable that systemic administration of CCL2 or local application of chemokine-loaded hydrogels (20) could be beneficial and help close endothelial gaps after injury by promoting CCR2-mediated EC migration. However, an increase in circulating CCL2 could lead to adverse effects due to increased mobilization of monocytes from the bone marrow (21).
ACKR3/CXCR7, a receptor for CXCL12, CXCL11, and also opioid peptides, is expressed by arterial and venous endothelial cells (Table 1). Recently, by using molecular reporters for ACKR3, ACKR4, and GPR182, the expression of these receptors in the endothelial system could be visualized and mapped (22). GPR182 corresponds to an endothelial atypical chemokine receptor that binds CXCL9, CXCL10, CXCL12, and CXCL13 (23, 24). Because ACKR4 and GPR182 are expressed predominantly by sinusoids and in endothelial cells of lymphatic organs, their expression pattern argues against a direct role in arterial and venous thrombosis. Nevertheless, a role in immunothrombosis to contain infection can be envisioned. When CXCR4 and ACKR3 are co-expressed, such as in endothelial cells, they associate to heteromers at the membrane level, and interact via activation of CXCR4 and downstream GPCR kinases (GRK) at the signaling level. The activation of GRK2 by CXCR4 leads to the phosphorylation of ACKR3, which in turn promotes the internalization and degradation of CXCL12 (25). This was shown in HEK transfectants, and it will be important to see how these mechanisms translate into the vascular environment where CXCR4 and ACKR3 are coexpressed by endothelial cells.
The CXCL12-CXCR4 pathway is essential, and the deficiency of either gene results in fatal embryonic vasculature malformations. CXCR4 is expressed by two types of endothelial cells: TIP cells, which are specialized for angiogenesis, and arterial endothelial cells where it protects against atherosclerosis by strengthening VE-cadherin VE-PTP interactions to maintain vessel integrity (26). Endothelium-specific effects of CXCR4 in in vivo thrombosis models have yet to be tested. However, a study has shown that a prevalent CXCL12 gene polymorphism linked to heightened CXCL12 production predisposes patients with chronic myeloproliferative disorders to arterial thrombosis, potentially due to the influence of VEGF (27). A prothrombotic role for the CXCL12/CXCR4/ACKR3 axis has also been proposed for the thrombotic microangiopathy caused by bacterial infections with Shiga toxin-producing E. coli that leads to the hemolytic uremic syndrome (HUS) (28). In this scenario, the exotoxin alters the endothelial phenotype and is implicated in the pathogenesis of microangiopathy in mouse models. The exotoxin stimulates the gene and protein expression of CXCL12, as well as that of its receptors CXCR4 and ACKR3, mimicking the conditions observed in children afflicted with E.coli O157:H7 who further develop HUS where CXCL12 levels are similarly elevated. In the animal model, blocking the CXCL12-CXCR4 pathway with AMD3100 prevents activation of endothelial cells, decreases permeability of endothelium, and enhances survival (28). The impact of the CXCL12-CXCR4 axis on endothelial permeability appears to vary according to the specific type of endothelial cells. In HMVEC and the microvasculature of the kidney and brain, activation of CXCR4 results in increased endothelial permeability, while in arterial endothelial cells of the aorta CXCR4 is crucial for maintaining vascular integrity (26, 29). A weakening of the endothelial barrier will predispose to plaque rupture and arterial thrombosis. In this respect, the increased expression of CXCL12 in unstable atherosclerotic plaques may play a role (30). Moreover, the CXCL12-CXCR4 axis also drives arterial thrombosis by inducing platelet activation (31). Therefore, targeting CXCL12-CXCR4 may prevent and impede thrombosis, although it is crucial to take into account the possible negative impacts on atherogenesis and bone marrow homeostasis. Healing following endothelial injury is a significant predisposing factor for thrombosis. The ability to restore the integrity of the vascular lining is enhanced by factors such as the release of CXCL12 from platelets, apoptotic vascular smooth muscle cells, and the presence of CXCR4 on circulating endothelial progenitor cells. These factors promote EPC recruitment and induce endothelial cell migration (32–34).
Laminar shear stress affects the expression of chemokines and chemokine receptors. For instance, high shear stress leads to a decrease in the expression of the CXCR4 gene and protein in endothelial cells (35). Therefore, investigating endothelial cell-specific knockouts of chemokine receptors in models of arterial thrombosis, venous thrombosis and thrombus resolution are required to understand the role of endothelial chemokine receptors as targets to prevent and treat thromboinflammation.
Under steady-state conditions, arterial, venous and capillary endothelial cells express the transmembrane chemokine CX3CL1 (fractalkine), which is upregulated in endothelial dysfunction. It contributes to the activation of platelets via its receptor CX3CR1 whereas platelet accumulation is mediated via a VWF-GPIb-dependent mechanism, independent its receptor CX3CR1 (36, 37). This mechanism of platelet adhesion has been associated with atherogenesis and likewise may play a role in platelet accumulation in thrombosis (36, 37). Additionally, the accumulation of myeloid cells has been identified as a crucial factor for thrombosis in mouse models, aside from platelet adhesion. One hour after the onset of flow restriction in a mouse model of venous thrombosis induced by blood stasis in the vena cava, monocytes and neutrophils begin to cover the intact endothelial surface. These leukocytes are crucially involved in the build-up and composition of the thrombus (38). Restricting venous flow increased levels of inflammatory molecules including IL-6 and the chemokines CXCL1, CXCL5, and CCL2, leading to inflammatory cell recruitment. The origin and precise role of these chemokines for this process and subsequent steps of thrombus formation and resolution remain to be elucidated (38).
Gene expression of CCL14, CCL23, CXCL1, CXCL2, CXCL3, and CXCL8 is robustly detectable in vascular endothelial cells, reflecting constitutive transcription. In contrast, other chemokine genes such as CCL5 and CXCL10 are barely expressed at rest but inducible by TNF-α activation (39).
It has been shown that chemokine secretion from human endothelial cells (HUVEC) originates from distinct granules. CXCL8 is stored in Weibel-Palade bodies, whereas CCL2, CXCL2 and CCL26 are kept in smaller vesicles released by secretagogues such as histamine, whereas CXCL10 and CCL5 when expressed are not sensitive to secretagogues (40).
Chemokines are avid binders of glycosaminoglycans (GAG), the linear sugar chains of proteoglycans that form a large part of the endothelial glycocalyx (41). GAG of the glycocalyx generate a scaffold and sink for chemokines so that an equilibrium of immobile and soluble chemokines forms a cloud around endothelial cells, which explains why GAG-chemokine interactions are essential for their function. Yet, in many instances, GAG binding competes with receptor binding (42). Chemokines can be released from circulating cells including platelets and deposited on the endothelial surface/glycocalyx. Examples are CCL5 and CXCL4, which are released from activated platelets or by their microparticles and bind to endothelial cells to subsequently activate and recruit leukocytes (43–45). CCL5, immobilized on endothelial cells, forms higher oligomeric structures that appear as strings and are shear flow resistant (46). The binding of chemokines to GAG and proteoglycans can occur not only on the endothelial glycocalyx, but proteoglycans are also constituents of eroded plaques that are substrates for coronary thrombosis (47).
Therefore, a multitude of chemokines is expressed in healthy veins by cells of the vascular wall and the endothelium. Chemokines are upregulated by flow disturbance, be it stasis or shear gradients in the nascent thrombus. Upregulation reflects inflammation and endothelial cell activation, which are the initial steps in deep vein thrombosis and likely also in microvascular thrombosis, where leukocyte adhesion is responsible for the ensuing steps of platelet aggregation, fibrin formation and thrombus build-up (Figure 1).
2.1 Chemokines affect coagulation through interaction with endothelial GAGs, plasma proteins and NETs
Soluble glycosaminoglycans (GAGs) are highly effective and frequently applied anticoagulants in clinical settings. They bind to antithrombotic proteins, including antithrombin (with the shortest effective unit being a pentasaccharide, which is used in fondaparinux) and heparin cofactor II (with the shortest effective unit being a hexasaccharide), inducing conformational changes that multiply their inhibitory effect. Antithrombin deactivates both thrombin and factor Xa when bound with heparin, whereas heparin cofactor II exclusively inhibits thrombin (48). This anticoagulant effect can be reversed by chemokines, which tightly bind heparin. For instance, CXCL4, a chemokine found in high abundance in blood plasma, can serve as an effective neutralizer of heparin (49, 50). Intravenous infusion of 5mg/kg CXCL4 fully reversed the anticoagulant activity of heparin in a small clinical trial in patients after cardiac surgery without any complications (51). Whether other abundant chemokines, such as CXCL7, would also result in heparin neutralization is, to our knowledge, unknown. Fluorescent probes enable the quantification of endogenous circulating GAG without differentiating the species or functional activity (52). In healthy human plasma, the concentration of circulating GAGs is in the range of 60 ng/ml. However, patients with pulmonary embolism, cancer, or liver disease may exhibit GAG levels exceeding 2 µg/ml (53).
2.2 Interactions between CXCL4 and anticoagulant and coagulant proteins
The use of homozygous and heterozygous knockouts as well as overexpressing knock-in mice, which either have a complete absence of CXCL4 or have reduced or increased CXCL4 levels, mimics the in vitro experiments in which a bell-shaped relationship between CXCL4 levels and prothrombotic function was observed (54). Compared with wild-type animals, both mice with increased and decreased CXCL4 expression showed a defect in arterial thrombosis formation (54). Despite full anticoagulation, infusion of heparin paradoxically enhanced thrombus formation in high CXCL4 mice, but potentiated the antithrombotic effect in low CXCL4 mice possibly by generating charge dependent attraction (54).
Another aspect of how CXCL4 affects the hemostatic system is by binding to proteins of the coagulation system that are simultaneously anti-inflammatory and fibrinolytic, such as activated protein C (APC), which can result in opposing effects. In humans, complete deficiency of APC, as in homozygous deficiency, results in microvascular thrombosis and hemorrhagic skin necrosis in infancy, whereas heterozygosity increases the risk of venous thrombosis in adults. Protein C is a vitamin K-dependent glycoprotein that is synthesized by the liver. After activation by thrombomodulin-chondroitinsulfate and its cofactor protein S, APC inactivates coagulation factors Va and VIIIa through proteolysis, resulting in reduced generation of thrombin, inhibition of thrombosis, and increased bleeding risk. CXCL4 has two effects on APC regulation. On one hand, CXCL4 enhances the thrombin/thrombomodulin dependent generation of APC on the endothelial surface and in vitro by interacting with the Gla domain of protein C and with chondroitinsulfate bound to thrombomodulin. This relationship does not follow a linear trend, as indicated by the bell-shaped curve, suggesting an optimal level for CXCL4 (55–57). This can be modulated by the presence of histones where low histone and high CXCL4 levels enhance APC formation and, in these models, high APC levels are associated with improved mouse survival (57). Extracellular histones activate platelets and induce thrombosis through a complement dependent mechanism. Mice deficient in complement component 5 (C5) are protected from histone-induced coagulopathy and lethal thrombosis (58). Platelets lacking C5R1 cannot be activated with C5 and therefore release less CXCL4. This mechanism has been shown to be important for angiogenesis but may also have implications for thrombosis (59).
By contrast, CXCL4 might foster blood clotting by impeding protein S’s augmenting effect on the anticoagulant function of APC through its interaction with APC’s Gla domain (60). Enhancing APC generation while simultaneously blocking its anticoagulant properties only makes sense if APC has multiple functional effects. Indeed, through its Gla domain, APC/Protein C binds to EPCR and PAR-1 on endothelial cells, thereby conferring cytoprotective effects. In this respect, it is noteworthy that CXCL4 did not block these cytoprotective functions of APC (60). It is possible that CXCL4 serves as a natural antagonist, blocking the risk of bleeding inherent to APC without jeopardizing protective functions. This is important under conditions of immunothrombosis/thromboinflammation, induced by activated platelets, when neutrophils expel web-like complexes of DNA, histones, chemokine and other mediators, a process termed NETosis (61, 62). Under these conditions, histones and CXCL4 additively modulate the rate of aPC formation. By inhibiting neutrophil EPCR, PAR3, and MAC-1, APC decreases NETosis. Consequently, in situations that result in platelet activation, this mechanism could serve as a crucial regulatory factor (63).
The role of CXCL4 in thrombosis and hemostasis is important but complex as it is involved in several systems regulating and keeping the balance in thrombosis and hemostasis by a corridor of concentrations that enhance platelet activation, whereas high and low concentrations will prevent this. Monitoring and adjusting blood concentrations would be required to therapeutically set an optimal balance between bleeding and thrombosis. Therefore, CXCL4 appears to be not an easy controllable ideal target.
