- 1Bacterial Vx Unit, GlaxoSmithKline, Siena, Italy
- 2Animal Resource Center, GlaxoSmithKline, Siena, Italy
- 3TRD, GlaxoSmithKline, Siena, Italy
- 4Infectious Disease Research Unit, GlaxoSmithKline, Upper Providence, PA, United States
Skin and soft tissue infections (SSTIs) are the most common diseases caused by Staphylococcus aureus (S. aureus), which can progress to threatening conditions due to recurrences and systemic complications. Staphylococcal protein A (SpA) is an immunomodulator antigen of S. aureus, which allows bacterial evasion from the immune system by interfering with different types of immune responses to pathogen antigens. Immunization with SpA could potentially unmask the pathogen to the immune system, leading to the production of antibodies that can protect from a second encounter with S. aureus, as it occurs in skin infection recurrences. Here, we describe a study in which mice are immunized with a mutated form of SpA mixed with the Adjuvant System 01 (SpAmut/AS01) before a primary S. aureus skin infection. Although mice are not protected from the infection under these conditions, they are able to mount a broader pathogen-specific functional immune response that results in protection against systemic dissemination of bacteria following an S. aureus second infection (recurrence). We show that this “hidden effect” of SpA can be partially explained by higher functionality of induced anti-SpA antibodies, which promotes better phagocytic activity. Moreover, a broader and stronger humoral response is elicited against several S. aureus antigens that during an infection are masked by SpA activity, which could prevent S. aureus spreading from the skin through the blood.
Introduction
Staphylococcus aureus (S. aureus) is a commensal human pathogen that persistently colonizes the skin and nares, as well as the gastrointestinal tract, of about one-third of the human population (1), and colonization is considered a key risk factor for infection (2). S. aureus is responsible for a wide variety of diseases, ranging from mild and common conditions to life-threatening diseases mainly thanks to its broad spectrum of virulence factors that can contribute to tissue colonization, infiltration, tissue damage, and distant diseases (3). Skin and soft tissue infections (SSTIs) are the most common S. aureus-mediated diseases. Uncomplicated SSTIs affect millions of patients of all ages worldwide every year (4–6), and the development of serious complications as systemic disseminations, even if relatively rare, can radically change the course of infection especially when caused by antibiotic-resistant strains (7). The capability of S. aureus to establish such a different kind of infection is mainly due to its plethora of virulence factors. Among these, staphylococcal protein A (SpA) plays a determinant role in establishing persistent and often recurrent infections, avoiding bacterial recognition and elimination by the host immune system. SpA, in fact, allows bacterial survival by exploiting two distinct antibody binding activities through its binding domains: (i) binding to immunoglobulins (in humans, all but IgD, IgE, and IgG3) through the Fcγ domain (8, 9), preventing opsonophagocytosis by sequestering antibodies and displaying them on the bacterial surface in an incorrect orientation (10–12); and (ii) binding to the VH3 domain of IgM/IgD present on the surface of B lymphocytes, triggering superantigen activity and causing cell necrosis (13–15). One of the main roles of the SpA is therefore to facilitate bacterial survival and to allow S. aureus to escape the host immune system. Previous works have shown that a nontoxigenic version of SpA (denominated SpAKKAA, hereby indicated as SpAmut), engineered by replacing essential amino acid residues in the binding domain, was able to elicit polyclonal antibodies that promote opsonophagocytosis and killing (OPK) of staphylococci and suppress their B-cell superantigen activity (11). Mouse monoclonal antibodies specific for SpAmut also neutralize SpA and promote OPK of S. aureus, together with the development of antibody responses against many different antigens (16). Furthermore, a previous work has shown that immunization with the non-toxic version of the SpA was able to protect guinea pigs from S. aureus-mediated systemic infection and to elicit a broader humoral response in animals vaccinated before infection as compared to only infected animals (8). Considering these observations, the development of a broader immune response after immunization with SpA and infection with S. aureus could represent an effective protection against a second exposure to the pathogen, as in recurrent skin infections. To understand if the immunomodulator properties of SpA could affect the humoral response after an S. aureus skin infection, we used a mouse model of skin infection and recurrence and characterized the humoral response in animals vaccinated with SpAmut.
Methods
Bacterial strains and preparation of inoculum for infection
S. aureus USA300 LAC strain was used for the in vivo model of skin infection and recurrence. Bacteria were grown in tryptic soy broth (TSB) at 37°C in agitation until the early exponential phase is reached and diluted 1:1 in a freezing solution, composed of phosphate-buffered saline (PBS) + 10% bovine serum albumin (BSA) + 10% L-glutamic acid. Aliquots were conserved at −80°C in cryovials until use.
For the inoculum preparation, 2-mL frozen stocks of bacteria were thawed and diluted in 48 mL of fresh TSB and incubated at 37°C at 250 rpm until bacteria reached the early exponential phase (OD600 = 2.0), at approximately 1.5–2 × 109 colony-forming units (CFUs)/mL. Bacteria were then washed once in sterile PBS and diluted with sterile PBS to obtain 4 × 108 CFUs/mL or 8 × 108 CFUs/mL for the skin infection or recurrence models, respectively.
S. aureus USA300 LAC strain expressing the green fluorescent protein (GFP) was used for the internalization assays. Bacteria were streaked on a tryptic soy agar (TSA) plate and incubated overnight (O/N) at 37°C + 5% CO2. The following day, pre-inocula were prepared from single colonies and they were incubated O/N at 37°C at 250 rpm. The following day, the cultures were diluted in fresh TSB and incubated at 37°C and 250 rpm for approximately 3 h, until they reached the early exponential phase (OD600 = 2). Bacteria were centrifuged at 2,400 rpm and 4°C for 10 min and washed twice with RPMI 1640 GlutaMAX + 25 mM HEPES + 0.05% BSA. After the final resuspension, the OD600 of bacteria was measured and adjusted to an OD600 of 1.0. Bacteria were aliquoted in 2-mL cryovials and stored at −20°C until use.
Animal care and ethical statements
Animal husbandry and experimental procedures were ethically reviewed and carried out in accordance with European Directive 2010/63/EU, Italian Decree 26/2014, and GSK Vaccines’ Policy on the Care, Welfare and Treatment of Animals, and were approved by the Italian Ministry of Health (authorization 123/2015-PR). Animals were kept in an AAALAC-accredited facility. Upon arrival, animals were randomly distributed in different experimental groups in individually ventilated cages (IVC, Sealsafe Plus GM500 by Tecniplast). The acclimation lasted for a period of 5 days. At the end of the acclimation period, each animal was identified by an individual tattoo. All animals had ad libitum access to GMP-grade food (Mucedola 4RF25 TOP CERTIFICATE) and bottled, filtered, tap water. Certified, irradiated cellulose bags containing Mucedola SCOBIS UNO bedding and carboard tunnels (ANTRUM) or plexiglass mouse houses were provided within the cages. A few food pellets in the cage were also used as enrichment for foraging and additional gnawing. Cage and bedding changes were performed once every 2 weeks in agreement with the cage supplier’s indications. Air supplied in IVC was 100% fresh air filtered by an EPA filter via the IVC system, with 60–75 air changes per hour. The animal room conditions were as follows: temperature, 21°C (± 3°C); relative humidity, 50% (range 30%–70%); and 12-h light/12-h dark cycle. Pressure, temperature, and relative humidity were recorded continuously by room probes, while the IVC system recorded the individual motors’ performance. The light cycle setting was ensured by a validated alarm system.
Mouse model of skin infection and recurrence
The mouse model of skin infection and recurrence was performed as depicted in the Supplementary Materials (see Supplementary Material Figure S1). Five-week-old female specific pathogen-free (SPF) C57BL/6 mice were immunized twice intramuscularly (IM), 28 days apart. Animals were immunized with 10 µg of a SpAmut adjuvanted with AS01, in a final volume of 50 µL, 25 µL/posterior leg. The control group (sham) was immunized with the formulation buffer alone (10 mM Na2HPO4 and 150 mM NaCl, pH 6.1).
For the in vivo vaccine efficacy studies, 4 weeks after the second immunization, the back of mice was shaved under anesthesia (3.5% isoflurane) using an electric razor and depilatory cream. For the primary skin infection, the day after shaving, mice were inoculated subcutaneously (SC) in the right flank with 2 × 107 CFU/50 µL of S. aureus USA300 LAC strain, under anesthesia (3.5% isoflurane). Six weeks after, mice were shaved and infected again as previously described in the opposite flank with 4 × 107 CFU/50 µL of S. aureus USA300 LAC strain to establish the model of skin infection recurrence.
Mice were observed daily for clinical signs of disease up to 14 days after infection. Mice were scored on the basis of pre-established parameters, and they were euthanized in case they exhibited pre-established humane endpoints in agreement with local Animal Welfare Policies (see Supplementary Materials Table 1). Dermonecrotic lesions were photographed and measured daily from day 4 to day 7 post-infection. At specific time points (which are shown in Supplementary Figure 1), blood was withdrawn from mice and serum was collected by centrifugation, for future serological analysis. At determined time points, mice were euthanized, and skin biopsies and kidneys were collected, homogenized, serially diluted and plated for bacterial burden, and expressed as CFU/mL for skin biopsies or as dissemination index for kidneys.
