- 1Laboratorio de Inmunología y Virología, Facultad de Ciencias Biológicas, Universidad Autónoma de Nuevo León, San Nicolás de los Garza, NL, Mexico
- 2The Institute for Obesity Research, Tecnológico de Monterrey, Monterrey, NL, Mexico
- 3Département d'Immunologie, Unité de Biologie Cellulaire des Lymphocytes, Pasteur Institute, Paris, France
Cancer immunotherapies include monoclonal antibodies, cytokines, oncolytic viruses, cellular therapies, and other biological and synthetic immunomodulators. These are traditionally studied for their effect on the immune system’s role in eliminating cancer cells. However, some of these therapies have the unique ability to directly induce cytotoxicity in cancer cells by inducing immunogenic cell death (ICD). Unlike general immune stimulation, ICD triggers specific therapy-induced cell death pathways, based on the release of damage-associated molecular patterns (DAMPs) from dying tumour cells. These activate innate pattern recognition receptors (PRRs) and subsequent adaptive immune responses, offering the promise of sustained anticancer drug efficacy and durable antitumour immune memory. Exploring how onco-immunotherapies can trigger ICD, enhances our understanding of their mechanisms and potential for combination strategies. This review explores the complexities of these immunotherapeutic approaches that induce ICD, highlighting their implications for the innate immune system, addressing challenges in cancer treatment, and emphasising the pivotal role of ICD in contemporary cancer research.
1 Introduction
Cancer immunotherapy aims to harness the patient’s own immune system to target and eliminate malignant cells. In 2013, cancer immunotherapy was named as “Breakthrough of the Year” by the journal Science for its promising potential in the field of oncology (1). Immunotherapy is often combined with other cancer treatments such as chemotherapy, surgery, radiotherapy, and targeted therapies, which aim to eliminate cancer cells by killing them. The combination of immune system activation with cancer cell-killing not only triggers the immune response but also precisely targets and eliminates cancer cells. This fusion demonstrates a markedly enhanced antitumor effect. In this way, cancer cells are eliminated by direct killing, while at the same time the immune system is activated.
Recently, ICD has been described as a promising form of therapy-induced anti-tumour immune system activation, and it is now considered to play a central role in various cancer treatment modalities. Although ICD targets cancer cells and mediates tumour‐specific immune responses, it occurs in the precise context of cell death induced by a specific therapy, making it conceptually distinct from cases of immune stimulation or inflammatory responses that do not rely on a therapy capable of inducing a specific modality of cell death (2). Thus, although ICD could be considered a form of immunotherapy, it has not been classified as such because it depends on a specific treatment that must be able to induce a specific cell death pathway that ends with a dying cancer cell that has sufficient antigenicity and adjuvanticity able to activate the immune system. ICD is characterized by the exposure and release of DAMPs from dying tumour cells that confer adjuvanticity. DAMPs are recognised by innate PRRs, resulting in the activation of innate cells and the subsequent activation of adaptive cells that mediate tumour‐specific immune responses. ICD is a promising strategy because it induces long‐term efficacy of anticancer drugs through the combination of the direct cancer cell killing and the activation of the antitumour immune system, leading to an anti-tumour immunological memory (3).
Some chemotherapies, radiotherapies, and targeted therapies have been shown to induce immunogenic cell death (4–6). Such ICD inductors have been successfully combined with different types of immunotherapies to promote better outcomes (7, 8). However, some immunotherapies, in parallel with their immunomodulatory effect that targets the immune system, can also be directly cytotoxic to the cancer cell and induce ICD. This article will review these specific types of immunotherapies, with an additional focus on their impact on the innate immune system.
2 Immunotherapies for cancer treatment
Oncology met immunology in 1891 when William B. Coley noticed that cancer patients who got infections after surgery seemed to do better than those who didn’t. So he tried immunotherapy for cancer by using erysipelas on a patient with inoperable sarcoma (9). He then created a filtered mixture of bacterial lysates called “Coley’s Toxins” to treat tumours. His first patient, John Ficken, with a large inoperable tumour (probably a malignant sarcoma) had a complete remission that lasted until his death from a heart attack 26 years later. Coley’s toxins may have stimulated the immune system to attack cancer cells. Thereafter, clinical interest in onco-immunotherapy waned, with research focusing on radiotherapy and chemotherapy (10–12), until 2013, when immunotherapy of cancer was named “Breakthrough of the Year” by Science (1).
Cancer is characterised by a number of features including activation of oncogenes, inactivation of tumour suppressor genes, resistance to cell death, angiogenesis, maintenance of proliferative signalling, immune suppression and avoidance of immune destruction (13). Even during cancer immunosurveillance, the most immunoevasive or highly mutagenic cancer cells may acquire the ability to evade immunosurveillance and thus generate a clinically relevant tumour. In this sense, cancer cells in an established tumour can evade anti-tumour immunity. In addition to the immunosuppressive microenvironment within the tumour, cancer cells can use several mechanisms for immunoevasion, which include (1): reducing their immunogenicity through the downregulation of tumour-associated antigens (TAAs) and major histocompatibility complex (MHC) class I expression (2), inducing tolerance by suppressing T cells (CD4+ and CD8+) through the promotion of immunosuppressive cytokines (e.g. IL-10 or TGFβ) or immune-checkpoints (e.g. regulated cell death 1, regulated cell death-ligand, cytotoxic T lymphocyte-associated protein-4), and (3) avoiding the immune cell-mediated lysis by overriding cell death pathways (14, 15), among others.
Pharmacological induction of cell death is the basis of almost all non-invasive cancer therapies. One of the major challenges in cancer treatment is to restore an anti-tumour immune response. In this sense, immunotherapies have transformed cancer treatment in recent years and have revitalised the field of tumour immunology (16). Cancer immunotherapy aims to stimulate the immune system in a controlled manner to eliminate cancer cells and prevent uncontrolled autoimmune inflammatory responses that lead to contraindications and therapeutic limitations (17). The main goals are to increase the quality or quantity of immune cells (especially effector cells), to generate tumour antigens and to eliminate mechanisms associated with immunosuppression, while minimising off-target effects. In addition, immunotherapies seek to induce long-lasting and durable responses in several cancer subsets, including solid and haematological malignancies. In this sense, several types of cancer immunotherapies have been developed with the aim of promoting cancer remission (18). These onco-immunotherapies are very vast and include (1) monoclonal antibodies (2), cytokines (3), oncolytic viruses (4), cellular therapies (5), and other biological and synthetic immunomodulators.
Activation of the anti-tumour immune system requires treatment strategies that can overcome the physiological barriers that control immune responses against tumour cells. Accordingly, immunotherapy uses strategies that target specific immunoregulatory processes to enhance anti-tumour immunity. However, cancer immunoediting can occur in response to immunotherapy as well as during tumour development. In this sense, immunotherapy may induce secondary (acquired) resistance that manifests as a clinical response followed by cancer progression (secondary escape) (19).
In general, the resistance of most cancers to immunotherapies and the lack of anti-tumour memory underline the need to overcome the immunosuppressive microenvironment, improve the immunogenicity of tumour cells and promote the induction of anti-tumour memory, rather than focusing only on stimulating broad and untargeted immune responses (20). In this sense, a novel strategy to induce immune system activation, antitumour memory and tumour microenvironment remodelling is the induction of a specific form of cell death called immunogenic cell death.
3 Immunogenic cell death in cancer
The immunogenicity of cancer cells has been identified as an essential factor in the development of anti-cancer therapies. Therefore, new research has focused on understanding the immunobiology of tumours in order to overcome the immunosuppressive function of the tumour microenvironment (TME) and increase the immunogenicity of cancer cells (21). In this sense, ICD is characterised by the increased immunogenicity of the cells (acting as a tumour vaccine) and the release of DAMPs, leading to the generation of immunological memory (21).
ICD is a type of cell death that can promote the antitumour immune response and induce immunological memory against endogenous (cellular) or exogenous (viral) antigens. The ability of ICD to stimulate adaptive immunity comprises two main parameters: antigenicity and adjuvanticity. Antigenicity is the ability of a molecule, such as a protein, to be recognised as an antigen and to promote an inflammatory response. This is provided by the production and presentation of antigens in the context of central tolerance in a given host that do not lead to clonal deletion, indicating that the host has naive T cell clones that can recognise such antigens. Adjuvanticity is mainly provided by the release or exposure of danger signals such as DAMPs due to cell damage or stress, and by pathogen-associated molecular patterns (PAMPs) in pathogen-derived ICD, which promote the recruitment and maturation of dendritic cells (DCs). These molecules have non-immunological effects within the cell, but, their exposure on the cell surface or their release into the extracellular space due to cellular stress allow their binding to receptors in immune cells (14, 21).
Cancer research has undergone a significant paradigm shift in recent years, with increasing emphasis on the importance of ICD in the context of cancer therapy. Both preclinical and clinical data have converged to support the notion that the way cancer cells undergo cell death in response to treatment carries is more important for long-term disease outcome than the proportion of cells that die. Given the challenge posed by the inability of current cancer therapies to achieve the utopian goal of eradicating 100% of cancer cells, there is a growing consensus among scientists for a strategic shift in focus. Rather than seeking cell death in isolation, the forefront of cancer research is now centred on the development of innovative combination therapeutic regimens designed to stimulate the antitumour immune system and induce cancer cell death (22–24).
Therefore, the use of immunotherapies that can both stimulate the immune system and induce ICD is of great interest because they can enhance the immune system’s ability to fight cancer while killing cancer cells. In the next section, we will focus on describing the role of the main onco-immunotherapies (monoclonal antibodies, cytokines, oncolytic viruses, cellular therapies, and other biological or synthetic immunomodulators) in immunogenic cell death induction and their role in modulating the innate immune system.
4 Dual action of immunotherapy: inducing immunogenic cell death and stimulating the innate immune response
4.1 Monoclonal antibodies
Monoclonal antibody (mAb)-based immunotherapies have recently emerged as one of the most important components of cancer therapy compared to surgery, radiation, and chemotherapy. Novel mAbs have been developed against neoantigens or overexpressed antigens in cancer cells that favour a variety of cell death mechanisms, including ICD (25, 26). The main clinically relevant mechanisms of action induced by mAbs on cancer cells are: antibody-dependent cellular cytotoxicity (ADCC), complement-dependent cytotoxicity (CDC), antibody-dependent cellular phagocytosis (ADCP), which involve the activation of innate cells such as natural killer (NK) cells, dendritic cells, and macrophages (27). As the aim of this review is to focus on immunotherapies that induce ICD, we will describe the principal mAbs that induce ICD and their role in innate immune responses (Figure 1).
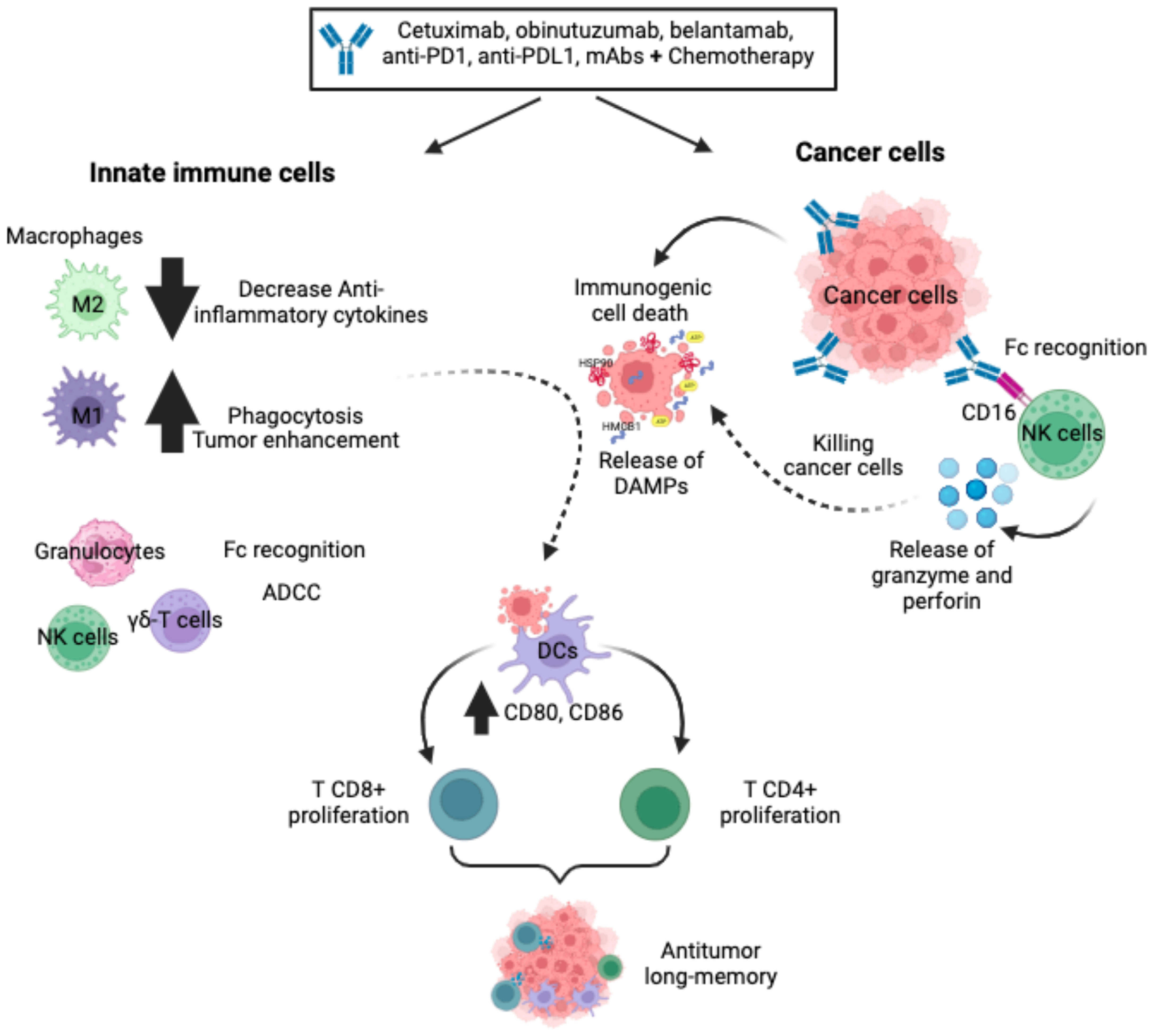
Figure 1 Monoclonal antibodies. The mAbs, alone or in combination with chemotherapy, improve innate cell recruitment, increase non-phagocytic tumour cell killing by neutrophils and NK cells, favour ADCC and reduce anti-inflammatory cytokines. In a variety of cancer cells, mAbs bind specifically to cancer cells, favouring Fc receptor recognition by NK cells and triggering ADCC via activating receptors. In addition, mAbs can induce ICD on cancer cells through the release and exposure of DAMPs to favour phagocytosis by DCs, triggering the stimulation of a protective T-cell (CD4+ and CD8+) memory immune response.
Some types of monoclonal antibodies can induce ICD as monotherapy. For example, belantamab mafodotin is a humanised mAb that targets B-cell maturation antigen (BCMA) in multiple myeloma and other B-cell malignancies. The anti-BCMA is afucosylated and linked to the microtubule polymerization inhibitor, MMAF via a protease-resistant maleimidocaproyl linker. After binding to the cell surface, anti-BCMA is internalised, leading to cell-cycle arrest and apoptosis. This type of cell death triggers cell surface exposure of calreticulin (CRT), heat shock protein 70 and 90 (HSP70, HSP90), and the release of the high mobility group box protein 1 (HMGB1), adenosine triphosphate (ATP), HSP70, and HSP90, triggering activation and maturation of DCs. This leads to host innate and adaptive immune responses through tumour recruitment of cytotoxic T lymphocytes, NK cells and DCs (28). In addition, the afucosylation favours binding to FcgRIIIa receptors on the surface of immune effector cells, which promotes immune cell recruitment, activates ADCC and ADCP and generates long-term immune memory (28). Obinutuzumab (GA101), the second generation of rituximab (anti-CD20 mAb) induces ICD, which is characterised by the release of DAMPs, such as HSP90, HMGB1 and ATP, which induce DCs maturation (enhancing CD86 and CD83 expression) and subsequent T-cell proliferation (29). In addition, GA101 potentiates cellular immune responses by binding to NK cells, promoting their activation and triggering ADCC more effectively than rituximab (30). It is also able to activate γδ T-cells and potentiate killing of lymphoma cells (31), and strongly engage monocytes and M1 macrophages, leading to high levels of nitric oxide and the elimination of CD20-expressing tumour cells (32).
Although some mAbs can induce ICD as a single treatment, most reports include their combination with other immunotherapies or chemotherapies to induce ICD on multiple cancer cells. For example, cetuximab has been shown to induce increased ICD in colorectal cancer when used in combination with leucovorin calcium (folinic acid), fluorouracil, and irinotecan hydrochloride (FOLFIRI). Cetuximab induced endoplasmic reticulum (ER) stress and CRT and ERp57 expression on the cell surface, favouring phagocytosis by DCs of dying cancer cells, which triggered the stimulation of a protective T-cell (CD8+) memory immune response observed alone and in combination with FOLFIRI (33). In addition to its direct ICD induction, cetuximab is able to repolarise tumour-associated macrophages (TAMs) from M2-like to M1-like phenotypes, mainly by suppressing IL-6 expression through NFκB and STAT3 pathways (34).
