- 1Biotherapy Center and Cancer Center, the First Affiliated Hospital of Zhengzhou University, Zhengzhou, China
- 2The First Clinical Medical College, Henan University of Chinese Medicine, Zhengzhou, China
- 3School of Life Sciences, Zhengzhou University, Zhengzhou, China
- 4School of Public Health, Zhengzhou University, Zhengzhou, China
- 5Engineering Key Laboratory for Cell Therapy of Henan Province, Zhengzhou, China
Objective: The coronavirus disease 2019 (COVID-19) spread rapidly and claimed millions of lives worldwide. Acute respiratory distress syndrome (ARDS) is the major cause of COVID-19-associated deaths. Due to the limitations of current drugs, developing effective therapeutic options that can be used rapidly and safely in clinics for treating severe acute respiratory syndrome coronavirus-2 (SARS-CoV-2) infections is necessary. This study aims to investigate the effects of two food-extracted immunomodulatory agents, ajoene-enriched garlic extract (AGE) and cruciferous vegetables-extracted sulforaphane (SFN), on anti-inflammatory and immune responses in a SARS-CoV-2 acute lung injury mouse model.
Methods: In this study, we established a mouse model to mimic the SARS-CoV-2 infection acute lung injury model via intratracheal injection of polyinosinic:polycytidylic acid (poly[I:C]) and SARS-CoV-2 recombinant spike protein (SP). After the different agents treatment, lung sections, bronchoalveolar lavage fluid (BALF) and fresh faeces were harvested. Then, H&E staining was used to examine symptoms of interstitial pneumonia. Flow cytometry was used to examine the change of immune cell populations. Multiplex cytokines assay was used to examine the inflammatory cytokines.16S rDNA high-throughput sequencing was used to examine the change of gut microbiome.
Results: Our results showed that AGE and SFN significantly suppressed the symptoms of interstitial pneumonia, effectively inhibited the production of inflammatory cytokines, decreased the percentage of inflammatory cell populations, and elevated T cell populations in the mouse model. Furthermore, we also observed that the gut microbiome of genus Paramuribaculum were enriched in the AGE-treated group.
Conclusion: Here, for the first time, we observed that these two novel, safe, and relatively inexpensive immunomodulatory agents exhibited the same effects on anti-inflammatory and immune responses as neutralizing monoclonal antibodies (mAbs) against interleukin 6 receptor (IL-6R), which have been suggested for treating COVID-19 patients. Our results revealed the therapeutic ability of these two immunomodulatory agents in a mouse model of SARS-CoV-2 acute lung injury by promoting anti-inflammatory and immune responses. These results suggest that AGE and SFN are promising candidates for the COVID-19 treatment.
Introduction
Coronavirus disease 2019 (COVID-19) was a public health emergency of international concern, causing over 632 million confirmed cases and 7 million deaths worldwide. Furthermore, COVID-19 has had a serious socioeconomic impact globally in the last three years (1). Severe acute respiratory syndrome coronavirus-2 (SARS-CoV-2) is the causative agent of COVID-19 and directly binds to the SARS-CoV-2 entry receptor, angiotensin-converting enzyme-2 (ACE2) (2, 3). Infected patients have clinical features ranging from asymptomatic to severe disease. Most critically ill patients deteriorated suddenly in the later stages of the disease and died in a short period of time due to acute respiratory distress syndrome (ARDS), which arises from cytokine release syndrome (CRS) and a complex immune-inflammatory response (4). Global efforts were made to rapidly develop specific anti-SARS-CoV2 antiviral drugs. However, the long process of validating the clinical efficacy and safety limited the drug’s development. Therefore, the current agents proved efficient and safe and required further evaluation in COVID-19 treatment. Emerging evidence has shown that excessive inflammation is a key driver of poor COVID-19 outcomes. The coagulation markers, proinflammatory cytokines, such as interleukin (IL)-6, IL-10, and tumor necrosis factor alpha (TNF‐α), and lymphopenia are associated with the severity of COVID-19 (5–7). Therapeutic immunomodulation is effective in the management of inflammatory diseases. Currently, the immunomodulatory agents include therapies targeting the IL-6/interleukin 6 receptor (IL-6R), TNF-α, and IL-1β pathways (8, 9). These agents have been suggested for treating the advanced stage of COVID-19 by reducing inflammatory responses (10, 11). The clinical outcomes of patients with COVID-19 were improved by these immunomodulatory therapies (4). However, multiple clinical trials of these agents have shown limited benefits (12, 13). Thus, effective, convenient, and inexpensive therapeutic strategies are required to treat SARS-CoV-2 infections.
Natural food products are excellent candidates for use in drug discovery. Medicinal plant extracts have been used to treat various diseases, including viral infection (14–16). Garlic has been consumed by humans for a thousand years as a functional food and a traditional remedy for preventing and treating various diseases since ancient times (17–19). Garlic and its active organosulfur compounds alleviate several viral infections in preclinical and clinical investigations (16). The World Health Organization considers it an essential component of any balanced diet with immunomodulatory properties (20). Ajoene [(E)-(Z)-4,5,9-trithiadodeca-1,6,11-triene-9-oxiade], a major bioactive compound extracted from garlic (21), is synthetically produced by the decomposition of allicin (22, 23). Ajoene has several biological functions, including anti-thrombotic, antioxidant, anti-microbial, anti-viral, anti-cancer, and anti-inflammatory activities, regulating the immune response (24–26). Ajoene exhibits a broad spectrum of in vitro and in vivo activities against several DNA and RNA viruses (18, 27, 28), including inhibitory activity against human immunodeficiency virus 1 (HIV-1) by blocking HIV-1 virus-cell attachment (29). Sulforaphane (SFN), which is extracted from cruciferous vegetables, including cabbage, broccoli, and Brussels sprouts, induces the activation of nuclear factor-erythroid 2-related factor 2 (NRF2), with acknowledged anti-inflammatory and anti-oxidant properties (30). However, whether ajoene-enriched garlic extract (AGE) and SFN play therapeutic roles in SARS-CoV-2 infection remains unclear.
In this study, we established an effective and biologically safe mouse model that mimicked the pathological processes of ARDS and CRS during SARS-CoV-2 infection (31). We utilized a SARS-CoV-2 acute lung injury mouse model to investigate the role of two immunomodulatory agents, AGE and SFN, in anti-inflammatory and immune response modulation. We examined whether interventions with these two agents could mitigate the inflammatory response and restore the immune system in SARS-CoV-2 in vivo. Here, for the first time, we observed that AGE and SFN significantly decreased the level of lung injury, effectively inhibited the production of inflammatory cytokines, decreased the percentage of inflammatory cell populations, and elevated the number of lymphocytes, such as CD4+T and CD8+T cells, in a SARS-CoV-2 ARDS mouse model. AGE and SFN treatment had the same effect as an antagonist of IL-6R, the neutralizing antibody suggested for treating advanced COVID-19. These results provide a theoretical basis for applying AGE and SFN in the treatment of patients with COVID-19.
Results
Poly(I:C) and SP-induced acute lung injury and CRS in humanized angiotensin-converting enzyme-2 mice
To mimic the SARS-CoV-2 infection acute lung injury model, we established a mouse model via intratracheal injection of polyinosinic:polycytidylic acid (poly[I:C]) and SARS-CoV-2 recombinant spike protein (SP) (Figure 1A). First, to examine the pathologic changes, we analyzed the results of hematoxylin and eosin (HE)-stained lung sections from the poly[I:C] and SP injected and uninjected mice. The results showed an inflammatory process similar to that previously described for this model after SARS-CoV-2 infection (32, 33). Poly(I:C) and SP injected mice showed increased pulmonary pathology with more alveolar and peribronchiolar inflammation than that in uninjected mice at different time points (Figure 1B). Lung inflammation emerged at 6 h and was most severe at 24 h. The lung inflammation gradually declined at 48 h and 5 days postinoculation (dpi). Then, as a measure of lung injury, bronchoalveolar lavage fluid (BALF) derived from injected mice showed a significantly increased level of inflammation cytokines, such as IL-6, interferon (IFN)-β, IL-23, TNF-α, IFN-γ, monocyte chemoattractant protein-1 (MCP1), IL-1β, IL-10, and granulocyte-macrophage colony-stimulating factor (GM-CSF) compared with those in uninjected controls (Figure 1C), indicating the formation of CRS. In addition, we observed that the percentage of CD11b+GR1+neutrophils was markedly elevated in the BALF after injection (Figures 1D, E). The accumulation of neutrophils is known to initiate and maintain lung inflammatory responses, including ARDS, and has been demonstrated in SARS-CoV-2 infections (34). We observed that CD3+ T, CD4+ T, and CD8+ T cells were reduced in the BALF and peripheral blood of injected mice, consistent with lymphopenia in patients with COVID-19 (6, 35) (Supplementary Figures 1, 2). These results confirmed the successful construction of an inflammatory mouse model mimicking the pathological characteristics of ARDS and CRS in COVID-19 patients.
