- College of Animal Science and Technology, Qingdao Agricultural University, Qingdao, China
Introduction: The prevention and mitigation of intestinal immune challenge is crucial for poultry production. This study investigated the effects of dietary Macleaya cordata extract (MCE) supplementation on the prevention of intestinal injury in broiler chickens challenged with lipopolysaccharide (LPS).
Methods: A total of 256 one-day-old male Arbor Acres broilers were randomly divided into 4 treatment groups using a 2×2 factorial design with 2 MCE supplemental levels (0 and 400 mg/kg) and 2 LPS challenge levels (0 and 1 mg/kg body weight). The experiment lasted for 21 d.
Results and discussion: The results showed that MCE supplementation increased the average daily feed intake during days 0-14. MCE supplementation and LPS challenge have an interaction on the average daily gain during days 15-21. MCE supplementation significantly alleviated the decreased average daily gain of broiler chickens induced by LPS. MCE supplementation increased the total antioxidant capacity and the activity of catalase and reduced the level of malondialdehyde in jejunal mucosa. MCE addition elevated the villus height and the ratio of villus height to crypt depth of the ileum. MCE supplementation decreased the mRNA expression of pro-inflammatory cytokines interleukin (IL)-6 and IL-8 in the jejunum. MCE addition mitigated LPS-induced mRNA up-expression of pro-inflammatory factors IL-1β and IL-17 in the jejunum. MCE supplementation increased the abundance of probiotic bacteria (such as Lactobacillus and Blautia) and reduced the abundance of pathogenic bacteria (such as Actinobacteriota, Peptostretococcaceae, and Rhodococcus), leading to alterations in gut microbiota composition. MCE addition altered several metabolic pathways such as Amino acid metabolism, Nucleotide metabolism, Energy metabolism, Carbohydrate metabolism, and Lipid metabolism in broilers. In these pathways, MCE supplementation increased the levels of L-aspartic acid, L-Glutamate, L-serine, etc., and reduced the levels of phosphatidylcholine, phosphatidylethanolamine, thromboxane B2, 13-(S)-HODPE, etc. In conclusion, dietary supplementation of 400 mg/kg MCE effectively improved the growth performance and intestinal function in LPS-challenged broiler chickens, probably due to the modulation of gut microbiota and plasma metabolites.
Introduction
The intestine is a key site for nutrient absorption and immune response in broiler chickens. However, many factors such as pathogens, mycotoxins and heat stress can damage the intestines of broiler chickens. Lipopolysaccharide (LPS), a structural part of the outer membrane of Gram-negative bacteria, often results in intestinal immune challenge, thereby compromising intestinal integrity, nutrient transport and utilization (1, 2). LPS is recognized by the LPS binding protein, which launches an intercellular cascade response, eventually leading to the release of pro-inflammatory cytokines. This process induces intestinal injury in animals (1, 3) and immune stress in cells (4). LPS is often used to establish the model of intestinal injury in various animals, such as mice (5), chickens (6) and pigs (1). Previous studies have reported that the negative effects induced by LPS in broilers can be mitigated by nutritional interventions, such as probiotics (7), polysaccharides (8), and natural plant extracts (9).
Macleaya cordata, a medicinal plant belonging to the Papaveraceae family, is traditional Chinese medicine and has been widely used as a natural feed additive in animal husbandry in China, Europe, and North America (10, 11). Numerous studies have shown that Macleaya cordata extract (MCE) decreases inflammation, increases antioxidant capacity, and improves intestinal health, thereby increasing the growth performance of livestock and poultry (10, 12, 13). The main biologically active components of MCE are sanguinarine and chelerythrine, both of which belong to the quaternary benzo[c]phen-anthridine (14). Sanguinarine and chelerythrine are reported to have physiological effects, including anti-inflammatory, anti-oxidative, antimicrobial and antiviral properties (15, 16). It has been reported that MCE increases the body weight gain, average daily gain (ADG), and average daily feed intake (ADFI) and decreases the feed-to-gain ratio (F/G) in broiler chickens (10, 12, 17). In addition, MCE enhances the antioxidant function of mice challenged with enterotoxigenic Escherichia coli, as indicated by elevated activities of superoxide dismutase (SOD), catalase (CAT), and glutathione peroxidase (GSH-Px), and decreased levels of malondialdehyde (MDA) (18). Moreover, supplementation of MCE increases the villus height and the villus height to crypt height ratio (VCR) and decreases the crypt depth in the jejunum of broiler chickens with necrotic enteritis (19). MCE supplementation regulates serum immunological indices, such as decreasing the serum TNF-α and IL-6 levels of laying hens (20), and increasing the serum IL-10, TGF-β and IgG levels of neonatal piglets (21). However, it is unclear whether MCE can relieve the detrimental effects of LPS on the intestinal function of broiler chickens.
The gastrointestinal tract is not only the largest immunological organ but also the primary site of digestion and absorption for animals (22). The microbes in the gut can be influenced by pathogens, inflammatory cytokines, diet, exercise, gastrointestinal peptides, and so on (23). There is a dynamic balance in the gut microbiota, and when the balance is broken, it will lead to dysbiosis, which can influence the host immunity, the development of the gastrointestinal tract, and the health status of animals (24, 25). It has been reported that MCE changes the composition of gut microbiota, increases the abundance of Lactobacillus, and decreases the abundance of Salmonella and Corynebacterium (26, 27). At the same time, MCE can change the metabolic pathways of amino acids, vitamins and lipids, and increase the contents of butyrate and secondary cholic acid, thereby improving the growth performance and health status of broiler chickens (27). However, it remains unknown how MCE affects gut microbes and plasma metabolites in broilers challenged with LPS.
Therefore, the purpose of this study was conducted to investigate the effects of dietary MCE supplementation on growth performance, intestinal antioxidant function, intestinal morphology, and inflammation in broiler chickens challenged with LPS, and further to detect gut microbiota and plasma metabolites to reveal the underlying mechanism. Our study will be of significance for a more comprehensive understanding of the anti-immune stress mechanism of MCE in broiler chickens.
Materials and methods
Experimental design and animals
All experimental procedures were approved by the Animal Care and Use Committee of Qingdao Agricultural University. A total of 256 one-day-old male Arbor Acres broilers were obtained from a local commercial hatchery. The broilers were weighed and randomly assigned into 4 groups, including the CON group, MCE group, LPS group, and MCE+LPS group. Each group consisted of 8 replicates, with 8 broiler chickens per replicate. There was no significant difference in initial body weights among the groups. Chickens in the MCE and MCE+LPS groups were fed diets supplemented with MCE (400 mg/kg) from day 0 to day 21. Chickens in the LPS and MCE+LPS groups were intraperitoneally injected with LPS (1 mg/kg body weight) on days 15, 17, 19, and 21. Chickens in the CON and MCE groups were intraperitoneally injected with an equal volume of sterile saline. MCE was provided by Hunan Micolta Biological Resources Co., Ltd. The contents of two active ingredients, sanguinarine and chelerythrine, are 1.5% and 0.075%, respectively. LPS was purchased from Sigma (L-2880, E. coli serotype O55:B5). The broilers were reared in wire-floored cages under a 23-h photoperiod and had free access to feed and water. The room temperature was 34°C for the first week and then reduced by 2°C each week. The diet composition and nutritional levels are listed in Table 1.
Sample collection
Chickens (one bird per replicate) with average body weight were randomly selected and slaughtered at 3 h after LPS injection on day 21. Blood samples were collected using sterile blood collection tubes containing sodium heparin anticoagulant before slaughtering. Heparin plasma was collected after centrifugation at 3000 rpm for 10 min at 4°C, aliquoted, and stored at -20°C until analysis. After blood collection, the broilers were sacrificed by exsanguination. The ileum was collected and fixed in 4% paraformaldehyde for intestinal morphology analysis. The jejunum was collected, immediately frozen in liquid nitrogen, and stored at -80°C for gene expression analysis. Jejunal mucosa was scraped by glass slides, rapidly frozen in liquid nitrogen, and stored at -20°C for antioxidant assays.
Growth performance
Body weight and feed consumption for each replicate cage were recorded on days 14 and 21. ADG, ADFI and F/G during days 0-14 and days 15-21 were calculated.
Antioxidant capacity
The activities of CAT (A007-1-1), GSH-Px (A005-1-2) and SOD (A001-1-1) and the levels of MDA (A003-1-1) and T-AOC (A015-1-2) in intestinal mucosa were determined using commercial kits according to the manufacturer’s protocols (Nanjing jiancheng Bioengineering Institute, Nanjing, China). Mucosal homogenates (10%) were prepared by homogenizing intestinal mucosa in ice-cold sterile saline. The homogenates were centrifuged at 3000 rpm for 10 min at 4°C, and the supernatants were then collected for further assays. The protein contents in the supernatants were quantified using a Bicinchoninic acid (BCA) protein assay kit (Cwbio, Beijing, China). Antioxidant indices in intestinal mucosa were expressed as units per milligram of protein in the sample.
Intestinal morphology
The intestinal tissues were dehydrated stepwise with ethanol, embedded in paraffin, and sectioned (5 μm). The tissue slices were stained with hematoxylin and eosin and sealed with gum. Images were captured using a light microscope (DM2000 LED, Leica, Germany) and analyzed using ZEISS ZEN 2011 (Blue version). Villus height was determined from the tip of the villus to the villus-crypt junction, and crypt depth was defined as the depth of the invagination between adjacent villi. and the VCR was calculated by dividing the villus height by the crypt depth. For each section, ten pairs of villi and crypts were observed, and the average was calculated as the final value.