Among the various antibodies used in combination, anti-PD-1 and anti-PD-L1 are the most used. For example, dinaciclib, a CDK1, -2, 5 and -9 kinase inhibitor, is a bona fide ICD inducer that, when combined with anti-PD1 mAbs, enhances DCs activation and favours antitumour response in a variety of murine syngeneic tumour models (35). Also, photodynamic therapy (PDT) enhances antitumour effects of the anti-PD-L1 mAb, inducing ICD in SCC7 cells by stimulating DCs maturation. In fact, the combination of PDT-DC vaccine and anti-PD-L1 mAb synergistically triggered an antitumour immune response and inhibited tumour progression (36). The PD-L1 mAb in combination with doxorubicin improved the immunosuppressive tumour microenvironment and promoted NK and T cell activation and proliferation. It also increased infiltrating CD8+ T cells through the secretion of CRT and HMGB1, and promoted tumour necrosis factor alpha (TNF-α) and interferon gamma (IFN-γ) production in tumour tissue in a hepatocarcinoma model (37). In addition to these effects, anti-PD-1 and anti-PD-L1 therapies increase the levels of M1-like macrophages markers and promote macrophage polarization towards the pro-inflammatory phenotype (38–40), improve NK cell anti-tumour efficacy and promote NK cell persistence and retention of their cytotoxic phenotype (41, 42). The principal antibodies that were related to ICD induction are summarized in Table 1.
Monoclonal antibodies can also activate cells of the innate immune system, such as NK cells, and trigger ADCC via NK cell-activating receptors, such as CD16. Even polymorphonuclear granulocytes such as eosinophils, neutrophils, macrophages, and monocytes can engage in Fc-mediated effector functions against antibody-opsonized tumour cells through multiple mechanisms (43, 44).
Several antibody therapeutics approved by the Food and Drug Administration (FDA) for various haematological and solid cancers have been reported (45), and have shown to activate innate immune cells. Among these, Rituximab was the first FDA approved mAb against B-cell lymphomas; its therapeutic activity in combination with chemotherapy improves innate cell recruitment (46). Human anti-CD47 antibodies enhance nonphagocytic tumour cell killing by neutrophils and NK cells in an acute myeloid leukaemia (47). Enavatuzumab is a humanized IgG1 antibody that exerts potent ADCC on TweakR positive tumour cells by monocytes and NK cells in vitro (48). Monalizumab, a humanized anti-NKG2A antibody, increased NK cell activity against cancer cells and established CD8+ T cell function in BALB/c mice bearing B cell lymphoma A20 cells in vivo (49).
The potential of mAbs to induce innate antitumour immune responses suggests that it may only be a matter of time before we fully exploit their capabilities to orchestrate a collaborative effort between innate and adaptive immune responses against cancer, ultimately generating long-term antitumour memory (Figure 1).
4.2 Cytokines
Cytokines are molecules that play a central role in cellular autocrine or paracrine signals that are released or produced in response to various stimuli, leading to differentiation, proliferation, activation, cell death and other effects. Cytokines also regulate innate and acquired immune responses such as pro- and anti-tumour effects. Thus, cytokine-based immunotherapies are a promising therapeutic approach that can be used to promote, enhance, maintain or regulate the establishment of an anti-tumour immune system (50).
In general, it has been reported that IL-2, IL-7, IL-12, IL-15, IL-18, and IL-21 induce the expansion and enhance the cytotoxicity of NK, NKT and T lymphocytes, whereas granulocyte macrophage colony-stimulating factor (GM-CSF) and granulocyte-colony stimulating factor (G-CSF) promote the expansion and activation of DCs, and most of them have recently been evaluated in various clinical trials (50, 51). However, most of the cytotoxic or antitumour evaluations of cytokines are in combination with other agents and the direct cytotoxic effect of cytokines in tumour cells is poorly evaluated.
In this sense, the combination of TNF-α and secondary mitochondria-derived activator of caspases (SMAC) mimetics has been reported to induce immunogenic cell death in fibrosarcoma, melanoma, liposarcoma, synovial sarcoma and patient-derived cutaneous squamous cell carcinoma. In vivo, the combination of TNF-α, SMAC mimetics and melphalan induced tumour shrinkage, promoted the activation of CD8+ T cells as well as NK cells and prolonged survival in a rat model of liposarcoma (52).
On the other hand, a cytokine-triggered inflammatory cell death pathway involving crosstalk between the machinery of pyroptosis, apoptosis and necroptosis cell death, termed PANoptosis, has recently been reported. Subbarao et al. showed that a cocktail of pro-inflammatory cytokines including TNF-α, IFN-γ, IL-1α, IL-1β, IL-18, IL-6, IL-8, and IL-15 induced cell death in NCI-60 colon cancer cells, while individual treatments or a cocktail lacking TNF-α and IFN-γ did not induce cell death, suggesting a synergistic effect of this cytokines signalling to induce cell death. The unique combination of TNF-α and IFN-γ induced PANoptosis in different cancer cell types such as colon, melanoma, lung cancer and leukemic cell lines, highlighting the robust cell death induction by TNF-α and IFN-γ in a wide range of cancer cells. Interestingly, the intratumorally administration of a combination of TNF-α and IFN-γ suppresses the tumour growth in a human colon cancer model (53), while independent treatment does not induce these effects. However, although pyroptosis and necroptosis are associated with immunogenicity, immunogenic cell death has not been assessed, but these reports shed light on the possibility that these combinations could lead to ICD.
4.3 Oncolytic viruses
Oncolytic viruses (OVs) are a novel immunotherapy strategy using competent or genetically modified oncolytic viruses that selectively infect, replicate, and induce cell death in tumour cells. OVs have a unique mechanism of action, combining direct tumour cell death, tumour-specific immune response, and antiviral immune system activation. OVs induce oncolysis through the production of viral particles that spread to surrounding tumour cells and promote immune system activation through the release of PAMPs, DAMPs, viral particles and neoantigens (54, 55) (Figure 2). OVs have been reported as ICD inducers and, depending on the type of virus (adenovirus, herpes simplex, semliki forest virus, vaccinia virus, reovirus, among others), they can induce cell death by different mechanisms. These cell death mechanisms include apoptosis, necroptosis, pyroptosis and autophagic cell death, but in general they all induce the exposure and release of DAMPs and PAMPs (54, 56).
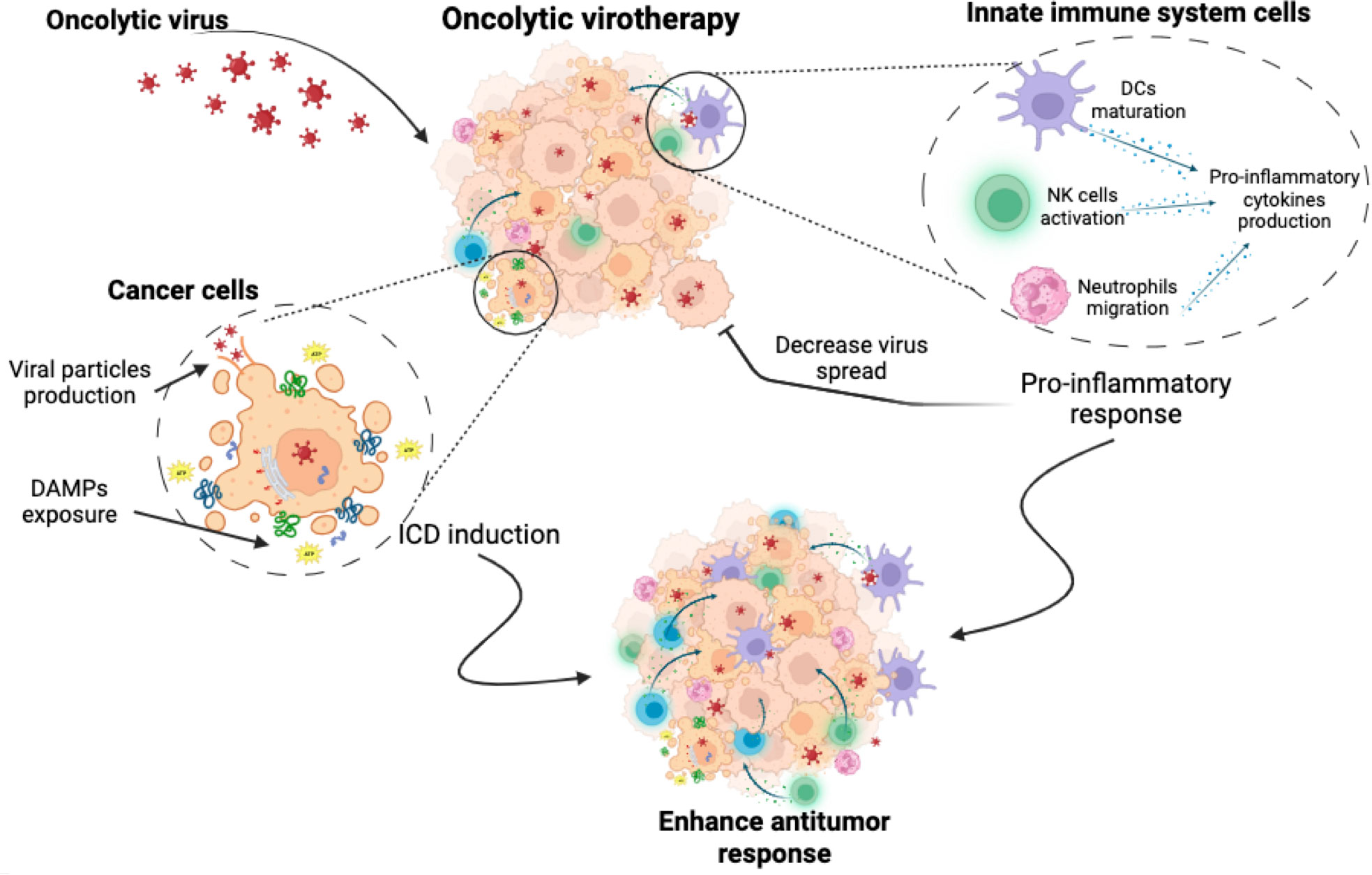
Figure 2 Oncolytic virotherapy. Oncolytic virotherapy has a dual antitumour effect. It induces a direct cytotoxic effect, causing cancer cell death through mechanisms involving the exposure and release of DAMPs, the production of viral particles and finally the induction of immunogenic cell death. At the same time, oncolytic virus triggers the activation of innate immune cells, leading to the stimulation of pro-inflammatory responses. While this may enhance the anti-tumour immune attack, it could also inhibit the virus spread, which could pose a challenge to treatment efficacy.
Currently, a diverse array of OVs has undergone extensive evaluation of their ability to induce ICD across a wide spectrum of tumour models. Notable examples include adenovirus OBP-702 in pancreatic cancer (57), adenovirus dl922-947 in mesothelioma (58), talimogene laherparepvec, and measles virus in melanoma (59), adenovirus serotype 5, semliki forest virus, and vaccinia virus in osteosarcoma (60), reovirus type 3 Dearing strain in lymphoma and prostate cancer (61), adenoviruses, Ad884 and Ad881 in colon cancer (62), oncolytic Newcastle disease virus (NDV) in human lung cancer (63), while herpes virus H-1PV, RH2, and VC2 in pancreas, squamous carcinoma, and melanoma cancer cells (64–66) (Table 2).
These viruses have demonstrated their capacity to trigger the release of critical DAMPs, such as ATP, HMGB1, calreticulin, HSP70, and HSP90 from dying cancer cells. This release enhance the phagocytosis and maturation of DCs, facilitating the infiltration of cytotoxic T cells, and bolstering the NK cell’s antitumor activity, particularly notable in the case of the measles virus, reovirus type 3 Dearing strain, and HSV-P10 (59, 61, 70). This immunogenic response leads to the secretion of pro-inflammatory cytokines such as IFN-γ, TNF-α. Table 2 offers a comprehensive compilation of various research studies delving into the utilization of oncolytic viruses as potent inducers of immunogenic cell death.
In addition to their primary role in killing cancer cells, OVs also serve as potent activators of the innate immune system (71). This is because OVs are pathogens specifically designed to infect and destroy cancer cells. When OVs infect tumour cells, they elicit an inflammatory response, leading to the localized production of cytokines and chemokines that promote the stimulation of the innate immune response trough different mechanisms. These mechanisms include the activation and recruitment of neutrophils (72), macrophages, and NK cells (71). Furthermore, OVs can initiate the activation of PRR in innate cells, triggering the activating receptors such as toll like receptors (TLRs).
Thus ICD-induction by OVs plus the antiviral immune response activated by OVs, promote an immune-stimulatory environment, leading to the uptake of TAAs and neo-antigens by PRR stimulated antigen presenting cells (APCs). Altogether, these events result in a dual immune system activation, on the one hand the generation of immune responses against virally infected cancer cells, and in the other hand immune responses against TAAs and neo-antigens of un-infected cancer cells. Thus, the ‘indirect’ effects of the antiviral immune response within the tumour site, including the release of pro-inflammatory cytokines and the cytotoxicity of infected tumour cells, can reverse the immunosuppressive TME. This in turn may enhance ICD-related properties, including stimulation of innate and adaptive immune cells, release of pro-inflammatory cytokines, and recruitment of immune cells into tumours (63, 73). The anti-viral immunity triggered against viral antigens from the resultant infection is also a key player during OV-based therapies, as tumour cell infection promotes the antiviral immune response, which can be seen as a negative response triggered against OVs, but it helps to settle an inflammatory site that turns “cold” tumours “warm” (74).
However if unbalanced, this immune response could induce premature clearance of OVs and compromise their antitumour efficacy (75), as it has been observed in herpes simplex virus (oHSV) therapy, where activated NK cells reduce the anti-tumour efficacy of HSV in glioblastoma cells (76). In this regard, the combination of transforming growth factor beta (TGFβ) and oHSV therapy inhibits NK cell recruitment and function, resulting in enhanced viral replication in glioblastoma mouse models (77). In addition, the downregulation of the NK cell-activating ligand CD155 inhibited NK cell recruitment in vitro, enhancing viral replication in a rat model of hepatocellular carcinoma (78). IFN-γ and TNF-α secretion also induce virotherapy resistance in different animal models (79, 80), being IFN-γ signalling modulators/inhibitors a strategy used to improve the success of OV treatment (81).
In summary, oncolytic viruses exert a wide range of direct and indirect antitumour effects, including tumour oncolysis, induction of tumour-specific immune responses and activation of the antiviral immune system. These effects culminate in the effective generation of neoantigens and the release of DAMPs and PAMPs, which in turn promote the recruitment of neutrophils, granulocytes, NK cells and APCs to the site of viral replication (56, 72, 81). This in turn enhances the activation of the T- and B-cell-mediated adaptive immune response (56). Finally, these combined effects can potentiate the anti-tumour immune response, which is further enhanced by the induction of ICD, ultimately leading to the establishment of anti-tumour memory.
4.4 Cellular immunotherapies
Cellular immunotherapy, or adoptive cell therapy, is a form of treatment that involves the infusion of live cells into a patient’s body to eliminate cancer. Some of these approaches involve the direct isolation and subsequent expansion of immune cells (T cell-based, NK cell-based, macrophage cell-based, and DC-based), while others use genetic engineering techniques, such as gene therapy, to enhance their cancer-fighting potential (such as chimeric antigen receptor (CAR)-based immunotherapies). CAR-based immunotherapies involve the genetic modification of the cells to confer tumour specificity and include CAR T cells, which comprise the innate T cell subsets such as the γδ T cells (γδCAR T cells) and natural killer T (CAR NKT), CAR NK, and CAR macrophages, which together enhance the potential of cellular immunotherapies (82, 83). In this section, we will focus on the role of cellular immunotherapies in ICD and the activation of innate immune cells.
4.4.1 CAR-based immunotherapies
4.4.1.1 CAR T-cell therapies
CAR T-cell therapy is a highly promising and rapidly advancing treatment approach primarily for haematologic malignancies (84, 85). CAR T cells eliminate cancer cells by binding to target cell surface antigens without the need for MHC restriction. Many articles have reviewed broadly their use, limitations and potential strategies (86–89), while in this review we will principally approach their possibility as ICD inductors, and CAR innate T cell subsets.
Although T cells predominate in CAR-based immunotherapies, innate T cell subsets can also be used for CAR redirection, such as γδCAR T cells and CAR NKT cells. It has been proposed that CARs in innate cells may be preferable than CAR T cells, because of a reduced cytokine release storms (CRS) and graft versus host disease (GvHD). CAR NKT cells simultaneously express the invariant TCR in addition to the CAR, thereby preserving their responsiveness to glycolipid antigens (90). CAR NKT cells in addition to presenting effects on liquid tumours like B-cell lymphoma and multiple myeloma, through CD19, CD38/BMCA, CEA, or HER2 targeting (91, 92), they can also target solid tumours like GD2 in neuroblastoma (93), CSPG4 in melanoma (94) due to their unique capabilities, like high infiltration into TME (91). On the other hand, γδCAR T cells can respond to CD19-positive and -negative tumour cells, suggesting that CD19-directed γδCAR T cells can target leukemic cells even after antigen loss (95). In addition to CD19 targeting, γδCAR T cells have also shown interesting results when targeting glypican-3 in hepatocellular carcinoma (96). In addition, γδCAR T cells produce less IFN-γ and other inflammatory cytokines when compared to conventional αβ CAR T-cells, which may result in a lower risk of CRS (97).
CAR T cells mediate their anti-tumour effects using similar mechanisms as native T cells, such as granular exocytosis and expression of death ligands (98). These mechanisms lead, in native T cells, to different cell death modalities that include apoptosis, necroptosis, pyroptosis, and ferroptosis (99), and can lead to immunogenic cell death in target cancer cells (100, 101). T lymphocytes promote calreticulin exposure, HMGB1 and IL-1β release, leading to DC uptake and cross presentation, providing a mechanism for amplification and self-perpetuation of the immune response against cancer neoantigens (100, 101). They also release cytokines that sensitise the tumour stroma and promote inflammatory signalling, such as IFN-γ which has also the ability to sensitize to cell death and directly trigger cell death alone or combined with other inflammatory molecules, such as TNF-α (99). Although there are no reports specifically describing whether CAR T, γδCAR T or CAR NKT therapies can induce ICD on cancer cells, it is likely that this could occur, as they share common mechanisms of cytotoxicity.