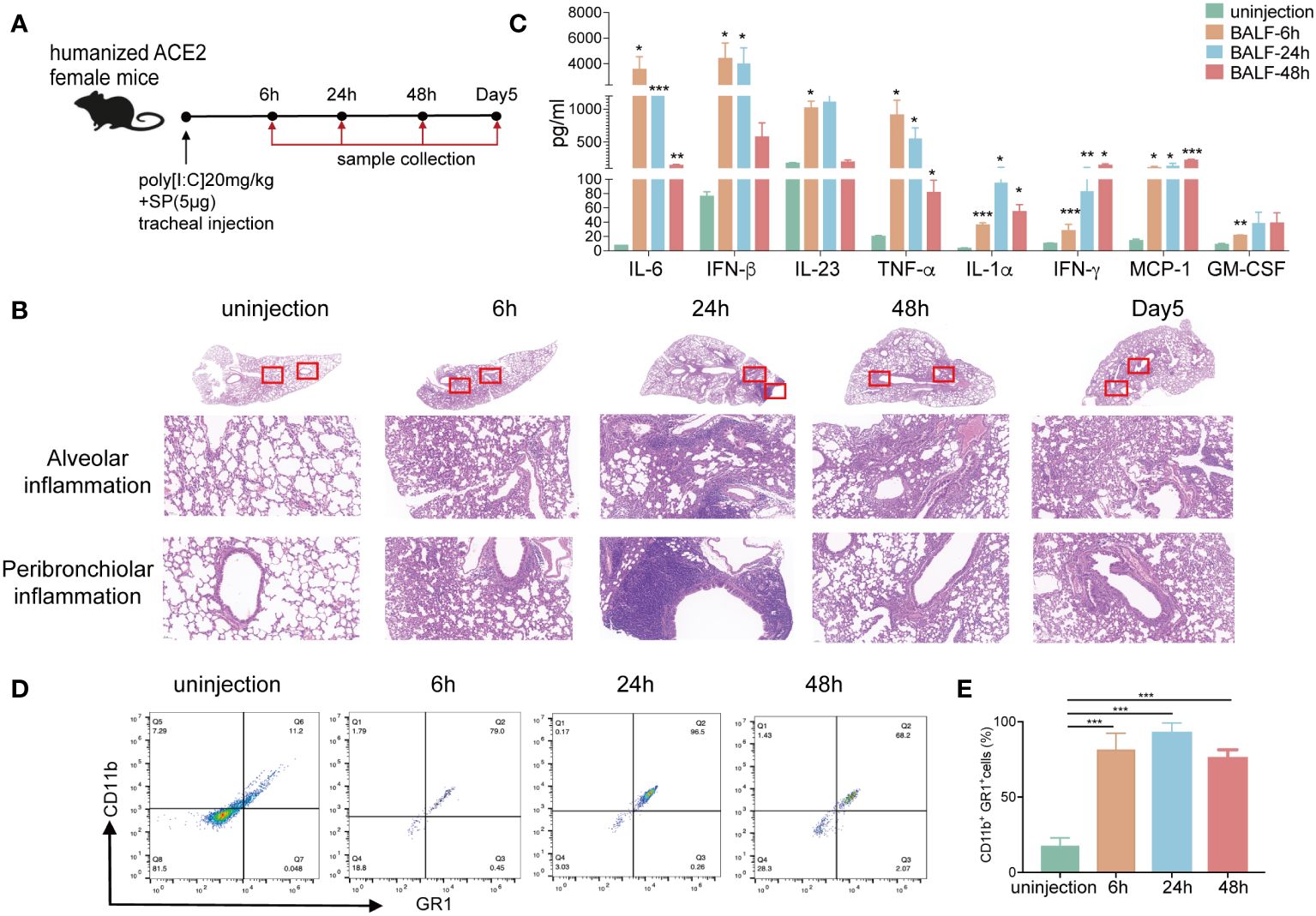
Figure 1 Poly(I:C) and SP-induced acute lung injury and CRS in hACE2 mice. (A) Schematic diagram showing the construction of an acute lung injury mouse model via intratracheal injection with 20 mg/kg poly(I:C) and 5 μg SARS-CoV-2 recombinant spike protein (SP). (B) Hematoxylin and eosin (HE) staining of histological lung sections of representative normal and injected mice at different time points. Regions of the lung anatomy where alveolar and peribronchiolar inflammation were assessed are highlighted in boxes. Images show low- (up panels) and high-power magnification (down panels) of the same tissue section. (C) The inflammation cytokines derived from BALF of the ARDS mouse model at different time points were measured using multiplex cytokines assays. (D–E) The percentage of CD11b+/GR1+ cells derived from the BALF of normal and injected mice was measured using flow cytometry. (n=5). *p <0.05, **p <0.01, and ***p <0.001. ARDS, acute respiratory distress syndrome; BALF, bronchoalveolar lavage fluid; hACE2, humanized angiotensin-converting enzyme-2; poly(I:C), polyinosinic:polycytidylic acid; SARS-CoV-2, severe acute respiratory syndrome coronavirus-2.
Anti-inflammatory effects of AGE and SFN in a SARS-CoV-2 acute lung injury mouse model
To evaluate the anti-inflammatory ability of AGE and SFN treatment in vivo, the SARS-CoV-2 acute lung injury model was used to administer AGE and SFN treatment, and an IL-6R antagonist was used as a positive control (Figure 2A). Lung tissues were harvested at different time points for HE staining. Analysis of HE-stained lung sections from these animals showed that the degree of alveolar and peribronchiolar inflammation was attenuated gradually over time after AGE and SFN treatment compared to that in the injected untreated and placebo groups, which showed the same effects as the IL-6R antagonist (Figure 2B). Histopathological analysis showed a significant reduction in lung inflammation in AGE- and SFN-treated mice compared to that in untreated controls (Figure 2C). Moreover, we examined the levels of inflammatory cytokines in the BALF following treatment with AGE and SFN. The results showed that AGE and SFN treatment had a favorable therapeutic effect in downregulating inflammatory cytokines in BALF, such as IL-1α, TNF-a, IL-6, IL-17A, IFN-β, and GM-CSF (Figures 3A–F). Therefore, these results demonstrated that AGE and SFN treatment effectively inhibited acute lung injury and CRS in SARS-CoV-2 injected mouse models by suppressing the degree of pulmonary pathology and inflammatory cytokine production.
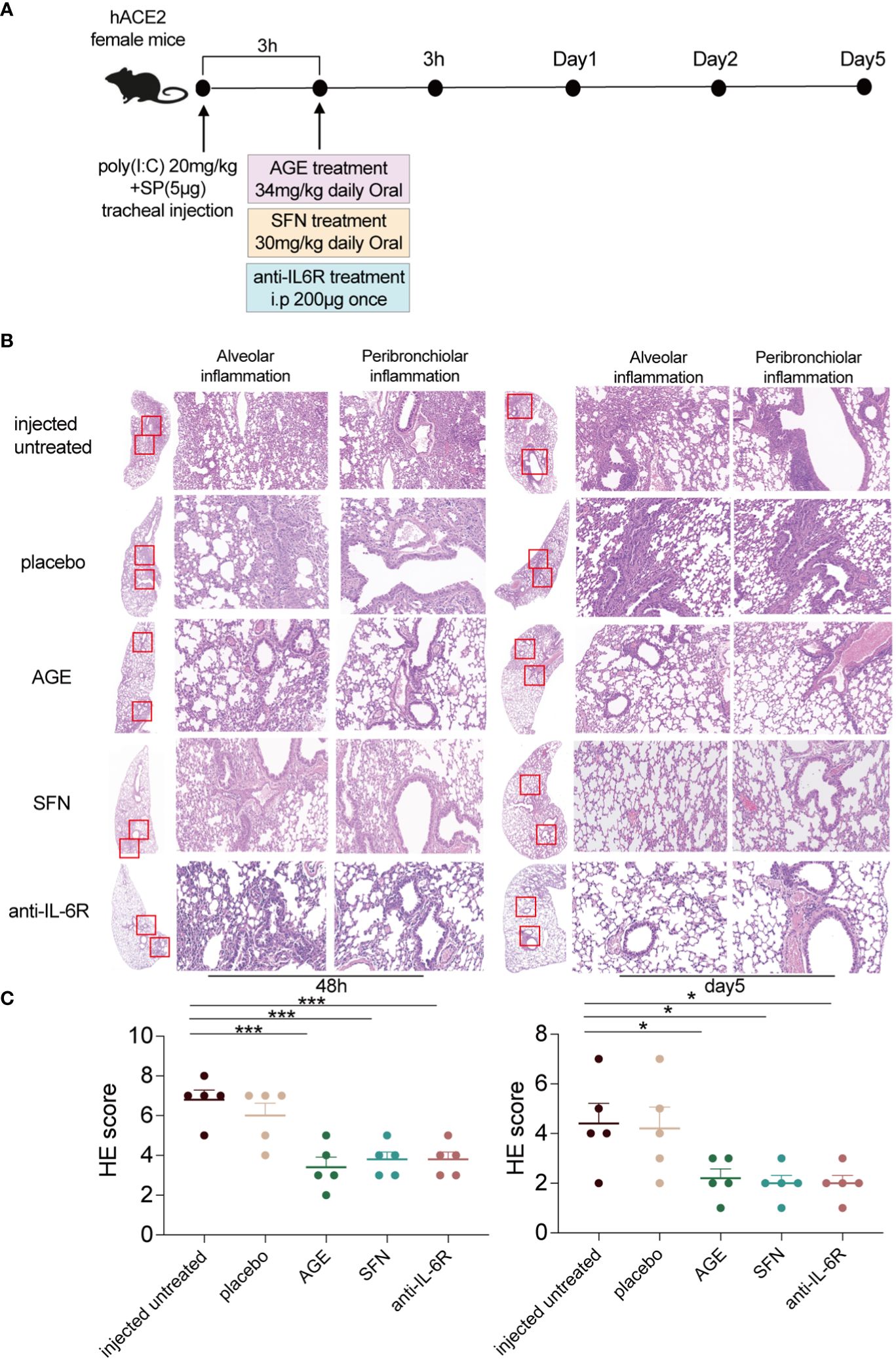
Figure 2 Anti-inflammatory effects of AGE and SFN in the SARS-CoV-2 acute lung injury mouse model. (A) Schematic diagram showing the treatment of AGE, SFN, and IL-6R antagonists in a SARS-CoV-2 acute lung injury mouse model. (B) HE staining of histological lung sections of the representative injected untreated mice and mice treated with the IL-6R neutralizing antibodies, AGE, and SFN at 48 h and 5 dpi. (C) Histopathological severity scoring was evaluated according to the pathological changes. Data from one independent experiment: injected untreated, injected treated. *p <0.05 and ***p <0.001. AGE, ajoene-enriched garlic extract; dpi, days postinoculation; IL-6R, interleukin 6 receptor; HE, hematoxylin and eosin; SARS-CoV-2, severe acute respiratory syndrome coronavirus-2; SFN, sulforaphane.
