- 1Department of Internal Medicine I, University Hospital Würzburg, Würzburg, Germany
- 2Institute for Experimental Cardiovascular Research, University Medical Center Hamburg-Eppendorf, Hamburg, Germany
- 3German Center for Cardiovascular Research (DZHK), partner site Hamburg/Kiel/Lübeck, Hamburg, Germany
- 4Comprehensive Heart Failure Center, University Hospital Würzburg, Würzburg, Germany
T-cell activation is a pivotal process of the adaptive immune response with 3′,5′-cyclic adenosine monophosphate (cAMP) as a key regulator of T-cell activation and function. It governs crucial control over T-cell differentiation and production of pro-inflammatory cytokines, such as IFN-γ. Intriguingly, levels of intracellular cAMP differ between regulatory (Treg) and conventional T-cells (Tcon). During cell-cell contact, cAMP is transferred via gap junctions between these T-cell subsets to mediate the immunosuppressive function of Treg. Moreover, the activation of T-cells via CD3 and CD28 co-stimulation leads to a transient upregulation of cAMP. Elevated intracellular cAMP levels are balanced precisely by phosphodiesterases (PDEs), a family of enzymes that hydrolyze cyclic nucleotides. Various PDEs play distinct roles in regulating cAMP and cyclic guanosine monophosphate (cGMP) in T-cells. Research on PDEs has gained growing interest due to their therapeutic potential to manipulate T-cell responses. So far, PDE4 is the best-described PDE in T-cells and the first PDE that is currently targeted in clinical practice to treat autoimmune diseases. But also, other PDE families harbor additional therapeutic potential. PDE2A is a dual-substrate phosphodiesterase which is selectively upregulated in Tcon upon activation. In this Mini-Review, we will highlight the impact of cAMP regulation on T-cell activation and function and summarize recent findings on different PDEs regulating intracellular cAMP levels in T-cells.
1 Introduction
Since its initial discovery in 1957, multifaceted roles in different cell types have been discovered for the second messenger 3′,5′-cyclic adenosine monophosphate (cAMP) (1). Importantly, cAMP as pivotal regulator of the adaptive immune system exerts control over T-cell activation, differentiation, and the production of pro-inflammatory cytokines like IFN-γ (2, 3). cAMP is generated from adenosine triphosphate (ATP) by adenylyl cyclases (ACs). Initiation of cAMP synthesis is facilitated by the binding of extracellular ligands, including cytokines, catecholamines, and adenosine, to various stimulatory G-protein coupled receptors (GPCRs). Ligand binding induces a conformational change of the GPCR and subsequent dissociation of Gα and Gβγ-subunits. Gα binds to ACs, leading to generation of cAMP (4). Among 10 mammalian AC isoforms, AC1-9 are membrane-bound and only AC10 is known to be soluble (5). In T-cells, AC7 is the predominant isoform, although AC3, AC6 and AC9 are also expressed (6, 7). The knock-out of AC7 in the hematopoietic system of mice exhibits a reduced total number of leukocytes and an impaired immune response against T-cell dependent antigens (7). Elevated intracellular cAMP levels activate several effector molecules. Notably, the binding of cAMP to the protein kinase A (PKA) is the best-characterized interaction downstream of the cAMP signaling pathway. The induced dissociation of the two regulatory subunits of PKA enables the phosphorylation of threonine and serine residues in various proteins e.g., cAMP-response element binding protein (CREB), cAMP-response element modulator/inducible cAMP early repressor (CREM/ICER) or the nuclear factor-κB (NF-κB) (8–10). High levels of cAMP are balanced by phosphodiesterases (PDEs), a superfamily of enzymes with the ability to degrade cyclic nucleotides, cAMP or cyclic guanosine monophosphate (cGMP) to 5’-AMP and 5’-GMP, respectively (11). Over 100 different isoforms are described in mammals (12). The distinct expression of PDEs, ACs and the A-kinase anchoring proteins enable to formation of local cAMP pools, allowing compartmentalization of cAMP signaling within micro- or nanodomains rather than eliciting a global response within the cell (13).
2 cAMP in different T-cell subsets
Naïve T-cells can differentiate into various T-cell subsets with a specific expression pattern of cytokine receptors after their contact with antigens presented by antigen-presenting cells (APCs). Ninety-five percent are conventional T-cells (Tcon) whereas the other five percent of the T-cell population are regulatory T-cells (Treg) expressing the transcription factor Forkhead box protein-3 (Foxp3), known to be indispensable for proper Treg development and function (14, 15). Tregs can either be derived from the thymus, or differentiated afterwards. Mice lacking Tregs develop a severe autoimmune disorder (16). Research in the last few years has been focused on how Tregs are able to fulfill their immunosuppressive function. Among other mechanisms, the cAMP signaling pathway plays a crucial role. Tregs harbor much higher cAMP levels compared to Tcon, which can be further increased after T-cell activation (17). The Treg- specific transcription factor Foxp3 governs the ability to downregulate PDE3B and subsequently block cAMP degradation by PDE3B in Treg (18). Concurrently, the single microRNA miR-142-3p, which is selectively expressed in Treg, elevates the cAMP levels by enhancing the expression of AC9 (6). The knock-out of miR-142-3p in murine T-cells leads to elevated gene expression related to the IFN-γ signaling pathway and high production of IFN-γ (19). Moreover, treatment of different T-cell subsets with IL-2 uncovers the upregulation of AC7 in Tregs and the downregulation in Tcon (20). Secondly, Treg express CD39 and CD73, ectoenzymes on the cell surface with the ability to convert ATP to adenosine (ADO). Subsequently, ADO activates A2AR on Tcon and APCs, which increases cAMP production (21). Lastly, there are no extracellular receptors known for cAMP, but cAMP can be transferred from Treg to Tcon via gap junctions. Co-culture of both T-cell subsets leads to increased cAMP levels in Tcon (17). This transfer and increase of cAMP influence the nuclear localization of CREM/ICER in activated T-cells and decrease IL-2 production, a cytokine presented to Tregs to increase its suppressive activity (22).
