- 1Michael E. DeBakey Department of Surgery, Baylor College of Medicine, Houston, TX, United States
- 2The Pelotonia Institute for Immuno-Oncology, Ohio State University Comprehensive Cancer Center, Columbus, OH, United States
- 3Dan L. Duncan Comprehensive Cancer Center, Baylor College of Medicine, Houston, TX, United States
- 4Systems Onco-Immunology Laboratory, David J. Sugarbaker Division of Thoracic Surgery, Michael E. DeBakey Department of Surgery, Baylor College of Medicine, Houston, TX, United States
- 5Baylor College of Medicine, Michael E. DeBakey VA Medical Center, Houston, TX, United States
Pancreatic adenocarcinoma (PDAC) is an aggressive tumor with poor survival and limited treatment options. PDAC resistance to immunotherapeutic strategies is multifactorial, but partially owed to an immunosuppressive tumor immune microenvironment (TiME). However, the PDAC TiME is heterogeneous and harbors favorable tumor-infiltrating lymphocyte (TIL) populations. Tertiary lymphoid structures (TLS) are organized aggregates of immune cells that develop within non-lymphoid tissue under chronic inflammation in multiple contexts, including cancers. Our current understanding of their role within the PDAC TiME remains limited; TLS are complex structures with multiple anatomic features such as location, density, and maturity that may impact clinical outcomes such as survival and therapy response in PDAC. Similarly, our understanding of methods to manipulate TLS is an actively developing field of research. TLS may function as anti-tumoral immune niches that can be leveraged as a therapeutic strategy to potentiate both existing chemotherapeutic regimens and potentiate future immune-based therapeutic strategies to improve patient outcomes. This review seeks to cover anatomy, relevant features, immune effects, translational significance, and future directions of understanding TLS within the context of PDAC.
1 Introduction
Although pancreatic adenocarcinoma (PDAC) accounts for approximately 3% of all cancer in the US, it is projected to become the 2nd leading cause of cancer-related deaths in the US by 2030 (1). The prognosis for patients with PDAC remains poor with <10% five-year overall survival and modest survival benefit from standard treatment regimens (2). Immunotherapy has changed the paradigm in modern oncologic therapy for cancers such as melanoma and non-small cell lung cancer (3–6). Unfortunately, immune checkpoint inhibitor (ICI) therapy has proven ineffective for PDAC outside of the rare few with high microsatellite instability (7–12). Additionally, other strategies such as TCR therapy, CAR-T therapy, tumor-infiltrating lymphocyte (TIL) therapy, and mRNA vaccines are still in their nascency (13–15).
Immunotherapy failure has partially been attributed to the immunosuppressive tumor immune microenvironment (TiME) of PDAC (16). The PDAC TiME is complex and significant tumor immune heterogeneity exists both within the tumor, the peritumoral microenvironment, and across patients (17–20). The cellular constituents of the PDAC TiME consists of multiple immunosuppressive cell lines, including regulatory T cells (Tregs), myeloid-derived suppressor cells (MDSCs), and tumor-associated macrophages (TAMs) (16, 21–30). These features of the TiME contribute to PDAC tumorigenesis, suppression of cytotoxic T cell priming and function (16, 31–34), and T cell exhaustion (29, 35, 36). However, favorable TILs also exist in the TiME such as CD4+ and CD8+ T cell infiltrates (17, 18, 37). Multiple reports have identified an association between higher degrees of CD4+ and CD8+ T cell infiltration with improved survival in PDAC (38–40). Furthermore, the spatial organization of these immune infiltrates impact survival (19, 38), suggesting that a deeper understanding of the architecture and spatial organization of immune cell populations within the PDAC TiME is required to better appreciate their clinical and translational relevance. Tertiary lymphoid structures (TLS) represent an essential component of this immune spatial organization and are organized aggregates of immune cells that arise in nonlymphoid tissue. A growing body of evidence link TLS formation to improved clinical outcomes in a variety of cancers (41–43). This review will define TLS anatomy, the clinical significance, and translational implications for PDAC TLS to establish a deeper understanding of opportunities to harness anti-tumoral immunity that can translate to improved patient outcomes.
2 Anatomy of tertiary lymphoid structures
TLS have been identified in multiple inflammatory disease states (44) such as rheumatoid arthritis (45), Sjogren’s disease (46), autoimmune diabetes (47), and various cancers (43). In contrast to lymph nodes, TLS are non-encapsulated organized lymphocyte aggregates that can form in non-lymphoid tissues undergoing chronic inflammatory stress such as infection, transplantation, and cancer (48). In human malignancies, TLS generally contain the major constituents of adaptive immunity, with zones containing B cells, T cells, and a stromal network of follicular dendritic cells (FDCs) (49). High endothelial venules (HEVs) can also surround TLS to allow immune cell trafficking (50). Interestingly, in PDAC, small nerve fibers have been shown to exist in these aggregates and the density of such fibers has been associated with a prolonged overall survival (51).
TLS formation can be broadly defined by three main phases: fibroblast activation, immune cell recruitment, and maturation (52) (Figure 1). Several cytokines such as IL-13, IL-17 and IL-22 are implicated in the initial priming of local fibroblasts by immune cells undergoing inflammatory stress (53, 54). At this stage, immature structures are formed, and their maintenance requires the retention of existing immune cells as well as the recruitment of further immune populations. Continuous local antigen presentation (55) and chemokines such as CXCL13 and others play a key role in these two processes respectively (56). If stressors persist, TLS maturation progresses with the formation of HEVs and germinal centers (GCs). Within PDAC, the B lymphocyte chemoattractant CXCL13 may be of particular relevance, as immunofluorescence studies of PDAC TLS demonstrate an abundance of CXCL13 distributed throughout TLS (57, 58). Furthermore, ectopic CXCL13 expression triggers TLS formation in the pancreas in both cancerous (59) and noncancerous (58) contexts. Other earlier studies also revealed the critical role of chemokines such as TCA4/SLC and CXCL13 (also known as B lymphocyte chemoattractant) (60) in pancreatic lymphoid neogenesis.
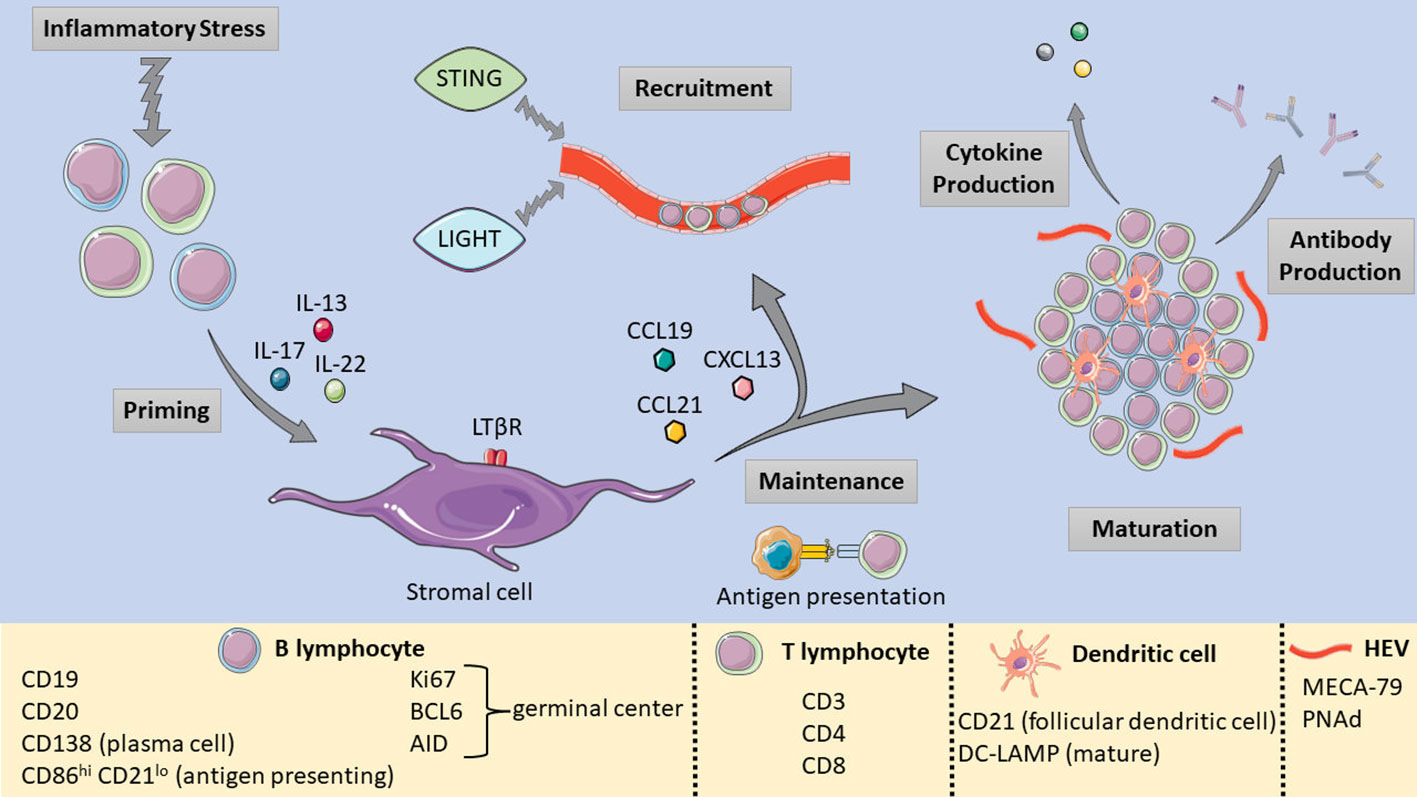
Figure 1 Pictorial summary of TLS formation, maturation, and the relevant markers used to identify TLS components. [Shapes and images are imported from Servier Medical Art by Servier (http://smart.servier.com/), accessed on May 19, 2023. Licensed under a Creative Commons Attribution 3.0 Unported License (https://creativecommons.org/licenses/by/3.0/)].
3 Mature and immature tertiary lymphoid structures
The maturation from a loose aggregate of B cells and T cells into a mature, functional lymphoid structure impacts the TiME and carries broader clinical significance. TLS can undergo multiple stages of maturity (Figure 2), which have been defined by different criteria from different research groups. Earlier distinctions were typically made based on the presence of GCs within mature TLS compared to immature TLS, which were vaguely organized lymphocytic clusters without GCs (61, 62). More recently, one group defined three phenotypes of TLS maturity in a lung cancer model based on immune cell constituents and anatomical organization (63). Early TLS were dense lymphocytic clusters of CD21− CD23− B and T cells without FDCs or GCs localized near CXCL13-expressing perivascular cells, intermediate-stage or “primary follicle-like” TLS contained CD21+ CD23− FDCs without GCs, and mature or “secondary follicle-like” TLS contained CD21+ CD23+ GCs in addition to the features of TLS in earlier stages of maturation. These maturity phenotypes were later validated in a colorectal cancer model from 109 patient-derived non-metastatic tumor specimens (64). Within studies of PDAC TLS, definitions of immature and mature TLS have varied between studies. Many groups defining PDAC TLS rely upon histological examination with hematoxylin and eosin staining to identify TLS with organized B cell and T cell zones, germinal centers, and HEVs, with immunohistochemical staining of surrogate markers such as peripheral lymph node addressin (PNAd) or Ki67 implying TLS maturity (65, 66), but do not directly identify early or intermediate TLS in comparison to mature TLS. Others fully characterize TLS based on distinct CD3+ and CD20+ B cell and CD8+ T cell zones with CD21+ FDCs and (PNAd+) HEVs (59, 67), providing a more specific definition of PDAC TLS maturity.
