- 1Section Molecular Allergology, Paul-Ehrlich-Institut, Langen, Germany
- 2Section Research Allergology (ALG 5), Division of Allergology, Paul-Ehrlich-Institut, Langen, Germany
Type I hypersensitivity, or so-called type I allergy, is caused by Th2-mediated immune responses directed against otherwise harmless environmental antigens. Currently, allergen-specific immunotherapy (AIT) is the only disease-modifying treatment with the potential to re-establish clinical tolerance towards the corresponding allergen(s). However, conventional AIT has certain drawbacks, including long treatment durations, the risk of inducing allergic side effects, and the fact that allergens by themselves have a rather low immunogenicity. To improve AIT, adjuvants can be a powerful tool not only to increase the immunogenicity of co-applied allergens but also to induce the desired immune activation, such as promoting allergen-specific Th1- or regulatory responses. This review summarizes the knowledge on adjuvants currently approved for use in human AIT: aluminum hydroxide, calcium phosphate, microcrystalline tyrosine, and MPLA, as well as novel adjuvants that have been studied in recent years: oil-in-water emulsions, virus-like particles, viral components, carbohydrate-based adjuvants (QS-21, glucans, and mannan) and TLR-ligands (flagellin and CpG-ODN). The investigated adjuvants show distinct properties, such as prolonging allergen release at the injection site, inducing allergen-specific IgG production while also reducing IgE levels, as well as promoting differentiation and activation of different immune cells. In the future, better understanding of the immunological mechanisms underlying the effects of these adjuvants in clinical settings may help us to improve AIT.
1 Introduction
Allergic reactions are an increasing health and economic problem in developed countries (1–4). Type I allergies, the most common form of allergies, are caused by exaggerated immune responses directed against otherwise harmless antigens from our environment (so-called allergens). Immunologically, these immune responses are characterized by the induction of allergen-specific Th2 cells and IgE antibodies, which trigger IgE-dependent mast cell degranulation with the associated allergic symptoms.
Currently, the only treatment affecting the underlying disease with disease-modifying potential is allergen-specific immunotherapy (AIT). In this form of treatment, patients are confronted with increasing amounts of allergen(s) by either oral, subcutaneous (s.c.), or sublingual (s.l.) routes over long periods of time. If successful, AIT results in the re-establishment of clinical tolerance towards the offending allergen(s) and is associated with (I) a late decline in allergen-specific IgE, (II) an early increase in allergen-specific IgG subclasses, and (III) the induction of regulatory T- and B cell subsets (5).
One of the major obstacles in achieving efficient allergen-specific immune modulation is the fact that most pure allergen molecules only display a low degree of immunogenicity (6). Here, adding adjuvants to the low-immunogenic allergen(s) is a promising strategy to either enhance or modify the overall immune responses induced by the allergen(s).
Adjuvants increase the magnitude of immune responses directed against the co-applied antigen(s), allowing for both the application of lower antigen doses (which may reduce side effects) and the induction of robust immune responses against otherwise non/low-immunogenic antigens (7, 8). Furthermore, adjuvants can change the overall type of immune response as the co-applied antigens are taken up, processed, and presented in the context of the adjuvant-induced immune cell activation.
Depending on their mode of action, adjuvants can be further distinguished into first- and second-generation adjuvants (Figure 1). First-generation adjuvants used in AIT, such as aluminum salts, calcium phosphate, or microcrystalline tyrosine (MCT) serve as carriers, adsorbing antigens and forming insoluble, micron-sized adjuvant-antigen aggregates (9). The adjuvant-antigen aggregates activate immune cells, leading to the secretion of cytokines and chemokines as well as the formation of nucleotide oligomerization domain (NOD)-, leucine rich repeat (LRR)- and pyrin domain-containing protein 3 (NLRP3) inflammasomes. Subsequently, antigen-presenting cells (APCs) are recruited and activated by the production of strongly pro-inflammatory, bioactive IL-1β and IL-18, cell death, and the release of damage-associated molecular patterns (DAMPs) such as uric acid and self-DNA (Figure 1) (10–12). In addition, adjuvant-antigen aggregates can not only be directly taken up by APCs, but also act as depots, causing a slow release and effective presentation of the incorporated antigen(s) to APCs (Figure 1) (12). Despite the fact that first-generation adjuvants efficiently boost immune responses, especially antibody production, their application has a number of drawbacks for AIT. For example, aluminum hydroxide (alum)-based adjuvants may induce Th2 responses and IgE-production depending on the co-applied antigen, and lack the ability to induce CD8+ T cell responses (13, 14).
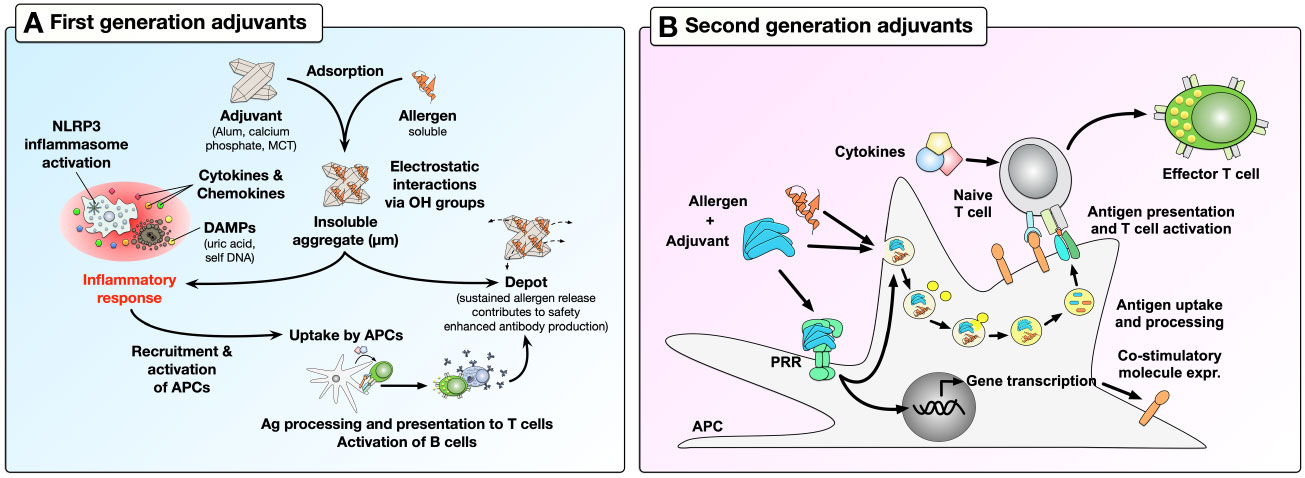
Figure 1 Boosting of immune responses by first- and second-generation adjuvants. First-generation adjuvants can adsorb soluble allergens through electrostatic interactions with the allergens hydroxyl groups to form insoluble aggregates (A). Micron-sized adjuvant-allergen aggregates can (I) induce activation of immune cells, which further recruit and activate APCs, (II) be directly taken up by APCs, or (III) act as depots to effectively enhance allergen-specific antibody production. In contrast, second-generation adjuvants activate immune cells by triggering the activation of APCs via their PRRs (B). Here, the binding of PAMPs (as second-generation adjuvants) to PRRs can induce transcriptional changes in APCs, leading to increased expression of co-stimulatory molecules and cytokine secretion. In addition, combining second-generation adjuvants with allergens can induce effective allergen uptake by APCs which then present allergen-derived peptides in the context of the adjuvant-induced APC activation. This results in effective T cell activation, thus triggering adaptive immune responses. For more information, see text. OH, hydroxyl group; MCT, microcrystalline tyrosine; NLRP3, NOD-, LRR- and pyrin domain-containing protein 3; PAMPs, pathogen-associated molecular patterns; DAMPs, damage-associated molecular patterns; APC, antigen-presenting cells; PRR, pathogen recognition receptor; Ag, antigen.
To improve first-generation adjuvants, recent studies have taken advantage of the discovery of pathogen recognition receptors (PRRs) (15). These receptors are abundantly expressed both on the surface and in the cytosolic compartments of APCs and other immune cells (B cells, T cells, neutrophils, mast cells, eosinophils, NK cells, innate-like lymphocytes), where they can promote immune responses by recognizing pathogen-associated molecular patterns (PAMPs) (15). Consequently, it was demonstrated that the use of different PAMPs (e.g., bacterial components, viral DNA/RNA) as second-generation adjuvants in combination with antigens could induce large-scale transcriptional changes in target cells, allowing them to alter their phenotype and function, e.g., by expressing co-inhibitory or co-stimulatory molecules, secreting cytokines, chemokines, and anti-microbial molecules; while also efficiently presenting antigens to T cells and thereby modulating T cell activation (Figure 1) (16).
Currently, adjuvant research is a topic of great interest not only for improving allergy treatment but also for the development of other vaccines. In this review, we shortly summarize the adjuvants already in use in human AIT and subsequently focus on those adjuvants currently under investigation for further improving AIT (see also Figure 2 for a general overview). The current state of clinical investigation of the discussed adjuvants is summarized in Table 1.
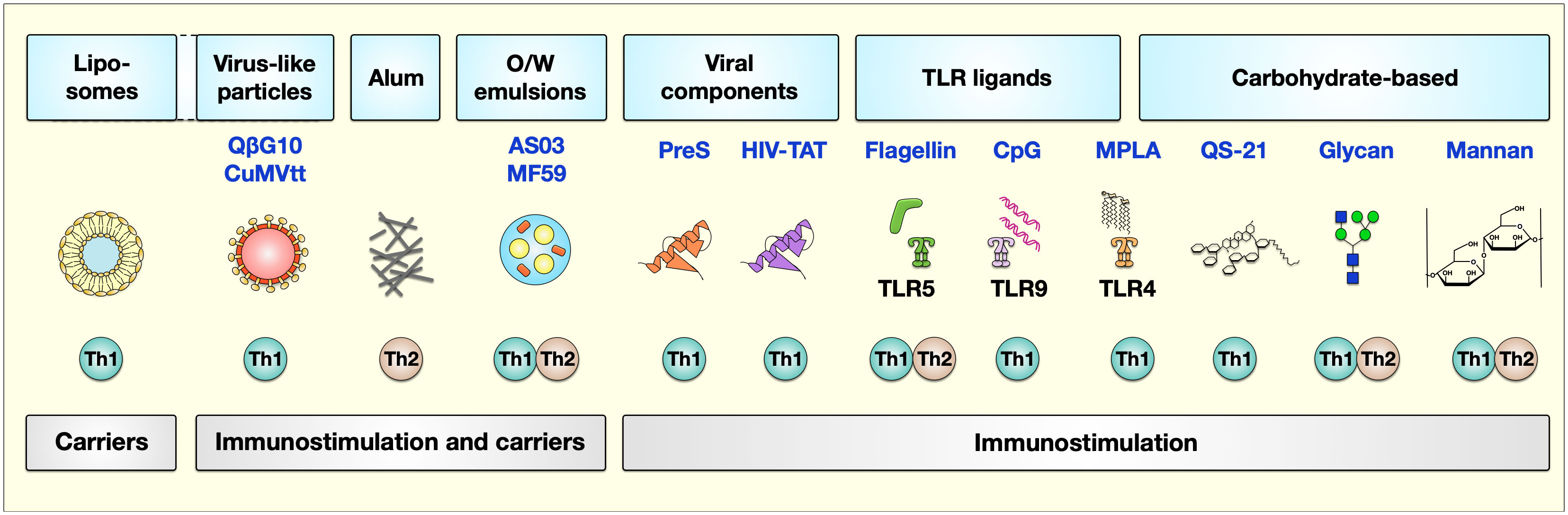
Figure 2 Adjuvants currently studied to improve AIT. The types of adjuvants can be classified based on their carrier or immunostimulatory capabilities. Here, liposomes serve as carriers, while virus-like particles, alum, and O/W emulsions have both carrier function and immune stimulatory characteristics. Besides viral components (the T-cell epitope derived from the hepatitis B virus PreS or the HIV-trans-activator of transcription (TAT)), TLR-ligands (flagellin, CpG, and MPLA), and carbohydrate-based adjuvants (QS-21, glycan, and mannan) have immunomodulatory properties triggering different kinds of immune responses. The described properties of these adjuvants to induce either Th1 or Th2 responses are indicated below the respective adjuvant. For more information, see text. AS, adjuvant system, alum: aluminum hydroxide; O/W, oil-in-water; Pres, HBV-derived T-cell epitope; TAT, HIV type 1 trans-activating regulatory protein; TLR, “Toll”-like receptor; MPLA, monophosphoryl lipid A. Figure modified after (17).
2 Adjuvants approved for human use in AIT
2.1 Aluminum hydroxide
Since Glenny et al. reported that alum could be used as an adjuvant in 1926 (23), it has become the most widely used adjuvant for different licensed human vaccines to prevent infectious diseases, such as diphtheria, tetanus, pertussis, hepatitis B, anthrax, and influenza (24). Alum adsorbs proteins via electrostatic interaction between alum and the proteins hydroxyl groups, thereby reducing allergen diffusion (lowering the chance of anaphylactic reactions) and prolonging the exposure of immune cells to these antigens at the injection site. Accordingly, alum has been included in most subcutaneous allergy immunotherapy (SCIT) products (25) in order to increase immune responses (12).
Recently, alum crystals were reported to also directly activate the NLRP3 inflammasome in mouse and human dendritic cells (DCs) and macrophages in vitro, resulting in secretion of the strongly pro-inflammatory and pyrogenic cytokines IL-1β and IL-18 [reviewed in (24)]. While mouse and human alum-stimulated DCs were shown to promote CD4+ T cell differentiation, especially towards Th2 cells via a prostaglandin E2-dependent mechanism [reviewed in (24, 26)], accumulated evidence from human application suggests alum to induce a more balanced Th1/Th2 immune profile depending on the formulation and the co-applied antigen (27, 28).
However, the use of alum is not without disadvantages. Alum frequently induces acute and chronic inflammation at the injection site, has a low biodegradability resulting in its accumulation upon repeated application (26, 29), and may have toxic effects; therefore, the application of alum as an adjuvant for type I allergy treatment is under ongoing investigation (30, 31).
2.2 Calcium phosphate
Calcium phosphate was first described as an adjuvant by Relyveld and colleagues in the 1960s (32–34). Although it is less commonly used than alum (35), it was used in AIT against seasonal rhinoconjunctivitis (36) as well as in vaccines against diphtheria, tetanus, pertussis, and poliomyelitis (37). Currently, because of reasons unknown to us, calcium phosphate adjuvanted AIT products are no longer used in the European market (Stallergenes Greer, personal communication).
Calcium phosphate is a natural component of the body with a better biodegradability and biocompatibility compared to alum, preventing its accumulation in the body upon repeated application (38, 39). While calcium phosphate displays a lower adjuvant activity than alum, it may still induce local adverse reactions, although of shorter duration (40). Upon subcutaneous injection, calcium phosphate induces active inflammation, involving the infiltration of neutrophils and macrophages (40). Earlier studies reported the induction of a similar IgG production in both animals and humans compared to alum (41, 42). However, the major advantage of calcium phosphate is its inability to induce the production of IgE in booster vaccinations (43) and immunotherapy (44). Furthermore, Wang et al. could show the induction of a cellular and humoral immune response as well as anti-cancer immunity upon the use of calcium phosphate-containing nanoparticles for vaccination purposes (45).
2.3 Microcrystalline tyrosine
MCT, the crystalline form of the non-essential amino acid L-tyrosine (46), is used as an adjuvant for AIT (47). Although MCT is less commonly used than alum (35), MCT has been shown to be both safe and effective for use in humans (46). It is a biodegradable depot adjuvant with a short half-life of 48 h (48), used in ultra-short course AIT for seasonal rhinitis (49, 50). The depot effect results in the slow release of the respective allergen(s), thereby prolonging their exposure to the immune system at the injection site (51).
Co-application of MCT with ovalbumin (OVA), a low immunogenic antigen, induces a B cell response leading to the production of antigen-specific IgG1, IgG2a, IgG2b, and IgG3 antibodies in mice that is comparable to alum, while inducing lower levels of both IgE and IL-4 (47). It induces lower levels of Th2 polarization compared to alum, which facilitates its use in AIT (52). Despite its more Th1-promoting adjuvant effects, MCT may induce both immediate and late local adverse reactions in patients undergoing AIT (53) and causes fewer, but still detectable, anaphylactic reactions in mice compared to alum (47). As was reported for alum, MCT was shown to induce caspase-dependent IL-1ß secretion in vitro, whereas the activation of the inflammasome was not essential for MCT-induced T or B cell response in vivo (47). In contrast to the TLR4-ligand monophosphoryl lipid A (MPLA) (see below), the promotion of T and B cell responses by either MCT or alum is independent of Toll-like receptor (TLR) signaling (47).
In AIT, MCT-adjuvanted native allergens or modified allergens (allergoids) are frequently used in combination with the TLR4-agonist MPLA, e.g. in the allergen therapeutic Pollinex® Quattro (PQ, Bencard Allergy GmbH, Munich, Germany) for the treatment of seasonal allergic rhinoconjunctivitis (49, 54). The combination of MCT as a Th1 promoting, first-generation adjuvant and MPLA as a Th1-promoting second-generation adjuvant in AIT has promoted dose reductions (55). Since MCT boosts T-cell mediated responses (47), its use in vaccination against different infectious diseases is currently investigated (56–58). Here, MCT-adjuvanted nanoparticles induced enhanced B and T cell responses in pre-clinical models of malaria (57, 58) and cancer (59).