3 cAMP during T-cell activation
APCs present pathogen fragments in a complex with major histocompatibility complex I or II (MHCI/II) to naïve T-cells for activation. These fragments stimulate T-cell receptors (TCR) leading to multiple intracellular signaling cascades. Additionally, a costimulatory signal of CD28 binding to B7.1/B7.2 is required for T-cell activation and Interleukin-2 (IL-2) synthesis (23, 24). Secreted IL-2 binds to IL-2 receptors on T-cells to promote T-cell differentiation. T-cell activation leads to transiently elevated cAMP levels (25). After stimulation of TCR, cAMP is produced in lipid rafts, which is followed by an increased raft-associated PKA activity (26). Among others, the C-terminal Src kinase (Csk) is activated by PKA phosphorylation and inhibits the activity of the lymphocyte-specific protein tyrosine kinase Lck, subsequently leading to downregulation of T-cell receptor signaling (13, 27). However, targeting of regulatory PKA subunit RIα by binding to Ezrin, an A-kinase anchoring protein, is needed for the transport to lipid rafts and for the inhibition of the T-cell activation by the PKA-Csk pathway (28). Additionally, the co-stimulation of CD28 plays a distinct role in balancing TCR-induced cAMP production. In CD3 and CD28 co-stimulated cells, lipid raft-associated PDE4 activity is increased. CD28 mediates the recruitment of a β-arrestin/PDE4D complex to lipid rafts to enhance cAMP degradation (26). β-arrestins are inhibitors of activated GPCRs and terminate their signal transduction. Hence, increased PDE4 activity leads to an inhibitory feedback loop and lower cAMP levels (29). Interestingly, the recruitment of β-arrestin/PDE4 to lipid rafts is also regulated by PI3K (30). Stimulation of CD28 increased PIP3 production via enhanced PI3K activity and β-arrestin can interact with PIP3 via the PH domain-containing protein (PKB). The siRNA mediated knock-down of β-arrestin 1 and 2 in primary T-cells leads to decreased IL-2 and IFN-γ production (30). Recently, it has been shown that high levels of cAMP upregulate the cytotoxic T-lymphocyte-associated protein 4 (CTLA-4) in Tcon and inhibit the binding of CD28 (31).
4 Physiological role of Phosphodiesterases in T-cells
Phosphodiesterases (PDEs), a superfamily of 11 enzyme families (PDE1-11), are responsible for balancing intracellular cyclic nucleotide levels. PDEs have different affinities for cAMP or cGMP. PDE 5, 6 and 9 hydrolyze only cGMP, whereas PDE4, 7 and 8 can selectively bind cAMP, and PDE1-3, 10 and 11 degrade both cyclic nucleotides. Importantly, the activity of dual-substrate PDEs can be influenced by the binding of cyclic nucleotides. In T-cells, several PDEs (PDE1-5, PDE7-8, PDE11) have been described.
4.1 PDE1
PDE1 with three different subfamilies PDE1A-PDE1C, is the only PDE family, which is known to be activated by calcium and calmodulin via its N-terminal calmodulin binding domain. In human T-cells, no PDE1 expression is detected on mRNA level, but it is inducible by activation via CD3/CD28 co-stimulation, and inhibition of PDE1 suppresses IL-13 production (32).
4.2 PDE2A
PDE2A is a unique subfamily with the ability to degrade both cAMP and cGMP. Three different splice variants of PDE2A with different N-terminal domains and different cellular localization are described: PDE2A1 is localized in the cytosol, PDE2A2 in mitochondria and PDE2A3 at the plasma membrane. After the discovery of PDE3 and PDE4 as the main PDEs in T-cells, the regulation of cAMP through PDE2A in T-cells was not in the focus of the research (33). But recently, PDE2A has been found in murine T-cells to be upregulated during activation of Tcon but not Treg (34).
4.3 PDE3
As mentioned above, PDE3B is inhibited in Treg by Foxp3 expression (18). A key finding to explain the elevated cAMP levels in this T-cell subset. Interestingly, inhibition of PDE3 in murine as well as in human T-cells led to differentiation of fully functional T-cells. Thus, PDE3 seems to be dispensable, but favoring for T-cell function since these Tregs harbor the potential to prevent allograft rejection (35).