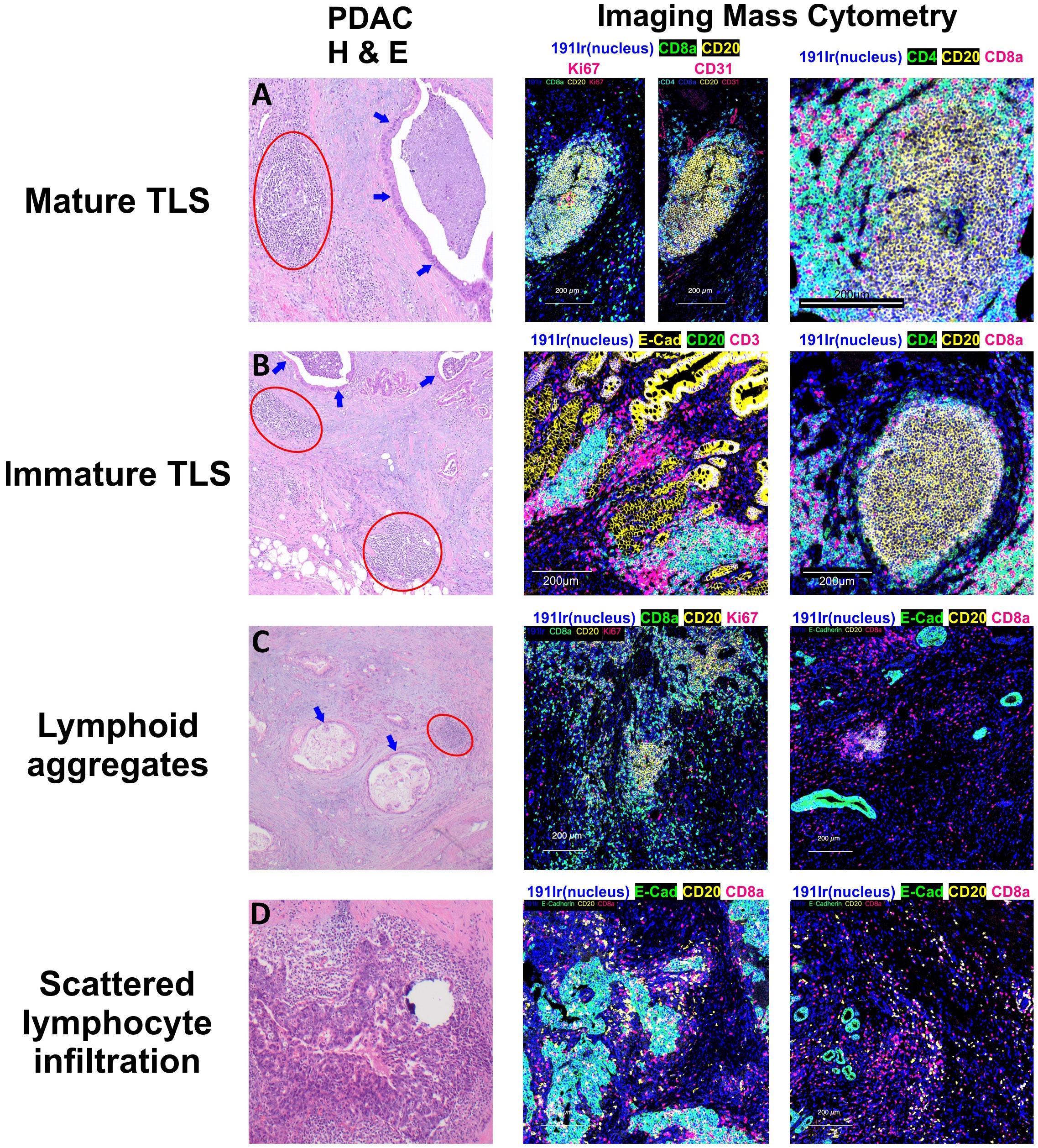
Figure 2 Patterns of Lymphocyte Infiltration in Pancreatic Adenocarcinoma. Tertiary lymphoid structures (TLS) in pancreatic adenocarcinoma display varying stages of maturation. (A) Mature TLSs comprise B cells, T cells, and follicular dendritic cells, and are distinguished by the presence of germinal centers containing Ki67(+) B cells. (B) In contrast, the majority of the observed TLSs are in an immature stage, lacking germinal centers but still exhibiting distinct B cell and T cell zones. (C) Apart from these structured aggregates, there are lymphoid aggregates which represent a disorganized accumulation of both B and T lymphocytes. (D) Moreover, individual B and T cells can also be observed dispersed within the tumor or surrounding the tumor cells. Circles in red show lymphoid structures, and arrows in blue display invasive adenocarcinoma with luminal necrosis.
GCs are an essential component of peripheral lymphoid organs and important sites of antigen-driven somatic hypermutation and memory B cell and plasma cell maturation (68). Similarly, the GCs of mature TLS contain proliferating B cells and DC-LAMP+ FDCs (69), characterized by Ki67, activation-induced cytidine deaminase (AID) and BCL6 expression (67, 70). HEVs are also present in these structures and are classically identified by the presence of an L-Selectin ligand, MECA-79, and PNAd (71). These HEVs play an essential role in mature TLS function by mediating immune cell trafficking (72) via binding of L-Selectin to PNAd (73).
Evidence from diabetic mice with autoimmune insulitis revealed that functional and mature TLS within the pancreas can harbor differentiated autoreactive B cells that express the activation-induced cytidine deaminase (AID) enzyme which mediates immunoglobulin class switching and affinity maturation (70). As with other neoplasms, expression of Ki67, BCL6 and CD21 in B cell zones is indicative of mature PDAC TLS, with GCs containing B cells undergoing affinity maturation and somatic hypermutation (67). In murine PDAC models, the presence of HEVs in mature TLS have been shown to facilitate lymphocytic infiltration (74) and enhance their activation via LTβR signaling (75).
However, TLS in cancers exhibit significant heterogeneity in maturation, and can also vary in maturity depending on tumor stage (76) or metastatic site (77). PDAC may present a unique challenge in understanding TLS maturity, as the PDAC TiME exhibits considerable immune heterogeneity (78, 79) and lower proportions of TLS are found in PDAC compared to other cancers (43). Relatively few of TLS found in PDAC are mature and possess GCs (67). A timeline of TLS maturity has been proposed (63). Although it remains unclear whether the various phenotypes in PDAC represent a stepwise progression of maturation or are instead terminally differentiated and unable to eventually transform into mature TLS. Understanding these nuances may improve both our knowledge of the PDAC TLS as well as broaden our understanding of the PDAC TiME as a whole.
4 Location and density
TLS can be located either intratumoral or peritumoral. TLS location has significant anatomical and functional implications. In cancers such as melanoma, increased peritumoral mature TLS density is associated with longer survival (74). PDAC TLS formation is more abundant in the invasive front of the tumor and peritumorally compared to the tumor center (80). One study conducted on more than 300 PDAC human samples demonstrated that 84% of the examined samples possessed only peritumoral TLS, while only 16% contained intratumoral TLS (65). Interestingly, a more recent study demonstrated that despite being less plentiful, the intratumoral TLS had a greater B and T cell infiltration, less immunosuppressive populations, a significant Th1 and Th17 pro-inflammatory genetic polarization, and were associated with improved survival (65). The intratumoral TLS were also more mature with intact nerve networks and organized vasculature formed by mature endothelial cells circled by pericytes. PDAC is characterized by a desmoplastic, fibrotic stroma (81–83), which may necessitate a spatial adjacency of TLS to tumor for its anti-tumoral effects to manifest.
5 Relevant cell populations within PDAC TLS
As TLS share morphological and cellular similarities with traditional secondary lymphoid organs, they may also recapitulate some of their functionality. B cells are an essential component of TLS and are present at all stages of TLS maturity (66, 69). Data from the study of TLS in autoimmune disease support the idea that B cells within the TLS GC undergo somatic hypermutation and clonal expansion in rheumatoid arthritis (84), Sjogren’s disease (85), myasthenia gravis (86), and autoimmune diabetes. TLS-associated B cells are also capable of terminal differentiation into antibody-secreting plasma cells (87). Similar to the data from autoimmune TLS research, cancer TLS-associated B cells are proliferative, experience somatic hypermutation, and undergo maturation into antibody-secreting plasma cells (69, 88). These plasma cells also produce tumor-specific antibodies (69, 88, 89) capable of binding to tumor cells and enhancing immunotherapy (88), suggesting that the TLS B cell population fulfills an antitumoral niche within the more generalized B cell infiltrate in tumors.
Data from retrospective studies and preclinical models in PDAC highlight the antitumoral function of the TLS-associated B cell population. One group showed that TLS+ PDAC tumors have a higher proportion of memory B cells and memory IgG1 class-switched B cells compared to TLS- tumors (67), while another group demonstrated that TLS-associated B cell infiltrates in a KPC PDAC murine model exhibited an immunostimulatory phenotype, with upregulation of proinflammatory (SPP1, IL6, CSF2, VEGFA, CCL4, PTGS2) and T cell chemotaxis (CXCL1, CXCL2, CXCL5, CCL2, CXCL12, CCL20) genes, along with downregulation of immunosuppressive genes (CD274 and IL12a) (90, 91).
T cells are an essential component of the TLS (49). As higher CD8 T cell tumor infiltration is associated with increased survival across cancers (92–94), understanding the interaction of CD8 T cell populations and TLS is of particular interest. Preclinical autoimmune diabetes models have linked TLS with naïve T cell recruitment and proliferation (95). Within a cancer-specific context, LIGHT expression in a fibrosarcoma model induced the formation of TLS, naïve T cell recruitment, T cell proliferation and priming, and subsequent tumor regression (96). A preclinical colorectal cancer model showed that TLS were associated with CD3+ T cell infiltration and mediated GFP+ splenocyte recruitment (97), and a preclinical melanoma model demonstrated an association between TLS and tumor-specific T cell responses independent of secondary lymphoid tissue (98).
Multiple retrospective studies from human PDAC samples show an association between TLS formation and increased CD8 T cell infiltration (91, 99, 100). For example, evidence from one group demonstrated that T cells were enriched in mature TLS with PNAd+ HEVs, TLS presence correlated with greater intratumoral and circulation CD8 T cell populations, and the TLS+ tumor stroma had a higher CD8/Treg ratio (100). Based on a PDAC tissue microarray, another investigation identified a “TLS rich” immune PDAC subtype with higher expression of T cells (CD8, CD3, and CD4) and B cells (CD20) markers and lower in Treg (FOXP3) markers (101).
The mechanistic interactions between CD8 T cells and the PDAC TLS in PDAC are just beginning to be explored and suggest that PDAC TLS are active participants in T cell maturation and trafficking. Naïve T cell infiltration appears dependent upon PNAd+ CCL21+ HEVs seen in mature TLS (102). Data from a tumor vaccine-induced human PDAC TLS cohort demonstrated high expression of the early T cell activation marker CD69 and T cell trafficking receptor CXCR3 within TLS, implicating their role in T cell activation (103). Furthermore, Vβ2-positive T cells are present in the center of PDAC TLS, suggesting that TLS are sites of T cell clonal expansion (104).