Real-time quantitative PCR
Total RNA was extracted from the jejunum using Trizol reagent (Invitrogen, Carlsbad, CA). The purity and concentration of total RNA were determined with a nanophotometer (Nano Photometer NP80, Implen, Germany). Reverse transcription into cDNA was performed using a PrimeScript RT reagent kit with gDNA Eraser (Takara, Osaka, Japan). Quantitative real-time PCR was performed using TB Green® Premix Ex TaqTM (Takara, Osaka, Japan) on a CFX96 Real-Time PCR Detection System (Bio-rad, USA). The reaction mixture (25 μL) contained 12.5 μL of TB Green Premix Ex Taq (Tli RNaseH Plus) (2X), 0.5 μL of forward and reverse primers (10 μmol/L), 2 μL of cDNA, and 9.5 μL of sterile nuclease-free water. The PCR cycling conditions were as follows: 95°C for 30 s, followed by 40 cycles of 95°C for 5 s and 60°C for 30 s. The specificity of the PCR products was assessed using a melting curve. The primer sequences for the target and reference genes are listed in Table 2. The relative mRNA expression levels of target genes were calculated using the 2–ΔΔCt method, and the housekeeping gene GAPDH was used as an internal control.
Gut microbiome
DNA extraction and high-throughput sequencing
Total microbial genomic DNA was extracted from ileal digesta using the E.Z.N.A.® soil DNA Kit (Omega Bio-tek, Norcross, GA, U.S.) according to the manufacturer’s instructions. The quality and concentration of DNA were determined by 1.0% agarose gel electrophoresis and a NanoDrop® ND-2000 spectrophotometer (Thermo Fisher Scientific, MA, USA). The hypervariable region V3-V4 of the bacterial 16S rRNA gene was amplified with primer pairs 338F (5’-ACTCCTACGGGAGGCAGCAG-3’) and 806R (5’-GGACTACHVGGGTWTCTAAT-3’) on an ABI GeneAmp® 9700 PCR thermocycler (ABI, CA, USA). PCR amplification was performed using TransStart® FastPfu DNA Polymerase kits (TransGen AP221-02) under the cycling conditions: 95°C for 3 min, followed by 27 cycles of 95°C for 30 s, 55°C for 30 s and 72°C for 45 s, and a final extension at 72°C for 10 min. All samples were amplified in triplicate. The PCR product was extracted from 2% agarose gel, purified using an AxyPrep DNA Gel Extraction kit (Axygen Biosciences, Union City, CA, USA) according to the manufacturer’s instructions, and quantified using the Quantus™ Fluorometer (Promega, USA). Purified amplicons were pooled in equimolar amounts and paired-end sequenced on an Illumina MiSeq PE300 platform (Illumina, San Diego, USA).
Sequence Processing and Bioinformatics Analysis
Raw FASTQ files were de-multiplexed using an in-house perl script, quality-filtered using fastp version 0.19.6, and merged using FLASH version 1.2.7. The optimized sequences were clustered into operational taxonomic units (OTUs) using UPARSE 7.1 at a 97% sequence similarity level. The taxonomy of each OTU representative sequence was analyzed using RDP Classifier version 2.2 against the 16S rRNA gene database (Silva v138) at a confidence threshold of 0.7. The α-diversity was analyzed using Mothur (1.30.2). The β-diversity and community barplot were analyzed using Qiime (1.9.1). The microbiota with linear discriminant analysis (LDA) scores > 2 were identified using the linear discriminant analysis effect size (LEfSe) method.
Detection of plasma metabolite composition by non-targeted metabolomics
To extract metabolites, 100 μL of plasma sample was mixed with 400 μL of extraction solution (acetonitrile: methanol = 1: 1, v/v) containing 0.02 mg/mL L-2-chlorophenylalanine as an internal standard, followed by vortexing for 30 s and sonication (40 kHz) for 30 min at 4°C. The mixture was incubated at -20°C for 30 min to precipitate the proteins. The mixture was centrifuged for 15 min (4°C, 13000 g). The supernatant was collected and blown dry under nitrogen. The samples were then re-dissolved in 100 µL of aqueous acetonitrile (acetonitrile: water = 1: 1, v/v) and sonicated (40 kHz)) for 5 min at 5°C, followed by centrifugation (13000 g, 4°C) for 10 min. The supernatant was collected and subjected to LC-MS/MS analysis. Quality control (QC) samples were analyzed at regular intervals (every 12 samples).
The LC-MS/MS analysis was conducted on a Thermo UHPLC-Q Exactive HF-X system equipped with an ACQUITY HSS T3 column (100 mm × 2.1 mm, i.d., 1.8 μm; Waters, USA) at Majorbio Bio-Pharm Technology Co. Ltd. (Shanghai, China). The mobile phases consisted of 0.1% formic acid in Solvent A (water: acetonitrile = 95: 5, v/v) and 0.1% formic acid in Solvent B (acetonitrile: isopropanol: water = 47.5: 47.5: 5, v/v/v). The flow rate was 0.40 mL/min, and the column temperature was 40°C. The injection volume was 3 μL. The LC-MS/MS data were acquired using a Thermo UHPLC-Q Exactive HF-X Mass Spectrometer equipped with an electrospray ionization (ESI) source operated in both positive and negative ion modes. The optimal conditions were set as follows: source temperature at 425°C; sheath gas flow rate at 50 arb; Aux gas flow rate at 13 arb; ion-spray voltage floating (ISVF) at -3 500 V in the negative ion mode and 3 500 V in the positive ion mode, respectively; Normalized collision energy, 20-40-60 V rolling for MS/MS. Full MS resolution was 60000, and MS/MS resolution was 7500. Data acquisition was performed in the Data-Dependent Acquisition mode. The detection was carried out over a mass range of 70-1 050 m/z.
LC/MS raw data were preprocessed using Progenesis QI (Waters Corporation, Milford, USA) and analyzed on the Majorbio cloud platform (Majorbio Biotech, Shanghai, China). The principal component analysis (PCA) and partial least squares-discriminant analysis (PLS-DA) were performed using the R package “ropls” (version 1.6.2), and 7-cycle interactive validation was used to evaluate the stability of the model. Differential metabolites in volcano plots were identified based on P values (P < 0.05, t-test) and VIP values (VIP > 1). Differential metabolites among the two groups were mapped into their biochemical pathways through metabolic enrichment and pathway analysis based on the KEGG database (http://www.genome.jp/kegg/). Python package “scipy.stats” (https://docs.scipy.org/doc/scipy/) was used to perform enrichment analysis.
Statistical analysis
Data are analyzed using SPSS version 26.0 (IBM Corp., Armonk, NY, USA) and expressed as mean ± SEM. Differences between groups were analyzed using a two-way analysis of variance (ANOVA), followed by Duncan’s multiple comparison test. The graphs were created using GraphPad Prism 8 Software (GraphPad Software Inc., La Jolla, CA, USA). Differences were considered statistically significant when P < 0.05.
Results
Growth performance
As shown in Table 3, MCE supplementation significantly increased the ADFI (P < 0.05) in broiler chickens, but did not affect the ADG and F/G (P > 0.05) during days 0-14. During days 15-21, there was an interaction between the LPS challenge and MCE supplementation on the ADG (P < 0.05). The LPS group significantly decreased the ADG in broiler chickens compared with the CON group, while the MCE+LPS group significantly suppressed the LPS-induced decrease in the ADG (P < 0.01). LPS challenge significantly decreased the ADFI in broiler chickens (P < 0.001) regardless of MCE supplementation. In addition, MCE supplementation, LPS challenge and their interaction had no effects on the F/G (P > 0.05) during days 0-21.
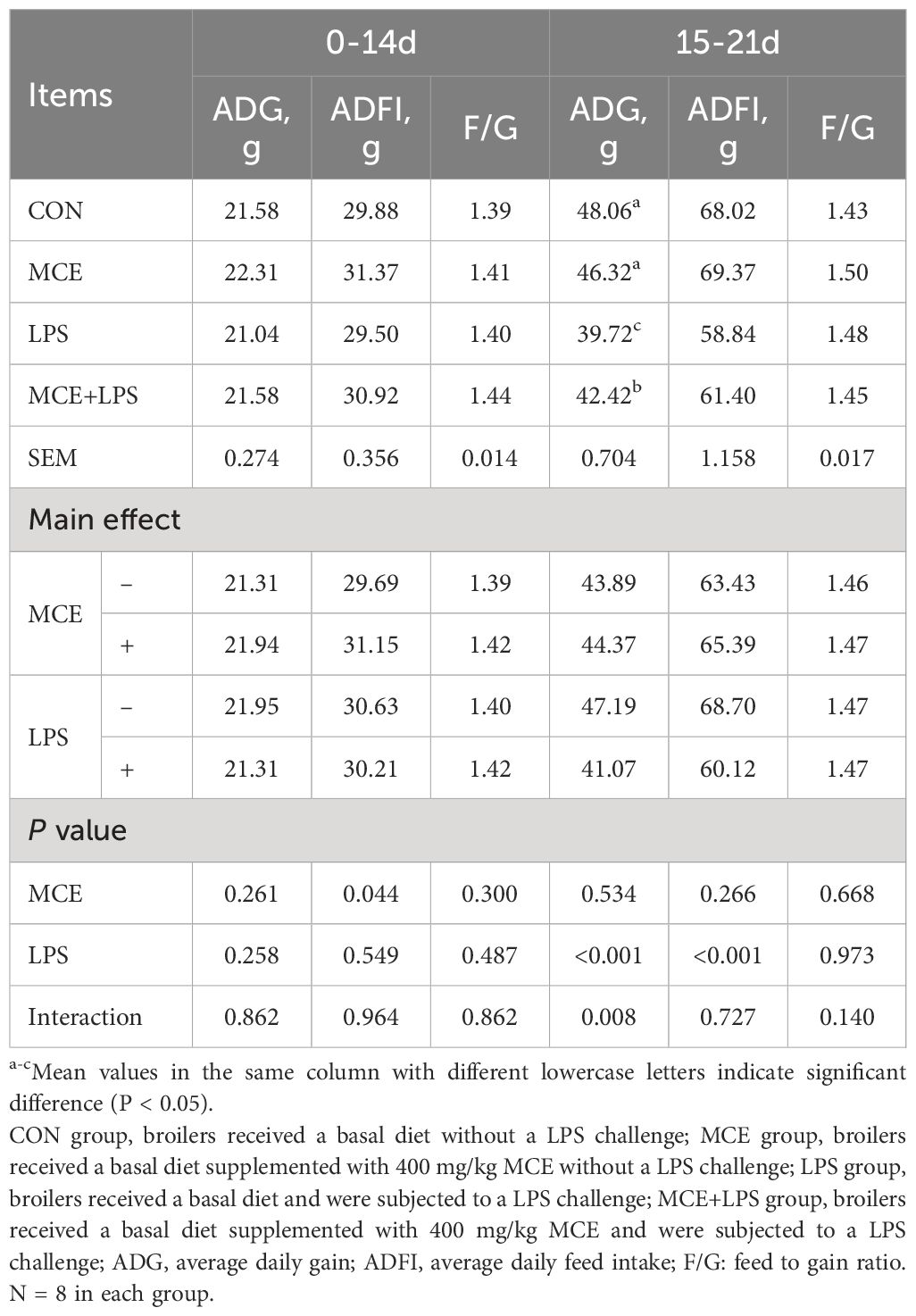
Table 3 Effects of Macleaya cordata extract (MCE) on growth performance in broiler chickens challenged with LPS.