4.4 PDE4
PDE4 is the best-characterized PDE. It comprises four different subfamilies PDE4A-PDE4D with multiple individual isoforms and distinct N-terminal domains. PDE4A, PDE4B and PDE4D are predominant subfamilies in T-cells (36). Notably, PDE4B is activated by T-cell receptor (TCR) signaling and controls IL-2 production (37). β-arrestin is able to form a complex with PDE4, which is recruited to lipid rafts after T-cell activation to balance cAMP levels and block PKA activity (26, 27, 38). Driver of the recruitment is CD28 stimulation (26). Three different PDE4 inhibitors, Rofumilast, Apremilast and Crisaborole, are approved to treat chronic obstructive pulmonary disorder (COPD), psoriasis and moderate atopic dermatitis, respectively (39).
4.5 PDE7
PDE7 is divided in its two different subfamilies, PDE7A and PDE7B, but only PDE7A is localized in the Golgi-apparatus of T-lymphocytes (40). PDE7A1 and PDE7A3 are upregulated during T-cell activation (41), which has been described also on mRNA level (32). Mice lacking PDE7 have normal T-cell function, indicating that PDE7 is dispensable for T-cell function. Nevertheless, PDE7 inhibitors can suppress T-cell proliferation by elevating cAMP levels (42, 43).
4.6 PDE8
The PDE8 can be subdivided into PDE8A and PDE8B subfamilies. For murine T-cells, expression of PDE8A is increased in Tcon. Activation of human T-cells is associated with an upregulation of PDE8A1 (41). In particular, the PDE8A subfamily controls T-cell motility due to the interaction of PDE8 and Raf-1 (44). The research conducted over the years focusing on the role of PDE8 on T-cell function was reviewed more in detail recently (45). Notably, the PDE8 inhibitor PF-04957325 is able to reduce the inflammatory lesion formation in the central nervous system in the experimental autoimmune encephalomyelitis (EAE) mouse model for multiple sclerosis (46). Table 1 summarizes previous findings about PDE expression and regulation during T cell activation.
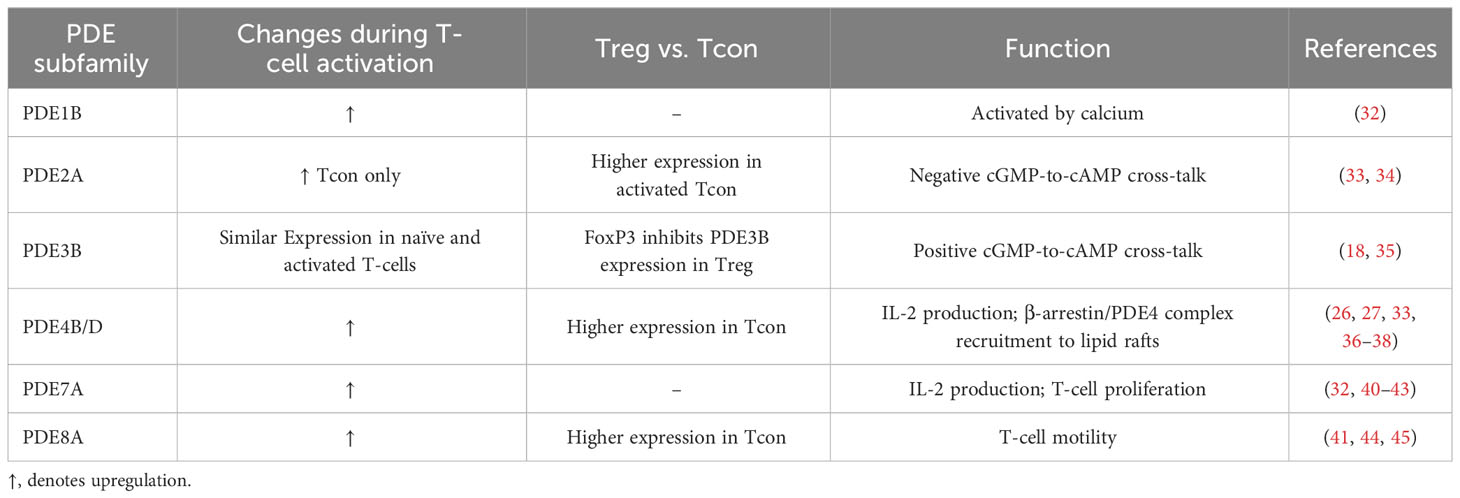
Table 1 Overview of changes in PDE expression depending on the T-cell subset, activation status and the function of the PDE family in T-cells.