Coupling of magnetic beads with SpAWT and SpAmut
For all the Luminex-based assays, 20 µg of recombinant SpAWT or SpAmut was chemically coupled to 1.25 × 106 MagPlex beads (Luminex Corporation), through an automated coupling method with a liquid handling workstation (Hamilton – Microlab STAR IVD). Briefly, antigens were coupled by a two-step carbodiimide procedure during which microsphere carboxyl groups are first activated with 1-ethyl-3-(3-dimethylaminopropryl) carbodiimide hydrochloride (EDC, Pierce), in the presence of sulfo-NHS (Pierce) to form a sulfo-NHS-ester intermediate. The reactive intermediate is then replaced by a reaction with the primary amine of the target molecule to form a covalent amide bond.
Serological analysis
Total SpAmut-specific IgG titers were evaluated with an automatized Luminex assay, incubating serially diluted mice sera with SpAmut-coupled beads for 1 h with vigorous shaking. After washing, IgG titers were detected using an R-Phycoerythrin AffiniPure F(ab′)2 Goat anti-mouse IgG (Jackson ImmunoResearch Labs, Cat #115-116-072, RRID: AB_2338627) through a FLEXMAP 3D reader (Luminex Corporation). Median fluorescence intensities (MFIs) of the samples, subtracted to the MFI of the blanks (MFI-Bkgd), were interpolated to a standard curve, expressing the IgG titer as relative light units per milliliter (RLU/mL). For each sample tested, the experiment was considered valid if a titer was observed in at least three points of the dilution and if these titers were interpolated in the linear portion of the standard curve; the final IgG titer of each value was expressed as the median value of the observed titers.
For the IgG subclass analysis, threefold serially diluted pools of sera collected after the second dose of immunization (D52, post II) or after skin infection (D72, 2 weeks post-infection) were incubated with SpAmut-coupled beads for 1 h with vigorous shaking, repeating the assay in five different 96-well plates. After washing, the specific IgG subclass was detected using the appropriate R-Phycoerythrin AffiniPure F(ab′)2 Goat anti-mouse IgG (Cat #115-116-072, RRID : AB_2338627), IgG1 (Cat #115-115-205, RRID : AB_2338620), IgG2a (Cat # 115-115-206, RRID : AB_2338621), IgG2b (Cat #115-115-207, RRID : AB_2338622), or IgG3 (Cat #115-115-209, RRID : AB_2338624) secondary antibody (Jackson ImmunoResearch Labs). Results were detected using a Luminex 200 instrument system (Luminex Corporation), and they were reported as the ratio between the RLU/mL of the different IgG subclasses.
For the characterization of the avidity, a mouse standard serum and mice sera obtained after immunization (D52, post II) and after skin infection (D72, 2 weeks post-infection) were threefold serially diluted and incubated in duplicate with SpAmut-coupled beads for 1 h with vigorous shaking. After washing, one-half of the samples was incubated with vigorous shaking either with 2 M ammonium thiocyanate or with PBS for 30 min. After washing, the remaining IgGs were detected with an R-Phycoerythrin AffiniPure F(ab′)2 Goat anti-mouse IgG (Jackson ImmunoResearch Labs) using a Luminex 200 reader (Luminex corporation). Results were reported as avidity percentage, calculated on the basis of the ratio of the antibody titers recovered after incubation with ammonium thiocyanate with respect to titers observed after incubation with PBS.
Luminex displacement assay
The displacement assay was performed as described elsewhere (17). Briefly, SpAWT-coupled beads (prepared as already described above) were saturated with 10 µg/mL of biotinylated-human IgGs for 30 min under vigorous shaking. After washing, saturated beads were incubated with threefold serially diluted total IgGs purified from mice sera collected after immunization (D52, post II), after skin infection (D72, 2 weeks post-infection) or with commercial total mouse IgGs as negative control (ChromPure Mouse IgG, ImmunoResearch Labs, Cat #015-000-003, RRID : AB_2337188), for 30 min with shaking. After washing, the amount of biotinylated-human IgGs bound to the SpAWT-coupled beads was detected using 10 µg/mL of Streptavidin, R-Phycoerythrin Conjugate (SA-PE), through a FLEXMAP 3D reader. Results are shown as the percentage of antibody displaced, normalized on the signal obtained without adding mouse IgGs.
Staphylococcal protein microarray
The staphylococcal protein microarray was generated by spotting relevant S. aureus antigens that were previously selected, expressed in competent E. coli cells, purified, and characterized as described elsewhere (18–20). Briefly, staphylococcal antigens were spotted randomly in quadruplicate on ultra-thin nitrocellulose-coated glass slides (Maine Manufacturing) using an ink-jet spotter (Arrayjet) in a cabinet at 18°C and 40% humidity (see Supplementary Table S2 for the complete list of spotted antigens). Slides were conserved in the dark at 4°C until use. After saturating the slides with a blocking buffer (BlockIt, Arraylt), diluted sera samples (n = 9) obtained 2 weeks after infection from immunized (SpAmut/AS01 and infected) or non-immunized (sham and infected) mice were incubated on slides for 1 h in the dark in a humid chamber. After washing, signals were detected with an Alexa Flour 647 anti-mouse IgG secondary antibody (Jackson ImmunoResearch Labs, Cat #115-605-006, RRID : AB_2338903) and slides were rinsed and left to dry. Slides were scanned using an InnoScan 710 AL (INNOPSYS) and images were generated by the Mapix Microarray Image Analysis Software. Fluorescence intensities of spots were determined using ImaGene 9.0 software (Biodiscovery Inc.) and data analysis was performed using R scripts.
Each spotted antigen was considered as “positive” if recognized by the majority of tested sera (at least five out of nine).
Opsonophagocytosis assay
THP-1 cells (ATCC TIB-202, RRID: CVCL_0006) were cultured in RPMI 1640 medium supplemented with GlutaMAX and HEPES (Gibco), 10% FBS, and 5 μg/mL of penicillin/streptomycin, according to manufacturer indications. Single sera collected from animal studies were heat-inactivated at 56°C for 30 min. The opsonophagocytosis assay was performed as described elsewhere (17). Briefly, 3–4 × 106 CFUs/mL of S. aureus USA300 GFP strain were pre-opsonized with mouse sera obtained from normal mice (Pre-immune), immunized mice (SpAmut/AS01 post II), 2 weeks after infection from non-immunized mice (sham and infected), and immunized mice (SpAmut/AS01 and infected) for 30 min at 37°C under vigorous shaking. A total of 3–4 × 104 THP-1 cells were added to allow opsonization of bacteria, and incubation continued for an additional 30 min (bacteria-to-cell ratio of 200:1). After adding lysostaphin (Sigma-Aldrich) to lyse non-internalized bacteria, bacteria and cells were fixed with 2% formaldehyde for 1 h on ice prior to acquisition at FACS CANTO II (Becton Dickinson) and data collected were analyzed using the FACSDiva 8.0.1 software. Gating strategy was as follows: (i) THP-1 cells; (ii) singlet cells; and (iii) FITC-A, representative of cells that have effectively internalized GFP-expressing bacteria. The median percentages of GFP+ THP-1 cells were normalized on the median signal obtained with pre-immune sera, and results were then represented as fold change with respect to the median of pre-immune sera.
Statistical analysis
Statistical analysis was performed using the GraphPad Prism Software version 8.0.0. The non-parametric Mann–Whitney U-test was used to assess differences between two groups, as for the evaluation of IgG titers against SpAmut (Figure 1). The one-way ANOVA Kruskal–Wallis and uncorrected Dunn’s post-test was used to assess differences between three or more groups, as in the in vivo results or in the internalization assay (Figures 2A, B, 3). Finally, the Fisher’s exact t-test was used to assess differences between frequencies, as in the dissemination severity index or the protein microarray results (Figures 2C, 4). A p (value) ≤ 0.05 was considered significant for all these analyses. Legend: *p ≤ 0.05; **p ≤ 0.01; ***p ≤ 0.001; ****p ≤ 0.0001; ns = not significant, p > 0.05.
