- 1School of Pharmacy, Bengbu Medical College, Bengbu, Anhui, China
- 2Department of Pharmacy, Third Affiliated Hospital of Naval Medical University, Shanghai, China
- 3Shanghai Skin Disease Hospital, School of Medicine, Tongji University, Shanghai, China
- 4Shanghai Engineering Research Center of External Chinese Medicine, Shanghai, China
Tumor immunotherapy is a promising approach for addressing the limitations of conventional tumor treatments, such as chemotherapy and radiotherapy, which often have side effects and fail to prevent recurrence and metastasis. However, the effectiveness and sustainability of immune activation in tumor immunotherapy remain challenging. Tumor immunogenic cell death, characterized by the release of immunogenic substances, damage associated molecular patterns (DAMPs), and tumor associated antigens, from dying tumor cells (DTCs), offers a potential solution. By enhancing the immunogenicity of DTCs through the inclusion of more immunogenic antigens and stimulating factors, immunogenic cell death (ICD) based cancer vaccines can be developed as a powerful tool for immunotherapy. Integrating ICD nanoinducers into conventional treatments like chemotherapy, photodynamic therapy, photothermal therapy, sonodynamic therapy, and radiotherapy presents a novel strategy to enhance treatment efficacy and potentially improve patient outcomes. Preclinical research has identified numerous potential ICD inducers. However, effectively translating these findings into clinically relevant applications remains a critical challenge. This review aims to contribute to this endeavor by providing valuable insights into the in vitro preparation of ICD-based cancer vaccines. We explored established tools for ICD induction, followed by an exploration of personalized ICD induction strategies and vaccine designs. By sharing this knowledge, we hope to stimulate further development and advancement in the field of ICD-based cancer vaccines.
1 Introduction
Cancer remains the most formidable disease globally, with over 19,292,789 new cases and approximately 9,958,133 cancer-related deaths recorded worldwide each year (1). As is well-known, tumor cells primarily evade immune surveillance by downregulating tumor-associated antigens (TAAs) and tumor-specific antigens (TSAs), and releasing soluble antigens and MHC molecules (2). Therefore, tumor immunotherapy has emerged as a crucial therapeutic approach for suppressing both primary and metastatic tumors. Moreover, immunotherapy can confer long-term immune protection for the body (3). Immune checkpoint blockade (ICB) represents a novel groundbreaking tumor immunotherapy that targets two key immune checkpoint pathways programmed cell death protein 1/programmed death ligand 1 (PD-1/PD-L1) and cytotoxic T lymphocyte-associated protein 4/B7 (CTLA-4/B7). By disrupting the mechanisms of tumor immune resistance, ICB can effectively promote antigen-specific T-cell immune responses. Currently, more than ten ICB drugs have been approved by the FDA for the treatment of a broad spectrum of tumors, offering new hope in the fight against cancer (4). However, the efficacy of ICB drugs appears to be less than satisfactory against late-stage patients in relevant clinical trials, with only a few patients benefiting from these treatments (5).
Cancer vaccines represent a promising emerging approach in tumor immunotherapy, offering extensive application prospects (6). The complexity of the preparation process, coupled with insufficient antigenic immune effects and immune cell dysfunction, have emerged as the primary factors limiting the development of cancer vaccines (7, 8). Nonetheless, the identification of immunogenic cell death (ICD) as a distinct form of tumor-regulated cell demise has offered new prospects to overcome the limitations hampering the advancement of cancer vaccines (9). In this regard, the capacity of chemotherapy, radiotherapy, photothermal therapy, and sonodynamic therapy to induce ICD, as evidenced in recent studies, could present a novel approach for reshaping conventional methods in oncology (10). It is now understood that the importance of ICD-based cancer vaccines lies in the in vivo application of ICD nanomedicine inducers and the production of dying tumor cells (DTCs) in vitro during tumor treatment (Figure 1).
ICD, induced by the chronic release and exposure of damage-associated molecular patterns (DAMPs) including calreticulin (CRT), adenosine triphosphate (ATP), high mobility histone 1 (HMGB1), heat shock protein (HSP) activates the recruitment and activation functions of neutrophils, macrophages, and dendritic cells. This orchestrated immune response associated within the tumor microenvironment exhibits characteristics akin to vaccination, a concept gaining significant traction within the research community (11). Simultaneously, during the study of ICD induction against tumor cells in vitro, it has been discovered that a substantial number of immunogenic DTCs are generated following ICD induction. These DTCs possess a strong ability to release immunogenic substances, such as DAMPs, which continuously trigger a potent immune stress response, remodel the immune microenvironment, and enhance the body’s immune surveillance capabilities. In summary, “ICD-based cancer vaccines” offer advantages such as broad-spectrum antigens and diverse induction conditions, which can mitigate numerous adverse factors in the development and application of cancer vaccines. Overall, the advent of ICD-based cancer vaccines has demonstrated significant potential for the immunotherapy of relevant tumors (12).
However, the pursuit of efficient and stable methods for ICD induction remains critical for developing and evaluating relevant ICD-stimulating nanomedicines. Since ICD inducers and induction strategies are key focuses in ICD-based cancer vaccine research, this review highlights recent advancements in ICD-based cancer vaccines from both in vivo and in vitro perspectives. Our goal is to provide more reliable preparation protocols and strategies for ICD-based cancer vaccines, thereby promoting their continued development and application. A crucial area of investigation for these vaccines, compared to other immunotherapies, lies in their potential to address tumor recurrence in long-term survivors of metastatic and invasive cancers To achieve curative potential, we advocate for the synergistic combination of immune-stimulating tools with ICD-based cancer vaccine therapy.
2 The role of ICD in anti-cancer immunity
Well-documented by numerous studies, immunogenic cell death plays a critical role in generating an immunogenic tumor phenotype, effectively overcoming immunosuppressive effects of a non-immunoreactive tumor microenvironment (TME).ICD is characterized by CRT exposure, ATP release, and leakage of HMGB1 and HSP (13). These processes facilitate the uptake of TAAs by adaptive immune cells, triggering a broad-spectrum antigen-specific immune response, promoting DC maturation, and enhancing the search for DTCs (14). Furthermore, ICB therapy relies on the production and activation of tumor antigen-specific T cells. Consequently, the release of specific antigens from ICD tumor cells is crucial for reshaping the TME and has been validated in the combination of ICB with chemotherapy and radiotherapy. The recruitment of adaptive immune cells, neutrophils, macrophages, and NK cells all can activate innate effector mechanisms (15).
Immunogenic substances released during ICD can be classified into constitutive DAMPs (cDAMPs) and inducible DAMPs (iDAMPs). cDAMPs consist of immune-stimulating molecules such as CRT, ATP, HMGB1, and HSP, which are expressed prior to tumor cell death (16). iDAMPs are endogenous molecules produced by underlying mechanisms during tumor cell death, primarily including cytotoxic T-lymphocytes (CTLs) with CD3+, CD4+, and CD8+, releasing interferon-α (IFN-α), granzymes, lysins, and perforins (17). In this respect, CRT and HSP emit eat-me signals. CRT-CD91 and HSP90-CD91 interactions promote endocytosis signals in tumor cells, inducing antigen presentation and specific CTL responses. Additionally, the release of tumor necrosis factor-α (TNF-α) and interleukin-6 (IL-6) (18); ATP emit energy signals, ATP-P2RY2 binding is involved in the recruitment of monocytes or macrophages, neutrophils, and promoting DC maturation (19); HMGB1, aided by chemokines, binds to pattern recognition receptor (PRR) (P2RX7, P2RY2), CD91, CD40, and Toll-like receptor 4 (TLR4) receptors on the surface of antigen-presenting cells (APCs) (20). Upon the functional activation of APCs, those APCs exposed to TAA and TSA immunostimulants initiate cross-presentation to CD4+/CD8+ T cells, enhancing DC antigen presentation and CTLs proliferation (14). Consequently, the transition from a “cold” to a “hot” tumor immune microenvironment occurs, accompanied by changes in the secretion levels of immunostimulatory and immunosuppressive factors (up-regulation of IFN-γ, TNF-α, and IL-12; down-regulation of IL-4, IL-6, and IL-10), as well as the depletion of myeloid-derived suppressor cells (MDSCs), regulatory T cells (Tregs), and tumor-associated macrophages 2 (TAM2) (21). The above studies overlap in their assertion that ICD, as an in-situ vaccine, possesses potential immunomodulatory abilities and holds great value in reversing the TME and improving the efficacy of tumor immunotherapy.