Additional anti-tumoral efficacy may be related to T follicular helper cells (Tfh), which are a component of TLS (105) associated with improved prognosis in cancers (43, 106, 107). PDAC Tfh from patients who received neoadjuvant chemotherapy exhibited increased CD8 T cell and B cell recruitment capability compared to treatment-naïve Tfh (108).
Interestingly, cancer-associated fibroblasts (CAFs) may also play a role in TLS function. PDAC CAFs are typically associated with tumorigenic (109) and immunosuppressive roles (110). However, diverse CAF subpopulations (111, 112) exist within PDAC. Nonspecific ablation of CAFs induces both immune remodeling and a more aggressive tumor phenotype (113, 114), suggesting that an immunologically favorable and tumor suppressive CAF subpopulation exists within PDAC. Similar to other cancers, where CAF subtypes can promote TLS formation (115), a link between PDAC CAFs and PDAC TLS may exist. A recent study described associations between CAF-derived TGF-β with increased TLS gene signatures within PDAC (57). Furthermore, the authors observed that the expression of CXCL13 on CD4 and CD8 T cells occurred in a TGF-β dependent fashion, highlighting the interaction between PDAC TLS and CAFs.
However, this immunologically favorable CAF-TLS interaction in PDAC is still relatively undefined. For one, if the immunologically favorable CAF subtype exists, it is not fully characterized. A potential biomarker of this putative CAF subtype may be podoplanin (PDPN), a lymphatic endothelial and CAF marker (116, 117). PDPN expression on fibroblasts can promote TLS establishment (54), while recent studies in PDAC demonstrated that a PDPN+ CAF subtype (112, 118) was associated with improved prognosis and enrichment of immune-related pathways, However, the role of PDPN in cancer is controversial, and associated with worse outcomes in multiple cancers, including PDAC (119, 120). A more thorough understanding of CAFs and CAF plasticity in PDAC (120) may provide clarification. 6 Clinical significance of PDAC TLS.
In a variety of cancers, mature TLS are associated with improved progression-free survival (41–43, 63, 64, 121) and response to immunotherapy (122). Similarly, a preponderance of data from human PDAC samples have linked TLS with improved survival (Table 1) (65, 67, 91, 99–101, 123, 124). Multiple groups demonstrated a survival benefit with the presence of mature TLS (98, 100, 103). Other have reported the prognostic presence of with intratumoral TLS (123). One of the first groups to report on TLS in PDAC examined surgical specimens from 308 treatment-naïve PDAC patients and identified mature TLS based on histopathological examination (65). Within their study cohort and an additional validation cohort of 226 patients, 84% of samples contained peritumoral TLS, while 16% contained intratumoral TLS. The presence of intratumoral TLS conferred a significant survival benefit, with a median survival of 42.7 months compared to 29.4 months for those with only peritumoral TLS. The presence of intratumoral TLS also correlated with increased CD4 and CD8 T cell infiltration and decreased Treg and M2 macrophage infiltration in the TiME, suggestive of a more anti-tumoral and immunogenic TiME. These findings are corroborated by other retrospective studies examining TLS in surgical PDAC specimens (67, 99, 101).
Importantly, many of these studies describing mature TLS are based upon histological examination and identification of TLS based on the presence of GCs and surrogate markers of maturity such as PNAd+ HEVs (65, 93, 101, 124) and are likely excluding early and immature TLS from analysis. Precise characterization of TLS maturity may have clinical ramifications, as one study reports early PDAC TLS having greater CD8+ T cell infiltration and being enriched for IgG1 class-switched memory B cells and memory CD4+ T cells (67). Using the Cancer Genome Atlas Program PDAC datasets, early TLS are found to harbor decreased tumor molecular burden, but mature TLS expressed more neoantigens and increased B cell somatic hypermutation (78).
Aside from their impact on CD8 T cells, mature TLS with GCs were enriched for other immunologically relevant immune cell phenotypes, including activated CD4+ memory cells, naïve B cells, and NK cells (67). The interaction between TLS and B cells is noteworthy; in cancers, the role of B cell infiltration is controversial, and has been linked to both pro-tumorigenic (125–127) and anti-tumorigenic (69, 91, 97) states. In human PDAC samples, the spatial distribution of B cells is predominantly within either TLS or at the tumor-stromal interface (91). Interestingly, only TLS-associated B cells were associated with improved prognosis, while an immune signature associated with increased TLS-associated B cells and low B cell TILs predicted longer overall survival (91), supporting the clinical relevance of a TLS-specific subset of B cells within PDAC.
6 Preclinical evidence for targeting TLS
As TLS are associated with favorable TiME characteristics such as an increased level of CD8+ TILs (43, 128), targeting PDAC TLS may improve patient outcomes. Multiple studies (129–132) support the claim that standard of care chemotherapy can favorably alter the PDAC TiME to create an anti-tumoral immune niche. Evidence from matched pre- and post-neoadjuvant treated APC germline mutated hepatoblastoma samples suggested that cisplatin can induced immature TLS formation (133). Within PDAC, a preclinical TLS model established that TLS formation can be induced after intratumor injections of chemokines CXCL13 and CCL21 in an orthotopic pancreatic cancer mouse model (60). Furthermore, coadministration of gemcitabine with these lymphoid chemokines led to significant tumor reduction (60). It should be noted that the sole administration of gemcitabine within this study globally reduced immune cell infiltration within the tumors, while combination chemokine and gemcitabine therapy ameliorated this effect.
Vaccine-based PDAC immunotherapy trials in PDAC also highlight the role of TLS in immunotherapy. Vaccinating mice that spontaneously develop PDAC KrasG12D Pdx1-Cre (KPC) tumors with an α-enolase (ENO1) encoding vector led to a spatial reorganization of the TiME that is marked by TLS induction. These TLS had a higher number of PD-1+ germinal center and vaccinated mice had an ameliorated infiltration of antigen-specific T cells (91). A randomized clinical trial with administration of a granulocyte-macrophage colony-stimulating factor (GM-CSF)-allogeneic pancreatic tumor cell vaccine (GVAX) demonstrated the induction of mature TLS formation alone or in combination with low dose cyclophosphamide (134). These vaccine-induced TLS possessed multiple antitumoral attributes such as effector T cell trafficking, T cell activation, and differential expression of immune-regulating pathways. These induced TLS also expressed PD-1 and PD-L1, suggesting the benefit of combination with ICI therapy.
7 PDAC TLS and therapy response
TLS presence is associated with neoadjuvant therapy response in cancers (96, 135, 136). Data on TLS and response to chemotherapy in PDAC remains limited. One group compared a total of 140 resected PDAC specimens from patients who received up-front surgery to those who received neoadjuvant therapy. The TLS from the neoadjuvant therapy group possessed higher proportions of CD8 T cells and lower proportions of PD-1 expressing lymphocytes compared to those from the up-front surgery group, suggesting the induction of an antitumoral niche within TLS through neoadjuvant therapy (66). Another retrospective analysis of 162 patients found that PDAC patients with TLS had longer cancer-specific survival when treated with 5FU-based therapy compared to gemcitabine-based or no adjuvant therapy (124). As platinum-based therapies such as cisplatin and oxaliplatin can interact with the TiME by inducing cytotoxic T cell activity through immunogenic cell death (137–140), the choice of chemotherapy may impact the predictive value of TLS. In contrast, a recent study of 380 treatment-naïve PDAC patients and 136 PDAC patients treated with predominantly gemcitabine-based neoadjuvant chemotherapy demonstrated significant alterations of the neoadjuvant-treated intratumoral TLS (123). The neoadjuvant-treated samples possessed fewer intratumoral TLS compared to the treatment-naïve samples, and the TLS within the neoadjuvant-treated group had significantly lower B cell proportions and higher Treg and macrophage proportions compared to their untreated counterparts (123). Furthermore, in contrast to the treatment-naïve group, the presence of intratumoral TLS within the neoadjuvant-treated group was no longer significantly associated with survival, suggesting that interactions between PDAC TLS and chemotherapy may depend on multiple factors such as the choice of chemotherapy, duration of therapy, or initiation with adjuncts such as corticosteroids (141).
Recent studies have also evaluated the favorable interaction between TLS and immunotherapy across a spectrum of cancers (142–144). As PDAC responds poorly to immune checkpoint inhibitors (ICIs) (9, 10), understanding the anti-tumoral and ICI-potentiating benefit of PDAC TLS becomes critical to improving treatment efficacy. Data from a cohort of 328 patients treated with immunotherapy, including a subset of patients diagnosed with PDAC, identified that mature TLS presence was correlated with increased CD8 T cell density, was predictive of response to ICIs, and an independent predictor of progression free and overall survival (122). These findings also suggest TLS may serve as marker for favorable outcome with FOLFIRINOX and can identify patients for future chemo-immunotherapy trials.
Similarly, as data are beginning to evolve regarding the role of TLS with immunotherapy responses in several aggressive cancer types, a number of clinical trials assessing immunotherapy as an effective treatment strategy for pancreatic ductal adenocarcinoma have been conducted (NCT03214250, NCT03404960, NCT02879318, NCT01836432). However, no trials have also investigated the role of immunotherapy with induction or presence of TLS or in respect to other modulations of the TiME in PDAC. This underscores the need for additional translational studies investigating the role of both TLS and TiME as they relate to immunotherapy responses in PDAC.
8 Future directions to enhance TLS function
As our understanding of the role of TLS in cancers improves, the translational utility of manipulating TLS has become an area of interest as well. As an initial step within the clinical setting, several well-known markers could be used to identify TLS. These could include CD3, CD4 and CD8 for T cells, CD19 or CD20 for B cells, CD21 for FDCs, CD138 for plasma cells, DC-LAMP for mature FDCs, as well as other activation markers (48). Antigen presenting B cells with a high expression of CD86 and a low expression of CD21 have been shown to localize in TLS in several cancers (145).
A chemokine-based “12-CK score” (CXCL9, CXCL10, CXCL11, CXCL13, CCL2, CCL3, CCL4, CCL5, CCL8, CCL18, CCL19 and CCL21) was initially proposed and developed based on colorectal cancer samples (146). Subsequently, this score has been validated as a TLS transcriptomic signature with prognostic significance in several neoplasms (147). A similar 12-chemokine gene signature (CCL2, CCL3, CCL4, CCL5, CCL8, CCL18, CCL19, CCL21, CXCL9, CXCL10, CXCL11, and CXCL13) was derived through metagene analysis and correlated with enhanced patient survival in colorectal cancer (146) independent of tumor stage, location, microsatellite stability status, or treatment. As a single marker, IL-2 levels could be used as a potential biomarker for PDAC TLS formation, as analysis of paired blood and tumor samples from PDAC patients revealed the existence of a “stroma-to-serum” gradient in patients that lack TLS and an association between lower serum IL-2 levels and TLS formation (99). These findings highlight the potential of using serum biomarkers to predict response to immunotherapy; however, additional work needs to be done to determine the optimal time of IL2 monitoring.
