- 1Department of Respiratory and Critical Care Medicine, The Affiliated Hospital of Southwest Medical University, Luzhou, Sichuan, China
- 2Inflammation & Allergic Diseases Research Unit, The Affiliated Hospital of Southwest Medical University, Luzhou, Sichuan, China
- 3Department of Respiratory and Critical Care Medicine, Liuzhou People’s Hospital, Guangxi Medical University, Liuzhou, Guangxi, China
- 4Key Laboratory of Diagnosis, Treatment and Research of Asthma and Chronic Obstructive Pulmonary Disease, Liuzhou, Guangxi, China
- 5Department of Dermatology, The Affiliated Hospital, Southwest Medical University, Luzhou, Sichuan, China
The prevalence rate of acute respiratory distress syndrome (ARDS) is estimated at approximately 10% in critically ill patients worldwide, with the mortality rate ranging from 17% to 39%. Currently, ARDS mortality is usually higher in patients with COVID-19, giving another challenge for ARDS treatment. However, the treatment efficacy for ARDS is far from satisfactory. The relationship between the gut microbiota and ARDS has been substantiated by relevant scientific studies. ARDS not only changes the distribution of gut microbiota, but also influences intestinal mucosal barrier through the alteration of gut microbiota. The modulation of gut microbiota can impact the onset and progression of ARDS by triggering dysfunctions in inflammatory response and immune cells, oxidative stress, cell apoptosis, autophagy, pyroptosis, and ferroptosis mechanisms. Meanwhile, ARDS may also influence the distribution of metabolic products of gut microbiota. In this review, we focus on the impact of ARDS on gut microbiota and how the alteration of gut microbiota further influences the immune function, cellular functions and related signaling pathways during ARDS. The roles of gut microbiota-derived metabolites in the development and occurrence of ARDS are also discussed.
1 Introduction
Acute respiratory distress syndrome (ARDS) is characterized by a severe inflammatory response, triggered by either primary or secondary acute injury to the lungs. This injury leads to damage to the alveolar wall and subsequent pulmonary congestion, culminating in gas exchange dysfunction (1). The clinical manifestations of ARDS typically include hypoxemia, respiratory distress, and pulmonary rales (2). Commonly associated conditions with ARDS encompass pneumonia, serious trauma, aspiration of gastric contents, or sepsis. Globally, the prevalence rate of ARDS is estimated to be around 10% among critically ill patients (3).
In intensive care unit (ICU) patients, the prevalence of ARDS exceeds 3%, and the incidence rates demonstrate a substantial variation, with differences exceeding 400% between different regions (4, 5). In USA, the incidence rate of ARDS elevated from 180.7/100,000 (in 2006) to 193.4/100,000 (in 2014) (6). A European study involving 3,504 hospitalized patients reported an incidence of ARDS at 32 cases per 100,000 individuals annually (7). In China, another investigation identified 672 out of 18,793 ICU patients as meeting the Berlin ARDS criteria (8). Additionally, the prevalence of ARDS is estimated at approximately 3% among pediatric intensive care unit patients (5). The mortality of ARDS is from 17% and 39% in different studies (9, 10). Presently, high mortality rate of ARDS is usually observed in COVID-19 patients, posing an additional challenge to the treatment of ARDS (11).
The management of ARDS encompasses early prevention, supportive care, pharmacological interventions, and mechanical ventilation (12). Given the substantial morbidity and mortality rates attributed to ARDS, prioritizing preventive strategies and optimized treatment measures are crucial. Observational studies have indicated that antiplatelet therapies, including aspirin, could be advantageous in averting ARDS among high-risk populations (13). In a randomized, double-blind, placebo-controlled trial, inhaled administration of salbutamol for 7 days did not elicit statistically significant differences in measured biomarkers of lung injury or inflammation compared to placebo, suggesting no detectable attenuation of the pathophysiological mechanisms underlying ARDS (14). Another investigation revealed that tracheotomy might extend survival in individuals diagnosed with ARDS (15).
The current therapeutic modalities for ARDS have proven suboptimal in addressing the clinical challenges associated with this condition. Given this unmet need, it is crucial to delve into the underlying pathogenesis and pathophysiological mechanisms of ARDS to identify innovative therapeutic targets. Emerging evidence underscores the integral role of gut microbiota in maintaining physiological homeostasis, modulating immune responses, and facilitating nutrient synthesis. Notably, a growing body of literature suggests that gut microbiota exerts a modulatory effect on the onset and progression of respiratory diseases through its influence on immune responses, oxidative stress, and inflammatory markers. Therefore, a comprehensive understanding of the mechanistic involvement of gut microbiota in ARDS and related respiratory conditions could pave the way for the development of targeted therapeutic interventions.
2 Lung microbiota, gut microbiota and the gut-lung axis
The lung microbiota plays a crucial regulatory role in lung and systemic immune modulation. The lung microbiota influences the development and function of immune cells such as macrophages, dendritic cells, etc., thereby impacting pulmonary and overall inflammatory responses (16). Lira et al. demonstrated that the lung microbiota plays a pivotal role in regulating immune responses, specifically influencing the delicate balance between pro-inflammatory and anti-inflammatory signals within the respiratory system (17). Khatiwada et al. confirmed that in the context of COVID-19, the lung microbiota can impact the risk and outcomes of diseases by activating both innate and adaptive immune responses (18). Furthermore, research has elucidated that the lung microbiota interacts with pattern recognition receptors on immune cells through the production of microbial molecules, influencing the host’s immune response (19). Studies have found an increase in the relative abundance of Enterobacteriaceae and Pasteurellaceae in the lung microbiome of ARDS samples, highlighting a potential shift in microbial composition (20). Dysbiosis refers to the disruption of the balance between beneficial and harmful microbes, with a relative increase or decrease in certain microbial populations. When the composition of the lung microbiota is disrupted, it may lead to immune imbalances, resulting in excessive inflammation and subsequent tissue damage (21).
In addition to the lung microbiota, the gut microbiota and its metabolites also play a regulatory role in lung immune function and inflammatory responses. Gut microbiota refers to the diverse group of microorganisms that reside in the human digestive tract, including bacteria, fungi, and viruses. These microorganisms play an important role in maintaining physiological balance, enhancing immune function, and synthesizing some essential nutrients in the human body (22, 23). In the present contexts, there are significant researches scrutiny directed towards elucidating the intricate interplays between the gut microbiota and diverse physiological systems, encompassing: gut -brain axis (24), gut -liver axis (25), gut -heart axis (26), and gut -skin axis (27) are currently hot topics in research. In this context, research on the gut-lung axis is also growing, aiming to elucidate the impact of gut microbiota and their metabolites on respiratory system diseases.
The gut-lung axis (28) refers to the anatomical independence of the gut and lungs, but the existence of complex interactions between them in terms of physiology and pathology. These interactions involve not only the host-microbe relationship but also the interplay between different microbial communities. The gut-lung axis functions through local regulation and distal effects, affecting the progression of respiratory diseases by modulating immune and inflammatory responses.
The balance of lung and gut microbiota, as well as the metabolic products and the barrier function of the gut microbiota, are essential components of overall health. When diseases occur, it can result in dysbiosis of the lung and gut microbiota, decreased production of beneficial gut microbiota metabolites, leading to impaired gut barrier function and increased intestinal permeability. This allows bacterial cell wall components such as lipopolysaccharide (LPS) and pathogen-associated molecular patterns, or even whole bacteria, to enter the bloodstream or lymphatic circulation, triggering systemic inflammation (29), and accelerating the progression of respiratory diseases (30). Pu et al. uncovered that specific bacteria within the gut microbiota stimulate the migration of group 2 innate lymphoid cells from the intestinal region to the lungs, contributing to host defense by regulating the cytokine IL-33 (31). In Ahlawat and Sharma’s study, they explored the role of the gut-lung axis in immunological coordination during SARS-CoV-2 infection, emphasizing the widespread distribution of the ACE2 receptor in both the gut and lungs. This research highlights how such interconnectivity affects the enteric microbiota and extrapulmonary symptoms in patients with COVID-19 (32). Oliveira and colleagues suggested that gut dysbiosis contributes to increased inflammation in both the gut and lungs or diminishes the capacity for anti-inflammatory processes, which plays a role in the immune response to SARS-CoV-2, potentially being either beneficial or detrimental (33). Yang et al. constructed a model of pulmonary infection and gut dysbiosis in 6-week-old mice, establishing a “gut-lung” communication axis through spleen and blood analyses. They demonstrated that gut dysbiosis disrupts the balance between Th1 and Th2 cells, exacerbating lung injury during infection. Furthermore, dysbiosis can have enduring impacts on the function of alveolar macrophages, which are vital in combating pulmonary infections (34). Similarly, Zhou et al. confirmed that dysbiosis of the gut microbiota exacerbates tracheal injury through the TLR4 signaling pathway, resulting in increased expression of inflammatory factors (34). It has been reported that SCFAs can effectively attenuate LPS-induced ARDS in rats by enhancing intestinal barrier function, modulating signaling pathways such as MAPK and NF-κB, leading to a decrease in the release of inflammatory factors and an increase in the production of anti-inflammatory factors, thus having a modulating effect on immunity and inflammation (35).
Under the coordinated action of the gut-lung axis, the balance of lung microbiota and gut microbiota, the metabolites of gut microbiota, and the intestinal barrier function are indispensable components for the health of the body and the maintenance of the lungs to perform their normal physiological functions. In the context of respiratory diseases, dysbiosis of the lung microbiota can trigger systemic inflammatory responses, thereby adversely affecting the composition of the gut microbiota. Conversely, dysbiosis of the gut microbiota can further disrupt immune system balance, consequently affecting the function and composition of the lung microbiota (36–39). Therefore, a comprehensive understanding and exploration of the regulatory mechanisms of the gut-lung axis in ARDS is expected to provide new therapeutic strategies and drug targets for the prevention and treatment of ARDS. This not only facilitates the precise and efficient treatment of ARDS but also alleviates the economic burden on both patients and society, ultimately enhancing the overall quality of life for affected individuals.
3 Gut microbiota in ARDS
In patients with ARDS, gut microbiota dysbiosis manifests as a reduction in beneficial symbiotic bacteria and an increase in opportunistic pathogens. Specifically, there is a decrease in beneficial bacterial populations such as Faecalibacterium prausnitzii, Bacteroides, and Bifidobacterium, which play a crucial role in maintaining the intestinal mucosal barrier and modulating local and systemic immune responses (40, 41). Concurrently, there is an increase in the abundance of pathogenic or conditionally pathogenic microbes, such as certain Enterobacteriaceae members, Clostridium difficile, and some Streptococcus species, which may lead to intestinal inflammation, impaired intestinal barrier function, and thereby affect systemic inflammatory status and immune responses (42, 43) (Table 1). Moreover, the altered gut microbiota landscape in ARDS patients plays a pivotal role in influencing cellular processes and recovery from injury, impacting oxidative stress responses, inflammatory cascades, and various types of cell death, such as apoptosis, pyroptosis, autophagy, and ferroptosis(Figure 1). Understanding these alterations and their reciprocal influence on cellular functions during ARDS progression is crucial for identifying novel therapeutic targets and strategies.