2.4 Monophosphoryl lipid A
MPLA is a carbohydrate-based adjuvant that activates TLR4 (60). It was developed by removing phosphate and fatty acid groups from the lipopolysaccharide (LPS) of Salmonella minnesota R595 by a series of organic extractions followed by mild acid and alkaline treatments (61). Although the TLR4-ligand LPS efficiently promotes immune responses when combined with antigens, its applicability as an adjuvant is strongly limited by its high toxicity (62). In contrast, MPLA maintains the adjuvant effect observed for LPS combined with a lower toxicity on human CD4+ T cells in vitro (63), and did not cause dangerous adverse effects in a human clinical trial (64). Therefore, several vaccines containing MPLA have either been tested in clinical trials or are already approved for human use like Fendrix® (vaccination against hepatitis B virus), Cervarix® (human papillomavirus-16 and -18), RTS,S® (Malaria), and Pollinex® Quattro (grass pollen allergy, in Germany only marketable according to therapy allergen ordinance but no marketing authorization) (50, 65–67). The overall immunological effects of MPLA are relatively well studied. It is known that MPLA can induce Th1-biased immune responses and promote both IgG1- and IgG4-dominated humoral immune responses without boosting IgE production (68, 69) and without the capacity to directly activate murine or human mast cells (70), making MPLA an attractive adjuvant for improving AIT. In vitro, MPLA was also shown to induce a pronounced, c-Jun N-terminal kinase mitogen-activated protein kinase (JNK-MAPK)- and mammalian target of rapamycin (mTOR)-dependent activation of glucose metabolism, IL-10 secretion, and CCL2 production in mDCs (71).
PQ is an allergen therapeutic containing glutaraldehyde-crosslinked pollen allergoids adsorbed to MCT that are further adjuvanted with MPLA (72). PQ was first developed in the 1970s for the treatment of grass pollen allergy, followed by the Pollinex-R, which included ragweed allergens (72). PQ was launched on the market in 1999 as named patient products for the treatment of allergic rhinitis (AR) caused by grass-, tree-, olive-, and weed allergens but so far has not received marketing authorization in Germany (72). Compared to traditional AIT approaches that normally require months to years of treatment, PQ is based on a “short-term specific immunotherapy (ST-SIT)” consisting of only four pre-seasonal injections with increasing allergen dosages (18). Clinical studies showed, that PQ treatment significantly reduced nasal and ocular clinical symptoms, while also shifting immune responses from allergic Th2- toward Th1-biased responses characterized by higher grass-pollen-specific IgG antibody production (18). Moreover, pre-clinical in vivo studies in rats and dogs showed no toxicological findings, and no significant local and systemic adverse events were reported in human clinical trials (18, 73).
Our own work demonstrated that both PQ and two commercial vaccines adjuvanted with MPLA could activate mDC metabolism (74). Activation of mDCs by PQ was mediated by a pronounced mTOR- and JNK-MAPK-dependent activation of glucose metabolism that regulated mDC-derived cytokine secretion (74). Finally, mDC glucose metabolism was also critical for the (Th1-biased) T cell priming capacity of PQ-stimulated mDCs (74).
Taken together, the available data for PQ show that combining allergen(s) with the adjuvants MPLA and MCT may improve major disadvantages of traditional AIT regarding long treatment periods, low patient adherence, and occurrence of side effects. However, so far, sufficient clinical human data fully supporting marketing authorization in Germany have not been reported. Moreover, as of January 2024, PQ has not been approved for marketing in any other European state (Bencard, personal communication).
3 Adjuvants currently investigated for use in human AIT
3.1 Liposomes
Liposomes are biodegradable nanoparticles (see (75) for a review of nanoparticles in AIT) that have been considered as adjuvants combining immune cell activation with effective packaging and delivering water-soluble antigens to target cells (76). Despite their potent immune-stimulatory capacity, liposomes may also cause severe side effects such as toxicity to cells of the mononuclear phagocyte system (monocytes, macrophages, and DCs), reducing the secretion of immune effector molecules by these cells, complement activation-related pseudoallergy, and organ damage to liver and spleen upon intravenous (i.v.) application [reviewed in (77–79)].
The potential of liposomes to carry allergens was first tested in 1991 by Audera’s group (80). In their experiments, house dust mite (HDM)- (Dermatophagoides pteronyssinus), grass- (Lolium perenne, Phleum pratense, Parietaria judaica, and Artemisia vulgaris), and cat dander-allergen extracts with or without liposome encapsulation were injected into BALB/c mice (80). Their results showed that liposome-encapsulated allergen extracts could induce higher levels of allergen-specific IgG paralleled by a lower IgE production (80). One disadvantage when using liposomes is their lack of inherent immune-activating capacity, which can be overcome by combining liposomes with additional adjuvants. For this, one liposome-based formulation, the commercial adjuvant system 01 (AS01), which additionally contains the TLR4-ligand MPLA (see above) and the natural saponin product QS-21 (see below) has already been approved for use in malaria- (MosquirixTM) and shingles (Shingrix(R)) vaccines (81). Besides, a recent in vivo study also encapsulated CpG oligodeoxynucleotides (CpG-ODN), a synthetic TLR9-agonist, together with OVA in liposomes to evaluate their potential for allergy treatment (82). Their results showed that OVA encapsulated in liposomes could significantly reduce cutaneous anaphylactic reactions in an in vivo OVA-induced asthma mouse model (82). Moreover, liposomes encapsulating OVA and CpG-ODN, but not the OVA plus CpG-ODN mixture alone, reversed OVA-induced allergic lung inflammation in a mouse model (82). The inflammation-suppressing effect of the liposomes was shown to be myeloid differentiation primary response protein 88 (MyD88)-dependent, and mediated by CD11c+ DCs (82). Therefore, liposomes may have potential as carriers that encapsulate allergens and other adjuvants to improve allergy treatment.
3.2 Oil-in-water emulsions
Besides alum and liposome-based AS01, two oil-in-water (O/W) emulsion adjuvants AS03 (containing squalene, alpha-tocopherol, and polysorbate 80) and MF59 (consisting of squalene and the non-toxic emulsifiers Tween 20 and Span 85), have also been licensed for clinical use, especially for various influenza vaccines (83). Mechanistically, O/W emulsions have the advantage of gradually releasing the combined antigen at the injection site, which reduces the chance of anaphylactic reactions while at the same time stimulating the activation of plasma cells producing antigen-specific antibodies and generating mixed Th1/Th2 responses (84, 85). Moreover, MF59 was also shown to induce DC recruitment and increase their antigen uptake activity in vivo (86). Potential safety risks concerning AS03 were raised after the use of the influenza vaccine Pandemrix(R) (adjuvanted with AS03) during the global indluenza A H1N1 pandemic in 2009. Pandemrix(R) was later found to be associated with increased frequencies of narcolepsy in patients with an HLA-DQB1*0602 haplotype (reviewed in (87)). Mechanistically, the vaccine was suggested to increase the frequency of antibodies to the hypocretin (HCRT) receptor 2 that both cause sleep dysregulation via a loss of HCRT-producing neurons and cross-react with a particular fragment of influenza nucleoprotein (reviewed in (87)).
Currently, there are limited studies regarding the use of O/W as adjuvants to improve AIT. Recently, O’Konek and Baker, Jr. described nanoscale O/W emulsions (NE), which induced potent Th1- and Th17-polarized immune responses (88). In vivo, treatment of mice with NE-formulated peanut allergens (PN-NE) suppressed allergic responses after both oral or systemic peanut allergen challenge (88). Furthermore, a decreased production of the Th2 cytokines IL-4 and IL-13, as well as a higher production of the Th1 cytokine IFN-γ and the anti-inflammatory cytokine IL-10, were observed in PN-NE-treated & peanut-allergic mice (88).
3.3 Virus-like particles and viral components
Virus-like particles (VLPs) are multimeric entities that have the morphology of a native virus but do not contain viral genomic material and are therefore unable to replicate in vivo. Consequently, VLP-based vaccines have shown an improved safety profile compared to either live-attenuated or inactivated vaccines (89). They can serve as effective delivery carriers while offering strong immune-modulating capacity due to their highly repetitive and ordered structure (90). Several VLPs have already been licensed for clinical use: Cervarix®, Gardasil®, and Gardasil9® for the prevention of human papillomavirus (HPV) infection, Hecolin® for the prevention of hepatitis E, Recombivax HB® and Sci-B-Vac™ for hepatitis B, and Mosquirix™ for malaria (90, 91). VLPs have also been studied for allergy treatment and the current strategies for using VLPs are reviewed in other publications (90). In brief, VLPs can be applied (I) alone, (II) packaging immune-stimulatory CpG-motifs, (III) display either cytokines or (IV) allergen(s) on their surface, (V) co-display both allergen(s) and immune-modulating proteins on their surface, (VI) package allergen(s) inside the VLPs, or (VII) mixed with allergen(s) to improve AIT (Figure 3) (90). Up to now, several studies applying different VLPs (except for VLPs displaying transforming growth factor beta 1 (TGF-β1) (95)) showed potential to improve allergic responses in allergic animal models in vivo, including reduced mast cell activation, suppressed Th2- while promoting Th1 responses, and upregulation of neutralizing antibody production [Figure 3 and reviewed in (90)].
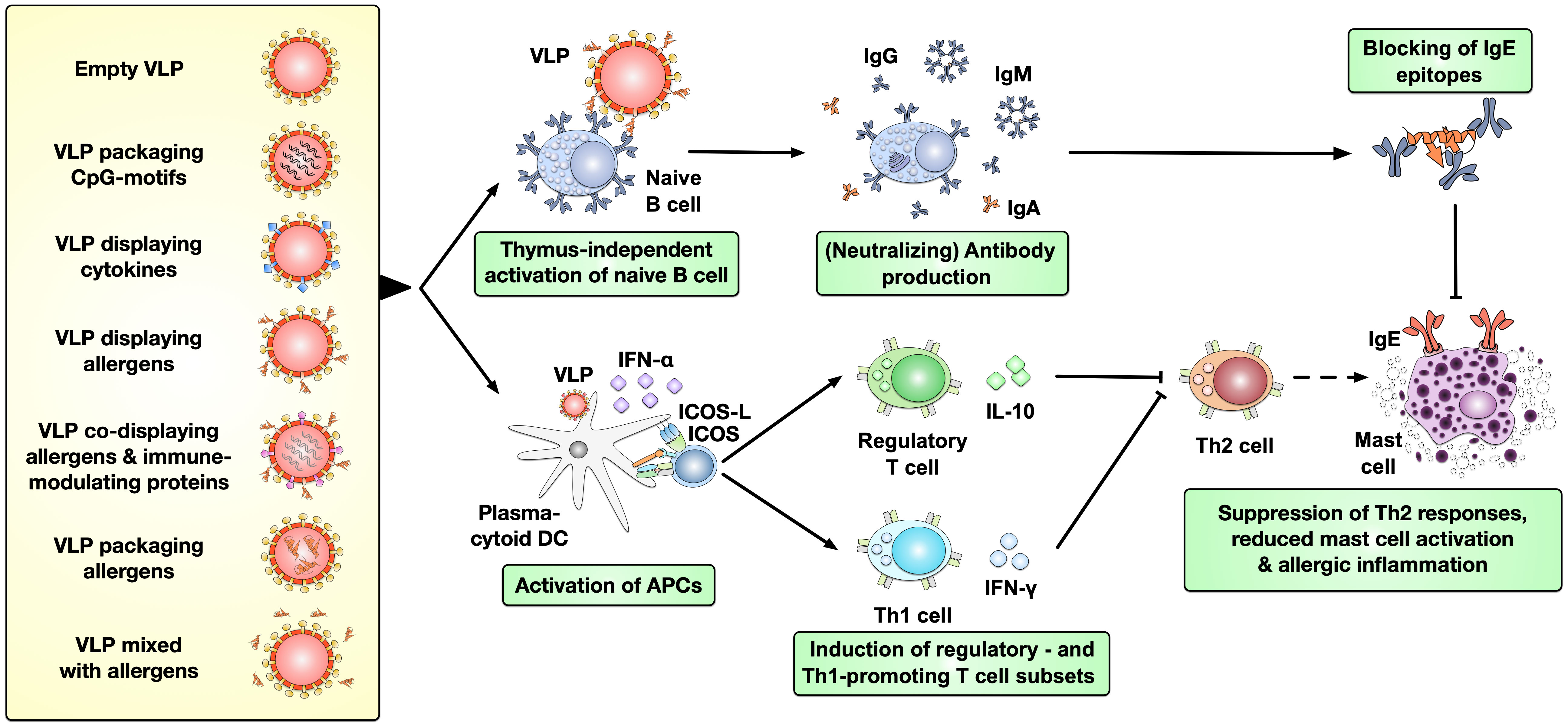
Figure 3 Types of VLPs investigated pre-clinically in AIT and their immunological effects. VLPs have been applied in pre-clinical studies to improve the treatment of allergies either as empty particles, packaging immune-stimulatory CpG-motifs, displaying either cytokines or allergen(s) on their surface, co-displaying both allergen(s) and immune-modulating proteins on their surface, packaging allergen(s) inside the VLPs, or mixed with allergen(s). VLPs were shown to thymus-independently activate naïve B cells, resulting in production of potentially neutralizing antibodies while also promoting the activation of plasmacytoid DCs (pDCs) (92–94). These APCs in turn favor the induction of regulatory- and Th1 cells which suppress the allergy-causing Th2 cells. Together these effects of VLPs may result in reduced mast cell activation and allergic inflammation [reviewed in (90)]. (p)DC, (plasmacytoid) dendritic cell; VLP, virus-like particle; IgG/A/E, immunoglobulin G/A/E.
The largest number of clinical studies on VLPs in the field of allergology have been performed on VLPs derived from the bacteriophage Q beta (QβG10, all financed by Cytos Biotechnology AG, Schlieren, Switzerland). These form particles of approximately 30 nm in size that were used to encapsulate approximately 60 molecules of immune-stimulating A-type CpG ODN (96). In contrast to B-type CpGs ODNs, A-type CpG ODNs were described to be less stable and induce the secretion of interferon alpha (IFN-α) from plasmacytoid DCs (pDCs) rather than IL-12 (96).
In an open-label phase I pilot trial 20 patients with HDM allergy were treated for 10 weeks with a mixture of QβG10 (termed CYT003) and an alum-adsorbed HDM extract (96). Here, the combination of QβG10, alum and allergens resulted in a 10-fold reduction in self-reported symptoms while at the same time increasing HDM-specific IgG1, IgG2, and IgG4 antibody production compared to before the treatment (96). These observations encouraged larger placebo-controlled trials, the first of which was published in 2011 by Klimek and coauthors. In a double-blind, randomized, placebo-controlled clinical trial enrolling 299 patients with HDM allergy, patients were treated for two months with either 0.5 or 1 mg of QβG10 alone. The authors reported QβG10 to dose-dependently reduce the average combined symptom medication score, while the dose of 1 mg QβG10 also resulted in a higher number of patients with increased allergen tolerance (97). Therefore, the authors suggested QβG10 to be an “allergen-independent treatment with favorable safety profile and therapeutic treatment benefit within 2 months” (97). In the second reported parallel-group, double-blind, randomized trial, 63 asthmatic patients undergoing steroid withdrawal were treated with 0.9 mg QβG10 (without additional allergen) (98). Also in this patient collective, QβG10 was reported to reduce asthma-related symptom scores while increasing the number of patients with well-controlled asthma (98). However, in a final double-blind phase IIb study with 365 patients with not sufficiently controlled, persistent moderate-to-severe allergic asthma, Casale et al. reported both no significant difference between the QβG10 treatment and placebo groups at week 12 and no significant differences in secondary outcomes (19). Therefore, the study was prematurely terminated due to a lack of efficacy compared to patients receiving standard inhaled glucocorticosteroid therapy with or without long-acting beta-agonists (19). It was stated by the authors that “continued clinical development of CYT003 in this indication is unlikely, but these results should not preclude further exploration of immune-modifying therapies in allergic asthma” (19).
In a related approach, Storni et al. under participation of Allergy Therapeutics Ltd, (Worthing, UK), recently published data on optimized cucumber mosaic virus (CuMV)-derived VLPs displaying allergen(s) (99). These VLPs encapsulate immune-stimulatory E. coli RNA while at the same time co-displaying tetanus toxin-derived epitopes (as universal antigens inducing recall responses in formerly tetanus-vaccinated individuals) as well as either the major peanut allergens Ara h 1, Ara h 2, or roasted peanut extract (99). In BALB/c mice sensitized with peanut extract plus alum intraperitoneal (i.p.), vaccinated with CuMV-VLPs s.c., and challenged with peanut extract i.v., CuMV-VLP treatment strongly reduced the peanut-induced temperature drop (99). Here, CuMV-VLPs displaying either Ara h 1, Ara h 2, or roasted peanut extract showed a similar efficacy, suggesting that vaccination against a single allergen was able to protect against challenge with the whole extract (99). In mechanistical studies, the CuMV-VLPs displaying Ara h 1 were shown to mediate their protective effects via a strong induction of peanut-specific IgG2a and IgG2b antibodies that down-regulated mast cell activation via FcγRIIB-dependent inhibitory signaling (99).
Finally, a phase I clinical trial sponsored by Allergy Therapeutics Ltd was initiated in January 2022 to analyze VLPs that display the major peanut allergen Ara h 2 for treating peanut-allergic patients (https://clinicaltrials.gov/ct2/show/NCT05476497). This trial may provide more insights in the potential of VLPs for allergy treatment.
Besides VLPs, viral components which have immune-activating capacity have also been proposed as adjuvants for allergy treatment. For example, the hepatitis B virus (HBV)-derived PreS T-cell epitope fused with different allergens was tested both in vivo (in mice and rabbits) (100, 101) and in a human phase II clinical trial sponsored by Biomay AG (Vienna, Austria) (20, 102). Two non-allergenic rFel d 1 (the major cat allergen)-derived peptides fused with PreS strongly suppressed basophil activation while inducing production of Fel d 1-specific IgG antibodies after injection into either mice or rabbits (101). Moreover, a fusion protein consisting of major birch pollen allergen Bet v 1-derived peptides and PreS (rBetv1:PreS) also induced higher production of Bet v 1-specific IgG antibodies from rabbits (100). More detailed in vitro analyses demonstrated rBetv1:PreS to reduce T cell activation in PBMCs from allergic patients, which was paralleled by both higher anti-inflammatory IL-10- and Th1 cytokine IFN-γ production (100). Moreover, BM32, a vaccine candidate fusing PreS with hypoallergenic peptides derived from several major timothy grass pollen allergens adsorbed to alum induced allergen-specific IgG4 responses in a two-year AIT approach (phase II study, sponsored by Biomay AG) (20, 102). BM32 also demonstrated a good safety profile with no reported anaphylactic reactions and T cell-mediated side effects while reducing both IgE reactivity and allergen-specific Th2 cytokine secretion during the two years of treatment (20).