3 Inducers of in situ ICD-based cancer vaccines
Over the years, a multitude of ICD inducers have been developed, especially when integrated with nanotechnology, to boost the effectiveness of ICDs. Administering ICD nano-inducers to the tumor site not only averts degradation and premature active ingredient release but also enhances their penetration and retention capabilities (EPR) (22). Presently, the primary driving methods of ICDs encompass chemotherapy, photodynamic, photothermal, radiotherapy, and sonodynamic therapies Table 1. Thus, a comprehensive investigation of ICD induction mechanisms and inducers holds substantial importance in advancing the development of “in situ ICD-based cancer vaccines”. The release of cancer cell immunogenic antigens induced by ICD and the presentation of these antigens by APCs constitute the fundamental stages of the “cancer vaccine-like function” observed in vivo (Figure 2). We introduce the ICD induction methods with some latest research to provide more evidence in the field of ICD-vaccine.
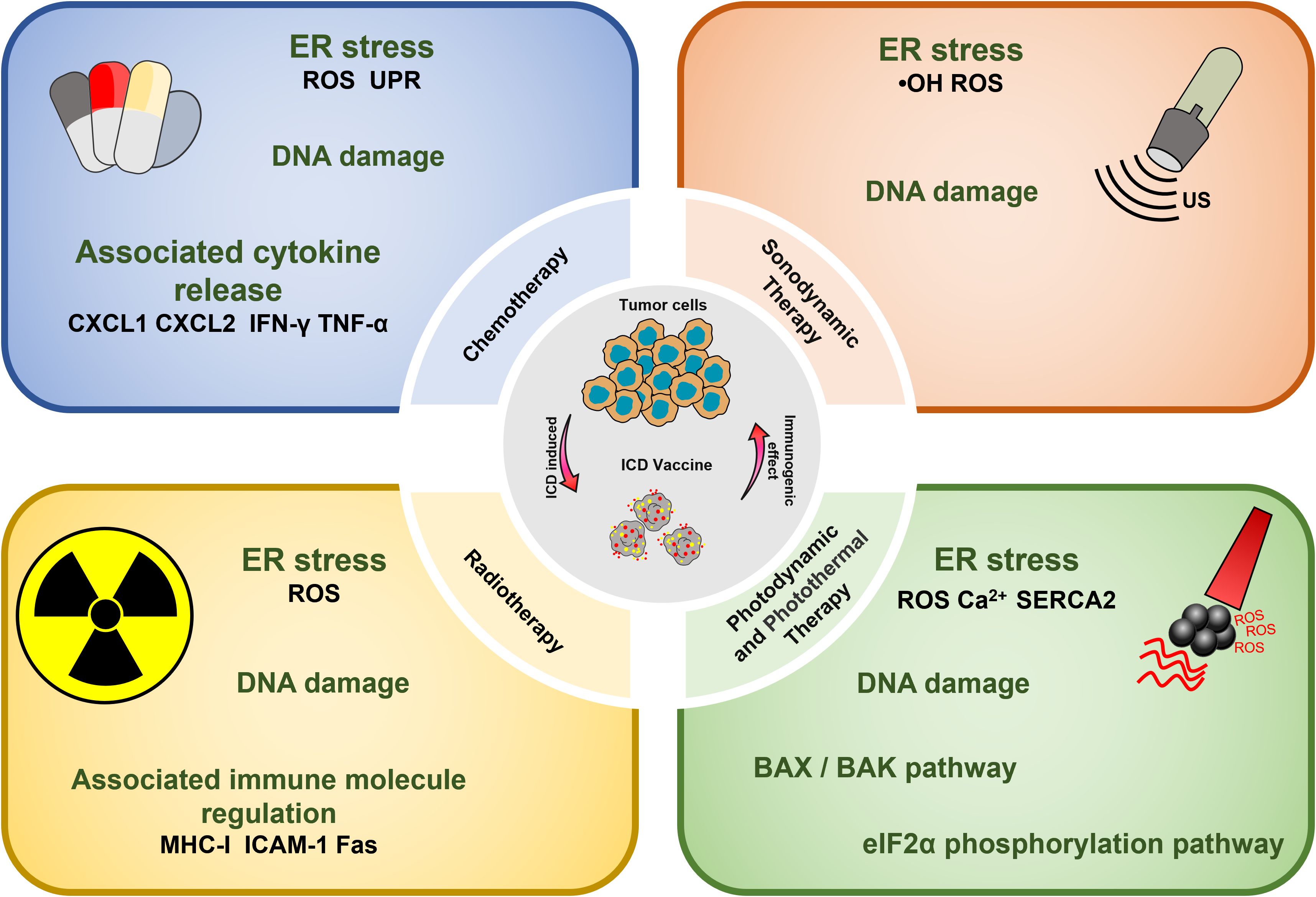
Figure 2 Overview of ICD-based cancer vaccines and the associated mechanisms involved in ICD regulation.
3.1 Chemotherapy-induced ICD-based cancer vaccines
Chemotherapeutic agents that have been shown to be effective for ICD induction include: Idarubicin; Epirubicin, Doxorubicin (DOX) (23), Mitoxantrone, Oxaliplatin (Oxp) (25), Bortezomib, Cyclophosphamide, and Paclitaxel (PTX) (27, 47). Studies have demonstrated that the induction of ICD by chemotherapeutic agents, particularly anthracyclines, is accompanied by phenomena such as the unfolded protein response within the endoplasmic reticulum (ER) and the generation of reactive oxygen species (ROS). These events are primarily caused by the DNA damage induced by chemotherapeutic agents to secondary structures, including the cytoplasm (28). Chemotherapy-induced ICD can also lead to the release of CRT, ATP, HMGB1, CXCL1, and CXCL2, which ultimately induces an immune stress response, triggering a sustained antitumor effect (48).
In experiments exploring the use of chemotherapeutic nanomedicines for ICD induction, some researchers employed nanoprecipitation technology to develop and design nanomedicines (called Nano-Folox), which contain Oxp derivatives and FnA. Nano-Folox not only induced ICD, but also synergistically interacted with free 5-Fu to induce a shift from cold to hot tumors. This ultimately led to a significant inhibition of tumor growth in CRC mouse models (29). Liu et al. synthesized liposomes carrying Oxp and coupled with indoximod (IND) precursors; the nanoparticles not only enhanced the ability of Oxp to induce ICD in pancreatic ductal carcinoma (PDAC) but also stimulated antitumor immune responses in PDAC (49). In recent years, researchers have designed nanoplatforms integrating OXA with polyethylene glycolated photosensitizer (PS) prodrugs, exhibiting good stability in blood circulationand able to complete drug release and ICD induction under near-infrared (NIR) irradiation. They also explored the enhancing effect of CD47 blockade on tumor ICD induction (50). Xie et al. designed nanoparticles MDP NPs self-assembled from DOX, MnO2 nanoparticles, Fe3+, and PEG-polyphenol ligands, which could enhance DOX-based tumor ICD induction and achieve high expression of TAAs, DC maturation, and infiltration of tumor-specific T-cells (51). Some researchers have constructed ROS-responsive polymers (R-SIP) using hydrophilic polyethylene glycol (PEG) and a hydrophobic self-immolative backbone, loaded with DOX. This nanoparticle could release DOX in response to spontaneous depolymerization of ROS and undergo depolymerization to produce azoquinone methyl ether derivatives that significantly deplete GSH, increase the level of oxidative stress, and ultimately enhance the induction of ICD in oncological treatments (52).