Jejunal mucosal antioxidant status
As shown in Table 4, LPS challenge significantly decreased the T-AOC (P < 0.001) and the activities of CAT (P < 0.01) and SOD (P < 0.01) and increased the level of MDA (P < 0.05) in broiler chickens. MCE supplementation significantly increased the T-AOC and the activity of CAT and reduced the level of MDA in broiler chickens (P < 0.05). MCE supplementation, LPS challenge and their interaction have no effects on GSH-Px activity (P > 0.05).
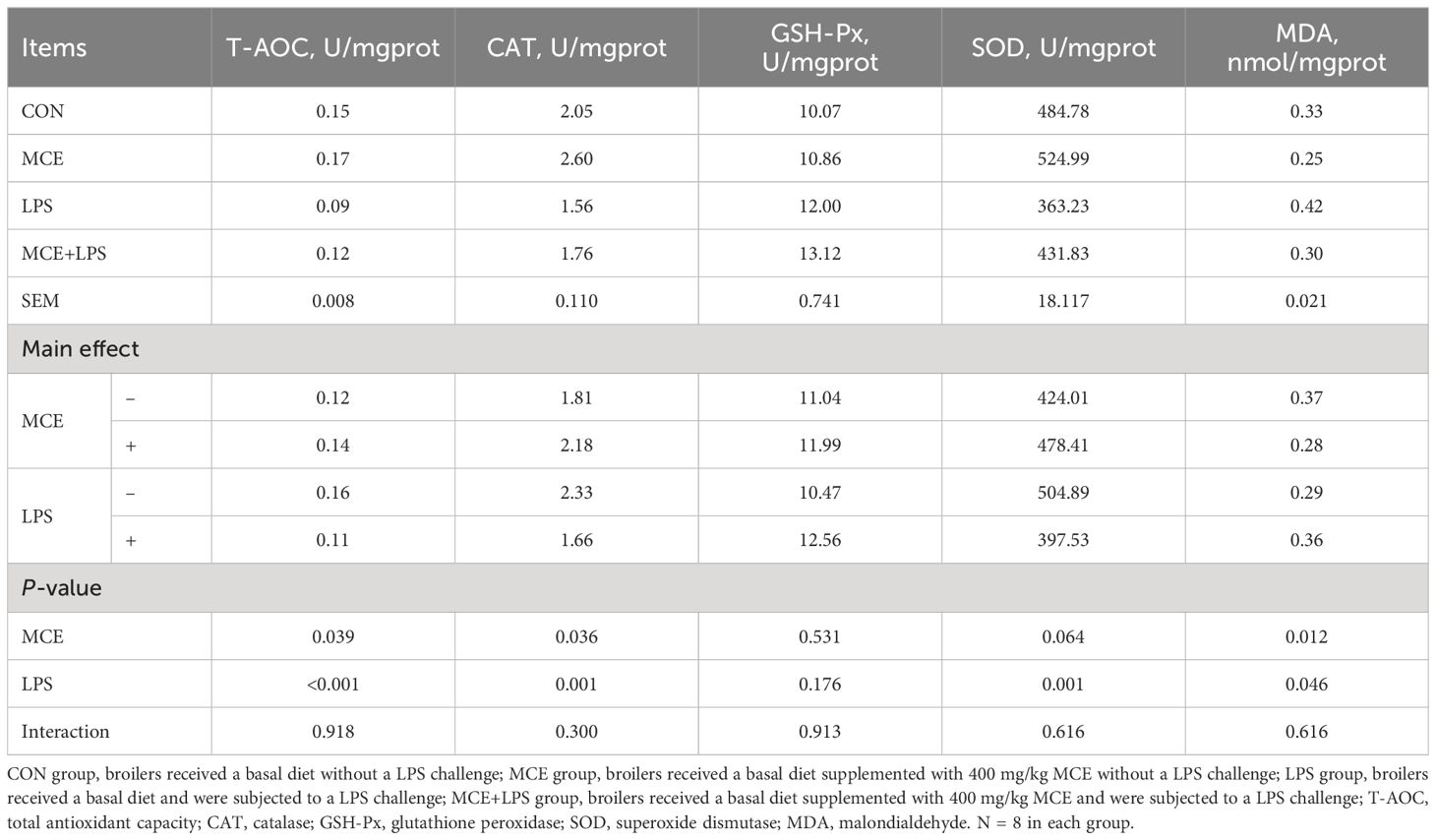
Table 4 Effects of Macleaya cordata extract (MCE) on the antioxidant ability of jejunum in broiler chickens challenged with LPS.
Ileal morphology
Hematoxylin and eosin staining results revealed nearly normal intestinal morphology in the CON and MCE groups. However, the LPS group exhibited significant intestinal mucosa damage, including damaged villus tips and villi atrophy. Nevertheless, intestinal mucosa damage was reduced in the MCE+LPS group (Figure 1). As shown in Table 5, LPS challenge significantly decreased the villus height in broiler chickens (P < 0.01), while MCE supplementation significantly increased the villus height (P < 0.05). LPS challenge significantly decreased the VCR in broiler chickens (P < 0.01), while MCE supplementation significantly increased the VCR (P < 0.05). MCE supplementation and LPS challenge have no effects on crypt depth (P > 0.05).
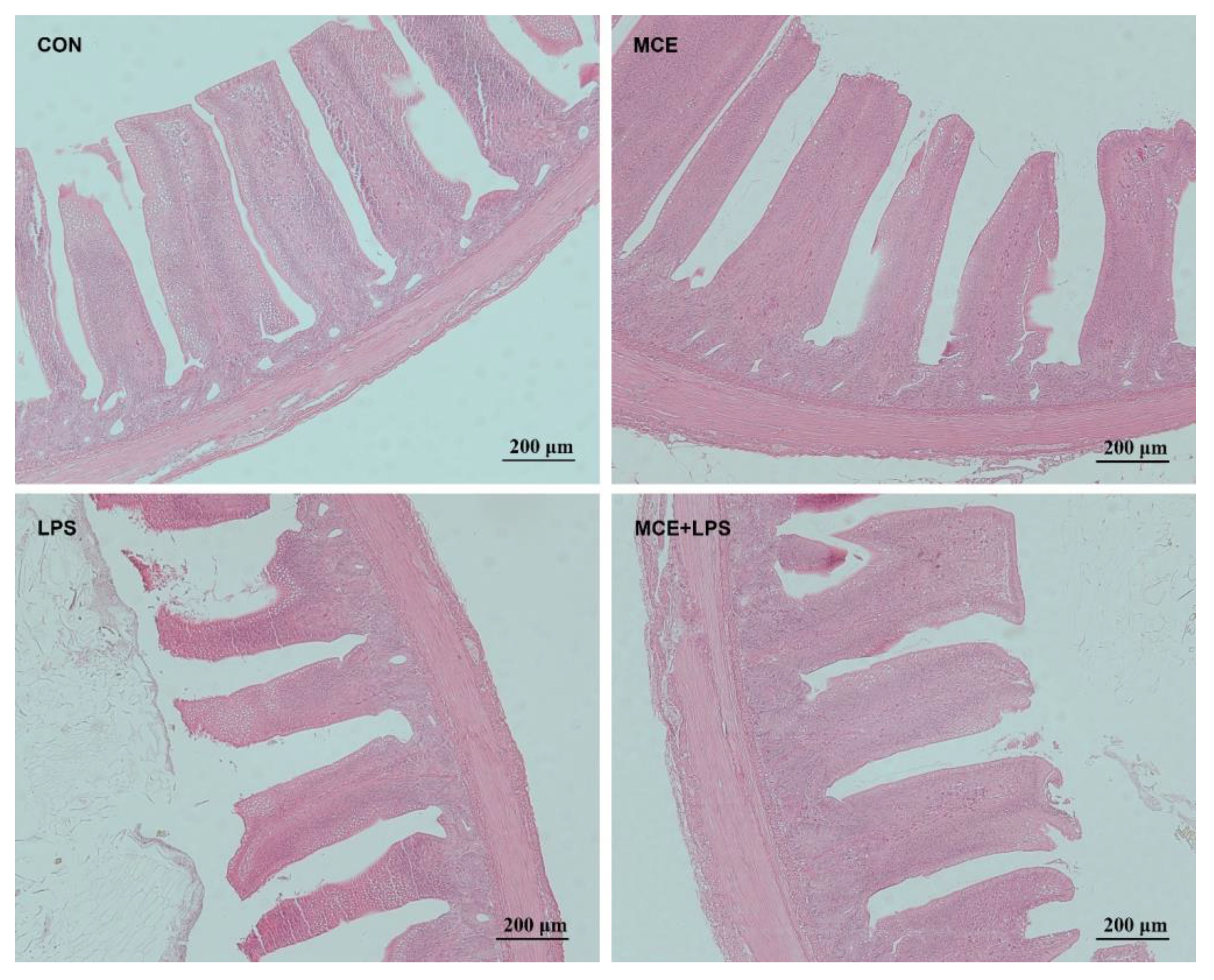
Figure 1 The representative images of ileal morphology by hematoxylin and eosin staining. Original magnification 100×, scale bar 200 μm. CON group, broilers received a basal diet without a LPS challenge; MCE group, broilers received a basal diet supplemented with 400 mg/kg MCE without a LPS challenge; LPS group, broilers received a basal diet and were subjected to a LPS challenge; MCE+LPS group, broilers received a basal diet supplemented with 400 mg/kg MCE and were subjected to a LPS challenge.