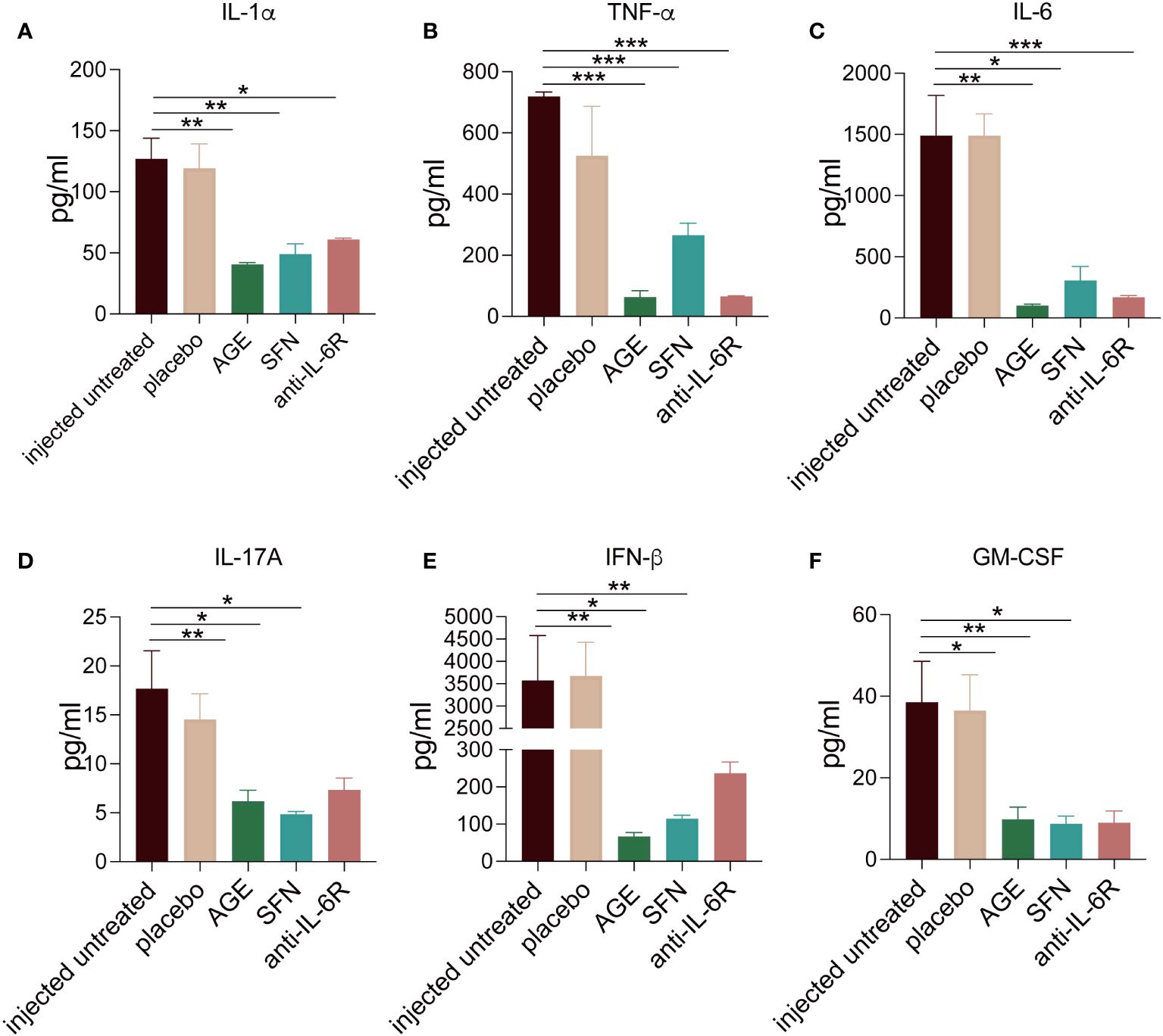
Figure 3 (A–F) The inflammatory cytokines changes in the SARS-CoV-2 acute lung injury mouse model BALF after AGE and SFN treatment. After the treatment with AGE, SFN, and IL-6R neutralizing antibodies, the inflammation cytokines from BALF were measured using a multiplex cytokines assay. *p <0.05, **p <0.01, and ***p <0.001. AGE, ajoene-enriched garlic extract; BALF, bronchoalveolar lavage fluid; IL-6R, interleukin 6 receptor; SARS-CoV-2, severe acute respiratory syndrome coronavirus-2; SFN, sulforaphane.
Effects of AGE and SFN treatment on immune response
Several studies have shown that ajoene has many biological functions, including anti-thrombotic, antioxidant, anti-microbial, anti-viral, anti-cancer, and anti-inflammatory activities, and regulates the immune response (24–26). SFN exhibits anti-tumor, anti-inflammatory, and anti-oxidative activities (36–38). Although extensive studies have been conducted, whether and how AGE and SFN modulate the immune response to SARS-CoV-2 infection remains unknown. Therefore, we hypothesized that AGE and SFN may regulate the anti-tumor immune response. To explore the immunomodulatory effects of AGE and SFN, we used flow cytometry to evaluate the changes in the immune response of the SARS-CoV-2 acute lung injury mouse model with different treatments and untreated controls. The results showed that CD11b+GR1+ neutrophils significantly decreased in BALF after treatment with both AGE and SFN at 24 h and 48 h with the same effects as the IL-6R antagonist (Figures 4A, D). We evaluated changes in the composition of other immune cells in BALF. In contrast, AGE and SFN treatment significantly increased the percentage of CD4+T and CD8+T cells among the total CD45+ immune cells in BALF (Figures 4B, C, E, F). In summary, these results demonstrated that the immunomodulatory effects of AGE and SFN have a local effect on the immune response in the lungs of a mouse model of SARS-CoV-2 acute lung injury.
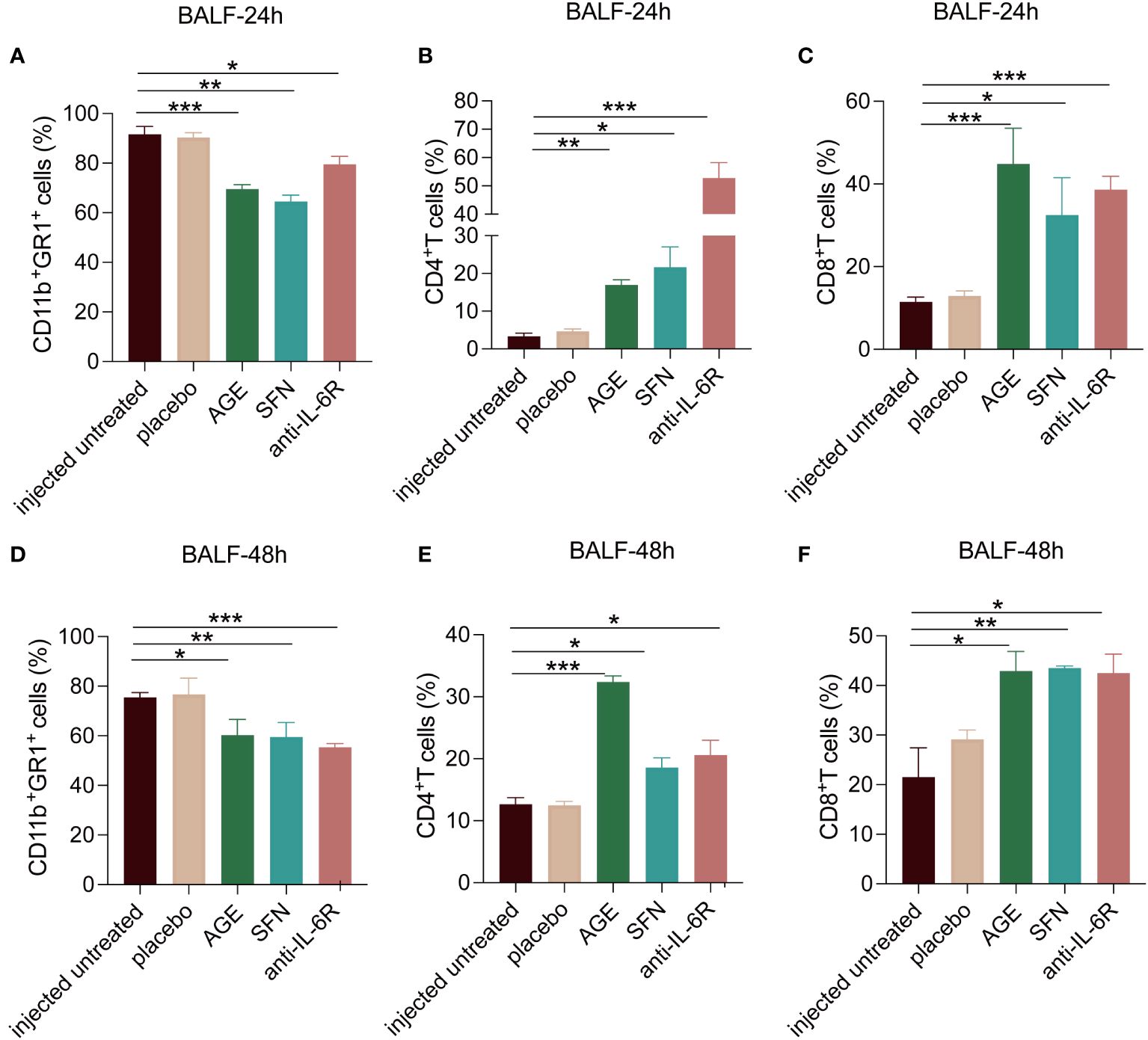
Figure 4 Effects of AGE and SFN treatment on immune response. The percentage of CD11b+GR1+, CD4+T, and CD8+T cells in BALF at 24 h (A–C) and 48 h (D–F) after AGE and SFN treatment was examined using flow cytometry. *p <0.05, **p <0.01, and ***p <0.001. AGE, ajoene-enriched garlic extract; BALF, bronchoalveolar lavage fluid; SFN, sulforaphane.