3.2 PDT-induced ICD-based cancer vaccines
PDT has shown effectiveness in inducing tumor cell death, solidifying its role as a viable clinical application in oncology. Currently, photosensitizers with the ability to induce cell death include Hypericin (33), 5-ALA (34), and Rose-Bengal acetate (36). The induction of ICD in tumor cells during PDT treatment is primarily attributed to the generation of intracellular ROS under NIR irradiation, which occurs after photosensitizer aggregation in the ER, causing disruptions in ER homeostasis, elevating calcium levels in the ER, and losing SERCA2 function. Mitochondrial dysfunction, characterized by oxidative damage to mtDNA and BAX/BAK-mediated apoptosis, triggers a series of events culminating in immunogenic stress. This process begins with the release of ATP and exposure of CRT on the mitochondrial surface. Subsequently, DAMPs, such as heat shock protein 70 (HSP70), are released, initiating a localized inflammatory response. Ultimately, these events contribute to the development of an immunogenic stress response (53). Beyond the intrinsic properties of the photosensitizer, the ROS generation and ICD-inducing effects can be severely limited by the constraints of hypoxia and insufficient tumor penetration in the TME (54). Therefore, it is crucial to address adverse factors in the TME to enhance ICD induction by PDT. Several strategies can be employed for this purpose, such as inducing mitochondrial damage that causes ER disorders and calcium overload (55); utilizing oxygen carriers like hemoglobin (Hb) as catalysts for ROS generation, and modifying the targeting effect of ER-targeting pardaxin (FAL) to enable more drugs to be internalized into the ER, thus increasing the efficacy of PDT (37).
Studies onPDT-induced ICD have explored the use of a smart semiconductor polymer nano-immunomodulator (SPNI) that could be activated under acidic TME. When it was used in the therapeutic process, SPNI exerted a photodynamic effect, directly ablating tumors and inducing ICD when exposed to NIR light treatment, while R837 promoted DC maturation and pro-inflammatory cytokine secretion (56). Qiu et al. incorporated the photosensitizer Chlorin e6 (Ce6) doped with the chemotherapeutic agent 10-hydroxycamptothecin (HCPT) into calcified nanocarriers CHC NPs. CHC NPs can generate ROS, causing mitochondrial dysfunction and inducing the ICD. This phenomenon is also crucial to compensate for the lack of results from insufficient immunogenic tumor microenvironment (ITME) in HCPT treatment (31). Zhu et al. demonstrated that a platelet membrane fusion liposome nanovesicle system (named TFL) loaded with type I AIE photosensitizer TBP-2. In this study, TBP-2 has the potential to increase the incidence of cuproptosis and induce the vaccine-function in tumor site (57, 58).
3.3 PTT-induced ICD-based cancer vaccines
PTT is a potential non-invasive treatment strategy that converts NIR energy into heat by photothermal agents, ultimately leading to tumor ablation. Interestingly, it was found that PTT could induce ICD and assume a role akin to a vaccine (59). The types of photothermal agents that can induce the photothermal effect at present include (1) precious metals Au, Ag, Pt, etc., which have high photothermal conversion efficiency and imaging; (2) Carbon materials graphene, carbon nanorods, with large photothermal conversion area but poor NIR absorption; (3) Metal and non-metal compounds, CuS, ZnS; with high photothermal and low cost; (4) Organic and inorganic nanomaterials. The ICD-inducing ability of PTT therapy may be attributed to several factors. Firstly, the photothermal agent initiates temperature changes at the tumor site, leading to a Fenton-like reaction, peroxidation reaction, and direct induction of H2O2 production in the TME (60). These results in intracellular Ca2+ overload within tumor organelles (61). The process involves mitochondria damage including reduced membrane potential and the generation of mitochondrial reactive oxygen species (mtROS) and the up-regulation of the PERK-mediated eukaryotic initiation factor 2α (eIF2α) phosphorylation pathway. Disruption of the ER structure is a hallmark of ICD. This process ultimately culminates in the release of a significant amount of DAMPs into the cytoplasm (62).
In recent years, the catalytic properties of Fenton metals, particularly copper and iron, have emerged as crucial mechanisms for inducing cell death processes termed copper death and iron death. The utilization of photo-activated copper, exhibiting exceptional photo-oxidation and reduction catalytic efficiency has ushered in a new era of photosensitizers. This advancement has significantly contributed to the burgeoning research in innovative photodynamic and photothermal therapies for tumors (63). One approach involves the synthesis of multifunctional nanoplatforms, such as Cu-PDA-FA, by combining polydopamine (PDA) with Cu2+ through chelation technology. Cu-PDA-FA not only induces ICD and cancer vaccine-like effects but also amplifies the efficiency of conversion (38). Some researchers designed CaO2 and Cu2Se conjugates, which showed the ability to induce ICD after being activated by NIR-II. Indeed, the Ca2+ overload in the ER enhances the immune activation capacity. Reinforcing this concept, research on photothermal materials (40). Zinc oxide (ZnO) has been identified as an efficient drug carrier responsive to tumor pH that significantly inhibits tumor growth. Building on these findings, scientists have designed a multifunctional composite nanoplatform (AuNP@mSiO2@DOX-ZnO) to harness the synergistic therapeutic effects. This platform could promote ICD, maturation of DCs, and proliferation of effector T cells, ultimately preventing tumor growth and metastasis (41). Ran et al. designed nano-platforms PBDB-T NPs using the organic photovoltaic material PBDB-T through a nanoprecipitation method. PBDB-T NPs exhibited favorable photothermal therapeutic effects and the ability to induce ICD. Importantly, they increased the efficiency of DAMPs production after mild-temperature PTT (mPTT) treatment (64). Tian et al. developed a mesoporous polydopamine nanoparticle MPDA. IR-780@MPDA not only induced ICD-activated CTLs in a therapeutic 4T1-homozygous mouse model under NIR treatment, but it also demonstrated its utility for in vivo photoacoustic (PA) imaging, as evidenced by the PA imaging tracings (39).
3.4 SDT-induced ICD-based cancer vaccines
It is well-established that SDT enables the concentration of ultrasound energy at the tumor site, leveraging the cavitation effect to elevate local temperatures and enhance drug decomposition. This process can generate free radicals and produce ROS under the action of endogenous substances in the cell, thereby achieving the purpose of local killing of the tumor. SDT offers several advantages, inducing high tissue penetration, low invasiveness, high controllability, and low costs (65). Consequently, SDT is safer than the traditional means of tumor treatment, such as chemotherapy, radiotherapy, and can minimize damage to normal tissues during the treatment process. Furthermore, ultrasound (US) has an ideal depth of tissue penetration (10 cm), which greatly mitigates the inhibitory effects of hypoxia, low pH, and other unfavorable factors in complex TME, which confers SDT a stronger ICD induction effect (66).
