- 1IRCCS Humanitas Research Hospital, Milan, Italy
- 2Department of Biomedical Sciences, Humanitas University, Milan, Italy
The tumor microenvironment is composed of tumor cells, stromal cells and leukocytes, including innate and adaptive immune cells, and represents an ecological niche that regulates tumor development and progression. In general, inflammatory cells are considered to contribute to tumor progression through various mechanisms, including the formation of an immunosuppressive microenvironment. Macrophages and neutrophils are important components of the tumor microenvironment and can act as a double-edged sword, promoting or inhibiting the development of the tumor. Targeting of the immune system is emerging as an important therapeutic strategy for cancer patients. However, the efficacy of the various immunotherapies available is still limited. Given the crucial importance of the crosstalk between macrophages and neutrophils and other immune cells in the formation of the anti-tumor immune response, targeting these interactions may represent a promising therapeutic approach against cancer. Here we will review the current knowledge of the role played by macrophages and neutrophils in cancer, focusing on their interaction with other immune cells.
Introduction
To develop and grow, tumor cells require constant support from cells of the surrounding environment (1–3). Immune cells are key players in this scene where they actively collaborate to either promote or inhibit tumor growth (4, 5). Through the production of numerous immunomodulatory molecules (e.g. cytokines, chemokines and growth factors), tumor cells can modulate the phenotype of immune cells and the positive or negative influence that they exert on the tumor microenvironment (TME) (6, 7). Additionally, tumor-infiltrated immune cells are engaged in a number of mutual interactions which further potentiate or reduce their pro-tumor or anti-tumor activities (8–10). Tumor-associated macrophages (TAMs) have long been identified as central players of this complex intercellular network (11–13). More recently, neutrophils, traditionally considered only as short-lived front-line fighters against pathogens, have received increasing attention due to their important role in regulating tumor development (14–17).
Being highly plastic and consequently heterogeneous, TAMs and tumor associated neutrophils (TANs) adapt their phenotype and activation state to the surrounding environment (11–21). TAMs and TANs have the capacity to act directly on tumor cells to promote or inhibit their proliferation, and to modulate the anti-tumor or pro-tumor activities of other immune cells (11, 14, 22). Targeting the immune system is now a reality and is emerging as an important therapeutic approach for the treatment of cancer (23, 24). However, a significant percentage of patients do not respond to current available treatments (25). Given the crucial importance of the intercellular crosstalk between immune cells within the TME, novel therapeutic approaches targeting these interactions might be beneficial. In this review, after a short overview concerning TAMs and TANs, we will focus on their complex intercellular interactions with other immune cells, with a particular emphasis on how these interactions can influence tumor development and progression.
Macrophages in the tumor microenvironment
Macrophages are large phagocytic cells of the innate immune system and are found in tissues where they play numerous roles, including defense against invading pathogens and maintenance of tissue homeostasis (19). Macrophages have long been identified as tumor-infiltrating cells and as one of the key regulators of the immune response within the TME (11–13). In a majority of human cancers, a high level of macrophage infiltration has been associated with a poor prognosis, including in gastric cancer, urogenital and head neck cancers, pancreatic ductal adenocarcinoma and breast carcinoma with some notable exceptions such as colorectal cancer and ovarian cancer (26–33).
The majority of TAMs derive from circulating bone marrow (BM)-derived monocytes that migrate into the tumor bed mainly under the influence of chemokines (e.g. C-C motif ligand 2 (CCL2)), cytokine colony stimulating factor 1 (CSF-1), or complement components (26, 34–39). In addition, numerous studies have shown that another important source of TAMs was represented by tissue-resident macrophages (TRMs), which derive from embryonic precursors and are maintained locally (40–45). Interestingly, a difference in origin can influence the function of TAMs within the TME (46, 47).
In addition to their heterogenous origin, TAMs have a high degree of plasticity and constantly adapt in response to the microenvironment further increasing their heterogeneity within the TME (11, 19, 48, 49). Historically, TAMs have been classified into two major polarization states, generally referred to as M1 (or classically activated) and M2 (or alternatively activated) (50–52). M1 polarization can be induced by bacterial products and interferon-γ (IFN-γ) and has been associated with tissue damage and anti-tumor activities (50, 51). In contrast, M2 polarization, induced by cytokines of the type 2 immune response, such as interleukin (IL)-13 and IL-4, has been associated with tissue repair and tumor-promotion (50, 51, 53). Although this dichotomous classification is still commonly used, it is nowadays fully recognized that it is too reductive and does not reflect the extraordinary complexity and heterogeneity of the different phenotypes and activation states of TAMs (13, 21, 52). Novel technologies, including single-cell RNA sequencing and spatial transcriptomic analyses, have led to a better understanding of macrophage complexity and heterogeneity in different tumor contexts. In addition, these technologies have facilitated insight into the localization of macrophages and their interactions with other cells within the TME (54–61).
Macrophages and immune cells cross-talk in the TME
Macrophages and lymphoid cells
The existence of a crosstalk between macrophages and lymphoid cells within the TME has been clearly defined and extensively studied. In various human cancers, TAMs were found in close proximity to different lymphoid cells, including natural killer (NK) cells and T cells (62–64). The final effect of the interaction between macrophages and lymphoid cells can differ depending on the type and the stage of tumors, the presence of immunomodulatory molecules and the location of TAMs within the TME.
A large body of evidence has shown that TAMs can inhibit the anti-tumor activity of lymphoid cells, thereby promoting cancer progression. As a matter of fact, the presence of monocyte/macrophage infiltration was found to be inversely correlated with the presence and activation of NK cells in different human cancers including lung cancer, gastric cancer and hepatocellular carcinoma (HCC) (64–66). In contrast, monocyte/macrophage infiltration was found positively correlated with the presence of regulatory CD4+ T lymphocytes (T-reg) in prostate cancer and colorectal cancer (67, 68). Consistently, CSF-1– CSF-1 receptor (CSF-1R) axis blockade or macrophage recruitment blockade via C-C chemokine receptor type 2 (CCR2)-inhibition could restore immune response against tumor in cancer models (69, 70).
The mechanisms by which TAMs can inhibit the anti-tumor activities of lymphoid cells are numerous ranging from the release of soluble inhibitory mediators and cell-cell contact to the modulation of their recruitment or their exclusion from the tumor bed (Figure 1). The release of tumor growth factor-β (TGF-β) by TAMs can directly inhibits T cell and NK cell effector functions (64, 71–74). In addition, macrophage-derived TGF-β can promote the generation of T-reg lymphocytes via the induction of SMAD3-mediated FOXP3 expression and the upregulation of PD-1 (68, 75). TGF-β availability within the TME can be further amplified by metalloproteinase 9 (MMP-9) secreted by macrophages, which can cleave and activate latent TGF-β present in the extracellular matrix (ECM) (76).
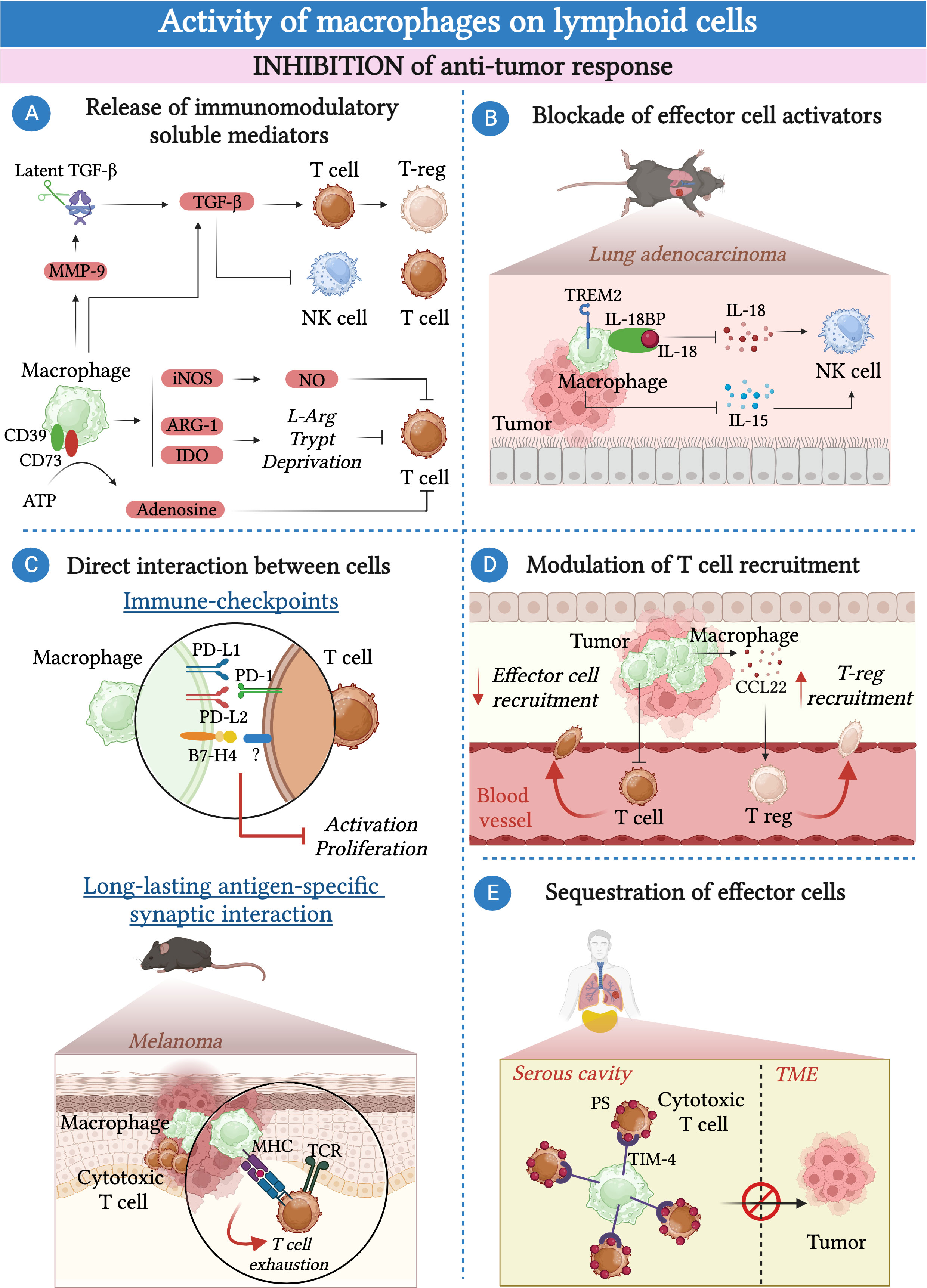
Figure 1 Macrophages inhibit the anti-tumor response of lymphoid cells. (A) Macrophages secrete a plethora of soluble molecules and immunomodulatory enzymes that inhibit effector cell functions including tumor growth factor β (TGF-β), inducible nitric oxide synthase (iNOS), indoleamine-2,3-dioxygenase (IDO) and arginase-1 (Arg-1). In addition, they produce metalloproteinase 9 (MMP-9) that induces the activation of the latent TGF-β present in the extracellular matrix (ECM). TAMs express CD39 and CD73, which convert the pro-inflammatory ATP present in the TME into the immunosuppressive adenosine. (B) In the context of lung carcinoma, triggering receptor expressed on myeloid cells 2 (TREM2)-expressing macrophages suppress the cytokine-mediated activation of NK-cells via the production of IL-18-binding protein (IL-18BP) and by limiting IL-15 production by dendritic cells. (C) Macrophages can inhibit the activation of effector cells in a contact-dependent manner. They express immune checkpoints such as programmed cell death ligand 1 (PD-L1), PD-L2 and B7-H4 that, by binding to their ligands, impair the activity of T cells and NK cells. In a mouse model of melanoma, the formation of an antigen-specific interaction between macrophages and T cells leads to T cell exhaustion. (D) Macrophages can inhibit the recruitment of effector T cells into the TME whereas they favor the recruitment of T-reg lymphocytes via the secretion of CCL22. (E) In pleural and peritoneal body cavities, macrophages expressing T-cell immunoglobulin and mucin domain containing 4 (TIM-4) can sequester CD8+ cytotoxic T cells away from the tumor by binding to phosphatidylserine (PS) expressed on their surface and inhibiting their proliferation.
TAMs can release the immunoregulatory enzymes Arginase-1 (Arg-1) and indoleamine 2,3-dioxygenase (IDO) that, by catalyzing the degradation of L-arginine (L-arg) and L-tryptophan respectively, deprive T cells of essential nutrients, leading to their functional impairment (77–79). A third important immunomodulatory enzyme produced by macrophages is the inducible nitric oxide synthase (iNOS), which catalyzes the production of nitric oxide (NO). In turn, NO has a direct inhibitory effect on T cell proliferation, as well as an indirect effect due to the secondary production of peroxynitrites able to impair the interaction of the major histocompatibility complex (MHC) with the T cell receptor (TCR) via nitration of tyrosines in the TCR-CD8 complex (80–82).
TAMs can exert their pro-tumor activity by blocking the activity of soluble factors that normally contribute to the activation of lymphoid cells. For instance, TAMs can express CD39 and CD73, which are essential in converting the pro-inflammatory adenosine triphosphate (ATP) present in the TME into immunosuppressive adenosine (83). In glioblastoma, kynurenine produced by cancer cells upregulates the expression of hydrocarbon receptor (AHR) in TAMs. In turn, AHR favors the expression of the ectonucleotidase CD39, which, in collaboration with CD73, leads to the dysfunction of CD8+ T cells via the production of adenosine (84, 85).
In a murine model of lung adenocarcinoma, efferocytosis of apoptotic cancer cell by macrophages induced a pro-tumorigenic program controlled by triggering receptor expressed on myeloid cells 2 (TREM2). In particular, TREM2+ macrophages can prevent the recruitment and activation of NK cells by blocking the activities of IL-18 and IL-15 through the production of IL-18 binding protein and by inhibiting the production of IL-15 by tumor-infiltrating dendritic cells (65).
The mechanism by which macrophages can dampen the anti-tumor response goes beyond the secretion of molecules or the interference with soluble mediators and often requires direct cell-cell contact.
TAMs have been found to express high levels of immune checkpoint molecules, including programmed cell death ligand 1 (PD-L1), PD-L2, V-domain Ig suppressor of T cell activation (VISTA) and B7-H4 (11, 86–93). The interaction of these immune checkpoints with their ligands expressed on T cells results in the suppression of the adaptive T cell immune response (94). Consistently, immune checkpoint blockade can restore T cell-mediated anti-tumor immune response (89, 91). In addition to T cells, PD-1, the ligand of PD-L1, can be expressed by a subset of fully mature NK cells, suggesting that NK cells can also serve as a target of PD-L1-mediated inhibition by macrophages (95).
In addition to the expression of immune checkpoints, other mechanisms involving cell-cell contact between macrophages and T cells have been identified and may lead to T cell exhaustion. For instance, TAMs and CD8+ T cells can be engaged in a long-lasting antigen-specific synaptic interaction. This interaction causes only weak stimulation of the TCR, which is insufficient to activate T cells and instead leads to their exhaustion (96).