5 cGMP-to-cAMP cross-talk via PDE2A/PDE3B in T-cells
cGMP formation is triggered by binding of natriuretic peptides (NPs) to guanylyl cyclases: atrial (ANP) and brain natriuretic peptides (BNP) bind to the guanylyl cyclase-A, C-type natriuretic peptides to guanylyl cyclase-B. Both NP receptors harbor an intracellular guanylyl cyclase domain and are also called particulate guanylyl cyclases (pGC). Alternatively, cGMP formation is catalyzed by nitric oxide sensitive or soluble (sGC) inside the cell. Dual-substrate phosphodiesterases, PDE1-PDE3, PDE10 and PDE11, allow the cross-talk between both cyclic nucleotides. PDE2A has a Km value of 30 µmol/L for cAMP and 10 µmol/L for cGMP hydrolysis, and is also called cGMP-stimulated PDE since the binding of cGMP to the regulatory GAF-B domain of PDE2A enhances the affinity to degrade cAMP (47, 48). With that unique characteristic PDE2A enables the negative cGMP-to-cAMP cross-talk. On the other hand, PDE3 is a cGMP-inhibited PDE because of lower Km value for cGMP. So, the competitive inhibition of cAMP hydrolysis by cGMP binding enables the positive cGMP-to-cAMP cross-talk (49). From a physiological point of view, the cGMP-to-cAMP cross-talk mediated by PDE2 is a key mediator in the heart and the adrenal cortex. For example, the secretion of aldosterone underlies the control of cAMP levels, which is balanced by ANP dependent PDE2A activation in adrenal zona glomerulosa cells (50). The impact of PDE3 in T-cells has been investigated, but so far, less research has focused on PDE2A and the real-time dynamics of cAMP-to-cGMP cross-talk in T-cells. With the use of a highly sensitive Förster Resonance Energy Transfer based sensor it was recently shown that PDE2A inhibition results in higher responses in CD3/CD28-activated than in non-activated T-cells (34). Moreover, PDE2A is selectively upregulated during activation in Tcon but not in Treg. Simultaneously, PDE3B is not upregulated upon CD3/CD28 stimulation. These findings open the question whether the regulation via PDE3B and PDE2A of the cAMP-to-cGMP-cross-talk undergoes a switch by the activation of T-cells (Figure 1).
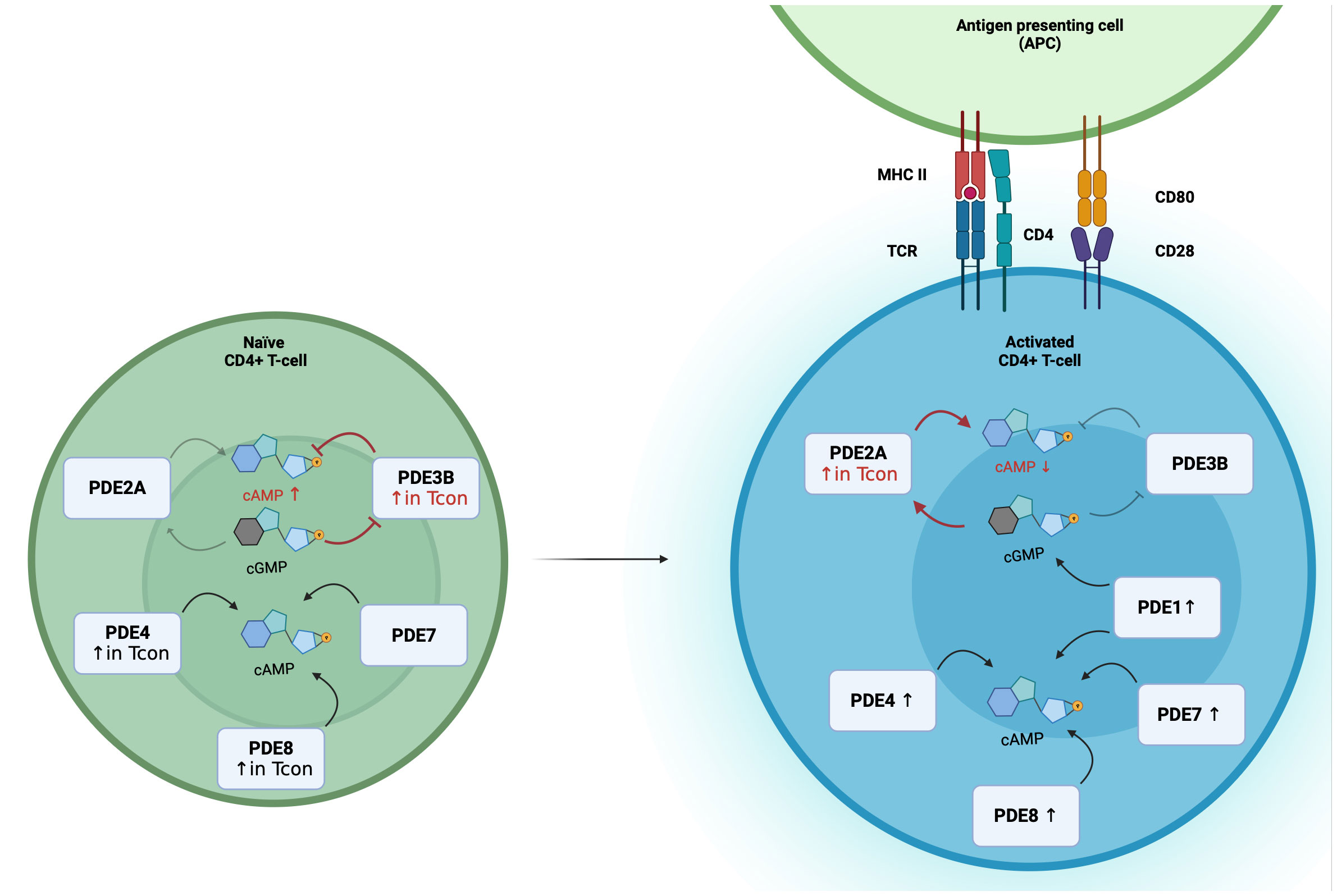
Figure 1 Differential PDE expression and the switch of the cGMP-to-cAMP cross-talk during T-cell activation. In Naïve CD4+ T-cells, PDE2A, PDE3B, PDE4, PDE7 and PDE8 are expressed, with higher expression of PDE3B, PDE4 and PDE8A in Tcon. During T-cell activation PDE1, PDE2A, PDE4, PDE7 and PDE8 expression are upregulated. In Tcon, the cGMP-to-cAMP switches during activation. In naïve Tcon, cGMP binds to PDE3B and acts as a competitive inhibitor of cAMP hydrolysis to enable the positive cGMP-to-cAMP cross-talk. During activation, elevated PDE2A levels result in the negative cGMP-to-cAMP cross-talk. Binding of cGMP to PDE2A leads to a higher cAMP degradation.