In addition to PreS, there are also two more pre-clinical mouse studies that generated fusion proteins combining viral proteins and allergen-derived peptides: Salari et al. used the HIV type 1 (HIV-1) trans-activating regulatory protein (TAT) fused with Chenopodium album pollen allergen Che a 3 (rTAT-Che a 3) (103), and Edlmayr et al. used rhinovirus viral protein 1 (VP1) fused with the major grass pollen allergen Phl p 1 peptide P5 (rVP1-P5) (104). Both approaches demonstrated the induction of higher allergen-specific IgG antibody production in mice by the respective fusion proteins (103, 104). Moreover, rTAT-Che a 3 was shown to enhance Treg-mediated immune responses, including lower production of the Th2 cytokine IL-4 as well as enhanced secretion of the Th1 cytokine IFN-γ (103). On the other hand, rVP1-P5 induced grass pollen-specific Th1-biased immune responses with reduced Th2 activation were observed in in vitro splenocyte cultures (104). Both studies were pre-clinical not reporting potential side effects, but Edlmayr and colleagues reported rVP1-P5 to not react with IgE antibodies from grass pollen allergic patients, to lack allergenic activity upon contact with basophils from allergic patients, and to induce protective IgG antibodies in mice or rabbits that blocked both IgE reactivity to Phl p 1 and Phl p 1-induced basophil degranulation (104).
In summary, these findings show that VPLs and certain viral proteins may be used as adjuvants for generating allergy vaccines.
3.4 Carbohydrate-based adjuvants
Carbohydrates are the most common type of biomolecule that can be found in nature. Accordingly, they have an important role in modulating both innate and adaptive immune responses (105). Carbohydrates combine a high biocompatibility with low toxicity, making them interesting novel adjuvant candidates. Currently, two carbohydrate-based adjuvants, QS-21 and MPLA (already discussed above), have already been licensed for clinical use. Moreover, several different other compounds have been investigated. The current state of knowledge is briefly summarized below.
3.4.1 Saponin-based adjuvants (QS-21)
QS-21 is a triterpene glycoside that can be purified from the soap bark tree (Quillaja saponaria) (106). It has been tested as a therapeutic vaccine adjuvant in clinical trials against cancer (lymphoma, leukemia, melanoma, breast-, prostate-, ovary-, or lung cancer) and infectious diseases (HIV-1 and hepatitis B) (81, 107).
The mechanisms underlying the adjuvant effects of QS-21 are not extensively studied. We know that induction of immune responses by QS-21 is mediated by a pronounced activation of innate immune cells: upon injection, QS-21 activates subcapsular CD11b+CD169+ macrophages in draining lymph nodes that in turn recruit neutrophils and DCs (108, 109). In DCs, QS-21 in turn triggers a MyD88-dependent inflammasome activation that involves a partial activation of the high-mobility group protein B1-TLR4 (109) and cathepsin B (shown for QS-21 in AS01), an inflammasome-dependent release of IL-1β, and the activation of both CD4+ and CD8+ T cells, and production of antibodies ((109) and reviewed in (110)). While QS-21 did not bind to either TLR2 or TLR4 (111), both lysosomal destabilization and spleen tyrosine kinase (Syk) activation were shown to be essential for both QS-21-mediated activation of monocyte-derived DCs (108), antigen cleavage, and subsequent presentation to CD8+ T cells (81)
However, there are several drawbacks that limit the application of QS-21, including (I) strong side effects when using high-dosages (e.g. local erythema, induration, and flu-like symptoms); (II) its chemical instability, especially in warm temperatures and pH values higher than 7.4; and (III) limited supplies due to difficulties extracting highly pure QS-21 from its natural source (107).
Therefore, in current clinical usage, QS-21 needs to be co-applied with other adjuvants and is most commonly applied packaged in liposomes in combination with MPLA (a TLR4-ligand, see above) as AS01. A study from Welsby et al. compared the effects of either QS-21 or MPLA on human DCs in culture, which were both packaged independently of each other in liposomes (108). Their results showed, that both QS-21- and MPLA-liposomes could induce higher IL-6, TNF-α, and IL-8 secretion, as well as CD86 expression (108). Interestingly these two liposome-packaged adjuvants showed different kinetics on cytokine production: MPLA-liposomes induced fast DC activation (two to four hours post-stimulation) while for QS-21-liposomes DC activation was observed at later time points (six hours post-stimulation) (108). Using microarray analysis the authors found, that QS-21- and MPLA-liposomes activated similar pathways such as cytokine-, NLR, and G-protein-couple receptor-signaling in human DCs (108). In contrast, the activation of the activator protein 1 (AP-1) and activating transcription factor 2 (ATF2)-transcription factor networks were found to be specific for QS-21-liposomes, while MPLA-liposomes specifically induced TLR- and IL-1-signaling in human DCs (108). The observed DC-activation by QS-21-liposomes was shown to depend on cholesterol-mediated endocytosis followed by lysosomal destabilization (108), which was different from MPLA since it is known to activate TLR4-signaling (60).
Although QS-21 is a strong adjuvant, it is well tolerated in clinical trials with systemic side effects being infrequent: Compared to placebo, QS-21 increased injection site pain and occurrence of diarrhea in two Pfizer-sponsored Alzheimer studies (NCT00479557 and NCT00498602) (meta-analysis in (112)). QS-21-mediated injection site pain was also reported in three phase I trials by Waite and colleagues (113) and upon intra-muscular application of QS-21 mixed with HIV-1 glycoprotein 120 (114). Also Gilewski reported local skin reactions at the injection site (duration 4 to 5 days) and mild flu-like symptoms (duration 1 to 2 days) upon application of a QS-21-adjuvanted vaccine to prevent breast cancer (115).
Although there is currently no study testing QS-21 as an adjuvant for allergy treatment, QS-21 was shown to induce Th1-biased immune responses with high titers of IgG2a and IgG2b antibodies (81), making it an attractive candidate for future research.
3.4.2 Glucans
Glucans are polysaccharides derived from either plants or microorganisms (such as bacteria, algae, or fungi) that are composed of D-glucose units linked by different glycosidic bonds (Figure 4) (116). Glucans encompass several different α-glucans (e.g., dextran, glycogen, pullulan, and starch) and β-glucans (e.g., cellulose, curdlan, laminarin, chrysolaminarin, lentinan, lichenin, pleuran, and zymosan) (116). The different glucan families considerably vary in structure, type of linkage, and length of the macromolecules. The mechanisms by which glucans activate immune cells are not fully understood. However, some glucans were reported to bind to either carbohydrate receptors or TLRs expressed on the surface of APCs, suggesting these molecules to have adjuvant potential (116). For example, Dillon et al. demonstrated that yeast zymosan could bind to both the Dectin-1 receptor and TLR2 on human and murine DCs, which induced higher secretion of IL-12(p70), IL-6, and IL-10 in vitro (117). Moreover, two studies used β-glucans chemically conjugated with either the detoxified diphtheria toxin CRM197 or the cancer-associated mucus protein mucin 1 as novel vaccines against either diphtheria or cancer (118, 119). Their results showed that, as adjuvants, β-glucans can serve as both carriers and immune activators promoting immune responses in vitro and in vivo, which enhanced the delivery of antigens by binding to APCs and enforced antigen-specific antibody production (118, 119).
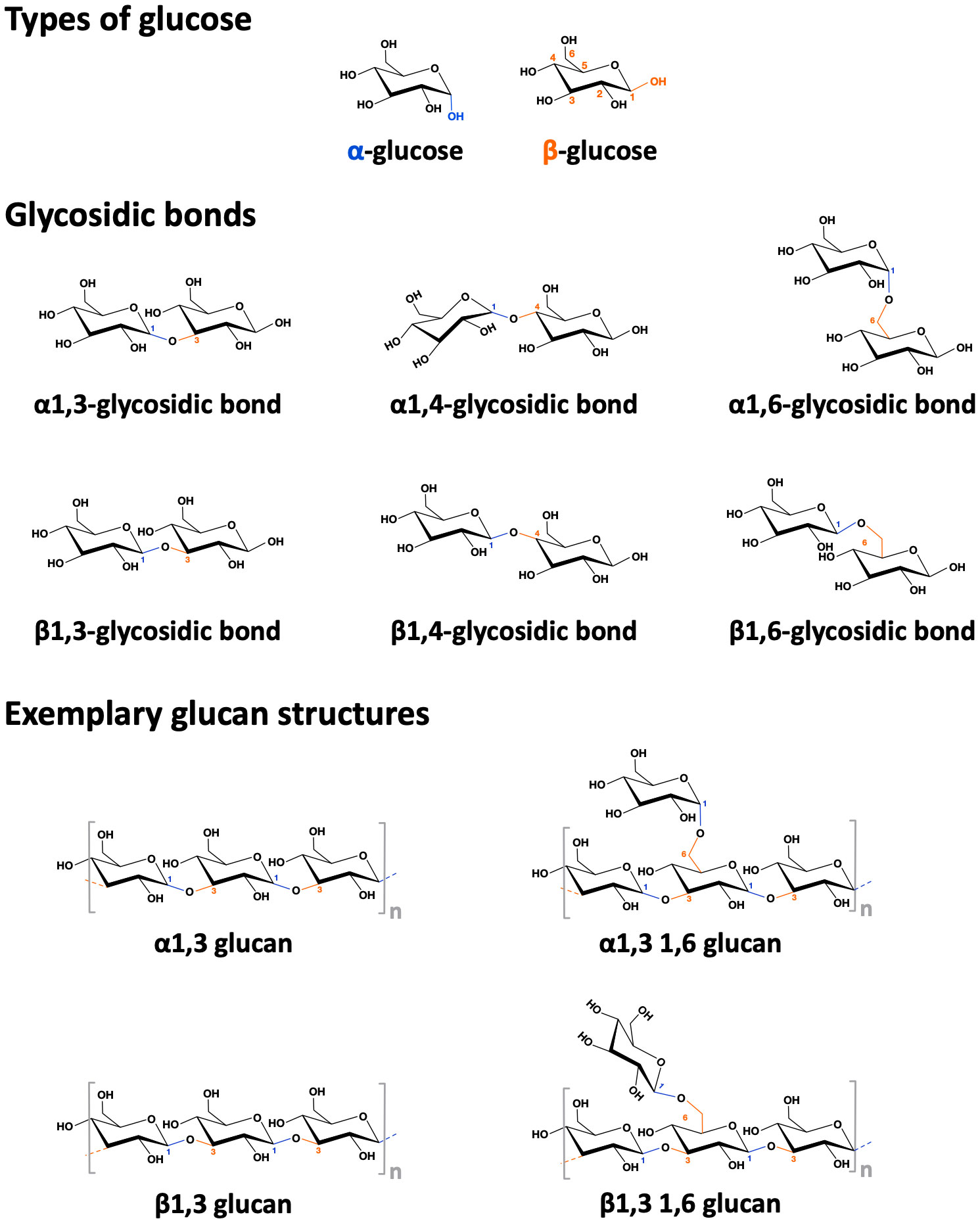
Figure 4 Structure of alpha- and beta-glucans. Depicted the difference between alpha- and beta-(D)-glucose, the different types of glycosidic bonds, and some simplified exemplary alpha- and beta-glucan structures.
The potential of glucans for allergen-specific immunotherapy is still controversial. Some researchers demonstrated β-(1,3)-glucans, which can be found in either HDM feces or pollen grains derived from several plant species, to exacerbate allergic inflammation by activating DCs in vitro and enhancing allergen-specific IgE antibody as well as Th2 cytokine production in vivo (120, 121). However, in another in vivo OVA-based food allergy mouse model, the authors applied either the low-molecular-weight β-(1,3)- or a 50–80% branched β-(1,6)-glucan (purified from Aureobasidium pullulans). Here, these two β-glucans were shown to suppress OVA-specific IgE production (122). In a non-industry-sponsored, multicenter open split-body study enrolling 105 patients with atopic dermatitis, regular topical application of a 0.25% β-glucan-based cream (Imunoglukan P4H® cream) over a period of 24 weeks reduced pruritus and severity of atopic dermatitis while increasing the number of days without exacerbation (123). The β-glucan-containing cream was well tolerated with only mild local side effects (contact dermatitis and other undisclosed skin side effects) that disappeared during regular application (123). In a randomized, double-blind, parallel group, placebo-controlled study without industry sponsorship 26 patients with seasonal AR sensitized to olive pollen were treated orally with 10 mg β-(1,3–1,6)-glucan twice a day for 12 weeks (124). β-glucan treatment significantly reduced levels of Th2 cytokines and eosinophils in nasal lavage fluid while increasing levels of IL-12 (124). Recently, a β-(1,3)-glucan and a β-(1,3-1,6)-glucan both derived from Saccharomyces cerevisiae were investigated in an OVA-based mouse intestinal allergy model: Here, oral treatment with β-(1,3)-glucan (but not β-(1,3-1,6)-glucan) was shown to further promote intestinal inflammation and allergic responses (125), suggesting that this β-(1,3)-glucan might not be suitable for immunotherapy purposes. Gut microbiome analysis in the allergen-sensitized and β-(1,3)-glucan fed group showed an increased ratio of Firmicutes to Bacteroidetes, a potential indication of dysbiosis (125). In a phase IV clinical study (sponsored by the Meiji University of Oriental Medicine, Kyoto, Japan) oral-administration of superfine dispersed β-(1,3)-glucan (particle-size: 0.08 μm), instead of non-dispersed shiitake extract (particle-size: 288 μm), was shown to alleviate allergic symptoms like rhinorrhea, sneezing, nasal congestion, and itchy watery eyes otherwise induced by Japanese cedar pollen in allergic patients (21). Along with the reduction of the aforementioned clinical symptoms, the superfine dispersed β-(1,3)-glucan treatment group also displayed lower pollen-specific IgE antibody levels (21). Based on these findings the authors speculated, that differences in size, structure, and purification method of carbohydrates derived from natural sources might lead to distinct immune responses (21, 122). Therefore, more detailed studies are needed to understand the adjuvant potential and potential side effects of glucans.
3.4.3 Mannan
Mannan is a β-(1,4)-mannose polysaccharide which can be found in the cell walls of both plant and fungal cells (81). Mannan can modulate immune responses towards both Th1 and Th2 responses (depending on its oxidation status, reduced mannan: Th2, oxidized mannan: Th1) by binding to both mannan- and C-type lectin receptors (DC-SIGN) expressed e.g., by APCs (116), which leads to activation of complement pathways (116), the NLRP3 inflammasome, as well as increased phagocytosis and cytokine secretion in APCs (81, 116). The first study using mannan as a vaccine adjuvant in mice was reported in 1992. Okawa et al. conjugated mannan with the HBV 139-147 peptide (126). The mannan:HBV peptide conjugate was shown to induce higher IgG titers in mice than corresponding dextran:HBV peptide-conjugates (126). So far, either native, oxidized, or reduced forms of mannan have been conjugated to different antigens to be tested as adjuvants for targeting APCs (reviewed in (81)). The results demonstrated both higher antigen uptake and presentation by human monocyte-derived DCs (127) coupled with the induction of distinct antigen-specific Th1- or Th2-responses in mice (128, 129).
There are some studies investigating mannan as an adjuvant for improving allergen immunotherapy. Weinberger and colleagues used oxidized mannan derived from the yeast Saccharomyces cerevisiae to generate reactive aldehyde groups for covalent attachment of OVA via amine-containing amino acid residues (MN–Ova) (130). Compared to OVA alone, MN–Ova was taken up more strongly by mouse DCs both in vitro and in vivo, while also inducing higher IgG antibody production in mice at the injection site (130).
In a second set of studies, the native form of Saccharomyces cerevisae mannan was conjugated to defatted grass pollen grain-based allergoids from Phleum pratense (PM) (127, 131, 132). PM reduced skin prick test reactivity compared to either grass pollen allergens or grass pollen-based allergoids in a phase II trial (sponsored by Inmunotek SL, Madrid Spain)(Figure 5) (127). Immunologically, in vitro stimulation of human monocyte-derived DCs (hmoDCs) with PM induced higher secretion of IL-6 and IL-10 paralleled by a lower production of the Th2 cytokine IL-4. PM additionally promoted a mTOR-dependent increase in hmoDC glycolysis, and was more efficiently taken up compared to either pollen extracts or pollen allergoids (Figure 5) (127, 131, 132). In vivo, PM promoted tolerogenic immune responses characterized by the differentiation of CD4+CD25highFOXP3+ Treg cells and increased pollen-specific IgG2a/IgE ratios in mice (Figure 5) (127). These immune-modulating properties were strongly dependent on the intact structure of the mannan, as conjugates containing oxidized mannan showed a strongly reduced uptake, IL-10 secretion, programmed death-ligand 1 (PD-L1) expression, and frequency of induced Tregs (127).