Looking ahead, preclinical and clinical studies of the molecular manipulation of TLS may inform PDAC TLS strategies. For example, Tregs play an important role in TLS maintenance and can dampen anti-tumor responses in secondary lymphoid organs, e.g., lymph nodes and spleen. Tregs express high amounts of IL2 receptor as well as immunoinhibitory receptor for PD-1. Infiltration of Tregs into lung adenocarcinoma mouse models was found to suppress anti-tumor responses in TLS; ablation of Tregs induces strong effector T cell responses and tumor destruction (148, 149). Treg deletion may be another promising therapeutic option.
Lymphotoxins belong to the tumor necrosis factor (TNF) superfamily and play an important role in lymphoid tissue organogenesis and may be associated with TLS development (150). Lymphotoxin beta receptor (LTβR) signaling has been shown to mediate the formation of HEVs (72) and FDCs (151). Another lymphotoxin, LIGHT/TNFSF24, increases lymphoid penetration and lead to changes in the TIME, such as the development of TLS (152, 153). Evidence from more than two decades ago revealed that the ectopic expression of chemokines like CCL19 and CCL21 in pancreatic islets led to small-sized infiltrates of lymphocytes with stromal cells and HEVs (154, 155). Other earlier studies also revealed the critical role of chemokines such as TCA4/SLC (60) in pancreatic lymphoid neogenesis. In an orthotopic murine mesothelioma model expressing human mesothelin, treatment with a mesothelin-targeted Fab linked to a toxin eradicated tumors and induced TLS formation (156). Knowing that mesothelin is expressed in almost all pancreatic ductal adenocarcinomas (157), its potential role as a therapeutic target to induce TLS formation in PDAC warrants further investigation.
Looking toward evidence from other preclinical cancer models, other methods to induce TLS formation may be applicable to PDAC. Stimulator of interferon genes, or STING, is a double-stranded DNA sensor, that along with STING agonists, can activate antitumoral immune responses (158). One group demonstrated that intratumoral injection of ADU S-100, a STING agonist, in a preclinical melanoma model promoted formation of CCL19+, CCL21+, PNAd+ TLS, although these TLS did not contain significant B cell infiltration or GCs (159). Similarly, oral administration of an oncolytic virus (160) carrying a vector expressing IL-15 produced similar results in lung cancer and melanoma mouse models (161). This is intriguing not only for the potential of inducing PDAC TLS formation through delivery of lymphogenic cytokines, but the use of oncolytic viruses in PDAC (162) may serve as an effective method to overcome barriers to drug delivery in PDAC (134, 163). This could also be adapted to potentiate the function of existing TLS by inducing immunogenic cell death (164) to enhance T cell mediated immune responses that may originate from pre-existing PDAC TLS.
Other future strategies to potentiate PDAC TLS function may target specific cell populations found within the TLS. The PDAC immune microenvironment possesses immune checkpoint heterogeneity (17), with a distinct subset of exhausted CD8 TILs expressing T-cell immunoglobulin and ITIM domain (TIGIT) (165). A recent study demonstrated the presence of CD8 T cells expressing both PD-1 and TIGIT which were located predominantly within PDAC TLS (166). PD-1+ TIGIT+ Cd8 T cells are associated with worse prognosis in cancer (167), and the relationship between this dual immune checkpoint expressing T cell phenotype and the PDAC TLS have yet to be elucidated. However, dual checkpoint blockade against PD-1 and TIGIT partially restored T cell proliferation and cytokine production (166), suggesting a potential therapeutic avenue for potentiating a TLS-mediated T cell response. CAF-specific therapy strategies targeting CAF plasticity may also be of potential benefit. Multiple phenotypically diverse CAF subtypes have been described in PDAC (112, 116, 118, 121). While the majority of CAF subtypes possess tumorigenic and immunosuppressive capabilities, evidence suggests that a minority of PDAC CAFs are associated with upregulation of immune signatures and TLS formation (57, 112, 118). Strategies involving CAF reprogramming (168, 169) may provide a useful adjunct to TLS-focused strategies in the future.
9 Conclusions
As our understanding of PDAC TLS continues to evolve, it is becoming increasingly evident that they hold promise as a prognostic marker and therapeutic target. The manipulation of TLS may pave the way for novel diagnostic tools, innovative immunotherapeutic strategies, combination treatments, and ultimately improving clinical outcomes for a patient population in need of improved outcomes. Moving forward, further research and clinical trials are warranted to capitalize on the potential of TLS as an integral element in the fight against PDAC.
Author contributions
ZG: Conceptualization, Writing – original draft, Writing – review & editing. JA: Visualization, Writing – review & editing. HZ: Writing – review & editing. SW-P: Writing – review & editing. SK: Data curation, Visualization, Writing – review & editing. CM: Visualization, Writing – review & editing. MR: Writing – review & editing. SM: Writing – review & editing. H-SL: Visualization, Writing – review & editing. EC: Writing – review & editing, Funding acquisition, Project administration, Supervision.
Funding
The author(s) declare financial support was received for the research, authorship, and/or publication of this article. This work was supported in part by Merit Review Award # I01 CX001880-01A1 from the United States (U.S.) Department of Veterans Affairs Biomedical Laboratory Research and Development Program (ERC), and by an award from the National Pancreatic Cancer Foundation. Additional support was provided by a pilot grant from a Cancer Prevention and Research Institute of Texas grant (Lee: CPRIT RP200443), an NIH R21 (Lee: R1AI159379), a US Department of Defense Impact Award (Lee: W81XWH-22-1-0657), the Helis Medical Research Foundation (Lee), and the Dan L Duncan Comprehensive Cancer Center, which is partly funded by the NIH grant P30 CA125123 (Camp & Lee). This project was also supported by the Cytometry and Cell Sorting Core at Baylor College of Medicine with funding from the NIH (NCI P30CA125123 and NCRR S10RR024574) and CPRIT (RP180672) and the assistance of Joel M. Sederstrom.
Conflict of interest
The authors declare that the research was conducted in the absence of any commercial or financial relationships that could be construed as a potential conflict of interest.
Publisher’s note
All claims expressed in this article are solely those of the authors and do not necessarily represent those of their affiliated organizations, or those of the publisher, the editors and the reviewers. Any product that may be evaluated in this article, or claim that may be made by its manufacturer, is not guaranteed or endorsed by the publisher.
References
1. Rahib L, Smith BD, Aizenberg R, Rosenzweig AB, Fleshman JM, Matrisian LM. Projecting cancer incidence and deaths to 2030: the unexpected burden of thyroid, liver, and pancreas cancers in the united states. Cancer Res (2014) 74(11):2913–21. doi: 10.1158/0008-5472.CAN-14-0155
2. Conroy T, Desseigne F, Ychou M, Bouche O, Guimbaud R, Becouarn Y, et al. FOLFIRINOX versus gemcitabine for metastatic pancreatic cancer. N Engl J Med (2011) 364(19):1817–25. doi: 10.1056/NEJMoa1011923
3. Couzin-Frankel J. Cancer immunotherapy. Science (2013) 342(6165):1432–3. doi: 10.1126/science.342.6165.1432
4. Topalian SL, Drake CG, Pardoll DM. Immune checkpoint blockade: a common denominator approach to cancer therapy. Cancer Cell (2015) 27(4):450–61. doi: 10.1016/j.ccell.2015.03.001
5. Ribas A, Wolchok JD. Cancer immunotherapy using checkpoint blockade. Science (2018) 359(6382):1350–5. doi: 10.1126/science.aar4060
6. Farkona S, Diamandis EP, Blasutig IM. Cancer immunotherapy: the beginning of the end of cancer? BMC Med (2016) 14(1):73. doi: 10.1186/s12916-016-0623-5
7. Le DT, Durham JN, Smith KN, Wang H, Bartlett BR, Aulakh LK, et al. Mismatch repair deficiency predicts response of solid tumors to PD-1 blockade. Science (2017) 357(6349):409–13. doi: 10.1126/science.aan6733
8. Brahmer JR, Tykodi SS, Chow LQ, Hwu WJ, Topalian SL, Hwu P, et al. Safety and activity of anti-PD-L1 antibody in patients with advanced cancer. N Engl J Med (2012) 366(26):2455–65. doi: 10.1056/NEJMoa1200694
9. O'Reilly EM, Oh DY, Dhani N, Renouf DJ, Lee MA, Sun W, et al. Durvalumab with or without tremelimumab for patients with metastatic pancreatic ductal adenocarcinoma: A phase 2 randomized clinical trial. JAMA Oncol (2019) 5(10):1431–8. doi: 10.1001/jamaoncol.2019.1588
10. Royal RE, Levy C, Turner K, Mathur A, Hughes M, Kammula US, et al. Phase 2 trial of single agent ipilimumab (anti-CTLA-4) for locally advanced or metastatic pancreatic adenocarcinoma. J Immunother. (2010) 33(8):828–33. doi: 10.1097/CJI.0b013e3181eec14c