In recent years, a new type of calreticulin nanoparticles (CRT-NP) has been developed, which can be activated by focused ultrasound (FUS) and induce ICD during melanoma immunotherapy. The CRT-NP in vivo therapeutic study of CRT-NP benefits from the non-invasiveness of has the advantage of ultrasound, couple with a thermal effect that transforms the TME to enhance TAA release, HSP expression, and up-regulation of CRT to stimulate tumor immune stress response (67). Some researchers designed perfluorocarbon nanoparticles (LIP-PFH NPs), which also exhibited tumor suppression effect and ICD induction effect on breast cancer cells by SDT (68). Besides, researchers have synthesized mitochondria-targeted liposome nanoparticles (MLipRIR NPs), which could be activated by ultrasound and released R162 that disrupted the glutaminolysis pathway in mitochondria and down-regulates glutathione peroxidase (GPx) enzyme expression. At the same time, IR780 generated large amounts of ROS in response to US treatment, disrupting normal mitochondrial function and inducing ICD (42). Building on the discovery that a combined photodynamic and sonodynamic therapy(PSDT) reduces sonosensitizer dose and energy loss, Zheng et al. developed the OIX-NP. This nanoparticle comprises poly(lactic-co-glycolic acid) (PLGA) encapsulating oxygen-carrying perfluoropentane (PFP), an ICG near-infrared dye and an Oxp chemoinducer. The OIX-NP not only efficiently induce ICD, but also demonstrated significant potential for imaging applications (44).
3.5 RT-induced ICD-based cancer vaccines
It has been reported that RT can accumulate energy in the tumor site by using high-energy X-rays, Y-rays, or other isoelectronic radiation (IR). Then RT induces DNA damage, destroying double-stranded DNA (dsDNA), leading to ICD. This process results in the release of key signaling molecules, including CRT, HSP70 and HMGB1, within the TME (69). The induction of ICD by RT mainly involves the up-regulation of histocompatibility complex I (MHC-I) molecule, intercellular adhesion molecule-1, and factor-related apoptosis (Fas) (70). This process contributes to the so-called “distant effect” and inhibits the development of metastasis. Thus, RT has the potential to act as an in-situ vaccine (71, 72).
While radiotherapy is a mainstay of local tumor treatment, its effectiveness in controlling distant tumor spread is limited. However, the recent discovery of RT’s ability to induce ICD offers a promising avenue to revitalize RT therapy. Importantly, research suggests that the degree of immune modulation within the tumor microenvironment by RT is dependent on the radiation dose (73). He et al. found that gold nanoparticles AuNPs were able to increase the expression of phosphorylated eIF2α (p-eIF2α) in G422 glioblastoma cells under RT treatment, promote eIF2α protein phosphorylation, and induce ICD. The study also verified the ICD-inducing ability of AuNPs under RT treatment in a therapeutic G422 tumor-bearing mice model (74).
4 In vitro induction of DTCs for cancer vaccines
“ICD-based cancer vaccines” involve DTCs for re-infusion vaccination in vivo, ultimately leading to tumor therapy. DTCs, produced after treatment with ICD inducers, exhibit excellent immunogenicity (Figure 3). In this respect, studies have reported that ICD-based cancer vaccines can induce robust immune activation in mouse models during prophylactic vaccination trials in vivo (75). ICD-based cancer vaccines offer a distinct advantage by inducing a comprehensive tumor cell antigen expression profile. This eliminates the dependence on a single antibody for recognition, a major hurdle in conventional immunotherapy. By promoting the expression of diverse tumor antigens, ICD vaccines significantly reduce the risk of tumor cells evading immune attack during treatment. The rationale behind this approach lies in the ability of ICD inducers to stimulate the spontaneous production of relevant antigens, DAMPs, and immune activation molecules within tumor cells. This comprehensive immune response translates to potent antitumor effects, establishment of long-term immune memory against the tumor, and aligns perfectly with the evolving paradigm of immunotherapy, which seeks to integrate preventive and therapeutic strategies.
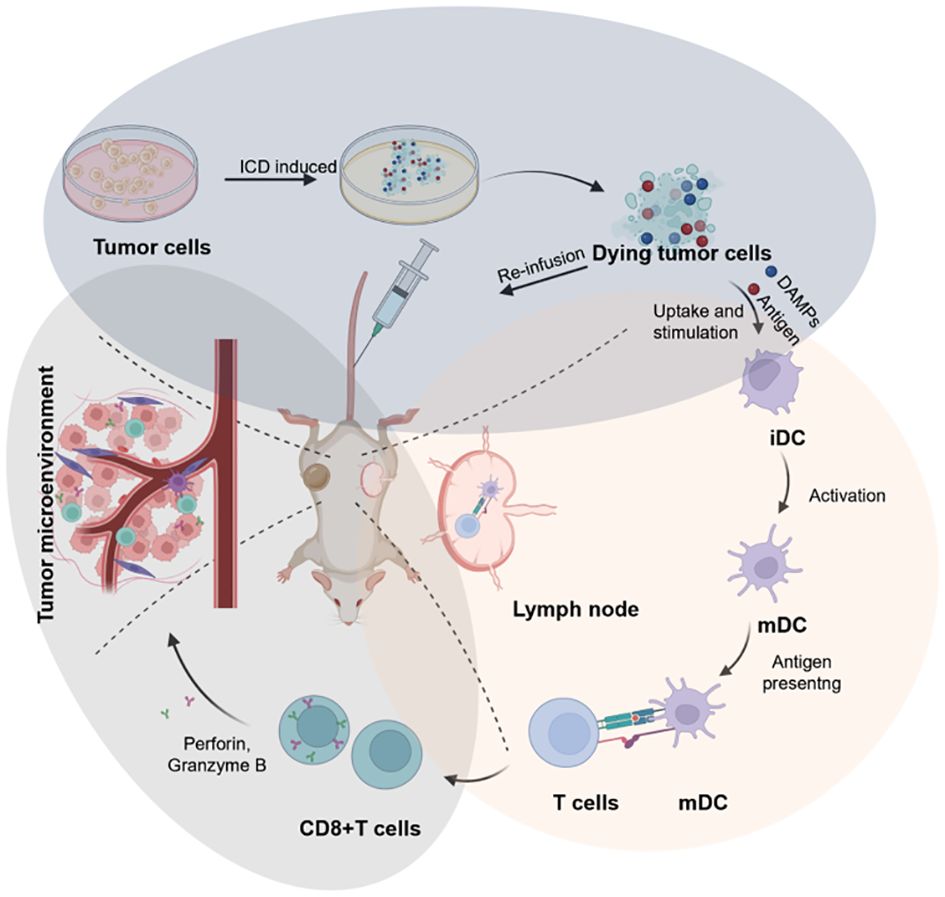
Figure 3 Schematic illustration of the design of cancer vaccines inspired by DTCs for eliciting humoral and cellular immunity, which can be broken into three key stages: the preparation of ICD-based cancer vaccines (blue circle), the immune activation include DC maturation and antigen presentation (red circle), and the immune effect of CD8+T cells in TME for anti-tumor therapy (grey circle). Created by © 2023 BioRender.