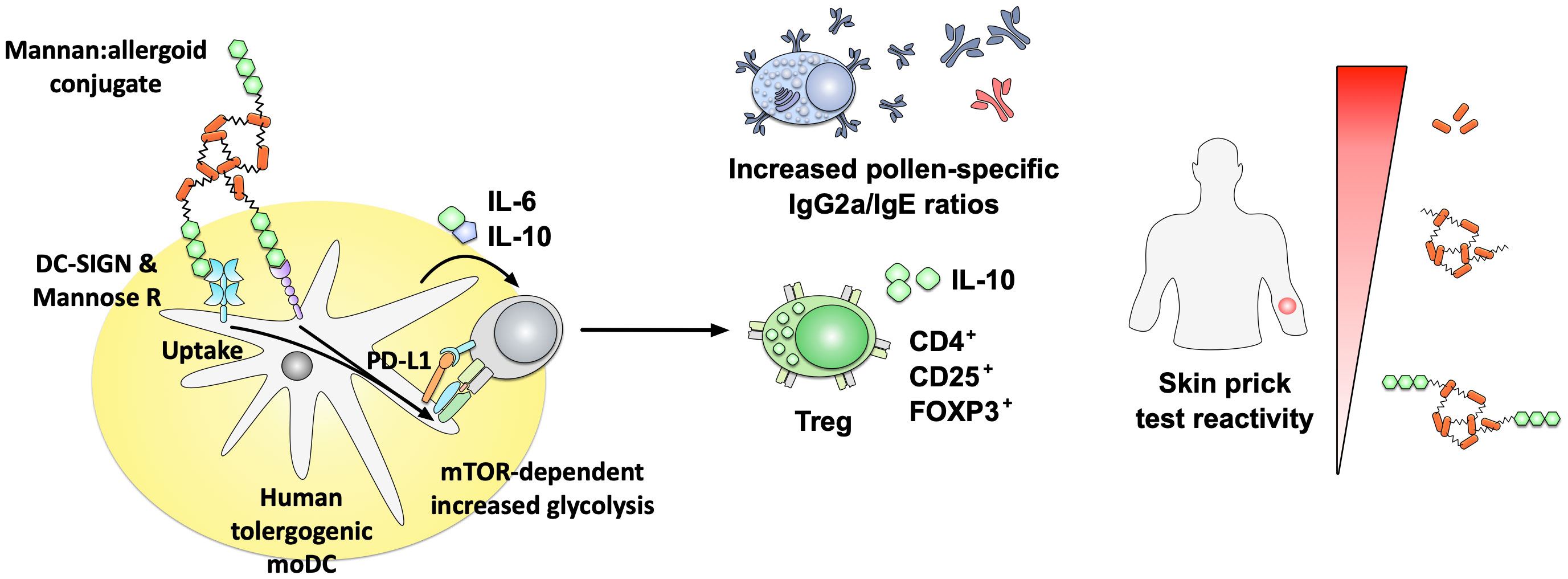
Figure 5 Immune modulatory properties of non-oxidized mannan:allergoid conjugates. Non-oxidized mannan:allergoid conjugates were shown to reduce skin prick test reactivity compared to either grass pollen allergens or grass pollen-based allergoids (right side). Immunologically, the mannan:allergoid conjugates taken up via DC-SIGN and mannose receptor induced higher secretion of IL-6 and IL-10 as well as surface expression of PD-L1 paralleled by an increase in mTOR-dependent glycolysis (left side). In in vivo analyses, mannan:allergoid conjugates stimulated the differentiation of CD4+CD25highFOXP3+ Treg cells, paralleled by increased pollen-specific IgG2a/IgE ratios. In grass pollen allergic patients, the mannan allergoid conjugates induced lower skin prick test reactivity compared to either non-modified allergens or the non-conjugated allergoids alone. Results summarized according to (127). For more information see text. DC-SIGN, dendritic cell-specific intercellular adhesion molecule-3-grabbing non-integrin; FOXP3, forkhead box protein 3; Mannose R, mannose receptor; mTOR, mammalian target of rapamycin.
In a follow-up pre-clinical study, Benito-Villalvilla et al. could show non-oxidized mannan conjugated to allergoids to induce the differentiation of tolerogenic DCs from monocytes (133). In the presence of IL-4, GM-CSF, and the mannan:allergoid conjugates, monocytes from both non-atopic and allergic donors differentiated into tolerogenic DCs with an increased mitochondrial oxidative phosphorylation (OXPHOS) and epigenetic reprogramming characterized by an open chromatin structure with both increased histone 3 lysine 4 (H3K4) trimethylation at the promotors of IL-10, PD-L1, suppressor of cytokine signaling 3 (SOCS3), and increased histone 3 lysine 27 (H3K27) acetylation at the promotor regions of PD-L1 and SOCS1 (133). Furthermore, monocyte-derived tolerogenic DCs expressed higher amounts of the microRNAs 146 a and b while having reduced levels of miR-155 and histone deacetylases (133). These tolerogenic DCs secreted IL-6 and IL-10 and promoted the differentiation of IL-10-producing CD4+CD25+FOXP3+ Tregs (133). These results suggest mannan, besides its direct immune activating effects, to also trigger extensive epigenetic modifications in the stimulated DCs that may (if also happening in their bone marrow progenitors) potentially result in long-term modification of the respective cells reactivity towards either mannan or the conjugated antigens (so called “trained immune responses”).
In a first multicenter, prospective, randomized, double-blind, double-dummy, placebo-controlled, phase II study encompassing 196 patients with HDM allergic rhinitis with or without asthma (financed by Inmunotek SL, Madrid, Spain), patients and healthy controls were treated with a HDM extract consisting of a mixture of 50% Der p and 50% Der f allergoids conjugated to non-oxidized mannan either sublingually or subcutaneously (22). Here, both s.c. and s.l. application of the mannan:allergoid conjugates increased the percentage of patients showing an improved nasal provocation test up to 50% and reduced combined symptom medication scores by 45 to 70%, depending on the dose and application route (22). Interestingly, s.l. application of the mannan conjugates induced lower levels of HDM-specific IgG4 antibodies than the s.c. application route (22). Reported side effects were grade I and II reactions (mostly delayed) with all grade II reactions occurring in the 1000 mTU/ml s.c. group (but not in groups receiving higher concentrations) (22). No grade III or IV systemic reaction were reported upon application of the mannan:allergoid conjugates (22). The majority of local reactions (observed in the s.c. treatment group) were mild and occurred after the first injections. However, six delayed reactions were described as severe and led to withdrawal of the respective patients from the trial (22).
Currently, three more phase II clinical trials (EudraCT nos. 2014-005471-88, 2018-002522-23, and 2020-004126-32) as well as two phase III clinical trials for allergoid-mannan conjugates for the treatment of patients with mild to moderate HDM-induced asthma and rhinitis/rhinoconjunctivitis (NCT05400811) or birch pollen-induced allergic rhinitis/rhinoconjunctivitis (EudraCT: 2021-002252-36) are either ongoing or finished. All studies are financed by Inmunotek SL (Madrid, Spain).
In summary, these studies demonstrated the potential of mannan as an adjuvant to improve AIT, confirming its efficacy in a first clinical study with more phase II and III studies currently ongoing.
3.5 Toll-like receptor agonists
Innate immune cells express a highly conserved set of PRRs (e.g., TLRs and NOD-like receptors (NLRs)) that directly recognize unique structures exclusively associated with foreign microorganisms, so-called PAMPs (134). After PAMPs bind to PRRs, innate immune cells become activated, present pathogen-derived peptides to naïve T cells, and initiate and regulate adaptive immune responses (134). In this context, different PRR-ligands induce distinct activation programs in APCs, resulting in the promotion of either Th1-, Th2-, and/or Th17-immune responses (134). Due to their intrinsic immune activating potential, PRR-ligands are highly interesting adjuvant candidates for future vaccine development. Here, we will present two TLR-ligands in more detail: the TLR5-ligand flagellin, and the TLR9-ligands CpG oligodeoxynucleotides, which have been studied for their potential to improve allergen immunotherapy.
3.5.1 Flagellin
The TLR5- and NLR family caspase activation and recruitment domain (CARD) containing 4 protein (NLRC4)-ligand flagellin is a bacterial motility protein forming the main body of the bacterial flagellum (135). Vaccines adjuvanted with flagellin were reported to be both safe and well-tolerated in clinical trials (136, 137) where flagellin was demonstrated to be an effective mucosal adjuvant triggering Th1-biased, protective immune responses in mice and monkeys (138–140).
Flagellin has also been analyzed pre-clinically as an adjuvant for improving traditional AIT. The available literature is divided into investigating (I) the mixture of flagellin and different allergens or (II) flagellin fused to allergens as part of fusion proteins. In this context, flagellin-containing fusion proteins were suggested as potential therapeutics combining the adjuvant activity of the TLR5- and NLRC4-ligand and the cargo antigen into a single molecule, allowing for the efficient targeting of antigens to, and simultaneous activation of TLR5+ APCs (140–143).
Lee et al. tested either intranasal or intralymphatic injection of Vibrio vulnificus flagellin B (FlaB) mixed with OVA in an OVA-based mouse model of airway inflammation (144, 145) where the OVA/FlaB mixture reduced OVA-induced airway hyper-responsiveness, inflammatory cell infiltration in lung tissue, as well as both systemic (IL-4, IL-5, IL-6, IL-17, and IFN-γ) and local (IL-4 and IL-5) cytokine production; while increasing OVA-specific IgA levels in serum (144, 145). Mechanistically, the ability of the OVA/FlaB mixture to suppress allergic responses was found to be dependent on TLR5 (146). Moreover, FlaB promoted the differentiation of IL-10- and TGF-β-producing tolerogenic DCs (tolDCs), which suppressed both Th1/Th2 responses and enhanced the activation of Treg cells (146). Besides DCs and T cells, a recent study demonstrated flagellin to stabilize OVA-induced eosinophil activation (releasing major basic protein (MBP) and peroxidase (EPX) in vitro and in vivo) and to reduce oxidative stress in OVA-sensitized eosinophils which was correlated with decreased allergic inflammation (147). Additionally, Zeng et al. found flagellin to diminish allergen-induced oxidative stress in Bregs from both a food allergy (FA) mouse model and patients’ blood samples (148). Here, adding flagellin to OVA improved traditional AIT in an OVA-induced food allergy model in vivo (148). These results showed that flagellin can activate different cell types, changing both their cytokine secretion and metabolic status, which correlated with improved treatment outcomes in AIT in mice.
On the other hand, flagellin:allergen fusion proteins have demonstrated an even higher potential to improve allergy treatment than the mixture of flagellin and allergen. Tan et al. generated a recombinant fusion protein consisting of FlaB fused to the C-terminus of the HDM allergen Der p 2 (rDerp2:FlaB) (149). Compared to the non-fused Der p 2 + FlaB mixture, rDerp2:FlaB more efficiently suppressed airway hyper-responsiveness, serum IgE levels, and secretion of Th2 cytokines into bronchoalveolar lavage fluid in a HDM-induced mouse asthma model (149).
Moreover, Kitzmüller and colleagues also demonstrated that Salmonella FliC (genetically modified to lack the middle hypervariable domain of flagellin described to induce neutralizing anti-flagellin antibody responses) fused to either the N- or C-terminus of Bet v 1 (rFliC:Betv1 or rBetv1:FliC, respectively) could activate monocyte-derived DCs from pollen-allergic patients (150). Immunization of mice with either rFliC:Betv1 or rBetv1:FliC fusion proteins induced lower serum levels of Bet v 1-specific IgE paralleled by a higher production of IgG antibodies (150), suggesting flagellin:allergen fusion proteins to be promising novel treatment options for pollen allergy.
In own previous studies we generated fusion proteins combining flagellin A (FlaA) from Listeria monocytogenes with different allergens (141–143, 151). We demonstrated that prophylactic vaccination with rFlaA:OVA, but not the mixture of both proteins, induced higher production of allergen-specific IgG2a antibodies paralleled with decreased levels of both IgE and Th2 cytokines (IL-5 and IL-13), resulting in the prevention of clinical symptoms such as softness of faces, body weight loss, and drop in core body temperature in an OVA-induced intestinal allergy mouse model (142, 152).
More in-depth analyses showed, that all generated rFlaA:allergen fusion proteins stimulated mDCs to secrete higher amounts of both pro- (IL-6, IL-1β, TNF-α, and IL-12) and anti-inflammatory (IL-10) cytokines (Figure 6) (141, 143, 151–153). rFlaA:allergen fusion protein-treated mDCs also displayed an activated phenotype with higher surface expression levels of the maturation markers CD40, CD69, CD80, and CD86 (Figure 6) (141, 151). Mechanistically, the induced mDC activation was mediated by intracellular MyD88-, MAPK-, and nuclear factor kappa-light-chain-enhancer of activated B cells (NFκB)-pathways while being largely TLR5-independent (143, 152, 153). The rFlaA:allergen fusion protein-stimulated mDCs efficiently modulated allergen-specific T cell responses, suppressing allergen-induced Th2 cytokine (IL-4, IL-5, and IL-13) production from ex vivo-isolated, Th2-biased CD4+ T cells in an IL-10-dependent manner (Figure 6) (142, 143, 151). The flagellin:antigen fusion proteins formed high-molecular aggregates possibly due to self-assembly of flagellin molecules into flagella-like structures, enabling the formation of intermolecular cysteine bridges between the fused allergens (FlaA itself does not contain cysteine residues) (143, 152). These aggregates were both taken up more strongly by mDCs and inhibition of aggregate uptake dose-dependently suppressed fusion protein-induced cytokine secretion (143). Therefore, these results suggest the strong activation of mDCs by the flagellin:allergen fusion proteins, while being mostly TLR5-independent, to be at least in part caused by aggregation-mediated enhanced uptake and subsequent triggering of above-described intracellular signaling cascades.
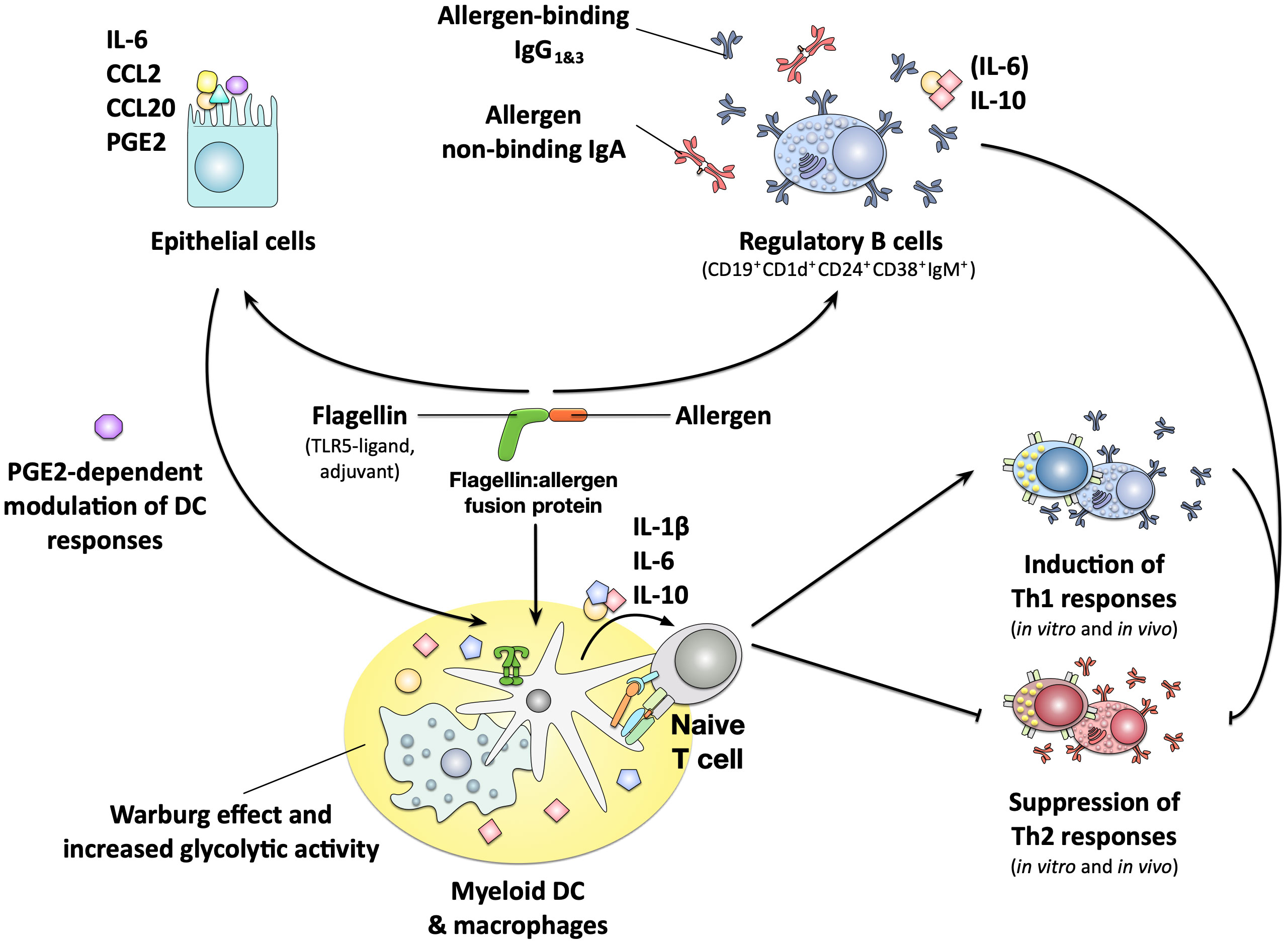
Figure 6 Immune modulating properties of flagellin:allergen fusion proteins. Flagellin:allergen fusion proteins combining the TLR5- and NLRC4-ligand flagellin and different allergens into a single molecule were shown to strongly activate both myeloid DCs and macrophages, resulting in the secretion of both pro- and anti-inflammatory cytokines and the expression of co-stimulatory molecules. The activated APCs promoted the induction of Th1 responses both in vitro and in vivo while also efficiently suppressing Th2 responses in an IL-10-dependent manner. This pro-tolerogenic phenotype of the APCs was critically dependent on a metabolic shift towards increased glycolytic activity termed the Warburg effect. The fusion protein consisting of flagellin A and the major birch pollen allergen Bet v 1 was also shown to strongly activate epithelial cells, resulting in the secretion of IL-6 and the chemokines CCL2 and CCL20. The activated epithelial cells furthermore produced prostaglandin E2 (PGE2) which was shown to modulate DC responses towards the fusion protein. Finally, B cells stimulated with the fusion protein were shown to form a CD19+CD1d+CD24+CD38+IgM+ subpopulation with regulatory properties that secreted anti-inflammatory IL-10, produced allergen-binding IgG1&3 antibodies and suppressed allergen-specific Th2 responses. For more information see text. PGE2, Prostaglandin E2.