4.4.1.2 CAR NK Cells
Natural killer cells are a vital component of the innate immune system, serving as the frontline defence against infected, transformed, and stressed cells. NK cell activation is initiated by stimulation of an activating receptor, often NK46 encoded by NCR1 (102, 103). Upon activation, NK cells release cytotoxic granules that contain perforin and granzymes to directly lyse cells (104, 105), or regulate the adaptive immune responses by releasing chemokines and cytokines such as IFN-γ and TNF-α. Importantly, NK cells are critical for tumour immunosurveillance, as increased cancer susceptibility and metastasis have been reported in mouse models and clinical trials with low NK activity (106, 107). Due to their inherent ability to target and destroy cancer cells, NK cell-based immunotherapies have been investigated for cancer treatment for decades, including therapies such as CAR NK cell therapy.
A wide range of tumour antigens have been targeted by CAR NK cells in pre-clinical studies for haematological malignancies and solid tumours (108). As for CAR T therapies CD19 is the most common target in CAR NK cells in both preclinical and clinical studies. Also, molecules such as CD20 and Flt3, have been developed as specific targets of CAR-NK against B-cell tumours (109), while CD38, CD138, B-cell maturation antigen, and signalling lymphocytic activation molecule family member 7 have been developed against acute myeloid leukaemia, and CD3, CD5 and CD7 for the cases of T-cell malignancies (110–113). Interestingly, in another therapeutic approach, CAR NK cells seek to eliminate myeloid-derived suppressor cells (MDSCs) (114) and M2TAMs (115) to reverse the immunosuppressive tumour microenvironment.
While detailed analyses of cell death induced by CAR NK cells have not been conducted, it is plausible that they share similar mechanisms with conventional NK cells, which execute cellular cytotoxicity through granule exocytosis and death ligands. NK cells possess the versatile ability to activate diverse cell death pathways, including apoptosis, necroptosis, and pyroptosis, and they are also capable of mediating immunogenic cell death by enhancing dendritic cell uptake of dying cells and facilitating antigen cross-presentation, ultimately leading to the development of immunogenic memory (101, 116). Similar to CAR T cells, there are no reports describing whether CAR NK therapies can induce ICD on cancer cells, but it is likely that this mechanism could also be applicable.
4.4.1.3 CAR macrophages
CAR macrophages (CAR M) are widely recognised as a potential treatment for solid tumours due to their prominent functions in immune regulation and their ability to infiltrate solid tumours. They are currently under clinical investigation as they retain phagocytic and M1 functions while migrating to both primary and metastatic tumours (117). Tumour antigen-specific CARs show significantly enhanced cytotoxicity against tumour antigen-expressing cells and have the potential to remodel the tumour microenvironment (118). CAR constructs used in CAR M cells principally include CD19 in non-solid tumours (119), HER 2 in breast (120) and ovarian cancer cells (121), and mesothelin in ovarian cancer cells (121).
The molecular mechanisms underlying the anti-tumour activity of macrophages are not fully understood. It has been established that macrophages have the ability to eliminate cancer cells through multiple mechanisms, including (1) indirect killing by recruiting cancer cell-killing immune cells such as innate (NK) and adaptive (T) cells (2), cytolysis through antibody (Ab)-dependent cellular cytotoxicity, and (3) direct cancer cell killing by releasing oxygen radicals such as nitric oxide, reactive oxygen species, IL-1β, and TNF-α (122). Although nitic oxide (NO), reactive oxygen species (ROS), IL-1β and TNF-α mediated cell death has been studied in cancer cells and may be associated with ICD induction, this cell death mechanism has not yet been elucidated in macrophage mediated cell death.
4.4.2 Dendritic cells
Dendritic cells are the major APCs that form the link between the innate and adaptive immune systems. These cells efficiently process and present antigens via histocompatibility complex I and II molecules to both innate and adaptive immune cells, thereby triggering the activation of both cellular and humoral immune responses (123).
In addition, DCs play a central role in the activation of the antitumour response during immunogenic cell death. Following the induction of cancer DAMPs exposure or release by various treatments (anthracyclines, oncolytic viruses, anticancer peptides, among others), DCs can be stimulated by different pathways (Table 3).
Due to the immunostimulatory effects of DCs, and their crucial role in the presentation of TAAs, DCs are an excellent means of enhancing the body’s natural anti-tumour responses. Therefore, DC-based immunotherapy focuses on harnessing the potential of DCs to effectively present tumour antigens and induce targeted anti-tumour immune responses.
In DC-based immunotherapy, the source of the DCs (peripheral blood monocytes, haematopoietic precursors, peripheral blood enriched DCs, etc.) and the stimulation with the antigen are crucial steps for the efficacy of the therapy. In addition, different sources of TAAs such as: whole tumour lysates, synthetic peptides, purified tumour antigens, genetically engineered DCs, among others, and different antigen-loading methods are used for stimulation (134). Interestingly, a very important source of TAAs for DC vaccines are cancer cells killed by ICD inducers or strategies. In particular, whole tumour vaccines are crucial for the stimulation of long-term anti-tumour immune responses by DC vaccines, as they serve as a potential reservoir of tumour antigens and could lead to enhanced anti-tumour T-cell responses (135). DCs vaccines loaded with doxorubicin-treated tumour cells are effective in a prophylactic application by reducing tumour development in neuroblastoma (NXS2) and melanoma (B16F10) cell-bearing mice in a prophylactic and therapeutic setting, respectively (136, 137). Also, a DCs vaccine loaded with shikonin (an ICD inductor) treated melanoma cells significantly promoted tumour reduction and improved survival of mice in a therapeutic application (137). Additionally, DCs stimulated with shikonin-treated breast cancer (4T1) cells suppressed metastasis and increased survival of breast cancer cells in an orthotopic tumour resection model (138).
On the other hand, although ICD was initially conceived as a form of chemotherapy-induced tumour cell death, physical anticancer approaches (radiotherapy, photodynamic therapy, among others) have demonstrated the capacity to generate an immune response that can be exploited in DC-based vaccine strategies (125). In this sense, it has been reported that DCs vaccines stimulated with killed squamous cell carcinoma cells by PDT promote tumour reduction and increase the survival of mice showing a better response that the application of tumour cell lysate (139), indicating that the use of DCs enhance the antitumour response. In addition, PDT-based DCs vaccine inhibits the growth of mesothelioma tumours and increases the survival of mice (140).
Furthermore, immunotherapy with DCs is currently being used in combination with ICD inducers. Mice treated with DCs and doxorubicin show an increase in CD8+ T lymphocytes within metastatic tumours and inhibition of metastatic growth (141). Similarly, an increase in serum IL-2, IL-12 and IFN-γ, as well as the proportion of IFN-γ+ CD8+ T cells, was observed in a randomized trial of oesophageal cancer patients treated with DCs vaccine and radiotherapy (142).
Finally, despite the diverse reports of DCs vaccine effects, most of the DCs activities in immunotherapy focus on the activation of the adaptive immune system. However, there are reports on the effect of DCs in different cells of the innate immune system. DCs activate and potentiate the cytotoxic activity of NK cells via IL-12, IL15 and IFN-γ (143). In addition, DCs can activate NKT cells through the expression of invariant CD1 molecules and the presentation of glycolipids (144). Thus, DCs play a critical role in the activation of both innate and adaptive immune responses. However, the activation of innate immune cells by DCs is poorly understood and further evaluation in the context of ICD induction is needed to expand the knowledge of the effect of DCs and to propose more efficient combinatorial treatments.
4.5 Other immunomodulators
4.5.1 Biological immunomodulators
Biological immunomodulators, also called biological therapies are a subset of immunotherapies obtained from biological entities, such as bacillus Calmette-Guérin (BCG), an attenuated Mycobacterium bovis derivative, and dialyzable leukocyte extracts, obtained from immune cells, among others. They are used in several diseases such as autoimmune diseases, viral and bacterial infections and recently in cancer (145, 146).
Bacille-Calmette-Guerin (BCG) is a live attenuated tuberculosis vaccine that is widely used in neonates to induce long-term immunity against pathogens such as Mycobacterium tuberculosis, Candida albicans and Staphylococcus aureus (147). Few reports have described its involvement in the cancer immune response. In this sense, it has been reported that it induces caspase-independent cell death with the release of HMGB1 into the extracellular space in a dose-dependent manner in urothelial carcinoma (UC) T24 and 253J cell lines. The authors also found urinary levels of HMGB1 in patients diagnosticated with UC at 24 hours after BCG therapy (148). In other hand, BCG vaccination triggers innate immune training in several types of immune cells, including monocytes, neutrophils, NK cells and dendritic cells. This training occurs through the interaction of various PRRs with PAMPs present in the bacterial cell wall (149). Immediately, innate cells respond by secreting pro-inflammatory cytokines, including IL-6 IL-1β, TNF-α, monocyte chemoattractant protein- 1 (MCP-1), and IL-8 (150). Consequently, cellular infiltration of T cells (CD3+), monocytes (CD14+), but predominantly CD15+ neutrophils occur at the vaccination site (151). In vitro studies show that human blood neutrophils obtained from BCG vaccination sites cooperate with dendritic cells to enhance antigen-specific T-cell responses (152)Indeed, BCG enhances innate immunity in the context of pathogen protection, however the implication of BCG vaccination and anti-tumour immunity is not yet described (148).
Other types of biological immunomodulators are animal extracts derived from the immune system. This group includes substances produced by immune system cells, also known as dialysable leukocyte extracts (DLE). DLE are a diverse mixture of low-molecular weight compounds derived from blood or lymphoid tissue with immunomodulatory properties (153). Several reports have shown that DLE derived from human blood or lymphoid tissue from different animals (crocodile, porcine or bovine) can regulate numerous molecular targets, thereby facilitating immunomodulatory effects in conditions such as autoimmune diseases, immunodeficiencies, asthma, bacterial infections and certain types of cancer (153–156). The bioactive peptides contained in DLE, irrespective of their source species, have displayed analogous effects on both mouse and human leukocytes, involving the activation of comparable signalling pathways associated with their immunomodulatory properties (155, 157). Recently, DLE have been shown to induce cytotoxicity in several cancer cell lines (156, 158–161).
Immunepotent CRP (I-CRP) is a DLE obtained from bovine spleen (bDLE), which has a wide range of applications in humans. Several studies have shown that it can modulate human and murine immune cells, while inducing cytotoxicity against human and murine tumour cell lines. In particular, its cytotoxic effect has been demonstrated in lung cancer (161), breast cancer (156, 159, 160), murine lymphoma (146), cervical cancer (160, 162) and leukemic cell lines (163). Currently, in a murine melanoma model, I-CRP has been shown to increase the release of DAMPs and the immunogenicity in combination with oxaliplatin (164). It also induces ICD in a murine breast cancer model, involving the DCs maturation in lymph nodes and the increase of CD8+ T cells in lymph nodes, peripheral blood and tumour site, favouring long-term memory (156). On the other hand, in human PBMC, ICRP increased the CD56Dim CD16- subset and modulated NKp30, NKp44, NKp46, NKG2D, NKG2C and KIR receptors, whereas there were no significant differences in CD160, CD85j and CD226 in human NK cells. These alterations revealed increased antitumour cytotoxic activity due to changes in the receptor repertoire of NK cells (155) (Figure 3).
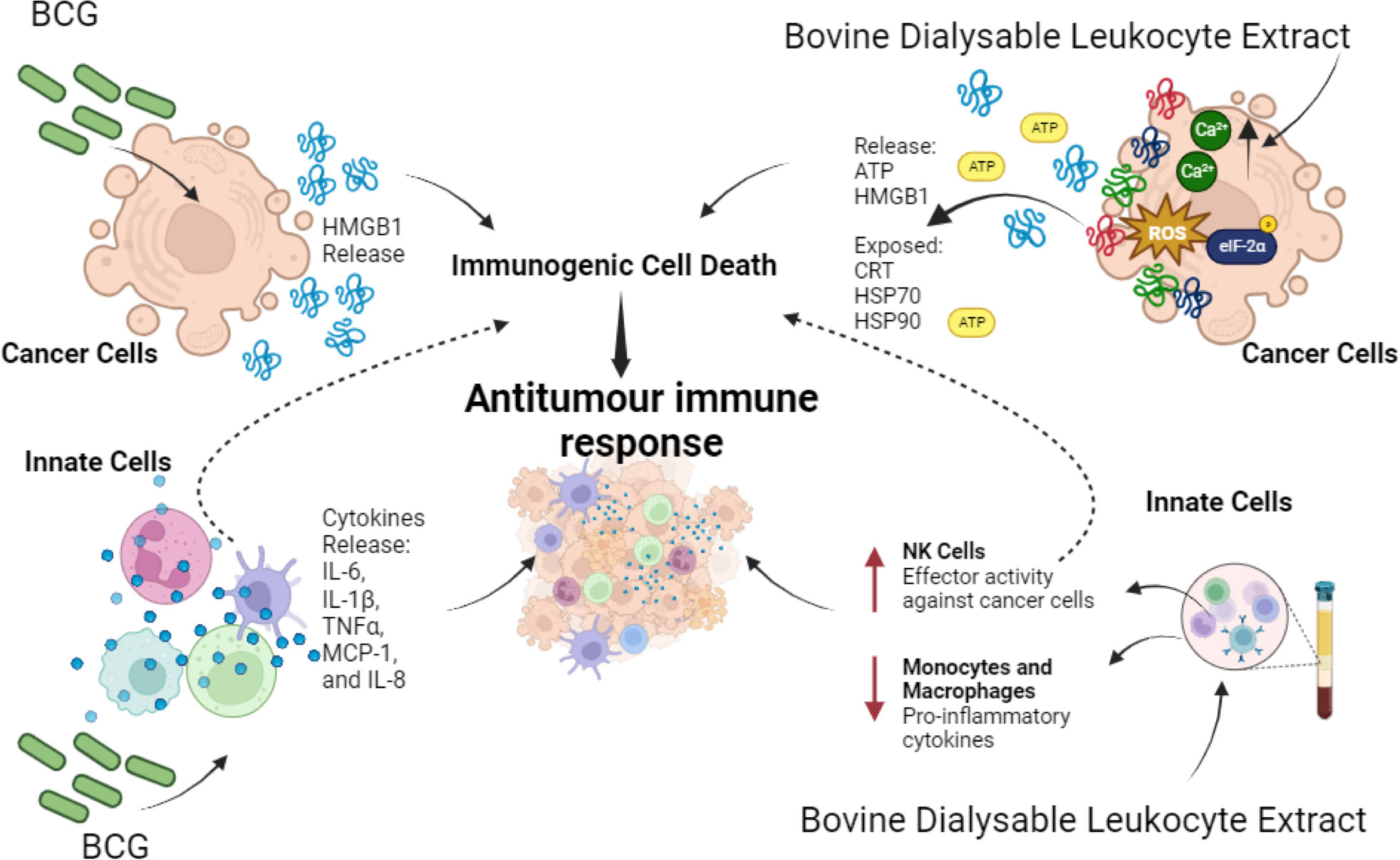
Figure 3 Effect of Biological Immunomodulators on cancer cells and innate immune cells. BCG induces HMGB1 release in cancer cells that promotes ICD. Also, on innate immune cells it induces pro-inflammatory cytokines’ release IL-6, IL-1β, TNF-α, MCP-1, and IL-8. On the other hand, bovine dialysable leukocyte extracts induce cytotoxicity on a variety of cancer cells, in most cases through immunogenic cell death induction, thereby enhancing antitumour immune responses. In parallel it enhances effector activity on NK cells against cancer cells while decreasing proinflammatory cytokines on macrophages and monocytes during lipopolysaccharide (LPS) stimulation.
Another type of DLE, derived from human blood cells (Transferon), suppressed tumour growth and promoted the differentiation of haematopoietic stem/progenitor cells into CD56+CD16+CD11c+ NK-like cells capable of eliminating tumour cells and stimulating the proliferation of γδ T lymphocytes (165). Besides, it decreased metastatic dissemination of intracardiac prostate epithelial cells and prevented tumour establishment of subcutaneous isotransplants. This effect has been associated with high levels of IL-12 and CXCL1, diminution of VEGF levels and changes in tumour infiltration of mononuclear cells and neutrophils (158). Also, Immodin, another human DLE, in combination with manumycin A suppressed tumour growth and prolonged survival in mammary tumour-bearing mice. This combination increased the infiltration of neutrophils and eosinophils into the TME, while independent treatments increased the phagocytic activity of monocytes and neutrophils (166). However, the immunogenicity of cell death has not been evaluated. In Figure 3 we can depict the effect of biological immunomodulators in cancer cells and innate immune cells.
4.5.2 Synthetic immunomodulators
Synthetic immunomodulators are chemical agents that can be derived from diverse sources and can modulate biological responses by interacting with specific cellular targets (167, 168). Synthetic immunomodulators, which may encompass peptides and small molecules, have been used for decades (167–170). They are now being employed in cancer immunotherapy, with a focus on targeting specific surface molecules on cancer cells. These compounds can directly influence signalling pathways and modulate immune cells to selectively target specific types of cancer cells (168, 170). Additionally, some of them show a cytotoxic effect and their role as ICD inducers has recently been explored.
One of these molecules is imiquimod (IMQ), the first member of the immune response modifier family to be approved by the FDA in 1997, for the treatment of external genital and perianal warts (171, 172). IMQ activates toll like receptor 7 (TLR7), which is overexpressed in different types of cancer (173), it also has potent antiviral and antitumour effects as shown in preclinical and clinical studies. Specifically, in human peripheral blood mononuclear cells (PBMC), IMQ has been shown to increase cytokine production including IFN-γ, TNF-α, IL-1, IL-6 and IL-12 by macrophages and monocytes. IMQ also stimulates NK cell activity against skin-infected cells and the activation of macrophages to produce nitric oxide (171, 174). Also, in acute and chronic infectious diseases it promotes anti-inflammatory molecules such as IL-10, and indoleamine 2,3-dioxygenase (IDO) (175–177).