11. Wong W, Alouani E, Wei A, Ryu YK, Chabot JA, Manji GA. Future of immunotherapy in pancreas cancer and the trials, tribulations and successes thus far. Semin Oncol (2021) 48(1):57–68. doi: 10.1053/j.seminoncol.2021.02.007
12. Patel GK, Perry JB, Abdul-Rahim O, Frankel AE, Cameron D, Taylor W, et al. Epidermal growth factor receptor-activating mutation(E746_T751>VP) in pancreatic ductal adenocarcinoma responds to erlotinib, followed by epidermal growth factor receptor resistance-mediating mutation (A647T): A case report and literature review. J Cancer Res Ther (2020) 16(4):950–4. doi: 10.4103/jcrt.JCRT_729_18
13. Leidner R, Sanjuan Silva N, Huang H, Sprott D, Zheng C, Shih YP, et al. Neoantigen t-cell receptor gene therapy in pancreatic cancer. N Engl J Med (2022) 386(22):2112–9. doi: 10.1056/NEJMoa2119662
14. Yeo D, Giardina C, Saxena P, Rasko JEJ. The next wave of cellular immunotherapies in pancreatic cancer. Mol Ther - Oncolytics. (2022) 24:561–76. doi: 10.1016/j.omto.2022.01.010
15. Rojas LA, Sethna Z, Soares KC, Olcese C, Pang N, Patterson E, et al. Personalized RNA neoantigen vaccines stimulate t cells in pancreatic cancer. Nature (2023) 618(7963):144–50. doi: 10.1038/s41586-023-06063-y
16. Ho WJ, Jaffee EM, Zheng L. The tumour microenvironment in pancreatic cancer - clinical challenges and opportunities. Nat Rev Clin Oncol (2020) 17(9):527–40. doi: 10.1038/s41571-020-0363-5
17. Steele NG, Carpenter ES, Kemp SB, Sirihorachai VR, The S, Delrosario L, et al. Multimodal mapping of the tumor and peripheral blood immune landscape in human pancreatic cancer. Nat Cancer. (2020) 1(11):1097–112. doi: 10.1038/s43018-020-00121-4
18. Bailey P, Chang DK, Nones K, Johns AL, Patch A-M, Gingras M-C, et al. Genomic analyses identify molecular subtypes of pancreatic cancer. Nature (2016) 531(7592):47–52. doi: 10.1038/nature16965
19. Carstens JL, Correa de Sampaio P, Yang D, Barua S, Wang H, Rao A, et al. Spatial computation of intratumoral t cells correlates with survival of patients with pancreatic cancer. Nat Commun (2017) 8(1):15095. doi: 10.1038/ncomms15095
20. Balli D, Rech AJ, Stanger BZ, Vonderheide RH. Immune cytolytic activity stratifies molecular subsets of human pancreatic cancer. Clin Cancer Res (2017) 23(12):3129–38. doi: 10.1158/1078-0432.CCR-16-2128
21. Bayne LJ, Beatty GL, Jhala N, Clark CE, Rhim AD, Stanger BZ, et al. Tumor-derived granulocyte-macrophage colony-stimulating factor regulates myeloid inflammation and t cell immunity in pancreatic cancer. Cancer Cell (2012) 21(6):822–35. doi: 10.1016/j.ccr.2012.04.025
22. Blair AB, Kim VM, Muth ST, Saung MT, Lokker N, Blouw B, et al. Dissecting the stromal signaling and regulation of myeloid cells and memory effector t cells in pancreatic cancer. Clin Cancer Res (2019) 25(17):5351–63. doi: 10.1158/1078-0432.CCR-18-4192
23. Clark CE, Hingorani SR, Mick R, Combs C, Tuveson DA, Vonderheide RH. Dynamics of the immune reaction to pancreatic cancer from inception to invasion. Cancer Res (2007) 67(19):9518–27. doi: 10.1158/0008-5472.CAN-07-0175
24. Vayrynen SA, Zhang J, Yuan C, Vayrynen JP, Dias Costa A, Williams H, et al. Composition, spatial characteristics, and prognostic significance of myeloid cell infiltration in pancreatic cancer. Clin Cancer Res (2021) 27(4):1069–81. doi: 10.1158/1078-0432.CCR-20-3141
25. Dias Carvalho P, Guimaraes CF, Cardoso AP, Mendonca S, Costa AM, Oliveira MJ, et al. KRAS oncogenic signaling extends beyond cancer cells to orchestrate the microenvironment. Cancer Res (2018) 78(1):7–14. doi: 10.1158/0008-5472.CAN-17-2084
26. Lee HS, Jang HJ, Choi JM, Zhang J, de Rosen VL, Wheeler TM, et al. Comprehensive immunoproteogenomic analyses of malignant pleural mesothelioma. JCI Insight (2018) 3(7). doi: 10.1172/jci.insight.98575
27. Zhang J, Wolfgang CL, Zheng L. Precision immuno-oncology: Prospects of individualized immunotherapy for pancreatic cancer. Cancers (Basel). (2018) 10(2). doi: 10.3390/cancers10020039
28. Dougan SK. The pancreatic cancer microenvironment. Cancer J (2017) 23(6):321–5. doi: 10.1097/PPO.0000000000000288
29. Saka D, Gokalp M, Piyade B, Cevik NC, Arik Sever E, Unutmaz D, et al. Mechanisms of t-cell exhaustion in pancreatic cancer. Cancers (Basel). (2020) 12(8). doi: 10.3390/cancers12082274
30. Bowers JS, Bailey SR, Rubinstein MP, Paulos CM, Camp ER. Genomics meets immunity in pancreatic cancer: Current research and future directions for pancreatic adenocarcinoma immunotherapy. Oncol Rev (2019) 13(2):430. doi: 10.4081/oncol.2019.430
31. Balachandran VP, Beatty GL, Dougan SK. Broadening the impact of immunotherapy to pancreatic cancer: Challenges and opportunities. Gastroenterology (2019) 156(7):2056–72. doi: 10.1053/j.gastro.2018.12.038
32. Sharma P, Hu-Lieskovan S, Wargo JA, Ribas A. Primary, adaptive, and acquired resistance to cancer immunotherapy. Cell (2017) 168(4):707–23. doi: 10.1016/j.cell.2017.01.017
33. Anderson KG, Stromnes IM, Greenberg PD. Obstacles posed by the tumor microenvironment to t cell activity: A case for synergistic therapies. Cancer Cell (2017) 31(3):311–25. doi: 10.1016/j.ccell.2017.02.008
34. Binnewies M, Roberts EW, Kersten K, Chan V, Fearon DF, Merad M, et al. Understanding the tumor immune microenvironment (TIME) for effective therapy. Nat Med (2018) 24(5):541–50. doi: 10.1038/s41591-018-0014-x
35. Zheng C, Fass JN, Shih YP, Gunderson AJ, Sanjuan Silva N, Huang H, et al. Transcriptomic profiles of neoantigen-reactive t cells in human gastrointestinal cancers. Cancer Cell (2022) 40(4):410–23 e7. doi: 10.1016/j.ccell.2022.03.005
36. Blando J, Sharma A, Higa MG, Zhao H, Vence L, Yadav SS, et al. Comparison of immune infiltrates in melanoma and pancreatic cancer highlights VISTA as a potential target in pancreatic cancer. Proc Natl Acad Sci USA (2019) 116(5):1692–7. doi: 10.1073/pnas.1811067116
37. Bailey P, Chang DK, Forget MA, Lucas FA, Alvarez HA, Haymaker C, et al. Exploiting the neoantigen landscape for immunotherapy of pancreatic ductal adenocarcinoma. Sci Rep (2016) 6:35848. doi: 10.1038/srep35848
38. Liu L, Zhao G, Wu W, Rong Y, Jin D, Wang D, et al. Low intratumoral regulatory t cells and high peritumoral CD8(+) t cells relate to long-term survival in patients with pancreatic ductal adenocarcinoma after pancreatectomy. Cancer Immunol Immunother. (2016) 65(1):73–82. doi: 10.1007/s00262-015-1775-4
39. Fukunaga A, Miyamoto M, Cho Y, Murakami S, Kawarada Y, Oshikiri T, et al. CD8+ tumor-infiltrating lymphocytes together with CD4+ tumor-infiltrating lymphocytes and dendritic cells improve the prognosis of patients with pancreatic adenocarcinoma. Pancreas (2004) 28(1):e26–31. doi: 10.1097/00006676-200401000-00023
40. Ino Y, Yamazaki-Itoh R, Shimada K, Iwasaki M, Kosuge T, Kanai Y, et al. Immune cell infiltration as an indicator of the immune microenvironment of pancreatic cancer. Br J Cancer. (2013) 108(4):914–23. doi: 10.1038/bjc.2013.32
41. Dieu-Nosjean M-C, Goc J, Giraldo NA, Sautès-Fridman C, Fridman WH. Tertiary lymphoid structures in cancer and beyond. Trends Immunol (2014) 35(11):571–80.
42. Schumacher TN, Thommen DS. Tertiary lymphoid structures in cancer. Science (2022) 375(6576):eabf9419.
43. Sautès-Fridman C, Petitprez F, Calderaro J, Fridman WH. Tertiary lymphoid structures in the era of cancer immunotherapy. Nat Rev Cancer. (2019) 19(6):307–25. doi: 10.1038/s41568-019-0144-6
44. Aloisi F, Pujol-Borrell R. Lymphoid neogenesis in chronic inflammatory diseases. Nat Rev Immunol (2006) 6(3):205–17. doi: 10.1038/nri1786
45. Humby F, Bombardieri M, Manzo A, Kelly S, Blades MC, Kirkham B, et al. Ectopic lymphoid structures support ongoing production of class-switched autoantibodies in rheumatoid synovium. PloS Med (2009) 6(1):e1. doi: 10.1371/journal.pmed.0060001
46. Bombardieri M, Barone F, Humby F, Kelly S, McGurk M, Morgan P, et al. Activation-induced cytidine deaminase expression in follicular dendritic cell networks and interfollicular large b cells supports functionality of ectopic lymphoid neogenesis in autoimmune sialoadenitis and MALT lymphoma in sjögren's syndrome. J Immunol (2007) 179(7):4929–38. doi: 10.4049/jimmunol.179.7.4929
47. Kendall PL, Yu G, Woodward EJ, Thomas JW. Tertiary lymphoid structures in the pancreas promote selection of b lymphocytes in autoimmune diabetes. J Immunol (2007) 178(9):5643–51. doi: 10.4049/jimmunol.178.9.5643
48. Colbeck EJ, Ager A, Gallimore A, Jones GW. Tertiary lymphoid structures in cancer: drivers of antitumor immunity, immunosuppression, or bystander sentinels in disease? Front Immunol (2017) 8:1830.
49. Drayton DL, Liao S, Mounzer RH, Ruddle NH. Lymphoid organ development: from ontogeny to neogenesis. Nat Immunol (2006) 7(4):344–53. doi: 10.1038/ni1330
50. Martinet L, Garrido I, Filleron T, Le Guellec S, Bellard E, Fournie JJ, et al. Human solid tumors contain high endothelial venules: association with t- and b-lymphocyte infiltration and favorable prognosis in breast cancer. Cancer Res (2011) 71(17):5678–87. doi: 10.1158/0008-5472.can-11-0431
51. Heij LR, Tan X, Kather JN, Niehues JM, Sivakumar S, Heussen N, et al. Nerve fibers in the tumor microenvironment are co-localized with lymphoid aggregates in pancreatic cancer. J Clin Med [Internet] (2021) 10:3.