6 Conclusion & further perspectives
Intracellular cAMP levels tightly control T-cell function and activation. cAMP is transiently upregulated during T-cell activation, but high levels of cAMP suppress T-cell activation, proliferation, and cytokine release. cAMP levels differ between T-cell subsets and can be transferred via gap junctions to mediate the suppressive function of Tregs. Thus, it is indispensable for the maintenance of the immune balance that cAMP levels in the cell are balanced precisely at a subcellular level by the interplay of cAMP production via ACs and cAMP degradation by PDEs. Research conducted over the last years has focused on PDE4 inhibition to mediate T-cell responses in the context of autoimmune diseases like chronic obstructive pulmonary disorder (COPD), psoriasis and atopic dermatitis. Ongoing research identified the importance of PDE8, alongside the well-characterized PDE4, for T-cell function suggesting it might be a beneficial drug target for multiple sclerosis (46). Moreover, changes in the cGMP-to-cAMP cross-talk could be especially relevant under pathological conditions such as inflammation caused by myocardial infarction where the differentiation of Tregs is promoted (51). High levels of catecholamines and NPs stimulating both cAMP and cGMP signaling at the same time might affect the immune response including the T-cell recruitment to the infarcted tissue. It will be exciting to get a better understanding how dual-substrate PDEs, such as PDE2A and PDE3B, mediate the cGMP-to-cAMP cross-talk to maintain the immune balance under this pathophysiological condition. Furthermore, enhanced PDE2A expression with constant PDE3B expression during activation indicates that the cAMP-to-cGMP cross-talk undergoes a switch during activation. From this perspective, PDE2A could be another important regulator of cAMP in T-cells and potential drug target for immunity and inflammation. Interestingly, PDE2A2 localized in mitochondria can specifically counterbalance local pool of cAMP produced by AC10. Since in several cell types high cAMP levels are known to induce reactive oxygen species production and affect cell apoptosis, similar important role of balanced cAMP signaling can be expected in T cells which can be also affected by autoimmune disease such as multiple sclerosis (52). Therefore, in the future, it will be exciting to study local real-time cAMP dynamics in various subcellular locations in healthy and diseased T-cells.
Author contributions
MB: Writing – original draft, Writing – review & editing. RK: Writing – review & editing. SF: Writing – review & editing. VN: Writing – original draft, Writing – review & editing.
Funding
The author(s) declare financial support was received for the research, authorship, and/or publication of this article. This study was funded by the German Research foundation (SFB1328 and SFB1525, grant numbers 335447717 and 453989101). We acknowledge financial support from the Open Access Publication Fund of UKE - Universitätsklinikum Hamburg-Eppendorf.
Acknowledgments
The figures were created with BioRender.com.
Conflict of interest
The authors declare that the research was conducted in the absence of any commercial or financial relationships that could be construed as a potential conflict of interest.
Publisher’s note
All claims expressed in this article are solely those of the authors and do not necessarily represent those of their affiliated organizations, or those of the publisher, the editors and the reviewers. Any product that may be evaluated in this article, or claim that may be made by its manufacturer, is not guaranteed or endorsed by the publisher.