2.3 CXCL7 variants bind and activate factor X
The other most abundant platelet chemokine CXCL7 (the gene is pro-platelet basic protein, PPBP), is stored as a full-length variant PBP consisting of residues 1-94, which become N-terminal truncated upon release to form CTAPIII (9-94) > β-TG (13-94) > NAP-2 (21-94) (64). The isoforms β-TG and CTAP III but not NAP-2 bind and activate coagulation factor X, thereby bypassing factor VIII and IX to support thrombin generation induced by the contact pathway, which may have relevance for hemophilia A and B (65). This effect was specific for CXCL7 as CXCL4 did not promote thrombin generation.
2.4 VWF forms thrombogenic complexes with CXCL4
Von Willebrand Factor (VWF) strings that form after endothelial injury under flow become decorated with CXCL4 (66). VWF is an anionic multidomain glycoprotein that forms multimeric end-to-end chains (concatemers) to achieve hemostasis after injury, but it can also provoke thrombosis. It is stored in endothelial Weibel-Palade bodies and in the α-granules of platelets, where it is folded into compact rods containing several thousand monomers (67, 68). Upon release, certain flow conditions unleash it into an elongated shape that exposes binding sites and enables it to function as a shear force sensor (67, 69). VWF binds factor VIII through its D modules and its C modules contain RGD motifs that recognize activated GPIIb/IIIa. Collagen binds to the A1 and A3 domains, platelet GP1b to the A1 domain, and the protease ADAMTS13 cleaves the A2 domain. ADAMTS13 regulates thrombosis and hemostasis by diminishing the size of the active VWF multimers and is, therefore, a critical antithrombotic molecule. Deficiency of ADAMTS13 leads to acquired thrombotic thrombocytopenic purpura (TTP). Consequently, it is important to note that CXCL4 binds to the A2 domain of VWF, thereby preventing its degradation (70). Moreover, circulating complexes of VWF and CXCL4 have been identified in patients with thrombocytopenic purpura, suggesting that CXCL4 contributes to microvascular thrombosis by inhibiting ADMTS13 (70). These circulating CXCL4•VWF complexes seem to mimic CXCL4•heparin complexes, as pathogenic antibodies isolated from the blood of HIT patients were also shown to recognize CXCL4•VWF (66).
3 Chemokines and platelets
3.1 Charge-driven effects of chemokines on platelets
CXCL4, along with proplatelet basic protein (PPBP), the full-length form of CXCL7, are signature chemokines of platelets. They are the most abundant circulating chemokines in the blood. With an isoelectric point (pI) of 8.8, mature CXCL4 is positively charged at physiological pH. This is thought to account for the contrasting effects of low and high CXCL4 levels on thrombosis investigated using transgenic mice either overexpressing human CXCL4 or being deficient for CXCL4 (54). CXCL7 is released as a proteolytic variant β-TG, which has an overall neutral charge with a pI of 6.9. The charge model proposes that the distance between platelets varies dependent on the extent of surface deposition of CXCL4. In the case of high or low CXCL4 levels, platelets are charged uniformly positive or negative, resulting in repulsive forces, whereas a moderate deposition level would allow attraction through mixed positive and negative charges (71).
3.2 Chemokine-chemokine receptor-mediated platelet activation
Of the 18 typical chemokine receptors that are coupled to G-proteins, specifically CCR1-10, CXCR1-CXCR6, XCR1, and CX3CR1 and the four chemokine receptors, ACKR1-4, that are considered atypical as they do not signal through G-proteins, RNA sequencing identified transcripts of all of them in human and mouse platelets, some only in traces and some at robust levels (Table 2).
Proteomics of human platelets (Table 2) picked up only CXCR4 and CCR4, the two receptors with the highest mRNA copy numbers suggesting that omics of platelets are unbiased but not completely specific or sensitive (72). Transcripts of CCR4 are missing in mice. Therefore, experiments exploring chemokines individually by functional and antibody-based tests are valuable. Presumably, those receptors with very low transcript copies will have no functional relevant protein expression, but drawing a cut-off line for relevant or irrelevant levels of transcripts is difficult.
Some of these chemokines have been used in platelet activation, aggregation, and signaling experiments to determine their biological function.
In an effort to comprehend the regulation of circulating platelet count, Gewirtz and colleagues searched and uncovered transcripts of CXCR1 and CXCR2 within human platelets and megakaryocytes. These receptors were responsive to CXCL7, which is one of the most highly expressed chemokines in platelets. They indeed found inhibition of megakaryocytopoiesis, suggesting a negative feedback loop through CXCR1 or CXCR2, although so far evidence of platelet activation by functional CXCR1/2 agonists CXCL8 or CXCL7 is elusive (79, 85). Later, Clemetson and colleagues (2000) revealed that CCL3, CCL4, CCL5, CCL11, CCL17, CCL22, and CXCL12 exert effects on aggregation and signaling in washed human platelets. These findings align with the expression of CCR1, CCR3, CCR4, and CXCR4 receptor mRNA or protein detected by the authors (78). Effects of CCL2 on platelet activation were judged ambiguous because CCR2 was not detectable, and the effects of CCL5 were not attributed to CCR5 because of lack of detectable expression and because CCR1 and CCR3 were sufficient to explain the effects (78). To date, CCL2’s effects on platelet activation have been reproduced, inhibited by CCR2 antagonists, and appear to be effective in vivo, as CCL2 knockout mice show mildly impaired hemostasis and arterial thrombosis (81).
CXCR4 is robustly expressed by platelets and megakaryocytes (Table 2). First studies identifying functional CXCR4 expressed by the MK/platelet lineage showed that the CXCL12-CXCR4 axis is a negative regulator of megakaryopoiesis/thrombopoiesis (77). Analyzing platelet function using washed platelets can deteriorate the sensitivity of an assay because methodical differences in washing procedures and analysis buffers can mask the effects of mediators with low potency. At least this is one explanation for why CXCL12-induced aggregation of washed platelets has only been observed as a synergistic increase in platelet response to a coagonist. In contrast, platelet aggregation was observed in platelet-rich plasma or whole blood when CXCL12 was used as the only agent (30, 31, 78, 86–90). In the flow chamber, CXCL12-induced thrombus formation mainly affects the 3D thrombus build-up while initial platelet deposition at the surface is only mildly reduced (31). Platelets are a relevant source of CXCL12, and using CXCR4 inhibitors and platelet-specific CXCL12- and CXCR4 knockout mice has clarified that an endogenous feedback loop exists (91). Solubilized plaque material mimicking atherosclerotic plaque rupture activates platelets that release CXCL12, thereby contributing to arterial thrombosis in a mouse model (31, 87). The activation of platelets through the CXCL12-CXCR4 axis leads to signals in the Gi-, PI3Kβ, BTK-, AKt-, and p38MAPK pathways that result in calcium influx, thromboxane and granule release and activation of GPIIb/IIIa (87, 89, 90). The phosphorylation of BTK after CXCR4 activation and the observation that platelet activation induced by CXCL12 can be eliminated with BTK inhibitors, suggests that BTK acts as a critical signaling molecule downstream of platelet chemokine receptors in addition to the recognized ITAM-coupled platelet immune receptors (31, 92, 93).
Beyond the platelet-dependent role of CXCL12 in atherothrombosis, platelets and likely also CXCL12 play an essential role in venous thrombosis (94). At the translational level, CXCL12 activity is regulated by microRNAs (miRs) and posttranslational by proteolytic processing with impact on venous thrombosis. Several circulating miRs including miR 103a-3p have been described to be biomarkers in patients with VTE. Circulating levels of miRNA 103a-3p are decreased in individuals with deep vein thrombosis, and CXCL12 is a target of miRNA 103a-3p, which may explain the observed increase in plasma CXCL12 levels in these patients (95). Appropriately, the overexpression of miR 103a-3p resulted in a reduction of CXCL12 protein levels in the bloodstream and inhibited venous thrombosis in mice, thus providing further support of the concept (95).
CXCL12 exists in various forms of different lengths. Truncation of the first two N-terminal residues leads to CXCL12[24-88], which exhibits unique binding properties and reduced activation of CXCR4. Additional proteolytic processing renders CXCL12 inactive or transforms it into an antagonist (96). Dipeptidylpeptidase 4 (DPP4) is a widely distributed enzyme found on both epithelial and endothelial cells. It cleaves after the first two aminoterminal residues in the consensus sequence of CXCL12 (KP-VSL…), resulting in the production of CXCL12[24-88] but also cleaves and deactivates the incretin GLP-1 (96, 97). Therefore DPP4-inhibitors are commonly used drugs in type 2 diabetics. Blocking DPP4 may potentially increase active CXCL12 levels and augment thrombus formation, as suggested by retrospective data from the WHO spontaneous reporting database. However, this is speculative and not confirmed by a later meta-analysis that analyzed 5 large cardiovascular outcome trials and did not find any signal regarding DPP4-associated VTE (98, 99).
CXCL12 (SDF) is the only typical chemokine that binds to and activates CXCR4. In comparison, the atypical chemokine MIF is another non-cognate ligand displaying partial agonism, and CXCL14 is an additional ambiguous candidate, indicated to synergize with CXCL12 or potentially manifest effects by directly binding CXCR4 (100–104). In contrast to classical chemokines, MIF lacks an N-terminal cysteine motif, though the structure of the MIF monomer bears a resemblance to that of a CXCL8 dimer. MIF is stored and produced by platelets, but the kinetics of secretion are slower than those of chemokines (105). Although MIF can bind to chemokine receptor complexes on platelets, it does not activate platelets. Rather, it keeps them in a resting state and attenuates in vitro thrombus formation at high shear flow on a collagen surface (83). In humans, MIF interacts with CXCL4L1 (also known as PF4V1 or PF4alt), a non-allelic variant of CXCL4, to produce prothrombotic and proinflammatory MIF-CXCL4L1 heterocomplexes. This mechanism is absent in mice, which lack the orthologue for CXCL4L1 (PF4V1) (106).
Furthermore, inhibition of CXCL12-induced platelet activation has been shown by the presence of CCL5, which can also be released by platelets (107, 108). CCL5 inhibits CXCL12 in a non-competitive manner (107), likely due to formation of a heterodimer (109). The carboxyterminal α-helix of CCL5 has been identified as an important region for this complex formation, and the generation of a bundled peptide of this sequence results in a peptide compound that binds to CXCL12 and CXCR4 at the same time (Figure 2), thereby partially preventing CXCR4-activation (31).