Studies have explored various methods for the preparation of ICD-based cancer vaccines include: One approach utilizes chemotherapy. Qing et al. demonstrated that DOX can induce tumor cells to become dendritic cell activators. They employed liquid nitrogen cryogenics to generate frozen dying tumor cells (FDTs), which achieved a 38% tumor elimination rate in the MC100 peritoneal carcinoma mouse model. Furthermore, in combination with cytokines IL-12 and aPD-L1, they achieved 100% eradication in the peritoneal metastasis model of colorectal carcinoma (76). Li et al. showed that tumor antigens CIAs induced by chemotherapeutic agents in vitro, triggering an immune response and demonstrating synergistic effects with anti-PD-1 therapy (77). Another approach involves using radiotherapy. Researchers have successfully prepared nano-vaccines in vitro using RT-induced tumor cells. These vaccines demonstrated efficacy in treating metastatic tumors and enhanced immunotherapeutic effects when combined with anti-PD-1 treatment (78).Although research on ICD inducers and strategies is more advanced, the development of ICD-based cancer vaccines in vitro is still in the preliminary stage. However, achieving high efficiency and low cost remains crucial research criteria for ICD-based cancer vaccines. Therefore, investigating whether photodynamic and sonodynamic therapy can be an ideal induction for ICD-based cancer vaccines is expected to be a worthy direction for more researchers to explore. Given the promising results of ICD induction using chemotherapy and radiotherapy, investigating the potential of PDT and SDT as triggers for ICD-based cancer vaccines warrants further exploration. we posit that the personalized design of these ICD-based cancer vaccines holds immense potential for advancing clinical translation in antitumor immunotherapy translation. As an example, previously mentioned strategies like encapsulating patient-specific nanoparticles and obtaining DC activators from tumors offer valuable avenues for personalization. While current personalized cancer vaccine development primarily focus on immune cells loaded with immune-stimulating agents, future breakthroughs lie in utilizing specific immune elements to create novel and highly effective therapeutic strategies.
5 ICD related clinical cancer therapy
In clinical immunotherapy studies involving non-small cell lung cancer, hepatocellular carcinoma, breast cancer, bladder cancer, melanoma, squamous carcinoma, and other solid tumors, the induction of ICD has demonstrated the ability to enhance the presentation function of APCs such as DCs, deplete Treg cells, and activate the vitality of immune cytotoxic effector cells. Thess combined effects ultimately lead to improved efficacy in tumor therapy.
As summarized in (Table 2), clinical trials have primarily focused on chemotherapy and radiotherapy for inducing ICD, demonstrating its clinical applicability. Researchers have further shown that physical treatments, like cryoablation, can also trigger ICD, potentially improving cost-effectiveness and clinical translation efficiency. However, significant progress is needed to develop and translate ICD-based cancer vaccines for clinical use. Therefore, exploring novel therapeutic approaches based on ICD-based cancer vaccines research and establishing a system to comprehensively evaluate the types and levels of immunogenic substance generated by ICD are crucial next steps.
6 Discussion and conclusion
The immunological adjuvant effect of ICD is now understood to be intricately linked to the exposure and release of cellular DAMPs. These DAMPs include exposed CRT, secreted ATP, ANXA1, TNF-α, and HMGB1, as well as phosphorylated eIF2α. However, additional valuable markers likely remain undiscovered. For example, low levels of autophagy can both protect cells and limit the release of immunogenic substances, while potentially increasing the risk of oncogenicity during treatment. Mounting evidence suggests that tumor autophagy, once thought to be similar to apoptosis, can also impact the effectiveness of antitumor immunotherapy. As expected, the autophagy inducer called STF-62247 (STF) can effectively convert protective autophagy into ICD, thereby enhancing antitumor immune activation. Furthermore, the uncertainty surrounding conventional methods of inducing ICD and determining the optimal dosage of ICD inducers makes it challenging to accurately quantify the release of associated immunogenic DAMPs. As ICD-based cancer therapy gains wider acceptance in clinical settings, it becomes increasingly important to select closely correlated assay secretions for screening ICD inducers that possess efficient induction capabilities for future development of ICD-based cancer vaccines.
Meanwhile, the development of safer and more reliable in vitro systems for ICD-based cancer vaccines, as well as the elimination of unfavorable factors in vivo, are areas that warrant attention and in-depth exploration. While some personalized ICD-based cancer vaccine research has been discussed above, providing potential mechanisms for their effectiveness, the activation of immunity by cancer vaccines is a complex process. For instance, studies have demonstrated that targeting the STING pathway can activate innate immune signaling in immune-infiltrating cells and within tumor cells, ultimately inducing ICD. However, the effectiveness of STING pathway activation within tumor cells may vary depending on the tumor type., Nanotechnology offers a potential solution by enhancing the delivery of STING activators to cells, thereby improving their efficacy (79). The involvement of the CXCL12/CXCR4 signaling pathway also plays a role in various physiological processes, such as tumor survival, invasion, metastasis, angiogenesis, and the creation of hypoxic environments (80). Additionally, CXCR4 expression can facilitate the transportation of MDSCs in different tumors, leading to the creation of an immunosuppressive TME and immune resistance (81). In gliomas, blocking CXCR4 signaling using nanoparticles loaded with CXCR4 inhibitors can reduce the infiltration of immunosuppressive MDSC and trigger an adaptive immune response (82).
In recent years, immunotherapy has made remarkable strides in the treatment of cancer, particularly with the emergence of cancer vaccines based on immune cell death. These vaccines hold great promise for expanding and enhancing tumor immunotherapy. In this article, we expounded on the mechanisms of ICD as a potent tool for regulating TME and explored the vast potential of ICD-based cancer vaccines. The successful clinical application of ICD-based cancer vaccines necessitates a rigorous evaluation of their therapeutic value. Therefore, we advocate for the identification of more robust clinical evaluation indicators, particularly those that assess the efficacy of ICD induction by these vaccines. A comprehensive assessment of both safety and efficacy is paramount in determining the transformative potential of this approach in cancer treatment. Further exploration of the intricate mechanisms underlying ICD and its role in tumor immunotherapy is crucial to unlocking the full potential of this innovative strategy. Our ongoing research endeavors to contribute to the expanding body of knowledge surrounding ICD-based cancer vaccines and ultimately pave the way for their successful translation into clinical practice through meticulous evaluation and analysis. In conclusion, the utilization of immunogenic patterns generated by ICD in tumor cells represents a paradigm shift in the field of tumor immunotherapy. By advancing the development of disease-specific cancer vaccines, we can harness the full potential of this approach to significantly improve treatment outcomes for cancer patients. Rigorous evaluation and analysis are essential to ensure the clinical relevance and applicability of these vaccines, ultimately leading to improved patient outcomes on a global scale.
Author contributions
JW: Software, Visualization, Writing – original draft, Writing – review & editing. JM: Investigation, Supervision, Writing – review & editing. FX: Supervision, Writing – review & editing. FM: Supervision, Writing – review & editing. LL: Supervision, Writing – review & editing. YH: Investigation, Writing – review & editing. XZ: Supervision, Writing – review & editing. JY: Supervision, Writing – review & editing. ZT: Supervision, Writing – review & editing. QZ: Funding acquisition, Writing – review & editing. LB: Funding acquisition, Supervision, Writing – review & editing.
Funding
The author(s) declare financial support was received for the research, authorship, and/or publication of this article. This review was financially supported by the National Natural Science Foundation of China (No. 82073385, 82172706 and 82003295), Natural Science Foundation of Shanghai (No. 23ZR1478100).
Conflict of interest
The authors declare that the research was conducted in the absence of any commercial or financial relationships that could be construed as a potential conflict of interest.
Publisher’s note
All claims expressed in this article are solely those of the authors and do not necessarily represent those of their affiliated organizations, or those of the publisher, the editors and the reviewers. Any product that may be evaluated in this article, or claim that may be made by its manufacturer, is not guaranteed or endorsed by the publisher.