References
1. Sutherland EW, Rall TW. Fractionation and characterization of a cyclic adenine ribonucleotide formed by tissue particles. J Biol Chem. (1958) 232:1077–91. doi: 10.1016/S0021-9258(19)77423-7
2. Vang T, Torgersen KM, Sundvold V, Saxena M, Levy FO, Skålhegg BS, et al. Activation of the COOH-terminal Src kinase (Csk) by cAMP-dependent protein kinase inhibits signaling through the T cell receptor. J Exp Med. (2001) 193:497–507. doi: 10.1084/jem.193.4.497
3. Aandahl EM, Moretto WJ, Haslett PA, Vang T, Bryn T, Tasken K, et al. Inhibition of antigen-specific T cell proliferation and cytokine production by protein kinase A type I. J Immunol. (2002) 169:802–8. doi: 10.4049/jimmunol.169.2.802
4. Kamenetsky M, Middelhaufe S, Bank EM, Levin LR, Buck J, Steegborn C. Molecular details of cAMP generation in mammalian cells: a tale of two systems. J Mol Biol. (2006) 362:623–39. doi: 10.1016/j.jmb.2006.07.045
5. Sunahara RK, Taussig R. Isoforms of mammalian adenylyl cyclase: multiplicities of signaling. Mol Interv. (2002) 2:168–84. doi: 10.1124/mi.2.3.168
6. Huang B, Zhao J, Lei Z, Shen S, Li D, Shen GX, et al. miR-142-3p restricts cAMP production in CD4+CD25- T cells and CD4+CD25+ Treg cells by targeting AC9 mRNA. EMBO Rep. (2009) 10:180–5. doi: 10.1038/embor.2008.224
7. Duan B, Davis R, Sadat EL, Collins J, Sternweis PC, Yuan D, et al. Distinct roles of adenylyl cyclase VII in regulating the immune responses in mice. J Immunol. (2010) 185:335–44. doi: 10.4049/jimmunol.0903474
8. Scott JD. Cyclic nucleotide-dependent protein kinases. Pharmacol Ther. (1991) 50:123–45. doi: 10.1016/0163-7258(91)90075-W
9. Taylor SS, Knighton DR, Zheng J, Ten Eyck LF, Sowadski JM. Structural framework for the protein kinase family. Annu Rev Cell Biol. (1992) 8:429–62. doi: 10.1146/annurev.cb.08.110192.002241
10. Shabb JB. Physiological substrates of cAMP-dependent protein kinase. Chem Rev. (2001) 101:2381–411. doi: 10.1021/cr000236l
11. Conti M, Jin SL. The molecular biology of cyclic nucleotide phosphodiesterases. Prog Nucleic Acid Res Mol Biol. (1999) 63:1–38. doi: 10.1016/S0079-6603(08)60718-7
12. Conti M, Beavo J. Biochemistry and physiology of cyclic nucleotide phosphodiesterases: essential components in cyclic nucleotide signaling. Annu Rev Biochem. (2007) 76:481–511. doi: 10.1146/annurev.biochem.76.060305.150444
13. Arumugham VB, Baldari CT. cAMP: a multifaceted modulator of immune synapse assembly and T cell activation. J Leukoc Biol. (2017) 101:1301–16. doi: 10.1189/jlb.2RU1116-474R
14. Fontenot JD, Rasmussen JP, Williams LM, Dooley JL, Farr AG, Rudensky AY. Regulatory T cell lineage specification by the forkhead transcription factor Foxp3. Immunity. (2005) 22:329–41. doi: 10.1016/j.immuni.2005.01.016
15. Josefowicz SZ, Rudensky A. Control of regulatory T cell lineage commitment and maintenance. Immunity. (2009) 30:616–25. doi: 10.1016/j.immuni.2009.04.009
16. Brunkow ME, Jeffery EW, Hjerrild KA, Paeper B, Clark LB, Yasayko SA, et al. Disruption of a new forkhead/winged-helix protein, scurfin, results in the fatal lymphoproliferative disorder of the scurfy mouse. Nat Genet. (2001) 27:68–73. doi: 10.1038/83784
17. Bopp T, Becker C, Klein M, Klein-Hessling S, Palmetshofer A, Serfling E, et al. Cyclic adenosine monophosphate is a key component of regulatory T cell-mediated suppression. J Exp Med. (2007) 204:1303–10. doi: 10.1084/jem.20062129
18. Gavin MA, Rasmussen JP, Fontenot JD, Vasta V, Manganiello VC, Beavo JA, et al. Foxp3-dependent program of regulatory T-cell differentiation. Nature. (2007) 445:771–5. doi: 10.1038/nature05543
19. Ma N, Wei T, Wang B, Jiang X, Zhou L, Zhong R. MicroRNA-142-3p inhibits IFN-gamma Production via targeting of rictor in aspergillus fumigatus activated CD4(+) T cells. Ann Transl Med. (2019) 7:649. doi: 10.21037/atm
20. Bazhin AV, Kahnert S, Kimpfler S, SChadendorf D, Umansky V. Distinct metabolism of cyclic adenosine monophosphate in regulatory and helper CD4+ T cells. Mol Immunol. (2010) 47:678–84. doi: 10.1016/j.molimm.2009.10.032
21. Ernst PB, Garrison JC, Thompson LF. Much ado about adenosine: adenosine synthesis and function in regulatory T cell biology. J Immunol. (2010) 185:1993–8. doi: 10.4049/jimmunol.1000108