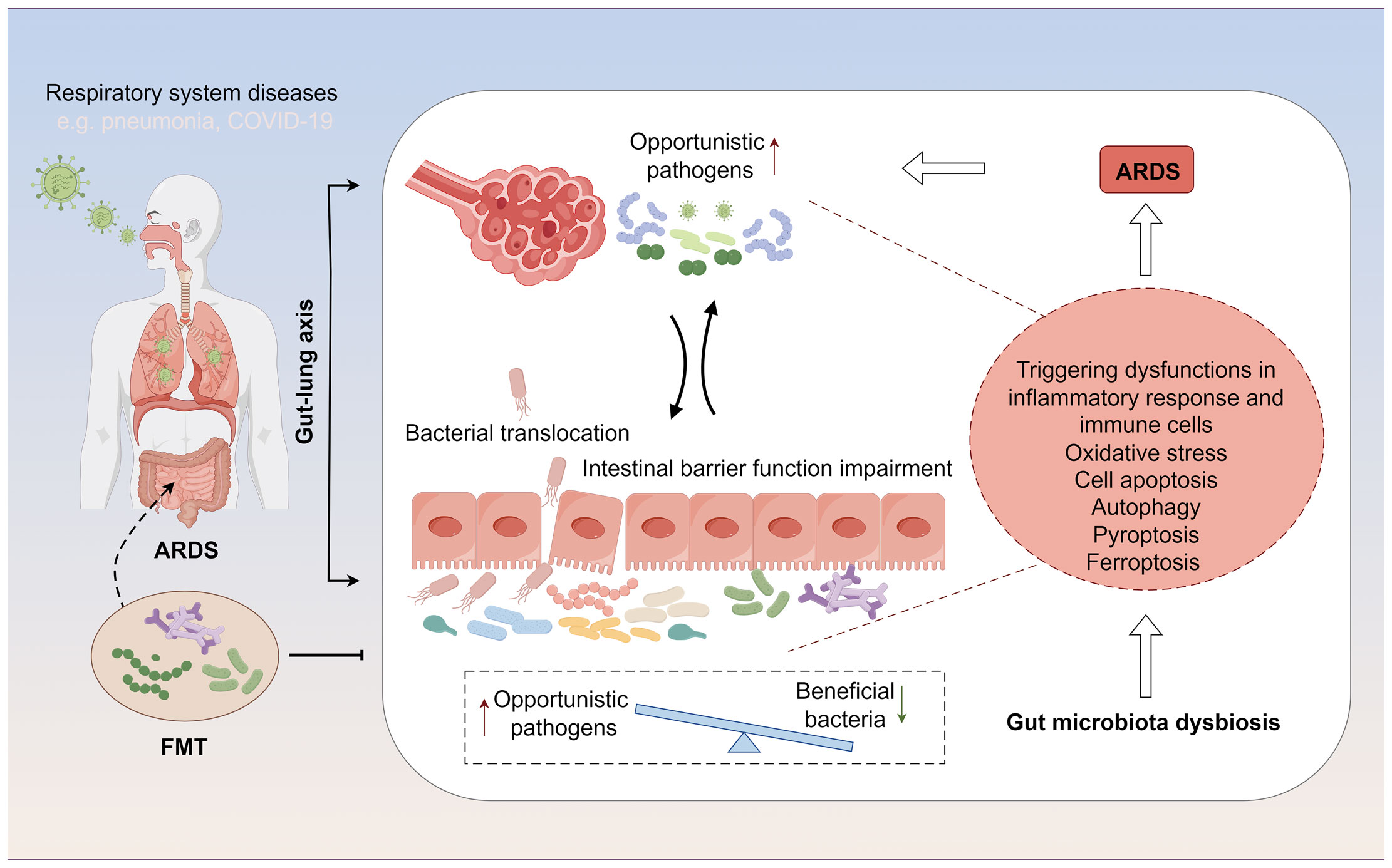
Figure 1 The gut-lung axis represents a bidirectional regulatory pathway between the gut microbiome and ARDS. On one hand, ARDS alters the distribution of the gut microbiome and affects the intestinal mucosal barrier through changes in the microbial composition. On the other hand, modulation of the gut microbiome can influence the development and progression of ARDS by further impacting immune functions, cellular functions, and related signaling pathways during ARDS.
3.1 The alteration of gut microbiota in ARDS
ARDS may exert influence on various gut microbiota, and these differences may be correlated with the distinct primary diseases that lead to ARDS. The study indicated that in ARDS caused by SARS-CoV-2 infection, Clostridia such as Clostridium hathewayi and Clostridium ramosum are upregulated in the intestinal tract of COVID-19 patients (49). A study by Hu et al. revealed specific alterations in the function of gut microbiota in acute pancreatitis patients who developed ARDS. In these AP-ARDS patients, there was a higher abundance of Proteobacteria, Enterobacteriaceae, Shigella, and Klebsiella Pneumoniae, but a lower abundance of Bifidobacterium. These findings suggest that changes in gut microbiota might serve as predictive indicators for the onset of ARDS (47). Panzer et al. demonstrated that the structure of the respiratory microbiota following severe blunt trauma was associated with the development and onset of ARDS (48). Zuo et al. demonstrated that the fecal microbiota in COVID-19 patients with ARDS underwent sustained changes during hospitalization and was related with disease severity compared to controls (48). Chen et al. provided evidence suggesting that a pre-existing diverse and rich gut microbiota may be advantageous in preventing severe COVID-19 and ARDS (50). Additionally, the supplementation with symbiotic microbial metabolites could potentially enhance the therapeutic approach to severe COVID-19 (50). The research findings of Kim et al. (45) and Tang et al. (46) further support the previously mentioned studies. They demonstrate that specific organisms, including Faecalibacterium prausnitzii, Clostridium butyricum, Clostridium leptum, and Eubacterium rectale, show altered abundance in COVID-19 patients with ARDS. These organisms may potentially serve as valuable diagnostic biomarkers.
3.2 The influence of gut microbiota on intestinal mucosal barrier in ARDS
The intestinal mucosal barrier is essential for maintaining gut health, balancing microbiota through antimicrobial peptides, and the combined action of the mucous layer and cell junctions (51). On the contrary, the intestinal mucosal barrier can also be regulated or attacked by changes in gut microbiota, increasing mucosal permeability and leading to the activation of the immune system and inflammatory response (52). A compromised barrier leads to intestinal inflammation and heightened exposure to external pathogens, while gut microbiota also affect bodily functions via mechanisms like inflammation and oxidative stress (53).
In various diseases, gut microbiota affects the intestinal barrier through cellular functions, inflammation, and oxidative stress. Cocaine use in mice upregulates NF-κB and IL-1β, altering gut microbiota and impairing intestinal permeability via the MAPK/ERK1/2 pathway (54). Scutellaria baicalensis (SC) helps diabetic rats by preserving the intestinal barrier, reducing inflammation via TLR-4/TRIF and TNFR-1/NF-κB pathways, and altering gut microbiota, affecting several bacterial genera (55). The study conducted by Yu et al. revealed that the sulfated derivative of Cyclocarya paliurus polysaccharide (SCP3) significantly modified the composition of the gut microbiota, restoring the intestinal barrier by enhancing oxidative stress resistance and upregulating tight junction proteins (56). Ge et al. showed that oxidative products in mice lead to weight gain, disruption of the intestinal barrier, and induction of inflammatory and oxidative stress, consequently altering the gut microbiota and impacting various bacteria, such as Akkermansia and Lactobacillus (44).. Furthermore, research has demonstrated that supplementation of conjugated linoleic acid can alleviate colitis by regulating cytokines and oxidative stress, maintaining the mucosal barrier, and rebalancing gut microbiota, including changes in Bacteroides, Bifidobacterium, and Odoribacter (57).
In ARDS, gut microbiota alterations affect the intestinal barrier and disease progression. Disrupted gut microbiota circadian rhythms in sepsis/ARDS patients are noted, but probiotics aid intestinal defense and immune response (58). COVID-19 impacts gut physiology, potentially causing dysbiosis and barrier damage, leading to immune and psychological issues (59). Chen et al. observed that lung inflammation in ARDS is exacerbated due to gut barrier dysfunction. They found that vancomycin could ameliorate this condition by altering the utilization of the gut microbial metabolite butyric acid (60). Tang et al. in an acute lung injury (ALI) animal model, suggested gut microbiota dysbiosis modulates the TLR4/NF-κB pathway in lungs, causing inflammation and stress, leading to lung injury (61). Zhou et al. found that in ALI, increased cytokines and lung microbiota changes cause gut dysbiosis and intestinal damage, exacerbating sepsis-induced ALI/ARDS (62).
3.3 The influence of gut microbiota on inflammatory response and immune cells in ARDS
In ARDS, an overactive inflammatory response causes immune system imbalance, worsening outcomes and prognosis. Disrupted intestinal barriers and gut microbiota changes can trigger local and systemic inflammation, promoting ARDS progression (63). In COVID-19, gut microenvironment balance significantly impacts systemic inflammation and ARDS onset (59). In a study conducted by Kyo et al., the lung microbiota in bronchoalveolar lavage fluid (BALF) from ARDS patients was examined. A significant correlation was found between the levels of Staphylococcus, Streptococcus, Enterobacteriaceae in the BALF and serum IL-6 levels (64). Another study revealed that antibiotics disrupt microbiota, altering host responses in LPS-induced lung inflammation and moderately increasing IL-6 in mice (65).
In fecal microbiota transplantation (FMT) treatments, probiotic mixtures containing bacteria such as Bifidobacteria, Lactobacilli, and Thermophilic Streptococci are commonly used (66). Additionally, ideal donors should have a high proportion of Lachnospiraceae, Ruminococcaceae, and Clostridium scindens, which are positively correlated with the secondary bile acids that inhibit Clostridium difficile infection spores (67). Integrating these specific microbial populations into FMT can enhance treatment effectiveness, especially in the context of increasing antimicrobial drug resistance. Li et al. demonstrated that in an ALI rat model, FMT not only reconstituted the gut microbiota structure and augmented bacterial gene abundance but also attenuated inflammatory cell infiltration and pulmonary exudation. Subsequent investigations revealed that FMT, by modulating the gut microbiota, could influence the TGF-β1/Smads/ERK signaling pathway, resulting in a reduction in the synthesis and secretion of inflammatory mediators in vivo. This, in turn, mitigated alveolar epithelial injury and ameliorated LPS-induced endotoxic ALI in the rats (68). Similarly, Tan et al. also indicated that FMT may affect the Keap1-Nrf2/ARE signaling pathway by modulating gut microbiota, enhancing the antioxidant stress response and reducing the production of oxidative damage-related products, thereby improving LPS-induced ALI in rats (69).
Lymphocytes, including T cells, Th cells, and Treg cells, play a pivotal role in mediating the inflammatory response in ARDS. Various studies have observed the distribution of T cells in ARDS. A recent investigation revealed that the Tregs/CD4+ percentage in the blood of ARDS patients was significantly higher compared to non-ARDS patients, while the Tregs/CD4+ percentage in the lung alveoli was lower (69). Similar research has also demonstrated significantly reduced CD4+, CD8+, and B lymphocyte counts in ARDS patients, along with activation of Th1 and Th2, inflammatory response cell apoptosis, cytotoxicity, and endothelial dysfunction (70, 71). Regarding gut microbiota, alterations in composition have been observed in ARDS patients, correlating with changes in lymphocyte counts, specifically CD3+ T cells, CD4+ T cells, and CD4+/CD8+ T cells (71). Nevertheless, further research is essential to gain a comprehensive understanding of this complex interaction.
3.4 Gut microbiota affects oxidative stress in ARDS
The activation of oxidative stress is intricately linked with the inflammatory response, leading to the increased production of pro-oxidants and the suppression of anti-oxidants (72, 73). In ARDS rat models, there is a marked promotion of oxidative stress, inflammatory response, cell apoptosis, and barrier disruption in alveolar epithelial cells (74), while LPS treatment significantly diminishes the activity of superoxide dismutase and glutathione peroxidase (75). Conversely, the inhibition of reactive oxygen species (ROS) and the enhancement of superoxide dismutase, catalase, and glutathione levels in lung tissue are considered beneficial in protecting animals from ARDS (68, 76). For ARDS patients, strategies aimed at reducing inflammation and oxidative stress can bolster the immune system, mitigating the severe impact of COVID-19 progression into ARDS, such approaches may also contribute to improving the treatment success and survival rates of COVID-19 patients (77, 78).