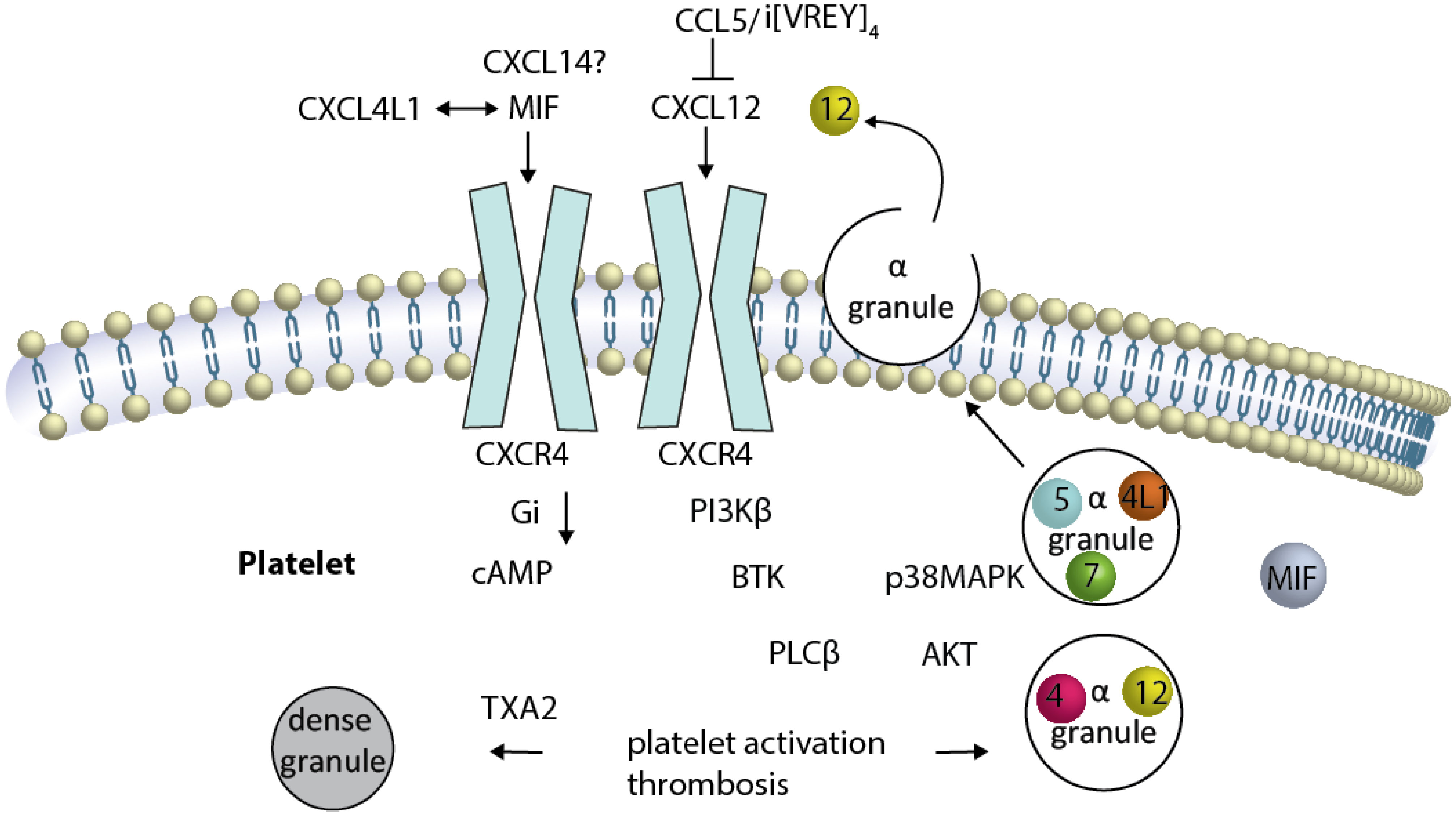
Figure 2 Platelet CXCR4 is a driver of platelet activation, and thrombosis CXCR4 is illustrated as a dimer (although this has not been formally shown in platelets). Activation of and Gαi-coupled CXCR4 by CXCL12 induces reduction of elevated cAMP. Several signaling pathways have been described to be activated either separately or by crosstalk of the signaling components, resulting in platelet activation and thrombosis. The sequence of events leading from CXCR4 activation to BTK-phosphorylation is unknown. CXCL12-induced platelet aggregation can be blunted by the inhibition of PI3K, BTK … TXA2. The release of alpha granules containing CXCL12, CXCL4, CCL5, CXCL7 (spheres) and other chemokines (not MIF), as well as the TXA2-mediated release of dense granules, results in platelet activation loops. The newly released CXCL12 activates CXCR4 in a positive-forward loop.
High levels of CXCL12 and CXCR4 on the surface of platelets are biomarkers that predict increased cardiovascular mortality due to arterial thrombosis. However, the functional significance remains unclear. It is possible that these biomarkers reflect a prothrombotic state resulting from increased platelet reactivity (110, 111).
Endowed with a platelet inhibiting function, ACKR3 (CXCR7) has been stained on the surface of platelets as a receptor for MIF, CXCL12, and CXCL11 and interacts with CXCR4 forming heterocomplexes (112–114). Stimulation of platelets with CXCL12 leads to internalization of CXCR4 but increased surface exposure of ACKR3 through crosstalk with CXCR4 (82). Although CXCR7 does not affect Gαi-signaling directly, interacting with CXCR4 inhibits CXCR4-signaling through Gαi (113). Whether and how CXCR4/CXCR7 assemble into heteromeric complexes in primary cells and platelets has yet to be established.
Finally, the CXCL16-CXCR6 axis has been described as instrumental for platelet activation in humans and mice but requires a co-agonist like ADP (84, 115). Platelet-CXCL16 levels are an independent predictor for all-cause of mortality in patients with stable and unstable CAD (116).
3.3 Platelet thrombi direct leukocytes into the vessel wall using CXCL7 gradients
Platelet-derived chemokines have the potential to establish gradients within platelet thrombi, with elevated concentrations bordering the vascular wall and lower concentrations on the luminal side (117). Neutrophil-platelet thrombus interactions were investigated using a CXCL7 knockout mouse model. The results indicated that neutrophils can bind to, invade, and transmigrate through platelet thrombi in a CXCL7-dependent manner (117). Whether this constitutes a regulatory aspect of a healing process or a harmful tissue inflammation and its effect on thrombus size and resolution are yet to be determined.
4 Leukocyte subsets of the innate and adaptive immune system are recruited to early thrombosis and affect thromboinflammation by complex formation in NETs, HIT and VITT
CXCL4 and, to a lesser extent, other chemokines (e.g., CXCL8) serve as antigens for pathogenic antibodies released by a limited number of B cell/plasma cell clones. This leads to the formation of immune complexes that activate platelets and occlusive platelet thrombi that can form in various apparently healthy vascular beds such as splanchnic veins, sinus veins and arteries (118–120). Entities of immune complex thrombosis comprise HIT which necessitates heparin-chemokine complexes, autoimmune HIT without therapeutic heparin as a trigger, and VITT. The latter came into view at the beginning of the COVID-19 vaccination campaign with adenovirus vaccines. The biological activity of the chemokine appears to be irrelevant in these thrombotic entities. The chemokine serves as a structural link between the antibodies and another component. In the case of HIT, this component may be heparin, while in the case of aHIT and VITT, it may be an unknown polyanionic molecule, such as non-heparin GAG, DNA, polyphosphate, or VWF. Presumably, the exceptionally high blood concentration, the tendency to form tetramers, and a very high affinity for heparin or another polyanion are the conditions that set CXCL4 above other chemokines in this regard. HIT, aHIT, and VITT may have varying polyanionic components that trigger the immune response, but the signaling pathways are highly similar. For the mechanism of platelet activation, the formation of antibody complexes is essential because the receptors that recognize these antibodies on platelets, particularly FcγRIIa (CD32A), require clustering to activate and initiate downstream signaling through the PI3K, BTK, and PLC. In this respect, it is noteworthy and therapeutically relevant that Btk inhibitors demonstrated encouraging outcomes in averting platelet activation through HIT and VITT sera (121–123). For more information on the pathogenesis, diagnostic methods, and therapeutic approaches to immune complex thrombosis, please refer to published reviews (124–126).
Leukocytes, particularly neutrophils, participate in immunothrombosis by releasing extracellular DNA traps (in the case of neutrophils termed NETs, NETosis) a tangle of DNA, histones citrullinated by PAD4, and antimicrobial granule proteins (Figure 1) (62). NETs are detectable in human ischemic stroke thrombi and in human coronaries and their presence has prognostic impact (127, 128). Blocking neutrophil rolling and adhesion during flow restriction in the vena cava has been demonstrated to decrease thrombosis rates and sizes by examining different leukocyte recruitment receptor knockouts. CXCR2, PSGL1, and the myeloid integrin CD11b (αMβ2), but not L-selectin knockouts, exhibit partial protection against venous thrombosis (129). See Table 3 for chemokine receptor expression in monocytes and neutrophils. Neutrophil rolling, facilitated by the P-selectin-PSGL-1-axis, is an essential initial step towards adhesion. During thrombosis, CXCL1-CXCR2 signaling and signals transmitted by the interaction of P-selectin-PSGL-1 not only cooperatively induce adhesion but also NETosis. The combination of both activation pathways can further enhance this process (129).
Activated platelets and several potent inflammatory mediators in sepsis and at high concentrations CXCL8 (IL-8) can activate neutrophils to release NETs (61, 130, 131). In COVID-19, the CXCL8-CXCR1/-2 axis plays an important role through an autocrine forward loop when neutrophils are simultaneously mobilized and activated by CXCL8 and contribute to microvascular thrombosis of the pulmonary vasculature by NETosis (132). This is an example of how NETs arise in immunothrombosis as a defense mechanism in infections and sepsis to protect the organism by trapping infectious pathogens (2). By incorporating red blood cells, NETs contribute to the formation of red thrombi and are major drivers of deep vein thrombosis. NETs are found in HIT, also in VITT models, and arterial thrombosis (38, 62, 133, 134). Therefore, they are important for sterile thromboinflammation in all vascular beds. Chemokines are at play because they bind to DNA and are found in NETs. Platelet chemokines, which are released during thrombosis, rapidly come into contact with NETs. CXCL4 specifically binds to cell-free DNA under flow and is integrated into NETs, making them more compact and resistant to nuclease degradation (133). CXCL4 organizes double-stranded self-DNA and bacterial DNA into liquid-crystalline supramolecular complexes (135). This is directly relevant to NETosis, thereby depleting free bacteria and improving outcome in murine models of sepsis (136). However, at the same time, this effect may enhance an ongoing thrombotic process as well. DNA•CXCL4 complexes facilitate cellular internalization, TLR9 binding, activation, and IFN-α release and therefore are thought to be more immunogenic and might brake tolerance to self-DNA, which has, in turn, implications for immunological thromboses (HIT, VITT) (135). This principle was initially demonstrated for CXCL4 and applies to some other chemokines as well. Possibly the heteromer formation of CCL5•CXCL4 also plays a role because inhibition of CCL5•CXCL4 preserves heart function after myocardial infarction by attenuating leukocyte recruitment and NETosis (137).
In addition to CXCL4, CXCL10, CXCL12 and CCL5 amplify TLR9-IFN-I responses that require their binding to DNA. Not all chemokines bind DNA. For instance CXCL7/Nap-2, CXCL9 and other chemokines have no IFN-I inducing effect, which excludes unspecific charge driven chemokine•DNA effects (138). In addition, and similar to CXCL4, CXCL10 binds bacterial DNA and enhances an adjuvant immune response mouse wound injury (139). CXCL10 and CXCL8 levels in the blood of septic patients and CXCL2 in mouse models (no CXCL8 gene in mice) correlate positively with septic NET formation, and simultaneous blockade of CXCR1 and CXCR2 with the allosteric inhibitor reparixin prevent NET formation in vitro and in vivo and improved survival in sepsis models (140). However, binding studies of these other chemokine DNA complexes, except for CXCL10, do not yet exist so that detailed structural insights on the epitopes and contact sites are missing. That heparin partly inhibits the release of interferon by several chemokines complexed with DNA implies that there are common binding sites that overlap (138).
Besides neutrophils, monocytes cover dysfunctional prothrombotic endothelium at the early stage of venous thrombosis and expose tissue factor (TF), a transmembrane receptor initiating the extrinsic pathway of coagulation (Figure 1) (38). Typically, TF is selectively expressed by perivascular and epithelial cells. However, monocytes are able to transiently express TF, when induced by LPS-mediated TLR activation or after surgery (141). Preferentially mouse Ly6Chigh, inflammatory, monocytes seem to express tissue factor (TF), at least after LPS treatment (142). They express highly CCR2. In addition, other leukocyte subsets, including dendritic cells, plasmacytoid dendritic cells, and NK cells, as well as inflamed endothelial cells, express CCR2 (143). Knocking out CCR2 results in sequestration of monocytes in the bone marrow and severe reduction in circulating monocyte count (143, 144). Reducing circulating monocytes through various methods, such as anti-CCR2, anti-CCL2, clodronate depletion, CCR2 knockout, or converting inflammatory Ly6Chigh monocytes to Ly6Clow monocytes using the Nur77 agonist Cytosporone B (Csnp), leads to slower thrombus growth and faster resolution (142, 145). Reducing monocytes to limit thrombosis and thrombus resolution seems counterintuitive since CCL2 promotes thrombus-invading monocytes to accelerate thrombolysis (16, 146). However, the CsnB-application after four days of thrombus growth showed indeed faster resolution of the venous thrombus (142). One speculative explanation would be that not inflammatory but rather patrolling Ly6Clow monocytes are the active force behind thrombus resolution or off-target effects of Csnp could be accountable.
The expression of CCR2 and CCL2 in monocytes and endothelial cells are both regulated by the vitamin K-dependent, Gla-domain containing plasmatic factor Gas6 which is the ligand for the myeloid receptor tyrosine kinases MerTK. Gas6 knockout leads to decreased expression of both the CCL2 chemokine and its corresponding receptor, CCR2. In venous thrombosis induced either in the vena cava through flow restriction or by FeCl3 injury, Gas6 deficiency results in smaller thrombi containing fewer monocytes but normal levels of neutrophils. This suggests that Gas6 specifically promotes the recruitment of inflammatory monocytes by regulating both CCR2 and CCL2 (145).