22. Vaeth M, Gogishvili T, Bopp T, Klein M, Berberich-Siebelt F, Gattenloehner S, et al. Regulatory T cells facilitate the nuclear accumulation of inducible cAMP early repressor (ICER) and suppress nuclear factor of activated T cell C1 (NFATc1). Proc Natl Acad Sci USA. (2011) 108:2480–5. doi: 10.1073/pnas.1009463108
23. Fraser JD, Irving BA, Crabtree GR, Weiss A. Regulation of interleukin-2 gene enhancer activity by the T cell accessory molecule CD28. Science. (1991) 251:313–6. doi: 10.1126/science.1846244
24. Harding FA, Mcarthur JG, Gross JA, Raulet DH, Allison JP. CD28-mediated signaling co-stimulates murine T cells and prevents induction of anergy in T-cell clones. Nature. (1992) 356:607–9. doi: 10.1038/356607a0
25. Wang T, Sheppard JR, Foker JE. Rise and fall of cyclic AMP required for onset of lymphocyte DNA synthesis. Science. (1978) 201:155–7. doi: 10.1126/science.208147
26. Abrahamsen H, Baillie G, Ngai J, Vang T, Nika K, Ruppelt A, et al. TCR- and CD28-mediated recruitment of phosphodiesterase 4 to lipid rafts potentiates tcr signaling. J Immunol. (2004) 173:4847–58. doi: 10.4049/jimmunol.173.8.4847
27. Wehbi VL, Taskén K. Molecular mechanisms for cAMP-mediated immunoregulation in T cells - role of anchored protein kinase A signaling units. Front Immunol. (2016) 7:222. doi: 10.3389/fimmu.2016.00222
28. Ruppelt A, Mosenden R, Grönholm M, Aandahl EM, Tobin D, Carlson CR, et al. Inhibition of T cell activation by cyclic adenosine 5'-monophosphate requires lipid raft targeting of protein kinase A type I by the A-kinase anchoring protein ezrin. J Immunol. (2007) 179:5159–68. doi: 10.4049/jimmunol.179.8.5159
29. Tasken K, Ruppelt A. Negative regulation of T-cell receptor activation by the cAMP-PKA-Csk signaling pathway in T-cell lipid rafts. Front Biosci. (2006) 11:2929–39. doi: 10.2741/2022
30. Bjørgo E, Solheim SA, Abrahamsen H, Baillie GS, Brown KM, Berge T, et al. Cross talk between phosphatidylinositol 3-kinase and cyclic AMP (cAMP)-protein kinase A signaling pathways at the level of a protein kinase B/beta-arrestin/cAMP phosphodiesterase 4 complex. Mol Cell Biol. (2010) 30:1660–72. doi: 10.1128/MCB.00696-09
31. Lorton D, Bellinger DL. Molecular mechanisms underlying ß-adrenergic receptor-mediated cross-talk between sympathetic neurons and immune cells. Int J Mol Sci. (2015) 16:5635–65. doi: 10.3390/ijms16035635
32. Kanda N, Watanabe S. Regulatory roles of adenylate cyclase and cyclic nucleotide phosphodiesterases 1 and 4 in interleukin-13 production by activated human T cells. Biochem Pharmacol. (2001) 62:495–507. doi: 10.1016/S0006-2952(01)00688-8
33. Michie AM, Lobban M, Müller T, Harnett MM, Houslay MD. Rapid regulation of PDE-2 and PDE-4 cyclic AMP phosphodiesterase activity following ligation of the T cell antigen receptor on thymocytes: analysis using the selective inhibitors erythro-9-(2-hydroxy-3-nonyl)-adenine (EHNA) and rolipram. Cell Signal. (1996) 8:97–110. doi: 10.1016/0898-6568(95)02032-2
34. Kurelic R, Krieg PF, Sonner JK, Bhaiyan G, Ramos GC, Frantz S, et al. Upregulation of phosphodiesterase 2A augments T cell activation by changing cGMP/cAMP cross-talk. Front Pharmacol. (2021) 12:748798. doi: 10.3389/fphar.2021.748798
35. Feng G, Nadig SN, Bäckdahl L, Beck S, Francis RS, Schiopu A, et al. Functional regulatory T cells produced by inhibiting cyclic nucleotide phosphodiesterase type 3 prevent allograft rejection. Sci Transl Med. (2011) 3:83ra40. doi: 10.1126/scitranslmed.3002099
36. Landells LJ, Szilagy CM, Jones NA, Banner KH, Allen JM, Doherty A, et al. Identification and quantification of phosphodiesterase 4 subtypes in CD4 and CD8 lymphocytes from healthy and asthmatic subjects. Br J Pharmacol. (2001) 133:722–9. doi: 10.1038/sj.bjp.0704120
37. Arp J, Kirchhof MG, Baroja ML, Nazarian SH, Chau TA, Strathdee CA, et al. Regulation of T-cell activation by phosphodiesterase 4B2 requires its dynamic redistribution during immunological synapse formation. Mol Cell Biol. (2003) 23:8042–57. doi: 10.1128/MCB.23.22.8042-8057.2003