Interestingly, the tolerogenic phenotype of mDCs stimulated with a flagellin fusion protein incorporating the major birch pollen allergen Bet v 1 (rFlaA:Betv1) was shown to depend on a JNK-MAPK-dependent activation of the mTOR pathway (143, 153). mTOR is a conserved serine/threonine protein kinase that belongs to the phosphatidylinositol 3-kinase-(PI3K) family. mTOR not only integrates various nutritional and environmental stimuli, including levels of growth factors, cellular energy reserves, and stress levels, but is also a master regulator of cellular metabolism that affects innate and adaptive immune responses (154). mTOR activation in rFlaA:Betv1-stimulated mDCs resulted in alterations of mDC metabolism with increased rates of glycolysis, Warburg metabolism, and reduced mitochondrial respiration (155). Inhibition of either glycolysis or mTOR activation suppressed rFlaA:Betv1-induced IL-10 secretion (143, 153, 155). In addition to glycolysis, fatty acid synthesis also significantly contributed to rFlaA:Betv1-mediated cytokine secretion, the production of antimicrobial molecules, and the modulation of T cell responses (155).
In line with the results presented for mDCs, stimulation of murine bone marrow-derived macrophages with rFlaA:Betv1 also triggered a MyD88-dependent, but only partly TLR5-dependent, cytokine production, stronger activation of hypoxia inducible factor 1 alpha (HIF-1a)-, MAPK-, NFκB-, and mTOR-signaling, and increased glucose metabolism (156). Therefore, macrophages also contribute to the strong immune-modulating effects of rFlaA:Betv1 previously observed in vivo and should be considered important target cells contributing to the re-establishment of allergen tolerance in AIT.
Moreover, the murine lung epithelial cell line LA-4 was shown to be activated by rFlaA:Betv1. Here, the fusion protein was shown to be taken up more strongly, triggering a MAPK-, NFκB-, and cyclo oxygenase 2 (COX2)-dependent activation of epithelial cells, characterized by a pronounced secretion of the cytokine IL-6, the myeloid chemo-attractants CCL2 and CCL20, as well as production of prostaglandin E2 (Figure 6) (157). rFlaA:Betv1-stimulated LA-4 cells modulated the activation of mDCs in a COX-2-dependent manner, resulting in lower secretion of the pro-inflammatory cytokines IL-12 and TNF-α, while the levels of the IL-1β and IL-10 remained unchanged (157). Therefore, epithelial cells also contribute to the immune modulating properties of potential allergen therapeutics and should be kept in mind as potential target cells for AIT approaches.
Finally, rFlaA:Betv1 was shown to induce a MyD88- and mTOR-dependent, but thymus-independent activation of ex vivo-isolated B cells, inducing a regulatory B cell phenotype (CD19+CD1d+CD24+CD38+IgM+) characterized by: (I) the secretion of the anti-inflammatory cytokine IL-10, (II) the production of antigen-binding IgG(1&3) and non-antigen-binding IgA antibodies, and (III) the capacity to suppress Bet v 1-induced Th2-responses (Figure 6) (158). To our knowledge, this is the first report showing the induction of Bregs by a potential therapeutic candidate for the treatment of allergic diseases.
So far Flagellin:antigen fusion proteins have not been investigated clinically for allergy treatment, but they were tested in several phase II clinical trials for the prevention of influenza infection (136, 137, 159–161). In these studies, the vaccines were well tolerated with lower doses (up to 3 µg) resulting in mild side effects (injection site pain, headache, fatigue, and myalgia) paralleled by increased serum CRP levels (136, 137, 159–161). Higher doses (10 µg) increased severity of fatigue, headache, myalgia, joint pain, fever, and gastrointestinal symptoms (nausea, vomiting, diarrhea) (136, 160). Symptoms resolved within 24 h of vaccination (136).
In summary, both flagellin and especially flagellin-containing fusion proteins incorporating different allergens were shown to efficiently activate many types of immune cells and thereby modulate immune responses, making these allergen-adjuvant conjugates interesting candidates for further development in AIT treatment.
3.5.2 CpG oligodeoxynucleotides
Bacterial DNA contains a high frequency of unmethylated CpG motifs that can bind to TLR9 and trigger immune responses (162). Therefore, synthetic immunostimulatory oligodeoxynucleotides containing unmethylated CpG motifs (CpG ODN) have been investigated to either mimic natural bacterial infection or as vaccine adjuvants (162). So far, several different types of CpG ODNs have been tested in clinical phase I/II trials as vaccine adjuvants against either infectious diseases (hepatitis B, malaria, and pneumonia) or cancer (melanoma, breast cancer, sarcoma, ovarian cancer, glioblastoma, and lymphoma) (162). The overall results showed that CpG ODN adjuvants only induced short-term mild-to-moderate adverse effects, while inducing Th1-biased immune responses and promoting CD8+ T cell activation (162).
The proposed advantages of using CpG ODN for allergen-specific immunotherapy include: (I) CpG ODN can activate DCs and macrophages via TLR9, promoting especially pDCs, to increase allergen uptake and produce IL-10, TGF-β, and indoleamine 2,3-dioxygenase (IDO) (Figure 7). This in turn drives differentiation and activation of Treg responses while also inducing DC-derived secretion of IFN, IL-6, IL-12, and IL-18 that promote the differentiation of IFN-γ producing Th1 cells and isotype-switch towards IgG2a (Figure 7) (162). (II) Since B cells express high levels of TLR9, CpG ODN can also directly activate B cells (Figure 7). An in vivo study that used ragweed pollen-sensitized mice demonstrated that combining CpG ODN with ragweed pollen could induce higher frequencies of IL-10-producing Breg (B220+CD19+CD23+IgM+CD40+MHCIIhi), which contribute to the suppression of allergen-induced inflammatory responses (163). However, CpG ODN application was also reported to result in increased serum levels of TNF-α that were associated with toxic shock in mice (Figure 7) (164, 165).
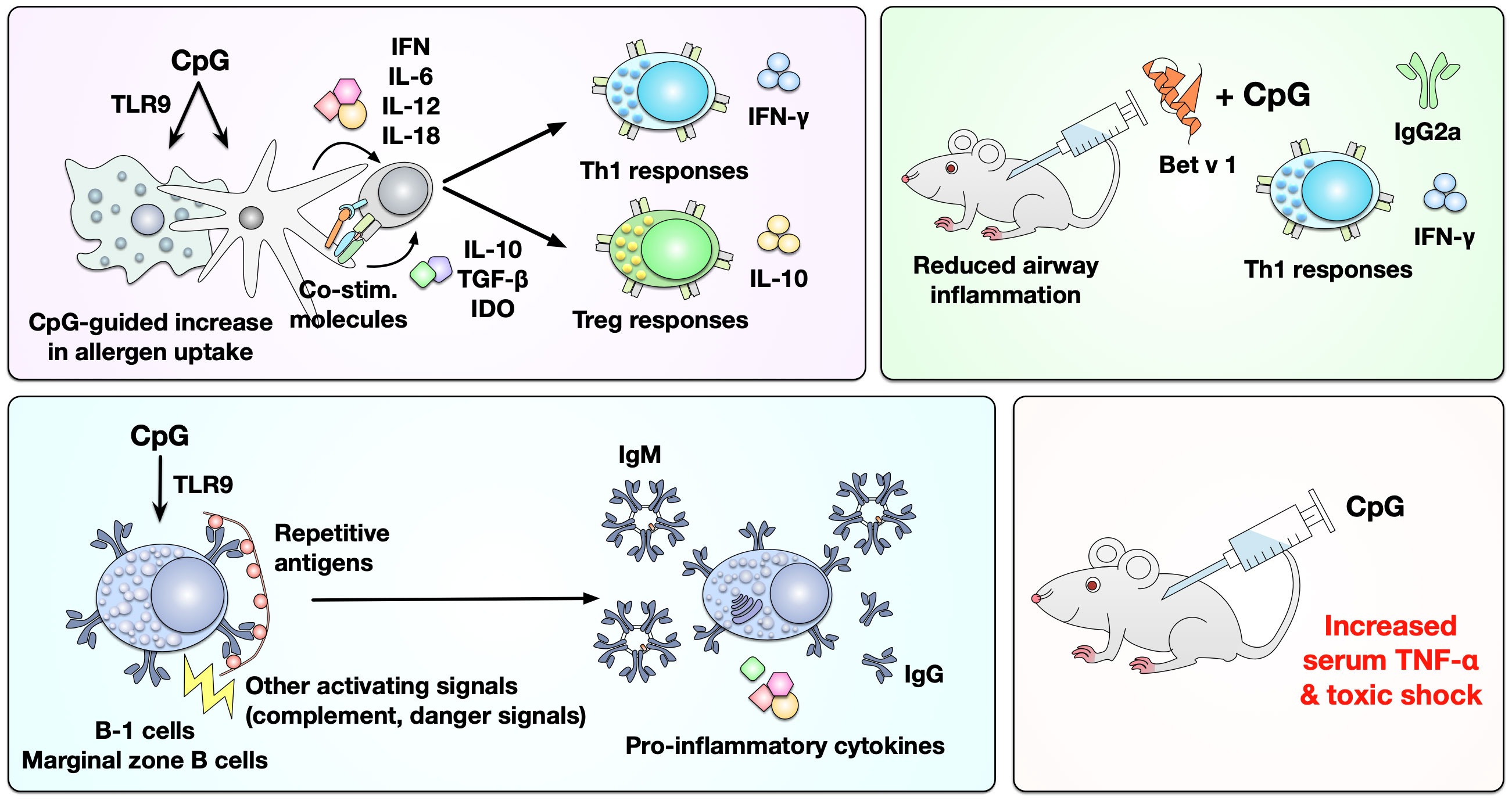
Figure 7 Immunological effects of CpG ODNs as adjuvants in mice and men. CpG ODNs can activate DCs and macrophages via TLR9, resulting in increased uptake of co-applied allergens and the differentiation of both allergen-specific Th1 and Treg cells. In mice the mixture of Bet v 1 and CpG ODN was shown to predominantly induce IFN-γ secreting Th1 cells and the production of Bet v 1-specific IgG2a antibodies. Furthermore, CpG ODNs can thymus-independently activate either B-1 or marginal zone B cells, promoting the secretion of antibodies and pro-inflammatory cytokines. Likely because of this strong capacity of CpG ODNs to activate multiple immune cells they were also reported to increase serum levels of TNF-α that were associated with toxic shock in mice. For more information see text. IDO, indoleamine 2,3-dioxygenase.
In a ragweed allergen-induced mouse asthma model, CpG ODN was shown to induce IFN-γ-dependent Th1-responses while suppressing allergic lung inflammation (166). As an approach to treat ragweed allergy, the immune-stimulating sequence 1018 (ISS-1018, 5’-TGACTGTGAACGTTCGAGATGA-3’) was chemically conjugated to the major ragweed allergen Amb a 1, resulting in an Amb a 1:CpG conjugate with a mean conjugation rate of 1 to 4 (167). This conjugate was termed TOLAMBA. In BALB/c mice, TOLAMBA induced Th1-biased immune responses characterized by the production of IFN-γ, IgG1- (also observed in rabbits and cynomolgus monkeys) and IgG2a-antibodies, while the non-conjugated mixture of Amb a 1 and ISS 1018 predominantly induced Th2-biased immune responses (167). In Amb a 1-sensitized and -challenged BALB/c mice, TOLAMBA reduced the production of IgG1 and IgE antibodies while boosting production of IgG2a (167). Similar results were described for Amb a 1-ISS-1080 by both Marshall et al. and Santeliz et al. (168, 169) as well as OVA-CpG ODN (1826) by Shirota et al. (170).
In 2006 Creticos and coworkers reported the results of a randomized, double-blind, placebo-controlled phase II trial testing TOLAMBA in 25 ragweed allergic patients (sponsored by the Johns Hopkins University School of Medicine, Baltimore, USA under participation of Dynavax Technologies, Berkeley, California, USA) (171). Patients were treated with six weekly injections of increasing doses of TOLAMBA (0.06, 0.3, 1.2, 3, 6, and 12 µg) and monitored during the following two ragweed seasons (171). Compared to placebo, TOLAMBA reduced rhinitis visual analogue scores by two thirds in both ragweed seasons, and also Amb a 1-specific IgE levels were lower during the first ragweed season (171). While the authors reported no vaccine-associated systemic adverse events or clinically significant abnormalities, also no effect on the primary endpoint (nasal vascular permeability as assessed by nasal-lavage albumin) was achieved (171).
To follow up on these results, a phase IIb study with TOLAMBA in 738 ragweed allergic patients was conducted (sponsored by Dynavax Technologies). It was initially reported in a conference abstract, that treatment during the first ragweed season was well tolerated in all groups without TOLAMBA-related serious adverse events (172). However, later on, the company reported problems with the study as they observed no measurable disease in any of the study groups during the ragweed season (http://investors.dynavax.com/releasedetail.cfm?releaseid=231013). In May 2008 the company reported, that although TOLAMBA showed a trend towards a reduction of the symptoms of ragweed allergic individuals relative to placebo, no statistical significance was achieved (https://investors.dynavax.com/news-releases/news-release-details/dynavax-tolambatm-chamber-study-misses-primary-endpoint-company). With no original paper published on the study so far, the company referred to “an unexpectedly high degree of variability in the data set possibly due to the subjective nature of symptom scoring used to assess efficacy” and decided to discontinue clinical development of TOLAMBA (https://investors.dynavax.com/news-releases/news-release-details/dynavax-tolambatm-chamber-study-misses-primary-endpoint-company).
As an approach to treat food allergy, Rodriguez et al. treated BALB/c mice sensitized to the major peach allergen Pru p 3 with a mixture of Pru p 3 peptides and CpG ODNs (173). In their experimental model, treatment with Pru p 3 peptides plus CpG ODNs suppressed the temperature drop induced by i.p. challenge with the allergen and strongly reduced Pru p 3-specific IgE levels (173). This CpG ODN-mediated immune modulation was accompanied by an increased production of Pru p 3-specific IgG2a antibodies as well as an increase in IL-10-producing CD4+CD25+ Tregs and IFN-γ-producing Th1 cells (173). Interestingly, the tolerance was maintained even after stopping the treatment for three weeks (173).
In a recent review article Montamat and colleagues recapitulated the development of CpG ODN adjuvants for allergy treatment in more detail (174). When analyzing the complex immune-stimulating properties of CpG ODN adjuvants, they concluded that the detrimental inflammation and Th1 responses observed upon application of CpG ODN adjuvants may be either preferentially triggered by low doses of CpG ODNs or be the byproduct of LPS potentially contaminating the used CPG ODN preparations (174). They concluded, that application of higher doses of CpG ODN may reliably induce the desired, pro-tolerogenic Treg responses (174). They proposed, that “doses between 0.5 and 1.5 mg/kg, dependent on the administration route, should induce the desired immune tolerance toward the allergen with a minimal risk of adverse reactions” (174).
In theory, the immune-activating properties of CpG ODNs are of interest in allergy treatment and initial mouse studies showed promising immune-modulating effects [induction of Th1 responses paralleled by suppression of Th2 responses (167)]. However, currently there are no clinical trials demonstrating a clear benefit of CpG ODNs in allergic patients. As neither an optimal dose nor application route of CpG ODNs are established, further studies improving on the published protocols should investigate the potential of CpG ODNs as adjuvants for advanced AIT treatment.
4 Summary and conclusion
Adjuvants have the potential to improve AIT by increasing the immunogenicity of isolated allergen(s), while at the same time reducing the number of injections and (cumulative) amounts of allergen(s) that need to be applied. Furthermore, adjuvants can reduce the probability of anaphylactic reactions by adsorbing the allergen(s) and modulate allergen-specific immune responses towards either predominantly tolerogenic or Th1-biassed immune responses.
The adjuvants currently approved for use in humans (aluminum hydroxide, calcium phosphate, MCT, and MPLA) show advantages for improving AIT, such as prolonging the contact time of immune cells with the allergen(s) at the injection site and inducing allergen-specific IgG production. However, some of them also show disadvantages, for example, the use of aluminum hydroxide can induce unwanted Th2 responses and IgE production.
Therefore, several different adjuvants (liposomes, O/W emulsions, VLPs, viral components, carbohydrate-based adjuvants, flagellin, and CpG ODN) have been tested in recent years not only in vitro and in vivo in respective animal models, but also in clinical trials. Liposomes, while usually lacking pronounced intrinsic immune-activating capacity, can both reduce cutaneous anaphylactic reactions and lung inflammation in mouse models when combined with other adjuvants (e.g. with QS-21 and MPLA in AS01). Similarly, O/W emulsions reduce the chance of anaphylactic reactions by slowly releasing the allergen, while generating mixed Th1/Th2 responses by increased APC recruitment and antigen uptake. So far, O/W emulsions have not seen widespread investigation for allergy treatment. VLPs are potent activators of DCs and B cells that have repeatedly shown promising results in pre-clinical trials. However, the only candidate so far tested in multiple clinical trials (QβG10) did not progress in clinical development after failing to show significant differences between the QβG10 treatment and placebo groups in a phase IIb study. However, there are currently several VLP-based therapeutics in different stages of pre-clinical and clinical development. Carbohydrate-based adjuvants like QS-21, glucans, and mannan induce a robust immune system activation with a tendency towards Th1-biassed immune responses. Here, especially mannan:allergoid conjugates have shown promising tolerogenic properties and are currently undergoing clinical evaluation in multiple trials. The TLR5-ligand flagellin fused to different allergens has shown promising pre-clinical results inducing the differentiation of tolerogenic DCs and macrophages and regulatory B cells. However, so far flagellin-containing therapeutics have not progressed to clinical trials in the field of allergology. Finally, TLR9-activating CpG oligodeoxynucleotides have shown promising immune-modulating effects in mice (induction of Th1 responses paralleled by suppression of Th2 responses), but so far there are no clinical trials demonstrating a clear benefit in allergic patients.