During the progression of ARDS, a complex network of interactions is observed between oxidative stress and alterations in gut microbiota. The imbalance in gut microbiota can trigger lung inflammation and oxidative stress, ultimately leading to gut-barrier-induced lung injury in animals with ALI (61). In a sequencing study utilizing fecal samples from 13 COVID-19 patients, Zhou et al. demonstrated that the metabolism of LPS, ketones, sphingolipids, and neutral amino acids was elevated, while the synthesis pathway of butyrate was anomalously diminished. These findings indicate that the gut microbiota of COVID-19 patients was in a state of oxidative stress (79).
3.5 Gut microbiota affects apoptosis in ARDS
Cellular apoptosis, a critical factor in the development of ARDS, has been found to contribute to the damage of the intestinal mucosal barrier. This damage, in turn, leads to subsequent alterations in gut microbiota, which can further exacerbate the condition. A study found that in LPS-induced ARDS models, inflammatory response, oxidative stress, cell apoptosis elevated, and barrier disruption in alveolar epithelial cells are promoted, while inhibition of cell apoptosis exerted a protective effect on ARDS, while use resveratrol could increase the abundance of Lactobacillus casei, which could be achieved by regulating the activation of the NLRP3 inflammasome and Rho GTPase axis, reducing the infiltration of inflammatory cells and the release of inflammatory factors, resveratrol helps to maintain the normal physiological function of the intestinal barrier, thereby reducing lung tissue damage and improving lung function (74). Studies have also suggested that in the BALB/c mouse model of ALI, the proportion of cell apoptosis was significantly increased, accompanied by an increase in the secretion of cytokines (80–82).
When alterations in gut microbiota occur during ARDS, these changes are typically associated with an increase in cell apoptosis within both lung and intestinal tissues. In a study involving staphylococcal enterotoxin B-induced ARDS in mice, researchers observed dysbiosis in both lung and gut microbiota, along with an increase in the proportion of cell apoptosis (83). Additionally, Xu et al. proposed that the administration of lysophosphatidylcholine could mitigate monocyte infiltration in the ARDS mouse model, an effect attributed to deoxynivalenol trichothecene mycotoxin galactosamine lipopolysaccharide bacteremia. This mitigation was achieved by modulating the MAPK/NF-κB signaling pathway and inhibiting monocyte apoptosis triggered by galactosamine lipopolysaccharide, subsequently enhancing survival rates and lung function (58).
3.6 The influence of gut microbiota on other cell functions (autophagy, pyroptosis, ferroptosis)
Cellular autophagy is often characterized as a ‘double-edged sword’ in various diseases and biological processes. It serves a protective role when functioning within normal limits but can become detrimental when overactivated (84, 85). The alteration of gut microbiota interacts with cellular autophagy, contributing to disease development. In research conducted by Cheng et al., it was discovered that in the piglet model treated with FMT, there was an increase in the abundance of beneficial microbes such as Lactobacillus and Veillonella and a decrease in Enterobacteriaceae and Proteobacteria. Furthermore, FMT also led to the production of short-chain fatty acids and other metabolic products. This activated AMPK, which in turn upregulated autophagy through pathways such as PGC-1α and mTOR. Additionally, FMT increased SIRT1 expression and promoted its deacetylation, activating PGC-1α and FOXO transcription factors. These changes enhanced mitochondrial biogenesis and the expression of autophagy-related genes, ultimately inducing autophagy (86). These findings indicate that FMT may enhance the composition of the gut microbiota, mitigate damage to the intestinal mucosal barrier, and diminish systemic inflammatory responses. This effect is achieved by modulating the metabolic function of the gut microbiota, particularly in the metabolism of linoleic acid. Cao et al. (87)demonstrated that berberine has the ability to inhibit the mammalian target of rapamycin complex 1 (mTORC1) axis. Such inhibition upregulates autophagy-related genes and proteins, such as Beclin-1, ATG5, and LC3II/I, in the intestinal epithelial cells of experimental animals, thereby inducing autophagy. Furthermore, berberine suppresses the TLR4/NF-κB signaling pathway, enhances the expression of tight junction proteins in the intestinal epithelial cells, reduces the phosphorylation of Claudin-2 and Occludin, and strengthens tight junctions and intestinal barrier function. It also stimulates the growth of beneficial bacteria like Lactobacillus and Bifidobacterium while inhibiting the growth of harmful bacteria such as Escherichia coli. This, in turn, increased the diversity and abundance of the gut microbiota in experimental animals. The study (87) also found that the use of 3-MA (an autophagy inhibitor) suppressed the expression of autophagy-related genes mentioned above, impeding the autophagy induction induced by berberine. Consequently, the anti-inflammatory and tissue-protective effects of berberine were weakened (87). Furthermore, a study conducted by Huang et al. confirmed that autophagy plays a vital role in enhancing the integrity of the intestinal mucosal barrier by mitigating oxidative stress in instances of acute severe pancreatitis (88). In the context of ARDS, studies have observed a significant suppression of autophagic activity in septic patients with this condition. The expression of autophagy-related markers such as LC3II, Beclin-1, RAB7, LAMP2, and p62 is markedly decreased. This finding indicates that the evaluation of autophagy-related proteins may hold diagnostic and prognostic significance for septic ARDS (89). Another study confirmed in an ARDS cell model and ALI rat model, treatment with penehyclidine hydrochloride enhanced proliferation and autophagy in ALI models, and reduced cell apoptosis and inflammation (90). However, how gut microbiota influence cell autophagy in ARDS now lacks adequate evidence.
Cell pyroptosis, a form of programmed cell death linked to inflammation, is mediated by inflammasomes like NLRP1, NLRP3, and AIM2, leading to caspase-1 activation and proinflammatory cytokine production, which results in excessive inflammation and cell death (91, 92). This process is crucial in ARDS, contributing to cytokine storms caused by various diseases (93, 94), and is evident in ARDS mouse models with increased NLRP3, IL-1β, and caspase-1 (95). Increased pyroptosis-related protein GSDMD-N in ARDS patients’ PBMCs suggests a role in exacerbating ARDS (96). However, the relationship between pyroptosis and gut microbiota in ARDS is less explored. Metabolite formononetin (FMN), a metabolite of Parabacteroides merdae, inhibits macrophage pyroptosis by interacting with NLRP3. Chen et al. found that reduced Parabacteroides merdae in pregnancy leads to less FMN, thereby facilitating pyroptosis and increasing septic responses and tissue damage (97). Understanding the link between cellular pyroptosis and gut microbiota alterations in ARDS is an emerging research area, potentially revealing new treatment approaches.
Ferroptosis, a newly recognized type of cell death characterized by iron-induced lipid peroxidation (98), is substantially activated in ARDS. This form of regulated cell death promotes inflammation and oxidative stress A parallel study has corroborated that ferroptosis plays a mediating role in inflammation within ALImouse models. By inhibiting ferroptosis, the expression of Nrf2 and HO-1 within the Keap1-Nrf2/HO-1 signaling pathway is augmented. This leads to the neutralization of hydroxyl radicals produced from excessive free iron, mitigating iron-induced cellular damage, and consequently exerting a protective effect against ALI (99). Furthermore, AUF1 plays a role in inhibiting the process of ferroptosis, thereby reducing sepsis-related ALI. This inhibition is achieved through the upregulation of the Nrf2-mediated antioxidant response and the downregulation of ATF3 expression, both of which are key mechanisms in controlling ferroptosis (100). In the contemporary landscape of medical research, the intricate interplay between ferroptosis and the gut microbiome in the context of ARDS remains a relatively uncharted domain. The exploration of how targeted modulation of the gut microbiome may influence ferroptosis, and consequently enhance the prognosis of ARDS, represents a promising avenue for future investigation. Such insights could herald innovative therapeutic strategies, offering new horizons in the management and treatment of ARDS.
4 The metabolic products of gut microbiota in ARDS
4.1 Short-chain fatty acids (butyric, acetic, and propionic acids) produced by gut microbiota in ARDS
The metabolic products of gut microbiota exert profound effects on the health and disease states of the host (human body). These metabolites include short-chain fatty acids (SCFAs), bile acids, amino acid metabolites, and vitamins, playing pivotal roles in maintaining intestinal health, regulating the immune system, and influencing systemic metabolic balance (101). SCFAs such as butyrate, propionate, and acetate, are products of the fermentation of cellulose by intestinal bacteria. They are crucial for the integrity of the intestinal mucosa, promoting the proliferation and repair of intestinal epithelial cells (102).
Butyric acid, a pivotal metabolite produced by gut microbiota, has been demonstrated to modulate the progression of ARDS in both clinical settings and in vitro or in vivo experimental studies. The regulation of this gut microbial metabolite by Vancomycin may attenuate the inflammatory response in ARDS, a mechanism potentially linked to the amelioration of gut barrier dysfunction in patients with ARDS (60). Another study identified a reduction in the synthesis of butyrate by gut microbiota in COVID-19 patients, indicative of an oxidative stress state potentially mediated through the dysfunction of butyrate metabolism (79). Subsequent research has revealed that butyrate may ameliorate inflammation and mitigate oxidative stress in ARDS caused by COVID-19. This suggests that butyrate could serve as an alternative to dexamethasone for treating severe infections, thereby potentially preventing the progression to severe ARDS and the resultant Multiple Organ Dysfunction Syndrome (MODS) (103). In cell and animal models, the gut microbiota metabolite butyrate promoted M2 macrophage polarization, facilitated goblet cell generation, and restored its mucosal barrier repair action in vivo and in vitro (104). This also suggests that butyrate is involved in the regulation of epithelial barrier integrity. Studies also found in ARDS mice there was a significant increase in pro-inflammatory cytokines such as IFN-γ and IL-17, which might be associated with the dysbiosis of the lung and gut microbiome, with a significant reduction in the production of propionate, isobutyrate, and butyrate (83). Furthermore, the administration of anandamide (AEA) has been demonstrated to augment the abundance of beneficial gut bacteria, such as Lactobacillus and Bifidobacterium. This enhancement is also associated with an improvement in the overall architecture of the gut microbiota and an elevation in the concentration of short-chain fatty acids, particularly butyrate. AEA’s involvement in the regulation of the gut-lung axis, its mitigation of inflammatory responses, and its protective role in ARDS have been substantiated (105) (Figure 2) (Table 2).