38. Bjørgo E, Taskén K. Role of cAMP phosphodiesterase 4 in regulation of T-cell function. Crit Rev Immunol. (2006) 26:443–51. doi: 10.1615/CritRevImmunol.v26.i5
39. Baillie GS, Tejeda GS, Kelly MP. Therapeutic targeting of 3',5'-cyclic nucleotide phosphodiesterases: inhibition and beyond. Nat Rev Drug Discov. (2019) 18:770–96. doi: 10.1038/s41573-019-0033-4
40. Szczypka M. Role of phosphodiesterase 7 (PDE7) in T cell activity. Effects of selective PDE7 inhibitors and dual PDE4/7 inhibitors on T cell functions. Int J Mol Sci. (2020) 21:6118. doi: 10.3390/ijms21176118
41. Glavas NA, Ostenson C, Schaefer JB, Vasta V, Beavo JA. T cell activation up-regulates cyclic nucleotide phosphodiesterases 8A1 and 7A3. Proc Natl Acad Sci USA. (2001) 98:6319–24. doi: 10.1073/pnas.101131098
42. Li L, Yee C, Beavo JA. CD3- and CD28-dependent induction of PDE7 required for T cell activation. Science. (1999) 283:848–51. doi: 10.1126/science.283.5403.848
43. Kadoshima-Yamaoka K, Murakawa M, Goto M, Tanaka Y, Inoue H, Murafuji H, et al. Effect of phosphodiesterase 7 inhibitor ASB16165 on development and function of cytotoxic T lymphocyte. Int Immunopharmacol. (2009) 9:97–102. doi: 10.1016/j.intimp.2008.10.005
44. Basole CP, Nguyen RK, Lamothe K, Vang A, Clark R, Baillie GS, et al. PDE8 controls CD4(+) T cell motility through the PDE8A-Raf-1 kinase signaling complex. Cell Signal. (2017) 40:62–72. doi: 10.1016/j.cellsig.2017.08.007
45. Epstein PM, Basole C, Brocke S. The role of PDE8 in T cell recruitment and function in inflammation. Front Cell Dev Biol. (2021) 9:636778. doi: 10.3389/fcell.2021.636778
46. Basole CP, Nguyen RK, Lamothe K, Billis P, Fujiwara M, Vang AG, et al. Treatment of experimental autoimmune encephalomyelitis with an inhibitor of phosphodiesterase-8 (PDE8). Cells. (2022) 11:660. doi: 10.3390/cells11040660
47. Martins TJ, Mumby MC, Beavo JA. Purification and characterization of a cyclic GMP-stimulated cyclic nucleotide phosphodiesterase from bovine tissues. J Biol Chem. (1982) 257:1973–9. doi: 10.1016/S0021-9258(19)68134-2
48. Martinez SE, Wu AY, Glavas NA, Tang XB, Turley S, Hol WG, et al. The two GAF domains in phosphodiesterase 2A have distinct roles in dimerization and in cGMP binding. Proc Natl Acad Sci USA. (2002) 99:13260–5. doi: 10.1073/pnas.192374899
49. Zaccolo M, Movsesian MA. cAMP and cGMP signaling cross-talk: role of phosphodiesterases and implications for cardiac pathophysiology. Circ Res. (2007) 100:1569–78. doi: 10.1161/CIRCRESAHA.106.144501
50. Macfarland RT, Zelus BD, Beavo JA. High concentrations of a cGMP-stimulated phosphodiesterase mediate ANP-induced decreases in cAMP and steroidogenesis in adrenal glomerulosa cells. J Biol Chem. (1991) 266:136–42. doi: 10.1016/S0021-9258(18)52413-3
51. Delgobo M, Weis E, Ashour D, Richter L, Popiolkowski L, ArAMPatzi P, et al. Myocardial milieu favors local differentiation of regulatory T cells. Circ Res. (2023) 132:565–82. doi: 10.1161/CIRCRESAHA.122.322183
Keywords: cAMP, phosphodiesterases, T-cell activation, T-cell subsets, cGMP-to-cAMP crosstalk, PDE2A
Citation: Bielenberg M, Kurelic R, Frantz S and Nikolaev VO (2024) A mini-review: phosphodiesterases in charge to balance intracellular cAMP during T-cell activation. Front. Immunol. 15:1365484. doi: 10.3389/fimmu.2024.1365484
Received: 04 January 2024; Accepted: 23 February 2024;
Published: 08 March 2024.
Edited by:
Christa Elisabeth Müller, University of Bonn, GermanyReviewed by:
Stefan Brocke, University of Connecticut Health Center, United StatesSubhashis Pal, Emory University, United States
Copyright © 2024 Bielenberg, Kurelic, Frantz and Nikolaev. This is an open-access article distributed under the terms of the Creative Commons Attribution License (CC BY). The use, distribution or reproduction in other forums is permitted, provided the original author(s) and the copyright owner(s) are credited and that the original publication in this journal is cited, in accordance with accepted academic practice. No use, distribution or reproduction is permitted which does not comply with these terms.
*Correspondence: Viacheslav O. Nikolaev, di5uaWtvbGFldkB1a2UuZGU=