Glossary
References
1. Sung H, Ferlay J, Siegel RL, Laversanne M, Soerjomataram I, Jemal A, et al. Global cancer statistics 2020: GLOBOCAN estimates of incidence and mortality worldwide for 36 cancers in 185 countries. CA Cancer J Clin May. (2021) 71:209–49. doi: 10.3322/caac.21660
2. Villadangos JA, Schnorrer P. Intrinsic and cooperative antigen-presenting functions of dendritic-cell subsets in vivo. Nat Rev Immunol Jul. (2007) 7:543–55. doi: 10.1038/nri2103
3. Ribas A, Wolchok JD. Cancer immunotherapy using checkpoint blockade. Science. Mar 23. (2018) 359:1350–5. doi: 10.1126/science.aar4060
4. Topalian SL, Forde PM, Emens LA, Yarchoan M, Smith KN, Pardoll DM. Neoadjuvant immune checkpoint blockade: A window of opportunity to advance cancer immunotherapy. Cancer Cell. (2023) 41:1551–66. doi: 10.1016/j.ccell.2023.07.011
5. Brahmer JR, Tykodi SS, Chow LQ, Hwu WJ, Topalian SL, Hwu P, et al. Safety and activity of anti-PD-L1 antibody in patients with advanced cancer. N Engl J Med. (2012) 366:2455–65. doi: 10.1056/NEJMoa1200694
6. Chen KS, Reinshagen C, Van Schaik TA, Rossignoli F, Borges P, Mendonca NC, et al. Bifunctional cancer cell-based vaccine concomitantly drives direct tumor killing and antitumor immunity. Sci Transl Med. (2023) 15:eabo4778. doi: 10.1126/scitranslmed.abo4778
7. Tanyi JL, Bobisse S, Ophir E, Tuyaerts S, Roberti A, Genolet R, et al. Personalized cancer vaccine effectively mobilizes antitumor T cell immunity in ovarian cancer. Sci Transl Med. (2018) 10:436. doi: 10.1126/scitranslmed.aao5931
8. Wang T, Wang D, Yu H, Feng B, Zhou F, Zhang H, et al. A cancer vaccine-mediated postoperative immunotherapy for recurrent and metastatic tumors. Nat Commun. (2018) 9:1532. doi: 10.1038/s41467-018-03915-4
9. Kroemer G, Galassi C, Zitvogel L, Galluzzi L. Immunogenic cell stress and death. Nat Immunol. (2022) 23:487–500. doi: 10.1038/s41590-022-01132-2
10. Choi B, Choi H, Yu B, Kim DH. Synergistic local combination of radiation and anti-programmed death ligand 1 immunotherapy using radiation-responsive splintery metallic nanocarriers. ACS Nano. (2020) 14:13115–26. doi: 10.1021/acsnano.0c04701
11. Obeid M, Tesniere A, Ghiringhelli F, Fimia GM, Apetoh L, Perfettini JL, et al. Calreticulin exposure dictates the immunogenicity of cancer cell death. Nat Med. (2007) 13:54–61. doi: 10.1038/nm1523
12. Huang Z, Wang Y, Yao D, Wu J, Hu Y, Yuan A. Nanoscale coordination polymers induce immunogenic cell death by amplifying radiation therapy mediated oxidative stress. Nat Commun. (2021) 12:145. doi: 10.1038/s41467-020-20243-8
13. Apetoh L, Ghiringhelli F, Tesniere A, Criollo A, Ortiz C, Lidereau R, et al. The interaction between HMGB1 and TLR4 dictates the outcome of anticancer chemotherapy and radiotherapy. Immunol Rev. (2007) 220:47–59. doi: 10.1111/j.1600-065X.2007.00573.x
14. Ghiringhelli F, Apetoh L, Tesniere A, Aymeric L, Ma Y, Ortiz C, et al. Activation of the NLRP3 inflammasome in dendritic cells induces IL-1beta-dependent adaptive immunity against tumors. Nat Med. (2009) 15:1170–8. doi: 10.1038/nm.2028
15. Garg AD, Vandenberk L, Fang S, Fasche T, Van Eygen S, Maes J, et al. Pathogen response-like recruitment and activation of neutrophils by sterile immunogenic dying cells drives neutrophil-mediated residual cell killing. Cell Death Differ. (2017) 24:832–43. doi: 10.1038/cdd.2017.15
16. Galluzzi L, Vitale I, Aaronson SA, Abrams JM, Adam D, Agostinis P, et al. Molecular mechanisms of cell death: recommendations of the Nomenclature Committee on Cell Death 2018. Cell Death Differ. (2018) 25:486–541. doi: 10.1038/s41418-017-0012-4
17. Wang Q, Ju X, Wang J, Fan Y, Ren M, Zhang H. Immunogenic cell death in anticancer chemotherapy and its impact on clinical studies. Cancer Lett. (2018) 438:17–23. doi: 10.1016/j.canlet.2018.08.028
18. Panaretakis T, Kepp O, Brockmeier U, Tesniere A, Bjorklund A, Chapman DC, et al. Mechanisms of pre-apoptotic calreticulin exposure in immunogenic cell death. EMBO J. (2009) 28:578–90. doi: 10.1038/emboj.2009.1
19. Martins I, Wang Y, Michaud M, Ma Y, Sukkurwala AQ, Shen S, et al. Molecular mechanisms of ATP secretion during immunogenic cell death. Cell Death Differ. (2014) 21:79–91. doi: 10.1038/cdd.2013.75
20. Apetoh L, Ghiringhelli F, Tesniere A, Obeid M, Ortiz C, Criollo A, et al. Toll-like receptor 4-dependent contribution of the immune system to anticancer chemotherapy and radiotherapy. Nat Med. (2007) 13:1050–9. doi: 10.1038/nm1622
21. Ahmed A, Tait SWG. Targeting immunogenic cell death in cancer. Mol Oncol. (2020) 14:2994–3006. doi: 10.1002/1878-0261.12851
22. Irvine DJ, Hanson MC, Rakhra K, Tokatlian T. Synthetic nanoparticles for vaccines and immunotherapy. Chem Rev. (2015) 115:11109–46. doi: 10.1021/acs.chemrev.5b00109
23. Banstola A, Pandit M, Duwa R, Chang JH, Jeong JH, Yook S. Reactive oxygen species-responsive dual-targeted nanosystem promoted immunogenic cell death against breast cancer. Bioeng Transl Med. (2023) 8:e10379. doi: 10.1002/btm2.10379
24. Liu M, Hao L, Zhao D, Li J, Lin Y. Self-assembled immunostimulatory tetrahedral framework nucleic acid vehicles for tumor chemo-immunotherapy. ACS Appl Mater Interfaces. (2022) 14:38506–14. doi: 10.1021/acsami.2c09462
25. Bains SJ, Abrahamsson H, Flatmark K, Dueland S, Hole KH, Seierstad T, et al. Immunogenic cell death by neoadjuvant oxaliplatin and radiation protects against metastatic failure in high-risk rectal cancer. Cancer Immunol Immunother. (2020) 69:355–64. doi: 10.1007/s00262-019-02458-x
26. Kim NR, Kim YJ. Oxaliplatin regulates myeloid-derived suppressor cell-mediated immunosuppression via downregulation of nuclear factor-kappaB signaling. Cancer Med. (2019) 8:276–88. doi: 10.1002/cam4.1878
27. Tang X, Rao J, Yin S. PD-L1 knockdown via hybrid micelle promotes paclitaxel induced Cancer-Immunity Cycle for melanoma treatment. Eur J Pharm Sci. (2019) 127:161–74. doi: 10.1016/j.ejps.2018.10.021
28. Vanmeerbeek I, Sprooten J, De Ruysscher D. Trial watch: chemotherapy-induced immunogenic cell death in immuno-oncology. Oncoimmunology. (2020) 9:1703449. doi: 10.1080/2162402X.2019.1703449
29. Guo J, Yu Z, Sun D, Zou Y, Liu Y, Huang L. Two nanoformulations induce reactive oxygen species and immunogenetic cell death for synergistic chemo-immunotherapy eradicating colorectal cancer and hepatocellular carcinoma. Mol Cancer. (2021) 20:10. doi: 10.1186/s12943-020-01297-0