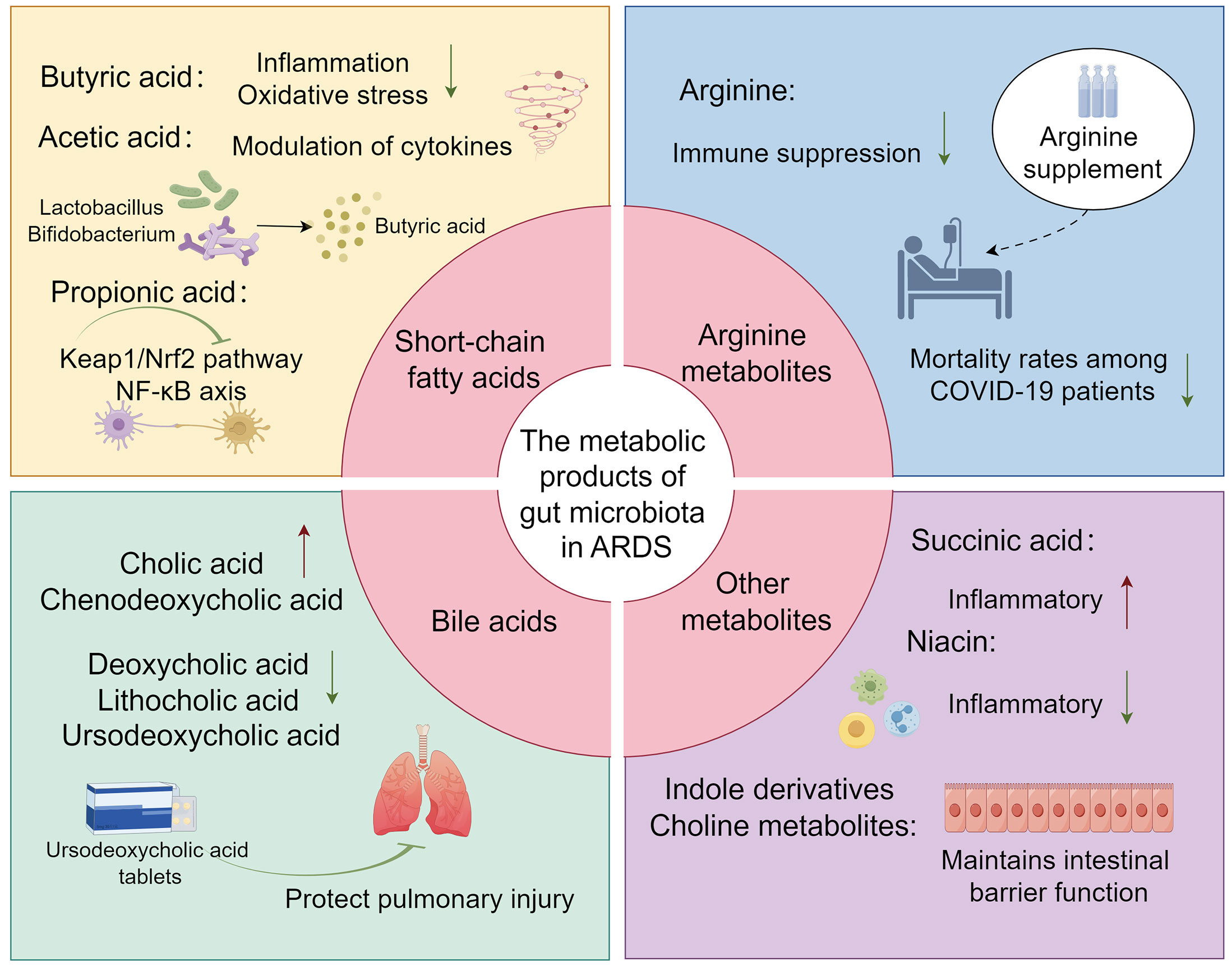
Figure 2 The overview image depicts several key metabolites produced by the gut microbiota, including short-chain fatty acids (such as Butyric, Acetic, and Propionic acids), Arginine, bile acids, and other potential metabolites (such as Succinic acid, Indole derivatives, Niacin, and Choline metabolites), and their roles in ARDS.
Evidence of acetate production by gut microbiota in relation to ARDS is scant in recent studies. Some investigations have hinted at a correlation between the levels of BALF cytokines and specific gut microbiota metabolites, such as acetate and butyrate, in LPS-induced ALI mice (106–108). While these initial explorations have begun to shed light on the association between acetate and butyrate with ARDS, the exact role of acetate within ARDS remains elusive and inadequately defined. The prevailing research, largely confined to animal models and particular human cohorts, has limited the generalizability of these insights. Therefore, further comprehensive and nuanced studies are urgently needed to unravel the intricate mechanisms through which gut microbiota metabolites, specifically acetate and butyrate, modulate ARDS at the molecular and cellular levels.
During the progression of ARDS, the synthesis of propionic acid is posited to ameliorate inflammatory responses and mitigate pulmonary injury. Mohammed et al. conducted an assessment of alterations in lung inflammation, gut/lung microbiota, and SCFA production in ARDS mice. Their findings revealed that propionate possesses the capability to suppress the inflammatory response induced by ARDS (109). Another study elucidated that FMT modulates the structure and function of the gut microbiota, fostering the growth of health-associated functional microbiota, thereby enhancing the production of acetic and propionic acids. This contributes to the protective effect on mice with ARDS. Specifically, propionic acid, acting on the GPR43 receptor on macrophages, inhibits the activation of NLRP3 inflammasomes, suppressing the release and synthesis of TNF-α, IL-1β, and IL-6. This attenuation of the inflammatory response leads to a reduction in lung injury during ARDS (110). Additionally, Zhang et al. demonstrated through animal experiments that sodium propionate has the ability to modulate the Keap1/Nrf2 pathway and inhibit NF-κB axis activation. This leads to a reduction in the inflammatory response and oxidative stress in rats with ALI, thereby ameliorating lipopolysaccharide (LPS)-induced ALI (111).
Propionic acid exerts protective effects in ARDS through multiple targets, including tissue repair, antioxidant and anti-inflammatory effects, maintenance of barrier integrity, modulation of receptor-mediated signaling, and regulation of immune cell function. These mechanisms interact with each other to collectively alleviate the occurrence and progression of ARDS. However, additional studies are necessary to validate and expand upon these mechanisms, as well as to better understand their specific manifestations and contributions. This will provide a robust theoretical foundation for potential clinical applications. Butyrate, acetate, and propionate, as representative products of short-chain fatty acids produced by gut microbiota metabolism, provide new targets for ARDS treatment. However, clinical research and application of these three compounds are still lacking. Future research is warranted to explore their efficacy and safety. In comparison, the study of acetate is relatively limited compared to propionate and butyrate, with unclear mechanisms. Therefore, more attention needs to be paid to the differential study of its role and mechanisms in ARDS and the speed of clinical translation.
4.2 Arginine metabolites produced by gut microbiota in ARDS
Arginine is an essential amino acid in the human body, playing a vital role in various physiological processes (112). It serves as a substrate for the synthesis of nitric oxide, a critical signaling molecule involved in numerous aspects of vascular and immune functions. Arginine may influence patients’ immune nutrition and nitric oxide metabolism to improve patients’ inflammatory condition (113). It also has the ability to inhibit the expression of the T-cell receptor complex CD3, thereby affecting the proliferation and differentiation of T-cells (114, 130). A clinical investigation encompassing 26 patients with COVID-19 revealed a direct correlation between the expansion of myeloid-derived suppressor cells (MDSCs) in association with COVID-19 and a reduction in lymphocytes, coupled with increased arginase activity. Furthermore, supplementation with arginine was observed to mitigate immune suppression, reduce the duration of mechanical ventilation, and decrease mortality rates among the patients (131). An additional study corroborated that curcumin confers protective effects in a rat model of ALI/ARDS by specifically targeting pulmonary M1 macrophages through the use of L-arginine-modified liposomes, thereby modulating immunological responses (115), playing an immunomodulatory role.
Glutamine acts as an essential precursor in the biosynthesis of arginine. Arginine can be synthesized from glutamine either through the enzymatic catalysis of glutamate dehydrogenase or via the conversion of ornithine derived from glutamine, facilitated by glutamine-ornithine transcarbamylase. While supplementation with both glutamine and arginine has demonstrated efficacy in improving clinical outcomes for patients with respiratory illnesses, the therapeutic potential of this approach in the treatment of ARDS warrants further comprehensive studies and rigorous scientific investigation (116, 117).
4.3 Bile acid synthesized by gut microbiota in ARDS
Bile acids are a group of complex molecules derived from cholesterol, primarily synthesized in the liver and secreted into the small intestine (132). They play a crucial role in the digestion and absorption of lipids and fat-soluble vitamins. Beyond their role in digestion, bile acids are increasingly recognized as signaling molecules that regulate metabolic processes within the host. The composition of the gut microbiota is modulated by bile acids; reciprocally, the microbiota exerts a regulatory function in bile acid metabolism (133). Both Clostridium scindens and Clostridium sordellii are gut bacteria with 7α-dehydroxylating capabilities, facilitating the biotransformation of primary bile acids into secondary bile acids. These bacterial species additionally secrete specific tryptophan-derived antibiotics, identified as 1-acetyl-β-carboline and turbomycin A. These antibiotics act by inhibiting the formation of the septum during bacterial cell division, thereby exerting a suppressive effect on the growth of Clostridium difficile as well as other constituents of the gut microbiota. This regulatory interaction plays a pivotal role in maintaining the homeostasis of the gastrointestinal microbiome (119). In patients with ARDS, existing evidence suggests a dysregulation in bile acid metabolism, characterized by elevated levels of primary bile acids such as cholic acid and chenodeoxycholic acid, and a concomitant reduction in secondary bile acids, including deoxycholic acid, lithocholic acid, and ursodeoxycholic acid. This distinct profile of plasma bile acid concentrations may function as an adaptive physiological response in the pathogenesis of ARDS (118). The research conducted by Niu F and colleagues demonstrated that ursodeoxycholic acid exerts a protective effect against pulmonary injury resulting from ARDS. In a clinical investigation conducted by Harnisch and colleagues, it was observed that patients with ARDS exhibited elevated levels of tauro-conjugated bile acids and a significantly diminished glycine-to-taurine conjugation ratio (G/T ratio) in comparison to the control cohort (134). In a study led by Kean and associates, it was elucidated that pediatric patients in critical condition, encompassing those diagnosed with ARDS, manifest gut microbiota dysbiosis. This imbalance is typified by a reduction in the genus Bifidobacterium and an augmentation in the genus Veillonella. Concurrently, this dysbiosis is associated with perturbations in bile acid metabolism, including a decline in dehydroxy bile acids and hydrogen ions, coupled with an elevation in hydroxylated hydrophobic bile acids (135).
The current body of research elucidates the putative roles of gut microbiota and bile acid metabolism in the pathophysiology of ARDS. These findings not only offer potential biomarkers for the diagnosis and therapeutic intervention of ARDS but also lay the groundwork for subsequent investigations. Future research endeavors should focus on delineating the precise mechanistic contributions of gut microbiota and bile acid metabolic pathways in the etiology of ARDS, thereby paving the way for the exploration of targeted modulation of these systems for prevention or treatment of the condition.
4.4 Potential roles of other metabolites (succinic acid, indole derivatives, niacin, and choline metabolites) produced by gut microbiota in ARDS
The occurrence of membrane-bound succinates is intricately linked with protein succinylation, a specific form of post-translational modification. This biochemical modification plays a pivotal role in the stabilization of hypoxia-inducible factortranscription factors, the initiation of pro-inflammatory signaling cascades, epigenetic modulation, and the production of reactive ROS (120–122). During episodes of intestinal ischemia/reperfusion (I/R), there is a marked elevation in plasma succinate concentrations. This increase functionally activates the succinate receptor 1 (SUCNR1) expressed on alveolar macrophages. Activation of SUCNR1 subsequently initiates the Phosphoinositide 3-Kinase-Protein Kinase B (PI3K-AKT)/Hypoxia-Inducible Factor-1αintracellular signaling cascade. This molecular eventuality culminates in the elicitation of pro-inflammatory responses within alveolar macrophages, thereby serving as a pathophysiological precursor to acute lung injury in the context of intestinal I/R (106, 123). According to the research conducted by Chouchani et al., succinate serves as a pivotal modulator in the induction of mitochondrial reactive ROS during the reperfusion phase subsequent to ischemic events. This accumulation of succinate is notably ascribed to the reversal of enzymatic activity of succinate dehydrogenase during the ischemic phase (136). The aforementioned study elucidates that the inhibition of succinate accumulation and the attenuation of mitochondrial ROS generation serve as potential therapeutic targets for diseases induced by ischemia-reperfusion injuries and related pathological conditions. In the presence of high-risk factors for ARDS or during the onset and progression of ARDS, the precise role of succinate warrants further comprehensive investigation for a more detailed understanding.