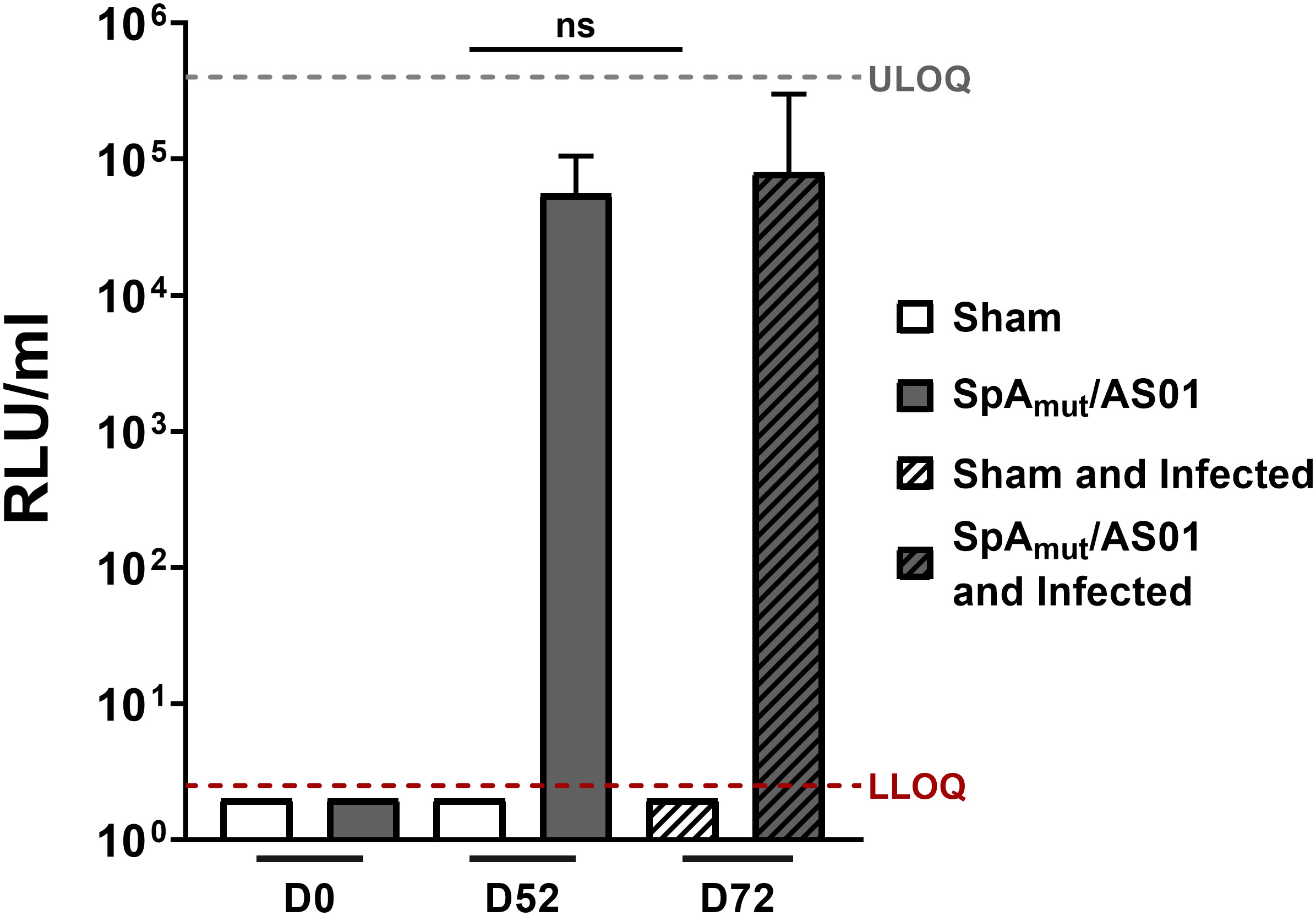
Figure 1 Subcutaneous infection with S. aureus does not induce anti-SpAmutIgG in mice. Anti-SpAmut IgG titration expressed as relative light units (RLU) per milliliter in sera of animals only immunized, only infected,or immunized and infected. Pre-immune (Day 0, D0), post II (D52), or post-infection (D72) sera were analyzed and titers against SpAmut were reported in the graph. Sham animals received only buffer, while SpAmut/AS01 animals were immunized with the proposed vaccine. The median value and the 95% confidence interval were reported for each group and condition. Groups were represented by at least 7 animals (range, 7–11 animals/group). LLOQ, lower limit of quantification; ULOQ, upper limit of quantification. n.s. pval > 0.05. The Kruskal–Wallis and uncorrected Dunn’s posttest was used to assess significance.
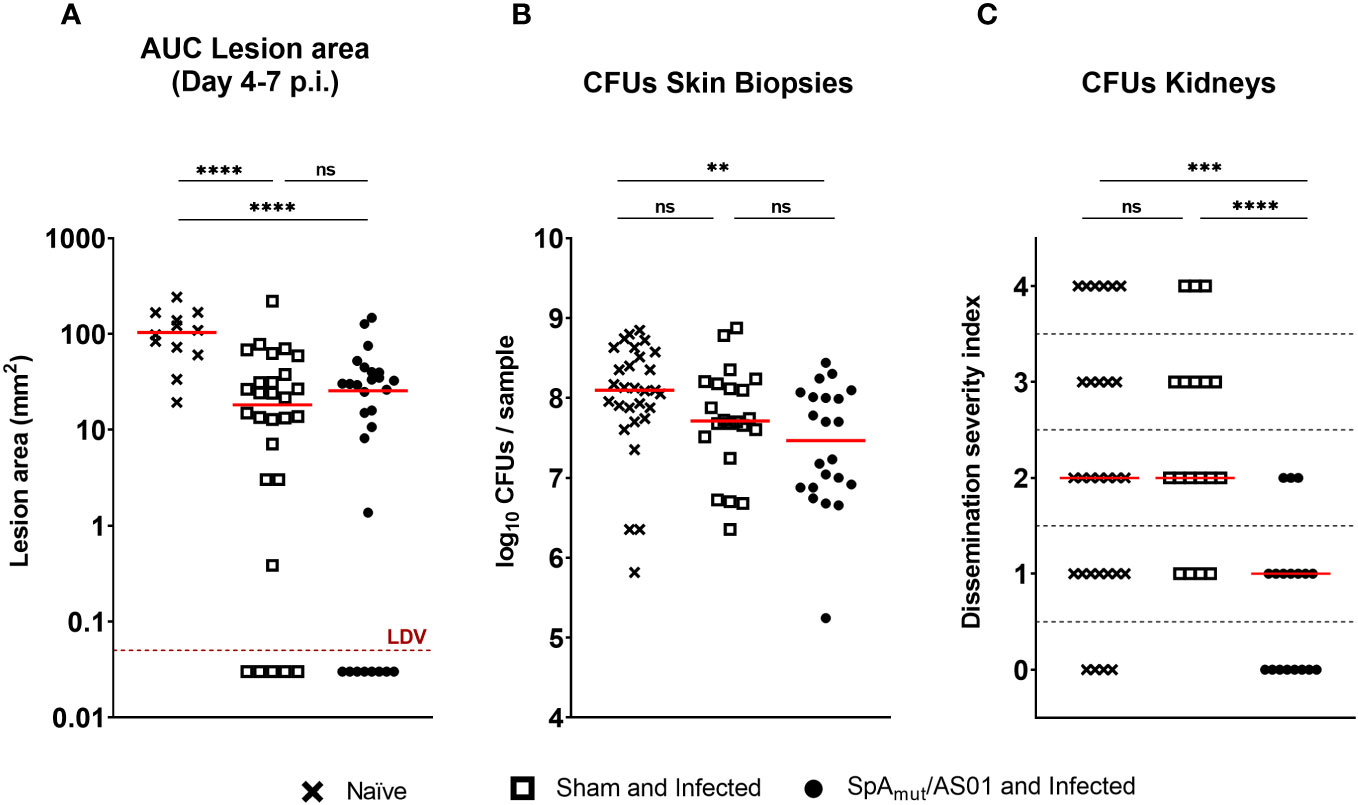
Figure 2 Vaccination with SpAmut/AS01 protects against local manifestations and systemic sequelae in a skin infection recurrence mouse model of S. aureus infection. Skin (A, B) and systemic (C) readouts used to assess protection of SpAmut/AS01 vaccine in the skin infection recurrence model. (A) Area under the curve (AUC) indicated as mm2 of dermonecrotic lesion developed from day 4 to day 7 after skin infection recurrence. The red dotted line represents the Lower Detectable Value (LDV). ****pval< 0.0001. (B) Log10 of colony-forming unit (CFU) counts enumerated in homogenized skin biopsies collected 7 days after skin infection recurrence. **pval< 0.01; n.s. pval > 0.05. (C) Dissemination severity index assigned to single mice based on CFU counts recovered in the kidneys of infected mice 7 days after skin infection recurrence. Score 0, no bacteria; Score 1, very low dissemination (1–10 CFU/kidneys); Score 2, minimal dissemination (11–100 CFU/kidneys); Score 3, mild dissemination (101–1,000 CFU/kidneys); Score 4 severe dissemination (>1,001 CFU/kidneys). ***pval< 0.001; ****pval< 0.0001; n.s. pval > 0.05. For all the graphs above, each single dot represented data from a single animal, and red lines were median values of the groups. A total of 29 animals belong to the age-paired naïve mice (Naïve) group, 18 animals make up the group of mice not vaccinated but exposed to S. aureus during the first infection (sham and infected), and 18 animals belong to the group of mice vaccinated and infected (SpAmut/AS01 and Infected). The Kruskal–Wallis and uncorrected Dunn’s post-test was used to assess significance among groups.