In the context of cancer cells, it can directly induce tumour autophagic cell death in melanoma (178), breast cancer (179) and colorectal cancer (180). It has also been shown that IMQ induces ICD by promoting ROS production, which triggers ER stress followed by surface-exposed CRT, ATP secretion and HMGB1 release (Figure 4), in BCC/KMC-1, AGS, HeLa and B16F10 cancer cells (181). Vaccination with IMQ-killed cancer cells also increased T lymphocyte proliferation, cytotoxic killing and immune cell infiltration into the tumour lesion in an in vivo melanoma model (181). In transgenic mice IMQ promoted breast cancer tumour regression, which progressed at the end of treatment due to CD4+ cells augmentation that enhanced IL-10 levels (182). On the other hand, IMQ could also improve the antitumour immune response by MAA peptide-pulsed DC immunotherapy (183). These effects are due to the stimulation of TLR7 in tumour cells and seem to depend on the type of cancer, the level of TLR7 expression, the downstream function of TLR7 signalling, or chemotaxis of suppressive cells into the tumour (173). Thus, although IMQ has promising cell death inducing and immunomodulatory effects, caution should be taken about these contrary effects reported.
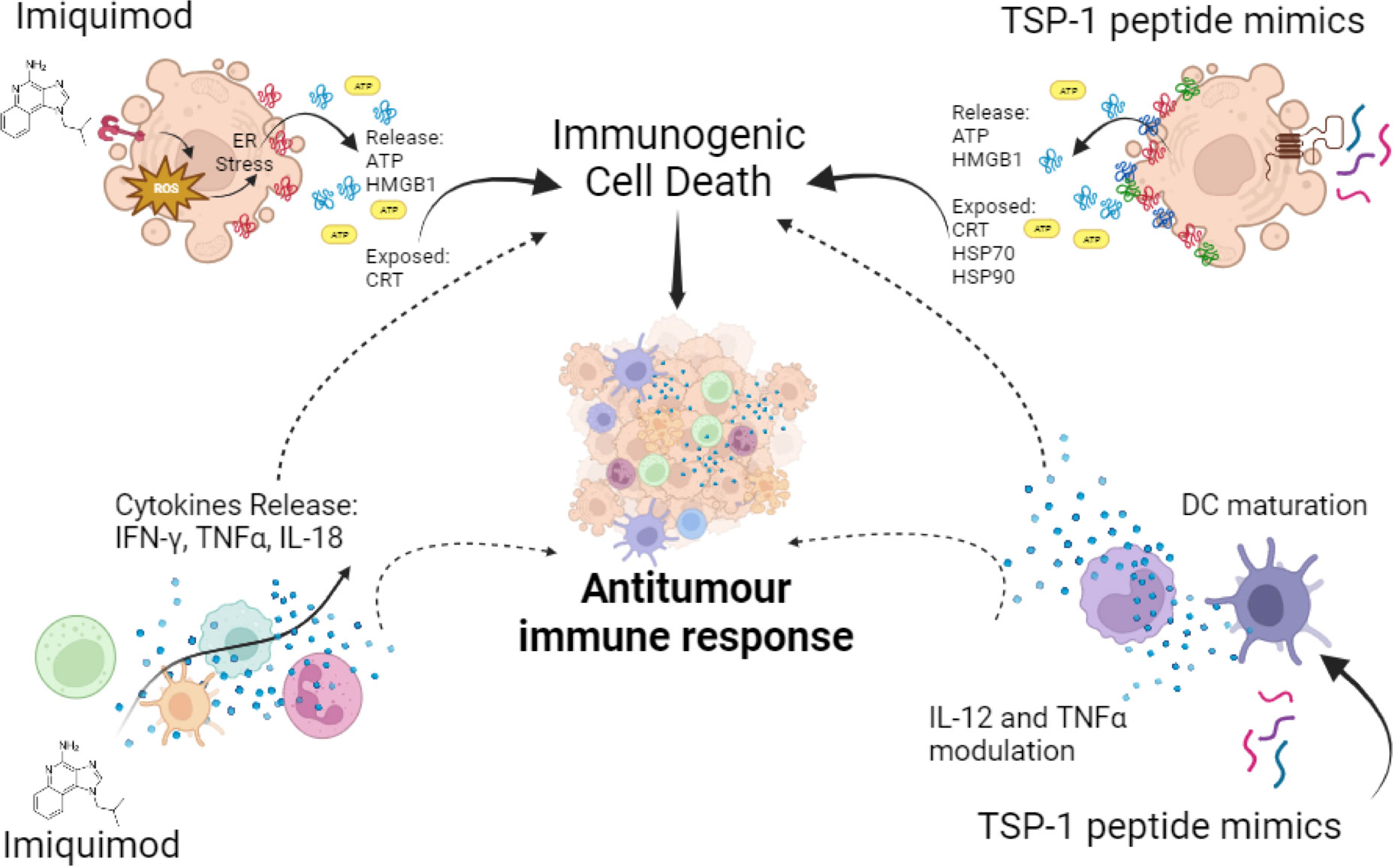
Figure 4 Synthetic immunomodulators in cancer cells and innate immune cells. Imiquimod induces ATP and HMGB1 release and CRT exposure, leading to ICD in cancer cells, also on innate immune cells it induces pro-inflammatory cytokines’ release favouring antitumour immune responses. TSP-1 peptide mimics induce cytotoxicity in a variety of cancer cells leading to ICD, through CRT exposure and ATP, HMGB1, HSP70 and HSP90 release, also in immune cells it induces the modulation of IL-12 and TNF-α and DC maturation. All these effects promote antitumour immune responses.
Other synthetic molecules such as thalidomide, lenalidomide and pomalidomide have demonstrated cytotoxicity in a variety of cancer subsets, however their activity as ICD inductors have not been described (184, 185). Recently, clinical trials have reported improved antitumour responses in multiple myeloma when used alone or in combination with other immunomodulatory agents (186–188). Thalidomide, in particular, was originally synthesised in the late 1950s as a non-addictive, non-barbiturate sedative (189). It is clinically useful in a number of cancers because of its antitumour activity, which is related to the secretion of various cytokines, including IL-2 and IFN-γ as well as inducing T-cell costimulatory and antiangiogenic activities (189, 190). These reports suggest that these molecules can provide satisfactory stimulation of innate immune cells and contribute to cancer elimination through ICD induction.
4.5.2.1 Peptide-based immunotherapies
Peptides are short-chain molecules, typically consisting of less than 50 amino acids. They have applications in the treatment of various conditions such as allergies, infections, tumours, and other diseases. Some peptides can induce cell death in bacteria, fungi, and tumour cells. In particular, peptides are gaining prominence in the field of immunotherapy due to their significant impact on the immune system (191). Therapeutic peptides have found application in immunotherapy, serving various purposes such as cancer vaccines, blocking or inhibiting agents, and inducers of cell death, among others (192). As this review focuses on immunotherapies that induce ICD, we aimed to describe immunotherapeutic peptides that are capable of inducing ICD.
4.5.2.2 Host defence peptides
An important source of ICD-inducing peptides are the host defence peptides (HDP), also known as antimicrobial peptides (AMPs), which are a conserved component of the innate immune system of a wide range of organisms (193), and they have specific physicochemical properties, such as a net positive charge and a specific distribution of cationic and hydrophobic amino acids (194), which enable their electrostatic interaction with cell membranes, membrane proteins or intracellular targets to promote cell lysis or regulated cell death.
Host defence peptides have immunomodulatory properties, such as modulation of inflammatory responses, chemokine expression, activation and differentiation of leukocytes, stimulation of antigen presentation, among others (193). Many HDP also have antitumour activities, most of them related to overcoming the immunosuppressive microenvironment, including: reduction of immunosuppressive cells, migration of phagocytic cells, reduction of pro-tumour molecules, recruitment of antitumour cytotoxic cells, among others (193). Among the HDP, some have been reported as ICD inducers, such as LTX-315 which, in addition to its effect in reducing pro-tumour immune cells, has also been reported to induce the emission of DAMPs (calreticulin, HMGB1 and ATP) and to induce in vivo myeloid and T lymphocyte tumour infiltration (195). The oncolytic peptides DTT-205 and DTT-304 induced calreticulin exposure and HMGB1 release, promoting tumour remission and the development of long-term immune memory against sarcoma and lung cancer cells in vivo (196). In addition, the peptide LTX-401 induced the release of ATP and HMGB1, and induced tumour remission with abscopal effect and promoted the establishment of antitumour memory against hepatocellular carcinoma cells in vivo (197). Taken together, the diverse effects of HDP could enhance their ICD properties to promote the antitumour immune system activation.
4.5.2.3 Thrombospondin-1 peptide mimics
Thrombospondin-1 (TSP-1) mimic peptides are synthetic sequences (natural or modified) designed to mimic the functions of the different motifs in the TSP-1. In this sense, two sequences with a VVM motif were identified within the C-terminal cell-binding domain (CBD) of TSP-1 (198, 199). This led to the generation of 7N3 (1102-FIRVVMYEGKK-1112) and 4N1 (1016-RFYVVMWK-1024) peptides. Then, a modified version of 4N1, called 4N1K (K-RFYVVMWK-K) was developed, containing two lysine (K) residues flanking the 4N1 sequence, to increase the peptide solubility (198, 200).
4N1K was found to induce cell death in leukemic cells in addition to the modulation of cytokines in DCs and microglial cells (201–204). Thus, to improve these effects a structure-activity relationship study led to the synthesis of the first serum-stable analogue of 4N1K, called PKHB1. PKHB1 is recognized as an ICD inducer in leukemic and breast cancer cells. This peptide induces a calcium-dependent and caspase-independent cell death mechanism. Furthermore, PKHB1-induced cell death exhibits key molecular hallmarks of ICD, including the exposure of calreticulin, HSP70, HSP90, and the release of ATP and HMGB1 (Figure 4) in various leukemic and breast cancer cell lines (205–207). Furthermore, PKHB1-treated cells promote DC maturation and stimulate the antitumour response of T-cells ex vivo. In a prophylactic context, PKHB1-treated cells prevent the tumour establishment in leukemic and breast cancer tumour bearing mice. PKHB1 also induce tumour shrinkage, increasing cytotoxic T-cell counts in blood and tumours, while reducing MDSCs and regulatory T-cells (T-regs) in breast cancer tumour-bearing mice (207). Notably, PKHB1 possess antiviral properties by triggering ICD in cases of infectious corneal disease caused by Herpes simplex virus type II. This event triggers an antiviral immune response, that reduces viral levels and mitigates the severity of the infection (208). Finally, PKHB1 also promotes the elimination of inflammatory macrophages in models of subretinal and peritoneal inflammation (209). Despite the evaluation of the immunogenicity and the antitumor effect of PKHB1, its impact on innate immune system cells and their role in the antitumor activity of PKHB1 have not been evaluated to date.
4.5.2.4 Other peptides as ICD-inductors
F-pY-T is a mitochondria-targeting peptide that has been reported as an ICD inducer, triggering calreticulin exposure (in vitro and in vivo), ATP and HMGB1 release. F-pY-T in vivo induced DC maturation and promoted the intratumoral infiltration of CD8+ cells, and inhibited tumour growth (210).
The recombinant human milk peptide lactaptin RL2 induced calreticulin exposure, ATP and HMGB1 release in breast cancer cells and promoted the phagocytosis of dying-cancer cells by macrophages. In vivo, vaccination with RL2-treated cells also increased the survival of mice (211).
The calmodulin binding peptide CBP501 has been reported as an ICD inducer, which promotes calreticulin exposure and HMGB1 release, and increases in vivo mouse survival in vaccination experiments (in combination with cisplatin).Also, the combination of cisplatin and CBP501 also reduces tumour growth and increases intratumoral CD8+ cell infiltration (212).
Other peptides that have been demonstrated to possess immunomodulatory proprieties and induce immunogenic cell death are peptide-based proteasome inhibitors. Proteasome inhibitors are a class of drugs whose main mechanism is to inhibit the multi-protease subunits of the proteosome, leading to the accumulation of undegraded proteins, affecting different cellular processes which lead to cell death (213). Bortezomib is a dipeptide boronic acid derivative that acts as a reversible inhibitor of the 26S proteasome and is the first FDA approved proteosome inhibitor (214). It has been shown to have various immunomodulatory effects in allogeneic stem cell transplantation, antibody-mediated graft rejection and various inflammatory diseases (215). Furthermore, it is considered an ICD inducer, as it (1) prevents breast cancer tumour establishment (216) (2); promotes HSP90 exposure, DC maturation and antitumour T-cell response against myeloma cells from patients (217) (3); triggers calreticulin exposure, induces DCs maturation (increase of CD83 and CD86) and the antitumour T-cell response, increasing the number of effector memory CD4+ and CD8+ cells (4); the in vivo vaccination with bortezomib-treated cells prevents tumour establishment and promotes long-term antitumour memory against multiple myeloma cells (218).
5 Discussion and concluding remarks
Since 1891, when Coley used the first immunotherapies, there have been tremendous advances and discoveries that have revealed the promising potential of immunotherapies for the prevention and treatment of cancer. Although these are usually combined with other cancer treatments capable of killing cancer cells to attack cancer cells from different perspectives, some immunotherapies have the capacity to be cytotoxic to cancer cells through immunogenic cell death induction. Although few in number, these ICD-inducing immunotherapies represent a promising and innovative approach in the fight against cancer, with the innate immune system playing a key role in their success.
ICD has the potential to induce a robust antitumor immune response (219). However, the principal challenge is associated with treatment resistance, which could hamper its therapeutic efficacy. This may be related with the ICD induction which depends on the host (for example immune perception of ICD), the tumour (for example DAMPs’ exposure), the ICD inductor (for example, its immunosuppressive effects), or the specific cancer microenvironment (the specific immunosuppressive cells present in the TME) (220). Cell death resistance could be addressed by combination regimens of therapeutic alternatives that could attack from different sources. For example, it has been demonstrated that bortezomib improves adoptive T cell therapy by sensitizing cancer cells to FasL cytotoxicity (221). Also, oncolytic viruses provide potent antitumor effects against brain tumours when combined with adoptive T-cell therapy (222). While, bovine dialyzable leukocyte extract, which induces ICD in breast cancer, when combined with cyclophosphamide induces synergic cell death (223). The combination of chemotherapy with immunotherapies is a primary approach evaluated to overcome cancer cell resistance (224–226). However, these combinations mostly look for ICD inducing chemotherapies with non-necessarily ICD inducing immunotherapies, yet combination of different ICD-inducing agents might promote better responses. Especially if combining immunotherapeutic and chemotherapeutic agents that possess ICD potential and immunomodulatory properties, as it has also been described for certain types of chemotherapies (227, 228). Immunotherapies may help to surpass tumour resistance mechanisms, as immunotherapies that stimulate the innate immune system may augment ICD by enhancing the effect triggered by DAMPs, thereby promoting a robust immune response (229). Additionally, they may activate DCs, enhancing antigen presentation and promoting the recruitment and activation of effector immune cells (125), like NK cells, which in turn can efficiently trigger cellular cytotoxicity, potentially leading to ICD (101). This synergy is a promising alternative to overcome ICD resistance, providing an interesting avenue for enhanced antitumor effects and improved therapeutic outcomes.
The intricate interplay between ICD and the innate immune response opens new avenues for the development of more effective and durable cancer treatments with promising potential. These onco-immunotherapies, including monoclonal antibodies, cytokines, oncolytic viruses, cellular immunotherapies, and other biological or synthetic immunomodulators, have clearly demonstrated their potential to harness the body’s natural defences against cancer cells. By triggering the ICD, these treatments also facilitate the release of tumour antigens and danger signals, stimulating innate immune cells such as dendritic cells, natural killer cells and macrophages. Activation of these innate first-line defence cells is critical for mounting a potent and sustained anti-cancer response, which involves the durable long-term memory of the adaptive immune system (Figure 5).