Indole, a pivotal metabolite in bacterial tryptophan metabolism, exerts a multifaceted regulatory influence on microbial behavior and host-pathogen interactions. Specifically, indole modulates bacterial motility, biofilm formation, antibiotic resistance, and exerts multifunctional effects on host cell invasion and the expression of virulence factors. Upon exposure to indole, normal cells are capable of enhancing the integrity of the host epithelial barrier by upregulating the transcriptional activity of genes associated with epithelial barrier function (124). Additionally, indole can regulate cytokines to improve inflammation and damage (62). Recent research indicated a significant reduction in the levels of indole-3-acetate and indole-3-propionate in both septic mice and patients (125, 126). Evidence suggested that in chronic lung disease, metabolites produced by the gut microbiota exhibited anti-inflammatory and anti-infective activity, and during this progress, indole compounds and their derivatives have been shown to activate the aryl hydrocarbon receptor signaling in astrocytes, leading to the inhibition of inflammation in the central nervous system (137–139). These results can imply potential roles of indole and the derivatives in ARDS, while both clinical and experimental works should be done to confirm it. Additionally, Nicotinic acid, a gut microbiota-derived short-chain fatty acid, serves as an agonist for G protein-coupled receptor 109A (GPR109A) expressed on colonic macrophages and dendritic cells. Activation of GPR109A leads to the suppression of NF-κB signaling pathways and a concomitant reduction in proinflammatory cytokine synthesis. Simultaneously, it enhances the generation of regulatory T cells and IL-10, thereby manifesting both anti-inflammatory and immunomodulatory properties. These effects hold potential for mitigating gut microbiota dysbiosis and immune-mediated inflammation commonly observed in COVID-19 patients (127). In ARDS, the metabolism and influence of niacin still lack evidence.
Choline is ubiquitously present in all cell membranes and various secretions, predominantly in the form of phosphatidylcholine and sphingomyelin, particularly in pulmonary tissues. In the lungs, it is found in pulmonary surfactants and alveolar lipoproteins. Certain probiotics in the gut microbiota, such as Bifidobacterium, are capable of producing choline. Beyond maintaining intestinal barrier function, choline also mitigates inflammation induced by bacterial translocation and exhibits anti-inflammatory properties. A widespread deficiency of choline is observed in patients with cystic fibrosis, with a reduction in choline-producing probiotics in the gut microbiota being one of the primary contributing factors (129). A recent report employing metabolomic analysis has elucidated distinct metabolic profiles between influenza-induced ARDS and COVID-19-induced ARDS. This finding serves as a foundational basis for investigating the influence of gut microbiota-derived metabolites on the pathophysiology of ARDS (128). Further investigation into the anti-inflammatory properties of choline and its regulatory effects on gut microbiota, along with the exploration of niacin’s impact on the gut-lung axis, will constitute focal points in future research within the realm of ARDS.
5 Conclusion and prospect
The intricate crosstalk between gut microbiota and ARDS exerts a synergistic influence on the trajectory of disease progression. ARDS instigates perturbations in the compositional architecture of the gut microbiota, subsequently precipitating disruptions in the integrity of the intestinal mucosal barrier, bacterial translocation, endotoxin absorption, and eliciting anomalies in humoral immunity. These disruptions further manifest as dysregulated inflammatory responses, heightened oxidative stress, and cellular processes including apoptosis, autophagy, pyroptosis, and ferroptosis. Conversely, specific microbial metabolites, such as butyrate, propionate, arginine, and bile acids, have demonstrated therapeutic efficacy in attenuating the inflammatory and oxidative sequelae associated with ARDS. Recent advancements in the field have earmarked gut microbiota modulation as a novel therapeutic paradigm for ARDS management. Interventions designed to re-establish gut microbial homeostasis—through probiotic supplementation, provision of probiotic-enriched dietary sources, and targeted inhibition of pathogenic bacterial proliferation—have shown promise in fortifying the intestinal barrier, mitigating inflammatory cascades, and improving the clinical prognosis of ARDS patients. Despite these advancements, the current evidence base remains inadequate for a comprehensive understanding of the multifaceted roles that gut microbiota play in ARDS, especially concerning the mechanistic impact of distinct metabolites and the molecular pathways implicated in this complex interplay.
To comprehensively elucidate the intricate roles of gut microbiota and their metabolic byproducts in the pathophysiology of ARDS, a series of methodologically rigorous empirical studies are urgently warranted. Initially, an exhaustive investigation into the multifaceted roles and interdependent mechanisms of specific microbial metabolites within the ARDS pathophysiological milieu is imperative. Concurrently, further scrutiny is required to understand the intracellular signaling cascades, receptor-ligand interactions, and cytokine-mediated regulatory pathways between the gut microbiota and ARDS. Subsequently, a systematic categorization and characterization of the gut microbiota across diverse ARDS patient cohorts should be undertaken. This would facilitate the identification of specific microbial taxa significantly correlated with clinical biomarkers, thereby laying the groundwork for microbiota-centric, precision medicine-based therapeutic interventions. Such interventions may encompass the application of FMT and the formulation of individualized probiotic regimens. In the realm of clinical research, a multidimensional approach incorporating metagenomics, metabolomics, transcriptomics, and epidemiological studies of the gut microbiota is advocated. The focus of therapeutic strategies should transition from conventional symptomatic treatments, such as restrictive ventilation and prone positioning, to more targeted interventions aimed at restoring immunological homeostasis, mitigating inflammatory cascade amplification, and re-establishing the ecological stability of the gut-lung axis. Modulating the gut microbiota and fortifying the integrity of the intestinal barrier may serve as pivotal mechanisms for minimizing bacterial translocation, reducing endotoxin exposure, and maintaining systemic immunological balance, thereby potentially decreasing the incidence and severity of ARDS. Consequently, further and comprehensive research endeavors are anticipated to provide groundbreaking insights into the early diagnosis, targeted therapeutic interventions, disease trajectory, prognostic evaluation, and rehabilitation strategies for ARDS.
Author contributions
D-WZ: Investigation, Project administration, Resources, Software, Supervision, Writing – review & editing. J-LL: Methodology, Software, Validation, Visualization, Writing – original draft. B-YD: Methodology, Project administration, Software, Visualization, Writing – original draft. M-YF: Conceptualization, Project administration, Writing – review & editing. XX: Formal Analysis, Methodology, Writing – review & editing. X-JQ: Funding acquisition, Methodology, Project administration, Writing – original draft. X-MF: Conceptualization, Methodology, Project administration, Resources, Writing – review & editing.
Funding
The author(s) declare financial support was received for the research, authorship, and/or publication of this article. This work was supported by Sichuan Science and Technology Program (No.2022YFS0631) and Natural Science Foundation of Sichuan Province of China (No. 2022NSFSC0046).
Conflict of interest
The authors declare that the research was conducted in the absence of any commercial or financial relationships that could be construed as a potential conflict of interest.
Publisher’s note
All claims expressed in this article are solely those of the authors and do not necessarily represent those of their affiliated organizations, or those of the publisher, the editors and the reviewers. Any product that may be evaluated in this article, or claim that may be made by its manufacturer, is not guaranteed or endorsed by the publisher.
References
1. Huppert LA, Matthay MA, Ware LB. Pathogenesis of acute respiratory distress syndrome. Semin Respir Crit Care Med (2019) 40(1):31–9. doi: 10.1055/s-0039-1683996
2. Lu S, Huang X, Liu R, Lan Y, Lei Y, Zeng F, et al. Comparison of COVID-19 induced respiratory failure and typical ARDS: Similarities and differences. Front Med (Lausanne) (2022) 9:829771. doi: 10.3389/fmed.2022.829771
3. Gragossian A, Siuba MT. Acute respiratory distress syndrome. Emerg Med Clin North Am (2022) 40(3):459–72. doi: 10.1016/j.emc.2022.05.002
4. Hendrickson KW, Peltan ID, Brown SM. The epidemiology of acute respiratory distress syndrome before and after coronavirus disease 2019. Crit Care Clin (2021) 37(4):703–16. doi: 10.1016/j.ccc.2021.05.001
5. Khemani RG, Smith L, Lopez-Fernandez YM, Kwok J, Morzov R, Klein MJ, et al. Paediatric acute respiratory distress syndrome incidence and epidemiology (PARDIE): An international, observational study. Lancet Respir Med (2019) 7(2):115–28. doi: 10.1016/s2213-2600(18)30344-8
6. Eworuke E, Major JM, Gilbert McClain LI. National incidence rates for Acute Respiratory Distress Syndrome (ARDS) and ARDS cause-specific factors in the United States (2006-2014). J Crit Care (2018) 47:192–7. doi: 10.1016/j.jcrc.2018.07.002
7. Hernu R, Wallet F, Thiollière F, Martin O, Richard JC, Schmitt Z, et al. An attempt to validate the modification of the American-European consensus definition of acute lung injury/acute respiratory distress syndrome by the Berlin definition in a university hospital. Intensive Care Med (2013) 39(12):2161–70. doi: 10.1007/s00134-013-3122-6
8. Huang X, Zhang R, Fan G, Wu D, Lu H, Wang D, et al. Incidence and outcomes of acute respiratory distress syndrome in intensive care units of mainland China: a multicentre prospective longitudinal study. Crit Care (2020) 24(1):515. doi: 10.1186/s13054-020-03112-0