Chemokines promote venous thrombosis by recruiting monocytes that contain tissue factor via the CCL2-CCR2 pathway. Simultaneously, they recruit and activate neutrophils that produce (NETs) via the CXCL1-CXCR2 axis. Targeting leukocyte adhesion by blocking rolling or the conversion from rolling to adhesion by antibodies against P-selectin or PSGL1 by blocking chemokines or their receptors or blocking NETosis are conceivable strategies in prevention and treatment of thrombosis. Advantages of anti-inflammatory therapeutics over anticoagulants could be that the sequelae of thrombosis would be targeted, i.e. damaged valves and the postthrombotic syndrome could benefit from an anti-recruitment therapy. Possible downsides are that inflammation is supposed to be a regulatory requirement for proper healing and hemostasis. It is yet unclear to what extent anti-inflammatory treatment is safe or if the intimate relationship between clotting and inflammation is a weakness in the system and a gateway to bleeding. Chemokines can also contribute to NETosis independently of any chemokine receptor by binding to DNA in NETs and affecting their structure. This process appears to be specific to a limited number of chemokines, despite the lack of systematic profiling regarding their affinity and the ambiguous nature of their binding sites. Other questions need to be answered in the future, too. Are chemokines that are entangled in NETs still able to bind to their receptors and functionally active? What occurs to the oligomeric nature of chemokines in these structures? Are chemokines present in NETs engaging in competition with other DNA-binding proteins, such as histones and myeloperoxidase (MPO), and subsequently releasing them?
Chemokines and their receptors are potential targets in NETosis and, consequently, in immunothrombosis and thromboinflammation (Figure 1). However, inhibiting NETosis may have both positive and negative effects, and the timing and balance between inflammatory and thrombotic conditions are crucial for the success of any inhibitory therapy. Chemokines are effectors of mutual interactions of platelets, leukocytes and endothelial thereby driving thromboinflammation and immunothrombosis (Figure 3).
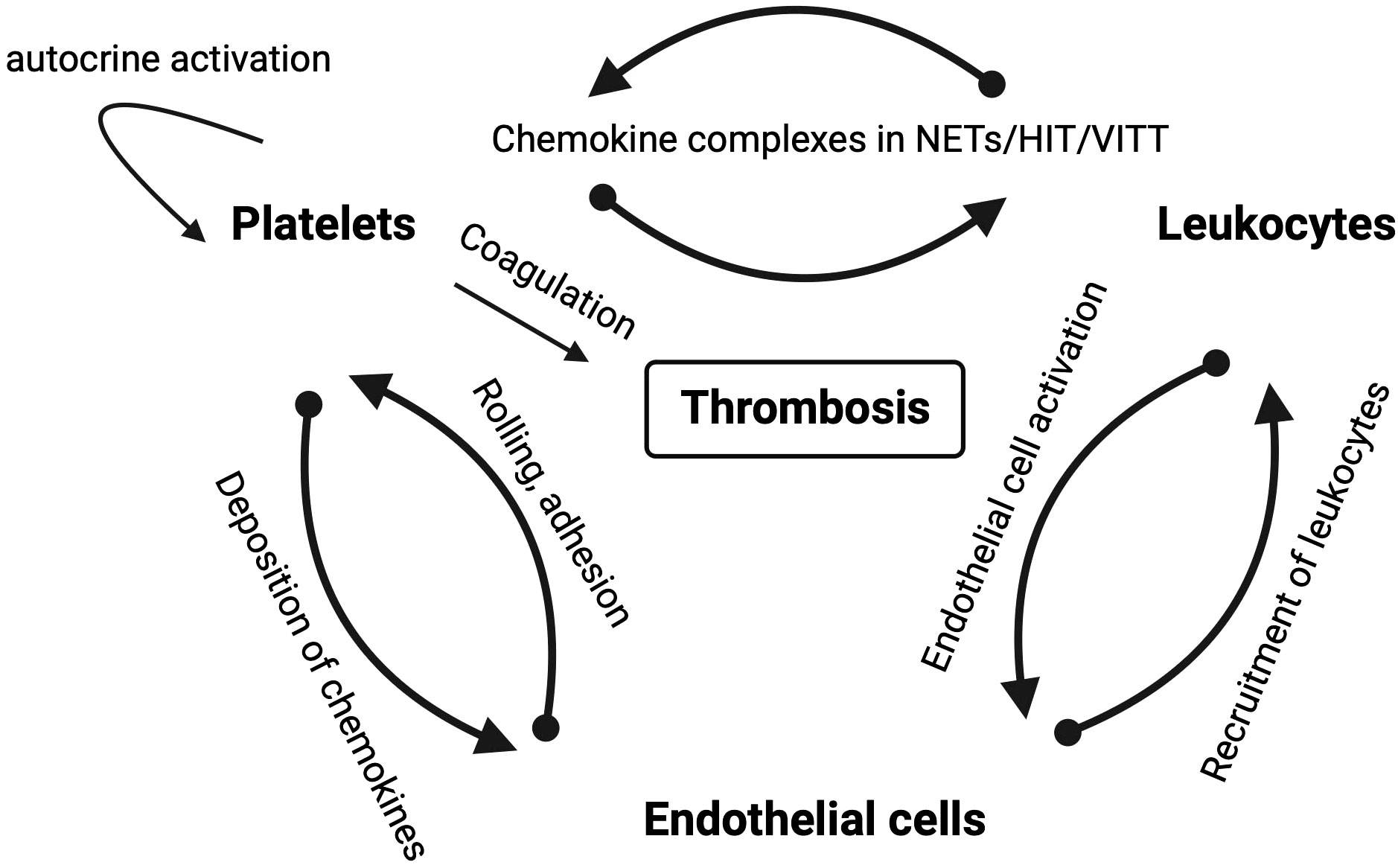
Figure 3 Schematic overview of mutual interactions of platelets, leukocytes and endothelial cells in thrombotic diseases in which chemokines play a role. Arrows depict the direction of the interaction labeled with the mode of interaction.
5 Chemokines, erythrocytes and ACKR1 in venous and arterial thrombosis
Traditionally, arterial thrombosis has been described as predominantly platelet-dependent due to the platelet-rich and erythrocyte-poor white morphology of thrombi. In contrast, venous thrombi show the opposite pattern and are characterized as red with low platelet and high erythrocyte content. Clinical data justify this concept because antiplatelet agents are proven superior over anticoagulation in preventing arterial thrombosis in myocardial infarction and atherosclerotic stroke. Thrombosis in the venous system, including deep vein thrombosis and atrial and auricular thrombus formation, can be treated and prevented more effectively with anticoagulants than with antiplatelet therapies. While this picture of arterial white platelet and red venous thrombi may hold some truth, it is known to be at least too simplistic and in angioscopic studies, coronary thrombi can be red or white with STEMI thrombi being more often red and those associated with unstable angina being more often white and antiplatelets have some efficacy in venous thrombosis (147, 148). Red blood cells in arterial thrombosis deserve attention. Their role has been demonstrated in FeCl3-induced endothelial injury, where the initial response came not from platelets, but from RBCs (149, 150).
Many chemokines and atypical chemokines, including MIF, may not be synthesized by RBCs or their precursors. Yet, they are impressively present at the protein level and represent a large reservoir in the blood (151, 152). ACKR1 plays an essential role in this respect. In addition to endothelial cells, ACKR1 is expressed at high levels by erythrocytes, except in a majority of West Africans or African Americans who carry a genetic polymorphism in the GATA-1 binding site that prevents ACKR1 expression on erythrocytes. ACKR1 binds to about 20 proinflammatory chemokines of the CC- and CXC-family such as CCL2, CCL5, CXCL5, and CXCL8 but not CXCL4, CXCL10 and CXCL12 (152–154). ACKR1 (gene Fy) possesses a common single nucleotide polymorphism in the extracellular N-terminal domain, which is important for chemokine binding. The major allele (FYB) translates at position 42 into aspartate, which is exchanged in the minor allele to a glycine (FYA). A GWAS study comparing several large cohorts found a strong association of this polymorphism with serum concentration of CCL2 and, to a lesser extent, with CCL5 and CXCL8 but not with CXCL10 that does not bind ACKR1. Notably, this observation was only accurate under coagulation conditions, as the correlation was solely observable in serum and not in plasma (155). This indicates that individuals who carry the FYB gene release higher levels of inflammatory chemokines while clotting due to an unknown factor, which may impact the development and resolution of thrombosis.
6 Conclusions
Inflammation and thrombosis are inextricably linked, and chemokines and their receptors are key regulators involved in several aspects of thromboinflammation and immunothrombosis by affecting circulating leukocyte numbers, leukocyte activation and recruitment during all stages of thrombosis and also by directly activating platelets and modulating the coagulation system. Although arterial thrombosis, as in HIT, as opposed to plaque rupture and atherothrombosis, microvascular thrombosis and venous thrombosis have different origins, there are many similarities in the progression of thrombosis. For example, shear gradients, NETosis, leukocyte recruitment, the contribution of red blood cells, activated endothelial cells, and activated platelets are relevant in each of these cases. However, we know that although anticoagulation and antiplatelet therapy (APT) have some efficacy in both arterial and venous thrombosis, anticoagulation is clearly preferred for venous thrombotic disease and APT for atherothrombosis whereas the treatment of immunocomplex thromboses is less standardized and depends on whether heparin is part of the pathology or not. Using the chemokine system as a target holds some promise but it needs to be tailored. Moreover, the knowledge we have gained is mostly from animal models of thrombosis and usually a comparison of thrombosis in distinct vascular beds and types of thrombosis is not available and a longer-term use and broader evaluation of effects on health are difficult to predict.
Most human chemokines also exist in mice and can bind and activate mouse chemokine receptors. However, transferability is not universally applicable, and some species differences have been identified. Differences in gene duplication may lead to different expression of CCL19, CCL22, and CCL27, which are the CCR7-ligands, between mice and humans. This disparity may complicate comparisons between the murine and human systems (156, 157). Furthermore, mice do not possess orthologs for human CXCL4L1, CXCL6, and CXCL8. Human CXCL1 and CXCL8 activate mouse CXCR1 upon binding, prompting the use of human chemokines for in vivo assays (158, 159). Another instance of interspecies variation lies in specific differences at the cellular level, exemplified by CCR4 (Table 2). While human platelets express this element, their mouse counterparts do not. Therefore, platelet activation via CCR4 by CCL17 should be operable only in humans. Mouse models are essential for investigating thrombosis in vivo, but the transferability of findings to the human system must be independently determined. In the translational process, clinical trials can provide valuable insight into the safety of utilizing chemokine therapeutics in other diseases. For instance, studies have investigated the prevention of diabetic nephropathy progression through the blocking of monocyte recruitment with small molecules, such as a specific CCR2 or dual CCR2/CCR5 antagonist (160, 161). Applying these antagonists to human subjects for either twelve or fifty-two weeks appears to be safe, with a notable decrease in circulating monocytes and a concomitant increase in CCL2 blood concentrations.
Targeting chemokines or their receptors may have an impact on circulating leukocyte numbers and potentially disrupt leukocyte homeostasis. Mobilization of leukocytes from the bone marrow is a finely tuned and temporally phased process that is amplified by inflammation as a trigger. The main cytokine axis in granulopoiesis is G-CSF/G-CSFR, which interacts with CXCR4 and CXCR2 to control the release of neutrophils from the bone marrow. The bone marrow CXCL12/CXCR4 axis restricts neutrophil mobilization. G-CSF downregulates the expression of CXCR4 on neutrophils and CXCL12 in bone marrow, which disrupts neutrophil retention (162). Similarly, certain CXCR4 antagonists result in neutrophil release whereas others such as the biased antagonist peptide i[VREY]4 do not (31). In contrast, the expression of CXCR2 by neutrophils leads to a rapid release from the bone marrow when CXCR2 ligands (CXCL8, CXCL1, CXCL2) are upregulated. Unexpectedly, G-CSF blocks CXCR2-signaling and regulates neutrophil mobilization negatively (163). Therefore, the cytokine G-CSF has opposing roles on neutrophil release depending on the phase by affecting more than on chemokine pathway. Monocytes are mobilized from the bone marrow into the circulation by CCL2 and CCL3 through monocytic CCR2 (164). Increased monocyte counts in the peripheral blood correlate with venous thrombosis in the mouse (142). It is unclear whether neutrophils will pave the way for monocytes or both accumulate simultaneously. As these leukocyte subsets express several chemokine receptors (Table 3) it could be necessary to block multiple receptors to achieve a meaningful effects. Blocking CXCL12 activity through small molecules inhibits CXCR4 completely, which inhibits platelet aggregation and arterial thrombosis, yet leads to leukocytosis whereas using biased or partial antagonists affect signaling differentially to preserve homeostatic functions and do not result in leukocytosis. When dealing with chemokine signaling, it is crucial to comprehend the points at which the distinct pathways diverge in order to target specific prothrombotic pathways for inhibition. Heparins are perhaps the oldest antithrombotics and chemokine scavengers that neutralize chemokine activity. However, due their unselective binding to virtual all chemokines and many other proteins their anti-chemokine role in thrombosis is difficult to dissect and engineering specific and orally available GAGs that do not affect coagulation will be difficult. Similarly, evasin peptides, which are multi-chemokine binders produced by ticks, have not yet undergone assessment for their potential role in thrombosis (165). It needs to be determined how targeting chemokines in thrombotic diseases will integrate into the current therapeutic strategies. Applying them earlier may have a greater impact on thrombosis, while resolution of the thrombus may be hindered at later timepoints. Potential adverse effects of effective chemokine inhibition may comprise bleeding and dysregulation of the immune system. Nevertheless, bleeding has not been described in any animal models utilizing anti-chemokine strategies. If combined with anticoagulants or antiplatelet therapy, however, there may be a collaborative antihemostatic effect.