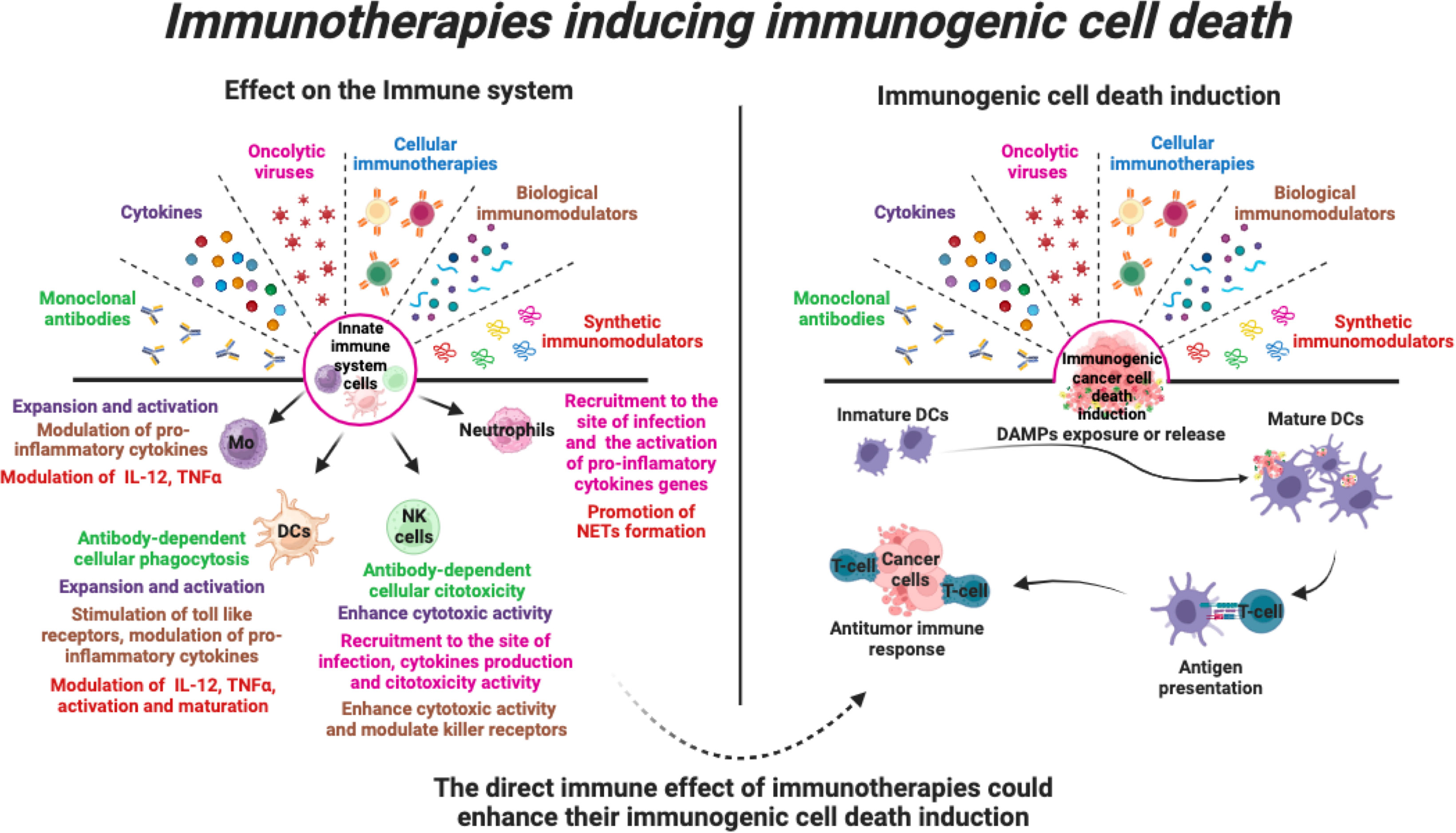
Figure 5 Immunotherapies that induce immunogenic cell death. Various immunotherapies promote the stimulation of various pro-inflammatory responses in cells of the innate immune system. In addition, immunotherapies can induce direct cell death of tumour cells through induction of immunogenic cell death, leading to activation of the anti-tumour immune system. Thus, the direct effect of immunotherapies on innate immune cells could enhance their ICD induction and enhance the anti-tumour immune response.
As these potential actions of immunotherapies have not been the primary focus, several challenges remain. For example, uncovering the potential role of immunotherapies in inducing immunogenic cell death is a significant challenge, given that only a few of them have been studied as ICD inducers. This is particularly striking when compared to the large body of evidence highlighting their role in the immune system. Another aspect is not only to elucidate their role in ICD induction, but also to propose combinations that enhance this dual action for a more comprehensive approach against a wide range of cancers. In conclusion, we recommend that these strategies be emphasised, as addressing these aspects will undoubtedly contribute to a deeper understanding, more effective use and further development of the enormous potential offered by these immunotherapies.
Author contributions
KC-R: Data curation, Investigation, Methodology, Validation, Writing – original draft, Writing – review and editing. HL-A: Data curation, Investigation, Methodology, Writing – original draft, Writing – review and editing. CR-P: Writing – review and editing. AM-T: Conceptualization, Data curation, Investigation, Methodology, Validation, Writing – original draft, Writing – review and editing. DS-A: Conceptualization, Validation, Writing – review and editing.
Funding
The author(s) declare financial support was received for the research, authorship, and/or publication of this article. ACMT and KMCR thank CONAHCYT, ANUIES, and ECOSNORD for financial support. DS-A thanks Agence Nationale de recherche sur le Sida, Hépatites Virales et Maladies Infectieuses Émergentes (ANRS-MIE) for financial support.
Acknowledgments
The authors would like to apologise to researchers whose relevant publications were not referenced due to the scope of this review, or who may have been inadvertently overlooked. Figures were created using BioRender.com.
Conflict of interest
The authors declare that the research was conducted in the absence of any commercial or financial relationships that could be construed as a potential conflict of interest.
The author(s) declared that they were an editorial board member of Frontiers, at the time of submission. This had no impact on the peer review process and the final decision.
Publisher’s note
All claims expressed in this article are solely those of the authors and do not necessarily represent those of their affiliated organizations, or those of the publisher, the editors and the reviewers. Any product that may be evaluated in this article, or claim that may be made by its manufacturer, is not guaranteed or endorsed by the publisher.
Glossary
References
1. Couzin-Frankel J. Breakthrough of the year 2013. Cancer Immunother Science. (2013) 342(6165):1432–3. doi: 10.1126/science.342.6165.1432
2. Galluzzi L, Kepp O, Hett E, Kroemer G, Marincola FM. Immunogenic cell death in cancer: concept and therapeutic implications. J Trans Med (2023) 21(1):162. doi: 10.1186/s12967-023-04017-6
3. Li L, Li Y, Yang CH, Radford DC, Wang J, Janát-Amsbury M, et al. Inhibition of immunosuppressive tumors by polymer-assisted inductions of immunogenic cell death and multivalent PD-L1 crosslinking. Adv Funct Mater (2020) 30(12):1908961. doi: 10.1002/adfm.201908961
4. Xu J, Xiong Y, Xu Z, Xing H, Zhou L, Zhang X. From targeted therapy to a novel way: Immunogenic cell death in lung cancer. Front Med (2022) 9. doi: 10.3389/fmed.2022.1102550
5. Chang X, Bian M, Liu L, Yang J, Yang Z, Wang Z, et al. Induction of immunogenic cell death by novel platinum-based anticancer agents. Pharmacol Res (2023) 187:106556. doi: 10.1016/j.phrs.2022.106556
6. Bao X, Xie L. Targeting purinergic pathway to enhance radiotherapy-induced immunogenic cancer cell death. J Exp Clin Cancer Res (2022) 41(1):222. doi: 10.1186/s13046-022-02430-1
7. Procureur A, Simonaggio A, Bibault JE, Oudard S, Vano YA. Enhance the immune checkpoint inhibitors efficacy with radiotherapy induced immunogenic cell death: A comprehensive review and latest developments. Cancers (2021) 13(4):678. doi: 10.3390/cancers13040678
8. Decraene B, Yang Y, De Smet F, Garg AD, Agostinis P, De Vleeschouwer S. Immunogenic cell death and its therapeutic or prognostic potential in high-grade glioma. Genes Immun (2022) 23(1):1–11. doi: 10.1038/s41435-021-00161-5
9. Coley Md WB. The treatment of Malignant tumors by repeated inoculations of erysipelas: With a report of ten original cases. Clin Orthop Relat Res (1893) 262):3–11. doi: 10.1097/00000658-189307000-00009
10. Richardson MA, Ramirez T, Russell NC, Moye LA. Coley toxins immunotherapy: A retrospective review. Altern Ther Health Med (1999) 5(3):42–7.
11. Kienle GS. Fever in cancer treatment: Coley’s therapy and epidemiologic observations. Global Adv In Health Med (2012) 1(1):92–100. doi: 10.7453/gahmj.2012.1.1.016
12. Wiemann B, Starnes CO. Coley’s toxins, tumor necrosis factor and cancer research: A historical perspective. Pharmacol Ther (1994) 64(3):529–64. doi: 10.1016/0163-7258(94)90023-x
13. Hanahan D. Hallmarks of cancer: new dimensions. Cancer Discovery (2022) 12(1):31–46. doi: 10.1158/2159-8290.CD-21-1059
14. Garg AD, Agostinis P. Cell death and immunity in cancer: From danger signals to mimicry of pathogen defense responses. Immunol Rev (2017) 280(1):126–48. doi: 10.1111/imr.12574
15. Garg AD, Dudek AM, Ferreira GB, Verfaillie T, Vandenabeele P, Krysko DV, et al. ROS-induced autophagy in cancer cells assists in evasion from determinants of immunogenic cell death. Autophagy. (2013) 9(9):1292–307. doi: 10.4161/auto.25399
16. Lee YT, Tan YJ, Oon CE. Molecular targeted therapy: Treating cancer with specificity. Eur J Pharmacol (2018) 834:188–96. doi: 10.1016/j.ejphar.2018.07.034
17. Zhang Y, Zhang Z. The history and advances in cancer immunotherapy: understanding the characteristics of tumor-infiltrating immune cells and their therapeutic implications. Cell Mol Immunol (2020) 17(8):807–21. doi: 10.1038/s41423-020-0488-6
18. Kennedy LB, Salama AKS. A review of cancer immunotherapy toxicity. CA Cancer J Clin (2020) 70(2):86–104. doi: 10.3322/caac.21596
19. Gubin MM, Vesely MD. Cancer immunoediting in the era of immuno-oncology. Clin Cancer Res (2022) 28(18):3917–28. doi: 10.1158/1078-0432.CCR-21-1804
20. Tan S, Li D, Zhu X. Cancer immunotherapy: Pros, cons and beyond. Biomedicine Pharmacotherapy (2020) 124:109821. doi: 10.1016/j.biopha.2020.109821
21. Kroemer G, Galluzzi L, Kepp O, Zitvogel L. Immunogenic cell death in cancer therapy. Annu Rev Immunol (2013) 31:51–72. doi: 10.4161/onci.26536
22. Asadzadeh Z, Safarzadeh E, Safaei S, Baradaran A, Mohammadi A, Hajiasgharzadeh K, et al. Current approaches for combination therapy of cancer: The role of immunogenic cell death. Cancers (2020) 12(4):1047. doi: 10.3390/cancers12041047
23. Vanneman M, Dranoff G. Combining immunotherapy and targeted therapies in cancer treatment. Nat Rev Cancer (2012) 12(4):237–51. doi: 10.1038/nrc3237
24. Fabian KP, Kowalczyk JT, Reynolds ST, Hodge JW. Dying of stress: chemotherapy, radiotherapy, and small-molecule inhibitors in immunogenic cell death and immunogenic modulation. Cells (2022) 11(23):3826. doi: 10.3390/cells11233826
25. Tsao LC, Force J, Hartman ZC. Mechanisms of therapeutic antitumor monoclonal antibodies. Cancer Res (2021) 81(18):4641–51. doi: 10.1158/0008-5472.CAN-21-1109
26. Trenevska I, Li D, Banham AH. Therapeutic antibodies against intracellular tumor antigens. Front Immunol (2017) 8. doi: 10.3389/fimmu.2017.01001
27. Chester C, Marabelle A, Houot R, Kohrt HE. Dual antibody therapy to harness the innate anti-tumor immune response to enhance antibody targeting of tumors. Curr Opin Immunol (2015) 33:1–8. doi: 10.1016/j.coi.2014.12.010
28. Montes de Oca R, Alavi AS, Vitali N, Bhattacharya S, Blackwell C, Patel K, et al. Belantamab mafodotin (GSK2857916) drives immunogenic cell death and immune-mediated antitumor responses in vivo. Mol Cancer Ther (2021) 20(10):1941–55. doi: 10.1158/1535-7163.MCT-21-0035
29. Cheadle EJ, Sidon L, Dovedi SJ, Melis MHM, Alduaij W, Illidge TM, et al. The induction of immunogenic cell death by type II anti-CD20 monoclonal antibodies has mechanistic differences compared with type I rituximab. Br J Haematology (2013) 162(6):842–5. doi: 10.1111/bjh.12427
30. Kern DJ, James BR, Blackwell S, Gassner C, Klein C, Weiner GJ. GA101 induces NK-cell activation and antibody-dependent cellular cytotoxicity more effectively than rituximab when complement is present. Leuk Lymphoma. (2013) 54(11):2500–5. doi: 10.3109/10428194.2013.781169
31. Braza MS, Klein B, Fiol G, Rossi JF. γδT-cell killing of primary follicular lymphoma cells is dramatically potentiated by GA101, a type II glycoengineered anti-CD20 monoclonal antibody. Haematologica. (2011) 96(3). doi: 10.3324/haematol.2010.029520
32. Herter S, Klein C, Umana P, Bacac M. Obinutuzumab (GA101) more potently engages phagocytic-lineage cells resulting in enhanced monocyte and macrophage activity when compared to rituximab and ofatumumab. Blood. (2013) 122(21):5136. doi: 10.1182/blood.V122.21.5136.5136
33. Pozzi C, Cuomo A, Spadoni I, Magni E, Silvola A, Conte A, et al. The EGFR-specific antibody cetuximab combined with chemotherapy triggers immunogenic cell death. Nat Med (2016) 22(6):624–31. doi: 10.1038/nm.4078
34. Zhao Y, Liu X, Huo M, Wang Y, Li Y, Xu N, et al. Cetuximab enhances the anti-tumor function of macrophages in an IL-6 dependent manner. Life Sci (2021) 267:118953. doi: 10.1016/j.lfs.2020.118953
35. Md Sakib Hossain D, Javaid S, Cai M, Zhang C, Sawant A, Hinton M, et al. Dinaciclib induces immunogenic cell death and enhances anti- PD1–mediated tumor suppression. J Clin Invest. (2018) 128(2):644–54. doi: 10.1172/JCI94586
36. Li S, Wang D, Cheng J, Sun J, Kalvakolanu DV, Zhao X, et al. A photodynamically sensitized dendritic cell vaccine that promotes the anti-tumor effects of anti-PD-L1 monoclonal antibody in a murine model of head and neck squamous cell carcinoma. J Transl Med (2022) 20(1):505. doi: 10.1186/s12967-022-03707-x
37. Chen Y, Luo X, Liu Y, Zou Y, Yang S, Zhao Y, et al. Targeted nanobubbles of PD-L1 mAb combined with doxorubicin as a synergistic tumor repressor in hepatocarcinoma. Int J Nanomedicine (2022) 17:3989–4008. doi: 10.2147/IJN.S376172
38. Zhang H, Liu L, Liu J, Dang P, Hu S, Yuan W, et al. Roles of tumor-associated macrophages in anti-PD-1/PD-L1 immunotherapy for solid cancers. Mol Cancer (2023) 22(1):58. doi: 10.1186/s12943-023-01725-x
39. Xiong H, Mittman S, Rodriguez R, Moskalenko M, Pacheco-Sanchez P, Yang Y, et al. Anti-PD-L1 treatment results in functional remodeling of the macrophage compartment. Cancer Res (2019) 79(7):1493–506. doi: 10.1158/0008-5472.CAN-18-3208
40. Dhupkar P, Gordon N, Stewart J, Kleinerman ES. Anti-PD-1 therapy redirects macrophages from an M2 to an M1 phenotype inducing regression of OS lung metastases. Cancer Med (2018) 7(6):2654–64. doi: 10.1002/cam4.1518
41. Oyer JL, Gitto SB, Altomare DA, Copik AJ. PD-L1 blockade enhances anti-tumor efficacy of NK cells. Oncoimmunology (2018) 7(11):e1509819. doi: 10.1080/2162402X.2018.1509819
42. Chung YM, Khan PP, Wang H, Bin TW, Qiao Y, Yu B, et al. Sensitizing tumors to anti-PD-1 therapy by promoting NK and CD8+ T cells via pharmacological activation of FOXO3. J Immunother Cancer (2021) 9(12):e002772. doi: 10.1136/jitc-2021-002772
43. Moynihan KD, Irvine DJ. Roles for innate immunity in combination immunotherapies. Cancer Res (2017) 77(19):5215–21. doi: 10.1158/0008-5472.CAN-17-1340
44. Malik B, Ghatol A. Understanding how monoclonal antibodies work. In: StatPearls [Internet]. Treasure Island (FL):StatPearls (2021).
45. The Antibody Society. Antibody therapeutics approved or in regulatory review in the EU or US. The Antibody Society (2023). Available at: https://www.antibodysociety.org/resources/approved-antibodies (Accessed May 2023).