9. Hasan SS, Capstick T, Ahmed R, Kow CS, Mazhar F, Merchant HA, et al. Mortality in COVID-19 patients with acute respiratory distress syndrome and corticosteroids use: a systematic review and meta-analysis. Expert Rev Respir Med (2020) 14(11):1149–63. doi: 10.1080/17476348.2020.1804365
10. Henry BM, Lippi G. Poor survival with extracorporeal membrane oxygenation in acute respiratory distress syndrome (ARDS) due to coronavirus disease 2019 (COVID-19): Pooled analysis of early reports. J Crit Care (2020) 58:27–8. doi: 10.1016/j.jcrc.2020.03.011
11. Qu W, Wang Z, Hare JM, Bu G, Mallea JM, Pascual JM, et al. Cell-based therapy to reduce mortality from COVID-19: Systematic review and meta-analysis of human studies on acute respiratory distress syndrome. Stem Cells Transl Med (2020) 9(9):1007–22. doi: 10.1002/sctm.20-0146
12. Fan E, Brodie D, Slutsky AS. Acute respiratory distress syndrome: Advances in diagnosis and treatment. Jama (2018) 319(7):698–710. doi: 10.1001/jama.2017.21907
13. Yadav H, Kor DJ. Platelets in the pathogenesis of acute respiratory distress syndrome. Am J Physiol Lung Cell Mol Physiol (2015) 309(9):L915–23. doi: 10.1152/ajplung.00266.2015
14. Perkins GD, McAuley DF, Thickett DR, Gao F. The beta-agonist lung injury trial (BALTI): a randomized placebo-controlled clinical trial. Am J Respir Crit Care Med (2006) 173(3):281–7. doi: 10.1164/rccm.200508-1302OC
15. Abe T, Madotto F, Pham T, Nagata I, Uchida M, Tamiya N, et al. Epidemiology and patterns of tracheostomy practice in patients with acute respiratory distress syndrome in ICUs across 50 countries. Crit Care (2018) 22(1):195. doi: 10.1186/s13054-018-2126-6
16. Segal LN, Clemente JC, Tsay JC, Koralov SB, Keller BC, Wu BG, et al. Enrichment of the lung microbiome with oral taxa is associated with lung inflammation of a Th17 phenotype. Nat Microbiol (2016) 1:16031. doi: 10.1038/nmicrobiol.2016.31
17. Lira-Lucio JA, Falfán-Valencia R, Ramírez-Venegas A, Buendía-Roldán I, Rojas-Serrano J, Mejía M, et al. Lung microbiome participation in local immune response regulation in respiratory diseases. Microorganisms (2020) 8(7):1059. doi: 10.3390/microorganisms8071059
18. Khatiwada S, Subedi A. Lung microbiome and coronavirus disease 2019 (COVID-19): Possible link and implications. Hum Microb J (2020) 17:100073. doi: 10.1016/j.humic.2020.100073
19. De Nuccio F, Piscitelli P, Toraldo DM. Gut-lung microbiota interactions in chronic obstructive pulmonary disease (COPD): Potential mechanisms driving progression to COPD and epidemiological data. Lung (2022) 200(6):773–81. doi: 10.1007/s00408-022-00581-8
20. Dickson RP, Schultz MJ, van der Poll T, Schouten LR, Falkowski NR, Luth JE, et al. Lung microbiota predict clinical outcomes in critically ill patients. Am J Respir Crit Care Med (2020) 201(5):555–63. doi: 10.1164/rccm.201907-1487OC
21. Yang D, Xing Y, Song X, Qian Y. The impact of lung microbiota dysbiosis on inflammation. Immunology (2020) 159(2):156–66. doi: 10.1111/imm.13139
22. García-Montero C, Fraile-Martínez O, Gómez-Lahoz AM, Pekarek L, Castellanos AJ, Noguerales-Fraguas F, et al. Nutritional components in western diet versus mediterranean diet at the gut microbiota-immune system interplay. Implications for health and disease. Nutrients (2021) 13(2):699. doi: 10.3390/nu13020699
23. Spencer SP, Fragiadakis GK, Sonnenburg JL. Pursuing human-relevant gut microbiota-immune interactions. Immunity (2019) 51(2):225–39. doi: 10.1016/j.immuni.2019.08.002
24. Agirman G, Yu KB, Hsiao EY. Signaling inflammation across the gut-brain axis. Science (2021) 374(6571):1087–92. doi: 10.1126/science.abi6087
25. Tilg H, Adolph TE, Trauner M. Gut-liver axis: Pathophysiological concepts and clinical implications. Cell Metab (2022) 34(11):1700–18. doi: 10.1016/j.cmet.2022.09.017
26. Chen W, Zhang S, Wu J, Ye T, Wang S, Wang P, et al. Butyrate-producing bacteria and the gut-heart axis in atherosclerosis. Clin Chim Acta (2020) 507:236–41. doi: 10.1016/j.cca.2020.04.037
27. Gao T, Wang X, Li Y, Ren F. The role of probiotics in skin health and related gut-skin axis: A review. Nutrients (2023) 15(14):3123. doi: 10.3390/nu15143123
28. Enaud R, Prevel R, Ciarlo E, Beaufils F, Wieërs G, Guery B, et al. The gut-lung axis in health and respiratory diseases: A place for inter-organ and inter-kingdom crosstalks. Front Cell Infect Microbiol (2020) 10:9. doi: 10.3389/fcimb.2020.00009
29. Li M, Zhang R, Li J, Li J. The role of C-type lectin receptor signaling in the intestinal microbiota-inflammation-cancer axis. Front Immunol (2022) 13:894445. doi: 10.3389/fimmu.2022.894445
30. Spiljar M, Merkler D, Trajkovski M. The immune system bridges the gut microbiota with systemic energy homeostasis: Focus on TLRs, mucosal barrier, and SCFAs. Front Immunol (2017) 8:1353. doi: 10.3389/fimmu.2017.01353
31. Pu Q, Lin P, Gao P, Wang Z, Guo K, Qin S, et al. Gut microbiota regulate gut-lung axis inflammatory responses by mediating ILC2 compartmental migration. J Immunol (2021) 207(1):257–67. doi: 10.4049/jimmunol.2001304
32. Ahlawat S, Asha, Sharma KK. Immunological co-ordination between gut and lungs in SARS-CoV-2 infection. Virus Res (2020) 286:198103. doi: 10.1016/j.virusres.2020.198103
33. de Oliveira GLV, Oliveira CNS, Pinzan CF, de Salis LVV, Cardoso CRB. Microbiota modulation of the gut-lung axis in COVID-19. Front Immunol (2021) 12:635471. doi: 10.3389/fimmu.2021.635471
34. Yang W, Ansari AR, Niu X, Zou W, Lu M, Dong L, et al. Interaction between gut microbiota dysbiosis and lung infection as gut-lung axis caused by Streptococcus suis in mouse model. Microbiol Res (2022) 261:127047. doi: 10.1016/j.micres.2022.127047
35. Zhou Y, Zhao X, Zhang M, Feng J. Gut microbiota dysbiosis exaggerates ammonia-induced tracheal injury Via TLR4 signaling pathway. Ecotoxicol Environ Saf (2022) 246:114206. doi: 10.1016/j.ecoenv.2022.114206
36. Sencio V, MaChado MG, Trottein F. The lung-gut axis during viral respiratory infections: the impact of gut dysbiosis on secondary disease outcomes. Mucosal Immunol (2021) 14(2):296–304. doi: 10.1038/s41385-020-00361-8
37. Aggarwal N, Kitano S, Puah GRY, Kittelmann S, Hwang IY, Chang MW. Microbiome and human health: Current understanding, engineering, and enabling technologies. Chem Rev (2023) 123(1):31–72. doi: 10.1021/acs.chemrev.2c00431
38. Ramírez-Labrada AG, Isla D, Artal A, Arias M, Rezusta A, Pardo J, et al. The influence of lung microbiota on lung carcinogenesis, immunity, and immunotherapy. Trends Cancer (2020) 6(2):86–97. doi: 10.1016/j.trecan.2019.12.007
39. Fabbrizzi A, Amedei A, Lavorini F, Renda T, Fontana G. The lung microbiome: clinical and therapeutic implications. Intern Emerg Med (2019) 14(8):1241–50. doi: 10.1007/s11739-019-02208-y
40. Gavzy SJ, Kensiski A, Lee ZL, Mongodin EF, Ma B, Bromberg JS. Bifidobacterium mechanisms of immune modulation and tolerance. Gut Microbes (2023) 15(2):2291164. doi: 10.1080/19490976.2023.2291164
41. Leylabadlo HE, Ghotaslou R, Feizabadi MM, Farajnia S, Moaddab SY, Ganbarov K, et al. The critical role of Faecalibacterium prausnitzii in human health: An overview. Microb Pathog (2020) 149:104344. doi: 10.1016/j.micpath.2020.104344
42. Lim SC, Knight DR, Riley TV. Clostridium difficile and one health. Clin Microbiol Infect (2020) 26(7):857–63. doi: 10.1016/j.cmi.2019.10.023
43. Mansour S, Asrar T, Elhenawy W. The multifaceted virulence of adherent-invasive Escherichia coli. Gut Microbes (2023) 15(1):2172669. doi: 10.1080/19490976.2023.2172669
44. Ge Y, Lin S, Li B, Yang Y, Tang X, Shi Y, et al. Oxidized pork induces oxidative stress and inflammation by altering gut microbiota in mice. Mol Nutr Food Res (2020) 64(2):e1901012. doi: 10.1002/mnfr.201901012
45. Kim HS. Do an altered gut microbiota and an associated leaky gut affect COVID-19 severity? mBio (2021) 12(1):e03022–20. doi: 10.1128/mbio.03022-20
46. Tang L, Gu S, Gong Y, Li B, Lu H, Li Q, et al. Clinical significance of the correlation between changes in the major intestinal bacteria species and COVID-19 severity. Engineering (2020) 6(10):1178–84. doi: 10.1016/j.eng.2020.05.013
47. Hu X, Han Z, Zhou R, Su W, Gong L, Yang Z, et al. Altered gut microbiota in the early stage of acute pancreatitis were related to the occurrence of acute respiratory distress syndrome. Front Cell Infection Microbiol (2023) 13:1127369. doi: 10.3389/fcimb.2023.1127369
48. Zuo T, Zhang F, Lui GCY, Yeoh YK, Li AYL, Zhan H, et al. Alterations in gut microbiota of patients with COVID-19 during time of hospitalization. Gastroenterology (2020) 159(3):944–55.e8. doi: 10.1053/j.gastro.2020.05.048
49. Panzer AR, Lynch SV, Langelier C, Christie JD, McCauley K, Nelson M, et al. Lung microbiota is related to smoking status and to development of acute respiratory distress syndrome in critically ill trauma patients. Am J Respir Crit Care Med (2018) 197(5):621–31. doi: 10.1164/rccm.201702-0441oc
50. Chen J, Hall S, Vitetta L. Altered gut microbial metabolites could mediate the effects of risk factors in Covid-19. Rev Med Virol (2021) 31(5):1–13. doi: 10.1002/rmv.2211
51. Albert-Bayo M, Paracuellos I, González-Castro AM, Rodríguez-Urrutia A, Rodríguez-Lagunas MJ, Alonso-Cotoner C, et al. Intestinal mucosal mast cells: Key modulators of barrier function and homeostasis. Cells (2019) 8(2):135. doi: 10.3390/cells8020135
52. Okumura R, Takeda K. Maintenance of intestinal homeostasis by mucosal barriers. Inflammation Regener (2018) 38:5. doi: 10.1186/s41232-018-0063-z
53. Ren Z, Guo C, Yu S, Zhu L, Wang Y, Hu H, et al. Progress in mycotoxins affecting intestinal mucosal barrier function. Int J Mol Sci (2019) 20(11):2777. doi: 10.3390/ijms20112777
54. Chivero ET, Ahmad R, Thangaraj A, Periyasamy P, Kumar B, Kroeger E, et al. Cocaine induces inflammatory gut milieu by compromising the mucosal barrier integrity and altering the gut microbiota colonization. Sci Rep (2019) 9(1):12187. doi: 10.1038/s41598-019-48428-2
55. Zhang B, Yue R, Chen Y, Huang X, Yang M, Shui J, et al. The herbal medicine scutellaria-coptis alleviates intestinal mucosal barrier damage in diabetic rats by inhibiting inflammation and modulating the gut microbiota. Evidence-Based Complementary Altern Med (2020) 2020:1–17. doi: 10.1155/2020/4568629