Furthermore, different lines of evidence suggested the involvement of TAMs in modulating the recruitment of lymphoid cells within the TME. In cervical and breast cancer models, CSF-1R-blockade has been shown to enhance CD8+ T cell infiltration (97). Consistently, LIF-mediated epigenetic silencing of CXCL9, one of the most important chemokines for CD8+ T cells, in macrophages resulted in decreased infiltration of CD8+ T cells into the tumor (98, 99). While they can reduce the recruitment of effector CD8+ T cells in the TME, macrophages have been suggested to facilitate the recruitment of T-reg lymphocytes via the secretion of CCL22 in human ovarian cancer (100). In a mouse model of melanoma, intra-tumoral administration of an anti-CCL22 antibody reduced the recruitment of T-reg lymphocytes and inhibited tumor growth (101).
Macrophages have been implicated in the sequestration of effector T cells away from the tumor bed. Recently, a study showed that serous cavity-resident macrophages expressed high levels of T-cell immunoglobulin and mucin domain containing 4 (TIM-4), a receptor for phosphatidylserine (PS). The interaction between TIM-4 and PS, highly expressed on cytotoxic CD8+ T cells, resulted in the sequestration of CD8+ T cells away from the tumor and inhibited their proliferation (102). Similarly, in samples of human non-small cell lung carcinoma (NSLC),TAMs reduced the motility of the CD8+ T cells present in the stroma surrounding the tumor, limiting their entry into the tumor bed (62).
Although in a large number of studies, TAMs appeared to reduce the anti-tumor activity of lymphoid cells, some works have suggested the existence of macrophages with an opposite function in different tumors (Figure 2). Recently, a study identified a discrete population of human tissue-resident FOL2R+ macrophages present in healthy mammary gland and breast cancer primary tumors endowed with an anti-tumor function. This population of macrophages was localized in the perivascular areas of the tumor stroma where they interacted with CD8+ T cells and promoted their activation (103). Of note, the presence of FOLR2+ macrophages has been associated with increased survival in breast cancer patients (103). In addition to T cells, macrophages have the potential to support the anti-tumor activity of NK cells either via the release of soluble molecules or via direct contact. For instance, in a model of mammary tumor, TAMs expressing the lipid transporter epidermal fatty acid binding proteins-(E-FABP) have been described to have an anti-tumor activity through the activation of NK cells. Specifically, the expression of E-FABP promoted the formation of lipid droplets in TAMs, leading to increased IFN-β production, which, in turn, favored the recruitment of effector cells, particularly NK cells (104). Remarkably, the same study showed that E-FABP was highly expressed in TAMs from women with early-stage disease, and that this expression decreased with disease progression (104). In in vitro co-culture experiments, M1-macrophages induced an IL-23 and IFN-β-dependent upregulation of natural killer group 2D (NKG2D) expression, an IL-1β-dependent upregulation of NKp44 expression, and sustained the production of IFN-γ by NK cells via the release of IFN-β and the engagement of the 2B4-CD48 pathway (105). Similarly, M0 and M2 macrophages reprogrammed to M1 via in vitro LPS stimulation can promote the cytotoxic activity of NK cells via a contact-dependent mechanism and drive the production of IFN-γ by NK cells via the interaction between DNAX accessory molecule-1 (DNAM-1) and 2B4 and the production of IL-18 (73, 106).
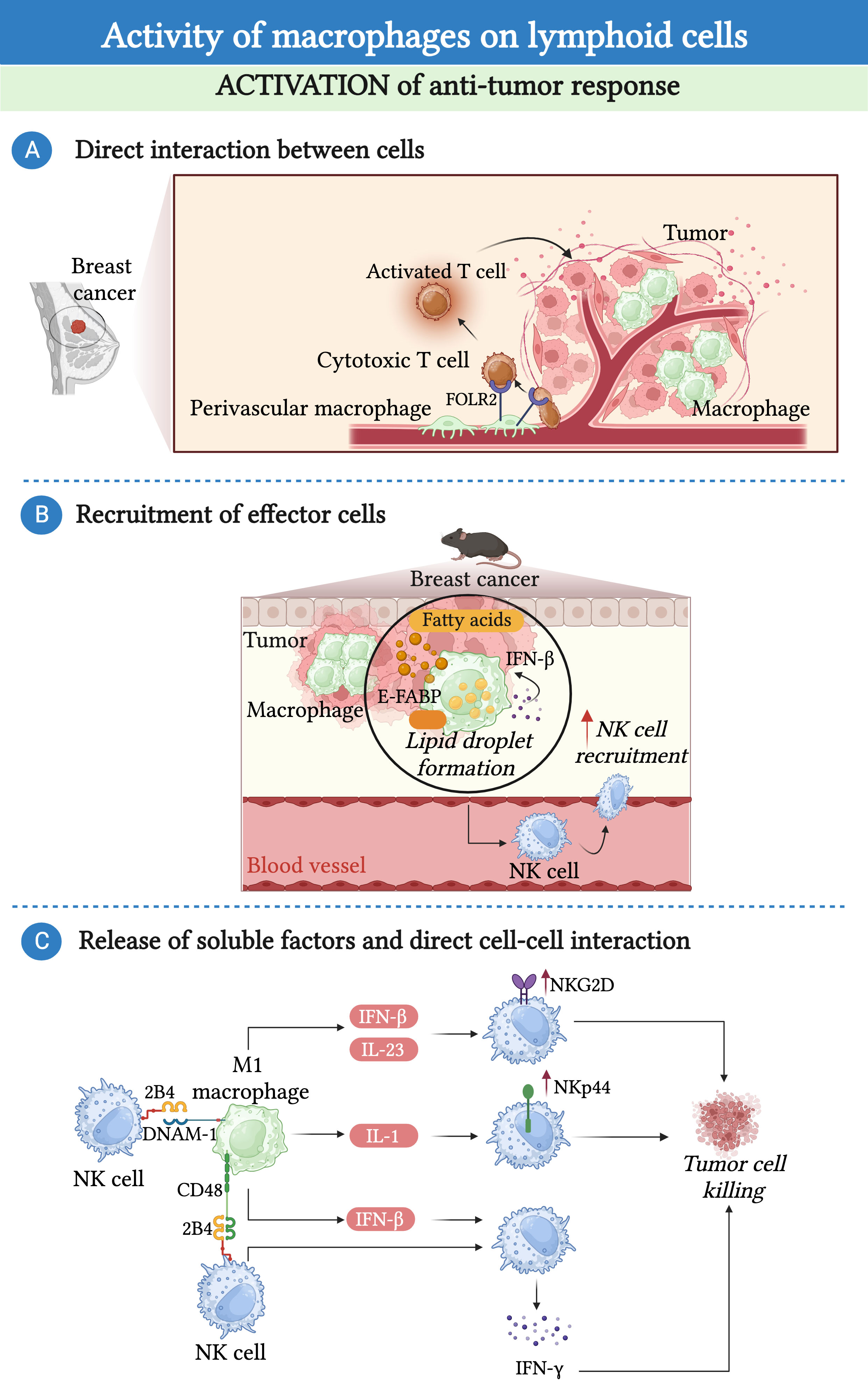
Figure 2 Macrophages promote the anti-tumor response of lymphoid cells. (A) In the context of breast cancer, a population of folate receptor 2 (FOLR2)-expressing macrophages endowed with anti-tumor properties can establish a prolonged interaction with cytotoxic T cells facilitating their recruitment and activation. (B) In a mouse model of mammary tumor, accumulation of lipid droplets in epidermal fatty acid binding proteins (E-FABP)-expressing TAMs induces the production of interferon-β (IFN-β), leading to the recruitment and activation of NK-cells. (C) Macrophages have the potential to support the anti-tumor activity of NK cells. M1-macrophages induce an IL-23 and IFN-β-dependent upregulation of NKG2D expression, an IL-1β-dependent upregulation of NKp44 expression, and sustained the production of IFN-γ by NK cells via the release of IFN-β and the interaction of CD48 and DNAX accessory molecule-1 (DNAM-1) with 2B4.
In addition to T cells and NK cells, some evidence suggested that macrophages could promote B cell proliferation via the release of B cell-activating factor (BAFF) or IL-6 (8, 107, 108). However, the relevance of this interaction within the TME remains to be clarified.
The interaction between macrophages and lymphoid cells within the TME is mutual and the phenotype and function of macrophages can also be affected by lymphoid cells (12, 109). For instance, the IFN-γ produced by T-helper (Th)-1 cells, NK cells and cytotoxic CD8+ T cells increases the presentation of antigens by macrophages, the production of pro-inflammatory cytokines and the cytotoxic activity of macrophages against tumor cells (48, 109, 110). In contrast, lymphoid cells found in the TME can also favor a pro-tumor phenotype of macrophages. For instance, CD4+ Th-2 cells, innate lymphoid cells (ILCs)-2- and T-reg lymphocytes that produce IL-4, IL-13 and IL-10 can sustain the formation of M2-like macrophages with a pro-tumor phenotype (111–115). Interestingly, it has been shown that mouse T-reg lymphocytes can indirectly promote the survival of M2-like pro-tumor macrophages. Indeed, by limiting the production of IFN-γ by CD8+ T cells, T- reg lymphocytes prevent the inhibition of sterol regulatory element binding protein 1 (SREBP1)-mediated fatty acid synthesis, which is crucial for the survival of M2-like macrophages (116).
Additionally, NK cells have been shown to be able to kill macrophages. Of note, this macrophage killing activity was found to be especially efficient toward M0 and M2 macrophage subtypes whereas M1 macrophages were more resistant to lysis due to the higher expression of human leukocytes antigen (HLA) class I molecules (106). Similarly, invariant natural killer T cells (iNKT) have been shown to exert anti-tumor activity by killing macrophages in a CD1d-dependent mechanism (117).
Finally, some studies have highlighted an effect of B cells on macrophages in tumor. In a mouse model of melanoma, adoptive transfer of a subtype of B cells can induce M2-like polarization of TAMs (118).
Macrophages and myeloid cells
While the interaction between macrophages and lymphoid cells has been the subject of numerous studies, their interaction with other myeloid cells has received less attention. Among myeloid cells, dendritic cells are key players in the orchestration of both innate and adaptive immune responses in cancer (119).TAMs produce high levels of vascular endothelial growth factor (VEGF), IL-10, IL-6 and TGF-β which are described to inhibit the activity of dendritic cells (11, 120–124). Besides dendritic cells, macrophages can interact with neutrophils (14). The interaction between these two important myeloid subtypes within the TME will be further discussed in this review.
Neutrophils in the tumor microenvironment
Neutrophils are the most abundant circulating leukocytes in humans. They have long been considered as simple first-line fighters against invading pathogens, but are now recognized as central players in the regulation of tumor development and progression (14, 16). TANs have been found in the TME of several human cancers, including renal cell carcinoma, hepatocellular carcinoma, lung cancer, melanoma, head and neck cancer, glioma, colorectal cancer, sarcomas, pancreatic cancer, breast cancer, gastric cancer, urothelial carcinoma and ovarian cancer (125–136). However, their role is still controversial and may depend on a number of factors, including the different types of cancer, the stage of development and the presence of other cells (14, 17, 137). While in a large number of studies, a high level of TANs has been associated with a poor prognosis for patients, in others, including colorectal cancer and undifferentiated pleomorphic sarcoma (UPS), it has been associated with a better outcome (14, 125, 130, 138–143).
Mature mouse and human neutrophils are constantly released from the BM where they differentiate and mature from progenitors in response to growth factors, in particular granulocyte-colony stimulating factor (G-CSF) and granulocyte macrophage-colony stimulating factor (GM-CSF) (16, 144–149). The process of neutrophil mobilization from the BM has been extensively investigated in mice and is highly dependent on the regulation of the expression of genes coding for CXCR4 and CXCR2 (150). Upon maturation, BM-neutrophils downregulate CXCR4 expression, which is the receptor for CXCL12 produced by BM stromal cells, and increase the expression of CXCR2. The expression of CXCR2 and the presence CXCL2, which is the ligand for CXCR2, in the circulation trigger the release of neutrophils into the peripheral blood (150, 151). The observation of alterations in neutrophil biology among patients with genetic mutations in CXCR4 and CXCR2 implies the significance of these molecules also in human (152, 153). Stress conditions, including cancer, trigger an “emergency granulopoiesis” program during which the process of neutrophil maturation and BM egress is altered, resulting in the release of immature neutrophils into the circulation (154).
Circulating neutrophils express high levels of CXCR1 and CXCR2, which play a major role in their recruitment into the TME (14, 16, 155). Numerous studies have shown the involvement of CXCR1 and CXCR2 ligands, including CXCL1, CXCL2, CXCL5, CXCL6 and CXCL8 (only for humans) in the recruitment of neutrophils into the TME (14, 156–162). Additionally, other inflammatory mediators, including the cytokines TNF-α, IL-17 and IL-1β have been implicated in the recruitment of neutrophils into the TME (14, 163, 164).
While neutrophils were traditionally considered as short-lived effector cells with limited plasticity, a large body of evidence challenged this view and recognized their considerable plasticity and heterogeneity (17, 18, 20). Based on their phenotype and function and mirroring the M1/M2 paradigm, TANs have been classified into anti-tumor (N1) and pro-tumor (N2) neutrophils (14, 165, 166). As mentioned above for macrophages, new studies based on state-of-the-art methodology, including single cell RNA sequencing, mass cytometry by time-of-flight (CyTOF), multiplex immunofluorescence and spatial transcriptomic have revealed a high degree of heterogeneity in TANs (14, 16, 167–171).
Pro-tumor neutrophils can directly promote tumor development by inducing tissue damage and genetic instability through the production of radical oxygen species (ROS) and miRNA, and by stimulating tumor growth through the secretion of cytokines and growth factors (172–177). Additionally, pro-tumor neutrophils can facilitate the formation of tumor metastasis through different mechanisms such as the induction of angiogenesis and ECM remodeling (178–181). In contrast, anti-tumor neutrophils can inhibit tumor growth through the direct killing of tumor cells via the production of ROS and NO or by trogocytosis of antibody-opsonized cancer cells (182, 183).
Besides their direct effect on tumor cells, TANs are engaged in dynamic and continuous interactions with a large variety of tumor infiltrating immune cells, affecting their phenotype and effector functions (14). In turn, these immune cells have a significant impact on neutrophil recruitment, phenotype and function (14, 17).
Neutrophils and immune cells cross-talk in tumor
Neutrophils and lymphoid cells
Neutrophils interact with a variety of lymphoid cells including CD4+ and CD8+ T cells, unconventional T cells, NK cells and B cells. In human samples, neutrophils have often been found co-localized with other lymphoid cells in the tumor bed or in the tumor-draining lymph nodes (139, 184–186).
Neutrophils have the capacity to either inhibit or activate the effector functions of these lymphoid cells (Figure 3) (15).