AGE treatment induces intestinal microbiome changes
There is a growing evidence showing that the gut microbiota, which plays a pivotal role in shaping the inflammation and immune systems, may significantly contribute to the COVID‐19. However, it remains unclear whether AGE treatment has an effect on the gut microbiota in the mouse model of SARS-CoV-2 acute lung injury. Therefore, we also explore the effects of AGE on the gut microbiome of acute lung injury mouse models by amplicon sequencing of 16S rRNA genes. We observed a significant difference between the AGE-treated and control groups in α-diversity, as indicated by the Chao1 indices observed and the Shannon and Simpson indices (Figure 5A). In contrast, β-diversity analysis showed no significant differences in the composition and abundance of the gut microbiota (Figure 5B). Linear discriminant analysis (LDA) effect size (LEfSe) revealed that the family Muribaculaceae, genus Paramuribaculum, and species Paramuribaculum_intestinale were enriched in the AGE-treated group (Figures 5C, D). Subsequent heat map and Sankey diagram analyses confirmed the enrichment of gut bacteria in the genus Paramuribaculum (Figures 5E–G). These results suggested that Paramuribaculum may be crucial for the anti-inflammatory and immunomodulatory effects of AGE.
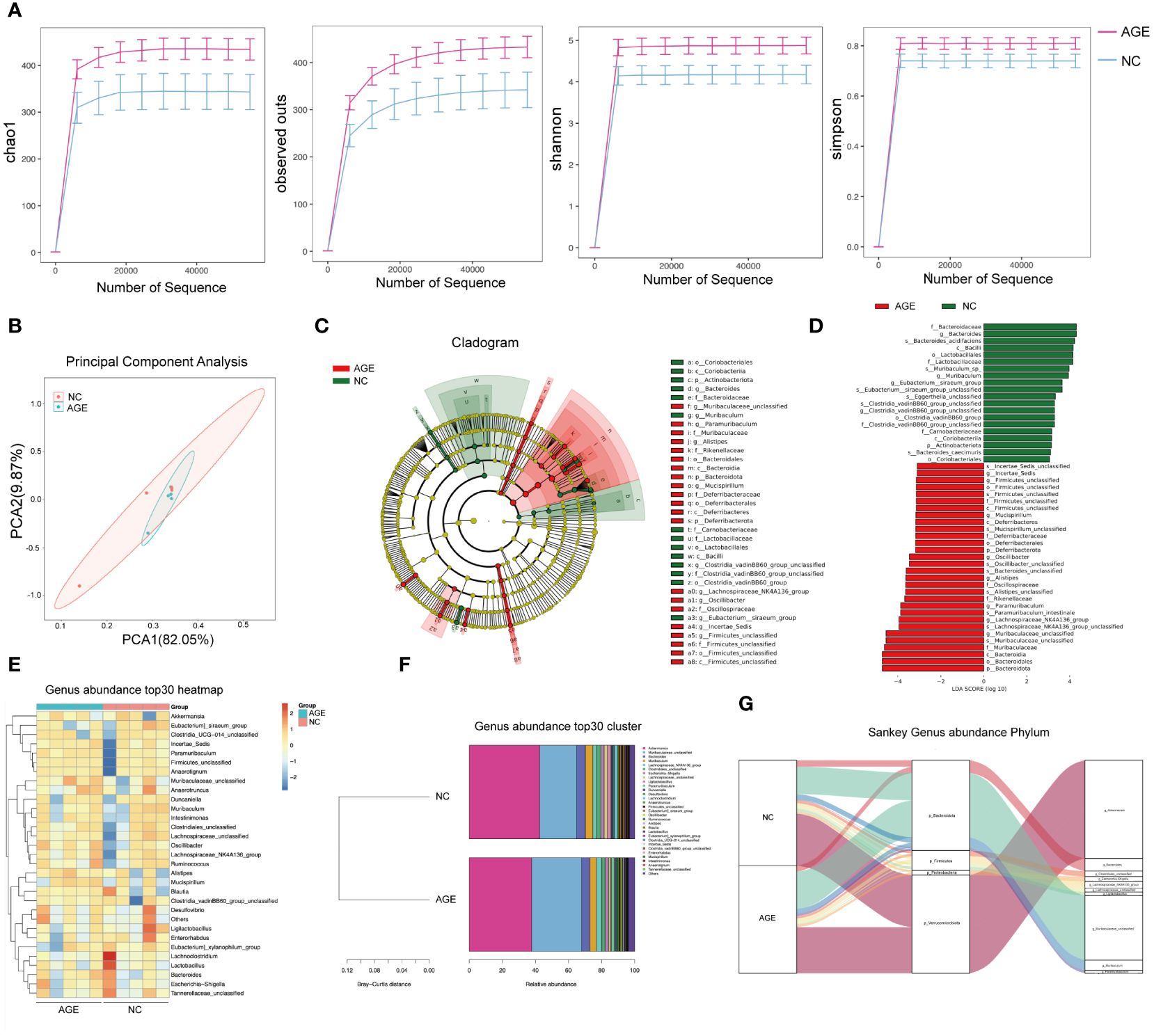
Figure 5 AGE treatment induces intestinal microbiome changes. (A) Chao1 indices were observed, and Shannon and Simpson indices were analyzed in the AGE-treated and control groups. (B) PCoA of β-diversity using the Bray–Curtis dissimilarity. (C, D). Overall exhibition of effect size (LefSe) analysis using a cladogram in the AGE and NC groups. (E–G). The genus present in the AGE and NC groups was analyzed using a heat map and Sankey diagram. AGE, ajoene-enriched garlic extract; NC, negative control; PCoA, principal coordinate analysis.
Discussion
The SARS-CoV-2 pandemic has substantially impacted global health, leading to a cumulative burden on medical resources, society, and the economy. Although numerous drug discovery efforts have led to the authorization of anti-viral drugs and monoclonal antibodies by the Food and Drug Administration (FDA), inexpensive therapeutics that can be rapidly and conveniently translated into clinical use are urgently required. The immunopathogenesis of COVID-19 involves acute respiratory distress syndrome (ARDS) and organ failure (39). We established a SARS-CoV-2 acute lung injury mouse model to mimic the pathological characteristics of SARS-CoV-2 infection. The mouse model exhibited obvious pathological changes, including alveolar pneumonia, characterized by stronger inflammatory responses, including typical alveolar and peribronchiolar inflammation, increased infiltration of neutrophils in BALF and blood, and serious CRS in the lung tissue, resulting in tissue damage and acute lung injury. A decreased percentage of lymphocytes, such as CD3+, CD4+, and CD8+ T cells, was observed in this model.
The immune system plays a pivotal role in the control and immunopathogenesis of SARS-CoV-2 infection. Lymphopenia is commonly observed in the blood of patients with COVID-19, and the degree of lymphopenia correlates with disease severity (40). Our results are consistent with those of patients with COVID-19 (41). Furthermore, this model provides greater safety and convenience for studying the pathogenic mechanisms and pharmacodynamics of agents against COVID-19 (31).
Garlic has been a common herb consumed as a functional food for thousands of years worldwide. Various garlic extracts have been reported to exhibit therapeutic efficacy against various diseases. Ajoene is a synthetic product of the decomposition of allicin. Growing evidence has demonstrated that allicin and ajoene have effective activities as anti-viral, anti-bacterial, anti-inflammatory, and anti-tumor agents (42, 43). Preclinical studies, both in vitro and in vivo, have shown that active organosulfur compounds derived from garlic, such as ajoene, allitridin, garlicin, and diallyl sulfide, possess potential anti-viral (28, 44–47), immune-enhancing, and other therapeutic activities (48–50). Ajoene shows a broad spectrum of in vitro and in vivo activities against zoopathogenic fungi, many yeast strains, zoopathogenic parasites, and several DNA and RNA viruses. Furthermore, in this study, we observed for the first time that AGE could restrain the inflammatory response and recover the immune system, leading to decreased levels of lung injury in SARS-CoV-2 acute lung injury mouse models. Garlic extract reduces neutrophil migration and blocks the biosynthesis of inflammatory cytokines, such as IL-6 and TNF-α (51–54), which supports our results.