52. Gago da Graca C, van Baarsen LGM, Mebius RE. Tertiary lymphoid structures: Diversity in their development, composition, and role. J Immunol (2021) 206(2):273–81. doi: 10.4049/jimmunol.2000873
53. Pikor Natalia B, Astarita Jillian L, Summers-Deluca L, Galicia G, Qu J, Ward Lesley A, et al. Integration of Th17- and lymphotoxin-derived signals initiates meningeal-resident stromal cell remodeling to propagate neuroinflammation. Immunity (2015) 43(6):1160–73. doi: 10.1016/j.immuni.2015.11.010
54. Nayar S, Campos J, Smith CG, Iannizzotto V, Gardner DH, Mourcin F, et al. Immunofibroblasts are pivotal drivers of tertiary lymphoid structure formation and local pathology. Proc Natl Acad Sci (2019) 116(27):13490–7. doi: 10.1073/pnas.1905301116
55. Koscso B, Kurapati S, Rodrigues RR, Nedjic J, Gowda K, Shin C, et al. Gut-resident CX3CR1hi macrophages induce tertiary lymphoid structures and IgA response in situ. Sci Immunol (2020) 5(46):10. doi: 10.1126/sciimmunol.aax0062
56. McDonald KG, McDonough JS, Dieckgraefe BK, Newberry RD. Dendritic cells produce CXCL13 and participate in the development of murine small intestine lymphoid tissues. Am J Pathology. (2010) 176(5):2367–77. doi: 10.2353/ajpath.2010.090723
57. Kinker GS, Vitiello GAF, Diniz AB, Cabral-Piccin MP, Pereira PHB, Carvalho MLetíciaR, et al. Mature tertiary lymphoid structures are key niches of tumour-specific immune responses in pancreatic ductal adenocarcinomas. Gut (2023) 72(10):1927. doi: 10.1136/gutjnl-2022-328697
58. Luther SA, Lopez T, Bai W, Hanahan D, Cyster JG. BLC expression in pancreatic islets causes b cell recruitment and lymphotoxin-dependent lymphoid neogenesis. Immunity (2000) 12(5):471–81. doi: 10.1016/s1074-7613(00)80199-5
59. Delvecchio FR, Fincham REA, Spear S, Clear A, Roy-Luzarraga M, Balkwill FR, et al. Pancreatic cancer chemotherapy is potentiated by induction of tertiary lymphoid structures in mice. Cell Mol Gastroenterol Hepatology. (2021) 12(5):1543–65. doi: 10.1016/j.jcmgh.2021.06.023
60. Fan L, Reilly CR, Luo Y, Dorf ME, Lo D. Cutting edge: ectopic expression of the chemokine TCA4/SLC is sufficient to trigger lymphoid neogenesis. J Immunol (2000) 164(8):3955–9. doi: 10.4049/jimmunol.164.8.3955
61. Finkin S, Yuan D, Stein I, Taniguchi K, Weber A, Unger K, et al. Ectopic lymphoid structures function as microniches for tumor progenitor cells in hepatocellular carcinoma. Nat Immunol (2015) 16(12):1235–44. doi: 10.1038/ni.3290
62. Murakami J, Shimizu Y, Kashii Y, Kato T, Minemura M, Okada K, et al. Functional b-cell response in intrahepatic lymphoid follicles in chronic hepatitis c. Hepatology (1999) 30(1):143–50. doi: 10.1002/hep.510300107
63. Siliņa K, Soltermann A, Attar FM, Casanova R, Uckeley ZM, Thut H, et al. Germinal centers determine the prognostic relevance of tertiary lymphoid structures and are impaired by corticosteroids in lung squamous cell carcinoma. Cancer Res (2018) 78(5):1308–20. doi: 10.1158/0008-5472.CAN-17-1987
64. Posch F, Silina K, Leibl S, Mündlein A, Moch H, Siebenhüner A, et al. Maturation of tertiary lymphoid structures and recurrence of stage II and III colorectal cancer. OncoImmunology (2018) 7(2):e1378844. doi: 10.1080/2162402X.2017.1378844
65. Hiraoka N, Ino Y, Yamazaki-Itoh R, Kanai Y, Kosuge T, Shimada K. Intratumoral tertiary lymphoid organ is a favourable prognosticator in patients with pancreatic cancer. Br J Cancer. (2015) 112(11):1782–90. doi: 10.1038/bjc.2015.145
66. Kuwabara S, Tsuchikawa T, Nakamura T, Hatanaka Y, Hatanaka KC, Sasaki K, et al. Prognostic relevance of tertiary lymphoid organs following neoadjuvant chemoradiotherapy in pancreatic ductal adenocarcinoma. Cancer Science. (2019) 110(6):1853–62. doi: 10.1111/cas.14023
67. JG A, Rajamanickam V, Bui C, Bernard B, Pucilowska J, Ballesteros-Merino C, et al. Germinal center reactions in tertiary lymphoid structures associate with neoantigen burden, humoral immunity and long-term survivorship in pancreatic cancer. Oncoimmunology (2021) 10(1):1900635. doi: 10.1080/2162402x.2021.1900635
68. Klein U, Dalla-Favera R. Germinal centres: role in b-cell physiology and malignancy. Nat Rev Immunol (2008) 8(1):22–33. doi: 10.1038/nri2217
69. Germain C, Gnjatic S, Tamzalit F, Knockaert S, Remark R, Goc J, et al. Presence of b cells in tertiary lymphoid structures is associated with a protective immunity in patients with lung cancer. Am J Respir Crit Care Med (2014) 189(7):832–44.
70. Astorri E, Bombardieri M, Gabba S, Peakman M, Pozzilli P, Pitzalis C. Evolution of ectopic lymphoid neogenesis and in situ autoantibody production in autoimmune nonobese diabetic mice: cellular and molecular characterization of tertiary lymphoid structures in pancreatic islets. J Immunol (2010) 185(6):3359–68. doi: 10.4049/jimmunol.1001836
71. Girard JP, Springer TA. High endothelial venules (HEVs): specialized endothelium for lymphocyte migration. Immunol Today (1995) 16(9):449–57. doi: 10.1016/0167-5699(95)80023-9
72. Browning JL, Allaire N, Ngam-ek A, Notidis E, Hunt J, Perrin S, et al. Lymphotoxin-β receptor signaling is required for the homeostatic control of HEV differentiation and function. Immunity (2005) 23(5):539–50. doi: 10.1016/j.immuni.2005.10.002
73. Jones E, Gallimore A, Ager A. Defining high endothelial venules and tertiary lymphoid structures in cancer. Methods Mol Biol (2018) 1845:99–118. doi: 10.1007/978-1-4939-8709-2_7
74. Ladányi A, Kiss J, Somlai B, Gilde K, Fejos Z, Mohos A, et al. Density of DC-LAMP(+) mature dendritic cells in combination with activated t lymphocytes infiltrating primary cutaneous melanoma is a strong independent prognostic factor. Cancer Immunol Immunother. (2007) 56(9):1459–69. doi: 10.1007/s00262-007-0286-3
75. Allen E, Jabouille A, Rivera LB, Lodewijckx I, Missiaen R, Steri V, et al. Combined antiangiogenic and anti-PD-L1 therapy stimulates tumor immunity through HEV formation. Sci Trans Med (2017) 9(385). doi: 10.1126/scitranslmed.aak9679
76. Wirsing AM, Ervik IK, Seppola M, Uhlin-Hansen L, Steigen SE, Hadler-Olsen E. Presence of high-endothelial venules correlates with a favorable immune microenvironment in oral squamous cell carcinoma. Modern Pathology. (2018) 31(6):910–22. doi: 10.1038/s41379-018-0019-5
77. Lee M, Heo SH, Song IH, Rajayi H, Park HS, Park IA, et al. Presence of tertiary lymphoid structures determines the level of tumor-infiltrating lymphocytes in primary breast cancer and metastasis. Mod Pathol (2019) 32(1):70–80. doi: 10.1038/s41379-018-0113-8
78. Masugi Y, Abe T, Ueno A, Fujii-Nishimura Y, Ojima H, Endo Y, et al. Characterization of spatial distribution of tumor-infiltrating CD8+ t cells refines their prognostic utility for pancreatic cancer survival. Modern Pathology. (2019) 32(10):1495–507. doi: 10.1038/s41379-019-0291-z
79. Liudahl SM, Betts CB, Sivagnanam S, Morales-Oyarvide V, da Silva A, Yuan C, et al. Leukocyte heterogeneity in pancreatic ductal adenocarcinoma: Phenotypic and spatial features associated with clinical outcome. Cancer Discovery. (2021) 11(8):2014–31. doi: 10.1158/2159-8290.CD-20-0841
80. Papalampros A, Vailas M, Ntostoglou K, Chiloeches ML, Sakellariou S, Chouliari NV, et al. Unique spatial immune profiling in pancreatic ductal adenocarcinoma with enrichment of exhausted and senescent t cells and diffused CD47-SIRPα expression. Cancers [Internet] (2020) 12:7.
81. Binkley CE, Zhang L, Greenson JK, Giordano TJ, Kuick R, Misek D, et al. The molecular basis of pancreatic fibrosis: common stromal gene expression in chronic pancreatitis and pancreatic adenocarcinoma. Pancreas (2004) 29(4):254–63.
82. Bachem MG, Schünemann M, Ramadani M, Siech M, Beger H, Buck A, et al. Pancreatic carcinoma cells induce fibrosis by stimulating proliferation and matrix synthesis of stellate cells. Gastroenterology (2005) 128(4):907–21.
83. Armstrong T, Packham G, Murphy LB, Bateman AC, Conti JA, Fine DR, et al. Type i collagen promotes the malignant phenotype of pancreatic ductal adenocarcinoma. Clin Cancer Res (2004) 10(21):7427–37.
84. Schröder AE, Greiner A, Seyfert C, Berek C. Differentiation of b cells in the nonlymphoid tissue of the synovial membrane of patients with rheumatoid arthritis. Proc Natl Acad Sci (1996) 93(1):221–5.
85. Dorner T, Hansen A, Jacobi A, Lipsky P. Immunglobulin repertoire analysis provides new insights into the immunopathogenesis of sjogren's syndrome. Autoimmun Rev (2002) 1(3):119–24.
86. Sims GP, Shiono H, Willcox N, Stott DI. Somatic hypermutation and selection of b cells in thymic germinal centers responding to acetylcholine receptor in myasthenia gravis. J Immunol (2001) 167(4):1935–44.
87. Kim H-J, Krenn V, Steinhauser G, Berek C. Plasma cell development in synovial germinal centers in patients with rheumatoid and reactive arthritis. J Immunol (1999) 162(5):3053–62.
88. Meylan M, Petitprez F, Becht E, Bougoüin A, Pupier G, Calvez A, et al. Tertiary lymphoid structures generate and propagate anti-tumor antibody-producing plasma cells in renal cell cancer. Immunity (2022) 55(3):527–41.e5. doi: 10.1016/j.immuni.2022.02.001
89. Montfort A, Pearce O, Maniati E, Vincent BG, Bixby L, Böhm S, et al. A strong b-cell response is part of the immune landscape in human high-grade serous ovarian metastases. Clin Cancer Res (2017) 23(1):250–62. doi: 10.1158/1078-0432.Ccr-16-0081
90. Spear S, Candido JB, McDermott JR, Ghirelli C, Maniati E, Beers SA, et al. Discrepancies in the tumor microenvironment of spontaneous and orthotopic murine models of pancreatic cancer uncover a new immunostimulatory phenotype for b cells. Front Immunol (2019) 10:542.
91. Castino GF, Cortese N, Capretti G, Serio S, Di Caro G, Mineri R, et al. Spatial distribution of b cells predicts prognosis in human pancreatic adenocarcinoma. OncoImmunology (2016) 5(4):e1085147. doi: 10.1080/2162402X.2015.1085147
92. Galon J, Costes A, Sanchez-Cabo F, Kirilovsky A, Mlecnik B, Lagorce-Pagès C, et al. Type, density, and location of immune cells within human colorectal tumors predict clinical outcome. Science (2006) 313(5795):1960–4. doi: 10.1126/science.1129139
93. Erdag G, Schaefer JT, Smolkin ME, Deacon DH, Shea SM, Dengel LT, et al. Immunotype and immunohistologic characteristics of tumor-infiltrating immune cells are associated with clinical outcome in metastatic melanoma. Cancer Res (2012) 72(5):1070–80. doi: 10.1158/0008-5472.Can-11-3218
94. Ali HR, Provenzano E, Dawson SJ, Blows FM, Liu B, Shah M, et al. Association between CD8+ t-cell infiltration and breast cancer survival in 12 439 patients. Ann Oncol (2014) 25(8):1536–43. doi: 10.1093/annonc/mdu191
95. Lee Y, Chin RK, Christiansen P, Sun Y, Tumanov AV, Wang J, et al. Recruitment and activation of naive t cells in the islets by lymphotoxin β receptor-dependent tertiary lymphoid structure. Immunity (2006) 25(3):499–509. doi: 10.1016/j.immuni.2006.06.016
96. Yu P, Lee Y, Liu W, Chin RK, Wang J, Wang Y, et al. Priming of naive t cells inside tumors leads to eradication of established tumors. Nat Immunol (2004) 5(2):141–9.