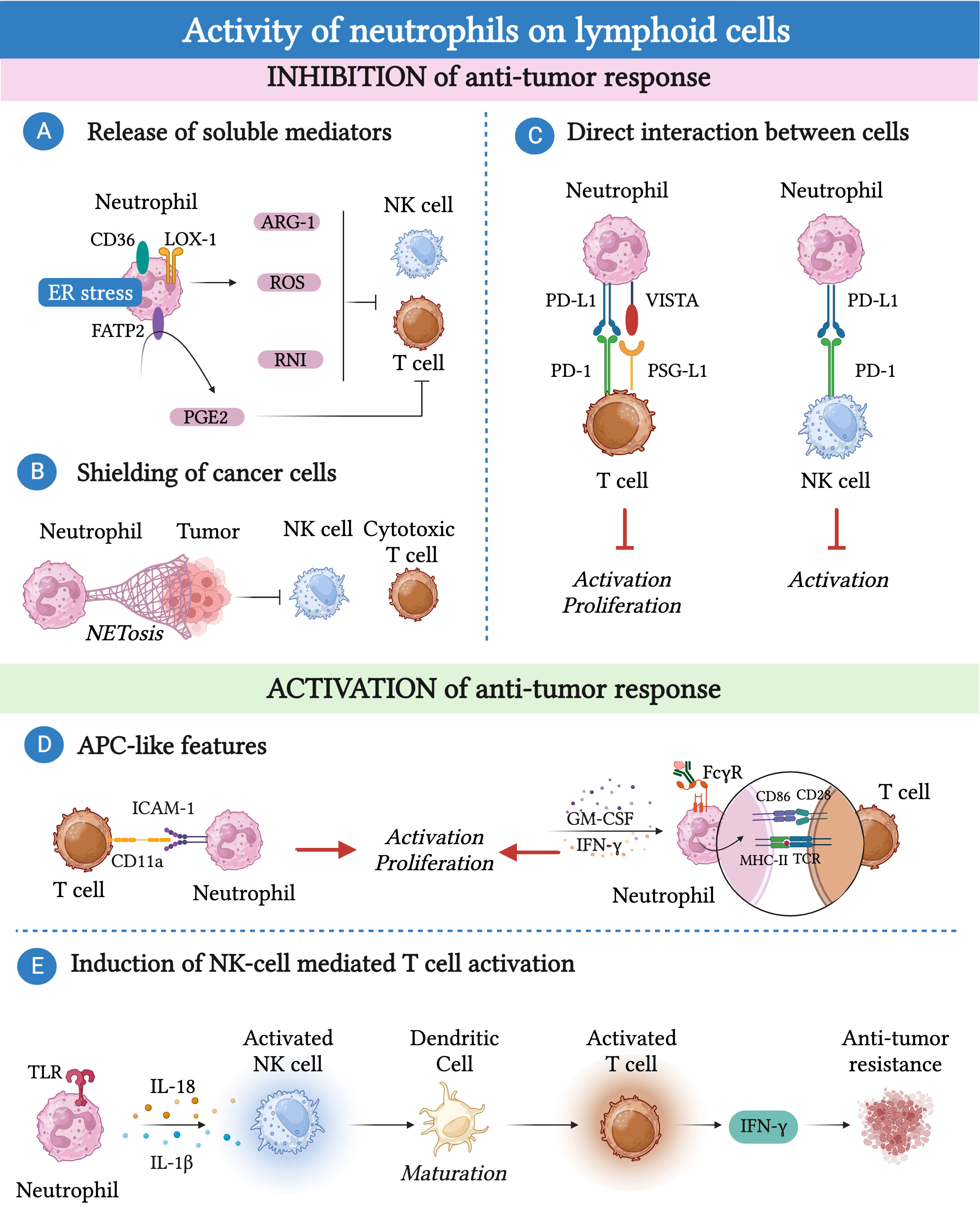
Figure 3 Neutrophils can promote or inhibit the anti-tumor response of lymphoid cells. (A) Immunosuppression can be mediated by neutrophils via the production of reactive oxygen species (ROS), reactive nitrogen intermediate (RNI) and arginase-1 (Arg-1) or through fatty acid transporter protein 2 (FATP2)-dependent production of prostaglandin E2 (PGE2). (B) Neutrophils can indirectly affect the cytotoxic activity of NK cells and CD8+ T cells by releasing neutrophil extracellular traps (NETs) that shield cancer cells. (C) Neutrophils express immune checkpoints such as programmed cell death 1 ligand 1 (PD-L1) or the V-domain immunoglobulin suppressor of T-cell activation (VISTA) that, by binding to their ligands PD-1 and P-selectin glycoprotein ligand-1 (PSG-L1), cause T cell and NK cell dysfunction. (D) Neutrophils can acquire antigen presenting cell-like (APC-like) features under the influence of GM-CSF or interferon (IFN)-γ or upon phagocytosis of antibody-antigen complexes via Fc gamma receptors (FcγRs). Similarly, neutrophils enhance TCR signaling in CD8+ T cells through the interaction between CD54/intercellular adhesion molecule 1 (ICAM-1) expressed on neutrophils and CD11a expressed on T cells. (E) Tool like receptor (TLR)-stimulated neutrophils can attract and activate NK cells which, in turn, trigger the maturation of dendritic cells resulting in T cell proliferation and IFN-γ production.
Several lines of evidence have suggested that human and mouse neutrophil-derived soluble mediators, such as Arg-1, ROS, reactive nitrogen intermediates (RNI) and prostaglandin E2 (PGE2), played a key role in suppressing the effector functions of T cells and NK cells (17, 165, 187–193). In different mouse tumor models, TANs respond to TGF-β by producing significant amounts of Arg-1, leading to a reduction in the availability of L-arginine (165). Given the fundamental role of L-arginine in T cell metabolism, its deprivation results in T cell dysfunction (165). Accordingly, a population of neutrophils expressing Arg-1 has been found in renal cell carcinoma and NSLC patients with a frequency that negatively correlates with the frequency of CD8+ cytotoxic T lymphocytes (191, 192).
Changes in neutrophil metabolism may be linked to their pro-tumor or anti-tumor activities. In a transplantable mouse model of breast cancer with limited glucose supply, c-Kit+ immature neutrophils exhibited increased mitochondrial fatty acid oxidation, resulting in higher production of ROS and inhibition of the T cell response (187). The production of RNI, through iNOS–dependent NO production, was found to hinder T cell activation in mammary tumor-bearing K14Cre; Cdh1F/F; Trp53F/F (KEP) mice (189). Neutrophils found within the TME can exhibit endoplasmic reticulum (ER) stress and altered lipid metabolism (188, 194). This phenomenon has been associated with the expression of proteins involved in lipid trafficking and metabolism, such as CD36, lectin-like oxidized low-density lipoprotein receptor-1 (LOX-1), and fatty acid transport protein 2 (FATP2), and with an immunosuppressive phenotype of neutrophils (195). For instance, ER-stressed neutrophils produce higher amounts of ROS and Arg-1, which inhibit T cell proliferation and cytokine production (195, 196). Remarkably, FATP2 expression on neutrophils induced the production of PGE2, which has potent immunosuppressive activity on NK cells and CD8+ T cells (188, 197).
Neutrophils can also indirectly affect NK cell and CD8+ T cell cytotoxic activity through the release of neutrophil extracellular traps (NETs) that shield cancer cells (198). In this context, CXCL chemokines produced by tumor cells induce NETosis in neutrophils, which can coat and protect cancer cells from the cytotoxic activity of NK cells and CD8+ T cells. Interestingly, blockade of protein arginine deiminase 4 (PAD-4), which is essential for the formation of NETs, increased the activity of anti-PD-1 and anti-Cytotoxic T-Lymphocyte Antigen 4 (CTLA-4) immunotherapy in a transplantable mouse model of breast cancer (198).
The interactions between neutrophils and lymphocytes extend beyond the release of soluble mediators, as neutrophils themselves express immune checkpoints such as PD-L1 or VISTA. These molecules can interact with their ligands expressed on T cells and NK cells, leading to their dysfunction (199–204). Neutrophils expressing PD-L1 or VISTA have been found in various types of human and murine cancer, including hepatocellular carcinoma, melanoma, and gastric cancer (199–203).
As mentioned earlier, neutrophils can also interact with lymphoid cells to activate their anti-tumor activity. For instance, in patients with NSLC, neutrophils with antigen-presenting cell (APC)-like features were found and shown to be capable to activate CD4+ and CD8+ T cells (184). Neutrophils can acquire these APC-like features in response to TME-derived GM-CSF and IFN-γ, which induce the expression of MHC-II and CD86 in neutrophils (184). The interaction between these TANs isolated from lung cancer tissue and activated T cells led to increased expression of the costimulatory molecules CD54, CD86, OX40L, and 4-1BBL on the neutrophil surface, which further enhanced T cell proliferation, creating a positive feedback loop (205). Similar findings were observed in colorectal cancer patients, where neutrophils enhanced TCR signaling in CD8+ T cells, in a cell-to-cell contact dependent manner through the interaction between CD54/intercellular adhesion molecule 1 (ICAM-1) expressed on neutrophils and CD11a expressed on T cells (139). Further investigations have demonstrated that the phagocytosis of antibody-antigen complexes via Fc gamma receptors (FcγRs) renders murine and human neutrophils more potent APC-like cells (206).
In addition to T cells, neutrophils can induce NK cell activation through various mechanisms (207, 208). For example, cytokine-stimulated NK cells and neutrophils exchange contact-dependent activation signals involving CD18, ICAM-1 and ICAM-3 (207).
Stimulated neutrophils can attract and activate NK cells trough release of soluble mediators, including IL-1β and IL-18 (208). In turn, activated NK cells can trigger the maturation of dendritic cells, resulting in T cell proliferation and IFN-γ production, suggesting an additional mechanism trough which neutrophils can indirectly control the T cell anti-tumor immune response (208).
In addition to conventional T cells, neutrophils can influence the polarization and activation state of a subset of unconventional T cells, leading to their secretion of IFN-γ. This mechanism required a tripartite interaction between neutrophils, macrophages and unconventional T cells (see below) (143).
The reasons for these dichotomous functions of neutrophils on lymphoid cells are not fully elucidated. Interestingly, a recent study conducted in head and neck cancer (HNC) patients described this neutrophil dual role in tumor-draining lymph nodes, where neutrophils can interact with T cells in a stage-dependent manner (185). In metastasis-free patients, neutrophils transmigrate to lymph-nodes, acquiring APC-like features and promoting T cell anti-tumor activity (185). In contrast, at a later stage, neutrophils acquire PD-L1 expression and suppress T cell activation (185).
Conversely, neutrophils can be influenced by lymphoid cells, which can modulate their recruitment and phenotype to the tumor bed under different conditions (Figure 4) (186, 189, 209, 210). For instance, in a mouse model of breast cancer in KEP mice, γδ T cells in response to IL-1β showed increased production of IL-17 which induced a G-CSF dependent accumulation of neutrophils with an immunosuppressive phenotype in the peripheral blood and metastatic lung (189). In CRC patients, IL-22 producing T cells induced the recruitment of neutrophils, by triggering the production of neutrophil-recruiting chemokines (i.e. CXCL1, CXCL2, CXCL3) by colorectal cancer cells. Importantly, the expression of IL-22 was found associated with the presence of neutrophils and T cells and a favorable prognosis (186).
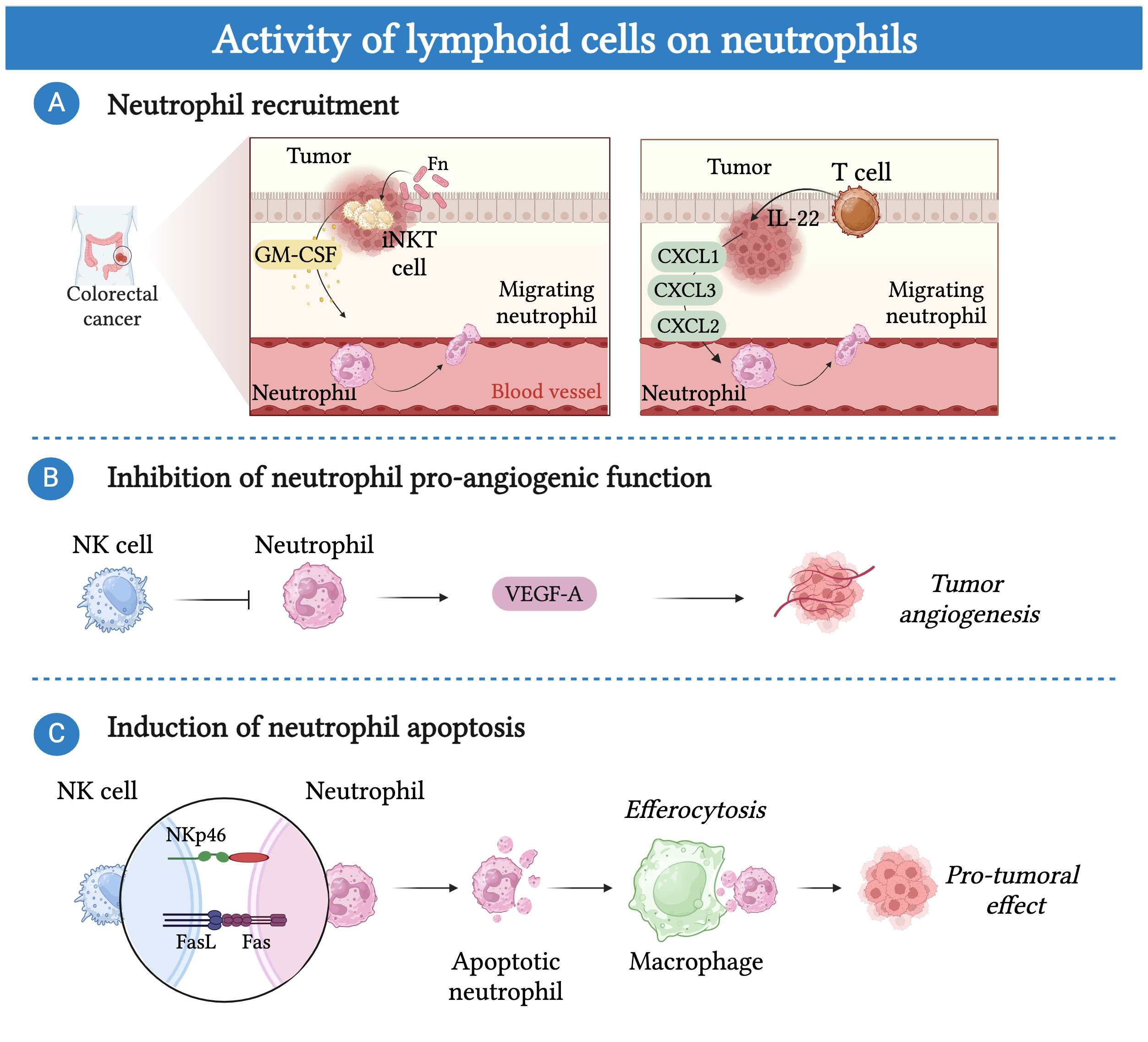
Figure 4 Lymphoid cells can modulate the activity of neutrophils. (A) Lymphoid cells can regulate the recruitment of neutrophils into the tumor bed. In CRC patients, tumor-associated pathobiont Fusobacterium nucleatum (Fn) induces the production of IL-17 and GM-CSF in invariant natural killer T cells (iNKT) resulting in increased neutrophil migration into the tumor bed. Additionally, IL-22 producing T cells induce the recruitment of neutrophils, by triggering the production of neutrophil-recruiting chemokines (i.e. CXCL1, CXCL2, CXCL3) by colorectal cancer cells. (B) NK cells control pro-tumor angiogenic function of neutrophils by blocking their secretion of vascular endothelial growth factor (VEGF). (C) NK-cells induce neutrophil apoptosis in a NKp46 and FAS-dependent manner. Efferocytosis of apoptotic neutrophils by macrophages promote a shift toward an M2-like pro-tumor phenotype.