56. Yu Y, Zhu H, Shen M, Yu Q, Chen Y, Xie J. Sulfation modification enhances the intestinal regulation of Cyclocarya paliurus polysaccharides in cyclophosphamide-treated mice via restoring intestinal mucosal barrier function and modulating gut microbiota. Food Funct (2021) 12(24):12278–90. doi: 10.1039/d1fo03042f
57. Chen Y, Yang B, Ross RP, Jin Y, Stanton C, Zhao J, et al. Orally administered CLA ameliorates DSS-induced colitis in mice via intestinal barrier improvement, oxidative stress reduction, and inflammatory cytokine and gut microbiota modulation. J Agric Food Chem (2019) 67(48):13282–98. doi: 10.1021/acs.jafc.9b05744
58. Mustansir Dawoodbhoy F, Patel BK, Patel K, Bhatia M, Lee CN, Moochhala SM. Gut microbiota dysbiosis as a target for improved post-surgical outcomes and improved patient care: A review of current literature. Shock (2021) 55(4):441–54. doi: 10.1097/shk.0000000000001654
59. Settanni CR, Ianiro G, Ponziani FR, Bibbò S, Segal JP, Cammarota G, et al. COVID-19 as a trigger of irritable bowel syndrome: A review of potential mechanisms. World J Gastroenterol (2021) 27(43):7433–45. doi: 10.3748/wjg.v27.i43.7433
60. Chen J, Zhang X, Xiong F, Zheng H, Zhang W, Shen Y, et al. Vancomycin protects against acute respiratory distress syndrome by promoting butyrate metabolism. (2022). doi: 10.21203/rs.3.rs-2364330/v1
61. Tang J, Xu L, Zeng Y, Gong F. Effect of gut microbiota on LPS-induced acute lung injury by regulating the TLR4/NF-kB signaling pathway. Int Immunopharmacol (2021) 91:107272. doi: 10.1016/j.intimp.2020.107272
62. Zhou X, Liao Y. Gut-lung crosstalk in sepsis-induced acute lung injury. Front Microbiol (2021) 12:779620. doi: 10.3389/fmicb.2021.779620
63. Kyo M, Nishioka K, Nakaya T, Kida Y, Tanabe Y, Ohshimo S, et al. Unique patterns of lower respiratory tract microbiota are associated with inflammation and hospital mortality in acute respiratory distress syndrome. Respir Res (2019) 20(1):246. doi: 10.1186/s12931-019-1203-y
64. Kyo M, Nishioka K, Nakaya T, Kida Y, Tanabe Y, Ohshimo S, et al. Unique patterns of lower respiratory tract microbiota are associated with inflammation and hospital mortality in acute respiratory distress syndrome. Respir Res (2019) 20(1):1–9. doi: 10.1186/s12931-019-1203-y
65. Jacobs MC, Lankelma JM, Wolff NS, Hugenholtz F, de Vos AF, van der Poll T, et al. Effect of antibiotic gut microbiota disruption on LPS-induced acute lung inflammation. PloS One (2020) 15(11):e0241748. doi: 10.1371/journal.pone.0241748
66. Chen D, Wu J, Jin D, Wang B, Cao H. Fecal microbiota transplantation in cancer management: Current status and perspectives. Int J Cancer (2019) 145(8):2021–31. doi: 10.1002/ijc.32003
67. Kazemian N, Kao D, Pakpour S. Fecal microbiota transplantation during and post-COVID-19 pandemic. Int J Mol Sci (2021) 22(6):3004. doi: 10.3390/ijms22063004
68. Erol N, Saglam L, Saglam YS, Erol HS, Altun S, Aktas MS, et al. The protection potential of antioxidant vitamins against acute respiratory distress syndrome: A rat trial. Inflammation (2019) 42(5):1585–94. doi: 10.1007/s10753-019-01020-2
69. Halter S, Aimade L, Barbié M, Brisson H, Rouby JJ, Langeron O, et al. T regulatory cells activation and distribution are modified in critically ill patients with acute respiratory distress syndrome: A prospective single-centre observational study. Anaesth Crit Care Pain Med (2020) 39(1):35–44. doi: 10.1016/j.accpm.2019.07.014
70. Bouadma L, Wiedemann A, Patrier J, Surénaud M, Wicky PH, Foucat E, et al. Immune alterations in a patient with SARS-CoV-2-related acute respiratory distress syndrome. J Clin Immunol (2020) 40(8):1082–92. doi: 10.1007/s10875-020-00839-x
71. Zhou Y, Shi X, Fu W, Xiang F, He X, Yang B, et al. Gut microbiota dysbiosis correlates with abnormal immune response in moderate COVID-19 patients with fever. J Inflammation Res (2021) 14:2619–31. doi: 10.2147/jir.s311518
72. Koutsaliaris IK, Moschonas IC, Pechlivani LM, Tsouka AN, Tselepis AD. Inflammation, oxidative stress, vascular aging and atherosclerotic ischemic stroke. Curr Med Chem (2022) 29(34):5496–509. doi: 10.2174/0929867328666210921161711
73. Chernyak BV, Popova EN, Prikhodko AS, Grebenchikov OA, Zinovkina LA, Zinovkin RA. COVID-19 and oxidative stress. Biochem (Mosc) (2020) 85(12):1543–53. doi: 10.1134/s0006297920120068
74. Shi X, Zhu L, Wang S, Zhu W, Li Q, Wei J, et al. Magnesium hydride ameliorates endotoxin-induced acute respiratory distress syndrome by inhibiting inflammation, oxidative stress, and cell apoptosis. Oxid Med Cell Longev (2022) 2022:5918954. doi: 10.1155/2022/5918954
75. Wang X, Lai R, Su X, Chen G, Liang Z. Edaravone attenuates lipopolysaccharide-induced acute respiratory distress syndrome associated early pulmonary fibrosis via amelioration of oxidative stress and transforming growth factor-β1/Smad3 signaling. Biochem Biophys Res Commun (2018) 495(1):706–12. doi: 10.1016/j.bbrc.2017.10.165
76. Wang X, Zhang C, Zou N, Chen Q, Wang C, Zhou X, et al. Lipocalin-2 silencing suppresses inflammation and oxidative stress of acute respiratory distress syndrome by ferroptosis via inhibition of MAPK/ERK pathway in neonatal mice. Bioengineered (2021) 13(1):508–20. doi: 10.1080/21655979.2021.2009970
77. Villapol S. Gastrointestinal symptoms associated with COVID-19: impact on the gut microbiome. Trans Res (2020) 226:57–69. doi: 10.1016/j.trsl.2020.08.004
78. Zabetakis I, Lordan R, Norton C, Tsoupras A. COVID-19: The inflammation link and the role of nutrition in potential mitigation. Nutrients (2020) 12(5):1466. doi: 10.3390/nu12051466
79. Zhou T, Wu J, Zeng Y, Li J, Yan J, Meng W, et al. SARS-CoV-2 triggered oxidative stress and abnormal energy metabolism in gut microbiota. MedComm (2022) 3(1):e112. doi: 10.1002/mco2.112
80. Li K, He Z, Wang X, Pineda M, Chen R, Liu H, et al. Apigenin C-glycosides of Microcos paniculata protects lipopolysaccharide induced apoptosis and inflammation in acute lung injury through TLR4 signaling pathway. Free Radical Biol Med (2018) 124:163–75. doi: 10.1016/j.freeradbiomed.2018.06.009
81. Ju Y-n, Gong J, Wang X-t, Zhu J-l, Gao W. Endothelial colony-forming cells attenuate ventilator-induced lung injury in rats with acute respiratory distress syndrome. Arch Med Res (2018) 49(3):172–81. doi: 10.1016/j.arcmed.2018.08.006
82. Mohammed A FK, Alghetaa H, Miranda K, Wilson K, Singh N P, Cai G, et al. Δ9-tetrahydrocannabinol prevents mortality from acute respiratory distress syndrome through the induction of apoptosis in immune cells, leading to cytokine storm suppression. Int J Mol Sci (2020) 21(17):6244. doi: 10.3390/ijms21176244
83. Alghetaa H, Mohammed A, Zhou J, Singh N, Nagarkatti M, Nagarkatti P. Resveratrol-mediated attenuation of superantigen-driven acute respiratory distress syndrome is mediated by microbiota in the lungs and gut. Pharmacol Res (2021) 167:105548. doi: 10.1016/j.phrs.2021.105548
84. Peng L, Hu G, Yao Q, Wu J, He Z, Law BY, et al. Microglia autophagy in ischemic stroke: A double-edged sword. Front Immunol (2022) 13:1013311. doi: 10.3389/fimmu.2022.1013311
85. Yan Q, Zhang Y, Wang Q, Yuan L. Autophagy: A double-edged sword in male reproduction. Int J Mol Sci (2022) 23(23):15273. doi: 10.3390/ijms232315273
86. Cheng S, Ma X, Geng S, Jiang X, Li Y, Hu L, et al. Fecal microbiota transplantation beneficially regulates intestinal mucosal autophagy and alleviates gut barrier injury. mSystems (2018) 3(5):e00137–18. doi: 10.1128/msystems.00137-18
87. Cao J, Chen M, Xu R, Guo M. Therapeutic mechanisms of berberine to improve the intestinal barrier function via modulating gut microbiota, TLR4/NF-κ B/MTORC pathway and autophagy in cats. Front Microbiol (2022) 13:961885. doi: 10.3389/fmicb.2022.961885
88. Huang L, Jiang Y, Sun Z, Gao Z, Wang J, Zhang D. Autophagy strengthens intestinal mucosal barrier by attenuating oxidative stress in severe acute pancreatitis. Digestive Dis Sci (2018) 63(4):910–9. doi: 10.1007/s10620-018-4962-2
89. Xu JL, Yu XL, Yao XQ, Xu LH, Jing M. Diagnostic value and prognostic evaluation of autophagy-related protein expression level in sepsis complicated with acute respiratory distress syndrome. Dis Markers (2022) 2022:8920926. doi: 10.1155/2022/8920926
90. Wang X, Liu F, Xu M, Wu L. Penehyclidine hydrochloride alleviates lipopolysaccharide−induced acute respiratory distress syndrome in cells via regulating autophagy−related pathway. Mol Med Rep (2021) 23(2):100. doi: 10.3892/mmr.2020.11739
91. Hsu SK, Li CY, Lin IL, Syue WJ, Chen YF, Cheng KC, et al. Inflammation-related pyroptosis, a novel programmed cell death pathway, and its crosstalk with immune therapy in cancer treatment. Theranostics (2021) 11(18):8813–35. doi: 10.7150/thno.62521
92. Kesavardhana S, Malireddi RKS, Kanneganti TD. Caspases in cell death, inflammation, and pyroptosis. Annu Rev Immunol (2020) 38:567–95. doi: 10.1146/annurev-immunol-073119-095439
93. Wei T, Zhang C, Song Y. Molecular mechanisms and roles of pyroptosis in acute lung injury. Chin Med J (2022) 135(20):2417–26. doi: 10.1097/cm9.0000000000002425
94. Yap JKY, Moriyama M, Iwasaki A. Inflammasomes and pyroptosis as therapeutic targets for COVID-19. J Immunol (2020) 205(2):307–12. doi: 10.4049/jimmunol.2000513
95. Li D, Ren W, Jiang Z, Zhu L. Regulation of the NLRP3 inflammasome and macrophage pyroptosis by the p38 MAPK signaling pathway in a mouse model of acute lung injury. Mol Med Rep (2018) 18(5):4399–409. doi: 10.3892/mmr.2018.9427
96. Jin Y, Sun M, Jiang X, Zhang Q, Feng D, Wen Z. Extracellular histones aggravate acute respiratory distress syndrome by inducing peripheral blood mononuclear cells pyroptosis. Zhonghua Wei Zhong Bing Ji Jiu Yi Xue (2019) 31(11):1357–62. doi: 10.3760/cma.j.issn.2095-4352.2019.11.009
97. Chen X, Wu R, Li L, Zeng Y, Chen J, Wei M, et al. Pregnancy-induced changes to the gut microbiota drive macrophage pyroptosis and exacerbate septic inflammation. Immunity (2023) 56(2):336–52.e9. doi: 10.1016/j.immuni.2023.01.015
98. Zheng Y, Huang Y, Xu Y, Sang L, Liu X, Li Y. Ferroptosis, pyroptosis and necroptosis in acute respiratory distress syndrome. Cell Death Discovery (2023) 9(1):91. doi: 10.1038/s41420-023-01369-2
99. Li J, Lu K, Sun F, Tan S, Zhang X, Sheng W, et al. Panaxydol attenuates ferroptosis against LPS-induced acute lung injury in mice by Keap1-Nrf2/HO-1 pathway. J Transl Med (2021) 19(1):96. doi: 10.1186/s12967-021-02745-1