The dual anti-viral and anti-inflammatory properties of ajoene against viral infections have been previously described. Ajoene prevents HIV-induced destruction of CD4+T cells and enhances cellular immunity in vitro. Additionally, it inhibits viral attachment to host cells and the reverse transcriptase of HIV-1 (29). Additionally, a non-organosulfur proteinaceous compound derived from garlic showed anti-viral activity against SARS-CoV by inhibiting early viral attachment and viral activity, which provides evidence for the potential anti-viral activity of ajoene in SARS-CoV-2 (45).
NF-κB activation is crucial for the inflammatory response to multiple viral infections, including COVID-19 (55). Ajoene and other garlic extracts directly inhibit NF-κB activation (56). A previous study showed that garlic extract exerts anti-inflammatory activity by inhibiting lipopolysaccharide (LPS)-induced toll-like receptor-4 dimerization, followed by the suppression of cyclooxygenase (COX)-2 (57). In addition, ajoene can target and covalently modify the signal transducer and activator of transcription 3 (STAT3) and COX-2, leading to an attenuated inflammatory response in macrophages (24). COX-2 catalyzes the synthesis of prostaglandins (PGs), which play a major role in mediating inflammatory responses. Coronaviruses, including SARS-CoV-2, enhance the expression of genes of COX-1, COX-2, and cytosolic prostaglandin E synthase (PTGES) (58). SARS-CoV-2 infection upregulates COX-2 expression in vivo in K18-hACE2 mice and in vitro in human cells (59). Similarly, COX-1, COX-2, and PTGES were upregulated in peripheral blood mononuclear cells isolated from patients with COVID-19 compared with healthy controls (60, 61). Therefore, these findings support the evidence that ajoene can treat SARS-CoV-2. However, further studies are required to investigate the cellular pathways involved in the antiviral and anti-inflammatory activities of ajoene against SARS-CoV-2.
In recent years, there is a growing evidence show that the gut microbiota, which plays a pivotal role in shaping both the innate and adaptive immune systems, may significantly contribute to the pathogenesis of COVID‐19 (62). The clinical researches found that a notable aspect of COVID‐19 is the prevalence of severe gastrointestinal symptoms reported by approximately 50.5% of affected individuals (63). Furthermore, clinical analyses have demonstrated that individuals with gastrointestinal involvement tend to experience a more severe disease course (64). A clear collection between SARS-CoV-2 infection and notable abnormalities in the gut microbiota, which marked as a decreased bacterial diversity, an increased opportunistic pathogens, a depletion of beneficial commensal organisms, and impaired biosynthesis of essential metabolites was established (65–70). In the humanized ACE2 knock‐in (hACE2‐KI) mouse model, a notable reduction in intra‐individual bacterial richness (α‐diversity) and distinct variations in intergroup microbiota composition (β‐diversity), was observed following SARS-CoV-2 infection (62). In our study, we found that α-diversity and β‐diversity were recovered with the AGE treatment. Garlic has shown the physiological function of balancing intestinal microbiota in other diseases. This is the first report about the effect of AGE on intestinal microbiota in the SARS-CoV-2 acute lung injury mouse model. However, the change of intestinal microbiota following the SARS-CoV-2 infection with the AGE treatment is still unknown and still needs further study.
SFN is an orally accessible dietary phytochemical observed in high amounts in cruciferous vegetables (71). SFN is commercially available at a low cost and has limited side effects (72, 73). SFN has several activities, such as anti-viral, anti-bacterial, anti-inflammatory, and anti-tumor. Ordonez et al. (74) reported that administration of SFN to K18-hACE2 mice before intranasal SARS-CoV2 infection significantly reduced the viral load in the respiratory tract, lung injury, and pulmonary pathology, suggesting that SFN is a potential agent for preventing COVID-19. However, whether SFN is therapeutic in the SARS-CoV-2 ARDS mouse model remains unclear. In this study, we observed that SFN restrained the inflammatory response and restored the immune system, leading to decreased levels of lung injury in a SARS-CoV-2 ARDS mouse model. SFN is an activator of NRF2, which is suppressed in the lung biopsies obtained from patients with COVID-19 (75). Under pathological conditions, reactive oxygen species (ROS) could damage the body through various pathways. When SARS‐CoV‐2 binds to ACE2, the angiotensin II receptor type 1 (AT1R) axis associated with oxidative stress is upregulated, leading to lung and endothelial damage. NRF2 can enhance the ability of cells to scavenge ROS and reduce oxidative damage. The therapeutic effects of NRF2 activation have been demonstrated in animal models of several lung disorders, including respiratory infections and ARDS (76). Sun et al. (30) reported that SFN exerts a significant anti-inflammatory effect on ARDS in rabbits by upregulating NRF2 expression, which is consistent with our results. NF-κB activation and its consequent release of cytokines contribute to the CRS in patients with COVID-19 (77). SFN inhibited NF-κB activation, endowing the anti-inflammatory properties of SFN, which can be another mechanism that explains the beneficial effects of SFN in patients with COVID-19. In addition, NLR family pyrin domain-containing 3 (NLRP3) inflammasome activation is downstream of SARS-CoV2 infection, which is important in inducing inflammatory responses in patients with COVID-19. NLRP3 is inhibited by SFN, independent of the NRF2 pathway. Gasparello et al. (78) reported that downstream cytokines of NLRP3 inflammasome activation, such as IL-6 and IL-8, which are involved in the cytokine storm, were inhibited by SFN. Therefore, targeting the NLPR3 inflammasome with SFN in bronchial cells had beneficial effects. These results are consistent with those of this study.
This study expanded the indications for the clinical application of AGE and SFN and demonstrated that AGE and SFN can inhibit the inflammatory response and recover the immune response in a SARS-CoV-2 acute lung injury model. Given that AGE and SFN are naturally extracted, orally bioavailable, commercially available, and have limited side effects, our results demonstrate that these two immunomodulatory agents can be promising alternative approaches for treating patients with COVID-19 and ARDS.
Materials and methods
Animal experiments
Female or male hACE2-All CDS-B6J 6–8-week-old mice were purchased from Cyagen Biosciences (Jiangsu, China). All the mouse experiments were approved by the Ethics Review Commission of the Laboratory Animal Center of Zhengzhou University (Approval number: ZZU-LAC20211015). Mice were housed and bred at the Academy of Medical Sciences, Zhengzhou University Center for Medical Animal Models. The animals were maintained under pathogen-free conditions, and care was provided following the International Association for Assessment and Accreditation of Laboratory Animal Care policies and certifications. The COVID-19 acute lung injury mouse models were constructed according to a previous report (31). Mice were intratracheally administered with the fresh mixture of Poly(I:C)-high molecular weight (HMW, 2.5 mg/mL, InvivoGen, tlrl-picw) and SARS-CoV-2 SP (5 μg, ECD, GeneScript, His&Flag Tag, Z03481). Subsequently, mice were euthanized via cervical dislocation, and samples were collected at different time points for different examinations. The different subgroups of animals received different treatments, including 200 μg neutralizing mAb against IL-6R once intraperitoneal (ip), 32 mg/kg daily AGE diluted in oil, 30 mg/kg daily SFN diluted in oil via oral gavage, and placebo oil. Both neutralizing mAb against IL-6R, AGE and SFN treatment angets were administered at 3h post Poly(I:C) and SARS-CoV-2 SP injection. AGE and SFN were obtained from Puer Qiyun Biotechnology Co., Ltd. (China). Neutralizing mAb against IL-6R (#BE0047) was obtained from Bio X cell (USA).
BALF sample collection
Mice were euthanized via cervical dislocation to obtain BALF. First, the tracheal lumen was exposed, and the airway was washed with 1 mL of saline solution via a 26G venous indwelling needle inserted into the tracheal lumen. The average total BALF volume was 1 mL per mouse. Then, BALF was centrifuged at 300 g for 10 min at 4°C to separate the supernatants and cells. The supernatants were collected and stored at –80°C for multiplex cytokine assays. The cells were collected for flow cytometry.
Multiplex cytokines assay
Cytokines or chemokines levels in the sera and BALF of mice were detected using the Mouse LEGENDplex™ Inflammation Panel (13-plex, Biolegend, Cat#740446) following the manufacturer’s instructions. The frozen samples were thawed completely, mixed, and centrifuged to remove particulates before use. The assay was performed using a V-bottom Plate at room temperature (25°C). Samples were read on a 13-laser flow cytometer (CytoFLEX S Flow Cytometer, Beckman), preferably on the same day as the assay. The data were analyzed using BioLegend’s LEGENDplex™ software.