Recently, in colorectal cancer patients, iNKT cells were found to increase the recruitment of neutrophils with immunosuppressive activity (209). The mechanism was related to the presence of the tumor-associated pathobiont Fusobacterium nucleatum, which induced the production of IL-17 and GM-CSF in iNKT. Importantly, the presence of iNKT cells and neutrophils correlated with a worse prognosis, suggesting that targeting this crosstalk could improve patient survival (209). On the other hand, NK cells have been involved in the control of neutrophil pro-tumor activity through an IFN-γ-dependent mechanism in mice (210). In a mouse model of transplantable sarcoma, the absence of NK cells induced neutrophils to acquire a pro-tumor phenotype characterized by the expression of VEGF-A (210) Interestingly, tumor-reprogrammed neutrophils that localize in a unique hypoxic and glycolytic niche exert a potent tumor-supporting, pro-angiogenic function through their high expression of VEGF-A (171). In contrast, NK cells have been suggested to induce neutrophil apoptosis via a NKp46 and FAS–dependent mechanism (211, 212). Remarkably, efferocytosis of apoptotic neutrophils by macrophages has been well documented to promote their shift toward an M2-like pro-tumor phenotype (213).
Neutrophils have been shown to interact also with B cells. Specifically, splenic neutrophils have been described to play a B-cell helper function, promoting the immunoglobulin class switching and the production of antibodies by activated B cells through a mechanism involving BAFF, APRIL and IL-21 (214). However, the involvement of this interaction in cancer has not been investigated.
Neutrophils and myeloid cells
As mentioned above for macrophages, while a significant amount of research has focused on the interaction between neutrophils and lymphoid cells, a limited number of studies have explored the cross-talk between neutrophils and other myeloid cells in the TME (Figure 5) (215).
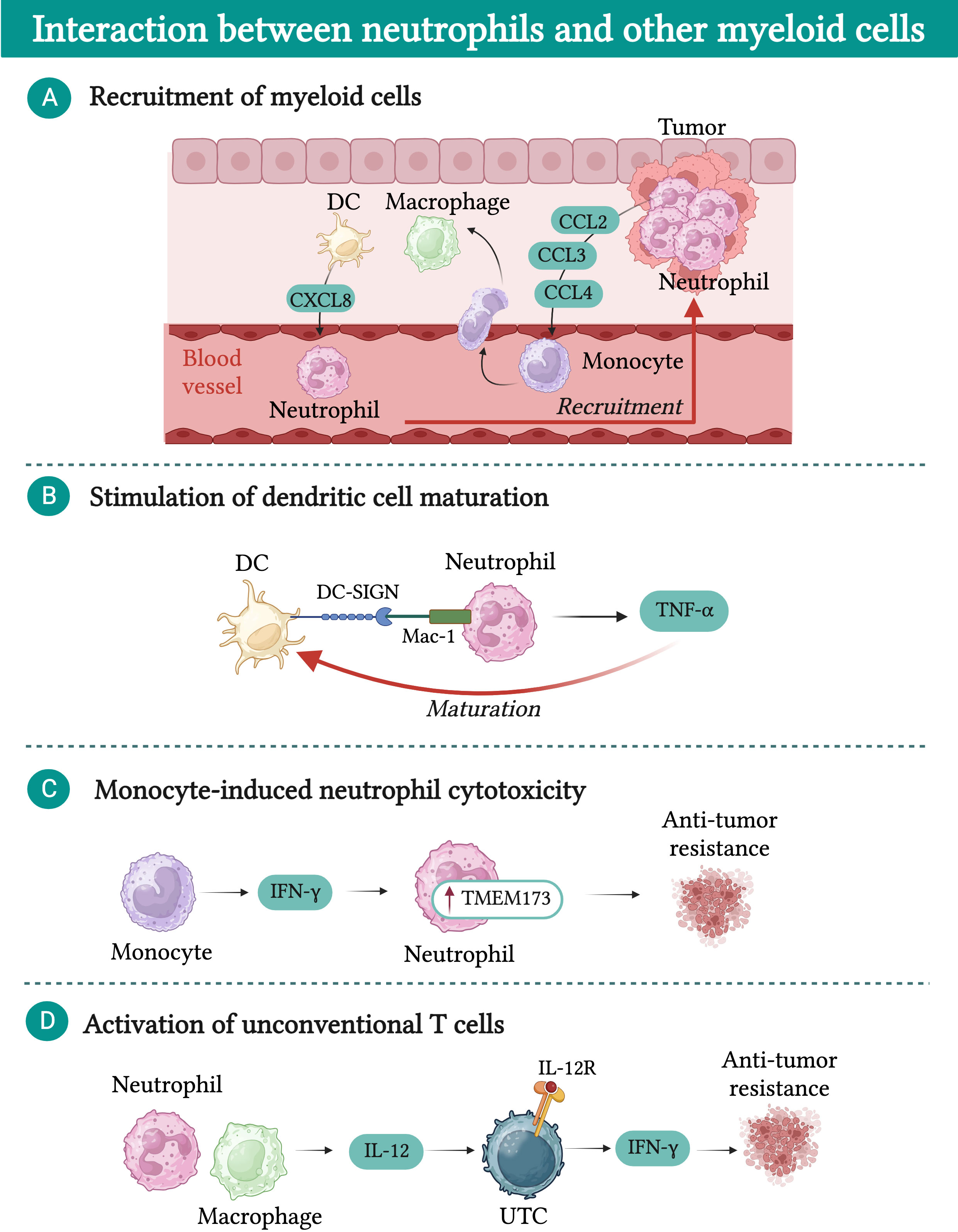
Figure 5 Interactions between neutrophils and other myeloid cells in cancer. (A) Macrophages and dendritic cells (DCs) are attracted into the tumor bed by chemokines released by neutrophils (e.g. CCL2, CCL3, and CCL4). In turn, macrophages and DCs produce chemokines (e.g. CXCL8) which further fuel neutrophil recruitment. (B) DCs physically interact with neutrophils via dendritic cell-specific intercellular adhesion molecule-3-grabbing non-integrin (DC-SIGN), resulting in increased release of tumor necrosis factor α (TNF-α). In turn, TNF-α promotes DC maturation. (C) IFN-γ produced by monocytes induces upregulation of transmembrane protein 173 (TMEM173) expression in neutrophils, unleashing their cytotoxic activity. (D) In a mouse model of sarcoma, neutrophil-macrophage interaction results in increased production of IL-12 by macrophages, which induces the expression of interferon γ (IFN-γ) in a subset of unconventional αβ T cells (UTCαβ) and favors their anti-tumor activity.
Neutrophils play a role in promoting the recruitment of other myeloid cells into the tumor bed through the release of chemokines such as CCL2, CCL3, and CCL4, which attract monocytes and dendritic cells (216). Once in the TME, monocytes and dendritic cells produce CXCL8, which favors the recruitment of additional neutrophils, creating a feedback loop that fosters the accumulation of inflammatory cells within the TME (217). Moreover, dendritic cells have been shown to physically interact with neutrophils via dendritic cell-specific intercellular adhesion molecule-3-grabbing non-integrin (DC-SIGN), leading to increased release of TNF-α by neutrophils (217). This interaction enhances the maturation of dendritic cells, thereby improving their capacity to effectively prime T cells and activate their anti-tumor response (217).
Another intriguing hypothesis is that neutrophils could amplify the source of antigens that dendritic cells process and present to T cells (218). Neutrophil-mediated trogoptosis of cancer cells may lead to an increased release of antigens and damage-associated molecular patterns (DAMPs) available for dendritic cells (219–222). This mechanism has been proposed as a potential therapeutic target and strategy to improve dendritic cell-based anti-cancer vaccines.
In a mouse model of breast cancer, it has been shown that neutrophil cytotoxic activity can be modulated by monocytes (223). Breast cancer cells with low spontaneous metastatic potential secrete high levels of CCL2, leading to the recruitment of IFN-γ-producing monocytes. Subsequently, neutrophils upregulate the expression of the transmembrane protein 173 (TMEM173, also known as stimulator of interferon response CGAMP interactor 1 (STING)), which then unleashes their cytotoxic activity (223).
Additionally, neutrophils were found to play a crucial role in potentiating the release of IL-12 by macrophages in the context of 3-methylcholanthrene (3-MCA)-induced sarcomagenesis (143). In turn, IL-12 can activate a subset of unconventional T cells (UTC) that express high levels of IL-12R, resulting in their production of IFN-γ and tumor control (143). To further underscore the importance of the interaction between neutrophils and other myeloid cells, two recent reports investigating the limited success of CSF-1R treatment in preclinical models of cancer revealed that upon TAM depletion, neutrophils acquired a highly immunosuppressive phenotype, counteracting the beneficial effect of macrophage depletion (224, 225).
Conclusion and perspectives
The TME represents a complex ecological niche composed of tumor cells, stromal cells and immune cells constantly engaged in mutual interactions that influence tumor development and progression. TAMs and TANs are crucial components of this niche and affect tumor progression by directly influencing tumor cell proliferation and by shaping the anti-tumor or pro-tumor response of other immune cells. In turn, the phenotypes and activities of TAMs and TANs are continuously regulated by other immune cells present in the TME.
Targeting the immune system represents a therapeutic strategy against cancer. However, a significant percentage of patients do not respond to current available treatments, underlining the need for new therapeutic approaches. As we uncover the complexity of the interactions between macrophages and neutrophils and other immune cells, it becomes evident that targeting these interactions may hold promise for developing novel and effective immunotherapeutic approaches to fight cancer.
Author contributions
ID: Writing – original draft. SC: Writing – original draft. AR: Writing – original draft. GG: Writing – original draft. PM: Writing – original draft. SJ: Writing – review & editing.
Funding
The author(s) declare financial support was received for the research, authorship, and/or publication of this article. SC is recipient of a fellowship from the Italian Association for Cancer Research. ID is financed by the HIgh Profile POst doc program – HIPPO Fondi 5x1000 Italian Ministry of University and Research. The Italian Ministry of Health GR-2018-12365588 and RF-2021-12372202 to SJ, the European Union - Next Generation EU - NRRP M6C2 - Investment 2.1 Enhancement and strengthening of biomedical research in the NHS - PNRR-MAD-2022-12375947 to SJ, the Italian Association for Cancer Research AIRC IG-22815 to SJ; Italian Ministry of University and Research - PRIN 2017K7FSYB to SJ, are gratefully acknowledged.
Acknowledgments
Figures were created with BioRender.com.
Conflict of interest
The authors declare that the research was conducted in the absence of any commercial or financial relationships that could be construed as a potential conflict of interest.
Publisher’s note
All claims expressed in this article are solely those of the authors and do not necessarily represent those of their affiliated organizations, or those of the publisher, the editors and the reviewers. Any product that may be evaluated in this article, or claim that may be made by its manufacturer, is not guaranteed or endorsed by the publisher.
References
1. Anderson NM, Simon MC. The tumor microenvironment. Curr Biol (2020) 30(16):R921–R5. doi: 10.1016/j.cub.2020.06.081
2. Baghban R, Roshangar L, Jahanban-Esfahlan R, Seidi K, Ebrahimi-Kalan A, Jaymand M, et al. Tumor microenvironment complexity and therapeutic implications at a glance. Cell Commun Signal (2020) 18(1):59. doi: 10.1186/s12964-020-0530-4
3. Giraldo NA, Sanchez-Salas R, Peske JD, Vano Y, Becht E, Petitprez F, et al. The clinical role of the TME in solid cancer. Br J Cancer (2019) 120(1):45–53. doi: 10.1038/s41416-018-0327-z
4. Galon J, Bruni D. Tumor immunology and tumor evolution: intertwined histories. Immunity (2020) 52(1):55–81. doi: 10.1016/j.immuni.2019.12.018
5. Pena-Romero AC, Orenes-Pinero E. Dual effect of immune cells within tumour microenvironment: pro- and anti-tumour effects and their triggers. Cancers (Basel) (2022) 14(7):1681. doi: 10.3390/cancers14071681
6. Wu B, Shi X, Jiang M, Liu H. Cross-talk between cancer stem cells and immune cells: potential therapeutic targets in the tumor immune microenvironment. Mol Cancer (2023) 22(1):38. doi: 10.1186/s12943-023-01748-4
7. Vinay DS, Ryan EP, Pawelec G, Talib WH, Stagg J, Elkord E, et al. Immune evasion in cancer: Mechanistic basis and therapeutic strategies. Semin Cancer Biol (2015) 35 Suppl:S185–S98. doi: 10.1016/j.semcancer.2015.03.004
8. Li M, Jiang P, Wei S, Wang J, Li C. The role of macrophages-mediated communications among cell compositions of tumor microenvironment in cancer progression. Front Immunol (2023) 14:1113312. doi: 10.3389/fimmu.2023.1113312
9. Zhou Y, Cheng L, Liu L, Li X. NK cells are never alone: crosstalk and communication in tumour microenvironments. Mol Cancer (2023) 22(1):34. doi: 10.1186/s12943-023-01737-7
10. Zhong J, Zong S, Wang J, Feng M, Wang J, Zhang H, et al. Role of neutrophils on cancer cells and other immune cells in the tumor microenvironment. Biochim Biophys Acta Mol Cell Res (2023) 1870(7):119493. doi: 10.1016/j.bbamcr.2023.119493
11. Mantovani A, Allavena P, Marchesi F, Garlanda C. Macrophages as tools and targets in cancer therapy. Nat Rev Drug Discovery (2022) 21(11):799–820. doi: 10.1038/s41573-022-00520-5
12. DeNardo DG, Ruffell B. Macrophages as regulators of tumour immunity and immunotherapy. Nat Rev Immunol (2019) 19(6):369–82. doi: 10.1038/s41577-019-0127-6
13. Cassetta L, Pollard JW. A timeline of tumour-associated macrophage biology. Nat Rev Cancer (2023) 23(4):238–57. doi: 10.1038/s41568-022-00547-1
14. Jaillon S, Ponzetta A, Di Mitri D, Santoni A, Bonecchi R, Mantovani A. Neutrophil diversity and plasticity in tumour progression and therapy. Nat Rev Cancer (2020) 20(9):485–503. doi: 10.1038/s41568-020-0281-y
15. Carnevale S, Ghasemi S, Rigatelli A, Jaillon S. The complexity of neutrophils in health and disease: Focus on cancer. Semin Immunol (2020) 48:101409. doi: 10.1016/j.smim.2020.101409
16. Carnevale S, Di Ceglie I, Grieco G, Rigatelli A, Bonavita E, Jaillon S. Neutrophil diversity in inflammation and cancer. Front Immunol (2023) 14:1180810. doi: 10.3389/fimmu.2023.1180810
17. Hedrick CC, Malanchi I. Neutrophils in cancer: heterogeneous and multifaceted. Nat Rev Immunol (2022) 22(3):173–87. doi: 10.1038/s41577-021-00571-6
18. Ng LG, Ostuni R, Hidalgo A. Heterogeneity of neutrophils. Nat Rev Immunol (2019) 19(4):255–65. doi: 10.1038/s41577-019-0141-8
19. Park MD, Silvin A, Ginhoux F, Merad M. Macrophages in health and disease. Cell (2022) 185(23):4259–79. doi: 10.1016/j.cell.2022.10.007
20. Beyrau M, Bodkin JV, Nourshargh S. Neutrophil heterogeneity in health and disease: a revitalized avenue in inflammation and immunity. Open Biol (2012) 2(11):120134. doi: 10.1098/rsob.120134
21. Kloosterman DJ, Akkari L. Macrophages at the interface of the co-evolving cancer ecosystem. Cell (2023) 186(8):1627–51. doi: 10.1016/j.cell.2023.02.020
22. Kazakova A, Sudarskikh T, Kovalev O, Kzhyshkowska J, Larionova I. Interaction of tumor−associated macrophages with stromal and immune components in solid tumors: Research progress (Review). Int J Oncol (2023) 62(2):32. doi: 10.3892/ijo.2023.5480
23. Abbott M, Ustoyev Y. Cancer and the immune system: the history and background of immunotherapy. Semin Oncol Nurs (2019) 35(5):150923. doi: 10.1016/j.soncn.2019.08.002
24. De Cicco P, Ercolano G, Ianaro A. The new era of cancer immunotherapy: targeting myeloid-derived suppressor cells to overcome immune evasion. Front Immunol (2020) 11:1680. doi: 10.3389/fimmu.2020.01680
25. Pilard C, Ancion M, Delvenne P, Jerusalem G, Hubert P, Herfs M. Cancer immunotherapy: it’s time to better predict patients’ response. Br J Cancer (2021) 125(7):927–38. doi: 10.1038/s41416-021-01413-x
26. Mantovani A, Marchesi F, Malesci A, Laghi L, Allavena P. Tumour-associated macrophages as treatment targets in oncology. Nat Rev Clin Oncol (2017) 14(7):399–416. doi: 10.1038/nrclinonc.2016.217
27. Zhang QW, Liu L, Gong CY, Shi HS, Zeng YH, Wang XZ, et al. Prognostic significance of tumor-associated macrophages in solid tumor: a meta-analysis of the literature. PloS One (2012) 7(12):e50946. doi: 10.1371/journal.pone.0050946
28. Di Caro G, Cortese N, Castino GF, Grizzi F, Gavazzi F, Ridolfi C, et al. Dual prognostic significance of tumour-associated macrophages in human pancreatic adenocarcinoma treated or untreated with chemotherapy. Gut (2016) 65(10):1710–20. doi: 10.1136/gutjnl-2015-309193
29. Leek RD, Lewis CE, Whitehouse R, Greenall M, Clarke J, Harris AL. Association of macrophage infiltration with angiogenesis and prognosis in invasive breast carcinoma. Cancer Res (1996) 56(20):4625–9.