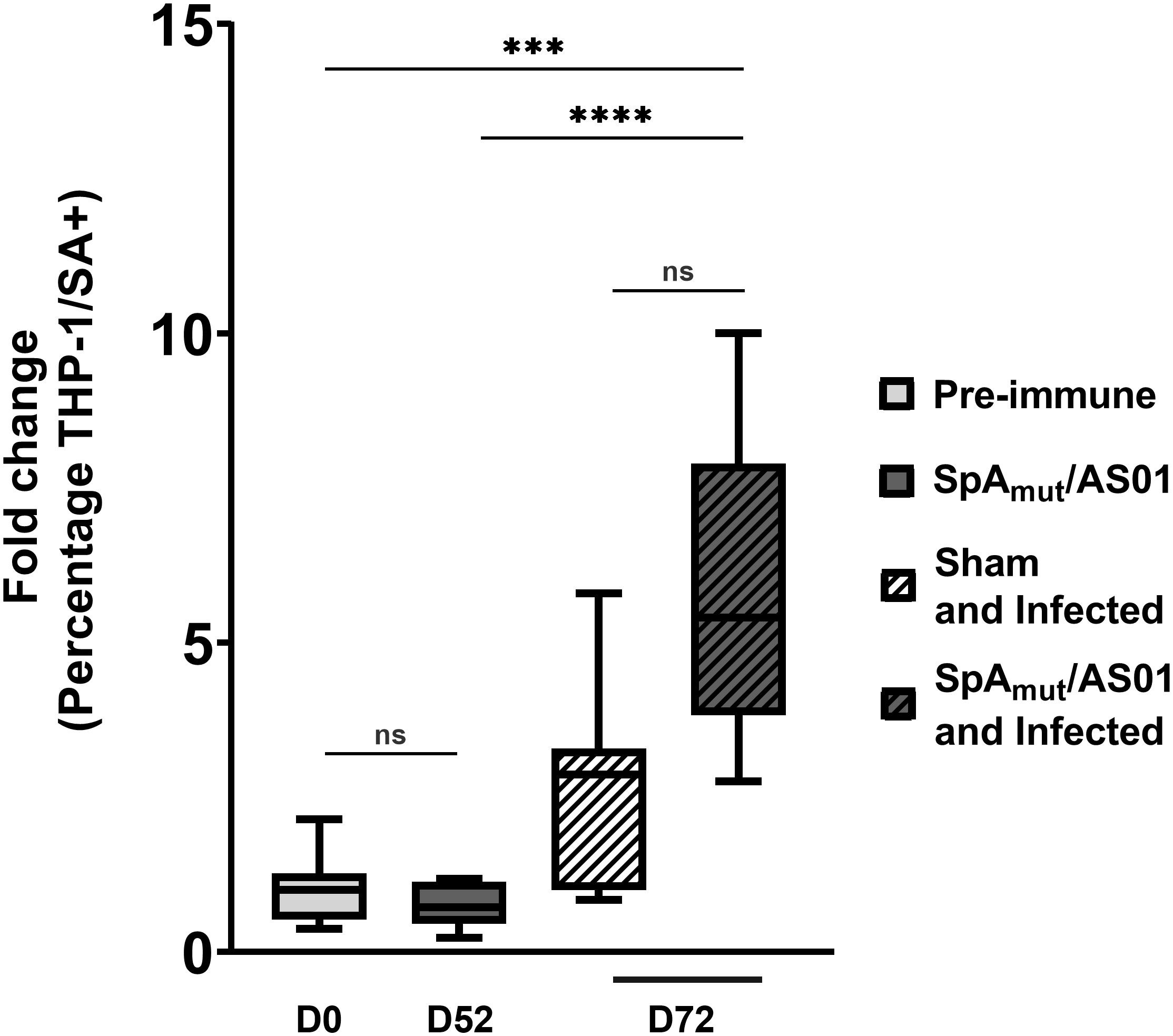
Figure 3 Antibodies in sera from SpAmut/AS01 and infected mice increase S. aureus phagocytosis by specialized human cells. Percentage of human THP-1 monocytic cells that internalized GFP-labeled S. aureus USA300 LAC strain in vitro. Box-and-whiskers representation of THP-1 cells that have internalized bacteria. Lines delimiting the boxes reported the median value of the group with 25 and 75 percentiles. The whiskers reported 10 and 90 percentiles. Eight pools (from at least five mouse sera for each group) were used for the assay. ***pval< 0.001; ****pval< 0.0001; n.s. pval > 0.05. The Kruskal–Wallis and uncorrected Dunn’s post-test was used to assess significance.
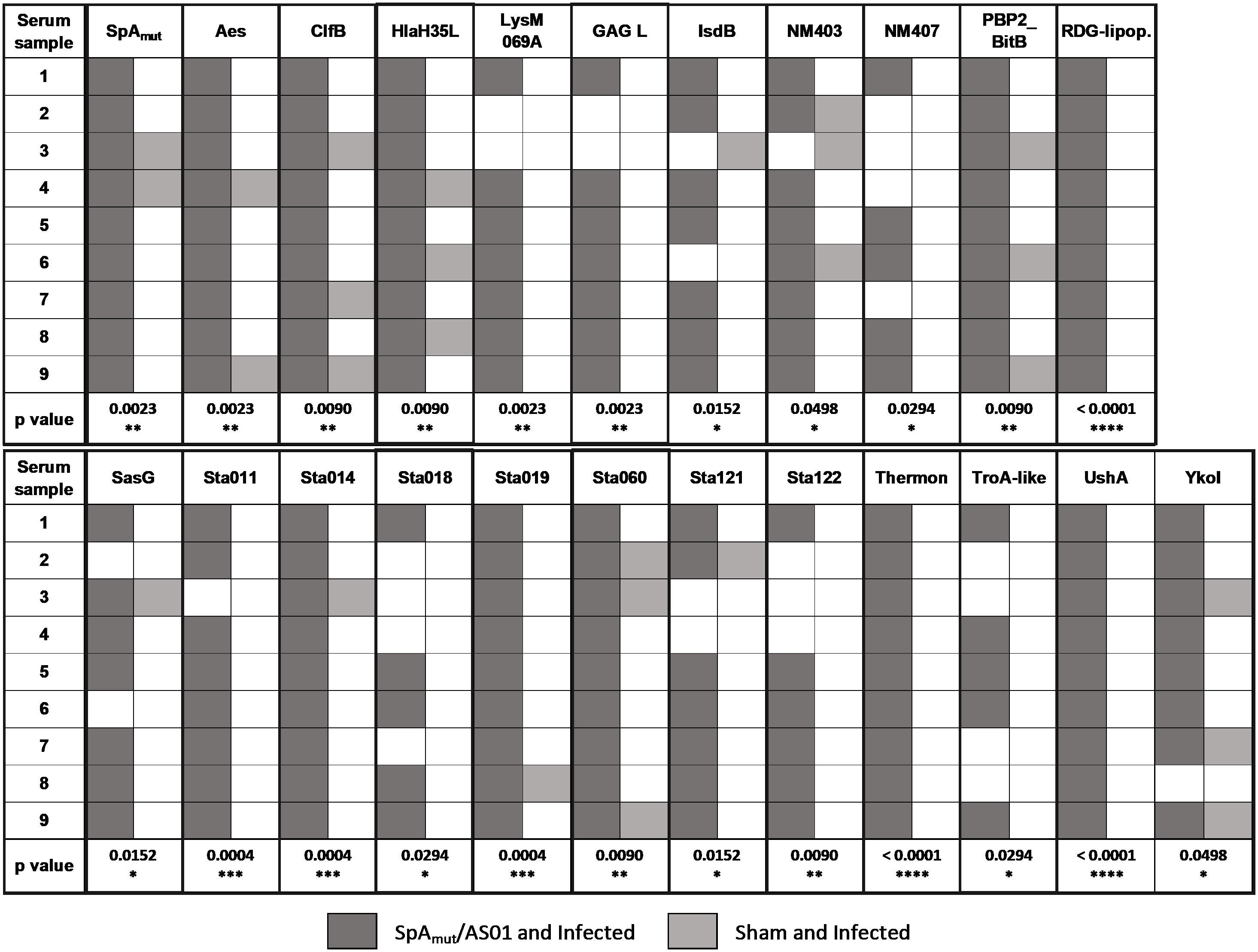
Figure 4 Vaccination with SpAmut/AS01 expands the antibody repertoire following an S. aureus infection. Nine single sera obtained from immunized and infected (SpAmut/AS01 and infected) and from infected-only animals (sham and infected) were assessed against the staphylococcal protein microarray. Out of the 96 antigens spotted on the array, 23 reacted with higher frequency with SpAmut/AS01 and infected sera, with respect to sera obtained from infected-only animals. Filled box: reactive serum; non-filled box: non-reactive serum. GAG L: Glycosaminoglycan lyase; RDG-lipop.: RDG lipoprotein; Thermon: thermonuclease. *pval< 0.05; **pval< 0.01; ***pval< 0.001; ****pval< 0.0001. Fisher’s exact t-test was used to evaluate significant differences between recognition frequencies for each antigen.
The area under the curve (AUC) was used to perform a cumulative data analysis on the dermonecrotic lesion areas (Figure 2A). The trapezoidal AUC of the dermonecrotic lesion areas, for a single day, was calculated using the following formula:
where B stands for major base, b denotes minor base, and t stands for time point.
The AUC was calculated for each time point after infection in the single animal and eventually summed together to have a final AUC value representing the whole time period after the infection.
Results
Immunization with SpAmut/AS01 protects mice against systemic sequelae in a model of Staphylococcus aureus skin infection and recurrences
Vaccination with SpAmut was shown to protect guinea pigs against systemic disease and to enable the host to develop a broader antibody repertoire against several staphylococcal antigens otherwise masked by SpA activity, which could protect from a second encounter with S. aureus (8). Given these premises, we developed an animal model to evaluate the contribution of SpA-specific antibodies in mediating the protection to systemic sequelae of skin infection recurrences.
To achieve this, C57BL/6 mice were immunized with SpAmut adjuvanted with AS01 (SpAmut/AS01). Four weeks after immunization, mice were inoculated subcutaneously (SC) in the right flank with S. aureus USA300 LAC strain (primary infection). Six weeks after the primary infection, mice were infected again with the same bacterial strain in the opposite flank (secondary infection), mimicking a skin infection recurrence (see Supplementary Figure 1 for the experimental design of the model). Different readouts were used to monitor the effect of vaccination and of the infection, both locally and systemically. Results were compared with those obtained with mock immunized mice, infected either once or twice.