H&E staining
After euthanasia, the lung tissues were collected and fixed with 10% neutral-buffered formalin. Tissues were dehydrated, embedded in paraffin, sectioned, and mounted on slides. Then, the lung sections were stained with H&E according to standard procedures for examination by digital scanning (Pannoramic MIDI, 3DHISTECH). Histological analysis was subjected by two independent skilled pathologists, in double-blind. Briefly, digital light microscopy scans of the lung were examined implementing a semi-quantitative, 5-point grading scheme (0-within normal limits, 1-mild, 2-moderate, 3-marked, 4-severe) based on what has been previously reported (79). The scoring system considered four different histopathological parameters: (1) perivascular inflammation, (2) bronchial or bronchiolar epithelial degeneration or necrosis, (3) bronchial or bronchiolar inflammation, and (4) alveolar inflammation.
Flow cytometry
Mice were deeply anesthetized using ketamine and xylazine. Then, the mouse’s chest was opened, the heart was exposed, and a needle was inserted into the right ventricle to collect blood. Blood was collected and treated with red blood cell lysis buffer to collect leukocytes. Leukocytes from the blood and BALF were incubated with fluorescent antibodies (5μL/test) for 30 min on ice in a flow buffer protected from light. Subsequently, the samples were washed with phosphate-buffered saline (PBS) twice and centrifuged to remove the supernatant. The samples were resuspended in 200 μL flow buffer and then ran on a 13-laser CytoFLEX S Flow Cytometer (Beckman). Flow cytometry standard (FCS) files were analyzed using Flowjo v10.6.2 software (BD). The flow cytometry antibodies as follow: FITC anti-mouse CD45 (Biolegend, Cat #103108), Brilliant Violet 510™ anti-mouse CD3 (Biolegend, Cat #100234), APC/Cyanine7 anti-mouse CD8a (Biolegend, Cat #100714), Alexa Fluor® 700 anti-mouse CD4 (Biolegend, Cat #100430), Brilliant Violet 605™ anti-mouse/human CD11b (Biolegend, Cat #101257), Brilliant Violet 650™ anti-mouse Ly-6G/Ly-6C (Gr-1)(Biolegend, Cat #108442),7-AAD Viability Staining Solution (Biolegend, Cat #420403).
16S rDNA high-throughput sequencing
Fresh faeces of three mice in each group were collected and stored at –80°C until sequencing. The 16S rDNA high-throughput sequencing was performed by LC-Bio Technology Co. Ltd. (Hangzhou, China). Briefly, DNA from different samples was extracted using trimethylammonium bromide (CTAB) according to the manufacturer’s instructions. The CTAB reagent, designed to extract DNA from trace amounts of the sample, is effective for DNA preparation from most bacteria. Nuclear-free water was used as the blank. The total DNA was eluted in 50 μL of elution buffer and stored at –80°C until measurement using polymerase chain reaction (PCR) performed by the LC-Bio Technology Co., Ltd. (Hang Zhou, Zhejiang Province, China). The 5’ ends of the primers were tagged with specific barcodes per sample and sequenced using universal primers. PCR amplification was performed in a total volume of 25 μL reaction mixture containing 25 ng of template DNA, 12.5 μL PCR Premix, 2.5 μL of each primer, and PCR-grade water to adjust the volume. The PCR conditions to amplify the prokaryotic 16S fragments were initial denaturation at 98°C for 30 s; 32 cycles for denaturation at 98°C for 10 s; annealing at 54°C for 30 s; extension at 72°C for 45 s; and then final extension at 72°C for 10 min. PCR products were confirmed using 2% agarose gel electrophoresis. Throughout the DNA extraction process, ultrapure water instead of a sample solution was used as the negative control to exclude the possibility of false-positive PCR results. The PCR products were purified using AMPure XT beads (Beckman Coulter Genomics, Danvers, MA, USA) and quantified using a Qubit (Invitrogen, USA). Amplicon pools were prepared for sequencing. The size and quantity of the amplicon library were assessed using an Agilent 2100 Bioanalyzer (Agilent, USA) and the Library Quantification Kit for Illumina (Kapa Biosciences, Woburn, MA, USA), respectively. Libraries were sequenced on a NovaSeq PE250 platform. Samples were sequenced on an Illumina NovaSeq platform (LC-Bio) according to the manufacturer’s recommendations.
Statistics
All data were analyzed using the GraphPad Prism 9.0 software (GraphPad, San Diego, CA, USA). Statistically significant differences were determined using the unpaired Student’s t-test and one-way analysis of variance (ANOVA) according to the experimental requirements. P-value <0.05 was considered statistically significant, *p<0.05, **p<0.01, and ***p<0.0001.
Data availability statement
The data presented in the study are deposited in the Genome Sequence Archive in National Genomics Data Center, China National Center for Bioinformation/Beijing Institute of Genomics, Chinese Academy of Sciences that are publicly accessible at https://ngdc.cncb.ac.cn/gsa. The accession number CRA016284.
Ethics statement
The animal study was approved by Ethics Review Commission of the Laboratory Animal Center of Zhengzhou University. The study was conducted in accordance with the local legislation and institutional requirements.
Author contributions
SL: Writing – original draft, Writing – review & editing. BW: Writing – original draft, Writing – review & editing. TC: Methodology, Writing – review & editing. HW: Writing – review & editing, Methodology. JL: Writing – review & editing, Methodology. XZ: Writing – original draft, Writing – review & editing. YZ: Writing – original draft, Writing – review & editing.
Funding
The author(s) declare financial support was received for the research, authorship, and/or publication of this article. This work was supported by grants from the Emergency Research Projects on the Prevention and Control of COVID-19 in Henan Province (No. 201100310900 and No. 211100310700).
Conflict of interest
The authors declare that the research was conducted in the absence of any commercial or financial relationships that could be construed as a potential conflict of interest.
The author(s) declared that they were an editorial board member of Frontiers, at the time of submission. This had no impact on the peer review process and the final decision.
Publisher’s note
All claims expressed in this article are solely those of the authors and do not necessarily represent those of their affiliated organizations, or those of the publisher, the editors and the reviewers. Any product that may be evaluated in this article, or claim that may be made by its manufacturer, is not guaranteed or endorsed by the publisher.
Supplementary material
The Supplementary Material for this article can be found online at: https://www.frontiersin.org/articles/10.3389/fimmu.2024.1374541/full#supplementary-material
References
2. Zhou P, Yang XL, Wang XG, Hu B, Zhang L, Zhang W, et al. A pneumonia outbreak associated with a new coronavirus of probable bat origin. Nature. (2020) 579:270–3. doi: 10.1038/s41586-020-2012-7
3. Zhang S, Liu Y, Wang X, Yang L, Li H, Wang Y, et al. SARS-CoV-2 binds platelet ACE2 to enhance thrombosis in COVID-19. J Hematol Oncol. (2020) 13:120. doi: 10.1186/s13045-020-00954-7
4. Lowery SA, Sariol A, Perlman S. Innate immune and inflammatory responses to SARS-CoV-2: Implications for COVID-19. Cell Host Microbe. (2021) 29:1052–62. doi: 10.1016/j.chom.2021.05.004
5. Yang L, Gou J, Gao J, Huang L, Zhu Z, Ji S, et al. Immune characteristics of severe and critical COVID-19 patients. Signal Transduct Target Ther. (2020) 5:179. doi: 10.1038/s41392-020-00296-3
6. Yang L, Liu S, Liu J, Zhang Z, Wan X, Huang B, et al. COVID-19: immunopathogenesis and immunotherapeutics. Signal Transduct Target Ther. (2020) 5:128. doi: 10.1038/s41392-020-00243-2
7. Huang L, Zhao X, Qi Y, Li H, Ye G, Liu Y, et al. Sepsis-associated severe interleukin-6 storm in critical coronavirus disease 2019. Cell Mol Immunol. (2020) 17:1092–4. doi: 10.1038/s41423-020-00522-6
8. Yang L, Xie X, Tu Z, Fu J, Xu D, Zhou Y. The signal pathways and treatment of cytokine storm in COVID-19. Signal Transduct Target Ther. (2021) 6:255. doi: 10.1038/s41392-021-00679-0
9. Mortaz E, Tabarsi P, Varahram M, Folkerts G, Adcock IM. The immune response and immunopathology of COVID-19. Front Immunol. (2020) 11:2037. doi: 10.3389/fimmu.2020.02037
10. Cao X. COVID-19: immunopathology and its implications for therapy. Nat Rev Immunol. (2020) 20:269–70. doi: 10.1038/s41577-020-0308-3
11. Murakami N, Hayden R, Hills T, Al-Samkari H, Casey J, Del Sorbo L, et al. Therapeutic advances in COVID-19. Nat Rev Nephrol. (2023) 19:38–52. doi: 10.1038/s41581-022-00642-4
12. Cavalli G, Larcher A, Tomelleri A, Campochiaro C, Della-Torre E, De Luca G, et al. Interleukin-1 and interleukin-6 inhibition compared with standard management in patients with COVID-19 and hyperinflammation: a cohort study. Lancet Rheumatol. (2021) 3:e253–61. doi: 10.1016/S2665-9913(21)00012-6
13. Li G, Hilgenfeld R, Whitley R, De Clercq E. Therapeutic strategies for COVID-19: progress and lessons learned. Nat Rev Drug Discov. (2023) 22:449–75. doi: 10.1038/s41573-023-00672-y
14. Ganjhu RK, Mudgal PP, Maity H, Dowarha D, Devadiga S, Nag S, et al. Herbal plants and plant preparations as remedial approach for viral diseases. Virusdisease. (2015) 26:225–36. doi: 10.1007/s13337-015-0276-6
15. Lin LT, Hsu WC, Lin CC. Antiviral natural products and herbal medicines. J Tradit Complement Med. (2014) 4:24–35. doi: 10.4103/2225-4110.124335
16. Rouf R, Uddin SJ, Sarker DK, Islam MT, Ali ES, Shilpi JA, et al. Antiviral potential of garlic (Allium sativum) and its organosulfur compounds: A systematic update of pre-clinical and clinical data. Trends Food Sci Technol. (2020) 104:219–34. doi: 10.1016/j.tifs.2020.08.006