Author contributions
PvH: Funding acquisition, Supervision, Visualization, Writing – original draft, Writing – review and editing. JL: Visualization, Writing – review and editing.
Funding
The author(s) declare financial support was received for the research, authorship, and/or publication of this article. The research highlighted herein was supported by the Deutsche Forschungsgemeinschaft SFB1123-A2 to PvH.
Acknowledgments
Figures were created with BioRender.com.
Conflict of interest
The authors declare that the research was conducted in the absence of any commercial or financial relationships that could be construed as a potential conflict of interest.
The author(s) declared that they were an editorial board member of Frontiers, at the time of submission. This had no impact on the peer review process and the final decision.
Publisher’s note
All claims expressed in this article are solely those of the authors and do not necessarily represent those of their affiliated organizations, or those of the publisher, the editors and the reviewers. Any product that may be evaluated in this article, or claim that may be made by its manufacturer, is not guaranteed or endorsed by the publisher.
References
1. Croce K, Libby P. Intertwining of thrombosis and inflammation in atherosclerosis. Curr Opin Hematol (2007) 14:55–61. doi: 10.1097/00062752-200701000-00011
2. Engelmann B, Massberg S. Thrombosis as an intravascular effector of innate immunity. Nat Rev Immunol (2013) 13:34–45. doi: 10.1038/nri3345
3. Stark K, Massberg S. Interplay between inflammation and thrombosis in cardiovascular pathology. Nat Rev Cardiol (2021) 18:666–82. doi: 10.1038/s41569-021-00552-1
4. Ackermann M, Verleden SE, Kuehnel M, Haverich A, Welte T, Laenger F, et al. Pulmonary vascular endothelialitis, thrombosis, and angiogenesis in Covid-19. N Engl J Med (2020) 383:120–8. doi: 10.1056/NEJMoa2015432
5. Michaelis UR. Mechanisms of endothelial cell migration. Cell Mol Life Sci (2014) 71:4131–48. doi: 10.1007/s00018-014-1678-0
6. Tabula Sapiens C, Jones RC, Karkanias J, Krasnow MA, Pisco AO, Quake SR, et al. The Tabula Sapiens: A multiple-organ, single-cell transcriptomic atlas of humans. Science (2022) 376:eabl4896. doi: 10.1126/science.abl4896
7. Gillitzer R, Goebeler M. Chemokines in cutaneous wound healing. J Leukoc Biol (2001) 69:513–21. doi: 10.1189/jlb.69.4.513
8. Rodrigues M, Kosaric N, Bonham CA and Gurtner GC. Wound healing: a cellular perspective. Physiol Rev (2019) 99:665–706. doi: 10.1152/physrev.00067.2017
9. Gencer S, van der Vorst EPC, Aslani M, Weber C, Doring Y and Duchene J. Atypical chemokine receptors in cardiovascular disease. Thromb Haemost (2019) 119:534–41. doi: 10.1055/s-0038-1676988
10. Thiriot A, Perdomo C, Cheng G, Novitzky-Basso I, McArdle S, Kishimoto JK, et al. Differential DARC/ACKR1 expression distinguishes venular from non-venular endothelial cells in murine tissues. BMC Biol (2017) 15:45. doi: 10.1186/s12915-017-0381-7
11. Pruenster M, Mudde L, Bombosi P, Dimitrova S, Zsak M, Middleton J, et al. The Duffy antigen receptor for chemokines transports chemokines and supports their promigratory activity. Nat Immunol (2009) 10:101–8. doi: 10.1038/ni.1675
12. Middleton J, Neil S, Wintle J, Clark-Lewis I, Moore H, Lam C, et al. Transcytosis and surface presentation of IL-8 by venular endothelial cells. Cell (1997) 91:385–95. doi: 10.1016/s0092-8674(00)80422-5
13. Cambier S, Gouwy M and Proost P. The chemokines CXCL8 and CXCL12: molecular and functional properties, role in disease and efforts towards pharmacological intervention. Cell Mol Immunol (2023) 20:217–51. doi: 10.1038/s41423-023-00974-6
14. Gutjahr JC, Crawford KS, Jensen DR, Naik P, Peterson FC, Samson GPB, et al. The dimeric form of CXCL12 binds to atypical chemokine receptor 1. Sci Signal (2021) 14:eabc9012. doi: 10.1126/scisignal.abc9012
15. Dickhout A, Kaczor DM, Heinzmann ACA, Brouns SLN, Heemskerk JWM, van Zandvoort M, et al. Rapid internalization and nuclear translocation of CCL5 and CXCL4 in endothelial cells. Int J Mol Sci (2021) 22:1–16. doi: 10.3390/ijms22147332
16. Henke PK, Pearce CG, Moaveni DM, Moore AJ, Lynch EM, Longo C, et al. Targeted deletion of CCR2 impairs deep vein thombosis resolution in a mouse model. J Immunol (2006) 177:3388–97. doi: 10.4049/jimmunol.177.5.3388
17. Henke PK, Wakefield T. Thrombus resolution and vein wall injury: dependence on chemokines and leukocytes. Thromb Res (2009) 123Suppl 4:S72–8. doi: 10.1016/s0049-3848(09)70148-3
18. Arderiu G, Pena E, Aledo R, Juan-Babot O, Badimon L. Tissue factor regulates microvessel formation and stabilization by induction of chemokine (C-C motif) ligand 2 expression. Arterioscler Thromb Vasc Biol (2011) 31:2607–15. doi: 10.1161/ATVBAHA.111.233536
19. Humphries J, McGuinness CL, Smith A, Waltham M, Poston R and Burnand KG. Monocyte chemotactic protein-1 (MCP-1) accelerates the organization and resolution of venous thrombi. J Vasc Surg (1999) 30:894–9. doi: 10.1016/s0741-5214(99)70014-5
20. Projahn D, Simsekyilmaz S, Singh S, Kanzler I, Kramp BK, Langer M, et al. Controlled intramyocardial release of engineered chemokines by biodegradable hydrogels as a treatment approach of myocardial infarction. J Cell Mol Med (2014) 18:790–800. doi: 10.1111/jcmm.12225
21. Weber KS, Nelson PJ, Grone HJ and Weber C. Expression of CCR2 by endothelial cells : implications for MCP-1 mediated wound injury repair and in vivo inflammatory activation of endothelium. Arterioscler Thromb Vasc Biol (1999) 19(9):2085–93. doi: 10.1161/01.atv.19.9.2085
22. Melgrati S, Radice E, Ameti R, Hub E, Thelen S, Pelczar P, et al. Atlas of the anatomical localization of atypical chemokine receptors in healthy mice. PloS Biol (2023) 21:e3002111. doi: 10.1371/journal.pbio.3002111
23. Melgrati S, Gerken OJ, Artinger M, Radice E, Szpakowska M, Chevigné A, et al. GPR182 is a broadly scavenging atypical chemokine receptor influencing T-independent immunity. Front Immunol (2023) 14:1242531. doi: 10.3389/fimmu.2023.1242531
24. Le Mercier A, Bonnavion R, Yu W, Alnouri MW, Ramas S, Zhang Y, et al. GPR182 is an endothelium-specific atypical chemokine receptor that maintains hematopoietic stem cell homeostasis. Proc Natl Acad Sci USA (2021) 118:e2021596118. doi: 10.1073/pnas.2021596118
25. Schafer CT, Chen Q, Tesmer JJG and Handel TM. Atypical chemokine receptor 3 'Senses' CXC chemokine receptor 4 activation through GPCR kinase phosphorylation. Mol Pharmacol (2023) 104(4):174–86. doi: 10.1124/molpharm.123.000710
26. Doring Y, Noels H, van der Vorst EPC, Neideck C, Egea V, Drechsler M, et al. Vascular CXCR4 limits atherosclerosis by maintaining arterial integrity: evidence from mouse and human studies. Circulation (2017) 136:388–403. doi: 10.1161/CIRCULATIONAHA.117.027646
27. Gerli G, Vanelli C, Turri O, Erario M, Gardellini A, Pugliano M, et al. SDF1-3'A gene polymorphism is associated with chronic myeloproliferative disease and thrombotic events. Clin Chem (2005) 51:2411–4. doi: 10.1373/clinchem.2005.057802
28. Petruzziello-Pellegrini TN, Yuen DA, Page AV, Patel S, Soltyk AM, Matouk CC, et al. The CXCR4/CXCR7/SDF-1 pathway contributes to the pathogenesis of Shiga toxin-associated hemolytic uremic syndrome in humans and mice. J Clin Invest (2012) 122:759–76. doi: 10.1172/JCI57313
29. Lee BC, Lee TH, Avraham S and Avraham HK. Involvement of the chemokine receptor CXCR4 and its ligand stromal cell-derived factor 1α in breast cancer cell migration through human brain microvascular endothelial cells. Mol Cancer Res (2004) 2:327–38. doi: 10.1158/1541-7786.327.2.6
30. Abi-Younes S, Sauty A, Mach F, Sukhova GK, Libby P, Luster AD. The stromal cell-derived factor-1 chemokine is a potent platelet agonist highly expressed in atherosclerotic plaques. Circ Res (2000) 86:131–8. doi: 10.1161/01.res.86.2.131
31. Leberzammer J, Agten SM, Blanchet X, Duan R, Ippel H, Megens RTA, et al. Targeting platelet-derived CXCL12 impedes arterial thrombosis. Blood (2022) 139:2691–705. doi: 10.1182/blood.2020010140
32. Massberg S, Konrad I, Schurzinger K, Lorenz M, Schneider S, Zohlnhoefer D, et al. Platelets secrete stromal cell-derived factor 1α and recruit bone marrow-derived progenitor cells to arterial thrombi in vivo. J Exp Med (2006) 203:1221–33. doi: 10.1084/jem.20051772
33. Zernecke A, Schober A, Bot I, von Hundelshausen P, Liehn EA, Mopps B, et al. SDF-1α/CXCR4 axis is instrumental in neointimal hyperplasia and recruitment of smooth muscle progenitor cells. Circ Res (2005) 96:784–91. doi: 10.1161/01.RES.0000162100.52009.38
34. Noels H, Zhou B, Tilstam PV, Theelen W, Li X, Pawig L, et al. Deficiency of endothelial CXCR4 reduces reendothelialization and enhances neointimal hyperplasia after vascular injury in atherosclerosis-prone mice. Arterioscler Thromb Vasc Biol (2014) 34:1209–20. doi: 10.1161/ATVBAHA.113.302878
35. Melchionna R, Porcelli D, Mangoni A, Carlini D, Liuzzo G, Spinetti G, et al. Laminar shear stress inhibits CXCR4 expression on endothelial cells: functional consequences for atherogenesis. FASEB J (2005) 19:629–31. doi: 10.1096/fj.04-2219fje
36. Meyer dos Santos S, Klinkhardt U, Scholich K, Nelson K, Monsefi N, Deckmyn H, et al. The CX3C chemokine fractalkine mediates platelet adhesion via the von Willebrand receptor glycoprotein Ib. Blood (2011) 117:4999–5008. doi: 10.1182/blood-2011-02-335471
37. Schulz C, Schafer A, Stolla M, Kerstan S, Lorenz M, von Bruhl ML, et al. Chemokine fractalkine mediates leukocyte recruitment to inflammatory endothelial cells in flowing whole blood: a critical role for P-selectin expressed on activated platelets. Circulation (2007) 116:764–73. doi: 10.1161/CIRCULATIONAHA.107.695189
38. von Bruhl ML, Stark K, Steinhart A, Chandraratne S, Konrad I, Lorenz M, et al. Monocytes, neutrophils, and platelets cooperate to initiate and propagate venous thrombosis in mice in vivo. J Exp Med (2012) 209:819–35. doi: 10.1084/jem.20112322
39. Hillyer P, Mordelet E, Flynn G and Male D. Chemokines, chemokine receptors and adhesion molecules on different human endothelia: discriminating the tissue-specific functions that affect leucocyte migration. Clin Exp Immunol (2003) 134:431–41. doi: 10.1111/j.1365-2249.2003.02323.x