A previous exposure to the pathogen contributed to reduce dermonecrotic lesions developed by mice during a second infection by approximately 80% (the median value of naïve mice was 103.3 mm2 and the median value of sham and infected mice was 18.25 mm2), but no additional effects due to a prior vaccination with SpAmut/AS01 were observed (Figure 2A). Mouse vaccination contributed nevertheless to significantly reduce bacterial load in the skin compared to the sham and infected mice (pval sham and infected vs. naïve was 0.07, pval SpAmut/AS01 and infected vs. naïve was 0.0057; Figure 2B). Notably, a pre-existing immunity present in sham and infected mice did not have any impact on the ability of S. aureus to migrate from the skin to the kidneys, while immunization with SpAmut/AS01 almost fully eradicated systemic complications during skin infection recurrence (Figure 2C).
We also evaluated a possible effect of immunization with the proposed vaccine in a model of skin infection but no reduction in dermonecrotic area size and CFU counts either in the skin or in the kidneys as observed (see Supplementary Material Figure 2).
S. aureus subcutaneous infection increases the affinity of anti-SpA antibodies following vaccination with SpAmut/AS01
Immunization with SpAmut/AS01 expanded the immune response to the pathogen after infection of mice, which overall contributed to control local disease (bacterial load in skin lesions) and bacterial dissemination to kidneys in a skin infection recurrence model.
In an attempt to understand the mechanisms underlying this observation, we firstly evaluated the antibody response generated against the vaccine candidate after immunization and infection. As shown in Figure 1, infection after vaccination did not boost the quantity of anti-SpAmut antibodies generated by vaccination itself. Interestingly, no antibodies against SpAmut were found in sera from sham and infected mice, underlying once more the ability of SpA to mask itself and, potentially, also other virulence factors to the host immune system (Figure 1).
We then wondered whether a change in the quality of anti-SpAmut IgG immune response could be detected after infection. IgG subclasses of anti-SpAmut specific antibodies were therefore analyzed in mouse sera, showing that no significant changes in the antibodies repertoire occurred during infection (Table 1). Eventually, affinity of anti-SpAmut IgG was characterized in sera collected from mice before and after subcutaneous infection. Importantly, anti-SpAmut antibodies strongly increased in affinity for their target during infection, with a relative percentage of IgG bound to the protein in the presence of 2 M ammonium thiocyanate that passed from 28% to 84%.
SpAmut/AS01 vaccination followed by S. aureus subcutaneous infection is associated with increased functionality and “broadness” of antibody response against the pathogen
The observed increased affinity might lead to more functional antibodies. To verify this hypothesis, the functionality of SpA-specific antibodies was evaluated in an in vitro displacement assay that measured the ability of anti-SpA IgG to displace human immunoglobulins bound to the wild-type SpA protein mainly through the Fc region (SpAWT). Figure 5 shows that immunoglobulins purified from vaccinated-only or infected-only mice sera displaced human IgG bound to the wild-type protein (40% at 500 µg/mL of purified IgGs) with a twofold increase as compared to the negative control group (almost 20% of background displacement). This effect was strongly enhanced when IgG purified from animals immunized and then infected were used with a 60% IgG displacement and an increase of threefold as compared to the negative control.
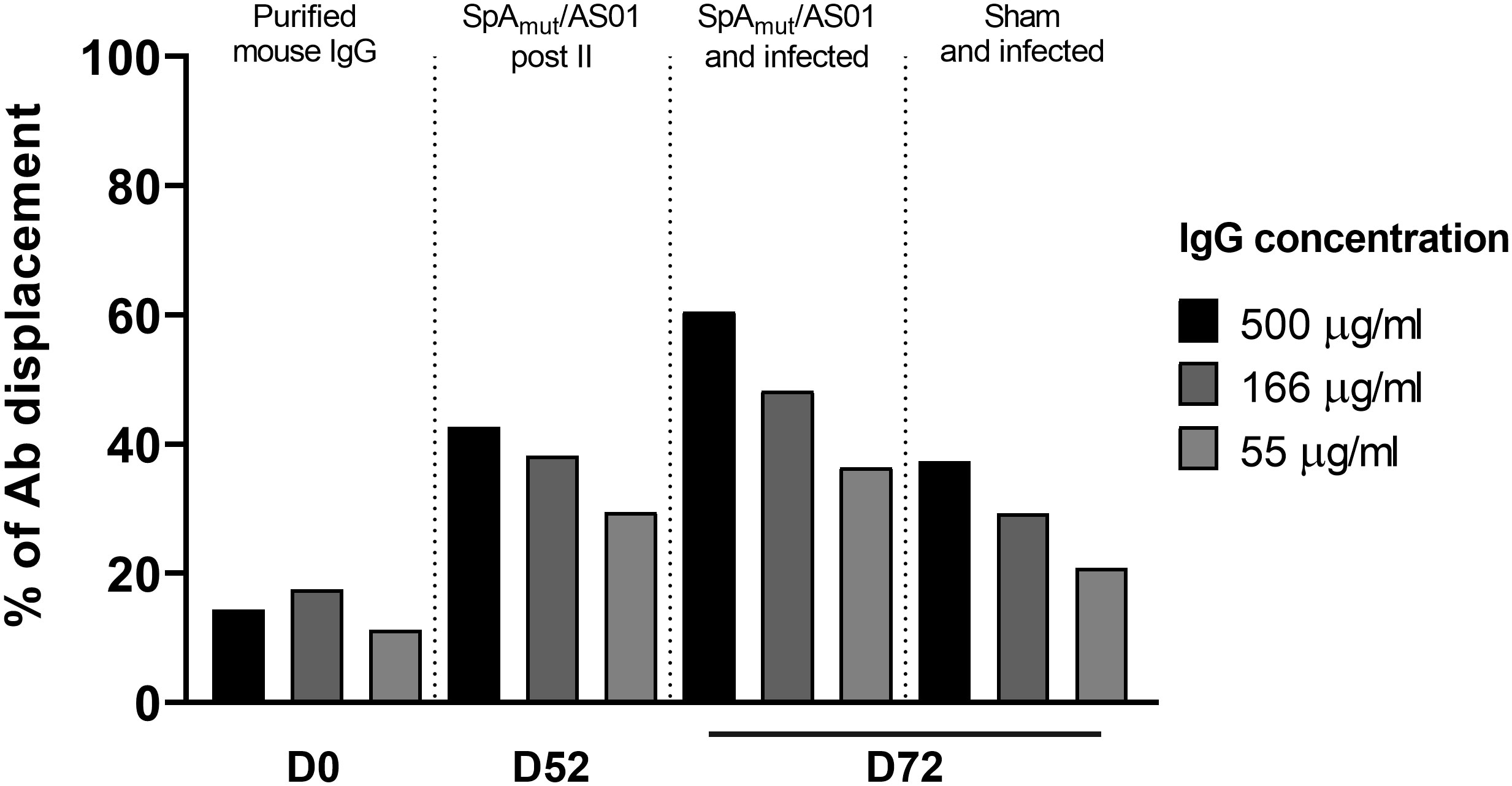
Figure 5 S. aureus infection increases the ability of vaccination to induce antibodies that allow in vitro human IgG displacement from SpA wild-type protein. Percentage of human IgG displaced from SpAWT-coupled beads by purified IgGs from sera of animals only immunized with SpAmut/AS01 or immunized and infected animals. Results were expressed as the percentage of human antibody displaced from the SpAWT, normalizing on the background signal.
Moreover, immunization with SpA derivatives followed by S. aureus infection was previously shown to increase antibody response against several other S. aureus virulence factors (1, 8). Sera from sham and infected (only infected animals) and SpAmut/AS01 and infected mice (animals immunized with SpAmut/AS01 before subcutaneous S. aureus infection) were therefore compared for their ability to recognize S. aureus antigens on a protein chip where up to 96 antigens were spotted (see Supplementary Material Table 2 for the complete list of antigens). Among these 96 virulence factors, 23 reacted with higher frequencies when sera from SpAmut/AS01 immunized and infected mice were used as compared to sera from sham and infected animals. Results with the 23 antigens are shown in Figure 4 and Table 2.

Table 2 List of the 23 antigens that reacted with higher frequency with sera from immunized and infected mice.
Sera from SpAmut/AS01 vaccinated and infected mice induces phagocytosis of S. aureus by human monocytic cells
Sera of SpAmut/AS01 and infected mice, as compared to sera from only vaccinated or sham and infected mice, elicited antibodies that (i) showed an increased affinity for SpA,(ii) reduced SpA ability to bind immunoglobulins (Figure 5), and (iii) recognized more S. aureus virulence factors both secreted and exposed on the bacterial surface (Figure 4; Table 2). Antibodies present in sera of these animals before the second subcutaneous infection (recurrence) could therefore facilitate the clearance of bacteria, and this might explain why spreading of S. aureus from skin to kidneys was highly impaired in SpAmut/AS01-immunized and infected animals.