30. Zhang F, Chen F, Yang C. Coordination and redox dual-responsive mesoporous organosilica nanoparticles amplify immunogenic cell death for cancer chemoimmunotherapy. Small. (2021) 17:e2100006. doi: 10.1002/smll.202100006
31. Qiu W, Liang M, Gao Y. Polyamino acid calcified nanohybrids induce immunogenic cell death for augmented chemotherapy and chemo-photodynamic synergistic therapy. Theranostics. (2021) 11:9652–66. doi: 10.7150/thno.64354
32. Ding D, Zhong H, Liang R. Multifunctional nanodrug mediates synergistic photodynamic therapy and MDSCs-targeting immunotherapy of colon cancer. Adv Sci (Weinh). (2021) 8:e2100712. doi: 10.1002/advs.202100712
33. Garg AD, Dudek AM, Ferreira GB. ROS-induced autophagy in cancer cells assists in evasion from determinants of immunogenic cell death. Autophagy. (2013) 9:1292–307. doi: 10.4161/auto.25399
34. Sun Z, Zhao M, Wang W. 5-ALA mediated photodynamic therapy with combined treatment improves anti-tumor efficacy of immunotherapy through boosting immunogenic cell death. Cancer Lett. (2023) 554:216032. doi: 10.1016/j.canlet.2022.216032
35. Ji J, Fan Z, Zhou F. Improvement of DC vaccine with ALA-PDT induced immunogenic apoptotic cells for skin squamous cell carcinoma. Oncotarget. (2015) 6:17135–46. doi: 10.18632/oncotarget.3529
36. Jin F, Qi J, Liu D, You Y, Shu G, Du Y, et al. Cancer-cell-biomimetic Upconversion nanoparticles combining chemo-photodynamic therapy and CD73 blockade for metastatic triple-negative breast cancer. J Control Release (2021) 337:90–104. doi: 10.1016/j.jconrel.2021.07.021
37. Li W, Yang J, Luo L. Targeting photodynamic and photothermal therapy to the endoplasmic reticulum enhances immunogenic cancer cell death. Nat Commun. (2019) 10:3349. doi: 10.1038/s41467-019-11269-8
38. Xu N, Hu A, Pu X. Cu-Chelated polydopamine nanoparticles as a photothermal medium and "immunogenic cell death" inducer for combined tumor therapy. J Mater Chem B. (2022) 10:3104–18. doi: 10.1039/D2TB00025C
39. Tian Y, Younis MR, Tang Y. Dye-loaded mesoporous polydopamine nanoparticles for multimodal tumor theranostics with enhanced immunogenic cell death. J Nanobiotechnology. (2021) 19:365. doi: 10.1186/s12951-021-01109-7
40. Feng X, Lin T, Chen D. Mitochondria-associated ER stress evokes immunogenic cell death through the ROS-PERK-eIF2alpha pathway under PTT/CDT combined therapy. Acta Biomater. (2023) 160:211–24. doi: 10.1016/j.actbio.2023.02.011
41. Zhang Y, Guo C, Liu L. ZnO-based multifunctional nanocomposites to inhibit progression and metastasis of melanoma by eliciting antitumor immunity via immunogenic cell death. Theranostics. (2020) 10:11197–214. doi: 10.7150/thno.44920
42. Ren J, Zhou J, Liu H. Ultrasound (US)-activated redox dyshomeostasis therapy reinforced by immunogenic cell death (ICD) through a mitochondrial targeting liposomal nanosystem. Theranostics. (2021) 11:9470–91. doi: 10.7150/thno.62984
43. Zheng J, Sun Y, Long T. Sonosensitizer nanoplatform-mediated sonodynamic therapy induced immunogenic cell death and tumor immune microenvironment variation. Drug Deliv. Dec. (2022) 29:1164–75. doi: 10.1080/10717544.2022.2058653
44. Zheng J, Sun J, Chen J. Oxygen and oxaliplatin-loaded nanoparticles combined with photo-sonodynamic inducing enhanced immunogenic cell death in syngeneic mouse models of ovarian cancer. J Control Release. (2021) 332:448–59. doi: 10.1016/j.jconrel.2021.02.032
45. Dai Z, Tang J, Gu Z. Eliciting immunogenic cell death via a unitized nanoinducer. Nano Lett. (2020) 20:6246–54. doi: 10.1021/acs.nanolett.0c00713
46. Xiao Y, Yao W, Lin M. Icaritin-loaded PLGA nanoparticles activate immunogenic cell death and facilitate tumor recruitment in mice with gastric cancer. Drug Deliv. (2022) 29:1712–25. doi: 10.1080/10717544.2022.2079769
47. Song Q, Yin Y, Shang L. Tumor microenvironment responsive nanogel for the combinatorial antitumor effect of chemotherapy and immunotherapy. Nano Lett. (2017) 17:6366–75. doi: 10.1021/acs.nanolett.7b03186
48. Jiang M, Zeng J, Zhao L. Chemotherapeutic drug-induced immunogenic cell death for nanomedicine-based cancer chemo-immunotherapy. Nanoscale. (2021) 13:17218–35. doi: 10.1039/D1NR05512G
49. Lu J, Liu X, Liao YP. Nano-enabled pancreas cancer immunotherapy using immunogenic cell death and reversing immunosuppression. Nat Commun. (2017) 8:1811. doi: 10.1038/s41467-017-01651-9
50. Zhou F, Feng B, Yu H. Tumor microenvironment-activatable prodrug vesicles for nanoenabled cancer chemoimmunotherapy combining immunogenic cell death induction and CD47 blockade. Adv Mater. (2019) 31:e1805888. doi: 10.1002/adma.201805888
51. Xie L, Wang G, Sang W. Phenolic immunogenic cell death nanoinducer for sensitizing tumor to PD-1 checkpoint blockade immunotherapy. Biomaterials. (2021) 269:120638. doi: 10.1016/j.biomaterials.2020.120638
52. Jeon J, Yoon B, Dey A. Self-immolative polymer-based immunogenic cell death inducer for regulation of redox homeostasis. Biomaterials. (2023) 295:122064. doi: 10.1016/j.biomaterials.2023.122064
53. Garg AD, Krysko DV, Vandenabeele P, Agostinis P. The emergence of phox-ER stress induced immunogenic apoptosis. Oncoimmunology. (2012) 1:786–8. doi: 10.4161/onci.19750
54. Deng H, Zhou Z, Yang W. Endoplasmic reticulum targeting to amplify immunogenic cell death for cancer immunotherapy. Nano Lett. (2020) 20:1928–33. doi: 10.1021/acs.nanolett.9b05210
55. Huang C, Lin B, Chen C. Synergistic reinforcing of immunogenic cell death and transforming tumor-associated macrophages via a multifunctional cascade bioreactor for optimizing cancer immunotherapy. Adv Mater Dec. (2022) 34:e2207593. doi: 10.1002/adma.202207593
56. Liu J, He S, Luo Y. Tumor-microenvironment-activatable polymer nano-immunomodulator for precision cancer photoimmunotherapy. Adv Mater. (2022) 34:e2106654. doi: 10.1002/adma.202106654
57. Chen H, Luo X, Huang Q. Platelet membrane fusion liposome loaded with type I AIE photosensitizer to induce chemoresistance cancer pyroptosis and immunogenic cell death for enhancing cancer immunotherapy. Chem Eng J. (2023) 476:146276. doi: 10.1016/j.cej.2023.146276
58. Ning S, Lyu M, Zhu D. Type-I AIE photosensitizer loaded biomimetic system boosting cuproptosis to inhibit breast cancer metastasis and rechallenge. ACS Nano. (2023) 17:10206–17. doi: 10.1021/acsnano.3c00326