40. Oynebraten I, Bakke O, Brandtzaeg P, Johansen FE, Haraldsen G. Rapid chemokine secretion from endothelial cells originates from 2 distinct compartments. Blood (2004) 104:314–20. doi: 10.1182/blood-2003-08-2891
41. Reitsma S, Slaaf DW, Vink H, van Zandvoort MA, oude Egbrink MG. The endothelial glycocalyx: composition, functions, and visualization. Pflugers Arch (2007) 454:345–59. doi: 10.1007/s00424-007-0212-8
42. Graham GJ, Handel TM and Proudfoot AEI. Leukocyte adhesion: reconceptualizing chemokine presentation by glycosaminoglycans. Trends Immunol (2019) 40:472–81. doi: 10.1016/j.it.2019.03.009
43. von Hundelshausen P, Weber KS, Huo Y, Proudfoot AE, Nelson PJ, Ley K, et al. RANTES deposition by platelets triggers monocyte arrest on inflamed and atherosclerotic endothelium. Circulation (2001) 103:1772–7. doi: 10.1161/01.cir.103.13.1772
44. Huo Y, Schober A, Forlow SB, Smith DF, Hyman MC, Jung S, et al. Circulating activated platelets exacerbate atherosclerosis in mice deficient in apolipoprotein E. Nat Med (2003) 9:61–7. doi: 10.1038/nm810
45. Mause SF, Ritzel E, Liehn EA, Hristov M, Bidzhekov K, Muller-Newen G, et al. Platelet microparticles enhance the vasoregenerative potential of angiogenic early outgrowth cells after vascular injury. Circulation (2010) 122:495–506. doi: 10.1161/CIRCULATIONAHA.109.909473
46. Oynebraten I, Barois N, Bergeland T, Kuchler AM, Bakke O, Haraldsen G. Oligomerized, filamentous surface presentation of RANTES/CCL5 on vascular endothelial cells. Sci Rep (2015) 5:9261. doi: 10.1038/srep09261
47. Burke AP, Farb A, Malcom GT, Liang YH, Smialek J and Virmani R. Coronary risk factors and plaque morphology in men with coronary disease who died suddenly. N Engl J Med (1997) 336:1276–82. doi: 10.1056/NEJM199705013361802
48. Sobczak AIS, Pitt SJ, Stewart AJ. Glycosaminoglycan neutralization in coagulation control. Arterioscler Thromb Vasc Biol (2018) 38:1258–70. doi: 10.1161/ATVBAHA.118.311102
49. Fiore MM, Kakkar VV. Platelet factor 4 neutralizes heparan sulfate-enhanced antithrombin inactivation of factor Xa by preventing interaction(s) of enzyme with polysaccharide. Biochem Biophys Res Commun (2003) 311:71–6. doi: 10.1016/j.bbrc.2003.09.171
50. Dehmer GJ, Fisher M, Tate DA, Teo S and Bonnem EM. Reversal of heparin anticoagulation by recombinant platelet factor 4 in humans. Circulation (1995) 91:2188–94. doi: 10.1161/01.CIR.91.8.2188
51. Demma L, Levy JH. A case series of recombinant platelet factor 4 for heparin reversal after cardiopulmonary bypass. Anesth Analg (2012) 115:1273–8. doi: 10.1213/ANE.0b013e3182662e1a
52. Warttinger U, Giese C, Harenberg J, Holmer E, Kramer R. A fluorescent probe assay (Heparin Red) for direct detection of heparins in human plasma. Anal Bioanal Chem (2016) 408:8241–51. doi: 10.1007/s00216-016-9940-y
53. Kantarcioglu B, Mehrotra S, Papineni C, Siddiqui F, Kouta A, Hoppensteadt D, et al. Endogenous glycosaminoglycans in various pathologic plasma samples as measured by a fluorescent quenching method. Clin Appl Thromb Hemost (2022) 28:10760296221144047. doi: 10.1177/10760296221144047
54. Eslin DE, Zhang C, Samuels KJ, Rauova L, Zhai L, Niewiarowski S, et al. Transgenic mice studies demonstrate a role for platelet factor 4 in thrombosis: dissociation between anticoagulant and antithrombotic effect of heparin. Blood (2004) 104:3173–80. doi: 10.1182/blood-2003-11-3994
55. Slungaard A, Key NS. Platelet factor 4 stimulates thrombomodulin protein C-activating cofactor activity. A structure-function analysis. J Biol Chem (1994) 269:25549–56. doi: 10.1016/S0021-9258(18)47284-5
56. Kowalska MA, Mahmud SA, Lambert MP, Poncz M, Slungaard A. Endogenous platelet factor 4 stimulates activated protein C generation in vivo and improves survival after thrombin or lipopolysaccharide challenge. Blood (2007) 110:1903–5. doi: 10.1182/blood-2007-03-081901
57. Kowalska MA, Zhao G, Zhai L, David G 3rd, Marcus S, Krishnaswamy S, et al. Modulation of protein C activation by histones, platelet factor 4, and heparinoids: new insights into activated protein C formation. Arterioscler Thromb Vasc Biol (2014) 34:120–6. doi: 10.1161/ATVBAHA.113.302236
58. Mizuno T, Yoshioka K, Mizuno M, Shimizu M, Nagano F, Okuda T, et al. Complement component 5 promotes lethal thrombosis. Sci Rep (2017) 7:42714. doi: 10.1038/srep42714
59. Nording H, Baron L, Haberthur D, Emschermann F, Mezger M, Sauter M, et al. The C5a/C5a receptor 1 axis controls tissue neovascularization through CXCL4 release from platelets. Nat Commun (2021) 12:3352. doi: 10.1038/s41467-021-23499-w
60. Preston RJ, Tran S, Johnson JA, Ni Ainle F, Harmon S, White B, et al. Platelet factor 4 impairs the anticoagulant activity of activated protein C. J Biol Chem (2009) 284:5869–75. doi: 10.1074/jbc.M804703200
61. Clark SR, Ma AC, Tavener SA, McDonald B, Goodarzi Z, Kelly MM, et al. Platelet TLR4 activates neutrophil extracellular traps to ensnare bacteria in septic blood. Nat Med (2007) 13:463–9. doi: 10.1038/nm1565
62. Fuchs TA, Brill A, Duerschmied D, Schatzberg D, Monestier M, Myers DD Jr., et al. Extracellular DNA traps promote thrombosis. Proc Natl Acad Sci USA (2010) 107:15880–5. doi: 10.1073/pnas.1005743107
63. Healy LD, Puy C, Fernandez JA, Mitrugno A, Keshari RS, Taku NA, et al. Activated protein C inhibits neutrophil extracellular trap formation in vitro and activation in vivo. J Biol Chem (2017) 292:8616–29. doi: 10.1074/jbc.M116.768309
64. Brandt E, Petersen F, Ludwig A, Ehlert JE, Bock L and Flad HD. The β-thromboglobulins and platelet factor 4: blood platelet-derived CXC chemokines with divergent roles in early neutrophil regulation. J Leukoc Biol (2000) 67:471–8. doi: 10.1002/jlb.67.4.471
65. Egan K, van Geffen JP, Ma H, Kevane B, Lennon A, Allen S, et al. Effect of platelet-derived β-thromboglobulins on coagulation. Thromb Res (2017) 154:7–15. doi: 10.1016/j.thromres.2017.03.023
66. Johnston I, Sarkar A, Hayes V, Koma GT, Arepally GM, Chen J, et al. Recognition of PF4-VWF complexes by heparin-induced thrombocytopenia antibodies contributes to thrombus propagation. Blood (2020) 135:1270–80. doi: 10.1182/blood.2018881607
67. Springer TA. von Willebrand factor, Jedi knight of the bloodstream. Blood (2014) 124:1412–25. doi: 10.1182/blood-2014-05-378638
68. Lenting PJ, Christophe OD and Denis CV. von Willebrand factor biosynthesis, secretion, and clearance: connecting the far ends. Blood (2015) 125:2019–28. doi: 10.1182/blood-2014-06-528406
69. Fu HX, Jiang Y, Yang DR, Scheiflinger F, Wong WP, Springer TA. Flow-induced elongation of von Willebrand factor precedes tension-dependent activation. Nat Commun (2017) 8:1–12. doi: 10.1038/s41467-017-00230-2
70. Nazy I, Elliott TD, Arnold DM. Platelet factor 4 inhibits ADAMTS13 activity and regulates the multimeric distribution of von Willebrand factor. Br J Haematol (2020) 190:594–8. doi: 10.1111/bjh.16553
71. Kowalska MA, Rauova L, Poncz M. Role of the platelet chemokine platelet factor 4 (PF4) in hemostasis and thrombosis. Thromb Res (2010) 125:292–6. doi: 10.1016/j.thromres.2009.11.023
72. Huang J, Swieringa F, Solari FA, Provenzale I, Grassi L, De Simone I, et al. Assessment of a complete and classified platelet proteome from genome-wide transcripts of human platelets and megakaryocytes covering platelet functions. Sci Rep (2021) 11:12358. doi: 10.1038/s41598-021-91661-x
73. Rowley JW, Oler AJ, Tolley ND, Hunter BN, Low EN, Nix DA, et al. Genome-wide RNA-seq analysis of human and mouse platelet transcriptomes. Blood (2011) 118:e101–11. doi: 10.1182/blood-2011-03-339705
74. Burkhart JM, Vaudel M, Gambaryan S, Radau S, Walter U, Martens L, et al. The first comprehensive and quantitative analysis of human platelet protein composition allows the comparative analysis of structural and functional pathways. Blood (2012) 120:e73–82. doi: 10.1182/blood-2012-04-416594
75. Lewandrowski U, Wortelkamp S, Lohrig K, Zahedi RP, Wolters DA, Walter U, et al. Platelet membrane proteomics: a novel repository for functional research. Blood (2009) 114:e10–9. doi: 10.1182/blood-2009-02-203828
76. Rieckmann JC, Geiger R, Hornburg D, Wolf T, Kveler K, Jarrossay D, et al. Social network architecture of human immune cells unveiled by quantitative proteomics. Nat Immunol (2017) 18:583–93. doi: 10.1038/ni.3693
77. Kowalska MA, Ratajczak J, Hoxie J, Brass LF, Gewirtz A, Poncz M, et al. Megakaryocyte precursors, megakaryocytes and platelets express the HIV co-receptor CXCR4 on their surface: determination of response to stromal-derived factor-1 by megakaryocytes and platelets. Br J Haematol (1999) 104:220–9. doi: 10.1046/j.1365-2141.1999.01169.x
78. Clemetson KJ, Clemetson JM, Proudfoot AE, Power CA, Baggiolini M, Wells TN. Functional expression of CCR1, CCR3, CCR4, and CXCR4 chemokine receptors on human platelets. Blood (2000) 96:4046–54. doi: 10.1182/blood.V96.13.4046
79. Gewirtz AM, Zhang J, Ratajczak J, Ratajczak M, Park KS, Li C, et al. Chemokine regulation of human megakaryocytopoiesis. Blood (1995) 86:2559–67. doi: 10.1182/blood.V86.7.2559.2559
80. Craig R, Cortens JP, Beavis RC. Open source system for analyzing, validating, and storing protein identification data. J Proteome Res (2004) 3:1234–42. doi: 10.1021/pr049882h
81. Liu D, Cao Y, Zhang X, Peng C, Tian X, Yan C, et al. Chemokine CC-motif ligand 2 participates in platelet function and arterial thrombosis by regulating PKCα-P38MAPK-HSP27 pathway. Biochim Biophys Acta Mol Basis Dis (2018) 1864:2901–12. doi: 10.1016/j.bbadis.2018.05.025
82. Chatterjee M, Seizer P, Borst O, Schonberger T, Mack A, Geisler T, et al. SDF-1α. induces differential trafficking of CXCR4-CXCR7 involving cyclophilin A, CXCR7 ubiquitination and promotes platelet survival. FASEB J (2014) 28:2864–78. doi: 10.1096/fj.14-249730
83. Chatterjee M, Borst O, Walker B, Fotinos A, Vogel S, Seizer P, et al. Macrophage migration inhibitory factor limits activation-induced apoptosis of platelets via CXCR7-dependent Akt signaling. Circ Res (2014) 115:939–49. doi: 10.1161/CIRCRESAHA.115.305171