To further evaluate the contribution of SpA-specific antibodies in the elimination of S. aureus from the blood circulation, a human monocytic cell line (THP-1) was used to evaluate bacterial internalization in the presence of sera from different groups of mice. As reported in Figure 3, neither vaccination of mice with SpAmut/AS01 (fold change equal to 0.7 with respect to the pre-immune sera, 10% percentile was 0.23, and 90% percentile was 1.18) nor bacterial infection (fold change equal to 3 with respect to the pre-immune sera, 10% percentile was 0.84, and 90% percentile was 5.8) significantly enhanced phagocytosis as compared to the negative control. Remarkably, the combination of vaccination and infection generated sera that significantly increased the phagocytic properties of THP-1 cells compared to the negative control (fold change equal to 5, 10% percentile was 2.8, and 90% percentile was 10, pval = 0.0007) and, interestingly, also to vaccination alone (pval< 0.0001).
Discussion
In the present study, we have demonstrated that the efficacy of a vaccine candidate, especially if it contains an immunomodulator virulence factor, could go beyond a direct effect of antibodies and cellular response against the antigen itself. A detoxified version of Staphylococcal protein A (SpAmut), adjuvanted with AS01, triggered the mouse immune system to mount a broader and more mature serological response against S. aureus during a first encounter with the pathogen, which importantly protected the animals against a second infection. Considering that skin infection and recurrences are one of the most frequent S. aureus-mediated disease in humans, the results obtained in an animal model somehow mimicking the human disease are particularly relevant.
Differently from mice, humans are often pre-exposed to S. aureus, and this can have an impact on the immune response elicited by a subsequent vaccination and on the effect mediated by SpA (“indirect” protection mediated by SpA-antibodies). Follow-up studies should be done to further address this point.
SpA is a well-known immunomodulator virulence factor of S. aureus that significantly contributes to make this opportunistic pathogen one of the best adapted bacteria to human hosts (36). When exposed on bacterial surface, SpA binds the Fc (Fragment crystallizable) portion of human immunoglobulins coating S. aureus with a kind of “host self-dress”, a mask that makes this pathogen (and its virulence factors) hidden to the host immune system (37). Additionally, when secreted, SpA binds the VH3 domain of the Fab (Fragment antigen binding) region present on IgM/IgD receptors on the surface of B lymphocytes inducing anergy and preventing the maturation of a long-lasting memory response (12).
Overall, the functionality of anti-S. aureus antibodies is impaired under these conditions, and human defenses against S. aureus are therefore often inefficient in clearing the infection.
Recurrences and bloodstream dissemination, both strictly connected to the ability of S. aureus to hide in the immune system, are the hallmark of SSTIs and exemplify the inability of infected hosts to establish a protective immunity (1, 38, 39).
SpA has already been tested as a vaccine candidate in animal models against S. aureus-mediated infections, particularly against systemic disease (8, 40, 41). In these publications, researchers demonstrated that a detoxified derivative of SpA protected animals against intravenous infection with S. aureus. Mice and guinea pigs immunized with SpA and infected with S. aureus, if compared to only infected animals, mounted a broader antibody response against several S. aureus virulence factors otherwise masked by SpA activity. Finally, a possible positive impact that this unmasking effect could have against future infections was speculated (8, 41).
Based on these observations, a mouse model of skin infection and recurrence has been chosen to (i) evaluate whether immunization with SpA could unmask the pathogen also in a model of infection that resembled the most frequent staphylococcal disease in humans; (ii) measure the impact that this unmasking effect could have against a future encounter with bacteria in such a model.
Vaccination with SpAmut/AS01 did not directly protect animals against skin infections and related sequelae but triggered the host to mount a protective immune response during this first encounter, which proved to have a crucial importance for a second infection (Figure 2; see also Supplementary Figure 1). In fact, the immunization with the proposed vaccine contributed not only to increase the number of S. aureus virulence factors recognized during infection but also to improve the quality of the mounted immune response, which strongly limited the ability of S. aureus to use its elusive properties when reinfecting the host.
Despite being highly immunogenic, vaccination with SpAmut/AS01 had, per se, no or very poor effect against a primary S. aureus skin infection and related systemic complications (Supplementary Figure 1). Infection with the pathogen did not quantitatively boost the IgG response against SpA over the level obtained by immunization itself but, nevertheless, positively affected the quality of these antibodies (Figure 1). Anti-SpA antibodies in sera of vaccinated mice after a first encounter with S. aureus had an increased affinity for the target and showed higher efficacy in displacing IgGs bound by SpA, presumably also in vivo (Figure 5). Anti-SpA-specific IgG indeed confirmed to “undress” the pathogen during infection since sera from vaccinated and infected animals can recognize more antigens during infection as compared to sera from negative control animals (Figure 4; Supplementary Table 2). This increment was quantitatively relevant: while 20 out of 96 antigens assessed induced detectable antibodies during S. aureus infection in at least five out of nine sera tested, this number grew up to 53 in the presence of anti-SpA specific IgGs (Fisher’s exact test, pval< 0.0001). Localization of these antigens on the bacteria is very broad. Out of 23, 9 (39.1%) were secreted molecules, which are indeed more easily recognized by the immune system, followed by cell-wall-associated antigens (8 out of 23, 34.8%), antigens located in the periplasmic space (3 out of 23, 13%), molecules anchored to the cell membrane (2 out of 23, 8.7%), and, finally, cytoplasmic proteins (1 out of 23, 4.3%). Additionally, most of them are well-known virulence factors involved in biofilm formation or known to contribute to S. aureus invasiveness and colonization (Table 2, Figure 6). Finally, sera from immunized and infected animals showed the highest capacity to favor phagocytosis of bacteria by human monocytic cells in vitro as compared to sera of all the other groups (Figure 3). The impact of the general improvement of the IgG response against the pathogen resulted in significant reduction of bacterial load in the site of infection and, surprisingly, an almost complete eradication of bacteria in the kidneys used as a marker of systemic dissemination (Figure 2). Taken together, these data suggest that all these positive effects could play an important role against different pathogenic mechanisms, increasing the ability of the host to clear the infection. The occurrence of both these results, indeed, required the animals not only to be vaccinated with SpA, but also to be infected. Interestingly, a trend in CFU count reduction in skin homogenates was also observed in infected-only animals as compared to naïve mice, but this effect did not correlate with a systemic protection (Figure 2). Since alpha toxin (Hla) plays a central role for the development of dermonecrotic lesions and bacteria growth in the site of infection and since infection per se was sufficient to induce detectable anti-Hla antibodies, we might postulate that these antibodies might have played an important role to control skin infection (42, 43). On the other hand, the control of systemic sequelae probably needed a more diverse and complete immune response, like that developed by vaccinated and infected mice.
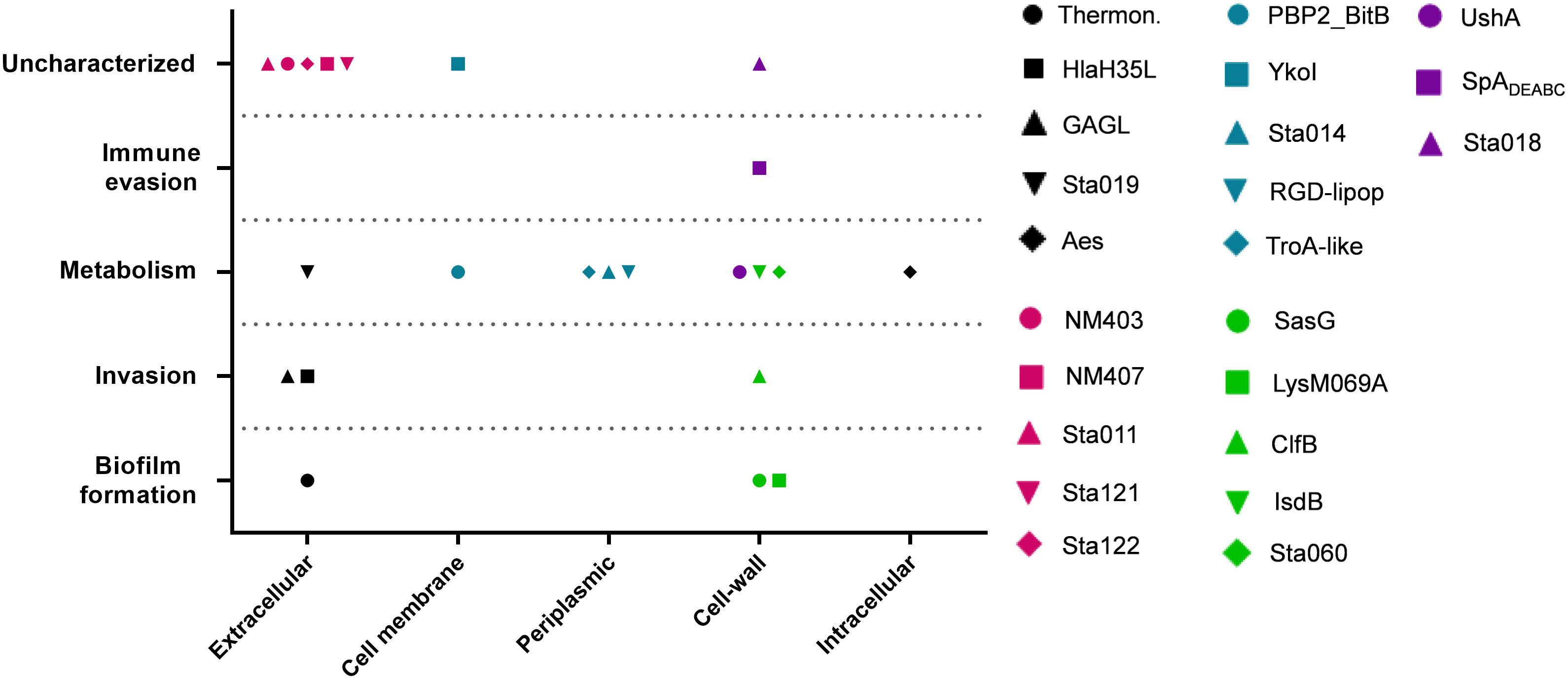
Figure 6 Correlation between cellular localization and functionality of recognized antigens. Graph summarizing cellular localization and pathogenic activity of the S. aureus virulence factors recognized by sera from SpAmut/AS01 immunized and S. aureus-infected mice. The 23 antigens recognized with higher frequency by sera from immunized and infected animals were further characterized by literature check and using the gene annotation present in NCBI data bank for their cellular localization (X axis) and in vivo/in vitro functionality (Y axis). Single dots reported information for single antigens as described in the legend. CSA family: conserved staphylococcal antigen family (32).