30. Hanada T, Nakagawa M, Emoto A, Nomura T, Nasu N, Nomura Y. Prognostic value of tumor-associated macrophage count in human bladder cancer. Int J Urol (2000) 7(7):263–9. doi: 10.1046/j.1442-2042.2000.00190.x
31. Forssell J, Oberg A, Henriksson ML, Stenling R, Jung A, Palmqvist R. High macrophage infiltration along the tumor front correlates with improved survival in colon cancer. Clin Cancer Res (2007) 13(5):1472–9. doi: 10.1158/1078-0432.CCR-06-2073
32. Malesci A, Bianchi P, Celesti G, Basso G, Marchesi F, Grizzi F, et al. Tumor-associated macrophages and response to 5-fluorouracil adjuvant therapy in stage III colorectal cancer. Oncoimmunology (2017) 6(12):e1342918. doi: 10.1080/2162402X.2017.1342918
33. Tanaka Y, Kobayashi H, Suzuki M, Kanayama N, Suzuki M, Terao T. Upregulation of bikunin in tumor-infiltrating macrophages as a factor of favorable prognosis in ovarian cancer. Gynecol Oncol (2004) 94(3):725–34. doi: 10.1016/j.ygyno.2004.06.012
34. Mantovani A, Bottazzi B, Colotta F, Sozzani S, Ruco L. The origin and function of tumor-associated macrophages. Immunol Today (1992) 13(7):265–70. doi: 10.1016/0167-5699(92)90008-U
35. Qian BZ, Li J, Zhang H, Kitamura T, Zhang J, Campion LR, et al. CCL2 recruits inflammatory monocytes to facilitate breast-tumour metastasis. Nature (2011) 475(7355):222–5. doi: 10.1038/nature10138
36. Bottazzi B, Polentarutti N, Acero R, Balsari A, Boraschi D, Ghezzi P, et al. Regulation of the macrophage content of neoplasms by chemoattractants. Science (1983) 220(4593):210–2. doi: 10.1126/science.6828888
37. Arwert EN, Harney AS, Entenberg D, Wang Y, Sahai E, Pollard JW, et al. A unidirectional transition from migratory to perivascular macrophage is required for tumor cell intravasation. Cell Rep (2018) 23(5):1239–48. doi: 10.1016/j.celrep.2018.04.007
38. Pena CG, Nakada Y, Saatcioglu HD, Aloisio GM, Cuevas I, Zhang S, et al. LKB1 loss promotes endometrial cancer progression via CCL2-dependent macrophage recruitment. J Clin Invest (2015) 125(11):4063–76. doi: 10.1172/JCI82152
39. Clarijs R, Schalkwijk L, Ruiter DJ, de Waal RM. EMAP-II expression is associated with macrophage accumulation in primary uveal melanoma. Invest Ophthalmol Vis Sci (2003) 44(5):1801–6. doi: 10.1167/iovs.02-0624
40. Ginhoux F, Guilliams M. Tissue-resident macrophage ontogeny and homeostasis. Immunity (2016) 44(3):439–49. doi: 10.1016/j.immuni.2016.02.024
41. Ginhoux F, Schultze JL, Murray PJ, Ochando J, Biswas SK. New insights into the multidimensional concept of macrophage ontogeny, activation and function. Nat Immunol (2016) 17(1):34–40. doi: 10.1038/ni.3324
42. Casanova-Acebes M, Dalla E, Leader AM, LeBerichel J, Nikolic J, Morales BM, et al. Tissue-resident macrophages provide a pro-tumorigenic niche to early NSCLC cells. Nature (2021) 595(7868):578–84. doi: 10.1038/s41586-021-03651-8
43. Zhu Y, Herndon JM, Sojka DK, Kim KW, Knolhoff BL, Zuo C, et al. Tissue-resident macrophages in pancreatic ductal adenocarcinoma originate from embryonic hematopoiesis and promote tumor progression. Immunity (2017) 47(2):323–38 e6. doi: 10.1016/j.immuni.2017.07.014
44. Loyher PL, Hamon P, Laviron M, Meghraoui-Kheddar A, Goncalves E, Deng Z, et al. Macrophages of distinct origins contribute to tumor development in the lung. J Exp Med (2018) 215(10):2536–53. doi: 10.1084/jem.20180534
45. Bowman RL, Klemm F, Akkari L, Pyonteck SM, Sevenich L, Quail DF, et al. Macrophage ontogeny underlies differences in tumor-specific education in brain Malignancies. Cell Rep (2016) 17(9):2445–59. doi: 10.1016/j.celrep.2016.10.052
46. Lahmar Q, Keirsse J, Laoui D, Movahedi K, Van Overmeire E, Van Ginderachter JA. Tissue-resident versus monocyte-derived macrophages in the tumor microenvironment. Biochim Biophys Acta (2016) 1865(1):23–34. doi: 10.1016/j.bbcan.2015.06.009
47. Franklin RA, Li MO. Ontogeny of tumor-associated macrophages and its implication in cancer regulation. Trends Cancer (2016) 2(1):20–34. doi: 10.1016/j.trecan.2015.11.004
48. Locati M, Curtale G, Mantovani A. Diversity, mechanisms, and significance of macrophage plasticity. Annu Rev Pathol (2020) 15:123–47. doi: 10.1146/annurev-pathmechdis-012418-012718
49. Masetti M, Carriero R, Portale F, Marelli G, Morina N, Pandini M, et al. Lipid-loaded tumor-associated macrophages sustain tumor growth and invasiveness in prostate cancer. J Exp Med (2022) 219(2):e20210564. doi: 10.1084/jem.20210564
50. Mantovani A, Sozzani S, Locati M, Allavena P, Sica A. Macrophage polarization: tumor-associated macrophages as a paradigm for polarized M2 mononuclear phagocytes. Trends Immunol (2002) 23(11):549–55. doi: 10.1016/S1471-4906(02)02302-5
51. Allavena P, Sica A, Garlanda C, Mantovani A. The Yin-Yang of tumor-associated macrophages in neoplastic progression and immune surveillance. Immunol Rev (2008) 222:155–61. doi: 10.1111/j.1600-065X.2008.00607.x
52. Murray PJ, Allen JE, Biswas SK, Fisher EA, Gilroy DW, Goerdt S, et al. Macrophage activation and polarization: nomenclature and experimental guidelines. Immunity (2014) 41(1):14–20. doi: 10.1016/j.immuni.2014.06.008
53. Stein M, Keshav S, Harris N, Gordon S. Interleukin 4 potently enhances murine macrophage mannose receptor activity: a marker of alternative immunologic macrophage activation. J Exp Med (1992) 176(1):287–92. doi: 10.1084/jem.176.1.287
54. Cheng S, Li Z, Gao R, Xing B, Gao Y, Yang Y, et al. A pan-cancer single-cell transcriptional atlas of tumor infiltrating myeloid cells. Cell (2021) 184(3):792–809 e23. doi: 10.1016/j.cell.2021.01.010
55. Ma RY, Black A, Qian BZ. Macrophage diversity in cancer revisited in the era of single-cell omics. Trends Immunol (2022) 43(7):546–63. doi: 10.1016/j.it.2022.04.008
56. Zhang Q, He Y, Luo N, Patel SJ, Han Y, Gao R, et al. Landscape and dynamics of single immune cells in hepatocellular carcinoma. Cell (2019) 179(4):829–45 e20. doi: 10.1016/j.cell.2019.10.003
57. Obradovic A, Chowdhury N, Haake SM, Ager C, Wang V, Vlahos L, et al. Single-cell protein activity analysis identifies recurrence-associated renal tumor macrophages. Cell (2021) 184(11):2988–3005 e16. doi: 10.1016/j.cell.2021.04.038
58. Mulder K, Patel AA, Kong WT, Piot C, Halitzki E, Dunsmore G, et al. Cross-tissue single-cell landscape of human monocytes and macrophages in health and disease. Immunity (2021) 54(8):1883–900 e5. doi: 10.1016/j.immuni.2021.07.007
59. Andersson A, Larsson L, Stenbeck L, Salmen F, Ehinger A, Wu SZ, et al. Spatial deconvolution of HER2-positive breast cancer delineates tumor-associated cell type interactions. Nat Commun (2021) 12(1):6012. doi: 10.1038/s41467-021-26271-2
60. Cortese N, Carriero R, Barbagallo M, Putignano AR, Costa G, Giavazzi F, et al. High-resolution analysis of mononuclear phagocytes reveals GPNMB as a prognostic marker in human colorectal liver metastasis. Cancer Immunol Res (2023) 11(4):405–20. doi: 10.1158/2326-6066.CIR-22-0462
61. Donadon M, Torzilli G, Cortese N, Soldani C, Di Tommaso L, Franceschini B, et al. Macrophage morphology correlates with single-cell diversity and prognosis in colorectal liver metastasis. J Exp Med (2020) 217(11):e20191847. doi: 10.1084/jem.20191847
62. Peranzoni E, Lemoine J, Vimeux L, Feuillet V, Barrin S, Kantari-Mimoun C, et al. Macrophages impede CD8 T cells from reaching tumor cells and limit the efficacy of anti-PD-1 treatment. Proc Natl Acad Sci U S A (2018) 115(17):E4041–E50. doi: 10.1073/pnas.1720948115
63. La Fleur L, Botling J, He F, Pelicano C, Zhou C, He C, et al. Targeting MARCO and IL37R on immunosuppressive macrophages in lung cancer blocks regulatory T cells and supports cytotoxic lymphocyte function. Cancer Res (2021) 81(4):956–67. doi: 10.1158/0008-5472.CAN-20-1885
64. Peng LS, Zhang JY, Teng YS, Zhao YL, Wang TT, Mao FY, et al. Tumor-associated monocytes/macrophages impair NK-cell function via TGFbeta1 in human gastric cancer. Cancer Immunol Res (2017) 5(3):248–56. doi: 10.1158/2326-6066.CIR-16-0152
65. Park MD, Reyes-Torres I, LeBerichel J, Hamon P, LaMarche NM, Hegde S, et al. TREM2 macrophages drive NK cell paucity and dysfunction in lung cancer. Nat Immunol (2023) 24(5):792–801. doi: 10.1038/s41590-023-01475-4
66. Wu Y, Kuang DM, Pan WD, Wan YL, Lao XM, Wang D, et al. Monocyte/macrophage-elicited natural killer cell dysfunction in hepatocellular carcinoma is mediated by CD48/2B4 interactions. Hepatology (2013) 57(3):1107–16. doi: 10.1002/hep.26192
67. Erlandsson A, Carlsson J, Lundholm M, Falt A, Andersson SO, Andren O, et al. M2 macrophages and regulatory T cells in lethal prostate cancer. Prostate (2019) 79(4):363–9. doi: 10.1002/pros.23742
68. Ma X, Gao Y, Chen Y, Liu J, Yang C, Bao C, et al. M2-type macrophages induce tregs generation by activating the TGF-beta/smad signalling pathway to promote colorectal cancer development. Onco Targets Ther (2021) 14:5391–402. doi: 10.2147/OTT.S336548
69. Zhu Y, Knolhoff BL, Meyer MA, Nywening TM, West BL, Luo J, et al. CSF1/CSF1R blockade reprograms tumor-infiltrating macrophages and improves response to T-cell checkpoint immunotherapy in pancreatic cancer models. Cancer Res (2014) 74(18):5057–69. doi: 10.1158/0008-5472.CAN-13-3723
70. Mitchem JB, Brennan DJ, Knolhoff BL, Belt BA, Zhu Y, Sanford DE, et al. Targeting tumor-infiltrating macrophages decreases tumor-initiating cells, relieves immunosuppression, and improves chemotherapeutic responses. Cancer Res (2013) 73(3):1128–41. doi: 10.1158/0008-5472.CAN-12-2731
71. Batlle E, Massague J. Transforming growth factor-beta signaling in immunity and cancer. Immunity (2019) 50(4):924–40. doi: 10.1016/j.immuni.2019.03.024
72. Krneta T, Gillgrass A, Poznanski S, Chew M, Lee AJ, Kolb M, et al. M2-polarized and tumor-associated macrophages alter NK cell phenotype and function in a contact-dependent manner. J Leukoc Biol (2017) 101(1):285–95. doi: 10.1189/jlb.3A1215-552R
73. Molgora M, Supino D, Mavilio D, Santoni A, Moretta L, Mantovani A, et al. The yin-yang of the interaction between myelomonocytic cells and NK cells. Scand J Immunol (2018) 88(3):e12705. doi: 10.1111/sji.12705
74. Li L, Yang L, Wang L, Wang F, Zhang Z, Li J, et al. Impaired T cell function in Malignant pleural effusion is caused by TGF-beta derived predominantly from macrophages. Int J Cancer (2016) 139(10):2261–9. doi: 10.1002/ijc.30289
75. Kos K, Salvagno C, Wellenstein MD, Aslam MA, Meijer DA, Hau CS, et al. Tumor-associated macrophages promote intratumoral conversion of conventional CD4(+) T cells into regulatory T cells via PD-1 signalling. Oncoimmunology (2022) 11(1):2063225. doi: 10.1080/2162402X.2022.2063225
76. Kessenbrock K, Plaks V, Werb Z. Matrix metalloproteinases: regulators of the tumor microenvironment. Cell (2010) 141(1):52–67. doi: 10.1016/j.cell.2010.03.015
77. Geiger R, Rieckmann JC, Wolf T, Basso C, Feng Y, Fuhrer T, et al. L-arginine modulates T cell metabolism and enhances survival and anti-tumor activity. Cell (2016) 167(3):829–42 e13. doi: 10.1016/j.cell.2016.09.031
78. Rodriguez PC, Quiceno DG, Zabaleta J, Ortiz B, Zea AH, Piazuelo MB, et al. Arginase I production in the tumor microenvironment by mature myeloid cells inhibits T-cell receptor expression and antigen-specific T-cell responses. Cancer Res (2004) 64(16):5839–49. doi: 10.1158/0008-5472.CAN-04-0465
79. Munn DH, Shafizadeh E, Attwood JT, Bondarev I, Pashine A, Mellor AL. Inhibition of T cell proliferation by macrophage tryptophan catabolism. J Exp Med (1999) 189(9):1363–72. doi: 10.1084/jem.189.9.1363
80. Lu T, Ramakrishnan R, Altiok S, Youn JI, Cheng P, Celis E, et al. Tumor-infiltrating myeloid cells induce tumor cell resistance to cytotoxic T cells in mice. J Clin Invest (2011) 121(10):4015–29. doi: 10.1172/JCI45862
81. Lu T, Gabrilovich DI. Molecular pathways: tumor-infiltrating myeloid cells and reactive oxygen species in regulation of tumor microenvironment. Clin Cancer Res (2012) 18(18):4877–82. doi: 10.1158/1078-0432.CCR-11-2939
82. Nagaraj S, Gupta K, Pisarev V, Kinarsky L, Sherman S, Kang L, et al. Altered recognition of antigen is a mechanism of CD8+ T cell tolerance in cancer. Nat Med (2007) 13(7):828–35. doi: 10.1038/nm1609
83. Antonioli L, Pacher P, Vizi ES, Hasko G. CD39 and CD73 in immunity and inflammation. Trends Mol Med (2013) 19(6):355–67. doi: 10.1016/j.molmed.2013.03.005
84. Takenaka MC, Gabriely G, Rothhammer V, Mascanfroni ID, Wheeler MA, Chao CC, et al. Author Correction: Control of tumor-associated macrophages and T cells in glioblastoma via AHR and CD39. Nat Neurosci (2019) 22(9):1533. doi: 10.1038/s41593-019-0446-8