In summary, these adjuvants showed the potential to improve AIT by (I) inducing higher levels of allergen-specific IgG while decreasing IgE production and (II) promoting the differentiation and activation of different regulatory immune cells, such as Tregs, tolerogenic DCs, macrophages, and Bregs. In the performed clinical studies, some adjuvants failed to show clinical efficacy (e.g., CpG, QβG10, Table 1), while some adjuvants have recently shown promising results in first clinical studies and could be useful as future adjuvants (e.g., VLPs displaying allergens, PreS, superfine dispersed β-(1,3)-glucan, mannan, Table 1). However, up to now, these results have not resulted in marketing authorization of novel adjuvanted AIT products. With a number of clinical studies currently ongoing to further test some of the described adjuvants, we may be able to better assess the potential of some of these adjuvants in the near future.
Author contributions
Y-JL: Data curation, Investigation, Visualization, Writing – original draft, Writing – review & editing. JZ: Data curation, Investigation, Writing – original draft, Writing – review & editing. SS: Conceptualization, Data curation, Project administration, Supervision, Validation, Visualization, Writing – original draft, Writing – review & editing.
Funding
The author(s) declare financial support was received for the research, authorship, and/or publication of this article. Y-JL was in part funded by the German Research Foundation (DFG SCHE637/4). Some results described in this manuscript were generated with funding from the German Research Foundation (DFG SCHE637/4 and DFG SCHU2951/4).
Acknowledgments
The authors would like to thank Prof. Vera Mahler (Paul-Ehrlich-Institut) for helpful suggestions on the manuscript draft.
Conflict of interest
The authors declare that the research was conducted in the absence of any commercial or financial relationships that could be construed as a potential conflict of interest.
Publisher’s note
All claims expressed in this article are solely those of the authors and do not necessarily represent those of their affiliated organizations, or those of the publisher, the editors and the reviewers. Any product that may be evaluated in this article, or claim that may be made by its manufacturer, is not guaranteed or endorsed by the publisher.
Author disclaimer
Opinions expressed in this paper are the personal views of the authors, not necessarily reflecting an official opinion of the Paul-Ehrlich-Institut or the German Federal Ministry of Health.
Glossary
References
1. Platts-Mills TAE. The allergy epidemics: 1870-2010. J Allergy Clin Immunol. (2015) 136:3–13. doi: 10.1016/j.jaci.2015.03.048
2. Eder W, Ege MJ, von Mutius E. The asthma epidemic. N Engl J Med. (2006) 355:2226–35. doi: 10.1056/NEJMra054308
3. Backman H, Räisänen P, Hedman L, Stridsman C, Andersson M, Lindberg A, et al. Increased prevalence of allergic asthma from 1996 to 2006 and further to 2016-results from three population surveys. Clin Exp Allergy. (2017) 47:1426–35. doi: 10.1111/cea.12963
4. Spolidoro GCI, Amera YT, Ali MM, Nyassi S, Lisik D, Ioannidou A, et al. Frequency of food allergy in Europe: An updated systematic review and meta-analysis. Allergy. (2023) 78:351–68. doi: 10.1111/all.15560
5. Kucuksezer UC, Ozdemir C, Cevhertas L, Ogulur I, Akdis M, Akdis CA. Mechanisms of allergen-specific immunotherapy and allergen tolerance. Allergology Int. (2020) 69:549–60. doi: 10.1016/j.alit.2020.08.002
6. Petrovsky N, Aguilar JC. Vaccine adjuvants: current state and future trends. Immunol Cell Biol. (2004) 82:488–96. doi: 10.1111/j.0818-9641.2004.01272.x
7. Gamazo C, D’Amelio C, Gastaminza G, Ferrer M, Irache JM. Adjuvants for allergy immunotherapeutics. Hum Vaccin Immunother. (2017) 13:2416–27. doi: 10.1080/21645515.2017.1348447
8. Bonam SR, Partidos CD, Halmuthur SKM, Muller S. An overview of novel adjuvants designed for improving vaccine efficacy. Trends Pharmacol Sci. (2017) 38:771–93. doi: 10.1016/j.tips.2017.06.002
9. Pulendran B, Arunachalam P S, O’Hagan DT. Emerging concepts in the science of vaccine adjuvants. Nat Rev Drug Discovery. (2021) 20:454–75. doi: 10.1038/s41573-021-00163-y
10. Spreafico R, Ricciardi-Castagnoli P, Mortellaro A. The controversial relationship between NLRP3, alum, danger signals and the next-generation adjuvants. Eur J Immunol. (2010) 40:638–42. doi: 10.1002/eji.200940039
11. Kool M, Pétrilli V, De Smedt T, Rolaz A, Hammad H, van Nimwegen M, et al. Cutting edge: alum adjuvant stimulates inflammatory dendritic cells through activation of the NALP3 inflammasome. J Immunol. (2008) 181:3755–9. doi: 10.4049/jimmunol.181.6.3755
12. HogenEsch H. Mechanisms of stimulation of the immune response by aluminum adjuvants. Vaccine. (2002) 20 Suppl 3:S34–39. doi: 10.1016/s0264-410x(02)00169-x
13. Shaw AR, Feinberg MB. 90 - Vaccines. In: Rich RR, Fleisher TA, Shearer WT, Schroeder HW, Frew AJ, Weyand CM editors. Clin Immunol 4th ed. London. (2013), 1095–121. doi: 10.1016/B978-0-7234-3691-1.00103-3
14. He Q, Mitchell AR, Johnson SL, Wagner-Bartak C, Morcol T, Bell SJ. Calcium phosphate nanoparticle adjuvant. Clin Diagn Lab Immunol. (2000) 7:899–903. doi: 10.1128/CDLI.7.6.899-903.2000
15. Takeuchi O, Akira S. Pattern recognition receptors and inflammation. Cell. (2010) 140:805–20. doi: 10.1016/j.cell.2010.01.022
16. Verma SK, Mahajan P, Singh NK, Gupta A, Aggarwal R, Rappuoli R, et al. New-age vaccine adjuvants, their development, and future perspective. Front Immunol. (2023) 14. doi: 10.3389/fimmu.2023.1043109
17. Lin Y-J. Immunological characterization of Man2 as a novel adjuvant and rFlaA:Betv1 as a therapeutic candidate for allergy treatment. PhD thesis Technical University Darmstadt, Darmstadt (2022).
18. Drachenberg KJ, Wheeler AW, Stuebner P, Horak F. A well-tolerated grass pollen-specific allergy vaccine containing a novel adjuvant, monophosphoryl lipid A, reduces allergic symptoms after only four preseasonal injections. Allergy. (2001) 56:498–505. doi: 10.1034/j.1398-9995.2001.056006498.x
19. Casale TB, Cole J, Beck E, Vogelmeier CF, Willers J, Lassen C, et al. CYT003, a TLR9 agonist, in persistent allergic asthma - a randomized placebo-controlled Phase 2b study. Allergy. (2015) 70:1160–8. doi: 10.1111/all.12663
20. Eckl-Dorna J, Weber M, Stanek V, Linhart B, Ristl R, Waltl EE, et al. Two years of treatment with the recombinant grass pollen allergy vaccine BM32 induces a continuously increasing allergen-specific IgG4 response. EBioMedicine. (2019) 50:421–32. doi: 10.1016/j.ebiom.2019.11.006
21. Yamada J, Hamuro J, Hatanaka H, Hamabata K, Kinoshita S. Alleviation of seasonal allergic symptoms with superfine beta-1,3-glucan: a randomized study. J Allergy Clin Immunol. (2007) 119:1119–26. doi: 10.1016/j.jaci.2007.02.005
22. Nieto A, Mazón Á, Nieto M, Ibáñez E, Jang D-T, Calaforra S, et al. First-in-human phase 2 trial with mite allergoids coupled to mannan in subcutaneous and sublingual immunotherapy. Allergy. (2022) 77:3096–107. doi: 10.1111/all.15374
23. Glenny AT, Pope CG, Waddington H, Wallace U. Immunological notes. XVII–XXIV. J Pathol Bacteriology. (1926) 29:31–40. doi: 10.1002/path.1700290106
24. Hogenesch H. Mechanism of immunopotentiation and safety of aluminum adjuvants. Front Immunol. (2012) 3:406. doi: 10.3389/fimmu.2012.00406
25. Moingeon P. Adjuvants for allergy vaccines. Hum Vaccin Immunother. (2012) 8:1492–8. doi: 10.4161/hv.21688
26. Jensen-Jarolim E. Aluminium in allergies and allergen immunotherapy. World Allergy Organ J. (2015) 8:7. doi: 10.1186/s40413-015-0060-5
27. Kooijman S, Brummelman J, van Els CACM, Marino F, Heck AJR, van Riet E, et al. Vaccine antigens modulate the innate response of monocytes to Al(OH)3. PloS One. (2018) 13:e0197885. doi: 10.1371/journal.pone.0197885
28. Fadugba OO, Wang L, Chen Q, Halasa NB. Immune responses to pertussis antigens in infants and toddlers after immunization with multicomponent acellular pertussis vaccine. Clin Vaccine Immunol. (2014) 21:1613–9. doi: 10.1128/CVI.00438-14
29. McDougall SA, Heath MD, Kramer MF, Skinner MA. Analysis of aluminium in rat following administration of allergen immunotherapy using either aluminium or microcrystalline-tyrosine-based adjuvants. Bioanalysis. (2016) 8:547–56. doi: 10.4155/bio.16.10
30. Weisser K, Stübler S, Matheis W, Huisinga W. Towards toxicokinetic modelling of aluminium exposure from adjuvants in medicinal products. Regul Toxicol Pharmacol. (2017) 88:310–21. doi: 10.1016/j.yrtph.2017.02.018
31. Hethey C, Hartung N, Wangorsch G, Weisser K, Huisinga W. Physiology-based toxicokinetic modelling of aluminium in rat and man. Arch Toxicol. (2021) 95:2977–3000. doi: 10.1007/s00204-021-03107-y
32. Relyveld EH, Henocq E, Raynaud M. [STUDY OF THE ANTIDIPHTHERIA VACCINATION OF ALLERGIC SUBJECTS WITH A PURE ANATOXIN ABSORBED ON CALCIUM PHOSPHATE]. Bull World Health Organ. (1964) 30:321–5.
33. Matić D, Skarica N, Relyveld EH, Raynaud M, Mravunac B. [Study of the vaccinating power of purified diphtheria toxoid adsorped on various adjuvants]. Bull World Health Organ. (1966) 34:21–32.
34. Relyveld EH, Martin R, Raynaud M, Damas JP, Thérond C, Hénocq E, et al. [Calcium phosphate as adjuvant in vaccinations in man]. Ann Inst Pasteur (Paris). (1969) 116:300–26.
35. Jensen-Jarolim E, Bachmann MF, Bonini S, Jacobsen L, Jutel M, Klimek L, et al. State-of-the-art in marketed adjuvants and formulations in Allergen Immunotherapy: A position paper of the European Academy of Allergy and Clinical Immunology (EAACI). Allergy. (2020) 75:746–60. doi: 10.1111/all.14134
36. Leynadier F, Banoun L, Dollois B, Terrier P, Epstein M, Guinnepain MT, et al. Immunotherapy with a calcium phosphate-adsorbed five-grass-pollen extract in seasonal rhinoconjunctivitis: a double-blind, placebo-controlled study. Clin Exp Allergy. (2001) 31:988–96. doi: 10.1046/j.1365-2222.2001.01145.x
37. Coursaget P, Yvonnet B, Relyveld EH, Barres JL, Diop-Mar I, Chiron JP. Simultaneous administration of diphtheria-tetanus-pertussis-polio and hepatitis B vaccines in a simplified immunization program: immune response to diphtheria toxoid, tetanus toxoid, pertussis, and hepatitis B surface antigen. Infect Immun. (1986) 51:784–7. doi: 10.1128/iai.51.3.784-787.1986
38. Jarcho M. Calcium phosphate ceramics as hard tissue prosthetics. Clin Orthop Relat Res. (1981) (157):259–78. doi: 10.1097/00003086-198106000-00037
39. Hayashi M, Aoshi T, Kogai Y, Nomi D, Haseda Y, Kuroda E, et al. Optimization of physiological properties of hydroxyapatite as a vaccine adjuvant. Vaccine. (2016) 34:306–12. doi: 10.1016/j.vaccine.2015.11.059
40. Goto N, Kato H, Maeyama J, Shibano M, Saito T, Yamaguchi J, et al. Local tissue irritating effects and adjuvant activities of calcium phosphate and aluminium hydroxide with different physical properties. Vaccine. (1997) 15:1364–71. doi: 10.1016/s0264-410x(97)00054-6
41. Aggerbeck H, Fenger C, Heron I. Booster vaccination against diphtheria and tetanus in man. Comparison of calcium phosphate and aluminium hydroxide as adjuvants–II. Vaccine. (1995) 13:1366–74. doi: 10.1016/0264-410x(94)00082-x
42. Gupta RK, Siber GR. Comparison of adjuvant activities of aluminium phosphate, calcium phosphate and stearyl tyrosine for tetanus toxoid. Biologicals. (1994) 22:53–63. doi: 10.1006/biol.1994.1008
43. Vassilev TL. Aluminium phosphate but not calcium phosphate stimulates the specific IgE response in Guinea pigs to tetanus toxoid. Allergy. (1978) 33:155–9. doi: 10.1111/j.1398-9995.1978.tb01527.x
44. Ickovic MR, Relyveld EH, Hénocq E, David B, Marie FN. Calcium-phosphate-adjuvanted allergens: total and specific IgE levels before and after immunotherapy with house dust and Dermatophagoides pteronyssinus extracts. Ann Immunol (Paris). (1983) 134D:385–98. doi: 10.1016/s0769-2625(83)80029-4
45. Wang X, Li X, Ito A, Watanabe Y, Sogo Y, Hirose M, et al. Rod-shaped and substituted hydroxyapatite nanoparticles stimulating type 1 and 2 cytokine secretion. Colloids Surf B Biointerfaces. (2016) 139:10–6. doi: 10.1016/j.colsurfb.2015.12.004
46. Baldrick P, Richardson D, Wheeler AW. Review of L-tyrosine confirming its safe human use as an adjuvant. J Appl Toxicol. (2002) 22:333–44. doi: 10.1002/jat.869
47. Leuthard DS, Duda A, Freiberger SN, Weiss S, Dommann I, Fenini G, et al. Microcrystalline tyrosine and aluminum as adjuvants in allergen-specific immunotherapy protect from igE-mediated reactivity in mouse models and act independently of inflammasome and TLR signaling. J Immunol. (2018) 200:3151–9. doi: 10.4049/jimmunol.1800035
48. Wheeler AW, Moran DM, Robins BE, Driscoll A. l-Tyrosine as an immunological adjuvant. Int Arch Allergy Appl Immunol. (1982) 69:113–9. doi: 10.1159/000233157
49. Rosewich M, Lee D, Zielen S. Pollinex Quattro: an innovative four injections immunotherapy in allergic rhinitis. Hum Vaccin Immunother. (2013) 9:1523–31. doi: 10.4161/hv.24631
50. Patel P, Salapatek AMF. Pollinex Quattro: a novel and well-tolerated, ultra short-course allergy vaccine. Expert Rev Vaccines. (2006) 5:617–29. doi: 10.1586/14760584.5.5.617
51. Awate S, Babiuk LA, Mutwiri G. Mechanisms of action of adjuvants. Front Immunol. (2013) 4:114. doi: 10.3389/fimmu.2013.00114
52. Shamji MH, Kappen JH, Akdis M, Jensen-Jarolim E, Knol EF, Kleine-Tebbe J, et al. Biomarkers for monitoring clinical efficacy of allergen immunotherapy for allergic rhinoconjunctivitis and allergic asthma: an EAACI Position Paper. Allergy. (2017) 72:1156–73. doi: 10.1111/all.13138
53. Becker S, Zieglmayer P, Canto G, Fassio F, Yong P, Acikel C, et al. A meta-analysis on allergen-specific immunotherapy using MCT® (MicroCrystalline Tyrosine)-adsorbed allergoids in pollen allergic patients suffering from allergic rhinoconjunctivitis. Clin Transl Allergy. (2021) 11:e12037. doi: 10.1002/clt2.12037
54. Zielen S, Kuna P, Aberer W, Lassmann S, Pfaar O, Klimek L, et al. Strong dose response after immunotherapy with PQ grass using conjunctival provocation testing. World Allergy Organ J. (2019) 12:100075. doi: 10.1016/j.waojou.2019.100075
55. DuBuske LM, Frew AJ, Horak F, Keith PK, Corrigan CJ, Aberer W, et al. Ultrashort-specific immunotherapy successfully treats seasonal allergic rhinoconjunctivitis to grass pollen. Allergy Asthma Proc. (2011) 32:239–47. doi: 10.2500/aap.2011.32.3453
56. Heath MD, Swan NJ, Marriott AC, Silman NJ, Hallis B, Prevosto C, et al. Comparison of a novel microcrystalline tyrosine adjuvant with aluminium hydroxide for enhancing vaccination against seasonal influenza. BMC Infect Dis. (2017) 17:232. doi: 10.1186/s12879-017-2329-5
57. Cabral-Miranda G, Heath MD, Gomes AC, Mohsen MO, Montoya-Diaz E, Salman AM, et al. Microcrystalline tyrosine (MCT®): A depot adjuvant in licensed allergy immunotherapy offers new opportunities in malaria. Vaccines (Basel). (2017) 5:32. doi: 10.3390/vaccines5040032
58. Cabral-Miranda G, Heath MD, Mohsen MO, Gomes AC, Engeroff P, Flaxman A, et al. Virus-Like Particle (VLP) Plus Microcrystalline Tyrosine (MCT) Adjuvants Enhance Vaccine Efficacy Improving T and B Cell Immunogenicity and Protection against Plasmodium berghei/vivax. Vaccines (Basel). (2017) 5:10. doi: 10.3390/vaccines5020010
59. Mohsen MO, Heath MD, Cabral-Miranda G, Lipp C, Zeltins A, Sande M, et al. Vaccination with nanoparticles combined with micro-adjuvants protects against cancer. J Immunother Cancer. (2019) 7:114. doi: 10.1186/s40425-019-0587-z
60. Mata-Haro V, Martin M, Chilton PM, Cekic C, Casella CR, Mitchell TC. The vaccine adjuvant monophosphoryl lipid A as a TRIF-biased agonist of TLR4. Science. (2007) 316:1628–32. doi: 10.1126/science.1138963
61. Ulrich JT, Myers KR. Monophosphoryl lipid A as an adjuvant. Past experiences and new directions. Pharm Biotechnol. (1995) 6:495–524.