Interestingly, since human neutrophils utilize the same FcγRs to trigger phagocytosis, we could hypothesize that this mechanism of protection could also be extended in humans during a systemic spreading of S. aureus, even if in vitro phagocytosis performed with a human monocytic cell line could not directly correlate with a possible protection in vaccinated people (44–47).
To conclude, even if we could not exclude the idea that the cellular response may have had a partial contribution on the overall observed protection, IgG-mediated opsonophagocytosis is certainly one of the most important mechanisms of bacterial clearance that could explain our results (16, 48). These data strongly support the proposal to use this antigen in a vaccine against S. aureus-mediated SSTIs, and it would be better if it was combined with additional antigens that could offer direct protection during a first encounter with the pathogen.
Data availability statement
The original contributions presented in the study are included in the article/Supplementary Material. Further inquiries can be directed to the corresponding author.
Ethics statement
The animal study was approved by Italian Ministry of Health (authorization 123/2015-PR). The study was conducted in accordance with the local legislation and institutional requirements.
Author contributions
AM: Data curation, Methodology, Writing – original draft, Writing – review & editing. GM: Data curation, Methodology, Writing – review & editing. MT: Methodology, Writing – review & editing. ST: Methodology, Writing – review & editing. DL: Writing – review & editing. FB: Writing – review & editing. OF: Writing – review & editing. GB: Writing – review & editing, Supervision. MB: Writing – review & editing, Supervision. EC: Data curation, Formal analysis, Methodology, Supervision, Writing – original draft, Writing – review & editing.
Funding
The author(s) declare that no financial support was received for the research, authorship, and/or publication of this article.
Acknowledgments
We thank Elena Boero for the support on the opsonophagocytosis assay, Viola Viviani per the protein microarray and related data analysis, Patrizia Giannetti for vaccine formulation, Bruna Clemente and Andrea G. O. Manetti for the displacement assay. We also thank Bruno Galletti, Fabiana Spensieri and Stefano Bonacci for supporting with all the Luminex analyses. A special acknowledgment is due to Raffaella Cecchi, Tommaso Pasquali and all the GSK Animal Resources Center, Siena, Italy for their excellent support with the in vivo studies. We also thank Professor Chiara Falciani for all the precious support and critically revising data. Finally, we deeply thank Laura Pancotto, who supported study design and analyses.
Conflict of interest
AM was a PhD student of Università degli Studi di Siena Italy at the time of the study and supervised by GSK. GM was a student of Università di Bologna Italy at the time of the study and participated in an internship at GSK. AM is now an employee of GiGroup SpA, a contract employment organization contracted by GSK. GM is now an employee of GSK. DL and GB were employee of GSK at the time of the study. All the other authors are employee of GSK. FB, MB, GB, EC report ownership of GSK shares. FB is listed as inventor on patents concerning S. aureus vaccine development.
The author(s) declared that they were an editorial board member of Frontiers, at the time of submission. This had no impact on the peer review process and the final decision.
Publisher’s note
All claims expressed in this article are solely those of the authors and do not necessarily represent those of their affiliated organizations, or those of the publisher, the editors and the reviewers. Any product that may be evaluated in this article, or claim that may be made by its manufacturer, is not guaranteed or endorsed by the publisher.
Supplementary material
The Supplementary Material for this article can be found online at: https://www.frontiersin.org/articles/10.3389/fimmu.2024.1355764/full#supplementary-material
References
1. Tong SYC, Davis JS, Eichenberger E, Holland TL, Fowler VG. Staphylococcus aureus infections: epidemiology, pathophysiology, clinical manifestations , and management. Clin Microbiol Rev. (2015) 28:603–61. doi: 10.1128/CMR.00134-14
2. van Belkum A, Melles DC, Nouwen J, van Leeuwen WB, van Wamel W, Vos MC, et al. Co-evolutionary aspects of human colonisation and infection by Staphylococcus aureus. Infect Genet Evol. (2009) 9:32–47. doi: 10.1016/j.meegid.2008.09.012
3. Broughan J, Anderson R, Anderson AS. Strategies for and advances in the development of Staphylococcus aureus prophylactic vaccines. Expert Rev Vaccines. (2011) 10:695–708. doi: 10.1586/erv.11.54
4. McCaig LF, McDonald LC, Mandal S, Jernigan DB. Staphylococcus aureus-associated skin and soft tissue infections in ambulatory care. Emerg Infect Dis. (2006) 12:1715–23. doi: 10.3201/eid1211.060190
5. Hersh AL, Chambers HF, Maselli JH, Gonzales R. National trends in ambulatory visits and antibiotic prescribing for skin and soft-tissue infections. Arch Intern Med. (2008) 168:1585–91. doi: 10.1001/archinte.168.14.1585
6. Ray GT, Suaya JA, Baxter R. Incidence, microbiology, and patient characteristics of skin and soft-tissue infections in a U.S. population: A retrospective population-based study. BMC Infect Dis. (2013) 13:1–11. doi: 10.1186/1471-2334-13-252
7. Tattevin P, Schwartz BS, Graber CJ, Volinski J, Bhukhen A, Bhukhen A, et al. Concurrent epidemics of skin and soft tissue infection and bloodstream infection due to staphylococcus aureus. Clin Infect Dis. (2012) 55:781–8. doi: 10.1093/cid/cis527
8. Kim HK, Falugi F, Thomer L, Missiakas DM, Schneewind O. Protein a suppresses immune responses during Staphylococcus aureus bloodstream infection in Guinea pigs. MBio. (2015) 6:1–11. doi: 10.1128/mBio.02369-14
9. Whitehouse J, Flaxman A, Rollier C, O’Shea MK, Fallowfield J, Lindsay M, et al. Population variation in anti-S. aureus IgG isotypes influences surface protein A mediated immune subversion. Vaccine. (2016) 34:1792–9. doi: 10.1016/j.vaccine.2016.02.034
10. Peterson PK, Verhoef J, Sabath LD, Quie PG. Effect of protein A on staphylococcal opsonization. Infect Immun. (1977) 15:760–4. doi: 10.1128/iai.15.3.760-764.1977
11. Falugi F, Kim HK, Missiakas DM, Schneewind O. Role of protein a in the evasion of host adaptive immune responses by Staphylococcus aureus. MBio. (2013) 4. doi: 10.1128/mBio.00575-13
12. Kobayashi SD, DeLeo FR. Staphylococcus aureus protein A promotes immune suppression. MBio. (2013) 4:4–6. doi: 10.1128/mBio.00764-13
13. Levinson AI, Kozlowski L, Zheng Y, Wheatley L. B-Cell superantigens: Definition and potential impact on the immune response. J Clin Immunol. (1995) 15:26–36. doi: 10.1007/BF01540891
14. Silverman GJ, Goodyear CS. Confounding B-cell defences: Lessons from a staphylococcal superantigen. Nat Rev Immunol. (2006) 6:465–75. doi: 10.1038/nri1853
15. Graille M, Stura EA, Corper AL, Sutton BJ, Taussig MJ, Charbonnier JB, et al. Crystal structure of a Staphylococcus aureus protein a domain complexed with the Fab fragment of a human IgM antibody: Structural basis for recognition of B-cell receptors and superantigen activity. Proc Natl Acad Sci U.S.A. (2000) 97:5399–404. doi: 10.1073/pnas.97.10.5399
16. Kim HK, Emolo C, DeDent AC, Falugi F, Missiakas DM, Schneewind O. Protein A-specific monoclonal antibodies and prevention of staphylococcus aureus disease in mice. Infect Immun. (2012) 80:3460–70. doi: 10.1128/IAI.00230-12
17. Boero E, Cruz AR, Pansegrau W, Giovani C, Rooijakkers SHM, van Kessel KPM, et al. Natural human immunity against staphylococcal protein A relies on effector functions triggered by igG3. Front Immunol. (2022) 13:834711. doi: 10.3389/fimmu.2022.834711
18. Scietti L, Sampieri K, Pinzuti I, Bartolini E, Benucci B, Liguori A, et al. Exploring host-pathogen interactions through genome wide protein microarray analysis. Sci Rep. (2016) 6:1–14. doi: 10.1038/srep27996