85. Mastelic-Gavillet B, Navarro Rodrigo B, Decombaz L, Wang H, Ercolano G, Ahmed R, et al. Adenosine mediates functional and metabolic suppression of peripheral and tumor-infiltrating CD8(+) T cells. J Immunother Cancer (2019) 7(1):257. doi: 10.1186/s40425-019-0719-5
86. Kuang DM, Zhao Q, Peng C, Xu J, Zhang JP, Wu C, et al. Activated monocytes in peritumoral stroma of hepatocellular carcinoma foster immune privilege and disease progression through PD-L1. J Exp Med (2009) 206(6):1327–37. doi: 10.1084/jem.20082173
87. Lin H, Wei S, Hurt EM, Green MD, Zhao L, Vatan L, et al. Host expression of PD-L1 determines efficacy of PD-L1 pathway blockade-mediated tumor regression. J Clin Invest (2018) 128(2):805–15. doi: 10.1172/JCI96113
88. Brom VC, Burger C, Wirtz DC, Schildberg FA. The role of immune checkpoint molecules on macrophages in cancer, infection, and autoimmune pathologies. Front Immunol (2022) 13:837645. doi: 10.3389/fimmu.2022.837645
89. Kryczek I, Zou L, Rodriguez P, Zhu G, Wei S, Mottram P, et al. B7-H4 expression identifies a novel suppressive macrophage population in human ovarian carcinoma. J Exp Med (2006) 203(4):871–81. doi: 10.1084/jem.20050930
90. Liu L, Li D, Chen S, Zhao R, Pang D, Li D, et al. B7-H4 expression in human infiltrating ductal carcinoma−associated macrophages. Mol Med Rep (2016) 14(3):2135–42. doi: 10.3892/mmr.2016.5510
91. Li J, Lee Y, Li Y, Jiang Y, Lu H, Zang W, et al. Co-inhibitory molecule B7 superfamily member 1 expressed by tumor-infiltrating myeloid cells induces dysfunction of anti-tumor CD8(+) T cells. Immunity (2018) 48(4):773–86 e5. doi: 10.1016/j.immuni.2018.03.018
92. Blando J, Sharma A, Higa MG, Zhao H, Vence L, Yadav SS, et al. Comparison of immune infiltrates in melanoma and pancreatic cancer highlights VISTA as a potential target in pancreatic cancer. Proc Natl Acad Sci U S A (2019) 116(5):1692–7. doi: 10.1073/pnas.1811067116
93. Xie X, Zhang J, Shi Z, Liu W, Hu X, Qie C, et al. The expression pattern and clinical significance of the immune checkpoint regulator VISTA in human breast cancer. Front Immunol (2020) 11:563044. doi: 10.3389/fimmu.2020.563044
94. Dyck L, Mills KHG. Immune checkpoints and their inhibition in cancer and infectious diseases. Eur J Immunol (2017) 47(5):765–79. doi: 10.1002/eji.201646875
95. Pesce S, Greppi M, Tabellini G, Rampinelli F, Parolini S, Olive D, et al. Identification of a subset of human natural killer cells expressing high levels of programmed death 1: A phenotypic and functional characterization. J Allergy Clin Immunol (2017) 139(1):335–46 e3. doi: 10.1016/j.jaci.2016.04.025
96. Kersten K, Hu KH, Combes AJ, Samad B, Harwin T, Ray A, et al. Spatiotemporal co-dependency between macrophages and exhausted CD8(+) T cells in cancer. Cancer Cell (2022) 40(6):624–38 e9. doi: 10.1016/j.ccell.2022.05.004
97. Strachan DC, Ruffell B, Oei Y, Bissell MJ, Coussens LM, Pryer N, et al. CSF1R inhibition delays cervical and mammary tumor growth in murine models by attenuating the turnover of tumor-associated macrophages and enhancing infiltration by CD8(+) T cells. Oncoimmunology (2013) 2(12):e26968. doi: 10.4161/onci.26968
98. Pascual-Garcia M, Bonfill-Teixidor E, Planas-Rigol E, Rubio-Perez C, Iurlaro R, Arias A, et al. LIF regulates CXCL9 in tumor-associated macrophages and prevents CD8(+) T cell tumor-infiltration impairing anti-PD1 therapy. Nat Commun (2019) 10(1):2416. doi: 10.1038/s41467-019-10369-9
99. Dangaj D, Bruand M, Grimm AJ, Ronet C, Barras D, Duttagupta PA, et al. Cooperation between constitutive and inducible chemokines enables T cell engraftment and immune attack in solid tumors. Cancer Cell (2019) 35(6):885–900 e10. doi: 10.1016/j.ccell.2019.05.004
100. Curiel TJ, Coukos G, Zou L, Alvarez X, Cheng P, Mottram P, et al. Specific recruitment of regulatory T cells in ovarian carcinoma fosters immune privilege and predicts reduced survival. Nat Med (2004) 10(9):942–9. doi: 10.1038/nm1093
101. Furudate S, Fujimura T, Kambayashi Y, Kakizaki A, Hidaka T, Aiba S. Immunomodulatory effect of imiquimod through CCL22 produced by tumor-associated macrophages in B16F10 melanomas. Anticancer Res (2017) 37(7):3461–71. doi: 10.21873/anticanres.11714
102. Chow A, SChad S, Green MD, Hellmann MD, Allaj V, Ceglia N, et al. Tim-4(+) cavity-resident macrophages impair anti-tumor CD8(+) T cell immunity. Cancer Cell (2021) 39(7):973–88 e9. doi: 10.1016/j.ccell.2021.05.006
103. Nalio Ramos R, Missolo-Koussou Y, Gerber-Ferder Y, Bromley CP, Bugatti M, Nunez NG, et al. Tissue-resident FOLR2(+) macrophages associate with CD8(+) T cell infiltration in human breast cancer. Cell (2022) 185(7):1189–207 e25. doi: 10.1016/j.cell.2022.02.021
104. Zhang Y, Sun Y, Rao E, Yan F, Li Q, Zhang Y, et al. Fatty acid-binding protein E-FABP restricts tumor growth by promoting IFN-beta responses in tumor-associated macrophages. Cancer Res (2014) 74(11):2986–98. doi: 10.1158/0008-5472.CAN-13-2689
105. Mattiola I, Pesant M, Tentorio PF, Molgora M, Marcenaro E, Lugli E, et al. Priming of human resting NK cells by autologous M1 macrophages via the engagement of IL-1beta, IFN-beta, and IL-15 pathways. J Immunol (2015) 195(6):2818–28. doi: 10.4049/jimmunol.1500325
106. Bellora F, Castriconi R, Dondero A, Reggiardo G, Moretta L, Mantovani A, et al. The interaction of human natural killer cells with either unpolarized or polarized macrophages results in different functional outcomes. Proc Natl Acad Sci U S A (2010) 107(50):21659–64. doi: 10.1073/pnas.1007654108
107. Craxton A, Magaletti D, Ryan EJ, Clark EA. Macrophage- and dendritic cell–dependent regulation of human B-cell proliferation requires the TNF family ligand BAFF. Blood (2003) 101(11):4464–71. doi: 10.1182/blood-2002-10-3123
108. Thies FG, Laurindo MF, Perez EC, Novaes e Brito RR, Mariano M, Popi AF. Cross talk between peritoneal macrophages and B-1 cells in vitro. PloS One (2013) 8(5):e62805. doi: 10.1371/journal.pone.0062805
109. Biswas SK, Mantovani A. Macrophage plasticity and interaction with lymphocyte subsets: cancer as a paradigm. Nat Immunol (2010) 11(10):889–96. doi: 10.1038/ni.1937
110. Ruffell B, Affara NI, Coussens LM. Differential macrophage programming in the tumor microenvironment. Trends Immunol (2012) 33(3):119–26. doi: 10.1016/j.it.2011.12.001
111. Gordon S, Martinez FO. Alternative activation of macrophages: mechanism and functions. Immunity (2010) 32(5):593–604. doi: 10.1016/j.immuni.2010.05.007
112. Dannenmann SR, Thielicke J, Stockli M, Matter C, von Boehmer L, Cecconi V, et al. Tumor-associated macrophages subvert T-cell function and correlate with reduced survival in clear cell renal cell carcinoma. Oncoimmunology (2013) 2(3):e23562. doi: 10.4161/onci.23562
113. DeNardo DG, Barreto JB, Andreu P, Vasquez L, Tawfik D, Kolhatkar N, et al. CD4(+) T cells regulate pulmonary metastasis of mammary carcinomas by enhancing protumor properties of macrophages. Cancer Cell (2009) 16(2):91–102. doi: 10.1016/j.ccr.2009.06.018
114. Yin G, Zhao C, Pei W. Crosstalk between macrophages and innate lymphoid cells (ILCs) in diseases. Int Immunopharmacol (2022) 110:108937. doi: 10.1016/j.intimp.2022.108937
115. Tiemessen MM, Jagger AL, Evans HG, van Herwijnen MJ, John S, Taams LS. CD4+CD25+Foxp3+ regulatory T cells induce alternative activation of human monocytes/macrophages. Proc Natl Acad Sci U S A (2007) 104(49):19446–51. doi: 10.1073/pnas.0706832104
116. Liu C, Chikina M, Deshpande R, Menk AV, Wang T, Tabib T, et al. Treg cells promote the SREBP1-dependent metabolic fitness of tumor-promoting macrophages via repression of CD8(+) T cell-derived interferon-gamma. Immunity (2019) 51(2):381–97 e6. doi: 10.1016/j.immuni.2019.06.017
117. Song L, Asgharzadeh S, Salo J, Engell K, Wu HW, Sposto R, et al. Valpha24-invariant NKT cells mediate antitumor activity via killing of tumor-associated macrophages. J Clin Invest (2009) 119(6):1524–36. doi: 10.1172/JCI37869
118. Wong SC, Puaux AL, Chittezhath M, Shalova I, Kajiji TS, Wang X, et al. Macrophage polarization to a unique phenotype driven by B cells. Eur J Immunol (2010) 40(8):2296–307. doi: 10.1002/eji.200940288
119. Wculek SK, Cueto FJ, Mujal AM, Melero I, Krummel MF, Sancho D. Dendritic cells in cancer immunology and immunotherapy. Nat Rev Immunol (2020) 20(1):7–24. doi: 10.1038/s41577-019-0210-z
120. Gabrilovich DI, Chen HL, Girgis KR, Cunningham HT, Meny GM, Nadaf S, et al. Production of vascular endothelial growth factor by human tumors inhibits the functional maturation of dendritic cells. Nat Med (1996) 2(10):1096–103. doi: 10.1038/nm1096-1096
121. Menetrier-Caux C, Montmain G, Dieu MC, Bain C, Favrot MC, Caux C, et al. Inhibition of the differentiation of dendritic cells from CD34(+) progenitors by tumor cells: role of interleukin-6 and macrophage colony-stimulating factor. Blood (1998) 92(12):4778–91. doi: 10.1182/blood.V92.12.4778
122. Song S, Wang Y, Wang J, Lian W, Liu S, Zhang Z, et al. Tumour-derived IL-10 within tumour microenvironment represses the antitumour immunity of Socs1-silenced and sustained antigen expressing DCs. Eur J Cancer (2012) 48(14):2252–9. doi: 10.1016/j.ejca.2011.12.009
123. Thepmalee C, Panya A, Junking M, Chieochansin T, Yenchitsomanus PT. Inhibition of IL-10 and TGF-beta receptors on dendritic cells enhances activation of effector T-cells to kill cholangiocarcinoma cells. Hum Vaccin Immunother (2018) 14(6):1423–31. doi: 10.1080/21645515.2018.1431598
124. Ruffell B, Chang-Strachan D, Chan V, Rosenbusch A, Ho CM, Pryer N, et al. Macrophage IL-10 blocks CD8+ T cell-dependent responses to chemotherapy by suppressing IL-12 expression in intratumoral dendritic cells. Cancer Cell (2014) 26(5):623–37. doi: 10.1016/j.ccell.2014.09.006
125. Jensen HK, Donskov F, Marcussen N, Nordsmark M, Lundbeck F, von der Maase H. Presence of intratumoral neutrophils is an independent prognostic factor in localized renal cell carcinoma. J Clin Oncol (2009) 27(28):4709–17. doi: 10.1200/JCO.2008.18.9498
126. Kuang DM, Zhao Q, Wu Y, Peng C, Wang J, Xu Z, et al. Peritumoral neutrophils link inflammatory response to disease progression by fostering angiogenesis in hepatocellular carcinoma. J Hepatol (2011) 54(5):948–55. doi: 10.1016/j.jhep.2010.08.041
127. Li YW, Qiu SJ, Fan J, Zhou J, Gao Q, Xiao YS, et al. Intratumoral neutrophils: a poor prognostic factor for hepatocellular carcinoma following resection. J Hepatol (2011) 54(3):497–505. doi: 10.1016/j.jhep.2010.07.044
128. Ilie M, Hofman V, Ortholan C, Bonnetaud C, Coelle C, Mouroux J, et al. Predictive clinical outcome of the intratumoral CD66b-positive neutrophil-to-CD8-positive T-cell ratio in patients with resectable nonsmall cell lung cancer. Cancer (2012) 118(6):1726–37. doi: 10.1002/cncr.26456
129. Jensen TO, Schmidt H, Moller HJ, Donskov F, Hoyer M, Sjoegren P, et al. Intratumoral neutrophils and plasmacytoid dendritic cells indicate poor prognosis and are associated with pSTAT3 expression in AJCC stage I/II melanoma. Cancer (2012) 118(9):2476–85. doi: 10.1002/cncr.26511
130. Trellakis S, Bruderek K, Dumitru CA, Gholaman H, Gu X, Bankfalvi A, et al. Polymorphonuclear granulocytes in human head and neck cancer: enhanced inflammatory activity, modulation by cancer cells and expansion in advanced disease. Int J Cancer (2011) 129(9):2183–93. doi: 10.1002/ijc.25892
131. Fossati G, Ricevuti G, Edwards SW, Walker C, Dalton A, Rossi ML. Neutrophil infiltration into human gliomas. Acta Neuropathol (1999) 98(4):349–54. doi: 10.1007/s004010051093
132. Rao HL, Chen JW, Li M, Xiao YB, Fu J, Zeng YX, et al. Increased intratumoral neutrophil in colorectal carcinomas correlates closely with Malignant phenotype and predicts patients’ adverse prognosis. PloS One (2012) 7(1):e30806. doi: 10.1371/journal.pone.0030806
133. Li S, Cong X, Gao H, Lan X, Li Z, Wang W, et al. Tumor-associated neutrophils induce EMT by IL-17a to promote migration and invasion in gastric cancer cells. J Exp Clin Cancer Res (2019) 38(1):6. doi: 10.1186/s13046-018-1003-0
134. Hassan WA, ElBanna AK, Noufal N, El-Assmy M, Lotfy H, Ali RI. Significance of tumor-associated neutrophils, lymphocytes, and neutrophil-to-lymphocyte ratio in non-invasive and invasive bladder urothelial carcinoma. J Pathol Transl Med (2023) 57(2):88–94. doi: 10.4132/jptm.2022.11.06
135. Yang M, Zhang G, Wang Y, He M, Xu Q, Lu J, et al. Tumour-associated neutrophils orchestrate intratumoural IL-8-driven immune evasion through Jagged2 activation in ovarian cancer. Br J Cancer (2020) 123(9):1404–16. doi: 10.1038/s41416-020-1026-0