46. Liang Y, Tedder TF. Identification of a CD20-, Fc∈RIβ-, and HTm4-related gene family: Sixteen new MS4A family members expressed in human and mouse. Genomics. (2001) 72(2):119–27. doi: 10.1006/geno.2000.6472
47. Luo X, Shen Y, Huang W, Bao Y, Mo J, Yao L, et al. Blocking CD47-SIRPα Signal axis as promising immunotherapy in ovarian cancer. Cancer Control. (2023) 30:10732748231159706. doi: 10.1177/10732748231159706
48. Ye S, Fox MI, Belmar NA, Sho M, Chao DT, Choi D, et al. Enavatuzumab, a humanized anti-TWEAK receptor monoclonal antibody, exerts antitumor activity through attracting and activating innate immune effector cells. J Immunol Res (2017) 2017:5737159. doi: 10.1155/2017/5737159
49. André P, Denis C, Soulas C, Bourbon-Caillet C, Lopez J, Arnoux T, et al. Anti-NKG2A mAb is a checkpoint inhibitor that promotes anti-tumor immunity by unleashing both T and NK cells. Cell. (2018) 175(7):1731–43.e13. doi: 10.1016/j.cell.2018.10.014
50. Berraondo P, Sanmamed MF, Ochoa MC, Etxeberria I, Aznar MA, Pérez-Gracia JL, et al. Cytokines in clinical cancer immunotherapy. Br J Cancer (2019) 120(1):6–15. doi: 10.1038/s41416-018-0328-y
51. Qiu Y, Su M, Liu L, Tang Y, Pan Y, Sun J. Clinical application of cytokines in cancer immunotherapy. Drug Design Dev Ther (2021) 15:2269–87. doi: 10.2147/DDDT.S308578
52. Smith HG, Jamal K, Dayal JH, Tenev T, Kyula-Currie J, Guppy N, et al. RIPK1-mediated immunogenic cell death promotes anti-tumour immunity against soft-tissue sarcoma. EMBO Mol Med (2020) 12(6):e10979. doi: 10.15252/emmm.201910979
53. Malireddi RKS, Karki R, Sundaram B, Kancharana B, Lee S, Samir P, et al. Inflammatory cell death, PANoptosis, mediated by cytokines in diverse cancer lineages inhibits tumor growth. ImmunoHorizons. (2021) 5(7):568–80. doi: 10.4049/immunohorizons.2100059
54. Guo ZS, Liu Z, Bartlett DL. Oncolytic immunotherapy: Dying the right way is a key to eliciting potent antitumor immunity. Front Oncol (2014) 4. doi: 10.3389/fonc.2014.00074
55. Mardi A, Shirokova AV, Mohammed RN, Keshavarz A, Zekiy AO, Thangavelu L, et al. Oncolytic viruses: priming time for cancer immunotherapy. Cancer Cell Int (2022) 22(1):168. doi: 10.1186/s12935-022-02585-z
56. Russell L, Peng KW, Russell SJ, Diaz RM. Oncolytic viruses: priming time for cancer immunotherapy. BioDrugs (2019) 33(5):485–501. doi: 10.1007/s40259-019-00367-0
57. Araki H, Tazawa H, Kanaya N, Kajiwara Y, Yamada M, Hashimoto M, et al. Oncolytic virus-mediated p53 overexpression promotes immunogenic cell death and efficacy of PD-1 blockade in pancreatic cancer. Mol Ther - Oncolytics. (2022) 27:3–13. doi: 10.1016/j.omto.2022.09.003
58. Di Somma S, Iannuzzi CA, Passaro C, Forte IM, Iannone R, Gigantino V, et al. The oncolytic virus dl922-947 triggers immunogenic cell death in mesothelioma and reduces Xenograft growth. Front Oncol (2019) 9. doi: 10.3389/fonc.2019.00564
59. Donnelly OG, Errington-Mais F, Steele L, Hadac E, Jennings V, Scott K, et al. Measles virus causes immunogenic cell death in human melanoma. Gene Ther (2013) 20(1):7–15. doi: 10.1038/gt.2011.205
60. Ma J, Ramachandran M, Jin C, Quijano-Rubio C, Martikainen M, Yu D, et al. Characterization of virus-mediated immunogenic cancer cell death and the consequences for oncolytic virus-based immunotherapy of cancer. Cell Death Dis (2020) 11(1):48. doi: 10.1038/s41419-020-2236-3
61. Errington F, Steele L, Prestwich R, Harrington KJ, Pandha HS, Vidal L, et al. Reovirus activates human dendritic cells to promote innate antitumor immunity. J Immunol (2008) 180(9):6018–26. doi: 10.4049/jimmunol.180.9.6018
62. Yamano T, Kubo S, Fukumoto M, Yano A, Mawatari-Furukawa Y, Okamura H, et al. Whole cell vaccination using immunogenic cell death by an oncolytic adenovirus is effective against a colorectal cancer model. Mol Ther - Oncolytics. (2016) 3:16031. doi: 10.1038/mto.2016.31
63. Ye T, Jiang K, Wei L, Barr MP, Xu Q, Zhang G, et al. Oncolytic Newcastle disease virus induces autophagy-dependent immunogenic cell death in lung cancer cells. Am J Cancer Res (2018) 8(8):1514–27.
64. Angelova AL, Grekova SP, Heller A, Kuhlmann O, Soyka E, Giese T, et al. Complementary induction of immunogenic cell death by oncolytic parvovirus H-1PV and gemcitabine in pancreatic cancer. J Virol (2014) 88(10):5263–76. doi: 10.1128/JVI.03688-13
65. Takasu A, Masui A, Hamada M, Imai T, Iwai S, Yura Y. Immunogenic cell death by oncolytic herpes simplex virus type 1 in squamous cell carcinoma cells. Cancer Gene Ther (2016) 23(4):107–13. doi: 10.1038/cgt.2016.8
66. Uche IK, Fowlkes N, Vu L, Watanabe T, Carossino M, Nabi R, et al. Novel oncolytic herpes simplex virus 1 VC2 promotes long-lasting, systemic anti-melanoma tumor immune responses and increased survival in an immunocompetent B16F10-derived mouse melanoma model. J Virol (2021) 95(3):e01359–20. doi: 10.1128/JVI.01359-20
67. Bommareddy PK, Zloza A, Rabkin SD, Kaufman HL. Oncolytic virus immunotherapy induces immunogenic cell death and overcomes STING deficiency in melanoma. Oncoimmunology. (2019) 8(7):1591875. doi: 10.1080/2162402X.2019.1591875
68. Annels NE, Simpson GR, Denyer M, Arif M, Coffey M, Melcher A, et al. Oncolytic reovirus-mediated recruitment of early innate immune responses reverses immunotherapy resistance in prostate tumors. Mol Ther - Oncolytics. (2021) 20:434–46. doi: 10.1016/j.omto.2020.09.010
69. van de Merbel AF, van der Horst G, van der Mark MH, Bots STF, van den Wollenberg DJM, de Ridder CMA, et al. Reovirus mutant jin-3 exhibits lytic and immune-stimulatory effects in preclinical human prostate cancer models. Cancer Gene Ther (2022) 29(6):793–802. doi: 10.1038/s41417-021-00360-2
70. Russell L, Swanner J, Jaime-Ramirez AC, Wang Y, Sprague A, Banasavadi-Siddegowda Y, et al. PTEN expression by an oncolytic herpesvirus directs T-cell mediated tumor clearance. Nat Commun (2018) 9(1):5006. doi: 10.1038/s41467-018-07344-1
71. Worschech A, Chen N, Yu YA, Zhang Q, Pos Z, Weibel S, et al. Systemic treatment of xenografts with vaccinia virus GLV-1h68 reveals the immunologic facet of oncolytic therapy. BMC Genomics (2009) 10:301. doi: 10.1186/1471-2164-10-301
72. Breitbach CJ, Paterson JM, Lemay CG, Falls TJ, McGuire A, Parato KA, et al. Targeted inflammation during oncolytic virus therapy severely compromises tumor blood flow. Mol Ther (2007) 15(9):1686–93. doi: 10.1038/sj.mt.6300215
73. Gujar S, Pol JG, Kim Y, Lee PW, Kroemer G. Antitumor benefits of antiviral immunity: an underappreciated aspect of oncolytic virotherapies. Trends Immunol (2018) 39(3):209–21. doi: 10.1016/j.it.2017.11.006
74. Breitbach CJ, Lichty BD, Bell JC. Oncolytic viruses: therapeutics with an identity crisis. EBioMedicine (2016) 9:31–6. doi: 10.1016/j.ebiom.2016.06.046
75. Warricker F, Khakoo SI, Blunt MD. The role of NK cells in oncolytic viral therapy: a focus on hepatocellular carcinoma. J Trans Genet Genomics (2021) 5:304–22. doi: 10.20517/jtgg.2021.27
76. Alvarez-Breckenridge CA, Yu J, Price R, Wojton J, Pradarelli J, Mao H, et al. NK cells impede glioblastoma virotherapy through NKp30 and NKp46 natural cytotoxicity receptors. Nat Med (2012) 18(12):1827–34. doi: 10.1038/nm.3013
77. Han J, Chen X, Chu J, Xu B, Meisen WH, Chen L, et al. TGFβ treatment enhances glioblastoma virotherapy by inhibiting the innate immune response. Cancer Res (2015) 75(24):5273–82. doi: 10.1158/0008-5472.CAN-15-0894
78. Altomonte J, Wu L, Meseck M, Chen L, Ebert O, Garcia-Sastre A, et al. Enhanced oncolytic potency of vesicular stomatitis virus through vector-mediated inhibition of NK and NKT cells. Cancer Gene Ther (2009) 16(3):266–78. doi: 10.1038/cgt.2008.74
79. Ebrahimi S, Ghorbani E, Khazaei M, Avan A, Ryzhikov M, Azadmanesh K, et al. Interferon-mediated tumor resistance to oncolytic virotherapy. J Cell Biochem (2017) 118(8):1994–9. doi: 10.1002/jcb.25917
80. Meisen WH, Wohleb ES, Jaime-Ramirez AC, Bolyard C, Yoo JY, Russell L, et al. The impact of macrophage- and microglia-secreted TNFα on oncolytic HSV-1 therapy in the glioblastoma tumor microenvironment. Clin Cancer Res (2015) 21(14):3274–85. doi: 10.1158/1078-0432.CCR-14-3118
81. Lemos de Matos A, Franco LS, McFadden G. Oncolytic viruses and the immune system: the dynamic duo. Mol Ther - Methods Clin Dev (2020) 17:349–58. doi: 10.1016/j.omtm.2020.01.001
82. Lin C, Zhang J. Chimeric antigen receptor engineered innate immune cells in cancer immunotherapy. Sci China Life Sci (2019) 62(5):633–9. doi: 10.1007/s11427-018-9451-0
83. Hayes C. Cellular immunotherapies for cancer. Irish J Med Sci (2021) 190(1):41–57. doi: 10.1007/s11845-020-02264-w
84. Khan AN, Chowdhury A, Karulkar A, Jaiswal AK, Banik A, Asija S, et al. Immunogenicity of CAR-T cell therapeutics: evidence, mechanism and mitigation. Front Immunol (2022) 13. doi: 10.3389/fimmu.2022.886546
85. Sadeqi Nezhad M, Yazdanifar M, Abdollahpour-Alitappeh M, Sattari A, Seifalian A, Bagheri N. Strengthening the CAR-T cell therapeutic application using CRISPR/Cas9 technology. Biotechnol Bioengineering (2021) 118(10):3691–705. doi: 10.22541/au.162058094.49226022/v1
86. Sterner RC, Sterner RM. CAR-T cell therapy: current limitations and potential strategies. Blood Cancer J (2021) 11(4):69. doi: 10.1038/s41408-021-00459-7
87. Mitra A, Barua A, Huang L, Ganguly S, Feng Q, He B. From bench to bedside: the history and progress of CAR T cell therapy. Front Immunol (2023) 14. doi: 10.3389/fimmu.2023.1188049
88. Alnefaie A, Albogami S, Asiri Y, Ahmad T, Alotaibi SS, Al-Sanea MM, et al. Chimeric antigen receptor T-cells: an overview of concepts, applications, limitations, and proposed solutions. Front Bioengineering Biotechnol (2022) 10. doi: 10.3389/fbioe.2022.797440
89. Cappell KM, Kochenderfer JN. Long-term outcomes following CAR T cell therapy: what we know so far. Nat Rev Clin Oncol (2023) 20(6):359–71. doi: 10.1038/s41571-023-00754-1
90. Cortés-Selva D, Dasgupta B, Singh S, Grewal IS. Innate and innate-like cells: the future of chimeric antigen receptor (CAR) cell therapy. Trends Pharmacol Sci (2021) 42(1):45–59. doi: 10.1016/j.tips.2020.11.004
91. Hadiloo K, Tahmasebi S, Esmaeilzadeh A. CAR-NKT cell therapy: a new promising paradigm of cancer immunotherapy. Cancer Cell Int (2023) 23(1):86. doi: 10.1186/s12935-023-02923-9
92. Du SH, Li Z, Chen C, Tan WK, Chi Z, Kwang TW, et al. Co-expansion of cytokine-induced killer cells and Vγ9Vδ2 T cells for CAR T-cell therapy. PloS One (2016) 11(9):e0161820. doi: 10.1371/journal.pone.0161820
93. Xu X, Huang W, Heczey A, Liu D, Guo L, Wood M, et al. NKT cells coexpressing a GD2-specific chimeric antigen receptor and IL15 show enhanced in vivo persistence and antitumor activity against neuroblastoma. Clin Cancer Res (2019) 25(23):7126–38. doi: 10.1158/1078-0432.CCR-19-0421
94. Simon B, Wiesinger M, März J, Wistuba-Hamprecht K, Weide B, Schuler-Thurner B, et al. The generation of CAR-transfected natural killer T cells for the immunotherapy of melanoma. Int J Mol Sci (2018) 19(8):2365. doi: 10.3390/ijms19082365
95. Rozenbaum M, Meir A, Aharony Y, Itzhaki O, Schachter J, Bank I, et al. Gamma-delta CAR-T cells show CAR-directed and independent activity against leukemia. Front Immunol (2020) 11. doi: 10.3389/fimmu.2020.01347
96. Makkouk A, Yang XC, Barca T, Lucas A, Turkoz M, Wong JTS, et al. Off-the-shelf Vδ 1 gamma delta T cells engineered with glypican-3 (GPC-3)-specific chimeric antigen receptor (CAR) and soluble IL-15 display robust antitumor efficacy against hepatocellular carcinoma. J Immunother Cancer. (2021) 9(12):e003441. doi: 10.1136/jitc-2021-003441
97. Saura-Esteller J, de Jong M, King LA, Ensing E, Winograd B, de Gruijl TD, et al. Gamma delta T-cell based cancer immunotherapy: past-present-future. Front Immunol (2022) 13. doi: 10.3389/fimmu.2022.915837
98. Korell F, Berger TR, Maus MV. Understanding CAR T cell-tumor interactions: Paving the way for successful clinical outcomes. Med (2022) 3(8):538–64. doi: 10.1016/j.medj.2022.05.001
99. de Miguel D, Ramirez-Labrada A, Uranga I, Hidalgo S, Santiago L, Galvez EM, et al. Inflammatory cell death induced by cytotoxic lymphocytes: a dangerous but necessary liaison. FEBS J (2022) 289(15):4398–415. doi: 10.1111/febs.16093
100. Jaime-Sanchez P, Uranga-Murillo I, Aguilo N, Khouili SC, Arias MA, Sancho D, et al. Cell death induced by cytotoxic CD8 + T cells is immunogenic and primes caspase-3-dependent spread immunity against endogenous tumor antigens. J Immunother Cancer. (2020) 8(1):e000528. doi: 10.1136/jitc-2020-000528
101. Minute L, Teijeira A, Sanchez-Paulete AR, Ochoa MC, Alvarez M, Otano I, et al. Cellular cytotoxicity is a form of immunogenic cell death. J Immunother Cancer. (2020) 8(1):e000325. doi: 10.1136/jitc-2019-000325
102. Barrow AD, Martin CJ, Colonna M. The natural cytotoxicity receptors in health and disease. Front Immunol (2019) 10. doi: 10.3389/fimmu.2019.00909
103. Sen Santara S, Lee DJ, Crespo Â, Hu JJ, Walker C, Ma X, et al. The NK cell receptor NKp46 recognizes ecto-calreticulin on ER-stressed cells. Nature (2023) 616(7956):348–56. doi: 10.1038/s41586-023-06151-z
104. Paul S, Lal G. The molecular mechanism of natural killer cells function and its importance in cancer immunotherapy. Front Immunol (2017) 8. doi: 10.3389/fimmu.2017.01124
105. Dogra P, Rancan C, Ma W, Toth M, Senda T, Carpenter DJ, et al. Tissue determinants of human NK cell development, function, and residence. Cell. (2020) 180(4):749–63.e13. doi: 10.1016/j.cell.2020.01.022
106. López-Soto A, Gonzalez S, Smyth MJ, Galluzzi L. Control of metastasis by NK cells. Cancer Cell (2017) 32(2):135–54. doi: 10.1016/j.ccell.2017.06.009
107. Imai K, Matsuyama S, Miyake S, Suga K, Nakachi K. Natural cytotoxic activity of peripheral-blood lymphocytes and cancer incidence: An 11-year follow-up study of a general population. Lancet (9244) 2000:356. doi: 10.1016/S0140-6736(00)03231-1
108. Liu S, Galat V, Galat4 Y, Lee YKA, Wainwright D, Wu J. NK cell-based cancer immunotherapy: from basic biology to clinical development. J Hematol Oncol (2021) 14(1):7. doi: 10.1186/s13045-020-01014-w
109. Li H, Song W, Li Z, Zhang M. Preclinical and clinical studies of CAR-NK-cell therapies for Malignancies. Front Immunol (2022) 13. doi: 10.3389/fimmu.2022.992232
110. Gurney M, Stikvoort A, Nolan E, Kirkham-McCarthy L, Khoruzhenko S, Shivakumar R, et al. CD38 knockout natural killer cells expressing an affinity optimized CD38 chimeric antigen receptor successfully target acute myeloid leukemia with reduced effector cell fratricide. Haematologica. (2022) 107(2):437–45. doi: 10.3324/haematol.2020.271908
111. Jiang H, Zhang W, Shang P, Zhang H, Fu W, Ye F, et al. Transfection of chimeric anti-CD138 gene enhances natural killer cell activation and killing of multiple myeloma cells. Mol Oncol (2014) 8(2):297–310. doi: 10.1016/j.molonc.2013.12.001
112. Ng YY, Du Z, Zhang X, Chng WJ, Wang S. CXCR4 and anti-BCMA CAR co-modified natural killer cells suppress multiple myeloma progression in a xenograft mouse model. Cancer Gene Ther (2022) 29(5):475–83. doi: 10.1038/s41417-021-00365-x