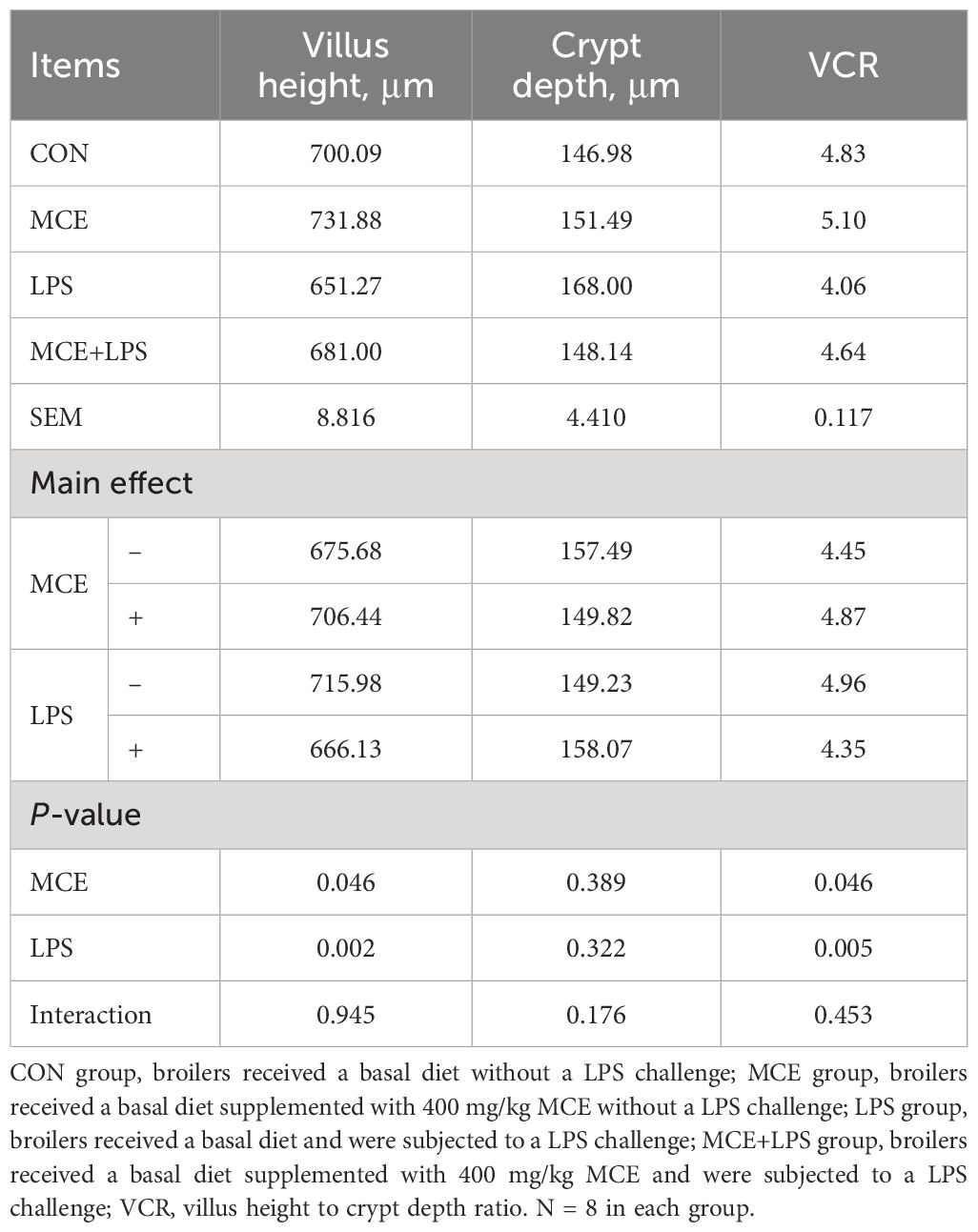
Table 5 Effects of Macleaya cordata extract (MCE) on the ileal morphology in broiler chickens challenged with LPS.
Relative mRNA expression of jejunal inflammatory genes
As shown in Table 6, MCE supplementation and LPS challenge have interactions on the mRNA expression of IL-1β and IL-17. Compared with the CON group, the LPS group significantly increased the mRNA expression of IL-1β and IL-17, while the MCE+LPS group significantly suppressed the increase of mRNA expression of IL-1β and IL-17 induced by LPS challenge (P < 0.01). LPS challenge significantly increased the mRNA expression of IL-8 (P < 0.01), IFN-γ (P < 0.05), and iNOS (P < 0.05). MCE supplementation significantly decreased the mRNA expression of IL-6 and IL-8 (P < 0.05).
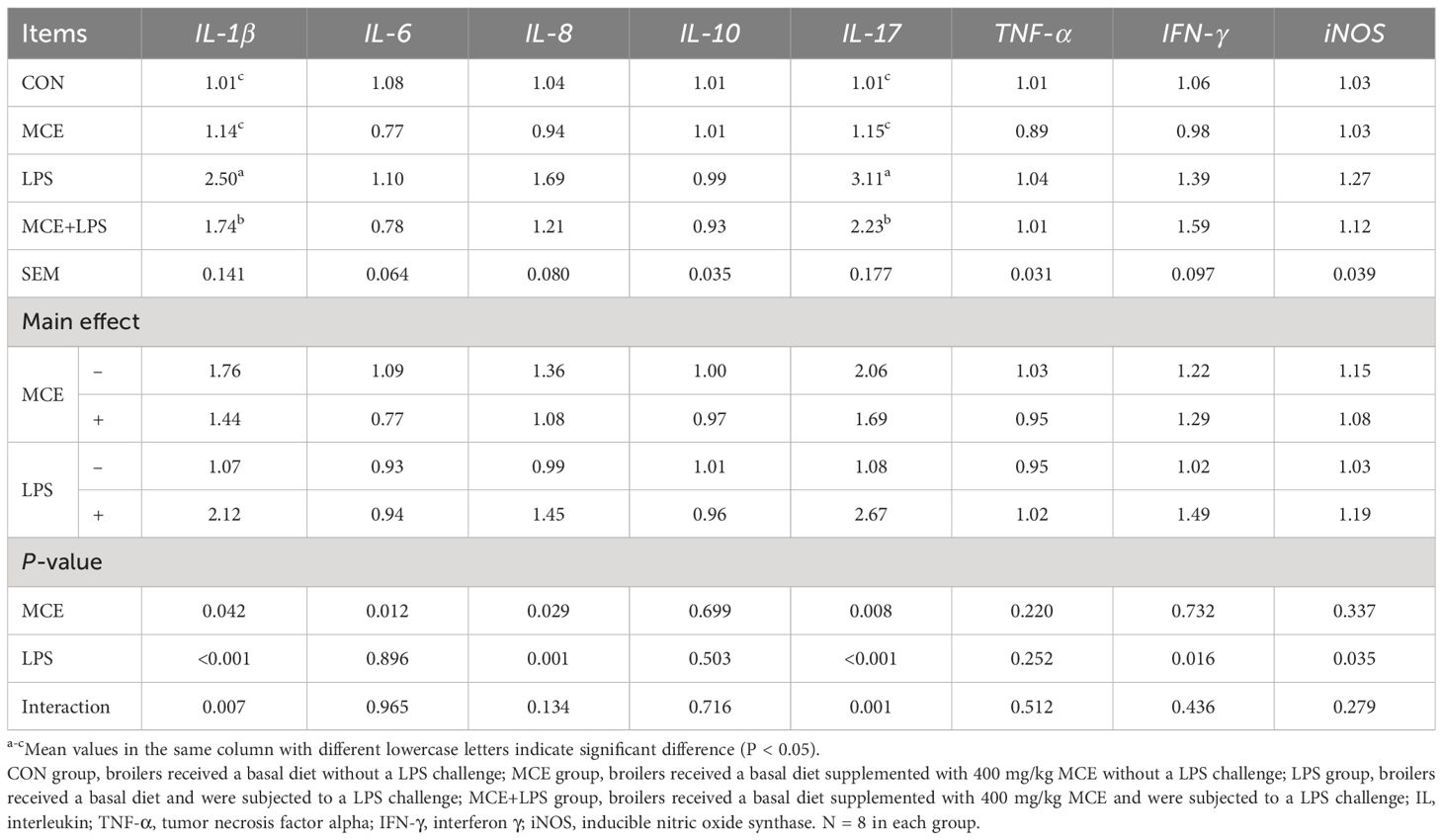
Table 6 Effects of Macleaya cordata extract (MCE) on the jejunal relative mRNA expression of inflammatory genes in broiler chickens challenged with LPS.
Gut microbiome
α-diversity and β-diversity
As shown in Figures 2A–E, LPS challenge significantly decreased the abundance-based coverage estimator (ACE) index (P < 0.05), Chao index (P < 0.01) and Shannon index (P < 0.05), while MCE supplementation significantly increased the ACE index (P < 0.05). MCE supplementation significantly elevated the Simpson index (P < 0.05) regardless of LPS challenge. There is an interaction on the Sobs index between MCE supplementation and LPS challenge (P < 0.05). The Sobs index was significantly higher in the MCE group than in other groups (P < 0.01). PCA and PCoA were used to examine the similarities and differences of gut microbiota between groups in broiler chickens and there was no distinct separation between groups (Figures 2F, G).