136. Khalid F, Takagi K, Sato A, Yamaguchi M, Guestini F, Miki Y, et al. Interleukin (IL)-17A in triple-negative breast cancer: a potent prognostic factor associated with intratumoral neutrophil infiltration. Breast Cancer (2023) 30(5):748–57. doi: 10.1007/s12282-023-01467-0
137. Powell DR, Huttenlocher A. Neutrophils in the tumor microenvironment. Trends Immunol (2016) 37(1):41–52. doi: 10.1016/j.it.2015.11.008
138. Schmidt H, Bastholt L, Geertsen P, Christensen IJ, Larsen S, Gehl J, et al. Elevated neutrophil and monocyte counts in peripheral blood are associated with poor survival in patients with metastatic melanoma: a prognostic model. Br J Cancer (2005) 93(3):273–8. doi: 10.1038/sj.bjc.6602702
139. Governa V, Trella E, Mele V, Tornillo L, Amicarella F, Cremonesi E, et al. The interplay between neutrophils and CD8(+) T cells improves survival in human colorectal cancer. Clin Cancer Res (2017) 23(14):3847–58. doi: 10.1158/1078-0432.CCR-16-2047
140. Droeser RA, Hirt C, Eppenberger-Castori S, Zlobec I, Viehl CT, Frey DM, et al. High myeloperoxidase positive cell infiltration in colorectal cancer is an independent favorable prognostic factor. PloS One (2013) 8(5):e64814. doi: 10.1371/journal.pone.0064814
141. Zhou G, Peng K, Song Y, Yang W, Shu W, Yu T, et al. CD177+ neutrophils suppress epithelial cell tumourigenesis in colitis-associated cancer and predict good prognosis in colorectal cancer. Carcinogenesis (2018) 39(2):272–82. doi: 10.1093/carcin/bgx142
142. Galdiero MR, Bianchi P, Grizzi F, Di Caro G, Basso G, Ponzetta A, et al. Occurrence and significance of tumor-associated neutrophils in patients with colorectal cancer. Int J Cancer (2016) 139(2):446–56. doi: 10.1002/ijc.30076
143. Ponzetta A, Carriero R, Carnevale S, Barbagallo M, Molgora M, Perucchini C, et al. Neutrophils driving unconventional T cells mediate resistance against murine sarcomas and selected human tumors. Cell (2019) 178(2):346–60 e24. doi: 10.1016/j.cell.2019.05.047
144. Yvan-Charvet L, Ng LG. Granulopoiesis and neutrophil homeostasis: A metabolic, daily balancing act. Trends Immunol (2019) 40(7):598–612. doi: 10.1016/j.it.2019.05.004
145. Liu F, Wu HY, Wesselschmidt R, Kornaga T, Link DC. Impaired production and increased apoptosis of neutrophils in granulocyte colony-stimulating factor receptor-deficient mice. Immunity (1996) 5(5):491–501. doi: 10.1016/S1074-7613(00)80504-X
146. Walker F, Zhang HH, Matthews V, Weinstock J, Nice EC, Ernst M, et al. IL6/sIL6R complex contributes to emergency granulopoietic responses in G-CSF- and GM-CSF-deficient mice. Blood (2008) 111(8):3978–85. doi: 10.1182/blood-2007-10-119636
147. Zhu YP, Padgett L, Dinh HQ, Marcovecchio P, Blatchley A, Wu R, et al. Identification of an early unipotent neutrophil progenitor with pro-tumoral activity in mouse and human bone marrow. Cell Rep (2018) 24(9):2329–41 e8. doi: 10.1016/j.celrep.2018.07.097
148. Calzetti F, Finotti G, Tamassia N, Bianchetto-Aguilera F, Castellucci M, Cane S, et al. CD66b(-)CD64(dim)CD115(-) cells in the human bone marrow represent neutrophil-committed progenitors. Nat Immunol (2022) 23(5):679–91. doi: 10.1038/s41590-022-01189-z
149. Evrard M, Kwok IWH, Chong SZ, Teng KWW, Becht E, Chen J, et al. Developmental analysis of bone marrow neutrophils reveals populations specialized in expansion, trafficking, and effector functions. Immunity (2018) 48(2):364–79 e8. doi: 10.1016/j.immuni.2018.02.002
150. Eash KJ, Greenbaum AM, Gopalan PK, Link DC. CXCR2 and CXCR4 antagonistically regulate neutrophil trafficking from murine bone marrow. J Clin Invest (2010) 120(7):2423–31. doi: 10.1172/JCI41649
151. Adrover JM, Del Fresno C, Crainiciuc G, Cuartero MI, Casanova-Acebes M, Weiss LA, et al. A neutrophil timer coordinates immune defense and vascular protection. Immunity (2019) 50(2):390–402 e10. doi: 10.1016/j.immuni.2019.01.002
152. Marin-Esteban V, Youn J, Beaupain B, Jaracz-Ros A, Barlogis V, Fenneteau O, et al. Biallelic CXCR2 loss-of-function mutations define a distinct congenital neutropenia entity. Haematologica (2022) 107(3):765–9. doi: 10.3324/haematol.2021.279254
153. De Filippo K, Rankin SM. CXCR4, the master regulator of neutrophil trafficking in homeostasis and disease. Eur J Clin Invest (2018) 48 Suppl 2(Suppl 2):e12949. doi: 10.1111/eci.12949
154. Manz MG, Boettcher S. Emergency granulopoiesis. Nat Rev Immunol (2014) 14(5):302–14. doi: 10.1038/nri3660
155. Capucetti A, Albano F, Bonecchi R. Multiple roles for chemokines in neutrophil biology. Front Immunol (2020) 11:1259. doi: 10.3389/fimmu.2020.01259
156. Mollica Poeta V, Massara M, Capucetti A, Bonecchi R. Chemokines and chemokine receptors: new targets for cancer immunotherapy. Front Immunol (2019) 10:379. doi: 10.3389/fimmu.2019.00379
157. Jamieson T, Clarke M, Steele CW, Samuel MS, Neumann J, Jung A, et al. Inhibition of CXCR2 profoundly suppresses inflammation-driven and spontaneous tumorigenesis. J Clin Invest (2012) 122(9):3127–44. doi: 10.1172/JCI61067
158. SenGupta S, Subramanian BC, Parent CA. Getting TANned: How the tumor microenvironment drives neutrophil recruitment. J Leukoc Biol (2019) 105(3):449–62. doi: 10.1002/JLB.3RI0718-282R
159. Yu PF, Huang Y, Han YY, Lin LY, Sun WH, Rabson AB, et al. TNFalpha-activated mesenchymal stromal cells promote breast cancer metastasis by recruiting CXCR2(+) neutrophils. Oncogene (2017) 36(4):482–90. doi: 10.1038/onc.2016.217
160. Gijsbers K, Gouwy M, Struyf S, Wuyts A, Proost P, Opdenakker G, et al. GCP-2/CXCL6 synergizes with other endothelial cell-derived chemokines in neutrophil mobilization and is associated with angiogenesis in gastrointestinal tumors. Exp Cell Res (2005) 303(2):331–42. doi: 10.1016/j.yexcr.2004.09.027
161. Zhou SL, Dai Z, Zhou ZJ, Chen Q, Wang Z, Xiao YS, et al. CXCL5 contributes to tumor metastasis and recurrence of intrahepatic cholangiocarcinoma by recruiting infiltrative intratumoral neutrophils. Carcinogenesis (2014) 35(3):597–605. doi: 10.1093/carcin/bgt397
162. Lee LF, Hellendall RP, Wang Y, Haskill JS, Mukaida N, Matsushima K, et al. IL-8 reduced tumorigenicity of human ovarian cancer in vivo due to neutrophil infiltration. J Immunol (2000) 164(5):2769–75. doi: 10.4049/jimmunol.164.5.2769
163. SenGupta S, Hein LE, Parent CA. The recruitment of neutrophils to the tumor microenvironment is regulated by multiple mediators. Front Immunol (2021) 12:734188. doi: 10.3389/fimmu.2021.734188
164. Charles KA, Kulbe H, Soper R, Escorcio-Correia M, Lawrence T, Schultheis A, et al. The tumor-promoting actions of TNF-alpha involve TNFR1 and IL-17 in ovarian cancer in mice and humans. J Clin Invest (2009) 119(10):3011–23. doi: 10.1172/JCI39065
165. Fridlender ZG, Sun J, Kim S, Kapoor V, Cheng G, Ling L, et al. Polarization of tumor-associated neutrophil phenotype by TGF-beta: “N1” versus “N2” TAN. Cancer Cell (2009) 16(3):183–94. doi: 10.1016/j.ccr.2009.06.017
166. Sionov RV, Fridlender ZG, Granot Z. The multifaceted roles neutrophils play in the tumor microenvironment. Cancer Microenviron (2015) 8(3):125–58. doi: 10.1007/s12307-014-0147-5
167. Montaldo E, Lusito E, Bianchessi V, Caronni N, Scala S, Basso-Ricci L, et al. Cellular and transcriptional dynamics of human neutrophils at steady state and upon stress. Nat Immunol (2022) 23(10):1470–83. doi: 10.1038/s41590-022-01311-1
168. Salcher S, Sturm G, Horvath L, Untergasser G, Kuempers C, Fotakis G, et al. High-resolution single-cell atlas reveals diversity and plasticity of tissue-resident neutrophils in non-small cell lung cancer. Cancer Cell (2022) 40(12):1503–20 e8. doi: 10.1016/j.ccell.2022.10.008
169. Peng H, Wu X, Liu S, He M, Tang C, Wen Y, et al. Cellular dynamics in tumour microenvironment along with lung cancer progression underscore spatial and evolutionary heterogeneity of neutrophil. Clin Transl Med (2023) 13(7):e1340. doi: 10.1002/ctm2.1340
170. Sorin M, Rezanejad M, Karimi E, Fiset B, Desharnais L, Perus LJM, et al. Single-cell spatial landscapes of the lung tumour immune microenvironment. Nature (2023) 614(7948):548–54. doi: 10.1038/s41586-022-05672-3
171. Ng MSF, Kwok I, Tan L, Shi C, Cerezo-Wallis D, Tan Y, et al. Deterministic reprogramming of neutrophils within tumors. Science (2024) 383(6679):eadf6493. doi: 10.1126/science.adf6493
172. Canli O, Nicolas AM, Gupta J, Finkelmeier F, Goncharova O, Pesic M, et al. Myeloid cell-derived reactive oxygen species induce epithelial mutagenesis. Cancer Cell (2017) 32(6):869–83 e5. doi: 10.1016/j.ccell.2017.11.004
173. Butin-Israeli V, Bui TM, Wiesolek HL, Mascarenhas L, Lee JJ, Mehl LC, et al. Neutrophil-induced genomic instability impedes resolution of inflammation and wound healing. J Clin Invest (2019) 129(2):712–26. doi: 10.1172/JCI122085
174. Wculek SK, Bridgeman VL, Peakman F, Malanchi I. Early neutrophil responses to chemical carcinogenesis shape long-term lung cancer susceptibility. iScience (2020) 23(7):101277. doi: 10.1016/j.isci.2020.101277
175. Houghton AM, Rzymkiewicz DM, Ji H, Gregory AD, Egea EE, Metz HE, et al. Neutrophil elastase-mediated degradation of IRS-1 accelerates lung tumor growth. Nat Med (2010) 16(2):219–23. doi: 10.1038/nm.2084
176. Lerman I, Garcia-Hernandez ML, Rangel-Moreno J, Chiriboga L, Pan C, Nastiuk KL, et al. Infiltrating myeloid cells exert protumorigenic actions via neutrophil elastase. Mol Cancer Res (2017) 15(9):1138–52. doi: 10.1158/1541-7786.MCR-17-0003
177. Wada Y, Yoshida K, Tsutani Y, Shigematsu H, Oeda M, Sanada Y, et al. Neutrophil elastase induces cell proliferation and migration by the release of TGF-alpha, PDGF and VEGF in esophageal cell lines. Oncol Rep (2007) 17(1):161–7. doi: 10.3892/or.17.1.161
178. El Rayes T, Catena R, Lee S, Stawowczyk M, Joshi N, Fischbach C, et al. Lung inflammation promotes metastasis through neutrophil protease-mediated degradation of Tsp-1. Proc Natl Acad Sci U S A (2015) 112(52):16000–5. doi: 10.1073/pnas.1507294112
179. Wculek SK, Malanchi I. Author Correction: Neutrophils support lung colonization of metastasis-initiating breast cancer cells. Nature (2019) 571(7763):E2. doi: 10.1038/s41586-019-1328-7
180. Ardi VC, Kupriyanova TA, Deryugina EI, Quigley JP. Human neutrophils uniquely release TIMP-free MMP-9 to provide a potent catalytic stimulator of angiogenesis. Proc Natl Acad Sci U S A (2007) 104(51):20262–7. doi: 10.1073/pnas.0706438104
181. Yang L, Liu Q, Zhang X, Liu X, Zhou B, Chen J, et al. DNA of neutrophil extracellular traps promotes cancer metastasis via CCDC25. Nature (2020) 583(7814):133–8. doi: 10.1038/s41586-020-2394-6
182. Gershkovitz M, Caspi Y, Fainsod-Levi T, Katz B, Michaeli J, Khawaled S, et al. TRPM2 mediates neutrophil killing of disseminated tumor cells. Cancer Res (2018) 78(10):2680–90. doi: 10.1158/0008-5472.CAN-17-3614
183. Matlung HL, Babes L, Zhao XW, van Houdt M, Treffers LW, van Rees DJ, et al. Neutrophils kill antibody-opsonized cancer cells by trogoptosis. Cell Rep (2018) 23(13):3946–59 e6. doi: 10.1016/j.celrep.2018.05.082
184. Singhal S, Bhojnagarwala PS, O’Brien S, Moon EK, Garfall AL, Rao AS, et al. Origin and role of a subset of tumor-associated neutrophils with antigen-presenting cell features in early-stage human lung cancer. Cancer Cell (2016) 30(1):120–35. doi: 10.1016/j.ccell.2016.06.001
185. Pylaeva E, Korschunow G, Spyra I, Bordbari S, Siakaeva E, Ozel I, et al. During early stages of cancer, neutrophils initiate anti-tumor immune responses in tumor-draining lymph nodes. Cell Rep (2022) 40(7):111171. doi: 10.1016/j.celrep.2022.111171
186. Tosti N, Cremonesi E, Governa V, Basso C, Kancherla V, Coto-Llerena M, et al. Infiltration by IL22-producing T cells promotes neutrophil recruitment and predicts favorable clinical outcome in human colorectal cancer. Cancer Immunol Res (2020) 8(11):1452–62. doi: 10.1158/2326-6066.CIR-19-0934
187. Rice CM, Davies LC, Subleski JJ, Maio N, Gonzalez-Cotto M, Andrews C, et al. Tumour-elicited neutrophils engage mitochondrial metabolism to circumvent nutrient limitations and maintain immune suppression. Nat Commun (2018) 9(1):5099. doi: 10.1038/s41467-018-07505-2
188. Veglia F, Tyurin VA, Blasi M, De Leo A, Kossenkov AV, Donthireddy L, et al. Fatty acid transport protein 2 reprograms neutrophils in cancer. Nature (2019) 569(7754):73–8. doi: 10.1038/s41586-019-1118-2
189. Coffelt SB, Kersten K, Doornebal CW, Weiden J, Vrijland K, Hau CS, et al. IL-17-producing gammadelta T cells and neutrophils conspire to promote breast cancer metastasis. Nature (2015) 522(7556):345–8. doi: 10.1038/nature14282
190. Schmielau J, Finn OJ. Activated granulocytes and granulocyte-derived hydrogen peroxide are the underlying mechanism of suppression of t-cell function in advanced cancer patients. Cancer Res (2001) 61(12):4756–60.