97. Di Caro G, Bergomas F, Grizzi F, Doni A, Bianchi P, Malesci A, et al. Occurrence of tertiary lymphoid tissue is associated with t-cell infiltration and predicts better prognosis in early-stage colorectal cancers. Clin Cancer Res (2014) 20(8):2147–58.
98. Schrama D, Voigt H, Eggert AO, Xiang R, Zhou H, Schumacher TN, et al. Immunological tumor destruction in a murine melanoma model by targeted LTalpha independent of secondary lymphoid tissue. Cancer Immunol Immunother. (2008) 57(1):85–95. doi: 10.1007/s00262-007-0352-x
99. Ahmed A, Köhler S, Klotz R, Giese N, Hackert T, Springfeld C, et al. Tertiary lymphoid structures and their association to immune phenotypes and circulatory IL2 levels in pancreatic ductal adenocarcinoma. OncoImmunology (2022) 11(1):2027148. doi: 10.1080/2162402X.2022.2027148
100. Stromnes IM, Hulbert A, Pierce RH, Greenberg PD, Hingorani SR. T-cell localization, activation, and clonal expansion in human pancreatic ductal adenocarcinoma. Cancer Immunol Res (2017) 5(11):978–91. doi: 10.1158/2326-6066.CIR-16-0322
101. Wartenberg M, Cibin S, Zlobec I, Vassella E, Eppenberger-Castori S, Terracciano L, et al. Integrated genomic and immunophenotypic classification of pancreatic cancer reveals three distinct subtypes with Prognostic/Predictive significance. Clin Cancer Res (2018) 24(18):4444–54. doi: 10.1158/1078-0432.Ccr-17-3401
102. Peske JD, Thompson ED, Gemta L, Baylis RA, Fu Y-X, Engelhard VH. Effector lymphocyte-induced lymph node-like vasculature enables naive t-cell entry into tumours and enhanced anti-tumour immunity. Nat Commun (2015) 6(1):7114. doi: 10.1038/ncomms8114
103. Lutz ER, Wu AA, Bigelow E, Sharma R, Mo G, Soares K, et al. Immunotherapy converts nonimmunogenic pancreatic tumors into immunogenic foci of immune regulation. Cancer Immunol Res (2014) 2(7):616–31. doi: 10.1158/2326-6066.CIR-14-0027
104. Poschke I, Faryna M, Bergmann F, Flossdorf M, Lauenstein C, Hermes J, et al. Identification of a tumor-reactive t-cell repertoire in the immune infiltrate of patients with resectable pancreatic ductal adenocarcinoma. OncoImmunology (2016) 5(12):e1240859. doi: 10.1080/2162402X.2016.1240859
105. Ma Q-Y, Huang D-Y, Zhang H-J, Chen J, Miller W, Chen X-F. Function of follicular helper t cell is impaired and correlates with survival time in non-small cell lung cancer. Int Immunopharmacology. (2016) 41:1–7. doi: 10.1016/j.intimp.2016.10.014
106. Duan Z, Gao J, Zhang L, Liang H, Huang X, Xu Q, et al. Phenotype and function of CXCR5+CD45RA–CD4+ t cells were altered in HBV-related hepatocellular carcinoma and elevated serum CXCL13 predicted better prognosis. Oncotarget (2015) 6(42).
107. Gu-Trantien C, Loi S, Garaud S, Equeter C, Libin M, de Wind A, et al. CD4+ follicular helper t cell infiltration predicts breast cancer survival. J Clin Invest (2013) 123(7):2873–92. doi: 10.1172/JCI67428
108. Lin X, Ye L, Wang X, Liao Z, Dong J, Yang Y, et al. Follicular helper t cells remodel the immune microenvironment of pancreatic cancer via secreting CXCL13 and IL-21. Cancers [Internet] (2021) 13:15.
109. Provenzano PP, Cuevas C, Chang AE, Goel VK, Von Hoff DD, Hingorani SR. Enzymatic targeting of the stroma ablates physical barriers to treatment of pancreatic ductal adenocarcinoma. Cancer Cell (2012) 21(3):418–29.
110. Jiang H, Hegde S, Knolhoff BL, Zhu Y, Herndon JM, Meyer MA, et al. Targeting focal adhesion kinase renders pancreatic cancers responsive to checkpoint immunotherapy. Nat Med (2016) 22(8):851–60. doi: 10.1038/nm.4123
111. Öhlund D, Handly-Santana A, Biffi G, Elyada E, Almeida AS, Ponz-Sarvise M, et al. Distinct populations of inflammatory fibroblasts and myofibroblasts in pancreatic cancer. J Exp Med (2017) 214(3):579–96. doi: 10.1084/jem.20162024
112. Neuzillet C, Tijeras-Raballand A, Ragulan C, Cros J, Patil Y, Martinet M, et al. Inter- and intra-tumoural heterogeneity in cancer-associated fibroblasts of human pancreatic ductal adenocarcinoma. J Pathology. (2019) 248(1):51–65. doi: 10.1002/path.5224
113. Özdemir BC, Pentcheva-Hoang T, Carstens JL, Zheng X, Wu C-C, Simpson TR, et al. Depletion of carcinoma-associated fibroblasts and fibrosis induces immunosuppression and accelerates pancreas cancer with reduced survival. Cancer Cell (2014) 25(6):719–34.
114. Rhim AD, Oberstein PE, Thomas DH, Mirek ET, Palermo CF, Sastra SA, et al. Stromal elements act to restrain, rather than support, pancreatic ductal adenocarcinoma. Cancer Cell (2014) 25(6):735–47.
115. Rodriguez AB, Peske JD, Woods AN, Leick KM, Mauldin IS, Meneveau MO, et al. Immune mechanisms orchestrate tertiary lymphoid structures in tumors via cancer-associated fibroblasts. Cell Rep (2021) 36(3).
116. Breiteneder-Geleff S, Soleiman A, Kowalski H, Horvat R, Amann G, Kriehuber E, et al. Angiosarcomas express mixed endothelial phenotypes of blood and lymphatic capillaries: podoplanin as a specific marker for lymphatic endothelium. Am J pathology. (1999) 154(2):385–94.
117. Pula B, Witkiewicz W, Dziegiel P, Podhorska-Okolow M. Significance of podoplanin expression in cancer-associated fibroblasts: A comprehensive review. Int J Oncol (2013) 42(6):1849–57. doi: 10.3892/ijo.2013.1887
118. Neuzillet C, Nicolle R, Raffenne J, Tijeras-Raballand A, Brunel A, Astorgues-Xerri L, et al. Periostin- and podoplanin-positive cancer-associated fibroblast subtypes cooperate to shape the inflamed tumor microenvironment in aggressive pancreatic adenocarcinoma. J Pathology. (2022) 258(4):408–25. doi: 10.1002/path.6011
119. Hirayama K, Kono H, Nakata Y, Akazawa Y, Wakana H, Fukushima H, et al. Expression of podoplanin in stromal fibroblasts plays a pivotal role in the prognosis of patients with pancreatic cancer. Surg Today (2018) 48:110–8.
120. Boyd LN, Andini KD, Peters GJ, Kazemier G, Giovannetti E. Heterogeneity and plasticity of cancer-associated fibroblasts in the pancreatic tumor microenvironment. In: Seminars in cancer biology;. Elsevier (2022). editors.
121. Horeweg N, Workel HH, Loiero D, Church DN, Vermij L, Léon-Castillo A, et al. Tertiary lymphoid structures critical for prognosis in endometrial cancer patients. Nat Commun (2022) 13(1):1373. doi: 10.1038/s41467-022-29040-x
122. Vanhersecke L, Brunet M, Guegan JP, Rey C, Bougouin A, Cousin S, et al. Mature tertiary lymphoid structures predict immune checkpoint inhibitor efficacy in solid tumors independently of PD-L1 expression. Nat Cancer. (2021) 2(8):794–802. doi: 10.1038/s43018-021-00232-6
123. Xuan Z, Xuan L, He C, Yusheng C, Ruijie W, Mingjian M, et al. Characterization of intratumoral tertiary lymphoid structures in pancreatic ductal adenocarcinoma: cellular properties and prognostic significance. J ImmunoTherapy Cancer. (2023) 11(6):e006698. doi: 10.1136/jitc-2023-006698
124. Tanaka T, Masuda A, Inoue J, Hamada T, Ikegawa T, Toyama H, et al. Integrated analysis of tertiary lymphoid structures in relation to tumor-infiltrating lymphocytes and patient survival in pancreatic ductal adenocarcinoma. J Gastroenterology. (2023) 58(3):277–91. doi: 10.1007/s00535-022-01939-8
125. Gunderson AJ, Kaneda MM, Tsujikawa T, Nguyen AV, Affara NI, Ruffell B, et al. Bruton tyrosine kinase–dependent immune cell cross-talk drives pancreas cancer. Cancer Discovery. (2016) 6(3):270–85. doi: 10.1158/2159-8290.CD-15-0827
126. Pylayeva-Gupta Y, Das S, Handler JS, Hajdu CH, Coffre M, Koralov SB, et al. IL35-producing b cells promote the development of pancreatic neoplasia. Cancer Discovery. (2016) 6(3):247–55. doi: 10.1158/2159-8290.CD-15-0843
127. Lee KE, Spata M, Bayne LJ, Buza EL, Durham AC, Allman D, et al. Hif1a deletion reveals pro-neoplastic function of b cells in pancreatic neoplasia. Cancer Discovery. (2016) 6(3):256–69. doi: 10.1158/2159-8290.CD-15-0822
128. Fridman WH, Pages F, Sautes-Fridman C, Galon J. The immune contexture in human tumours: impact on clinical outcome. Nat Rev Cancer. (2012) 12(4):298–306. doi: 10.1038/nrc3245
129. Mota Reyes C, Teller S, Muckenhuber A, Konukiewitz B, Safak O, Weichert W, et al. Neoadjuvant therapy remodels the pancreatic cancer microenvironment via depletion of protumorigenic immune cells. Clin Cancer Res (2020) 26(1):220–31.
130. Dias Costa A, Väyrynen SA, Chawla A, Zhang J, Väyrynen JP, Lau MC, et al. Neoadjuvant chemotherapy is associated with altered immune cell infiltration and an anti-tumorigenic microenvironment in resected pancreatic cancer. Clin Cancer Res (2022) 28(23):5167–79.
131. Farren MR, Sayegh L, Ware MB, Chen H-R, Gong J, Liang Y, et al. Immunologic alterations in the pancreatic cancer microenvironment of patients treated with neoadjuvant chemotherapy and radiotherapy. JCI Insight (2020) 5(1).
132. Peng H, James CA, Cullinan DR, Hogg GD, Mudd JL, Zuo C, et al. Neoadjuvant FOLFIRINOX therapy is associated with increased effector t cells and reduced suppressor cells in patients with pancreatic cancer. Clin Cancer Res (2021) 27(24):6761–71.
133. Morcrette G, Hirsch TZ, Badour E, Pilet J, Caruso S, Calderaro J, et al. APC germline hepatoblastomas demonstrate cisplatin-induced intratumor tertiary lymphoid structures. OncoImmunology (2019) 8(6):e1583547. doi: 10.1080/2162402X.2019.1583547
134. Rucki AA, Zheng L. Pancreatic cancer stroma: understanding biology leads to new therapeutic strategies. World J Gastroenterol (2014) 20(9):2237–46. doi: 10.3748/wjg.v20.i9.2237
135. Song IH, Heo S-H, Bang WS, Park HS, Park IA, Kim Y-A, et al. Predictive value of tertiary lymphoid structures assessed by high endothelial venule counts in the neoadjuvant setting of triple-negative breast cancer. Cancer Res treatment: Off J Korean Cancer Assoc (2017) 49(2):399–407.