84. Borst O, Munzer P, Gatidis S, Schmidt EM, Schonberger T, Schmid E, et al. The inflammatory chemokine CXC motif ligand 16 triggers platelet activation and adhesion via CXC motif receptor 6-dependent phosphatidylinositide 3-kinase/Akt signaling. Circ Res (2012) 111:1297–307. doi: 10.1161/CIRCRESAHA.112.276444
85. Gleissner CA, von Hundelshausen P, Ley K. Platelet chemokines in vascular disease. Arterioscler Thromb Vasc Biol (2008) 28:1920–7. doi: 10.1161/ATVBAHA.108.169417
86. Kowalska MA, Ratajczak MZ, Majka M, Jin J, Kunapuli S, Brass L, et al. Stromal cell-derived factor-1 and macrophage-derived chemokine: 2 chemokines that activate platelets. Blood (2000) 96:50–7. doi: 10.1182/blood.V96.1.50
87. Walsh TG, Harper MT, Poole AW. SDF-1α is a novel autocrine activator of platelets operating through its receptor CXCR4. Cell Signal (2015) 27:37–46. doi: 10.1016/j.cellsig.2014.09.021
88. Rath D, Chatterjee M, Borst O, Muller K, Stellos K, Mack AF, et al. Expression of stromal cell-derived factor-1 receptors CXCR4 and CXCR7 on circulating platelets of patients with acute coronary syndrome and association with left ventricular functional recovery. Eur Heart J (2014) 35:386–94. doi: 10.1093/eurheartj/eht448
89. Ohtsuka H, Iguchi T, Hayashi M, Kaneda M, Iida K, Shimonaka M, et al. SDF-1α/CXCR4 signaling in lipid rafts induces platelet aggregation via PI3 kinase-dependent Akt phosphorylation. PloS One (2017) 12:e0169609. doi: 10.1371/journal.pone.0169609
90. Nakashima D, Onuma T, Tanabe K, Kito Y, Uematsu K, Mizutani D, et al. Synergistic effect of collagen and CXCL12 in the low doses on human platelet activation. PloS One (2020) 15:e0241139. doi: 10.1371/journal.pone.0241139
91. Walsh TG, Poole AW. Do platelets promote cardiac recovery after myocardial infarction: roles beyond occlusive ischemic damage. Am J Physiol Heart Circ Physiol (2018) 314:H1043–8. doi: 10.1152/ajpheart.00134.2018
92. Nore BF, Vargas L, Mohamed AJ, Branden LJ, Backesjo CM, Islam TC, et al. Redistribution of Bruton's tyrosine kinase by activation of phosphatidylinositol 3-kinase and Rho-family GTPases. Eur J Immunol (2000) 30:145–54. doi: 10.1002/1521-4141(200001)30:1<145::AID-IMMU145>3.0.CO;2-0
93. Rayes J, Watson SP and Nieswandt B. Functional significance of the platelet immune receptors GPVI and CLEC-2. J Clin Invest (2019) 129:12–23. doi: 10.1172/JCI122955
94. Heestermans M, Poenou G, Duchez AC, Hamzeh-Cognasse H, Bertoletti L, Cognasse F. Immunothrombosis and the role of platelets in venous thromboembolic diseases. Int J Mol Sci (2022) 23:13176. doi: 10.3390/ijms232113176
95. Sun S, Chai S, Zhang F, Lu L. Overexpressed microRNA-103a-3p inhibits acute lower-extremity deep venous thrombosis via inhibition of CXCL12. IUBMB Life (2020) 72:492–504. doi: 10.1002/iub.2168
96. Richter R, Jochheim-Richter A, Ciuculescu F, Kollar K, Seifried E, Forssmann U, et al. Identification and characterization of circulating variants of CXCL12 from human plasma: effects on chemotaxis and mobilization of hematopoietic stem and progenitor cells. Stem Cells Dev (2014) 23:1959–74. doi: 10.1089/scd.2013.0524
97. Zhong J, Rajagopalan S. Dipeptidyl peptidase-4 regulation of SDF-1/CXCR4 axis: implications for cardiovascular disease. Front Immunol (2015) 6:477. doi: 10.3389/fimmu.2015.00477
98. Xin L, Sun S, Wang J, Lu W, Wang T, Tang H. Dipeptidyl peptidase 4 inhibitors and venous thromboembolism risk in patients with type 2 diabetes: a meta-analysis of cardiovascular outcomes trials. Thromb Haemost (2021) 121:106–8. doi: 10.1055/s-0040-1715444
99. Gouverneur A, Lair A, Arnaud M, Bégaud B, Raschi E, Pariente A, et al. DPP-4 inhibitors and venous thromboembolism: an analysis of the WHO spontaneous reporting database. Lancet Diabetes Endocrinol (2020) 8:365–7. doi: 10.1016/s2213-8587(20)30112-1
100. Bernhagen J, Krohn R, Lue H, Gregory JL, Zernecke A, Koenen RR, et al. MIF is a noncognate ligand of CXC chemokine receptors in inflammatory and atherogenic cell recruitment. Nat Med (2007) 13:587–96. doi: 10.1038/nm1567
101. Rajasekaran D, Groning S, Schmitz C, Zierow S, Drucker N, Bakou M, et al. Macrophage migration inhibitory factor-CXCR4 receptor interactions: evidence for partial allosteric agonism in comparison with CXCL12 chemokine. J Biol Chem (2016) 291:15881–95. doi: 10.1074/jbc.M116.717751
102. Witte A, Rohlfing AK, Dannenmann B, Dicenta V, Nasri M, Kolb K, et al. The chemokine CXCL14 mediates platelet function and migration via direct interaction with CXCR4. Cardiovasc Res (2021) 117:903–17. doi: 10.1093/cvr/cvaa080
103. Collins PJ, McCully ML, Martínez-Muñoz L, Santiago C, Wheeldon J, Caucheteux S, et al. Epithelial chemokine CXCL14 synergizes with CXCL12 via allosteric modulation of CXCR4. FASEB J (2017) 31:3084–97. doi: 10.1096/fj.201700013R
104. Otte M, Kliewer A, Schütz D, Reimann C, Schulz S, Stumm R. CXCL14 is no direct modulator of CXCR4. FEBS Lett (2014) 588:4769–75. doi: 10.1016/j.febslet.2014.11.009
105. Wirtz TH, Tillmann S, Strussmann T, Kraemer S, Heemskerk JW, Grottke O, et al. Platelet-derived MIF: a novel platelet chemokine with distinct recruitment properties. Atherosclerosis (2015) 239:1–10. doi: 10.1016/j.atherosclerosis.2014.12.039
106. Brandhofer M, Hoffmann A, Blanchet X, Siminkovitch E, Rohlfing AK, El Bounkari O, et al. Heterocomplexes between the atypical chemokine MIF and the CXC-motif chemokine CXCL4L1 regulate inflammation and thrombus formation. Cell Mol Life Sci (2022) 79:512. doi: 10.1007/s00018-022-04539-0
107. Shenkman B, Brill A, Brill G, Lider O, Savion N and Varon D. Differential response of platelets to chemokines: RANTES non-competitively inhibits stimulatory effect of SDF-1α. J Thromb Haemost (2004) 2:154–60. doi: 10.1111/j.1538-7836.2004.00527.x
108. Kameyoshi Y, Dorschner A, Mallet AI, Christophers E, Schroder JM. Cytokine RANTES released by thrombin-stimulated platelets is a potent attractant for human eosinophils. J Exp Med (1992) 176:587–92. doi: 10.1084/jem.176.2.587
109. von Hundelshausen P, Agten SM, Eckardt V, Blanchet X, Schmitt MM, Ippel H, et al. Chemokine interactome mapping enables tailored intervention in acute and chronic inflammation. Sci Transl Med (2017) 9:eaah6650. doi: 10.1126/scitranslmed.aah6650
110. Rath D, Chatterjee M, Bongartz A, Muller K, Droppa M, Stimpfle F, et al. Platelet surface expression of SDF-1 is associated with clinical outcomes in the patients with cardiovascular disease. Platelets (2017) 28:34–9. doi: 10.1080/09537104.2016.1203399
111. Rath D, Chatterjee M, Borst O, Muller K, Langer H, Mack AF, et al. Platelet surface expression of stromal cell-derived factor-1 receptors CXCR4 and CXCR7 is associated with clinical outcomes in patients with coronary artery disease. J Thromb Haemost (2015) 13:719–28. doi: 10.1111/jth.12870
112. Chatterjee M, Rath D, Gawaz M. Role of chemokine receptors CXCR4 and CXCR7 for platelet function. Biochem Soc Trans (2015) 43:720–6. doi: 10.1042/BST20150113
113. Levoye A, Balabanian K, Baleux F, Bachelerie F and Lagane B. CXCR7 heterodimerizes with CXCR4 and regulates CXCL12-mediated G protein signaling. Blood (2009) 113:6085–93. doi: 10.1182/blood-2008-12-196618
114. Decaillot FM, Kazmi MA, Lin Y, Ray-Saha S, Sakmar TP, Sachdev P. CXCR7/CXCR4 heterodimer constitutively recruits beta-arrestin to enhance cell migration. J Biol Chem (2011) 286:32188–97. doi: 10.1074/jbc.M111.277038
115. Collado A, Marques P, Escudero P, Rius C, Domingo E, Martinez-Hervas S, et al. Functional role of endothelial CXCL16/CXCR6-platelet-leucocyte axis in angiotensin II-associated metabolic disorders. Cardiovasc Res (2018) 114:1764–75. doi: 10.1093/cvr/cvy135
116. Guan TY, Emschermann F, Schories C, Groga-Bada P, Martus P, Borst O, et al. Platelet SR-PSOX/CXCL16-CXCR6 axis influences thrombotic propensity and prognosis in coronary artery disease. Int J Mol Sci (2022) 23:1–23. doi: 10.3390/ijms231911066
117. Ghasemzadeh M, Kaplan ZS, Alwis I, Schoenwaelder SM, Ashworth KJ, Westein E, et al. The CXCR1/2 ligand NAP-2 promotes directed intravascular leukocyte migration through platelet thrombi. Blood (2013) 121:4555–66. doi: 10.1182/blood-2012-09-459636
118. Wang JJ, Armour B, Chataway T, Troelnikov A, Colella A, Yacoub O, et al. Vaccine-induced immune thrombotic thrombocytopenia is mediated by a stereotyped clonotypic antibody. Blood (2022) 140:1738–42. doi: 10.1182/blood.2022016474
119. Zheng Y, Wang AW, Yu M, Padmanabhan A, Tourdot BE, Newman DK, et al. B-cell tolerance regulates production of antibodies causing heparin-induced thrombocytopenia. Blood (2014) 123:931–4. doi: 10.1182/blood-2013-11-540781
120. Regnault V, de Maistre E, Carteaux JP, Gruel Y, Nguyen P, Tardy B, et al. Platelet activation induced by human antibodies to interleukin-8. Blood (2003) 101:1419–21. doi: 10.1182/blood-2002-02-0620
121. Goldmann L, Duan R, Kragh T, Wittmann G, Weber C, Lorenz R, et al. Oral Bruton tyrosine kinase inhibitors block activation of the platelet Fc receptor CD32a (FcgammaRIIA): a new option in HIT? Blood Adv (2019) 3:4021–33. doi: 10.1182/bloodadvances.2019000617
122. Weber C, von Hundelshausen P, Siess W. VITT after ChAdOx1 nCoV-19 vaccination. N Engl J Med (2021) 385:2203–4. doi: 10.1056/NEJMc2111026
123. von Hundelshausen P, Lorenz R, Siess W, Weber C. Vaccine-induced immune thrombotic thrombocytopenia (VITT): targeting pathomechanisms with bruton tyrosine kinase inhibitors. Thromb Haemost (2021) 121:1395–9. doi: 10.1055/a-1481-3039
124. Arepally GM, Cines DB. Pathogenesis of heparin-induced thrombocytopenia. Transl Res (2020) 225:131–40. doi: 10.1016/j.trsl.2020.04.014
125. Greinacher A, Selleng K, Warkentin TE. Autoimmune heparin-induced thrombocytopenia. J Thromb Haemost (2017) 15:2099–114. doi: 10.1111/jth.13813
126. Cines DB, Greinacher A. Vaccine-induced immune thrombotic thrombocytopenia. Blood (2023) 141:1659–65. doi: 10.1182/blood.2022017696
127. Mangold A, Alias S, Scherz T, Hofbauer M, Jakowitsch J, Panzenbock A, et al. Coronary neutrophil extracellular trap burden and deoxyribonuclease activity in ST-elevation acute coronary syndrome are predictors of ST-segment resolution and infarct size. Circ Res (2015) 116:1182–92. doi: 10.1161/CIRCRESAHA.116.304944