19. Rigat F, Bartolini E, Dalsass M, Kumar N, Marchi S, Speziale P, et al. Retrospective identification of a broad IgG repertoire differentiating patients with S. aureus skin and soft tissue infections from controls. Front Immunol. (2019) 10:114. doi: 10.3389/fimmu.2019.00114
20. Bagnoli F, Fontana MR, Soldaini E, Mishra RPN, Fiaschi L, Cartocci E, et al. Vaccine composition formulated with a novel TLR7-dependent adjuvant induces high and broad protection against Staphylococcus aureus. Proc Natl Acad Sci U.S.A. (2015) 112:3680–5. doi: 10.1073/pnas.1424924112
21. Tang F, Li L, Meng X, Li B, Wang C, Wang S, et al. Microbial Pathogenesis Inhibition of alpha-hemolysin expression by resveratrol attenuates Staphylococcus aureus virulence. Microb Pathog. (2019) 127:85–90. doi: 10.1016/j.micpath.2018.11.027
22. Adhikari RP, Thompson CD, Aman MJ, Lee JC. Protective efficacy of a novel alpha hemolysin subunit vaccine ( AT62 ) against Staphylococcus aureus skin and soft tissue infections. Vaccine. (2016) 34:6402–7. doi: 10.1016/j.vaccine.2016.09.061
23. Tran VG, Venkatasubramaniam A, Adhikari RP, Krishnan S, Wang X, Le VTM, et al. Efficacy of active immunization with attenuated α-hemolysin and panton-valentine leukocidin in a rabbit model of staphylococcus aureus necrotizing pneumonia. J Infect Dis. (2020) 221:267–75. doi: 10.1093/infdis/jiz437
24. Porayath C, Suresh MK, Biswas R, Nair BG, Mishra N, Pal S. Autolysin mediated adherence of Staphylococcus aureus with Fibronectin, Gelatin and Heparin. Int J Biol Macromol. (2018) 110:179–84. doi: 10.1016/j.ijbiomac.2018.01.047
25. Pulia MS, Anderson J, Ye Z, Elsayed NS, Le T, Patitucci J, et al. Expression of staphylococcal virulence genes in situ in human skin and soft tissue infections. Antibiotics. (2022) 11:1–11. doi: 10.3390/antibiotics11040527
26. Zheng X, Ma X, John S. The major autolysin atl regulates the virulence of staphylococcus aureus by controlling the sorting of lukAB. Infect Immun. (2022) 90:1–13. doi: 10.1128/iai.00056-22
27. Ibberson CB, Parlet CP, Kwiecinski J, Crosby HA, Meyerholz DK, Horswill AR. Hyaluronan modulation impacts Staphylococcus aureus biofilm infection. Infect Immun. (2016) 84:1917–29. doi: 10.1128/IAI.01418-15
28. Torres VJ, Pishchany G, Humayun M, Schneewind O, Skaar EP. Staphylococcus aureus isdB is a hemoglobin receptor required for heme iron utilization. J Bacteriol. (2006) 188:8421–9. doi: 10.1128/JB.01335-06
29. Tsai CM, Caldera JR, Hajam IA, Chiang AWT, Tsai CH, Li H, et al. Non-protective immune imprint underlies failure of Staphylococcus aureus IsdB vaccine. Cell Host Microbe. (2022) 30:1163–72. doi: 10.1016/j.chom.2022.06.006
30. Lacey KA, Mulcahy ME, Towell AM, Geoghegan JA, McLoughlin RM. Clumping factor B is an important virulence factor during Staphylococcus aureus skin infection and a promising vaccine target. PloS Pathog. (2019) 15:1–20. doi: 10.1371/journal.ppat.1007713
31. Schaffer AC, Solinga RM, Cocchiaro J, Portoles M, Kiser KB, Risley A, et al. Immunization with staphylococcus aureus clumping factor B , a major determinant in nasal carriage , reduces nasal colonization in a murine model. Infect Immun. (2006) 74:2145–53. doi: 10.1128/IAI.74.4.2145
32. Schluepen C, Malito E, Marongiu A, Schirle M, McWhinnie E, Lo Surdo P, et al. Mining the bacterial unknown proteome: identification and characterization of a novel family of highly conserved protective antigens in Staphylococcus aureus. Biochem J. (2013) 455:273–84. doi: 10.1042/BJ20130540
33. Schaeffer CR, Woods KM, Longo GM, Kiedrowski MR, Paharik AE, Büttner H, et al. Accumulation-associated protein enhances Staphylococcus epidermidis biofilm formation under dynamic conditions and is required for infection in a rat catheter model. Infect Immun. (2015) 83:214–26. doi: 10.1128/IAI.02177-14
34. Wang M, Buist G, van Dijl JM. Staphylococcus aureus cell wall maintenance – the multifaceted roles of peptidoglycan hydrolases in bacterial growth, fitness, and virulence. FEMS Microbiol Rev. (2022) 46(5):1–19. doi: 10.1093/femsre/fuac025
35. Yu J, Jiang F, Zhang F, Hamushan M, Du J, Mao Y, et al. Thermonucleases contribute to staphylococcus aureus biofilm formation in implant-associated infections–A redundant and complementary story. Front Microbiol. (2021) 12:687888. doi: 10.3389/fmicb.2021.687888
36. Howden BP, Giulieri SG, Wong Fok Lung T, Baines SL, Sharkey LK, Lee JYH, et al. Staphylococcus aureus host interactions and adaptation. Nat Rev Microbiol. (2023) 21:380–95. doi: 10.1038/s41579-023-00852-y
37. De Jong NWM, Van Kessel KPM, Van Strijp JAG. Immune evasion by staphylococcus aureus. Gram-Positive Pathog. (2019) 1991:618–39. doi: 10.1128/9781683670131.ch39
38. Missiakas D, Schneewind O. Staphylococcus aureus vaccines: Deviating from the carol. J Exp Med. (2016) 213:1645–53. doi: 10.1084/jem.20160569
39. Ote H, Ito H, Akira T, Sugai M, Hisatsune J, Uehara Y, et al. A fatal case of disseminated infection caused by community-associated methicillin-resistant Staphylococcus aureus USA300 clone. Jpn J Infect Dis. (2023) 79(4):1–15. doi: 10.7883/yoken.JJID.2022.578
40. Shi M, Chen X, Sun Y, Kim HK, Schneewind O, Missiakas D. A protein A based Staphylococcus aureus vaccine with improved safety. Vaccine. (2021) 39:3907–15. doi: 10.1016/j.vaccine.2021.05.072
41. Kim HK, Cheng AG, Kim HY, Missiakas DM, Schneewind O. Nontoxigenic protein A vaccine for methicillin-resistant staphylococcus aureus infections in mice. J Exp Med. (2010) 207:1863–70. doi: 10.1084/jem.20092514
42. Sampedro GR, De Dent AC, Becker REN, Berube BJ, Gebhardt MJ, Cao H, et al. Targeting Staphylococcus aureus α-toxin as a novel approach to reduce severity of recurrent skin and soft-tissue infections. J Infect Dis. (2014) 210:1012–8. doi: 10.1093/infdis/jiu223
43. Teymournejad O, Li Z, Beesetty P, Yang C, Montgomery CP. Toxin expression during Staphylococcus aureus infection imprints host immunity to inhibit vaccine efficacy. NPJ Vaccines. (2023) 8. doi: 10.1038/s41541-022-00598-3
44. Fleit HB, Kobasiuk CD. The human monocyte-like cell line THP-1 expresses Fc gamma RI and Fc gamma RII The Human Cell Line THP-1 Expresses FcyRl and FcyRIl. J Leukoc Biol. (1991) 49:556–65. doi: 10.1002/jlb.49.6.556
45. Wang Y, Jönsson F. Expression, role, and regulation of neutrophil Fcγ receptors. Front Immunol. (2019) 10:1958. doi: 10.3389/fimmu.2019.01958
46. Forrester MA, Wassall HJ, Hall LS, Cao H, Wilson HM, Barker RN, et al. Similarities and differences in surface receptor expression by THP-1 monocytes and differentiated macrophages polarized using seven different conditioning regimens. Cell Immunol. (2018) 332:58–76. doi: 10.1016/j.cellimm.2018.07.008
47. Lee WL, Harrison RE, Grinstein S. Phagocytosis by neutrophils. Microbes Infect. (2003) 5:1299–306. doi: 10.1016/j.micinf.2003.09.014
Keywords: immunomodulators, SSTIs, mouse model, Staphylococcus, bacteremia
Citation: Mandelli AP, Magri G, Tortoli M, Torricelli S, Laera D, Bagnoli F, Finco O, Bensi G, Brazzoli M and Chiarot E (2024) Vaccination with staphylococcal protein A protects mice against systemic complications of skin infection recurrences. Front. Immunol. 15:1355764. doi: 10.3389/fimmu.2024.1355764
Received: 14 December 2023; Accepted: 16 February 2024;
Published: 11 March 2024.
Edited by:
Giampiero Pietrocola, University of Pavia, ItalyReviewed by:
Timothy J. Foster, Trinity College Dublin, IrelandJohn Fraser, The University of Auckland, New Zealand
Copyright © 2024 Mandelli, Magri, Tortoli, Torricelli, Laera, Bagnoli, Finco, Bensi, Brazzoli and Chiarot. This is an open-access article distributed under the terms of the Creative Commons Attribution License (CC BY). The use, distribution or reproduction in other forums is permitted, provided the original author(s) and the copyright owner(s) are credited and that the original publication in this journal is cited, in accordance with accepted academic practice. No use, distribution or reproduction is permitted which does not comply with these terms.
*Correspondence: Emiliano Chiarot, ZW1pbGlhbm8ueC5jaGlhcm90QGdzay5jb20=