17. Ayaz E, Alpsoy HC. Garlic (Allium sativum) and traditional medicine. Turkiye Parazitol Derg. (2007) 31:145–9.
18. Tsai Y, Cole LL, Davis LE, Lockwood SJ, Simmons V, Wild GC. Antiviral properties of garlic: in vitro effects on influenza B, herpes simplex and coxsackie viruses. Planta Med. (1985) 51:460–1. doi: 10.1055/s-2007-969553
19. Verma T, Aggarwal A, Dey P, Chauhan AK, Rashid S, Chen KT, et al. Medicinal and therapeutic properties of garlic, garlic essential oil, and garlic-based snack food: An updated review. Front Nutr. (2023) 10:1120377. doi: 10.3389/fnut.2023.1120377
20. Del Rayo Camacho-Corona M, Camacho-Morales A, Gongora-Rivera F, Escamilla-Garcia E, Morales-Landa JL, Andrade-Medina M, et al. Immunomodulatory Effects of Allium sativum L. and its Constituents against Viral Infections and Metabolic Diseases. Curr Top Med Chem. (2022) 22:109–31. doi: 10.2174/1568026621666211122163156
21. Li M, Yun W, Wang G, Li A, Gao J, He Q. Roles and mechanisms of garlic and its extracts on atherosclerosis: A review. Front Pharmacol. (2022) 13:954938. doi: 10.3389/fphar.2022.954938
22. Apitz-Castro R, Escalante J, Vargas R, Jain MK. Ajoene, the antiplatelet principle of garlic, synergistically potentiates the antiaggregatory action of prostacyclin, forskolin, indomethacin and dypiridamole on human platelets. Thromb Res. (1986) 42:303–11. doi: 10.1016/0049-3848(86)90259-8
23. Srivastava KC, Tyagi OD. Effects of a garlic-derived principle (ajoene) on aggregation and arachidonic acid metabolism in human blood platelets. Prostaglandins Leukot Essent Fatty Acids. (1993) 49:587–95. doi: 10.1016/0952-3278(93)90165-S
24. Hitchcock JK, Mkwanazi N, Barnett C, Graham LM, Katz AA, Hunter R, et al. S-thiolates COX2 and STAT3 and dampens the inflammatory response in RAW264.7 macrophages. Mol Nutr Food Res. (2021) 65:e2000854. doi: 10.1002/mnfr.202000854
25. Agarwal KC. Therapeutic actions of garlic constituents. Med Res Rev. (1996) 16:111–24. doi: 10.1002/(ISSN)1098-1128
26. Hwang JW, Cho H, Lee JY, Jeon Y, Kim SN, Lee SJ, et al. The synthetic ajoene analog SPA3015 induces apoptotic cell death through crosstalk between NF-kappaB and PPARgamma in multidrug-resistant cancer cells. Food Chem Toxicol. (2016) 96:35–42. doi: 10.1016/j.fct.2016.07.020
27. Weber ND, Andersen DO, North JA, Murray BK, Lawson LD, Hughes BG. In vitro virucidal effects of Allium sativum (garlic) extract and compounds. Planta Med. (1992) 58:417–23. doi: 10.1055/s-2006-961504
28. Walder R, Kalvatchev Z, Garzaro D, Barrios M, Apitz-Castro R. In vitro suppression of HIV-1 replication by ajoene [(e)-(z)-4,5,9-trithiadodeca-1,6,11-triene-9 oxide]. BioMed Pharmacother. (1997) 51:397–403. doi: 10.1016/S0753-3322(97)89433-4
29. Bachar SC, Mazumder K, Bachar R, Aktar A, Al Mahtab M. A review of medicinal plants with antiviral activity available in Bangladesh and mechanistic insight into their bioactive metabolites on SARS-coV-2, HIV and HBV. Front Pharmacol. (2021) 12:732891. doi: 10.3389/fphar.2021.732891
30. Sun Z, Niu Z, Wu S, Shan S. Protective mechanism of sulforaphane in Nrf2 and anti-lung injury in ARDS rabbits. Exp Ther Med. (2018) 15:4911–5. doi: 10.3892/etm
31. Gu T, Zhao S, Jin G, Song M, Zhi Y, Zhao R, et al. Cytokine signature induced by SARS-coV-2 spike protein in a mouse model. Front Immunol. (2020) 11:621441. doi: 10.3389/fimmu.2020.621441
32. Zheng J, Wong LR, Li K, Verma AK, Ortiz ME, Wohlford-Lenane C, et al. COVID-19 treatments and pathogenesis including anosmia in K18-hACE2 mice. Nature. (2021) 589:603–7. doi: 10.1038/s41586-020-2943-z
33. Winkler ES, Bailey AL, Kafai NM, Nair S, McCune BT, Yu J, et al. SARS-CoV-2 infection of human ACE2-transgenic mice causes severe lung inflammation and impaired function. Nat Immunol. (2020) 21:1327–35. doi: 10.1038/s41590-020-0778-2
34. D’Alessio FR, Heller NM. COVID-19 and myeloid cells: complex interplay correlates with lung severity. J Clin Invest. (2020) 130:6214–7. doi: 10.1172/JCI143361
35. Braun J, Loyal L, Frentsch M, Wendisch D, Georg P, Kurth F, et al. SARS-CoV-2-reactive T cells in healthy donors and patients with COVID-19. Nature. (2020) 587:270–4. doi: 10.1038/s41586-020-2598-9
36. Mahn A, Castillo A. Potential of sulforaphane as a natural immune system enhancer: A review. Molecules. (2021) 26:752. doi: 10.3390/molecules26030752
37. Shen C, Zhang Z, Tian Y, Li F, Zhou L, Jiang W, et al. Sulforaphane enhances the antitumor response of chimeric antigen receptor T cells by regulating PD-1/PD-L1 pathway. BMC Med. (2021) 19:283. doi: 10.1186/s12916-021-02161-8
38. Houghton CA. Sulforaphane: its “Coming of age” as a clinically relevant nutraceutical in the prevention and treatment of chronic disease. Oxid Med Cell Longev. (2019) 2019:2716870. doi: 10.1155/2019/2716870
39. Zhao X, Zhang Y. Mesenchymal stem cells represent a potential therapeutic option for coronavirus disease 2019-related acute respiratory distress syndrome. Eng (Beijing). (2020) 6:1073–5. doi: 10.1016/j.eng.2020.05.015
40. Qin G, Liu S, Yang L, Yu W, Zhang Y. Myeloid cells in COVID-19 microenvironment. Signal Transduct Target Ther. (2021) 6:372. doi: 10.1038/s41392-021-00792-0
41. Wang F, Nie J, Wang H, Zhao Q, Xiong Y, Deng L, et al. Characteristics of peripheral lymphocyte subset alteration in COVID-19 pneumonia. J Infect Dis. (2020) 221:1762–9. doi: 10.1093/infdis/jiaa150
42. Kaschula CH, Hunter R, Parker MI. Garlic-derived anticancer agents: structure and biological activity of ajoene. Biofactors. (2010) 36:78–85. doi: 10.1002/biof.76
43. Yi L, Su Q. Molecular mechanisms for the anti-cancer effects of diallyl disulfide. Food Chem Toxicol. (2013) 57:362–70. doi: 10.1016/j.fct.2013.04.001
44. Fang F, Li H, Cui W, Dong Y. Treatment of hepatitis caused by cytomegalovirus with allitridin injection–an experimental study. J Tongji Med Univ. (1999) 19:271–4. doi: 10.1007/BF02886960
45. Keyaerts E, Vijgen L, Pannecouque C, Van Damme E, Peumans W, Egberink H, et al. Plant lectins are potent inhibitors of coronaviruses by interfering with two targets in the viral replication cycle. Antiviral Res. (2007) 75:179–87. doi: 10.1016/j.antiviral.2007.03.003
46. Liu ZF, Fang F, Dong YS, Li G, Zhen H. Experimental study on the prevention and treatment of murine cytomegalovirus hepatitis by using allitridin. Antiviral Res. (2004) 61:125–8. doi: 10.1016/S0166-3542(03)00087-1
47. Terrasson J, Xu B, Li M, Allart S, Davignon JL, Zhang LH, et al. Activities of Z-ajoene against tumour and viral spreading in vitro. Fundam Clin Pharmacol. (2007) 21:281–9. doi: 10.1111/j.1472-8206.2007.00470.x
48. Hall A, Troupin A, Londono-Renteria B, Colpitts TM. Garlic organosulfur compounds reduce inflammation and oxidative stress during dengue virus infection. Viruses. (2017) 9:159. doi: 10.3390/v9060159
49. Li YN, Huang F, Liu XL, Shu SN, Huang YJ, Cheng HJ, et al. Allium sativum-derived allitridin inhibits Treg amplification in cytomegalovirus infection. J Med Virol. (2013) 85:493–500. doi: 10.1002/jmv.23480
50. Yi X, Feng F, Xiang Z, Ge L. The effects of allitridin on the expression of transcription factors T-bet and GATA-3 in mice infected by murine cytomegalovirus. J Med Food. (2005) 8:332–6. doi: 10.1089/jmf.2005.8.332
51. Hobauer R, Frass M, Gmeiner B, Kaye AD, Frost EA. Garlic extract (allium sativum) reduces migration of neutrophils through endothelial cell monolayers. Middle East J Anaesthesiol. (2000) 15:649–58.