59. Guo R, Wang S, Zhao L. Engineered nanomaterials for synergistic photo-immunotherapy. Biomaterials. (2022) 282:121425. doi: 10.1016/j.biomaterials.2022.121425
60. Zhu D, Chen H, Huang C. H2O2 self-producing single-atom nanozyme hydrogels as light-controlled oxidative stress amplifier for enhanced synergistic therapy by transforming “Cold” Tumors. Advanced Funct Materials. (2022) 32:2110268. doi: 10.1002/adfm.202110268
61. Wang X, Zhong X, Lei H. Hollow cu2Se nanozymes for tumor photothermal-catalytic therapy. Chem Materials. (2019) 31:6174–86. doi: 10.1021/acs.chemmater.9b01958
62. Zhou J, Lei M, Peng XL, Wei DX, Yan LK. Fenton reaction induced by fe-based nanoparticles for tumor therapy. J BioMed Nanotechnol. (2021) 17:1510–24. doi: 10.1166/jbn.2021.3130
63. Beaudelot J, Oger S, Perusko S. Photoactive copper complexes: properties and applications. Chem Rev. (2022) 122:16365–609. doi: 10.1021/acs.chemrev.2c00033
64. Ran J, Liu T, Song C, et al. Rhythm mild-temperature photothermal therapy enhancing immunogenic cell death response in oral squamous cell carcinoma. Adv Healthc Mater. (2023) 12:e2202360. doi: 10.1002/adhm.202202360
65. Canavese G, Ancona A, Racca L, Canta M, Dumontel B, Barbaresco F, et al. Nanoparticle-assisted ultrasound: A special focus on sonodynamic therapy against cancer. Chem Eng J. (2018) 340:155–72. doi: 10.1016/j.cej.2018.01.060
66. Bilmin K, Kujawska T, Grieb P. Sonodynamic therapy for gliomas. Perspectives and prospects of selective sonosensitization of glioma cells. Cells. (2019) 8:11. doi: 10.3390/cells8111428
67. Sethuraman SN, Singh MP, Patil G, et al. Novel calreticulin-nanoparticle in combination with focused ultrasound induces immunogenic cell death in melanoma to enhance antitumor immunity. Theranostics. (2020) 10:3397–412. doi: 10.7150/thno.42243
68. Sethuraman SN, Singh MP, Patil G, Li S, Fiering S, Hoopes PJ, et al. Phase-transformation nanoparticle-mediated sonodynamic therapy: an effective modality to enhance anti-tumor immune response by inducing immunogenic cell death in breast cancer. Int J Nanomedicine. (2021) 16:1913–26. doi: 10.2147/IJN.S297933
69. Galluzzi L, Buque A, Kepp O, Zitvogel L, Kroemer G. Immunogenic cell death in cancer and infectious disease. Nat Rev Immunol. (2017) 17:97–111. doi: 10.1038/nri.2016.107
70. Schaue D, Ratikan JA, Iwamoto KS, McBride WH. Maximizing tumor immunity with fractionated radiation. Int J Radiat Oncol Biol Phys. (2012) 83:1306–10. doi: 10.1016/j.ijrobp.2011.09.049
71. Rodriguez-Ruiz ME, Vitale I, Harrington KJ, Melero I, Galluzzi L. Immunological impact of cell death signaling driven by radiation on the tumor microenvironment. Nat Immunol. (2020) 21:120–34. doi: 10.1038/s41590-019-0561-4
72. Sharabi AB, Lim M, DeWeese TL, Drake CG. Radiation and checkpoint blockade immunotherapy: radiosensitisation and potential mechanisms of synergy. Lancet Oncol. (2015) 16:e498–509. doi: 10.1016/S1470-2045(15)00007-8
73. Demaria S, Formenti SC. Radiation as an immunological adjuvant: current evidence on dose and fractionation. Front Oncol. (2012) 2:153. doi: 10.3389/fonc.2012.00153
74. He C, Ding H, Li L, Chen J, Mo X, Ding Y, et al. Gold nanoparticles enhance the ability of radiotherapy to induce immunogenic cell death in glioblastoma. Int J Nanomedicine. (2023) 18:5701–12. doi: 10.2147/IJN.S419712
75. Fan Y, Kuai R, Xu Y, Ochyl LJ, Irvine DJ, Moon JJ. Immunogenic cell death amplified by co-localized adjuvant delivery for cancer immunotherapy. Nano Lett. (2017) 17:7387–93. doi: 10.1021/acs.nanolett.7b03218
76. Qin X, Yang T, Xu H, Zhang R, Zhao S, Kong L, et al. Dying tumor cells-inspired vaccine for boosting humoral and cellular immunity against cancer. J Control Release. (2023) 359:359–72. doi: 10.1016/j.jconrel.2023.05.044
77. omrat Li R, Hao Y, Roche K, Chen G, Pan W, Wang AZ, et al. Chemotherapy-induced nanovaccines implement immunogenicity equivalence for improving cancer chemoimmunotherapy. Biomaterials. (2023) 301:122290. doi: 10.1016/j.biomaterials.2023.122290
78. Min Y, Roche KC, Tian S, Eblan MJ, McKinnon KP, Caster JM, et al. Antigen-capturing nanoparticles improve the abscopal effect and cancer immunotherapy. Nat Nanotechnol. (2017) 12:877–82. doi: 10.1038/nnano.2017.113
79. Chattopadhyay S, Liu YH, Fang ZS, Lin CL, Lin JC, Yao BY, et al. Synthetic immunogenic cell death mediated by intracellular delivery of STING agonist nanoshells enhances anticancer chemo-immunotherapy. Nano Lett. (2020) 20:2246–56. doi: 10.1021/acs.nanolett.9b04094
80. Huang X, Xiong M, Jin Y, Deng C, Xu H, An C, et al. Evidence that high-migration drug-surviving MOLT4 leukemia cells exhibit cancer stem cell-like properties. Int J Oncol. (2016) 49:343–51. doi: 10.3892/ijo.2016.3526
81. Gupta SK, Lysko PG, Pillarisetti K, Ohlstein E, Stadel JM. Chemokine receptors in human endothelial cells. Functional expression of CXCR4 and its transcriptional regulation by inflammatory cytokines. J Biol Chem. (1998) 273:4282–7. doi: 10.1074/jbc.273.7.4282
Keywords: immunogenic cell death, cancer vaccine, dying tumor cells, immunotherapy, nanoinducers
Citation: Wang J, Ma J, Xie F, Miao F, lv L, Huang Y, Zhang X, Yu J, Tai Z, Zhu Q and Bao L (2024) Immunogenic cell death-based cancer vaccines: promising prospect in cancer therapy. Front. Immunol. 15:1389173. doi: 10.3389/fimmu.2024.1389173
Received: 21 February 2024; Accepted: 10 April 2024;
Published: 29 April 2024.
Edited by:
Shensi Shen, Sichuan University, ChinaReviewed by:
Daoming Zhu, Southern Medical University, ChinaJoanna Rossowska, Polish Academy of Sciences, Poland
Copyright © 2024 Wang, Ma, Xie, Miao, lv, Huang, Zhang, Yu, Tai, Zhu and Bao. This is an open-access article distributed under the terms of the Creative Commons Attribution License (CC BY). The use, distribution or reproduction in other forums is permitted, provided the original author(s) and the copyright owner(s) are credited and that the original publication in this journal is cited, in accordance with accepted academic practice. No use, distribution or reproduction is permitted which does not comply with these terms.
*Correspondence: Leilei Bao, YW5uYWJhbzIxMkAxMjYuY29t; Quangang Zhu, emh1cWdAc2hza2luLmNvbQ==; Zongguang Tai, dGFpem9uZ2d1YW5nQDEyNi5jb20=
†These authors have contributed equally to this work