113. Chu J, Deng Y, Benson DM, He S, Hughes TL, Zhang J, et al. CS1-specific chimeric antigen receptor (CAR)-engineered NK cells and T cells enhance in vitro and in vivo anti-tumor activity against human multiple myeloma. Blood. (2013) 122(21):917–27. doi: 10.1182/blood.V122.21.14.14
114. Parihar R, Rivas C, Huynh M, Omer B, Lapteva N, Metelitsa LS, et al. NK cells expressing a chimeric activating receptor eliminate MDSCs and rescue impaired CAR-T cell activity against solid tumors. Cancer Immunol Res (2019) 7(3):363–75. doi: 10.1158/2326-6066.CIR-18-0572
115. Zhang P, Zhao S, Wu C, Li J, Li Z, Wen C, et al. Effects of CSF1R-targeted chimeric antigen receptor-modified NK92MI & T cells on tumor-associated macrophages. Immunotherapy. (2018) 10(11):935–49. doi: 10.2217/imt-2018-0012
116. Ramírez-Labrada A, Pesini C, Santiago L, Hidalgo S, Calvo-Pérez A, Oñate C, et al. All about (NK cell-mediated) death in two acts and an unexpected encore: initiation, execution and activation of adaptive immunity. Front Immunol (2022) 13. doi: 10.3389/fimmu.2022.896228
117. Mantovani A, Allavena P, Marchesi F, Garlanda C. Macrophages as tools and targets in cancer therapy. Nat Rev Drug Discov (2022) 21(11):799–820. doi: 10.1038/s41573-022-00520-5
118. Su S, Lei A, Wang X, Lu H, Wang S, Yang Y, et al. Induced CAR-macrophages as a novel therapeutic cell type for cancer immune cell therapies. Cells. (2022) 11(10):1652. doi: 10.3390/cells11101652
119. Morrissey MA, Williamson AP, Steinbach AM, Roberts EW, Kern N, Headley MB, et al. Chimeric antigen receptors that trigger phagocytosis. Elife. (2018) 7:e36688. doi: 10.7554/eLife.36688
120. Zhang W, Liu L, Su HF, Liu Q, Shen J, Dai H, et al. Chimeric antigen receptor macrophage therapy for breast tumours mediated by targeting the tumour extracellular matrix. Br J Cancer (2019) 121(10):837–45. doi: 10.1038/s41416-019-0578-3
121. Klichinsky M, Ruella M, Shestova O, Lu XM, Best A, Zeeman M, et al. Human chimeric antigen receptor macrophages for cancer immunotherapy. Nat Biotechnol (2020) 38(8):947–53. doi: 10.1038/s41587-020-0462-y
122. Aminin D, Wang YM. Macrophages as a “weapon” in anticancer cellular immunotherapy. Kaohsiung J Med Sci (2021) 37(9):749–58. doi: 10.1002/kjm2.12405
123. Yu J, Sun H, Cao W, Song Y, Jiang Z. Research progress on dendritic cell vaccines in cancer immunotherapy. Exp Hematol Oncol (2022) 11(1):3. doi: 10.1186/s40164-022-00257-2
124. Saéz PJ, Vargas P, Shoji KF, Harcha PA, Lennon-Duméni AM, Saéz JC. ATP promotes the fast migration of dendritic cells through the activity of pannexin 1 channels and P2X7 receptors. Sci Signal (2017) 10(506):eaah7107. doi: 10.1126/scisignal.aah7107
125. Lamberti MJ, Nigro A, Mentucci FM, Vittar NBR, Casolaro V, Col JD. Dendritic cells and immunogenic cancer cell death: A combination for improving antitumor immunity. Pharmaceutics (2020) 12(3):256. doi: 10.3390/pharmaceutics12030256
126. Fucikova J, Kepp O, Kasikova L, Petroni G, Yamazaki T, Liu P, et al. Detection of immunogenic cell death and its relevance for cancer therapy. Cell Death Dis (2020) 11(11):1013. doi: 10.1038/s41419-020-03221-2
127. Liu X, Li J, Liu Y, Ding J, Tong Z, Liu Y, et al. Calreticulin acts as an adjuvant to promote dendritic cell maturation and enhances antigen-specific cytotoxic T lymphocyte responses against non-small cell lung cancer cells. Cell Immunol (2016) 300:46–53. doi: 10.1016/j.cellimm.2015.12.003
128. Obeid M, Tesniere A, Ghiringhelli F, Fimia GM, Apetoh L, Perfettini J-L, et al. Calreticulin exposure dictates the immunogenicity of cancer cell death. Nat Med (2007) 13(1):54–61. doi: 10.1038/nm1523
129. Salimu J, Spary LK, Al-Taei S, Clayton A, Mason MD, Staffurth J, et al. Cross-presentation of the oncofetal tumor antigen 5T4 from irradiated prostate cancer cells-a key role for heat-shock protein 70 and receptor CD91. Cancer Immunol Res (2015) 3(6):678–88. doi: 10.1158/2326-6066.CIR-14-0079
130. Hou W, Zhang Q, Yan Z, Chen R, Zeh HJ, Kang R, et al. Strange attractors: DAMPs and autophagy link tumor cell death and immunity. Cell Death Dis (2013) 4(12):e966. doi: 10.1038/cddis.2013.493
131. Garg AD, Dudek-Peric AM, Romano E, Agostinis P. Immunogenic cell death. Int J Dev Biol (2015) 59(1-3):131–40. doi: 10.1387/ijdb.150061pa
132. Apetoh L, Ghiringhelli F, Tesniere A, Obeid M, Ortiz C, Criollo A, et al. Toll-like receptor 4-dependent contribution of the immune system to anticancer chemotherapy and radiotherapy. Nat Med (2007) 13(9):1050–9. doi: 10.1038/nm1622
133. Del Prete A, Salvi V, Soriani A, Laffranchi M, Sozio F, Bosisio D, et al. Dendritic cell subsets in cancer immunity and tumor antigen sensing. Cell Mol Immunol (2023) 20(5):432–47. doi: 10.1038/s41423-023-00990-6
134. Huber A, Dammeijer F, Aerts JGJV, Vroman H. Current state of dendritic cell-based immunotherapy: opportunities for in vitro antigen loading of different DC subsets? Front Immunol (2018) 9. doi: 10.3389/fimmu.2018.02804
135. Keenan BP, Jaffee EM. Whole cell vaccines - Past progress and future strategies. Semin Oncol (2012) 39(3):276–86. doi: 10.1053/j.seminoncol.2012.02.007
136. Komorowski M, Tisonczyk J, Kolakowska A, Drozdz R, Kozbor D. Modulation of the tumor microenvironment by CXCR4 antagonist-armed viral oncotherapy enhances the antitumor efficacy of dendritic cell vaccines against neuroblastoma in syngeneic mice. Viruses. (2018) 10(9):455. doi: 10.3390/v10090455
137. Chen H-M, Wang P-H, Chen S-S, Wen C-C, Chen Y-H, Yang W-C, et al. Shikonin induces immunogenic cell death in tumor cells and enhances dendritic cell-based cancer vaccine. Cancer Immunol Immunother (2012) 61(11):1989–2002. doi: 10.1007/s00262-012-1258-9
138. Lin TJ, Lin HT, Chang WT, Pradeep Mitapalli S, Hsiao PW, Yin SY, et al. Shikonin-enhanced cell immunogenicity of tumor vaccine is mediated by the differential effects of DAMP components. Mol Cancer (2015) 14(1):174. doi: 10.1186/s12943-015-0435-9
139. Doix B, Trempolec N, Riant O, Feron O. Low photosensitizer dose and early radiotherapy enhance antitumor immune response of photodynamic therapy-based dendritic cell vaccination. Front Oncol (2019) 9(AUG). doi: 10.3389/fonc.2019.00811
140. Trempolec N, Doix B, Degavre C, Brusa D, Bouzin C, Riant O, et al. Photodynamic therapy-based dendritic cell vaccination suited to treat peritoneal mesothelioma. Cancers (Basel). (2020) 12(3):545. doi: 10.3390/cancers12030545
141. Kawano M, Tanaka K, Itonaga I, Iwasaki T, Miyazaki M, Ikeda S, et al. Dendritic cells combined with doxorubicin induces immunogenic cell death and exhibits antitumor effects for osteosarcoma. Oncol Lett (2016) 11(3):2169–75. doi: 10.3892/ol.2016.4175
142. Wang C, Pu J, Yu H, Liu Y, Yan H, He Z, et al. A dendritic cell vaccine combined with radiotherapy activates the specific immune response in patients with esophageal cancer. J Immunother (2017) 40(2):71–6. doi: 10.1097/CJI.0000000000000155
143. Münz C, Dao T, Ferlazzo G, De Cos MA, Goodman K, Young JW. Mature myeloid dendritic cell subsets have distinct roles for activation and viability of circulating human natural killer cells. Blood. (2005) 105(1):266–73. doi: 10.1182/blood-2004-06-2492
144. Fujii SI, Shimizu K, Kronenberg M, Steinman RM. Prolonged IFN-γ-producing NKT response induced with α-galactosylceramide-loaded DCs. Nat Immunol (2002) 3(9):867–74. doi: 10.1038/ni827
145. Chen J, Gao L, Wu X, Fan Y, Liu M, Peng L, et al. BCG-induced trained immunity: history, mechanisms and potential applications. J Trans Med (2023) 21(1):106. doi: 10.1186/s12967-022-03842-5
146. Franco-Molina MA, Mendoza-Gamboa E, Miranda-Hernández D, Zapata-Benavides P, Castillo-León L, Isaza-Brando C, et al. In vitro effects of bovine dialyzable leukocyte extract (bDLE) in cancer cells. Cytotherapy. (2006) 8(4):408–14. doi: 10.1080/14653240600847266
147. Dye C. Making wider use of the world’s most widely used vaccine: Bacille Calmette-Guérin revaccination reconsidered. J R Soc Interface (2013) 10(87):20130365. doi: 10.1098/rsif.2013.0365
148. See WA, Zhang G, Chen F, Cao Y, Langenstroer P, Sandlow J. Bacille-Calmette Guèrin induces caspase-independent cell death in urothelial carcinoma cells together with release of the necrosis-associated chemokine high molecular group box protein 1. BJU Int (2009) 103(12):1714–20. doi: 10.1111/j.1464-410X.2008.08274.x
149. Dockrell HM, Smith SG. What have we learnt about BCG vaccination in the last 20 years? Front Immunol (2017) 8. doi: 10.3389/fimmu.2017.01134
150. Covián C, Fernández-Fierro A, Retamal-Díaz A, Díaz FE, Vasquez AE, Lay MK, et al. BCG-induced cross-protection and development of trained immunity: implication for vaccine design. Front Immunol (2019) 10:2806. doi: 10.3389/fimmu.2019.02806
151. Minassian AM, Satti I, Poulton ID, Meyer J, Hill AVS, McShane H. A human challenge model for Mycobacterium tuberculosis using Mycobacterium bovis bacille Calmette-Guérin. J Infect Dis (2012) 205(7):1035–42. doi: 10.1093/infdis/jis012
152. Morel C, Badell E, Abadie V, Robledo M, Setterblad N, Gluckman JC, et al. Mycobacterium bovis BCG-infected neutrophils and dendritic cells cooperate to induced specific T cell responses in humans and mice. Eur J Immunol (2008) 38(2):437–47. doi: 10.1002/eji.200737905
153. Arnaudov A. Immunotherapy with dialyzable leukocyte extracts containing transfer factor. Immunother - Myth Reality Ideas Futur (2017). doi: 10.5772/66524
154. Mačák Kubašková T, Mudroňová D, Velebný S, Hrčková G. The utilisation of human dialyzable leukocyte extract (IMMODIN) as adjuvant in albendazole therapy on mouse model of larval cestode infection: Immunomodulatory and hepatoprotective effects. Int Immunopharmacol. (2018) 65:148–58. doi: 10.1016/j.intimp.2018.09.045
155. Lorenzo-Anota HY, Martínez-Loria AB, Tamez-Guerra RS, Scott-Algara D, Martínez-Torres AC, Rodríguez-Padilla C. Changes in the natural killer cell repertoire and function induced by the cancer immune adjuvant candidate IMMUNEPOTENT-CRP. Cell Immunol (2022) 374:104511. doi: 10.1016/j.cellimm.2022.104511
156. Reyes-Ruiz A, Calvillo-Rodriguez KM, Martínez-Torres AC, Rodríguez-Padilla C. The bovine dialysable leukocyte extract IMMUNEPOTENT CRP induces immunogenic cell death in breast cancer cells leading to long-term antitumour memory. Br J Cancer (2021) 124(8):1398–410. doi: 10.1038/s41416-020-01256-y
157. Castrejón Vázquez MI, Reséndiz-Albor AA, Ynga-Durand MA, Arciniega Martínez IMI, Orellana-Villazon VI, García López CA, et al. Dialyzable leukocyte extract (TransferonTM) administration in sepsis: experience from a single referral pediatric intensive care unit. BioMed Res Int (2019) 2019:8980506. doi: 10.1155/2019/8980506
158. Hernández-Esquivel MA, Pérez-Torres A, Romero-Romero L, Reyes-Matute A, Loaiza B, Mellado-Sánchez G, et al. The dialyzable leukocyte extract TransferonTM inhibits tumor growth and brain metastasis in a murine model of prostate cancer. BioMed Pharmacother. (2018) 101:938–44. doi: 10.1016/j.biopha.2018.03.012
159. Mendoza-Gamboa E, Franco-Molina MA, Zapata-Benavides P, Castillo-Tello P, Vera-García ME, Tamez-Guerra RS, et al. Bovine dialyzable leukocyte extract modulates AP-1 DNA-binding activity and nuclear transcription factor expression in MCF-7 breast cancer cells. Cytotherapy. (2008) 10(2):212–9. doi: 10.1080/14653240801891659
160. Martínez-Torres AC, Reyes-Ruiz A, Calvillo-Rodriguez KM, Alvarez-Valadez KM, Uscanga-Palomeque AC, Tamez-Guerra RS, et al. IMMUNEPOTENT CRP induces DAMPS release and ROS-dependent autophagosome formation in HeLa and MCF-7 cells. BMC Cancer (2020) 20(1):1–11. doi: 10.1186/s12885-020-07124-5
161. Martinez-Torres AC, Gomez-Morales L, Martinez-Loria AB, Uscanga-Palomeque AC, Vazquez-Guillen JM, Rodriguez-Padilla C. Cytotoxic activity of IMMUNEPOTENT CRP against non-small cell lung cancer cell lines. PeerJ (2019) 7(9):e7759. doi: 10.7717/peerj.7759
162. Martínez-Torres AC, Reyes-Ruiz A, Benítez-Londoño M, Franco-Molina MA, Rodríguez-Padilla C. IMMUNEPOTENT CRP induces cell cycle arrest and caspase-independent regulated cell death in HeLa cells through reactive oxygen species production. BMC Cancer (2018) 18(1):13. doi: 10.1186/s12885-017-3954-5
163. Lorenzo-Anota HY, Martínez-Torres AC, Scott-Algara D, Tamez-Guerra RS, Rodríguez-Padilla C. Bovine dialyzable leukocyte extract IMMUNEPOTENT-CRP induces selective ROS-dependent apoptosis in T-acute lymphoblastic leukemia cell lines. J Oncol (2020) 2020:1598503. doi: 10.1155/2020/1598503
164. Rodríguez-Salazar MDC, Franco-Molina MA, Mendoza-Gamboa E, Martínez-Torres AC, Zapata-Benavides P, López-González JS, et al. The novel immunomodulator IMMUNEPOTENT CRP combined with chemotherapy agent increased the rate of immunogenic cell death and prevented melanoma growth. Oncol Lett (2017) 14(1):844–52. doi: 10.3892/ol.2017.6202
165. Ramírez-Ramírez D, Vadillo E, Arriaga-Pizano LA, Mayani H, Estrada-Parra S, Velasco-Velázquez MA, et al. Early differentiation of human CD11c+NK cells with γδ T cell activation properties is promoted by dialyzable leukocyte extracts. J Immunol Res (2016) 2016:4097642. doi: 10.1155/2016/4097642
166. Solár P, Sačková V, Hrčková G, Demečková V, Kassayová M, Bojková B, et al. Antitumor effect of the combination of manumycin A and Immodin is associated with antiplatelet activity and increased granulocyte tumor infiltration in a 4T1 breast tumor model. Oncol Rep (2017) 37(1):368–78. doi: 10.3892/or.2016.5265
167. Kayser O, Masihi KN, Kiderlen AF. Natural products and synthetic compounds as immunomodulators. Expert Rev Anti-Infective Ther (2003) 1(2):319–35. doi: 10.1586/14787210.1.2.319
168. Iyer V. Small molecules for immunomodulation in cancer: A review. Anticancer Agents Med Chem (2014) 15(4):433–52. doi: 10.2174/1871520615666141210152128
169. Lefrancier P. Chemistry of immunomodulators. Comp Immunol Microbiol Infect Dis (1985) 8(2):171–85. doi: 10.1016/0147-9571(85)90043-8
170. Gokhale AS, Satyanarayanajois S. Peptides and peptidomimetics as immunomodulators. Immunotherapy (2014) 6(6):755–74. doi: 10.2217/imt.14.37
171. Miller R. Imiquimod stimulates innate and cell mediated immunity which controls virus infections and tumors. Int J Dermatol (2002) 41(Suppl 1):3–6. doi: 10.1111/j.1365-4632.2002.00017.x
172. Yoon HK, Shim YS, Kim PH, Park SR. The TLR7 agonist imiquimod selectively inhibits IL-4-induced IgE production by suppressing IgG1/IgE class switching and germline ϵ transcription through the induction of BCL6 expression in B cells. Cell Immunol (2019) 338:1–8. doi: 10.1016/j.cellimm.2019.02.006
173. Dajon M, Iribarren K, Cremer I. Dual roles of TLR7 in the lung cancer microenvironment. Oncoimmunology (2015) 4(3):e991615. doi: 10.4161/2162402X.2014.991615
174. Miller RL, Gerster JF, Owens ML, Slade HB, Tomai MA. Review article Imiquimod applied topically: A novel immune response modifier and new class of drug. Int J Immunopharmacol (1999) 21(1):1–14. doi: 10.1016/s0192-0561(98)00068-x
175. López V, Alonso V, Jordá E. Efficacy of topical imiquimod 5% in a patient with chronic radiodermatitis on the hands. Actas Dermo-Sifiliográficas (2012) 103(5):441–2.