62. McAleer JP, Vella AT. Understanding how lipopolysaccharide impacts CD4 T-cell immunity. Crit Rev Immunol. (2008) 28:281–99. doi: 10.1615/critrevimmunol.v28.i4.20
63. Thompson BS, Evans JT, Mitchell TC, Chilton PM, Ward JR. The low-toxicity versions of LPS, MPL adjuvant and RC529, are efficient adjuvants for CD4+ T cells. J Leukoc Biol (2005) 78:1273–80. doi: 10.1189/jlb.0305172.1
64. Vernacchio L, Bernstein H, Pelton S, Allen C, MacDonald K, Dunn J, et al. Effect of monophosphoryl lipid A (MPL) on T-helper cells when administered as an adjuvant with pneumocococcal-CRM197 conjugate vaccine in healthy toddlers. Vaccine. (2002) 20:3658–67. doi: 10.1016/S0264-410X(02)00413-9
65. Kundi M. New hepatitis B vaccine formulated with an improved adjuvant system. Expert Rev Vaccines. (2007) 6:133–40. doi: 10.1586/14760584.6.2.133
66. Agnandji ST, Lell B, Soulanoudjingar SS, Fernandes JF, Abossolo BP, Conzelmann C, et al. First results of phase 3 trial of RTS,S/AS01 malaria vaccine in African children. N Engl J Med. (2011) 365:1863–75. doi: 10.1056/NEJMoa1102287
67. GlaxoSmithKline Vaccine HPV-007 Study Group, Romanowski B, de Borba PC, Naud PS, Roteli-Martins CM, De Carvalho NS, et al. Sustained efficacy and immunogenicity of the human papillomavirus (HPV)-16/18 AS04-adjuvanted vaccine: analysis of a randomised placebo-controlled trial up to 6.4 years. Lancet. (2009) 374:1975–85. doi: 10.1016/S0140-6736(09)61567-1
68. Mothes N, Heinzkill M, Drachenberg KJ, Sperr WR, Krauth MT, Majlesi Y, et al. Allergen-specific immunotherapy with a monophosphoryl lipid A-adjuvanted vaccine: reduced seasonally boosted immunoglobulin E production and inhibition of basophil histamine release by therapy-induced blocking antibodies. Clin Exp Allergy. (2003) 33:1198–208. doi: 10.1046/j.1365-2222.2003.01699.x
69. de la Torre MV, Baeza ML, Nájera L, Zubeldia JM. Comparative study of adjuvants for allergen-specific immunotherapy in a murine model. Immunotherapy. (2018) 10:1219–28. doi: 10.2217/imt-2018-0072
70. Schülke S, Flaczyk A, Vogel L, Gaudenzio N, Angers I, Löschner B, et al. MPLA shows attenuated pro-inflammatory properties and diminished capacity to activate mast cells in comparison with LPS. Allergy. (2015) 70:1259–68. doi: 10.1111/all.12675
71. Blanco-Pérez F, Goretzki A, Wolfheimer S, Schülke S. The vaccine adjuvant MPLA activates glycolytic metabolism in mouse mDC by a JNK-dependent activation of mTOR-signaling. Mol Immunol. (2019) 106:159–69. doi: 10.1016/j.molimm.2018.12.029
72. Gawchik SM, Saccar CL. Pollinex Quattro Tree: allergy vaccine. Expert Opin Biol Ther. (2009) 9:377–82. doi: 10.1517/14712590802699596
73. Baldrick P, Richardson D, Woroniecki SR, Lees B. Pollinex Quattro Ragweed: safety evaluation of a new allergy vaccine adjuvanted with monophosphoryl lipid A (MPL) for the treatment of ragweed pollen allergy. J Appl Toxicol. (2007) 27:399–409. doi: 10.1002/jat.1223
74. Zimmermann J, Goretzki A, Meier C, Wolfheimer S, Lin Y-J, Rainer H, et al. Modulation of dendritic cell metabolism by an MPLA-adjuvanted allergen product for specific immunotherapy. Front Immunol. (2022) 13:916491. doi: 10.3389/fimmu.2022.916491
75. Pohlit H, Bellinghausen I, Frey H, Saloga J. Recent advances in the use of nanoparticles for allergen-specific immunotherapy. Allergy. (2017) 72:1461–74. doi: 10.1111/all.13199
76. Wang N, Chen M, Wang T. Liposomes used as a vaccine adjuvant-delivery system: From basics to clinical immunization. J Control Release. (2019) 303:130–50. doi: 10.1016/j.jconrel.2019.04.025
77. Szebeni J. Complement activation-related pseudoallergy: a stress reaction in blood triggered by nanomedicines and biologicals. Mol Immunol. (2014) 61:163–73. doi: 10.1016/j.molimm.2014.06.038
78. Inglut CT, Sorrin AJ, Kuruppu T, Vig S, Cicalo J, Ahmad H, et al. Immunological and toxicological considerations for the design of liposomes. Nanomaterials (Basel). (2020) 10:190. doi: 10.3390/nano10020190
79. Mohamed M, Abu Lila AS, Shimizu T, Alaaeldin E, Hussein A, Sarhan HA, et al. PEGylated liposomes: immunological responses. Sci Technol Adv Mater. (2019) 20:710–24. doi: 10.1080/14686996.2019.1627174
80. Audera C, Ramírez J, Soler E, Carreira J. Liposomes as carriers for allergy immunotherapy. Clin Exp Allergy. (1991) 21:139–44. doi: 10.1111/j.1365-2222.1991.tb00816.x
81. Pifferi C, Fuentes R, Fernández-Tejada A. Natural and synthetic carbohydrate-based vaccine adjuvants and their mechanisms of action. Nat Rev Chem. (2021) 5:197–216. doi: 10.1038/s41570-020-00244-3
82. Alberca-Custodio RW, Faustino LD, Gomes E, Nunes FPB, de Siqueira MK, Labrada A, et al. Allergen-specific immunotherapy with liposome containing cpG-ODN in murine model of asthma relies on myD88 signaling in dendritic cells. Front Immunol. (2020) 11:692. doi: 10.3389/fimmu.2020.00692
83. Wilkins AL, Kazmin D, Napolitani G, Clutterbuck EA, Pulendran B, Siegrist C-A, et al. AS03- and MF59-adjuvanted influenza vaccines in children. Front Immunol. (2017) 8:1760. doi: 10.3389/fimmu.2017.01760
84. Alam S, Lukawska J, Corrigan C. Adjuvants in allergy: state of the art. Curr Treat Options Allergy. (2014) 1:39–47. doi: 10.1007/s40521-013-0008-3
85. Feng Z, Yi X, Hajavi J. New and old adjuvants in allergen-specific immunotherapy: With a focus on nanoparticles. J Cell Physiol. (2021) 236:863–76. doi: 10.1002/jcp.29941
86. Mosca F, Tritto E, Muzzi A, Monaci E, Bagnoli F, Iavarone C, et al. Molecular and cellular signatures of human vaccine adjuvants. Proc Natl Acad Sci U.S.A. (2008) 105:10501–6. doi: 10.1073/pnas.0804699105
87. Ahmed SS, Steinman L. Mechanistic insights into influenza vaccine-associated narcolepsy. Hum Vaccin Immunother. (2016) 12:3196–201. doi: 10.1080/21645515.2016.1171439
88. O’Konek JJ, Baker JR. Treatment of allergic disease with nanoemulsion adjuvant vaccines. Allergy. (2020) 75:246–9. doi: 10.1111/all.13977
89. Donaldson B, Lateef Z, Walker GF, Young SL, Ward VK. Virus-like particle vaccines: immunology and formulation for clinical translation. Expert Rev Vaccines. (2018) 17:833–49. doi: 10.1080/14760584.2018.1516552
90. Anzaghe M, Schülke S, Scheurer S. Virus-like particles as carrier systems to enhance immunomodulation in allergen immunotherapy. Curr Allergy Asthma Rep. (2018) 18:71. doi: 10.1007/s11882-018-0827-1
91. Mohsen MO, Zha L, Cabral-Miranda G, Bachmann MF. Major findings and recent advances in virus–like particle (VLP)-based vaccines. Semin Immunol. (2017) 34:123–32. doi: 10.1016/j.smim.2017.08.014
92. Zhang S, Cubas R, Li M, Chen C, Yao Q. Virus-like particle vaccine activates conventional B2 cells and promotes B cell differentiation to IgG2a producing plasma cells. Mol Immunol 46(10):1988–2001. doi: 10.1016/j.molimm.2009.03.008
93. Liao W, Hua Z, Liu C, Lin L, Chen R, Hou B. Characterization of T-dependent and T-independent B cell responses to a virus-like particle. J Immunol. (2017) 198:3846–56. doi: 10.4049/jimmunol.1601852
94. Bontkes HJ, Ruizendaal JJ, Kramer D, Meijer CJLM, Hooijberg E. Plasmacytoid dendritic cells are present in cervical carcinoma and become activated by human papillomavirus type 16 virus-like particles. Gynecol Oncol. (2005) 96:897–901. doi: 10.1016/j.ygyno.2004.10.040
95. Ma Y, Huang W, Liu C, Li Y, Xia Y, Yang X, et al. Immunization against TGF-β1 reduces collagen deposition but increases sustained inflammation in a murine asthma model. Hum Vaccin Immunother. (2016) 12:1876–85. doi: 10.1080/21645515.2016.1145849
96. Senti G, Johansen P, Haug S, Bull C, Gottschaller C, Müller P, et al. Use of A-type CpG oligodeoxynucleotides as an adjuvant in allergen-specific immunotherapy in humans: a phase I/IIa clinical trial. Clin Exp Allergy. (2009) 39:562–70. doi: 10.1111/j.1365-2222.2008.03191.x
97. Klimek L, Willers J, Hammann-Haenni A, Pfaar O, Stocker H, Mueller P, et al. Assessment of clinical efficacy of CYT003-QbG10 in patients with allergic rhinoconjunctivitis: a phase IIb study. Clin Exp Allergy. (2011) 41:1305–12. doi: 10.1111/j.1365-2222.2011.03783.x
98. Beeh K-M, Kanniess F, Wagner F, Schilder C, Naudts I, Hammann-Haenni A, et al. The novel TLR-9 agonist QbG10 shows clinical efficacy in persistent allergic asthma. J Allergy Clin Immunol. (2013) 131:866–74. doi: 10.1016/j.jaci.2012.12.1561
99. Storni F, Zeltins A, Balke I, Heath MD, Kramer MF, Skinner MA, et al. Vaccine against peanut allergy based on engineered virus-like particles displaying single major peanut allergens. J Allergy Clin Immunol. (2020) 145:1240–1253.e3. doi: 10.1016/j.jaci.2019.12.007
100. Marth K, Breyer I, Focke-Tejkl M, Blatt K, Shamji MH, Layhadi J, et al. A nonallergenic birch pollen allergy vaccine consisting of hepatitis PreS-fused Bet v 1 peptides focuses blocking IgG toward IgE epitopes and shifts immune responses to a tolerogenic and Th1 phenotype. J Immunol. (2013) 190:3068–78. doi: 10.4049/jimmunol.1202441
101. Niespodziana K, Focke-Tejkl M, Linhart B, Civaj V, Blatt K, Valent P, et al. A hypoallergenic cat vaccine based on Fel d 1–derived peptides fused to hepatitis B PreS. J Allergy Clin Immunol. (2011) 127:1562–1570.e6. doi: 10.1016/j.jaci.2011.02.004
102. Niederberger V, Neubauer A, Gevaert P, Zidarn M, Worm M, Aberer W, et al. Safety and efficacy of immunotherapy with the recombinant B-cell epitope-based grass pollen vaccine BM32. J Allergy Clin Immunol. (2018) 142:497–509.e9. doi: 10.1016/j.jaci.2017.09.052
103. Salari F, Vahedi F, Varasteh A-R, Ketabdar H, Chamani J, Sankian M. Enhanced sublingual immunotherapy by TAT-fused recombinant allergen in a murine rhinitis model. Int Immunopharmacol. (2017) 48:118–25. doi: 10.1016/j.intimp.2017.04.011
104. Edlmayr J, Niespodziana K, Linhart B, Focke-Tejkl M, Westritschnig K, Scheiblhofer S, et al. A combination vaccine for allergy and rhinovirus infections based on rhinovirus-derived surface protein VP1 and a nonallergenic peptide of the major timothy grass pollen allergen Phl p 1. J Immunol. (2009) 182:6298–306. doi: 10.4049/jimmunol.0713622
105. van Kooyk Y, Rabinovich GA. Protein-glycan interactions in the control of innate and adaptive immune responses. Nat Immunol. (2008) 9:593–601. doi: 10.1038/ni.f.203
106. Kensil CR, Patel U, Lennick M, Marciani D. Separation and characterization of saponins with adjuvant activity from Quillaja saponaria Molina cortex. J Immunol. (1991) 146:431–7.
107. Ragupathi G, Gardner JR, Livingston PO, Gin DY. Natural and synthetic saponin adjuvant QS-21 for vaccines against cancer. Expert Rev Vaccines. (2011) 10:463–70. doi: 10.1586/erv.11.18
108. Welsby I, Detienne S, N’Kuli F, Thomas S, Wouters S, Bechtold V, et al. Lysosome-dependent activation of human dendritic cells by the vaccine adjuvant QS-21. Front Immunol. (2016) 7:663. doi: 10.3389/fimmu.2016.00663
109. Detienne S, Welsby I, Collignon C, Wouters S, Coccia M, Delhaye S, et al. Central role of CD169+ Lymph node resident macrophages in the adjuvanticity of the QS-21 component of AS01. Sci Rep. (2016) 6:39475. doi: 10.1038/srep39475
110. Bonam SR, Rénia L, Tadepalli G, Bayry J, Kumar HMS. Plasmodium falciparum malaria vaccines and vaccine adjuvants. Vaccines. (2021) 9:1072. doi: 10.3390/vaccines9101072
111. Pink JR, Kieny M-P. 4th meeting on Novel Adjuvants Currently in/close to Human Clinical Testing World Health Organization – organisation Mondiale de la Santé Fondation Mérieux, Annecy, France, 23-25, June 2003. Vaccine. (2004) 22:2097–102. doi: 10.1016/j.vaccine.2004.01.021
112. Bigaeva E, van DE, Liu H, Hak E. Meta-analysis on randomized controlled trials of vaccines with QS-21 or ISCOMATRIX adjuvant: safety and tolerability. PloS One. (2016) 11:e0154757. doi: 10.1371/journal.pone.0154757
113. Waite DC, Jacobson EW, Ennis FA, Edelman R, White B, Kammer R, et al. Three double-blind, randomized trials evaluating the safety and tolerance of different formulations of the saponin adjuvant QS-21. Vaccine. (2001) 19:3957–67. doi: 10.1016/s0264-410x(01)00142-6
114. Keefer MC, Wolff M, Gorse GJ, Graham BS, Corey L, Clements-Mann ML, et al. Safety profile of phase I and II preventive HIV type 1 envelope vaccination: experience of the NIAID AIDS Vaccine Evaluation Group. AIDS Res Hum Retroviruses. (1997) 13:1163–77. doi: 10.1089/aid.1997.13.1163
115. Gilewski T, Adluri S, Ragupathi G, Zhang S, Yao TJ, Panageas K, et al. Vaccination of high-risk breast cancer patients with mucin-1 (MUC1) keyhole limpet hemocyanin conjugate plus QS-21. Clin Cancer Res. (2000) 6:1693–701.
116. Petrovsky N, Cooper PD. Carbohydrate-based immune adjuvants. Expert Rev Vaccines. (2011) 10:523–37. doi: 10.1586/erv.11.30
117. Dillon S, Agrawal S, Banerjee K, Letterio J, Denning TL, Oswald-Richter K, et al. Yeast zymosan, a stimulus for TLR2 and dectin-1, induces regulatory antigen-presenting cells and immunological tolerance. J Clin Invest. (2006) 116:916–28. doi: 10.1172/JCI27203
118. Donadei A, Gallorini S, Berti F, O’Hagan DT, Adamo R, Baudner BC. Rational design of adjuvant for skin delivery: conjugation of synthetic β-glucan dectin-1 agonist to protein antigen. Mol Pharm. (2015) 12:1662–72. doi: 10.1021/acs.molpharmaceut.5b00072
119. Wang H, Yang B, Wang Y, Liu F, Fernández-Tejada A, Dong S. β-Glucan as an immune activator and a carrier in the construction of a synthetic MUC1 vaccine. Chem Commun (Camb). (2018) 55:253–6. doi: 10.1039/c8cc07691j
120. Kanno T, Adachi Y, Ohashi-Doi K, Matsuhara H, Hiratsuka R, Ishibashi K-I, et al. Latent 1,3-β-D-glucan acts as an adjuvant for allergen-specific IgE production induced by Japanese cedar pollen exposure. Allergol Int. (2021) 70:105–13. doi: 10.1016/j.alit.2020.08.004
121. Hadebe S, Kirstein F, Fierens K, Redelinghuys P, Murray GI, Williams DL, et al. β-Glucan exacerbates allergic airway responses to house dust mite allergen. Respir Res. (2016) 17:35. doi: 10.1186/s12931-016-0352-5
122. Kimura Y, Sumiyoshi M, Suzuki T, Suzuki T, Sakanaka M. Inhibitory effects of water-soluble low-molecular-weight beta-(1,3-1,6) d-glucan purified from Aureobasidium pullulans GM-NH-1A1 strain on food allergic reactions in mice. Int Immunopharmacol. (2007) 7:963–72. doi: 10.1016/j.intimp.2007.03.003
123. Jesenak M, Urbancek S, Majtan J, Banovcin P, Hercogova J. β-Glucan-based cream (containing pleuran isolated from pleurotus ostreatus) in supportive treatment of mild-to-moderate atopic dermatitis. J Dermatolog Treat. (2016) 27:351–4. doi: 10.3109/09546634.2015.1117565
124. Kirmaz C, Bayrak P, Yilmaz O, Yuksel H. Effects of glucan treatment on the Th1/Th2 balance in patients with allergic rhinitis: a double-blind placebo-controlled study. Eur Cytokine Netw. (2005) 16:128–34.