52. Hofbauer R, Frass M, Gmeiner B, Kaye AD, Frost EA. Effects of garlic extract (Allium sativum) on neutrophil migration at the cellular level. Heart Dis. (2001) 3:14–7. doi: 10.1097/00132580-200101000-00003
53. Hodge G, Hodge S, Han P. Allium sativum (garlic) suppresses leukocyte inflammatory cytokine production in vitro: potential therapeutic use in the treatment of inflammatory bowel disease. Cytometry. (2002) 48:209–15. doi: 10.1002/cyto.10133
54. Jeong YY, Ryu JH, Shin JH, Kang MJ, Kang JR, Han J, et al. Comparison of anti-oxidant and anti-inflammatory effects between fresh and aged black garlic extracts. Molecules. (2016) 21:430. doi: 10.3390/molecules21040430
55. Kircheis R, Haasbach E, Lueftenegger D, Heyken WT, Ocker M, Planz O. NF-kappaB pathway as a potential target for treatment of critical stage COVID-19 patients. Front Immunol. (2020) 11:598444. doi: 10.3389/fimmu.2020.598444
56. Ide N, Lau BH. Garlic compounds minimize intracellular oxidative stress and inhibit nuclear factor-kappa b activation. J Nutr. (2001) 131:1020S–6S. doi: 10.1093/jn/131.3.1020S
57. Youn HS, Lim HJ, Lee HJ, Hwang D, Yang M, Jeon R, et al. Garlic (Allium sativum) extract inhibits lipopolysaccharide-induced Toll-like receptor 4 dimerization. Biosci Biotechnol Biochem. (2008) 72:368–75. doi: 10.1271/bbb.70434
58. Theken KN, FitzGerald GA. Bioactive lipids in antiviral immunity. Sci (New York NY). (2021) 371:237–8. doi: 10.1126/science.abf3192
59. Chen JS, Alfajaro MM, Chow RD, Wei J, Filler RB, Eisenbarth SC, et al. Non-steroidal anti-inflammatory drugs dampen the cytokine and antibody response to SARS-CoV-2 infection. J Virol. (2021) 95(7):e00014–21. doi: 10.1128/JVI.00014-21
60. Perico N, Cortinovis M, Suter F, Remuzzi G. Home as the new frontier for the treatment of COVID-19: the case for anti-inflammatory agents. Lancet Infect Dis. (2022) 23(1):e22–e33. doi: 10.1016/S1473-3099(22)00433-9
61. Yan Q, Li P, Ye X, Huang X, Feng B, Ji T, et al. Longitudinal peripheral blood transcriptional analysis reveals molecular signatures of disease progression in COVID-19 patients. J Immunol (Baltimore Md: 1950). (2021) 206:2146–59. doi: 10.4049/jimmunol.2001325
62. Zhang Y, Ma Y, Sun W, Zhou X, Wang R, Xie P, et al. Exploring gut-lung axis crosstalk in SARS-CoV-2 infection: Insights from a hACE2 mouse model. J Med Virol. (2024) 96:e29336. doi: 10.1002/jmv.29336
63. Groff A, Kavanaugh M, Ramgobin D, McClafferty B, Aggarwal CS, Golamari R, et al. Gastrointestinal manifestations of COVID-19: A review of what we know. Ochsner J. (2021) 21:177–80. doi: 10.31486/toj.20.0086
64. Mao R, Qiu Y, He JS, Tan JY, Li XH, Liang J, et al. Manifestations and prognosis of gastrointestinal and liver involvement in patients with COVID-19: a systematic review and meta-analysis. Lancet Gastroenterol Hepatol. (2020) 5:667–78. doi: 10.1016/S2468-1253(20)30126-6
65. Gu S, Chen Y, Wu Z, Chen Y, Gao H, Lv L, et al. Alterations of the gut microbiota in patients with coronavirus disease 2019 or H1N1 influenza. Clin Infect Dis. (2020) 71:2669–78. doi: 10.1093/cid/ciaa709
66. Nagata N, Takeuchi T, Masuoka H, Aoki R, Ishikane M, Iwamoto N, et al. Human gut microbiota and its metabolites impact immune responses in COVID-19 and its complications. Gastroenterology. (2023) 164:272–88. doi: 10.1053/j.gastro.2022.09.024
67. Ren Z, Wang H, Cui G, Lu H, Wang L, Luo H, et al. Alterations in the human oral and gut microbiomes and lipidomics in COVID-19. Gut. (2021) 70:1253–65. doi: 10.1136/gutjnl-2020-323826
68. Zhang F, Wan Y, Zuo T, Yeoh YK, Liu Q, Zhang L, et al. Prolonged impairment of short-chain fatty acid and L-isoleucine biosynthesis in gut microbiome in patients with COVID-19. Gastroenterology. (2022) 162:548–561 e544. doi: 10.1053/j.gastro.2021.10.013
69. Zuo T, Wu X, Wen W, Lan P. Gut microbiome alterations in COVID-19. Genomics Proteomics Bioinf. (2021) 19:679–88. doi: 10.1016/j.gpb.2021.09.004
70. Zuo T, Zhang F, Lui GCY, Yeoh YK, Li AYL, Zhan H, et al. Alterations in gut microbiota of patients with COVID-19 during time of hospitalization. Gastroenterology. (2020) 159:944–955 e948. doi: 10.1053/j.gastro.2020.05.048
71. Kiser C, Gonul CP, Olcum M, Genc S. Inhibitory effects of sulforaphane on NLRP3 inflammasome activation. Mol Immunol. (2021) 140:175–85. doi: 10.1016/j.molimm.2021.10.014
72. Axelsson AS, Tubbs E, Mecham B, Chacko S, Nenonen HA, Tang Y, et al. Sulforaphane reduces hepatic glucose production and improves glucose control in patients with type 2 diabetes. Sci Trans Med. (2017) 9:eaah4477. doi: 10.1126/scitranslmed.aah4477
73. Singh K, Connors SL, Macklin EA, Smith KD, Fahey JW, Talalay P, et al. Sulforaphane treatment of autism spectrum disorder (ASD). Proc Natl Acad Sci United States America. (2014) 111:15550–5. doi: 10.1073/pnas.1416940111
74. Ordonez AA, Bullen CK, Villabona-Rueda AF, Thompson EA, Turner ML, Merino VF, et al. Sulforaphane exhibits antiviral activity against pandemic SARS-CoV-2 and seasonal HCoV-OC43 coronaviruses in vitro and in mice. Commun Biol. (2022) 5:242. doi: 10.1038/s42003-022-03189-z
75. Cuadrado A, Pajares M, Benito C, Jimenez-Villegas J, Escoll M, Fernandez-Gines R, et al. Can activation of NRF2 be a strategy against COVID-19? Trends Pharmacol Sci. (2020) 41:598–610. doi: 10.1016/j.tips.2020.07.003
76. Liu Q, Gao Y, Ci X. Role of nrf2 and its activators in respiratory diseases. Oxid Med Cell Longevity. (2019) 2019:7090534. doi: 10.1155/2019/7090534
77. Su CM, Wang L, Yoo D. Activation of NF-kappaB and induction of proinflammatory cytokine expressions mediated by ORF7a protein of SARS-CoV-2. Sci Rep. (2021) 11:13464. doi: 10.1038/s41598-021-92941-2
78. Gasparello J, D’Aversa E, Papi C, Gambari L, Grigolo B, Borgatti M, et al. Sulforaphane inhibits the expression of interleukin-6 and interleukin-8 induced in bronchial epithelial IB3-1 cells by exposure to the SARS-CoV-2 Spike protein. Phytomedicine. (2021) 87:153583. doi: 10.1016/j.phymed.2021.153583
Keywords: COVID-19, ajoene-enriched garlic extract, sulforaphane, hACE2, anti-inflammatory
Citation: Liu S, Wang B, Chen T, Wang H, Liu J, Zhao X and Zhang Y (2024) Two new and effective food-extracted immunomodulatory agents exhibit anti-inflammatory response activity in the hACE2 acute lung injury murine model of COVID-19. Front. Immunol. 15:1374541. doi: 10.3389/fimmu.2024.1374541
Received: 23 January 2024; Accepted: 25 April 2024;
Published: 14 May 2024.
Edited by:
Fabrício Souza Silva, Federal University of São Francisco Valley, BrazilReviewed by:
Mohan Tulapurkar, University of Maryland, United StatesDiego De Queiroz, Universidade de Pernambuco, Brazil
Copyright © 2024 Liu, Wang, Chen, Wang, Liu, Zhao and Zhang. This is an open-access article distributed under the terms of the Creative Commons Attribution License (CC BY). The use, distribution or reproduction in other forums is permitted, provided the original author(s) and the copyright owner(s) are credited and that the original publication in this journal is cited, in accordance with accepted academic practice. No use, distribution or reproduction is permitted which does not comply with these terms.
*Correspondence: Yi Zhang, yizhang@zzu.edu.cn; Xuan Zhao, zhaoxuan929@foxmail.com
†These authors have contributed equally to this work