128. Laridan E, Denorme F, Desender L, Francois O, Andersson T, Deckmyn H, et al. Neutrophil extracellular traps in ischemic stroke thrombi. Ann Neurol (2017) 82:223–32. doi: 10.1002/ana.24993
129. Yago T, Liu Z, Ahamed J, McEver RP. Cooperative PSGL-1 and CXCR2 signaling in neutrophils promotes deep vein thrombosis in mice. Blood (2018) 132:1426–37. doi: 10.1182/blood-2018-05-850859
130. Brinkmann V, Reichard U, Goosmann C, Fauler B, Uhlemann Y, Weiss DS, et al. Neutrophil extracellular traps kill bacteria. Science (2004) 303:1532–5. doi: 10.1126/science.1092385
131. Teijeira A, Garasa S, Ochoa MDC, Cirella A, Olivera I, Glez-Vaz J, et al. Differential Interleukin-8 thresholds for chemotaxis and netosis in human neutrophils. Eur J Immunol (2021) 51:2274–80. doi: 10.1002/eji.202049029
132. Kaiser R, Leunig A, Pekayvaz K, Popp O, Joppich M, Polewka V, et al. Self-sustaining IL-8 loops drive a prothrombotic neutrophil phenotype in severe COVID-19. JCI Insight (2021) 6:1–15. doi: 10.1172/jci.insight.150862
133. Gollomp K, Kim M, Johnston I, Hayes V, Welsh J, Arepally GM, et al. Neutrophil accumulation and NET release contribute to thrombosis in HIT. JCI Insight (2018) 3:1–14. doi: 10.1172/jci.insight.99445
134. Leung HHL, Perdomo J, Ahmadi Z, Zheng SYS, Rashid FN, Enjeti A, et al. NETosis and thrombosis in vaccine-induced immune thrombotic thrombocytopenia. Nat Commun (2022) 13:1–11. doi: 10.1038/s41467-022-32946-1
135. Lande R, Lee EY, Palazzo R, Marinari B, Pietraforte I, Santos GS, et al. CXCL4 assembles DNA into liquid crystalline complexes to amplify TLR9-mediated interferon-α production in systemic sclerosis. Nat Commun (2019) 10:1731. doi: 10.1038/s41467-019-09683-z
136. Gollomp K, Sarkar A, Harikumar S, Seeholzer SH, Arepally GM, Hudock K, et al. Fc-modified HIT-like monoclonal antibody as a novel treatment for sepsis. Blood (2020) 135:743–54. doi: 10.1182/blood.2019002329
137. Vajen T, Koenen RR, Werner I, Staudt M, Projahn D, Curaj A, et al. Blocking CCL5-CXCL4 heteromerization preserves heart function after myocardial infarction by attenuating leukocyte recruitment and NETosis. Sci Rep (2018) 8:10647. doi: 10.1038/s41598-018-29026-0
138. Du Y, Ah Kioon MD, Laurent P, Chaudhary V, Pierides M, Yang C, et al. Chemokines form nanoparticles with DNA and can superinduce TLR-driven immune inflammation. J Exp Med (2022) 219:1–18. doi: 10.1084/jem.20212142
139. Di Domizio J, Belkhodja C, Chenuet P, Fries A, Murray T, Mondejar PM, et al. The commensal skin microbiota triggers type I IFN-dependent innate repair responses in injured skin. Nat Immunol (2020) 21:1034–45. doi: 10.1038/s41590-020-0721-6
140. Alsabani M, Abrams ST, Cheng Z, Morton B, Lane S, Alosaimi S, et al. Reduction of NETosis by targeting CXCR1/2 reduces thrombosis, lung injury, and mortality in experimental human and murine sepsis. Br J Anaesth (2022) 128:283–93. doi: 10.1016/j.bja.2021.10.039
141. Sachetto ATA, Mackman N. Monocyte tissue factor expression: lipopolysaccharide induction and roles in pathological activation of coagulation. Thromb Haemost (2023), 1–17. doi: 10.1055/a-2091-7006
142. Shahneh F, Christian Probst H, Wiesmann SC, AG N, Ruf W, Steinbrink K, et al. Inflammatory monocyte counts determine venous blood clot formation and resolution. Arterioscler Thromb Vasc Biol (2022) 42:145–55. doi: 10.1161/ATVBAHA.121.317176
143. Fujimura N, Xu B, Dalman J, Deng H, Aoyama K, Dalman RL. CCR2 inhibition sequesters multiple subsets of leukocytes in the bone marrow. Sci Rep (2015) 5:11664. doi: 10.1038/srep11664
144. Serbina NV, Pamer EG. Monocyte emigration from bone marrow during bacterial infection requires signals mediated by chemokine receptor CCR2. Nat Immunol (2006) 7:311–7. doi: 10.1038/ni1309
145. Laurance S, Bertin FR, Ebrahimian T, Kassim Y, Rys RN, Lehoux S, et al. Gas6 promotes inflammatory CCR2hiCX3CR1lo monocyte recruitment in venous thrombosis. Arterioscler Thromb Vasc Biol (2017) 37:1315–22. doi: 10.1161/ATVBAHA.116.308925
146. Wang ZW, Wang JJ, Zhang JZ, Xue ZJ, Miao J, Li L, et al. Thrombolysis of deep vein thrombosis and inhibiting chemotaxis of macrophage by MCP-1 blockage. Eur Rev Med Pharmacol Sci (2017) 21:1695–701.
147. Lippi G, Favaloro EJ. Venous and arterial thromboses: two sides of the same coin? Semin Thromb Hemost (2018) 44:239–48. doi: 10.1055/s-0037-1607202
148. Mizuno K, Satomura K, Miyamoto A, Arakawa K, Shibuya T, Arai T, et al. Angioscopic evaluation of coronary-artery thrombi in acute coronary syndromes. N Engl J Med (1992) 326:287–91. doi: 10.1056/nejm199201303260502
149. Barr JD, Chauhan AK, Schaeffer GV, Hansen JK, Motto DG. Red blood cells mediate the onset of thrombosis in the ferric chloride murine model. Blood (2013) 121:3733–41. doi: 10.1182/blood-2012-11-468983
150. Weisel JW, Litvinov RI. Red blood cells: the forgotten player in hemostasis and thrombosis. J Thromb Haemost (2019) 17:271–82. doi: 10.1111/jth.14360
151. Karsten E, Hill CJ, Herbert BR. Red blood cells: The primary reservoir of macrophage migration inhibitory factor in whole blood. Cytokine (2018) 102:34–40. doi: 10.1016/j.cyto.2017.12.005
152. Bachelerie F, Ben-Baruch A, Burkhardt AM, Combadiere C, Farber JM, Graham GJ, et al. International Union of Basic and Clinical Pharmacology. [corrected]. LXXXIX. Update on the extended family of chemokine receptors and introducing a new nomenclature for atypical chemokine receptors. Pharmacol Rev (2014) 66:1–79. doi: 10.1124/pr.113.007724
153. Horuk R, Colby TJ, Darbonne WC, Schall TJ, Neote K. The human erythrocyte inflammatory peptide (chemokine) receptor. Biochemical characterization, solubilization, and development of a binding assay for the soluble receptor. Biochemistry (1993) 32:5733–8. doi: 10.1021/bi00073a002
154. Gardner L, Patterson AM, Ashton BA, Stone MA, Middleton J. The human Duffy antigen binds selected inflammatory but not homeostatic chemokines. Biochem Biophys Res Commun (2004) 321:306–12. doi: 10.1016/j.bbrc.2004.06.146
155. Schnabel RB, Baumert J, Barbalic M, Dupuis J, Ellinor PT, Durda P, et al. Duffy antigen receptor for chemokines (Darc) polymorphism regulates circulating concentrations of monocyte chemoattractant protein-1 and other inflammatory mediators. Blood (2010) 115:5289–99. doi: 10.1182/blood-2009-05-221382
156. Nakano H, Gunn MD. Gene duplications at the chemokine locus on mouse chromosome 4: multiple strain-specific haplotypes and the deletion of secondary lymphoid-organ chemokine and EBI-1 ligand chemokine genes in the plt mutation. J Immunol (2001) 166:361–9. doi: 10.4049/jimmunol.166.1.361
157. Zlotnik A, Yoshie O, Nomiyama H. The chemokine and chemokine receptor superfamilies and their molecular evolution. Genome Biol (2006) 7:243. doi: 10.1186/gb-2006-7-12-243
158. Fan X, Patera AC, Pong-Kennedy A, Deno G, Gonsiorek W, Manfra DJ, et al. Murine CXCR1 is a functional receptor for GCP-2/CXCL6 and interleukin-8/CXCL8. J Biol Chem (2007) 282:11658–66. doi: 10.1074/jbc.M607705200
159. Sawant KV, Poluri KM, Dutta AK, Sepuru KM, Troshkina A, Garofalo RP, et al. Chemokine CXCL1 mediated neutrophil recruitment: Role of glycosaminoglycan interactions. Sci Rep (2016) 6:33123. doi: 10.1038/srep33123
160. Gale JD, Gilbert S, Blumenthal S, Elliott T, Pergola PE, Goteti K, et al. Effect of PF-04634817, an oral CCR2/5 chemokine receptor antagonist, on albuminuria in adults with overt diabetic nephropathy. Kidney Int Rep (2018) 3:1316–27. doi: 10.1016/j.ekir.2018.07.010
161. de Zeeuw D, Bekker P, Henkel E, Hasslacher C, Gouni-Berthold I, Mehling H, et al. The effect of CCR2 inhibitor CCX140-B on residual albuminuria in patients with type 2 diabetes and nephropathy: a randomised trial. Lancet Diabetes Endocrinol (2015) 3:687–96. doi: 10.1016/S2213-8587(15)00261-2
162. Semerad CL, Liu F, Gregory AD, Stumpf K, Link DC. G-CSF is an essential regulator of neutrophil trafficking from the bone marrow to the blood. Immunity (2002) 17:413–23. doi: 10.1016/s1074-7613(02)00424-7
163. Bajrami B, Zhu H, Kwak HJ, Mondal S, Hou Q, Geng G, et al. G-CSF maintains controlled neutrophil mobilization during acute inflammation by negatively regulating CXCR2 signaling. J Exp Med (2016) 213:1999–2018. doi: 10.1084/jem.20160393
164. Tsou CL, Peters W, Si Y, Slaymaker S, Aslanian AM, Weisberg SP, et al. Critical roles for CCR2 and MCP-3 in monocyte mobilization from bone marrow and recruitment to inflammatory sites. J Clin Invest (2007) 117:902–9. doi: 10.1172/jci29919
165. Denisov SS, Heinzmann ACA, Vajen T, Vries MHM, Megens RTA, Suylen D, et al. Tick saliva protein evasin-3 allows for visualization of inflammation in arteries through interactions with CXC-type chemokines deposited on activated endothelium. Bioconjug Chem (2020) 31:948–55. doi: 10.1021/acs.bioconjchem.0c00095
Keywords: platelet, atherothrombosis, inflammation, leukocyte, red blood cell, endothelial cell
Citation: Leberzammer J and von Hundelshausen P (2023) Chemokines, molecular drivers of thromboinflammation and immunothrombosis. Front. Immunol. 14:1276353. doi: 10.3389/fimmu.2023.1276353
Received: 11 August 2023; Accepted: 12 October 2023;
Published: 26 October 2023.
Edited by:
James David McFadyen, Baker Heart and Diabetes Institute, AustraliaReviewed by:
Konstantinos Stellos, University of Heidelberg, GermanyFlorian Gaertner, Institute of Science and Technology Austria (IST Austria), Austria
Copyright © 2023 Leberzammer and von Hundelshausen. This is an open-access article distributed under the terms of the Creative Commons Attribution License (CC BY). The use, distribution or reproduction in other forums is permitted, provided the original author(s) and the copyright owner(s) are credited and that the original publication in this journal is cited, in accordance with accepted academic practice. No use, distribution or reproduction is permitted which does not comply with these terms.
*Correspondence: Philipp von Hundelshausen, UGhpbGlwcC52b25fSHVuZGVsc2hhdXNlbkBtZWQudW5pLW11ZW5jaGVuLmRl