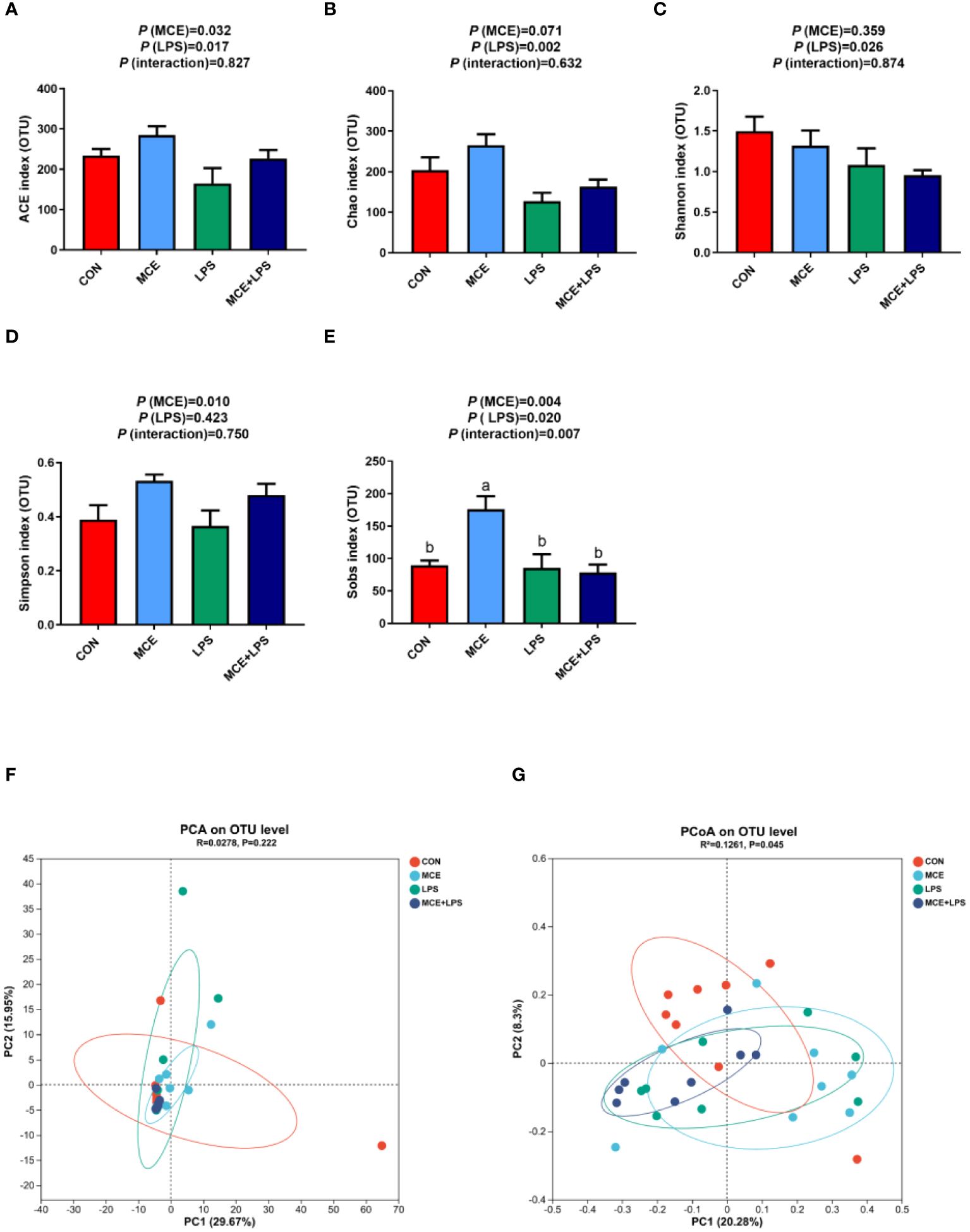
Figure 2 Effects of Macleaya cordata extract (MCE) on α-diversity and β-diversity of gut microbiota in broiler chickens challenged with LPS. Changes in α-diversity: (A) ACE index, (B) Chao index, (C) Shannon index, (D) Simpson index, and (E) Sobs index α-diversity. (F) PCA and (G) PCoA of the β-diversity. CON group, broilers received a basal diet without a LPS challenge; MCE group, broilers received a basal diet supplemented with 400 mg/kg MCE without a LPS challenge; LPS group, broilers received a basal diet and were subjected to a LPS challenge; MCE+LPS group, broilers received a basal diet supplemented with 400 mg/kg MCE and were subjected to a LPS challenge. a, b Mean values with no common lowercase letters indicate significant difference (P < 0.05). N = 8 in each group.
The composition of gut microbiota and LEfSe analysis
As illustrated in the Venn diagram, a total of 180 OTUs were identified in all groups, with 36, 26, 47 and 10 particular OTUs in the CON, MCE, LPS, and MCE+LPS groups, respectively (Figure 3A). The dominant microbiota in all groups mainly included Firmicutes, Proteobacteria, Actinobacteriota, Patescibacteria at the phylum level (Figure 3B), and Lactobacillus, Peptostreptococcaceae, Bacillus and Romboutsia at the genus level (Figure 3C). The LDA scores were used to identify taxonomic biomarkers contributing to the differences between groups (Figure 3D). Blautia, GCA-900066575, Anaerostipes butyraticus, Anaerostipes, and Clostridium_sensu_stricto_1 had higher LDA scores in the MCE group. Actinobacteriota, Staphylococcus_sciuri, Micrococcales, Streptococcus, Alphaproteobacteria, Rhizobiales, Microbacterium, Rhizobiaceae, Christensenellaceae_R-7_group, and Allorhizobium-Neorhizobium-Pararhizobium-Rhizobium had higher LDA scores in the LPS group. Kurthia had a higher LDA score in the MCE+LPS group.
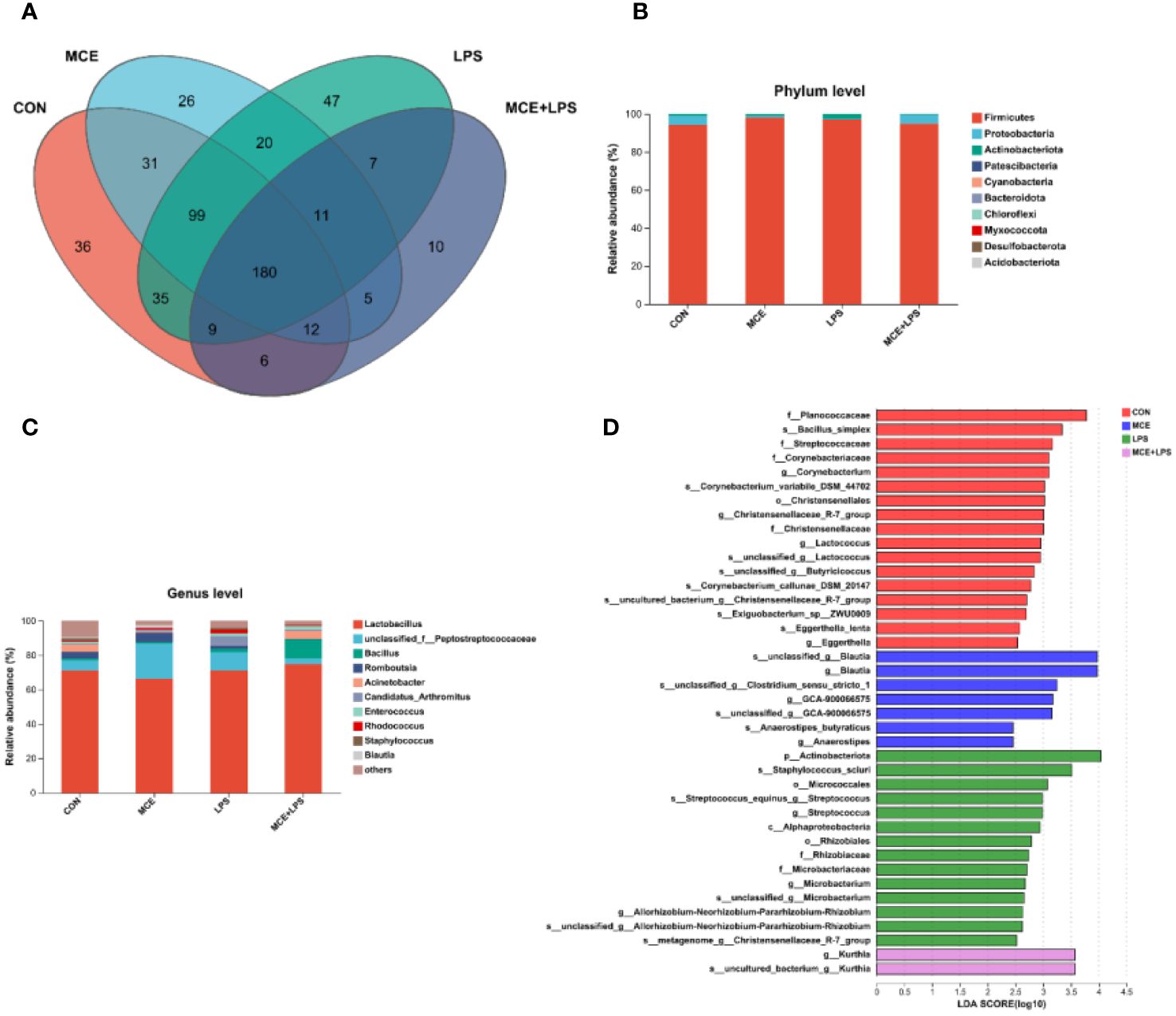
Figure 3 Effects of Macleaya cordata extract (MCE) on the composition of gut microbiota in broiler chickens challenged with LPS. (A) the Venn diagram of gut microbiota, (B) the composition of gut microbiota at the phylum level, (C) the composition of gut microbiota at the genus level, and (D) the LEfSe analysis of gut microbiota. CON group, broilers received a basal diet without a LPS challenge; MCE group, broilers received a basal diet supplemented with 400 mg/kg MCE without a LPS challenge; LPS group, broilers received a basal diet and were subjected to a LPS challenge; MCE+LPS group, broilers received a basal diet supplemented with 400 mg/kg MCE and were subjected to a LPS challenge. N = 8 in each group.