191. Liu CY, Wang YM, Wang CL, Feng PH, Ko HW, Liu YH, et al. Population alterations of L-arginase- and inducible nitric oxide synthase-expressed CD11b+/CD14(-)/CD15+/CD33+ myeloid-derived suppressor cells and CD8+ T lymphocytes in patients with advanced-stage non-small cell lung cancer. J Cancer Res Clin Oncol (2010) 136(1):35–45. doi: 10.1007/s00432-009-0634-0
192. Rodriguez PC, Ernstoff MS, Hernandez C, Atkins M, Zabaleta J, Sierra R, et al. Arginase I-producing myeloid-derived suppressor cells in renal cell carcinoma are a subpopulation of activated granulocytes. Cancer Res (2009) 69(4):1553–60. doi: 10.1158/0008-5472.CAN-08-1921
193. Li P, Lu M, Shi J, Hua L, Gong Z, Li Q, et al. Dual roles of neutrophils in metastatic colonization are governed by the host NK cell status. Nat Commun (2020) 11(1):4387. doi: 10.1038/s41467-020-18125-0
194. Condamine T, Kumar V, Ramachandran IR, Youn JI, Celis E, Finnberg N, et al. ER stress regulates myeloid-derived suppressor cell fate through TRAIL-R-mediated apoptosis. J Clin Invest (2014) 124(6):2626–39. doi: 10.1172/JCI74056
195. Veglia F, Perego M, Gabrilovich D. Myeloid-derived suppressor cells coming of age. Nat Immunol (2018) 19(2):108–19. doi: 10.1038/s41590-017-0022-x
196. Lee BR, Chang SY, Hong EH, Kwon BE, Kim HM, Kim YJ, et al. Elevated endoplasmic reticulum stress reinforced immunosuppression in the tumor microenvironment via myeloid-derived suppressor cells. Oncotarget (2014) 5(23):12331–45. doi: 10.18632/oncotarget.2589
197. Bonavita E, Bromley CP, Jonsson G, Pelly VS, Sahoo S, Walwyn-Brown K, et al. Antagonistic inflammatory phenotypes dictate tumor fate and response to immune checkpoint blockade. Immunity (2020) 53(6):1215–29 e8. doi: 10.1016/j.immuni.2020.10.020
198. Teijeira A, Garasa S, Gato M, Alfaro C, Migueliz I, Cirella A, et al. CXCR1 and CXCR2 chemokine receptor agonists produced by tumors induce neutrophil extracellular traps that interfere with immune cytotoxicity. Immunity (2020) 52(5):856–71 e8. doi: 10.1016/j.immuni.2020.03.001
199. Cheng Y, Li H, Deng Y, Tai Y, Zeng K, Zhang Y, et al. Cancer-associated fibroblasts induce PDL1+ neutrophils through the IL6-STAT3 pathway that foster immune suppression in hepatocellular carcinoma. Cell Death Dis (2018) 9(4):422. doi: 10.1038/s41419-018-0458-4
200. He G, Zhang H, Zhou J, Wang B, Chen Y, Kong Y, et al. Peritumoural neutrophils negatively regulate adaptive immunity via the PD-L1/PD-1 signalling pathway in hepatocellular carcinoma. J Exp Clin Cancer Res (2015) 34:141. doi: 10.1186/s13046-015-0256-0
201. Noman MZ, Desantis G, Janji B, Hasmim M, Karray S, Dessen P, et al. PD-L1 is a novel direct target of HIF-1alpha, and its blockade under hypoxia enhanced MDSC-mediated T cell activation. J Exp Med (2014) 211(5):781–90. doi: 10.1084/jem.20131916
202. Xu W, Dong J, Zheng Y, Zhou J, Yuan Y, Ta HM, et al. Immune-checkpoint protein VISTA regulates antitumor immunity by controlling myeloid cell-mediated inflammation and immunosuppression. Cancer Immunol Res (2019) 7(9):1497–510. doi: 10.1158/2326-6066.CIR-18-0489
203. Wang TT, Zhao YL, Peng LS, Chen N, Chen W, Lv YP, et al. Tumour-activated neutrophils in gastric cancer foster immune suppression and disease progression through GM-CSF-PD-L1 pathway. Gut (2017) 66(11):1900–11. doi: 10.1136/gutjnl-2016-313075
204. Sun R, Xiong Y, Liu H, Gao C, Su L, Weng J, et al. Tumor-associated neutrophils suppress antitumor immunity of NK cells through the PD-L1/PD-1 axis. Transl Oncol (2020) 13(10):100825. doi: 10.1016/j.tranon.2020.100825
205. Eruslanov EB, Bhojnagarwala PS, Quatromoni JG, Stephen TL, Ranganathan A, Deshpande C, et al. Tumor-associated neutrophils stimulate T cell responses in early-stage human lung cancer. J Clin Invest (2014) 124(12):5466–80. doi: 10.1172/JCI77053
206. Mysore V, Cullere X, Mears J, Rosetti F, Okubo K, Liew PX, et al. FcgammaR engagement reprograms neutrophils into antigen cross-presenting cells that elicit acquired anti-tumor immunity. Nat Commun (2021) 12(1):4791. doi: 10.1038/s41467-021-24591-x
207. Costantini C, Calzetti F, Perbellini O, Micheletti A, Scarponi C, Lonardi S, et al. Human neutrophils interact with both 6-sulfo LacNAc+ DC and NK cells to amplify NK-derived IFNgamma: role of CD18, ICAM-1, and ICAM-3. Blood (2011) 117(5):1677–86. doi: 10.1182/blood-2010-06-287243
208. Riise RE, Bernson E, Aurelius J, Martner A, Pesce S, Della Chiesa M, et al. TLR-stimulated neutrophils instruct NK cells to trigger dendritic cell maturation and promote adaptive T cell responses. J Immunol (2015) 195(3):1121–8. doi: 10.4049/jimmunol.1500709
209. Lattanzi G, Strati F, Diaz-Basabe A, Perillo F, Amoroso C, Protti G, et al. iNKT cell-neutrophil crosstalk promotes colorectal cancer pathogenesis. Mucosal Immunol (2023) 16(3):326–40. doi: 10.1016/j.mucimm.2023.03.006
210. Ogura K, Sato-Matsushita M, Yamamoto S, Hori T, Sasahara M, Iwakura Y, et al. NK cells control tumor-promoting function of neutrophils in mice. Cancer Immunol Res (2018) 6(3):348–57. doi: 10.1158/2326-6066.CIR-17-0204
211. Thoren FB, Riise RE, Ousback J, Della Chiesa M, Alsterholm M, Marcenaro E, et al. Human NK Cells induce neutrophil apoptosis via an NKp46- and Fas-dependent mechanism. J Immunol (2012) 188(4):1668–74. doi: 10.4049/jimmunol.1102002
212. Bernson E, Christenson K, Pesce S, Pasanen M, Marcenaro E, Sivori S, et al. Downregulation of HLA class I renders inflammatory neutrophils more susceptible to NK cell-induced apoptosis. Front Immunol (2019) 10:2444. doi: 10.3389/fimmu.2019.02444
213. Brostjan C, Oehler R. The role of neutrophil death in chronic inflammation and cancer. Cell Death Discovery (2020) 6:26. doi: 10.1038/s41420-020-0255-6
214. Puga I, Cols M, Barra CM, He B, Cassis L, Gentile M, et al. B cell-helper neutrophils stimulate the diversification and production of immunoglobulin in the marginal zone of the spleen. Nat Immunol (2011) 13(2):170–80. doi: 10.1038/ni.2194
215. Garner H, de Visser KE. Immune crosstalk in cancer progression and metastatic spread: a complex conversation. Nat Rev Immunol (2020) 20(8):483–97. doi: 10.1038/s41577-019-0271-z
216. Mantovani A, Cassatella MA, Costantini C, Jaillon S. Neutrophils in the activation and regulation of innate and adaptive immunity. Nat Rev Immunol (2011) 11(8):519–31. doi: 10.1038/nri3024
217. van Gisbergen KP, Sanchez-Hernandez M, Geijtenbeek TB, van Kooyk Y. Neutrophils mediate immune modulation of dendritic cells through glycosylation-dependent interactions between Mac-1 and DC-SIGN. J Exp Med (2005) 201(8):1281–92. doi: 10.1084/jem.20041276
218. Gong T, Liu L, Jiang W, Zhou R. DAMP-sensing receptors in sterile inflammation and inflammatory diseases. Nat Rev Immunol (2020) 20(2):95–112. doi: 10.1038/s41577-019-0215-7
219. Chen GY, Nunez G. Sterile inflammation: sensing and reacting to damage. Nat Rev Immunol (2010) 10(12):826–37. doi: 10.1038/nri2873
220. Moynihan KD, Opel CF, Szeto GL, Tzeng A, Zhu EF, Engreitz JM, et al. Eradication of large established tumors in mice by combination immunotherapy that engages innate and adaptive immune responses. Nat Med (2016) 22(12):1402–10. doi: 10.1038/nm.4200
221. Carmi Y, Spitzer MH, Linde IL, Burt BM, Prestwood TR, Perlman N, et al. Allogeneic IgG combined with dendritic cell stimuli induce antitumour T-cell immunity. Nature (2015) 521(7550):99–104. doi: 10.1038/nature14424
222. Zhu EF, Gai SA, Opel CF, Kwan BH, Surana R, Mihm MC, et al. Synergistic innate and adaptive immune response to combination immunotherapy with anti-tumor antigen antibodies and extended serum half-life IL-2. Cancer Cell (2015) 27(4):489–501. doi: 10.1016/j.ccell.2015.03.004
223. Hagerling C, Gonzalez H, Salari K, Wang CY, Lin C, Robles I, et al. Immune effector monocyte-neutrophil cooperation induced by the primary tumor prevents metastatic progression of breast cancer. Proc Natl Acad Sci U S A (2019) 116(43):21704–14. doi: 10.1073/pnas.1907660116
224. Kumar V, Donthireddy L, Marvel D, Condamine T, Wang F, Lavilla-Alonso S, et al. Cancer-associated fibroblasts neutralize the anti-tumor effect of CSF1 receptor blockade by inducing PMN-MDSC infiltration of tumors. Cancer Cell (2017) 32(5):654–68 e5. doi: 10.1016/j.ccell.2017.10.005
Keywords: tumor microenvironment, anti-tumor immunity, tumor-associated macrophages, tumor-associated neutrophils, immune cell network
Citation: Di Ceglie I, Carnevale S, Rigatelli A, Grieco G, Molisso P and Jaillon S (2024) Immune cell networking in solid tumors: focus on macrophages and neutrophils. Front. Immunol. 15:1341390. doi: 10.3389/fimmu.2024.1341390
Received: 20 November 2023; Accepted: 29 January 2024;
Published: 14 February 2024.
Edited by:
Silvia Pesce, University of Genoa, ItalyReviewed by:
Yves Delneste, Institut National de la Santé et de la Recherche Médicale (INSERM), FranceNicola Tamassia, University of Verona, Italy
Copyright © 2024 Di Ceglie, Carnevale, Rigatelli, Grieco, Molisso and Jaillon. This is an open-access article distributed under the terms of the Creative Commons Attribution License (CC BY). The use, distribution or reproduction in other forums is permitted, provided the original author(s) and the copyright owner(s) are credited and that the original publication in this journal is cited, in accordance with accepted academic practice. No use, distribution or reproduction is permitted which does not comply with these terms.
*Correspondence: Sebastien Jaillon, c2ViYXN0aWVuLmphaWxsb25AaHVtYW5pdGFzcmVzZWFyY2guaXQ=
†These authors have contributed equally to this work