136. Sun X, Liu W, Sun L, Mo H, Feng Y, Wu X, et al. Maturation and abundance of tertiary lymphoid structures are associated with the efficacy of neoadjuvant chemoimmunotherapy in resectable non-small cell lung cancer. J Immunother Cancer. (2022) 10(11). doi: 10.1136/jitc-2022-005531
137. Dosset M, Vargas TR, Lagrange A, Boidot R, Vegran F, Roussey A, et al. PD-1/PD-L1 pathway: an adaptive immune resistance mechanism to immunogenic chemotherapy in colorectal cancer. Oncoimmunology (2018) 7(6):e1433981. doi: 10.1080/2162402X.2018.1433981
138. Tesniere A, Schlemmer F, Boige V, Kepp O, Martins I, Ghiringhelli F, et al. Immunogenic death of colon cancer cells treated with oxaliplatin. Oncogene (2010) 29(4):482–91. doi: 10.1038/onc.2009.356
139. Pfirschke C, Engblom C, Rickelt S, Cortez-Retamozo V, Garris C, Pucci F, et al. Immunogenic chemotherapy sensitizes tumors to checkpoint blockade therapy. Immunity (2016) 44(2):343–54. doi: 10.1016/j.immuni.2015.11.024
140. Song W, Shen L, Wang Y, Liu Q, Goodwin TJ, Li J, et al. Synergistic and low adverse effect cancer immunotherapy by immunogenic chemotherapy and locally expressed PD-L1 trap. Nat Commun (2018) 9(1):2237. doi: 10.1038/s41467-018-04605-x
141. Cook AM, McDonnell AM, Lake RA, Nowak AK. Dexamethasone co-medication in cancer patients undergoing chemotherapy causes substantial immunomodulatory effects with implications for chemo-immunotherapy strategies. Oncoimmunology (2016) 5(3):e1066062. doi: 10.1080/2162402x.2015.1066062
142. Helmink BA, Reddy SM, Gao J, Zhang S, Basar R, Thakur R, et al. B cells and tertiary lymphoid structures promote immunotherapy response. Nature (2020) 577(7791):549–55. doi: 10.1038/s41586-019-1922-8
143. Cabrita R, Lauss M, Sanna A, Donia M, Skaarup Larsen M, Mitra S, et al. Tertiary lymphoid structures improve immunotherapy and survival in melanoma. Nature (2020) 577(7791):561–5.
144. Petitprez F, de Reyniès A, Keung EZ, Chen TW-W, Sun C-M, Calderaro J, et al. B cells are associated with survival and immunotherapy response in sarcoma. Nature (2020) 577(7791):556–60.
145. Wennhold K, Thelen M, Lehmann J, Schran S, Preugszat E, Garcia-Marquez M, et al. CD86+ antigen-presenting b cells are increased in cancer, localize in tertiary lymphoid structures, and induce specific t-cell responses. Cancer Immunol Res (2021) 9(9):1098–108. doi: 10.1158/2326-6066.CIR-20-0949
146. Coppola D, Nebozhyn M, Khalil F, Dai H, Yeatman T, Loboda A, et al. Unique ectopic lymph node-like structures present in human primary colorectal carcinoma are identified by immune gene array profiling. Am J Pathol (2011) 179(1):37–45. doi: 10.1016/j.ajpath.2011.03.007
147. Li R, Berglund A, Zemp L, Dhillon J, Putney R, Kim Y, et al. The 12-CK score: Global measurement of tertiary lymphoid structures. Front Immunol (2021) 12:org/10.3389/fimmu.2021.694079. doi: 10.3389/fimmu.2021.694079
148. Joshi N, Akama-Garren E, Lu Y, Lee D-Y, Chang G, Li A, et al. Regulatory t cells in tumor-associated tertiary lymphoid structures suppress anti-tumor t cell responses. Immunity (2015) 43(3):579–90.
149. Jing F, Choi EY. Potential of cells and Cytokines/Chemokines to regulate tertiary lymphoid structures in human diseases. Immune Netw (2016) 16(5):271–80. doi: 10.4110/in.2016.16.5.271
150. Tang H, Zhu M, Qiao J, Fu YX. Lymphotoxin signalling in tertiary lymphoid structures and immunotherapy. Cell Mol Immunol (2017) 14(10):809–18. doi: 10.1038/cmi.2017.13
151. Krautler Nike J, Kana V, Kranich J, Tian Y, Perera D, Lemm D, et al. Follicular dendritic cells emerge from ubiquitous perivascular precursors. Cell (2012) 150(1):194–206. doi: 10.1016/j.cell.2012.05.032
152. Ware CF. Targeting lymphocyte activation through the lymphotoxin and LIGHT pathways. Immunol Rev (2008) 223:186–201. doi: 10.1111/j.1600-065X.2008.00629.x
153. Filderman JN, Appleman M, Chelvanambi M, Taylor JL, Storkus WJ. STINGing the tumor microenvironment to promote therapeutic tertiary lymphoid structure development. Front Immunol (2021) 12:690105. doi: 10.3389/fimmu.2021.690105
154. Luther SA, Bidgol A, Hargreaves DC, Schmidt A, Xu Y, Paniyadi J, et al. Differing activities of homeostatic chemokines CCL19, CCL21, and CXCL12 in lymphocyte and dendritic cell recruitment and lymphoid Neogenesis1. J Immunol (2002) 169(1):424–33. doi: 10.4049/jimmunol.169.1.424
155. Chen SC, Vassileva G, Kinsley D, Holzmann S, Manfra D, Wiekowski MT, et al. Ectopic expression of the murine chemokines CCL21a and CCL21b induces the formation of lymph node-like structures in pancreas, but not skin, of transgenic mice. J Immunol (2002) 168(3):1001–8. doi: 10.4049/jimmunol.168.3.1001
156. Liu W, Tai CH, Liu X, Pastan I. Anti-mesothelin immunotoxin induces mesothelioma eradication, anti-tumor immunity, and the development of tertiary lymphoid structures. Proc Natl Acad Sci United States America. (2022) 119(48):e2214928119. doi: 10.1073/pnas.2214928119
157. Argani P, Iacobuzio-Donahue C, Ryu B, Rosty C, Goggins M, Wilentz RE, et al. Mesothelin is overexpressed in the vast majority of ductal adenocarcinomas of the pancreas: identification of a new pancreatic cancer marker by serial analysis of gene expression (SAGE). Clin Cancer Res (2001) 7(12):3862–8.
158. Zhu Y, An X, Zhang X, Qiao Y, Zheng T, Li X. STING: a master regulator in the cancer-immunity cycle. Mol Cancer. (2019) 18(1):152. doi: 10.1186/s12943-019-1087-y
159. Chelvanambi M, Fecek RJ, Taylor JL, Storkus WJ. STING agonist-based treatment promotes vascular normalization and tertiary lymphoid structure formation in the therapeutic melanoma microenvironment. J Immunother Cancer. (2021) 9(2). doi: 10.1136/jitc-2020-001906
160. Kaufman HL, Kohlhapp FJ, Zloza A. Oncolytic viruses: a new class of immunotherapy drugs. Nat Rev Drug discovery. (2015) 14(9):642–62.
161. He T, Hao Z, Lin M, Xin Z, Chen Y, Ouyang W, et al. Oncolytic adenovirus promotes vascular normalization and nonclassical tertiary lymphoid structure formation through STING-mediated DC activation. OncoImmunology (2022) 11(1):2093054. doi: 10.1080/2162402X.2022.2093054
162. Santos J, Heiniö C, Quixabeira D, Zafar S, Clubb J, Pakola S, et al. Systemic delivery of oncolytic adenovirus to tumors using tumor-infiltrating lymphocytes as carriers. Cells (2021) 10(5). doi: 10.3390/cells10050978
163. Von Ahrens D, Bhagat TD, Nagrath D, Maitra A, Verma A. The role of stromal cancer-associated fibroblasts in pancreatic cancer. J Hematol Oncol (2017) 10(1):1–8.
164. Ma J, Ramachandran M, Jin C, Quijano-Rubio C, Martikainen M, Yu D, et al. Characterization of virus-mediated immunogenic cancer cell death and the consequences for oncolytic virus-based immunotherapy of cancer. Cell Death Disease. (2020) 11(1):48. doi: 10.1038/s41419-020-2236-3
165. Manieri NA, Chiang EY, Grogan JL. TIGIT: A key inhibitor of the cancer immunity cycle. Trends Immunol (2017) 38(1):20–8. doi: 10.1016/j.it.2016.10.002
166. Pearce H, Croft W, Nicol SM, Margielewska-Davies S, Powell R, Cornall R, et al. Tissue-resident memory t cells in pancreatic ductal adenocarcinoma coexpress PD-1 and TIGIT and functional inhibition is reversible by dual antibody blockade. Cancer Immunol Res (2023) 11(4):435–49. doi: 10.1158/2326-6066.Cir-22-0121
167. Liu X, Li M, Wang X, Dang Z, Jiang Y, Wang X, et al. PD-1(+) TIGIT(+) CD8(+) t cells are associated with pathogenesis and progression of patients with hepatitis b virus-related hepatocellular carcinoma. Cancer Immunol Immunother. (2019) 68(12):2041–54. doi: 10.1007/s00262-019-02426-5
168. Ford K, Hanley CJ, Mellone M, Szyndralewiez C, Heitz F, Wiesel P, et al. NOX4 inhibition potentiates immunotherapy by overcoming cancer-associated fibroblast-mediated CD8 t-cell exclusion from tumors. Cancer Res (2020) 80(9):1846–60.
Keywords: tertiary lymphoid structures, pancreatic adenocarcinoma, pancreatic cancer, translational ability, immune microenvironment
Citation: Gao Z, Azar J, Zhu H, Williams-Perez S, Kang SW, Marginean C, Rubinstein MP, Makawita S, Lee H-S and Camp ER (2024) Translational and oncologic significance of tertiary lymphoid structures in pancreatic adenocarcinoma. Front. Immunol. 15:1324093. doi: 10.3389/fimmu.2024.1324093
Received: 18 October 2023; Accepted: 11 January 2024;
Published: 01 February 2024.
Edited by:
Hui Zhao, University of Texas MD Anderson Cancer Center, United StatesReviewed by:
Feng Wei, Tianjin Medical University Cancer Institute and Hospital, ChinaAnthony B. Rodriguez, University of Virginia, United States
Copyright © 2024 Gao, Azar, Zhu, Williams-Perez, Kang, Marginean, Rubinstein, Makawita, Lee and Camp. This is an open-access article distributed under the terms of the Creative Commons Attribution License (CC BY). The use, distribution or reproduction in other forums is permitted, provided the original author(s) and the copyright owner(s) are credited and that the original publication in this journal is cited, in accordance with accepted academic practice. No use, distribution or reproduction is permitted which does not comply with these terms.
*Correspondence: E. Ramsay Camp, UmFtc2F5LkNhbXBAYmNtLmVkdQ==