125. He C, Liu Y, Schülke S, Nishio S, Guo Y, Rainer H, et al. β-1,3-glucan, but not β-1,3/1,6-glucan, exacerbates experimental food allergy, while both increase IgA induction. Allergy. 79(2):503–6. doi: 10.1111/all.15841
126. Okawa Y, Howard CR, Steward MW. Production of anti-peptide specific antibody in mice following immunization with peptides conjugated to mannan. J Immunol Methods. (1992) 149:127–31. doi: 10.1016/s0022-1759(12)80057-3
127. Sirvent S, Soria I, Cirauqui C, Cases B, Manzano AI, Diez-Rivero CM, et al. Novel vaccines targeting dendritic cells by coupling allergoids to nonoxidized mannan enhance allergen uptake and induce functional regulatory T cells through programmed death ligand 1. J Allergy Clin Immunol. (2016) 0. doi: 10.1016/j.jaci.2016.02.029
128. Apostolopoulos V, Pietersz GA, Loveland BE, Sandrin MS, McKenzie IF. Oxidative/reductive conjugation of mannan to antigen selects for T1 or T2 immune responses. Proc Natl Acad Sci U.S.A. (1995) 92:10128–32. doi: 10.1073/pnas.92.22.10128
129. Ahlén G, Strindelius L, Johansson T, Nilsson A, Chatzissavidou N, Sjöblom M, et al. Mannosylated mucin-type immunoglobulin fusion proteins enhance antigen-specific antibody and T lymphocyte responses. PloS One. (2012) 7:e46959. doi: 10.1371/journal.pone.0046959
130. Weinberger EE, Himly M, Myschik J, Hauser M, Altmann F, Isakovic A, et al. Generation of hypoallergenic neoglycoconjugates for dendritic cell targeted vaccination: a novel tool for specific immunotherapy. J Control Release. (2013) 165:101–9. doi: 10.1016/j.jconrel.2012.11.002
131. Manzano AI, Javier Cañada F, Cases B, Sirvent S, Soria I, Palomares O, et al. Structural studies of novel glycoconjugates from polymerized allergens (allergoids) and mannans as allergy vaccines. Glycoconj J. (2016) 33:93–101. doi: 10.1007/s10719-015-9640-4
132. Benito-Villalvilla C, Soria I, Pérez-Diego M, Fernández-Caldas E, Subiza JL, Palomares O. Alum impairs tolerogenic properties induced by allergoid-mannan conjugates inhibiting mTOR and metabolic reprogramming in human DCs. Allergy. (2020) 75:648–59. doi: 10.1111/all.14036
133. Benito-Villalvilla C, Pérez-Diego M, Angelina A, Kisand K, Rebane A, Subiza JL, et al. Allergoid-mannan conjugates reprogram monocytes into tolerogenic dendritic cells via epigenetic and metabolic rewiring. J Allergy Clin Immunol. (2022) 149:212–222.e9. doi: 10.1016/j.jaci.2021.06.012
134. Li D, Wu M. Pattern recognition receptors in health and diseases. Signal Transduct Target Ther. (2021) 6:291. doi: 10.1038/s41392-021-00687-0
135. Hayashi F, Smith KD, Ozinsky A, Hawn TR, Yi EC, Goodlett DR, et al. The innate immune response to bacterial flagellin is mediated by Toll-like receptor 5. Nature. (2001) 410:1099–103. doi: 10.1038/35074106
136. Turley CB, Rupp RE, Johnson C, Taylor DN, Wolfson J, Tussey L, et al. Safety and immunogenicity of a recombinant M2e-flagellin influenza vaccine (STF2.4xM2e) in healthy adults. Vaccine. (2011) 29:5145–52. doi: 10.1016/j.vaccine.2011.05.041
137. Tussey L, Strout C, Davis M, Johnson C, Lucksinger G, Umlauf S, et al. Phase 1 safety and immunogenicity study of a quadrivalent seasonal flu vaccine comprising recombinant hemagglutinin-flagellin fusion proteins. Open Forum Infect Dis. (2016) 3:ofw015. doi: 10.1093/ofid/ofw015
138. Lee SE, Kim SY, Jeong BC, Kim YR, Bae SJ, Ahn OS, et al. A bacterial flagellin , vibrio vulnificus flaB , has a strong mucosal adjuvant activity to induce protective immunity. Infect Immun (2006) 74:694–702. doi: 10.1128/IAI.74.1.694
139. Honko AN, Sriranganathan N, Lees CJ, Mizel SB. Flagellin is an effective adjuvant for immunization against lethal respiratory challenge with Yersinia pestis. Infection Immun. (2006) 74:1113–20. doi: 10.1128/IAI.74.2.1113-1120.2006
140. Huleatt JW, Jacobs AR, Tang J, Desai P, Kopp EB, Huang Y, et al. Vaccination with recombinant fusion proteins incorporating Toll-like receptor ligands induces rapid cellular and humoral immunity. Vaccine. (2007) 25:763–75. doi: 10.1016/j.vaccine.2006.08.013
141. Schülke S, Waibler Z, Mende M-S, Zoccatelli G, Vieths S, Toda M, et al. Fusion protein of TLR5-ligand and allergen potentiates activation and IL-10 secretion in murine myeloid DC. Mol Immunol. (2010) 48:341–50. doi: 10.1016/j.molimm.2010.07.006
142. Schülke S, Burggraf M, Waibler Z, Wangorsch A, Wolfheimer S, Kalinke U, et al. A fusion protein of flagellin and ovalbumin suppresses the TH2 response and prevents murine intestinal allergy. J Allergy Clin Immunol. (2011) 128:1340–1348.e12. doi: 10.1016/j.jaci.2011.07.036
143. Schülke S, Fiedler A-H, Junker A-C, Flaczyk A, Wolfheimer S, Wangorsch A, et al. Critical role of mammalian target of rapamycin for IL-10 dendritic cell induction by a flagellin A conjugate in preventing allergic sensitization. J Allergy Clin Immunol. (2018) 141:1786–1798.e11. doi: 10.1016/j.jaci.2017.07.002
144. Kim EH, Kim JH, Samivel R, Bae J-S, Chung Y-J, Chung P-S, et al. Intralymphatic treatment of flagellin-ovalbumin mixture reduced allergic inflammation in murine model of allergic rhinitis. Allergy. (2016) 71:629–39. doi: 10.1111/all.12839
145. Bae SJ, Koh JT, Ryu H-J, Choy HE, Lee SE, Rhee JH, et al. Inhibition of airway allergic disease by co-administration of flagellin with allergen. J Clin Immunol. (2007) 28:157–65. doi: 10.1007/s10875-007-9138-3
146. Shim J-U, Lee SE, Hwang W, Lee C, Park J-W, Sohn J-H, et al. Flagellin suppresses experimental asthma by generating regulatory dendritic cells and T cells. J Allergy Clin Immunol. (2016) 137:426–35. doi: 10.1016/j.jaci.2015.07.010
147. Luo X-Q, Liu J, Mo L-H, Yang G, Ma F, Ning Y, et al. Flagellin alleviates airway allergic response by stabilizing eosinophils through modulating oxidative stress. J Innate Immun. (2021) 13:333–44. doi: 10.1159/000515463
148. Zeng H-T, Liu Y, Zhao M, Liu J-Q, Jin Q-R, Liu Z-Q, et al. Modulating oxidative stress in B cells promotes immunotherapy in food allergy. Oxid Med Cell Longev. (2022) 2022:3605977. doi: 10.1155/2022/3605977
149. Tan W, Zheng JH, Duong TMN, Koh YI, Lee SE, Rhee JH. A fusion protein of derp2 allergen and flagellin suppresses experimental allergic asthma. Allergy Asthma Immunol Res. (2019) 11:254–66. doi: 10.4168/aair.2019.11.2.254
150. Kitzmüller C, Kalser J, Mutschlechner S, Hauser M, Zlabinger GJ, Ferreira F, et al. Fusion proteins of flagellin and the major birch pollen allergen Bet v 1 show enhanced immunogenicity, reduced allergenicity, and intrinsic adjuvanticity. J Allergy Clin Immunol. (2018) 141:293–299.e6. doi: 10.1016/j.jaci.2017.02.044
151. Schülke S, Kuttich K, Wolfheimer S, Duschek N, Wangorsch A, Reuter A, et al. Author Correction: Conjugation of wildtype and hypoallergenic mugwort allergen Art v 1 to flagellin induces IL-10-DC and suppresses allergen-specific TH2-responses in vivo. Sci Rep. (2018) 8:2745. doi: 10.1038/s41598-018-20635-3
152. Schülke S, Wolfheimer S, Gadermaier G, Wangorsch A, Siebeneicher S, Briza P, et al. Prevention of intestinal allergy in mice by rflaA:Ova is associated with enforced antigen processing and TLR5-dependent IL-10 secretion by mDC. PloS One. (2014) 9:e87822. doi: 10.1371/journal.pone.0087822
153. Moeller T, Wolfheimer S, Goretzki A, Scheurer S, Schülke S. NFκB- and MAP-kinase signaling contribute to the activation of murine myeloid dendritic cells by a flagellin A:Allergen fusion protein. Cells. (2019) 8(4):355. doi: 10.3390/cells8040355
154. Säemann MD, Haidinger M, Hecking M, Hörl WH, Weichhart T. The multifunctional role of mTOR in innate immunity: implications for transplant immunity. Am J Transplant. (2009) 9:2655–61. doi: 10.1111/j.1600-6143.2009.02832.x
155. Goretzki A, Lin Y-J, Zimmermann J, Rainer H, Junker A-C, Wolfheimer S, et al. Role of glycolysis and fatty acid synthesis in the activation and T cell-modulating potential of dendritic cells stimulated with a TLR5-ligand allergen fusion protein. Int J Mol Sci. (2022) 23:12695. doi: 10.3390/ijms232012695
156. Lin Y-J, Papp G, Miskey C, Fiedler A, Goretzki A, Wolfheimer S, et al. The flagellin:Allergen fusion protein rFlaA:Betv1 induces a myD88– and MAPK-dependent activation of glucose metabolism in macrophages. Cells. (2021) 10(10):2614. doi: 10.3390/cells10102614
157. Lin Y-J, Flaczyk A, Wolfheimer S, Goretzki A, Jamin A, Wangorsch A, et al. The Fusion Protein rFlaA:Betv1 Modulates DC Responses by a p38-MAPK and COX2-Dependent Secretion of PGE2 from Epithelial Cells. Cells. (2021) 10:3415. doi: 10.3390/cells10123415
158. Goretzki A, Lin Y-J, Meier C, Dorn B, Wolfheimer S, Jamin A, et al. Stimulation of naïve B cells with a fusion protein consisting of FlaA and Bet v 1 induces regulatory B cells ex vivo. Allergy. (2023) 78:663–81. doi: 10.1111/all.15542
159. Taylor DN, Treanor JJ, Strout C, Johnson C, Fitzgerald T, Kavita U, et al. Induction of a potent immune response in the elderly using the TLR-5 agonist, flagellin, with a recombinant hemagglutinin influenza-flagellin fusion vaccine (VAX125, STF2.HA1 SI). Vaccine. (2011) 29:4897–902. doi: 10.1016/j.vaccine.2011.05.001
160. Taylor DN, Treanor JJ, Sheldon EA, Johnson C, Umlauf S, Song L, et al. Development of VAX128, a recombinant hemagglutinin (HA) influenza-flagellin fusion vaccine with improved safety and immune response. Vaccine. (2012) 30:5761–9. doi: 10.1016/j.vaccine.2012.06.086
161. Treanor JJ, Taylor DN, Tussey L, Hay C, Nolan C, Fitzgerald T, et al. Safety and immunogenicity of a recombinant hemagglutinin influenza-flagellin fusion vaccine (VAX125) in healthy young adults. Vaccine. (2010) 28:8268–74. doi: 10.1016/j.vaccine.2010.10.009
162. Bode C, Zhao G, Steinhagen F, Kinjo T, Klinman DM. CpG DNA as a vaccine adjuvant. Expert Rev Vaccines. (2011) 10:499–511. doi: 10.1586/erv.10.174
163. Miyazaki D, Kuo CH, Tominaga T, Inoue Y, Ono SJ. Regulatory function of CpG-activated B cells in late-phase experimental allergic conjunctivitis. Invest Ophthalmol Vis Sci. (2009) 50:1626–35. doi: 10.1167/iovs.08-2701
164. Sparwasser T, Miethke T, Lipford G, Erdmann A, Häcker H, Heeg K, et al. Macrophages sense pathogens via DNA motifs: induction of tumor necrosis factor-alpha-mediated shock. Eur J Immunol. (1997) 27:1671–9. doi: 10.1002/eji.1830270712
165. Lipford GB, Sparwasser T, Bauer M, Zimmermann S, Koch ES, Heeg K, et al. Immunostimulatory DNA: sequence-dependent production of potentially harmful or useful cytokines. Eur J Immunol. (1997) 27:3420–6. doi: 10.1002/eji.1830271242
166. Sur S, Wild JS, Choudhury BK, Sur N, Alam R, Klinman DM. Long term prevention of allergic lung inflammation in a mouse model of asthma by CpG oligodeoxynucleotides. J Immunol. (1999) 162:6284–93.
167. Tighe H, Takabayashi K, Schwartz D, Marsden R, Beck L, Corbeil J, et al. Conjugation of protein to immunostimulatory DNA results in a rapid, long-lasting and potent induction of cell-mediated and humoral immunity. Eur J Immunol. (2000) 30:1939–47. doi: 10.1002/1521-4141(200007)30:7<1939::AID-IMMU1939>3.0.CO;2-
168. Marshall JD, Abtahi S, Eiden JJ, Tuck S, Milley R, Haycock F, et al. Immunostimulatory sequence DNA linked to the Amb a 1 allergen promotes T(H)1 cytokine expression while downregulating T(H)2 cytokine expression in PBMCs from human patients with ragweed allergy. J Allergy Clin Immunol. (2001) 108:191–7. doi: 10.1067/mai.2001.116984
169. Santeliz JV, Van Nest G, Traquina P, Larsen E, Wills-Karp M. Amb a 1-linked CpG oligodeoxynucleotides reverse established airway hyperresponsiveness in a murine model of asthma. J Allergy Clin Immunol. (2002) 109(3):455–62. doi: 10.1067/mai.2002.122156
170. Shirota H, Sano K, Kikuchi T, Tamura G, Shirato K. Regulation of murine airway eosinophilia and Th2 cells by antigen-conjugated CpG oligodeoxynucleotides as a novel antigen-specific immunomodulator. J Immunol. (2000) 164:5575–82. doi: 10.4049/jimmunol.164.11.5575
171. Creticos PS, Schroeder JT, Hamilton RG, Balcer-Whaley SL, Khattignavong AP, Lindblad R, et al. Immunotherapy with a ragweed-toll-like receptor 9 agonist vaccine for allergic rhinitis. N Engl J Med. (2006) 355:1445–55. doi: 10.1056/NEJMoa052916
172. Bernstein DI, Segall N, Nayak A, Casale TB, Korenblatt P, Martins E. Safety and efficacy of the novel vaccine TOLAMBATM in ragweed allergic adults, a dose finding study. J Allergy Clin Immunol 119(1):S78–9. doi: 10.1016/j.jaci.2006.11.332
173. Rodriguez MJ, Mascaraque A, Ramos-Soriano J, Torres MJ, Perkins JR, Gomez F, et al. Pru p 3-Epitope-based sublingual immunotherapy in a murine model for the treatment of peach allergy. Mol Nutr Food Res. (2017) 61. doi: 10.1002/mnfr.201700110
Keywords: type I hypersensitivity, allergen-specific immunotherapy, adjuvant, CpG, virus-like particle, mannan, flagellin, Th1/Th2 responses
Citation: Lin Y-J, Zimmermann J and Schülke S (2024) Novel adjuvants in allergen-specific immunotherapy: where do we stand? Front. Immunol. 15:1348305. doi: 10.3389/fimmu.2024.1348305
Received: 02 December 2023; Accepted: 05 February 2024;
Published: 23 February 2024.
Edited by:
Mrinmoy Sanyal, Stanford University, United StatesReviewed by:
Ronald L. Rabin, United States Food and Drug Administration, United StatesSrinivasa Reddy Bonam, University of Texas Medical Branch at Galveston, United States
Lilit Hovhannisyan, Institute of Biotechnology and Molecular Medicine (IBMM), Poland
Copyright © 2024 Lin, Zimmermann and Schülke. This is an open-access article distributed under the terms of the Creative Commons Attribution License (CC BY). The use, distribution or reproduction in other forums is permitted, provided the original author(s) and the copyright owner(s) are credited and that the original publication in this journal is cited, in accordance with accepted academic practice. No use, distribution or reproduction is permitted which does not comply with these terms.
*Correspondence: Stefan Schülke, c3RlZmFuLnNjaHVlbGtlQHBlaS5kZQ==