100. Wang Y, Chen D, Xie H, Jia M, Sun X, Peng F, et al. AUF1 protects against ferroptosis to alleviate sepsis-induced acute lung injury by regulating NRF2 and ATF3. Cell Mol Life Sci (2022) 79(5):228. doi: 10.1007/s00018-022-04248-8
101. Wang J, Zhu N, Su X, Gao Y, Yang R. Gut-microbiota-derived metabolites maintain gut and systemic immune homeostasis. Cells (2023) 12(5):793. doi: 10.3390/cells12050793
102. Tan JK, Macia L, Mackay CR. Dietary fiber and SCFAs in the regulation of mucosal immunity. J Allergy Clin Immunol (2023) 151(2):361–70. doi: 10.1016/j.jaci.2022.11.007
103. NK K, Patil P, Bhandary SK, Haridas V, N SK, E S, et al. Is butyrate a natural alternative to dexamethasone in the management of CoVID-19? F1000Res (2021) 10:273. doi: 10.12688/f1000research.51786.1
104. Liang L, Liu L, Zhou W, Yang C, Mai G, Li H, et al. Gut microbiota-derived butyrate regulates gut mucus barrier repair by activating the macrophage/WNT/ERK signaling pathway. Clin Sci (Lond) (2022) 136(4):291–307. doi: 10.1042/cs20210778
105. Sultan M, Wilson K, Abdulla OA, Busbee PB, Hall A, Carter T, et al. Endocannabinoid anandamide attenuates acute respiratory distress syndrome through modulation of microbiome in the gut-lung axis. Cells (2021) 10(12):3305. doi: 10.3390/cells10123305
106. Hashimoto Y, Eguchi A, Wei Y, Shinno-Hashimoto H, Fujita Y, Ishima T, et al. Antibiotic-induced microbiome depletion improves LPS-induced acute lung injury via gut-lung axis. Life Sci (2022) 307:120885. doi: 10.1016/j.lfs.2022.120885
107. Negatu DA, Liu JJJ, Zimmerman M, Kaya F, Dartois V, Aldrich CC, et al. Whole-cell screen of fragment library identifies gut microbiota metabolite indole propionic acid as antitubercular. Antimicrob Agents Chemother (2018) 62(3):e01571–17. doi: 10.1128/aac.01571-17
108. Li N, Dai Z, Wang Z, Deng Z, Zhang J, Pu J, et al. Gut microbiota dysbiosis contributes to the development of chronic obstructive pulmonary disease. Respir Res (2021) 22(1):274. doi: 10.1186/s12931-021-01872-z
109. Mohammed A, Alghetaa HK, Zhou J, Chatterjee S, Nagarkatti P, Nagarkatti M. Protective effects of Δ(9) -tetrahydrocannabinol against enterotoxin-induced acute respiratory distress syndrome are mediated by modulation of microbiota. Br J Pharmacol (2020) 177(22):5078–95. doi: 10.1111/bph.15226
110. Zhang Y, Zhang L, Mao L, Fan J, Jiang X, Li N, et al. Intestinal microbiota-derived propionic acid protects against zinc oxide nanoparticle-induced lung injury. Am J Respir Cell Mol Biol (2022) 67(6):680–94. doi: 10.1165/rcmb.2021-0515OC
111. Tingting ZHANG, Xiaoqin XIANG, Jiangshan ZHONG, Xuemei GUI, Xianming F. Effects of sodium propionate on oxidative stress and inflammation in LPS induced acute lung injury rats. Acad J Chin PLA Med School (2022) 43(3):6. doi: 10.1117.R.20220324.1749.014
112. Park HY, Kim SW, Seo J, Jung YP, Kim H, Kim AJ, et al. Dietary arginine and citrulline supplements for cardiovascular health and athletic performance: A narrative review. Nutrients (2023) 15(5):1268. doi: 10.3390/nu15051268
113. Pontes-Arruda A, Demichele S, Seth A, Singer P. The use of an inflammation-modulating diet in patients with acute lung injury or acute respiratory distress syndrome: A meta-analysis of outcome data. JPEN J Parenter Enteral Nutr (2008) 32(6):596–605. doi: 10.1177/0148607108324203
114. Battaglini D, Robba C, Fedele A, Trancǎ S, Sukkar SG, Di Pilato V, et al. The role of dysbiosis in critically ill patients with COVID-19 and acute respiratory distress syndrome. Front Med (Lausanne) (2021) 8:671714. doi: 10.3389/fmed.2021.671714
115. Jiang L, Guo P, Ju J, Zhu X, Wu S, Dai J. Inhalation of L-arginine-modified liposomes targeting M1 macrophages to enhance curcumin therapeutic efficacy in ALI. Eur J Pharm Biopharm (2023) 182:21–31. doi: 10.1016/j.ejpb.2022.11.017
116. Iyer R, Bansal A. What do we know about optimal nutritional strategies in children with pediatric acute respiratory distress syndrome? Ann Transl Med (2019) 7(19):510. doi: 10.21037/atm.2019.08.25
117. Oliveira GP, de Abreu MG, Pelosi P, Rocco PR. Exogenous glutamine in respiratory diseases: Myth or reality? Nutrients (2016) 8(2):76. doi: 10.3390/nu8020076
118. Harnisch LO, Mihaylov D, Bein T, Apfelbacher C, Kiehntopf M, Bauer M, et al. Determination of individual bile acids in acute respiratory distress syndrome reveals a specific pattern of primary and secondary bile acids and a shift to the acidic pathway as an adaptive response to the critical condition. Clin Chem Lab Med (2022) 60(6):891–900. doi: 10.1515/cclm-2021-1176
119. Kang JD, Myers CJ, Harris SC, Kakiyama G, Lee IK, Yun BS, et al. Bile acid 7α-dehydroxylating gut bacteria secrete antibiotics that inhibit clostridium difficile: Role of secondary bile acids. Cell Chem Biol (2019) 26(1):27–34.e4. doi: 10.1016/j.chembiol.2018.10.003
120. Littlewood-Evans A, Sarret S, Apfel V, Loesle P, Dawson J, Zhang J, et al. GPR91 senses extracellular succinate released from inflammatory macrophages and exacerbates rheumatoid arthritis. J Exp Med (2016) 213(9):1655–62. doi: 10.1084/jem.20160061
121. Murphy MP, O’Neill LAJ. Krebs cycle reimagined: The emerging roles of succinate and itaconate as signal transducers. Cell (2018) 174(4):780–4. doi: 10.1016/j.cell.2018.07.030
122. Tannahill GM, Curtis AM, Adamik J, Palsson-McDermott EM, McGettrick AF, Goel G, et al. Succinate is an inflammatory signal that induces IL-1β through HIF-1α. Nature (2013) 496(7444):238–42. doi: 10.1038/nature11986
123. Wang YH, Yan ZZ, Luo SD, Hu JJ, Wu M, Zhao J, et al. Gut microbiota-derived succinate aggravates acute lung injury after intestinal ischaemia/reperfusion in mice. Eur Respir J (2023) 61(2):2200840. doi: 10.1183/13993003.00840-2022
124. Su X, Gao Y, Yang R. Gut microbiota-derived tryptophan metabolites maintain gut and systemic homeostasis. Cells (2022) 11(15):2296. doi: 10.3390/cells11152296
125. Elmassry MM, Mudaliar NS, Colmer-Hamood JA, San Francisco MJ, Griswold JA, Dissanaike S, et al. New markers for sepsis caused by Pseudomonas aeruginosa during burn infection. Metabolomics (2020) 16(3):40. doi: 10.1007/s11306-020-01658-2
126. Gao J, Xu K, Liu H, Liu G, Bai M, Peng C, et al. Impact of the gut microbiota on intestinal immunity mediated by tryptophan metabolism. Front Cell Infection Microbiol (2018) 8:13. doi: 10.3389/fcimb.2018.00013
127. Zhao S, Feng P, Meng W, Jin W, Li X, Li X. Modulated gut microbiota for potential COVID-19 prevention and treatment. Front Med (Lausanne) (2022) 9:811176. doi: 10.3389/fmed.2022.811176
128. Lorente JA, Nin N, Villa P, Vasco D, Miguel-Coello AB, Rodriguez I, et al. Metabolomic diferences between COVID-19 and H1N1 influenza induced ARDS. Crit Care (2021) 25(1):390. doi: 10.1186/s13054-021-03810-3
129. Bernhard W. Choline in cystic fibrosis: relations to pancreas insufficiency, enterohepatic cycle, PEMT and intestinal microbiota. Eur J Nutr (2021) 60(4):1737–59. doi: 10.1007/s00394-020-02358-2
130. van Waardenburg DA, de Betue CT, Luiking YC, Engel M, Deutz NE. Plasma arginine and citrulline concentrations in critically ill children: strong relation with inflammation. Am J Clin Nutr (2007) 86(5):1438–44. doi: 10.1093/ajcn/86.5.1438
131. Reizine F, LesouHaitier M, Gregoire M, Pinceaux K, Gacouin A, Maamar A, et al. SARS-CoV-2-induced ARDS associates with MDSC expansion, lymphocyte dysfunction, and arginine shortage. J Clin Immunol (2021) 41(3):515–25. doi: 10.1007/s10875-020-00920-5
132. Li T, Chiang JYL. Bile acids as metabolic regulators: an update. Curr Opin Gastroenterol (2023) 39(3):249–55. doi: 10.1097/mog.0000000000000934
133. Urdaneta V, Casadesús J. Interactions between bacteria and bile salts in the gastrointestinal and hepatobiliary tracts. Front Med (Lausanne) (2017) 4:163. doi: 10.3389/fmed.2017.00163
134. Harnisch LO, Mihaylov D, Bein T, Apfelbacher C, Moerer O, Quintel M. A reduced glycine-to-taurine ratio of conjugated serum bile acids signifies an adaptive mechanism and is an early marker of outcome in acute respiratory distress syndrome. Intern Emerg Med (2023) 18(2):607–15. doi: 10.1007/s11739-022-03152-0
135. Kean IRL, Wagner J, Wijeyesekera A, De Goffau M, Thurston S, Clark JA, et al. Profiling gut microbiota and bile acid metabolism in critically ill children. Sci Rep (2022) 12(1):10432. doi: 10.1038/s41598-022-13640-0
136. Chouchani ET, Pell VR, Gaude E, Aksentijević D, Sundier SY, Robb EL, et al. Ischaemic accumulation of succinate controls reperfusion injury through mitochondrial ROS. Nature (2014) 515(7527):431–5. doi: 10.1038/nature13909
137. Rothhammer V, Mascanfroni ID, Bunse L, Takenaka MC, Kenison JE, Mayo L, et al. Type I interferons and microbial metabolites of tryptophan modulate astrocyte activity and central nervous system inflammation via the aryl hydrocarbon receptor. Nat Med (2016) 22(6):586–97. doi: 10.1038/nm.4106
138. Tsoyi K, Liang X, De Rossi G, Ryter SW, Xiong K, Chu SG, et al. CD148 deficiency in fibroblasts promotes the development of pulmonary fibrosis. Am J Respir Crit Care Med (2021) 204(3):312–25. doi: 10.1164/rccm.202008-3100oc
Keywords: acute respiratory distress syndrome (ARDS), gut microbiota, metabolic products, gut-lung, axis
Citation: Zhang D-W, Lu J-L, Dong B-Y, Fang M-Y, Xiong X, Qin X-J and Fan X-M (2024) Gut microbiota and its metabolic products in acute respiratory distress syndrome. Front. Immunol. 15:1330021. doi: 10.3389/fimmu.2024.1330021
Received: 30 October 2023; Accepted: 30 January 2024;
Published: 16 February 2024.
Edited by:
Susanta Pahari, Texas Biomedical Research Institute, United StatesReviewed by:
Tomasz Piotr Wypych, Polish Academy of Sciences, PolandAgata Mulak, Wroclaw Medical University, Poland
Copyright © 2024 Zhang, Lu, Dong, Fang, Xiong, Qin and Fan. This is an open-access article distributed under the terms of the Creative Commons Attribution License (CC BY). The use, distribution or reproduction in other forums is permitted, provided the original author(s) and the copyright owner(s) are credited and that the original publication in this journal is cited, in accordance with accepted academic practice. No use, distribution or reproduction is permitted which does not comply with these terms.
*Correspondence: Xian-Ming Fan, ZnhtMTI5QDE2My5jb20=; Xue-Jun Qin, MjgzNjI4Nzg5QHFxLmNvbQ==
†These authors have contributed equally to this work