The relative abundance of gut microbiota at the phylum and genus levels
As depicted in Figures 4A, B, at the phylum level, MCE supplementation significantly increased the relative abundance of Firmicutes (P < 0.05). Compared with the CON group, the LPS group significantly increased the relative abundance of Actinobacteriota, while the MCE+LPS group significantly suppressed the increase induced by LPS challenge (P < 0.05). As shown in Figures 4C–F, at the genus level, MCE supplementation significantly increased the relative abundance of Lactobacillus (P < 0.05). Interactions have been observed in the relative abundance of Peptostretococcaceae, Rhodococcus and Blautia between MCE supplementation and LPS challenge (P < 0.05). Compared with the CON group, the LPS group significantly increased the relative abundance of Peptostretococcaceae and Rhodococcus, while the MCE+LPS group suppressed the increase induced by LPS challenge (P < 0.05). Blautia had a higher relative abundance in the MCE group than in other groups (P < 0.05).
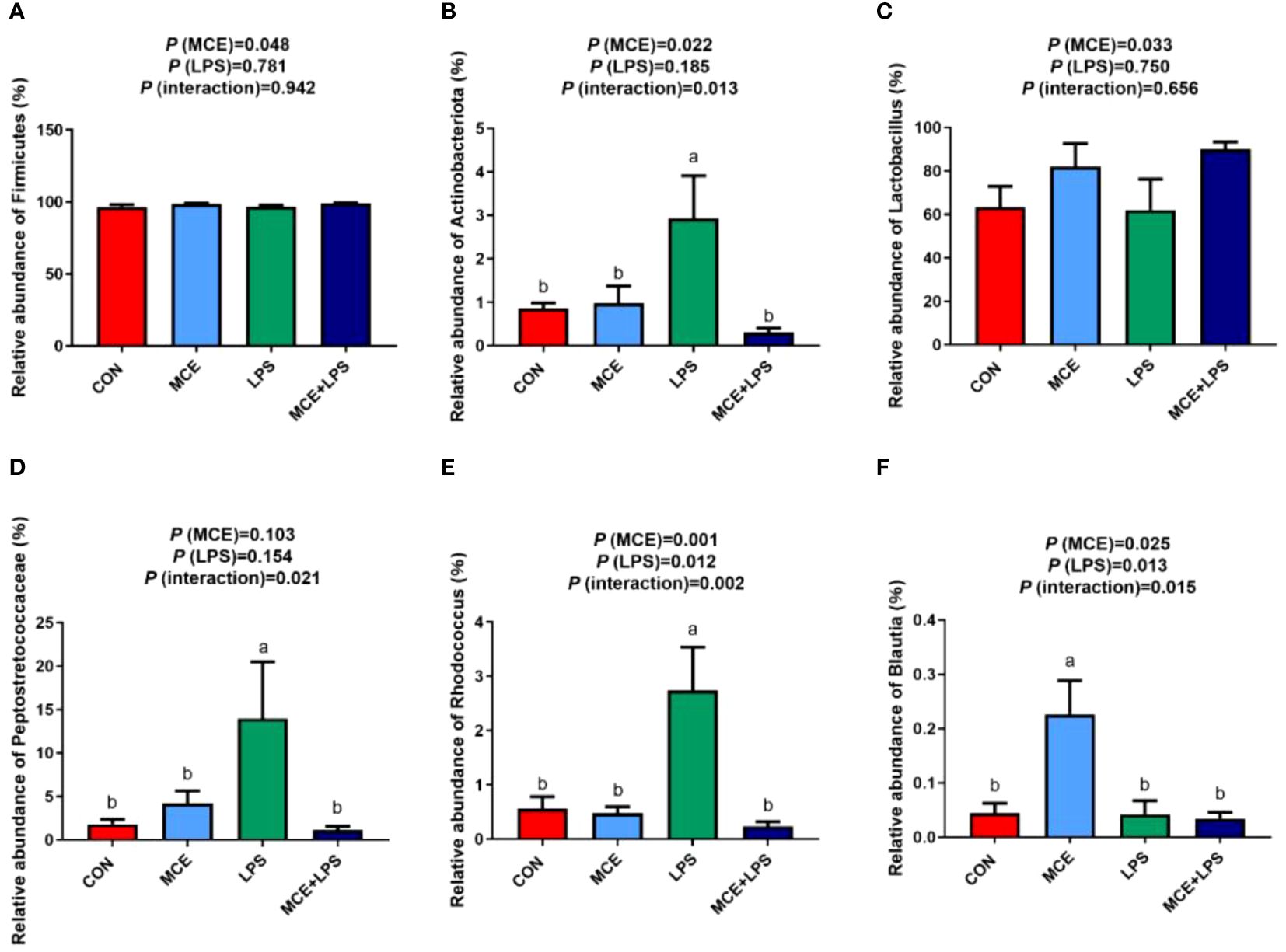
Figure 4 Effects of Macleaya cordata extract (MCE) on the relative abundance of bacteria at the phylum and genus levels in broiler chickens challenged with LPS. (A) Firmicutes and (B) Actinobacteriota at the phylum level. (C) Lactobacillus, (D) Peptostretococcaceae, (E) Rhodococcus, and (F) Blautia at the genus level. CON group, broilers received a basal diet without a LPS challenge; MCE group, broilers received a basal diet supplemented with 400 mg/kg MCE without a LPS challenge; LPS group, broilers received a basal diet and were subjected to a LPS challenge; MCE+LPS group, broilers received a basal diet supplemented with 400 mg/kg MCE and were subjected to a LPS challenge. a, b Mean values with no common lowercase letters indicate significant difference (P < 0.05). N = 8 in each group.
Non-targeted metabolomics in plasma
PCA and PLS-DA plots
There were 551 metabolites identified in the positive mode and 217 metabolites identified in the negative mode in all groups. The differences in metabolites between groups are shown in the PCA and PLS-DA plots (Figure 5). There was a distinct separation between the CON group and the LPS group in both positive and negative modes. A clear separation was also observed in the negative mode between the LPS and MCE+LPS groups. There was a clear separation between the CON and MCE groups in PLS-DA score plots in the positive mode.
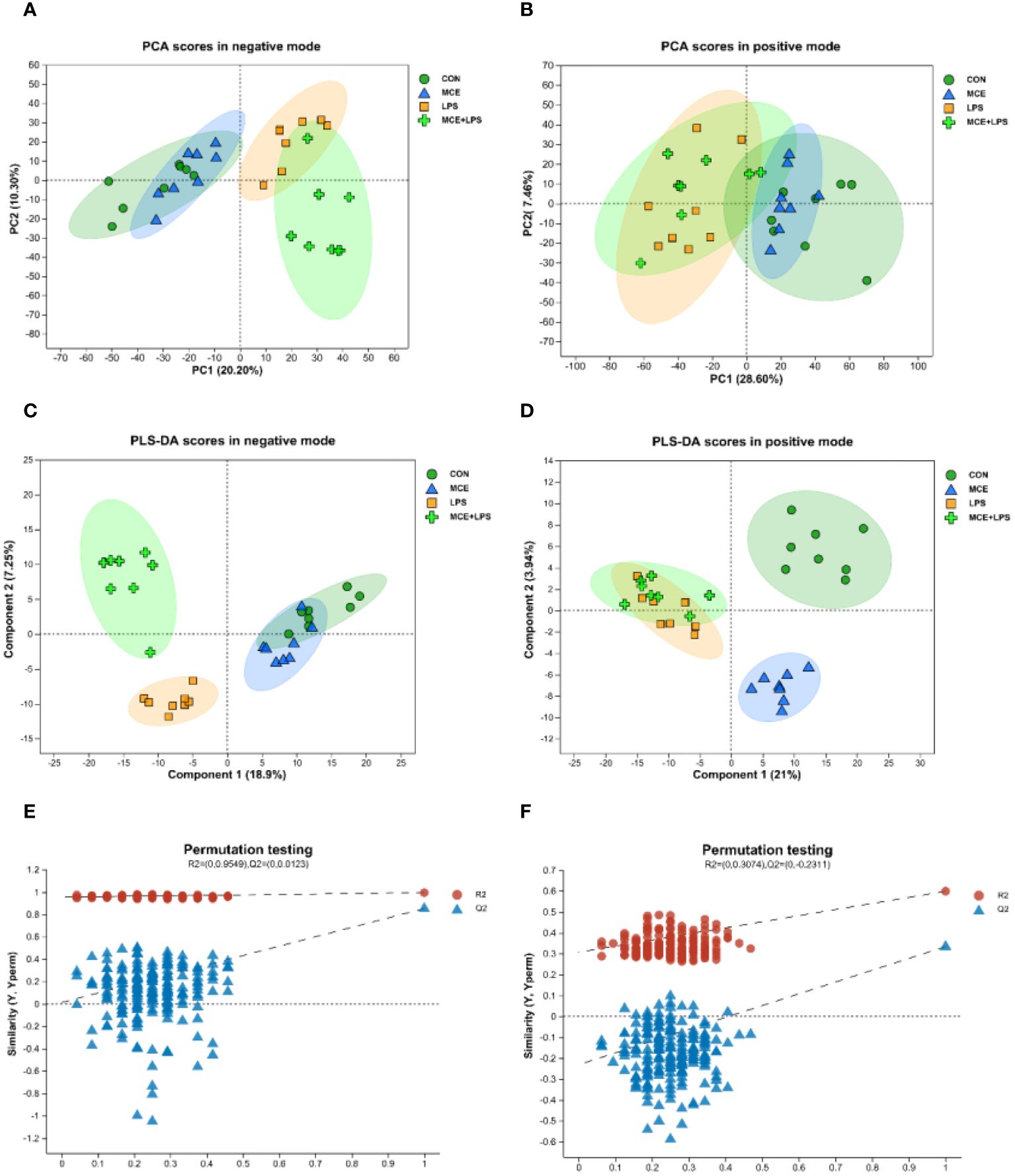
Figure 5 PCA and PLS-DA score plots of plasma metabolites in broiler chickens. (A, B) PCA scores in negative and positive modes. (C, D) PLS-DA scores in negative and positive modes. (E, F) Permutation test plots of PLS-DA scores in negative and positive modes. CON group, broilers received a basal diet without a LPS challenge; MCE group, broilers received a basal diet supplemented with 400 mg/kg MCE without a LPS challenge; LPS group, broilers received a basal diet and were subjected to a LPS challenge; MCE+LPS group, broilers received a basal diet supplemented with 400 mg/kg MCE and were subjected to a LPS challenge. N = 8 in each group.