176. De Sousa MDGT, Belda W, Spina R, Lota PR, Valente NS, Brown GD, et al. Topical application of imiquimod as a treatment for chromoblastomycosis. Clin Infect Dis (2014) 58(12):1734–7. doi: 10.1093/cid/ciu168
177. Nieto-Fontarigo JJ, Tillgren S, Cerps S, Sverrild A, Hvidtfeldt M, Ramu S, et al. Imiquimod boosts interferon response, and decreases ACE2 and pro-inflammatory response of human bronchial epithelium in asthma. Front Immunol (2021) 12:743890. doi: 10.3389/fimmu.2021.743890
178. Cho JH, Lee HJ, Ko HJ, Il YB, Choe J, Kim KC, et al. The TLR7 agonist imiquimod induces anti-cancer effects via autophagic cell death and enhances anti-tumoral and systemic immunity during radiotherapy for melanoma. Oncotarget. (2017) 8(15). doi: 10.18632/oncotarget.15326
179. Kang SJ, Tak JH, Cho JH, Lee HJ, Jung YJ. Stimulation of the endosomal TLR pathway enhances autophagy-induced cell death in radiotherapy of breast cancer. Genes Genomics (2010) 32(6):599–606. doi: 10.1007/s13258-010-0139-x
180. Yi JY, Jung YJ, Choi SS, Hwang J, Chung E. Autophagy-mediated anti-tumoral activity of imiquimod in Caco-2 cells. Biochem Biophys Res Commun (2009) 386(3):455–8. doi: 10.1016/j.bbrc.2009.06.046
181. Huang SW, Wang ST, Chang SH, Chuang KC, Wang HY, Kao JK, et al. Imiquimod exerts antitumor effects by inducing immunogenic cell death and is enhanced by the glycolytic inhibitor 2-deoxyglucose. J Invest Dermatol (2020) 140(9):1771–83.e6. doi: 10.1016/j.jid.2019.12.039
182. Lu H, Wagner WM, Gad E, Yang Y, Duan H, Amon LM, et al. Treatment failure of a TLR-7 agonist occurs due to self-regulation of acute inflammation and can be overcome by IL-10 blockade. J Immunol (2010) 184(9):5360–7. doi: 10.4049/jimmunol.0902997
183. Prins RM, Craft N, Bruhn KW, Khan-Farooqi H, Koya RC, Stripecke R, et al. The TLR-7 agonist, imiquimod, enhances dendritic cell survival and promotes tumor antigen-specific T cell priming: relation to central nervous system antitumor immunity. J Immunol (2006) 176(1):157–64. doi: 10.4049/jimmunol.176.1.157
184. Mitsiades N, Mitsiades CS, Poulaki V, Chauhan D, Richardson PG, Hideshima T, et al. Apoptotic signaling induced by immunomodulatory thalidomide analogs in human multiple myeloma cells: Therapeutic implications. Blood. (2002) 99(12):4525–30. doi: 10.1182/blood.V99.12.4525
185. Marriott JB, Clarke IA, Czajka A, Dredge K, Childs K, Man HW, et al. A novel subclass of thalidomide analogue with anti-solid tumor activity in which caspase-dependent apoptosis is associated with altered expression of bcl-2 family proteins. Cancer Res (2003) 63(3):593–9.
186. Dimopoulos MA, Dytfeld D, Grosicki S, Moreau P, Takezako N, Hori M, et al. Elotuzumab plus pomalidomide and dexamethasone for relapsed/refractory multiple myeloma: final overall survival analysis from the randomized phase II ELOQUENT-3 trial. J Clin Oncol (2023) 41(3):568–78. doi: 10.1200/JCO.21.02815
187. Bahlis NJ, Siegel DS, Schiller GJ, Samaras C, Sebag M, Berdeja J, et al. Pomalidomide, dexamethasone, and daratumumab immediately after lenalidomide-based treatment in patients with multiple myeloma: updated efficacy, safety, and health-related quality of life results from the phase 2 MM-014 trial. Leuk Lymphoma. (2022) 63(6):1407–17. doi: 10.1080/10428194.2022.2030477
188. Wang D, Hu X, Yin X, Cui C, Yang X, Li Y, et al. Effectiveness of thalidomide for ankylosing spondylitis: a meta-analysis of randomized controlled trials in China. Clin Rheumatol (2022) 41(10):2929–38. doi: 10.1007/s10067-022-06220-0
189. Wang X, Shen Y, Li S, Lv M, Zhang X, Yeng J, et al. Importance of the interaction between immune cells and tumor vasculature mediated by thalidomide in cancer treatment (Review). Int J Mol Med (2016) 38(4):1021–9. doi: 10.3892/ijmm.2016.2724
190. Haslett PAJ, Klausner JD, Makonkawkeyoon S, Moreira A, Metatratip P, Boyle B, et al. Thalidomide stimulates T cell responses and interleukin 12 production in HIV-infected patients. AIDS Res Hum Retroviruses (1999) 15(13):1169–79. doi: 10.1089/088922299310269
191. Aria H, Rezaei M. Immunogenic cell death inducer peptides: A new approach for cancer therapy, current status and future perspectives. Biomedicine Pharmacotherapy (2023) 161:114503. doi: 10.1016/j.biopha.2023.114503
192. Luthra R, Datta S, Roy A. Role of different peptides for cancer immunotherapy. Int J Pept Res Ther (2021) 27:2777–93. doi: 10.1007/s10989-021-10289-7
193. Martell EM, González-Garcia M, Ständker L, Otero-González AJ. Host defense peptides as immunomodulators: The other side of the coin. Peptides. (2021) 146:170644. doi: 10.1016/j.peptides.2021.170644
194. Vitale I, Yamazaki T, Wennerberg E, Sveinbjørnsson B, Rekdal Ø, Demaria S, et al. Targeting cancer heterogeneity with immune responses driven by oncolytic peptides. Trends Cancer (2021) 7(6):557–72. doi: 10.1016/j.trecan.2020.12.012
195. Zhou H, Forveille S, Sauvat A, Yamazaki T, Senovilla L, Ma Y, et al. The oncolytic peptide LTX-315 triggers immunogenic cell death. Cell Death Dis (2016) 7(3):e2134. doi: 10.1038/cddis.2016.47
196. Zhou H, Mondragón L, Xie W, Mauseth B, Leduc M, Sauvat A, et al. Oncolysis with DTT-205 and DTT-304 generates immunological memory in cured animals. Cell Death Dis (2018) 9(11):1086. doi: 10.1038/s41419-018-1127-3
197. Mauseth B, Camilio KA, Shi J, Hammarström CL, Rekdal Ø, Sveinbjørnsson B, et al. The novel oncolytic compound LTX-401 induces antitumor immune responses in experimental hepatocellular carcinoma. Mol Ther - Oncolytics. (2019) 14:139–48. doi: 10.1016/j.omto.2019.05.002
198. Gao AG, Frazier WA. Identification of a receptor candidate for the carboxyl-terminal cell binding domain of thrombospondins. J Biol Chem (1994) 269(47):29650–7. doi: 10.1016/S0021-9258(18)43930-0
199. Kosfeld MD, Frazier WA. Identification of a new cell adhesion motif in two homologous peptides from the COOH-terminal cell binding domain of human thrombospondin. J Biol Chem (1993) 268(12):8808–14. doi: 10.1016/S0021-9258(18)52946-X
200. Gao AG, Lindberg FP, Finn MB, Blystone SD, Brown EJ, Frazier WA. Integrin-associated protein is a receptor for the C-terminal domain of thrombospondin. J Biol Chem (1996) 271(1):21–4. doi: 10.1074/jbc.271.1.21
201. Armant M, Avice MN, Hermann P, Rubio M, Kiniwa M, Delespesse G, et al. CD47 ligation selectively downregulates human interleukin 12 production. J Exp Med (1999) 190(8):1175–82. doi: 10.1084/jem.190.8.1175
202. Demeure CE, Tanaka H, Mateo V, Rubio M, Delespesse G, Sarfati M. CD47 engagement inhibits cytokine production and maturation of human dendritic cells. J Immunol (2000) 164(4):2193–9. doi: 10.4049/jimmunol.164.4.2193
203. Johansson U, Londei M. Ligation of CD47 during Monocyte Differentiation into Dendritic Cells Results in Reduced Capacity for Interleukin-12 Production. Scand J Immunol (2004) 59(1):50–7. doi: 10.1111/j.0300-9475.2004.01354.x
204. Karki S, Nichols MR. CD47 does not mediate amyloid-β(1-42) protofibril-stimulated microglial cytokine release. Biochem Biophys Res Commun (2014) 454(1):239–44. doi: 10.1016/j.bbrc.2014.10.081
205. Martinez-Torres A-C, Quiney C, Attout T, Boullet H, Herbi L, Vela L, et al. CD47 agonist peptides induce programmed cell death in refractory chronic lymphocytic leukemia B cells via PLCγ1 activation: evidence from mice and humans. PloS Med (2015) 12(3):e1001796. doi: 10.1371/journal.pmed.1001796
206. Uscanga-Palomeque AC, Calvillo-Rodríguez KM, Gómez-Morales L, Lardé E, Denèfle T, Caballero-Hernández D, et al. CD47 agonist peptide PKHB1 induces immunogenic cell death in T-cell acute lymphoblastic leukemia cells. Cancer Sci (2019) 110(1):256–68. doi: 10.1111/cas.13885
207. Calvillo-Rodríguez KM, Mendoza-Reveles R, Gómez-Morales L, Uscanga-Palomeque AC, Karoyan P, Martínez-Torres AC, et al. PKHB1, a thrombospondin-1 peptide mimic, induces anti-tumor effect through immunogenic cell death induction in breast cancer cells. Oncoimmunology. (2022) 11(1):2054305. doi: 10.1080/2162402X.2022.2054305
208. He Y, Wang C, Liang Q, Guo R, Jiang J, Shen W, et al. PKHB1 peptide induces antiviral effects through induction of immunogenic cell death in herpes simplex keratitis. Front Pharmacol (2022) 13. doi: 10.3389/fphar.2022.1048978
209. Calippe B, Augustin S, Beguier F, Charles-Messance H, Poupel L, Conart JB, et al. Complement factor H inhibits CD47-mediated resolution of inflammation. Immunity (2017) 46(2):261–72. doi: 10.1016/j.immuni.2017.01.006
210. Zheng D, Liu J, Xie L, Wang Y, Ding Y, Peng R, et al. Enzyme-instructed and mitochondria-targeting peptide self-assembly to efficiently induce immunogenic cell death. Acta Pharm Sin B (2022) 12(6):2740–50. doi: 10.1016/j.apsb.2021.07.005
211. Troitskaya O, Varlamov M, Nushtaeva A, Richter V, Koval O. Recombinant lactaptin induces immunogenic cell death and creates an antitumor vaccination effect in vivo with enhancement by an IDO inhibitor. Molecules (2020) 25(12):2804. doi: 10.3390/molecules25122804
212. Sakakibara K, Sato T, Kufe DW, VonHoff DD, Kawabe T. CBP501 induces immunogenic tumor cell death and CD8 T cell infiltration into tumors in combination with platinum, and increases the efficacy of immune checkpoint inhibitors against tumors in mice. Oncotargets (2017) 8(45):78277–88. doi: 10.18632/oncotarget.20968
213. Fricker LD. Proteasome inhibitor drugs. Annu Rev Pharmacol Toxicol (2020) 60:457–76. doi: 10.1146/annurev-pharmtox-010919-023603
214. Chen D, Frezza M, Schmitt S, Kanwar J, P. Dou Q. Bortezomib as the first proteasome inhibitor anticancer drug: current status and future perspectives. Curr Cancer Drug Targets (2011) 11(3):239–53. doi: 10.2174/156800911794519752
215. Mohty M, Brissot E, Savani BN, Gaugler B. Effects of bortezomib on the immune system: A focus on immune regulation. Biol Blood Marrow Transplant (2013) 19(10):1416–20. doi: 10.1016/j.bbmt.2013.05.011
216. Demaria S, Santori FR, Ng B, Liebes L, Formenti SC, Vukmanovic S. Select forms of tumor cell apoptosis induce dendritic cell maturation. J Leukoc Biol (2004) 77(3):361–8. doi: 10.1189/jlb.0804478
217. Spisek R, Charalambous A, Mazumder A, Vesole DH, Jagannath S, Dhodapkar MV. Bortezomib enhances dendritic cell (DC)-mediated induction of immunity to human myeloma via exposure of cell surface heat shock protein 90 on dying tumor cells: therapeutic implications. Blood (2007) 109(11):4839–45. doi: 10.1182/blood-2006-10-054221
218. Gulla A, Morelli E, Samur MK, Botta C, Hideshima T, Bianchi G, et al. Bortezomib induces anti–multiple myeloma immune response mediated by cGAS/STING pathway activation. Blood Cancer Discovery (2021) 2(5):468–83. doi: 10.1158/2643-3230.BCD-21-0047
219. Vanmeerbeek I, Sprooten J, De Ruysscher D, Tejpar S, Vandenberghe P, Fucikova J, et al. Trial watch: chemotherapy-induced immunogenic cell death in immuno-oncology. Oncoimmunology (2020) 9(1):1703449. doi: 10.1080/2162402X.2019.1703449
220. Johnstone M, Vinaixa D, Turi M, Morelli E, Anderson KC, Gulla A. Promises and challenges of immunogenic chemotherapy in multiple myeloma. Cells (2022) 11(16):2519. doi: 10.3390/cells11162519
221. Shanker A, Pellom ST, Dudimah DF, Thounaojam MC, De Kluyver RL, Brooks AD, et al. Bortezomib improves adoptive T-Cell therapy by sensitizing cancer cells to FasL cytotoxicity. Cancer Res (2015) 75(24):5260–72. doi: 10.1158/0008-5472.CAN-15-0794
222. Tang B, Guo ZS, Bartlett DL, Yan DZ, Schane CP, Thomas DL, et al. Synergistic combination of oncolytic virotherapy and immunotherapy for glioma. Clin Cancer Res (2020) 26(9):2216–30. doi: 10.1158/1078-0432.CCR-18-3626
223. Rivera-Lazarín AL, Martínez-Torres AC, de la Hoz-Camacho R, Guzmán-Aguillón OL, Franco-Molina MA, Rodríguez-Padilla C. The bovine dialyzable leukocyte extract, immunepotent crp, synergically enhances cyclophosphamide-induced breast cancer cell death, through A caspase-independent mechanism. EXCLI J (2023) 22:131–45. doi: 10.17179/excli2022-5389
224. Chen Q, Zhang Z, Li X, Bu L. Chemotherapy combined with immunotherapy as a first-line treatment brings benefits to patients with lung squamous cell carcinoma but different risks of adverse reactions: A systematic review and meta-analysis. Front Pharmacol (2022) 13. doi: 10.3389/fphar.2022.940567
225. Sordo-Bahamonde C, Lorenzo-Herrero S, Gonzalez-Rodriguez AP, Martínez-Pérez A, Rodrigo JP, García-Pedrero JM, et al. Chemo-immunotherapy: A new trend in cancer treatment. Cancers (2023) 15(11):2912. doi: 10.3390/cancers15112912
226. Salas-Benito D, Pérez-Gracia JL, Ponz-Sarvisé M, Rodriguez-Ruiz ME, Martínez-Forero I, Castañón E, et al. Paradigms on immunotherapy combinations with chemotherapy. Cancer Discovery (2021) 11(6):1353–67. doi: 10.1158/2159-8290.CD-20-1312
227. Fabian KP, Wolfson B, Hodge JW. From immunogenic cell death to immunogenic modulation: select chemotherapy regimens induce a spectrum of immune-enhancing activities in the tumor microenvironment. Front Oncol (2021) 11. doi: 10.3389/fonc.2021.728018
228. Kersten K, Salvagno C, De Visser KE. Exploiting the immunomodulatory properties of chemotherapeutic drugs to improve the success of cancer immunotherapy. Front Immunol (2015) 6. doi: 10.3389/fimmu.2015.00516
Keywords: onco-immunotherapy, immunogenic cell death, innate immune system, monoclonal antibodies, cytokines, oncolytic virus, cellular therapies, immunomodulators
Citation: Calvillo-Rodríguez KM, Lorenzo-Anota HY, Rodríguez-Padilla C, Martínez-Torres AC and Scott-Algara D (2023) Immunotherapies inducing immunogenic cell death in cancer: insight of the innate immune system. Front. Immunol. 14:1294434. doi: 10.3389/fimmu.2023.1294434
Received: 14 September 2023; Accepted: 01 November 2023;
Published: 23 November 2023.
Edited by:
Virginie Lafont, Institut National de la Santé et de la Recherche Médicale (INSERM), FranceReviewed by:
Azam Roohi, Tehran University of Medical Sciences, IranJian Yu, University of Southern California, United States
Copyright © 2023 Calvillo-Rodríguez, Lorenzo-Anota, Rodríguez-Padilla, Martínez-Torres and Scott-Algara. This is an open-access article distributed under the terms of the Creative Commons Attribution License (CC BY). The use, distribution or reproduction in other forums is permitted, provided the original author(s) and the copyright owner(s) are credited and that the original publication in this journal is cited, in accordance with accepted academic practice. No use, distribution or reproduction is permitted which does not comply with these terms.
*Correspondence: Ana Carolina Martínez-Torres, YW5hLm1hcnRpbmV6dG9AdWFubC5lZHUubXg=; Daniel Scott-Algara, ZGFuaWVsLnNjb3R0LWFsZ2FyYUBwYXN0ZXVyLmZy
†These authors share first authorship
‡These authors share senior authorship