Alteration in plasma metabolic composition
The significantly differential metabolites between groups are shown in the volcano maps (Figure 6). As shown in Figure 6A, in the negative mode, compared with the CON group, the MCE group had 6 up-regulated metabolites (2,3-Dihydroxybenzoic acid, (25R)-3beta-hydroxycholest-5-en-7-one-26-oate, Ganoderic acid C1, L-Ascorbic acid, Myo-Inositol, and Pyrocatechol sulfate) and 29 down-regulated metabolites (such as Uridine, 13(S)-HODPE, Xanthosine, Xanthine, and Pseudouridine). As shown in Figure 6B, in the positive mode, compared with the CON group, the MCE group had 16 up-regulated metabolites, (such as Thr-Thr-Lys-Phe, Capsianoside III, Beta-Alanine, 5-Aminolevulinic acid, and Pro-Tyr-Gly) and 45 down-regulated metabolites (such as Hypoxanthine, Octadecenoylcarnitine, 13Z-Docosenamide, Tranexamic acid, and Uracil).
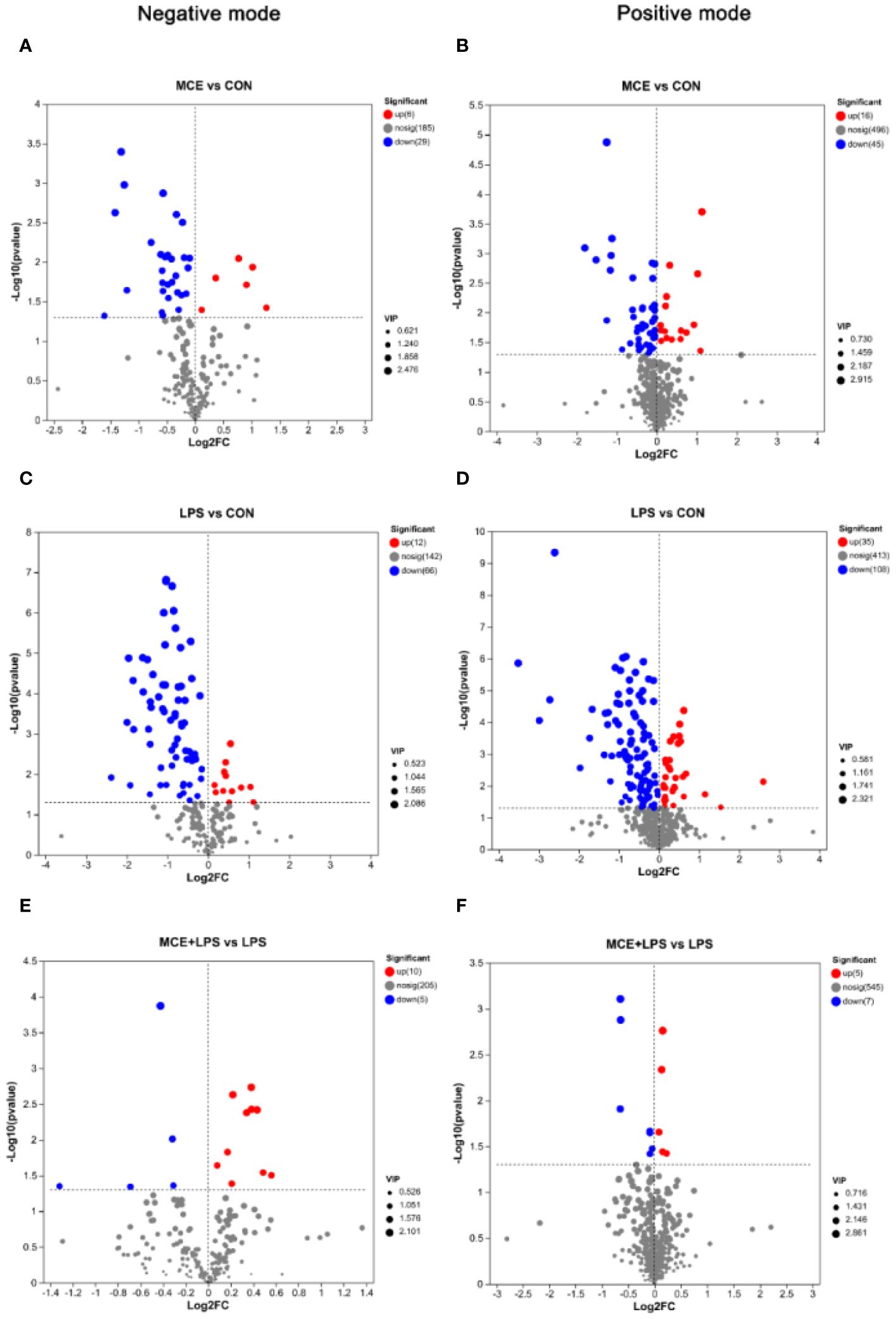
Figure 6 Volcano plots showing significantly up-regulated and down-regulated metabolites between different groups of broiler chickens. (A, B) significantly differential metabolites between the MCE and CON groups in negative and positive modes. (C, D) significantly differential metabolites between the LPS and CON groups in negative and positive modes. (E, F) significantly differential metabolites between the MCE+LPS and LPS groups in negative and positive modes. CON group, broilers received a basal diet without a LPS challenge; MCE group, broilers received a basal diet supplemented with 400 mg/kg MCE without a LPS challenge; LPS group, broilers received a basal diet and were subjected to a LPS challenge; MCE+LPS group, broilers received a basal diet supplemented with 400 mg/kg MCE and were subjected to a LPS challenge. N = 8 in each group.
As shown in Figure 6C, in the negative mode, compared with the CON group, LPS challenge increased the levels of 12 metabolites (such as Citramalic acid, Ketosantalic acid, Ribothymidine, Pyrocatechol sulfate, and Glutaric acid) and decreased the levels of 66 metabolites, (such as Inosine, Stearic acid, Pseudouridine, L-Glutamate, and Threoninyl-Tyrosine). As shown in Figure 6D, in the positive mode, compared with the CON group, the LPS group had 35 up-regulated metabolites (such as (E)-2-Methylglutaconic acid, Deoxypyridinoline, 2-Naphthylamine, Stigmastanol, and 2-Deoxybrassinolide) and 108 down-regulated metabolites (such as Pantothenic Acid, Asperagenin, Octadecenoylcarnitine, Acetylcarnitine, and Val Glu Val).
As presented in Figure 6E and Table 7, in the negative mode, compared with the LPS group, the MCE+LPS group had 10 up-regulated metabolites (Gamma-Glutamylthreonine, D-altro-D-manno-Heptose, N-acetylaspartate, (1R,2S,3S,4R)-p-Menthane-2,3-diol, Stearic acid, Threoninyl-Tyrosine, L-Ribulose, L-Aspartic Acid, L-Glutamate and Myo-Inositol) and 5 down-regulated metabolites (TXB2, Lamivudine sulfoxide, Ribothymidine, 6-(3-ethenylphenoxy)-3,4,5-trihydroxyoxane-2-carboxylic acid and 5-Methoxynoracronycine). As presented in Figure 6F and Table 7, in the positive mode, compared with the LPS group, the MCE+LPS group had 5 up-regulated metabolites (Cucurbic acid, L-Serine, Corchorifatty acid A, Cyclohex-2-enone and Vinylacetylglycine) and 7 down-regulated metabolites (Sphingosine-1-phosphate, 1-Methyladenine, Oryzalexin E, Sphinganine-phosphate, Cycloalliin, (8alpha,10beta,11beta)-3-Hydroxy-4,15-dinor-1(5)-xanthen-12,8-olide and 20-Hydroxy-PGF2a).
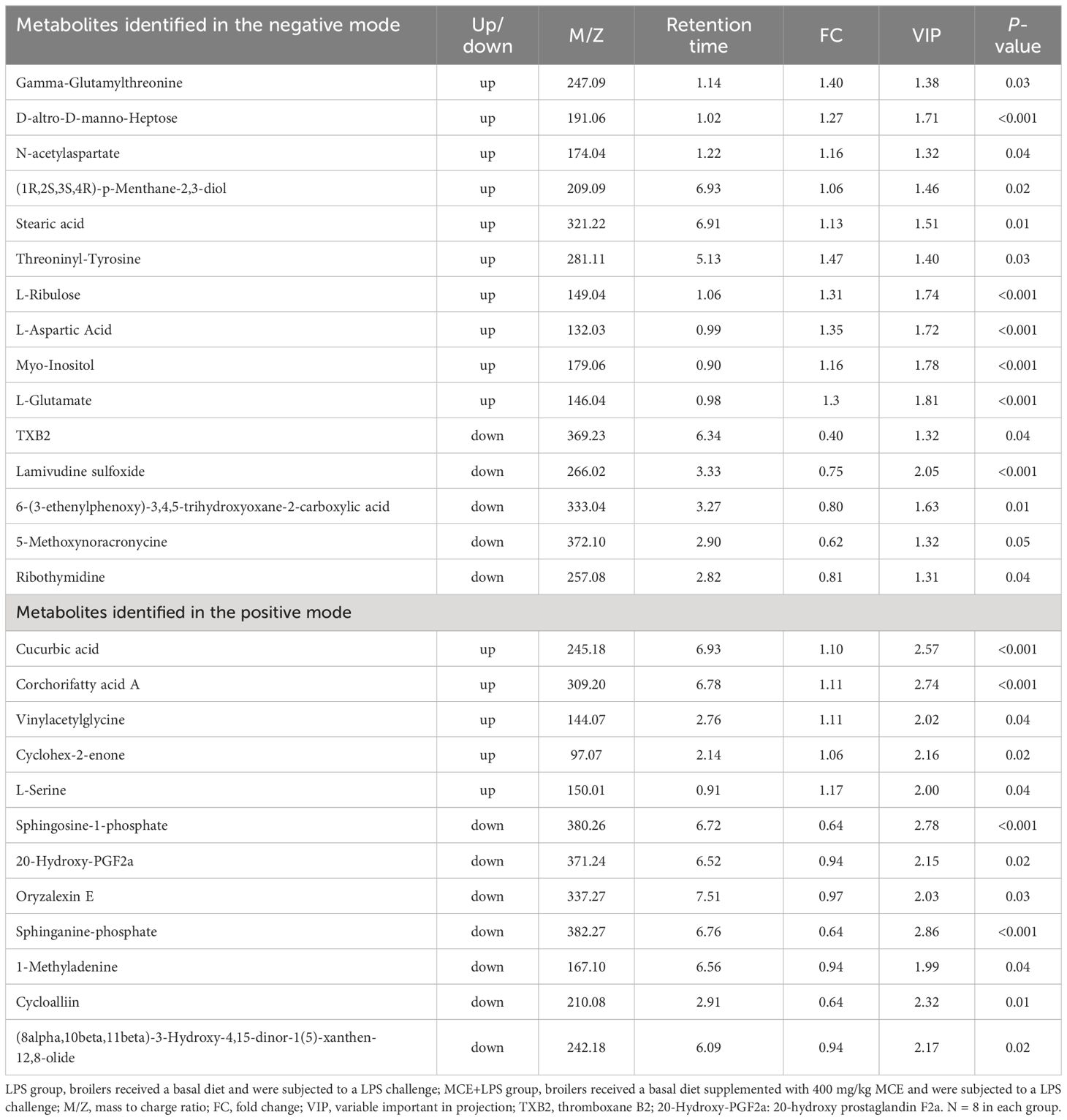
Table 7 Up- and down-regulated plasma metabolites in broilers between the MCE+LPS and LPS groups in negative and positive modes.
KEGG enrichment of differential metabolites
As presented in Figure 7, the KEGG metabolic pathway enrichment analysis demonstrated that significantly differential metabolites between the MCE and CON groups were enriched in Nucleotide metabolism, Lipid metabolism, Metabolism of cofactors and vitamins, Metabolism of other amino acids, Carbohydrate metabolism, Amino acid metabolism and others. MCE supplementation altered the pathways of Pyrimidine metabolism, ABC transporters, Caffeine metabolism, Purine metabolism, Pantothenate and CoA biosynthesis, beta-Alanine metabolism and others.
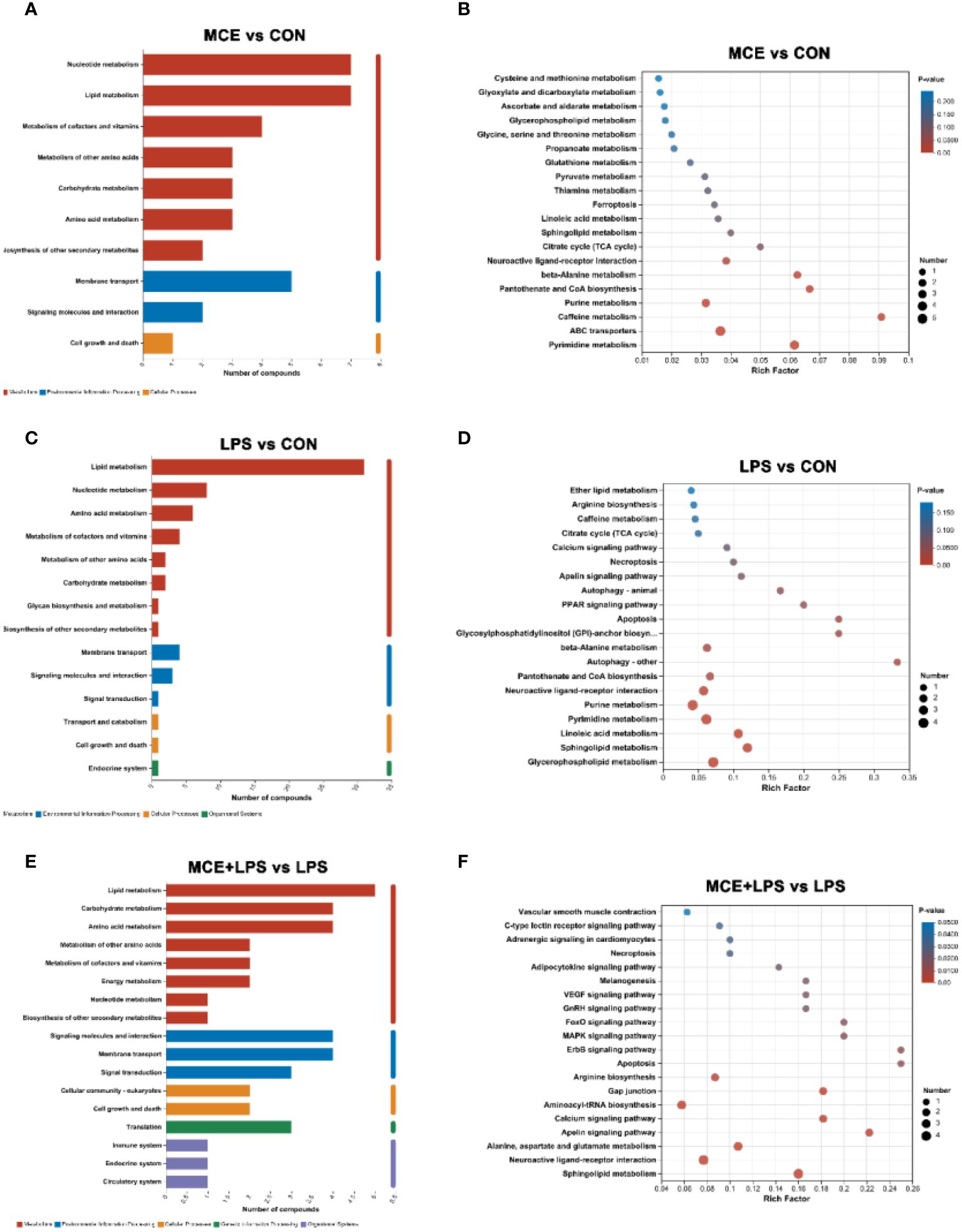
Figure 7 Effects of Macleaya cordata extract (MCE) on KEGG pathways enrichment for differential plasma metabolites in broiler chickens. The first and second (A) and third (B) classification categories of the KEGG pathway between MCE and CON groups. The first and second (C) and third (D) classification categories of the KEGG pathway between LPS and CON groups.The first and second (E) and third (F) classification categories of the KEGG pathway between MCE+LPS and LPS groups. CON group, broilers received a basal diet without a LPS challenge; MCE group, broilers received a basal diet supplemented with 400 mg/kg MCE without a LPS challenge; LPS group, broilers received a basal diet and were subjected to a LPS challenge; MCE+LPS group, broilers received a basal diet supplemented with 400 mg/kg MCE and were subjected to a LPS challenge. N = 8 in each group.
The significantly differential metabolites between the LPS and CON groups were enriched in Lipid metabolism, Nucleotide metabolism, Amino acid metabolism, Metabolism of cofactors and vitamins, Metabolism of other amino acids, Carbohydrate metabolism and others. Compared with the CON group, LPS challenge altered the pathways of Glycerophospholipid metabolism, Sphingolipid metabolism, Linoleic acid metabolism, Pyrimidine metabolism, Purine metabolism, Neuroactive ligand-receptor interaction, Pantothenate and CoA biosynthesis and others.
The significantly differential metabolites between the MCE+LPS and LPS groups were enriched in Lipid metabolism, Carbohydrate metabolism, Amino acid metabolism, Metabolism of other amino acids, Metabolism of cofactors and vitamins, Energy metabolism, and others. Compared with the LPS group, MCE supplementation altered the pathways of Sphingolipid metabolism, Neuroactive ligand-receptor interaction, Alanine, aspartate and glutamate metabolism, Apelin signaling pathway, Calcium signaling pathway, Aminoacyl-tRNA biosynthesis, Gap junction, Arginine biosynthesis, Apoptosis, MAPK signaling pathway and others.
Correlation analysis between gut microbiota and metabolites
As shown in Figure 8A, at the phylum level, in the negative mode, the relative abundance of Firmicutes was negatively correlated with N-Desthienylethyl-rotigotine. A negative correlation was observed between the relative abundance of Proteobacteria and (+/-)-Tryptophan. The relative abundance of Actinobacteriota was positively correlated with lysophosphatidylcholine (LysoPC)(17:0) and LysoPC(18:0) and was negatively correlated with Myo-Inositol. The relative abundance of Bacteroidota showed a negative correlation with gamma-glutamylphenylalanine. As shown in Figure 8B, in the positive mode, A positive correlation was observed between the relative abundance of Firmicutes and 2-Hydroxycinnamic acid. The relative abundance of Actinobacteriota was positively correlated with LysoPC(18:1(11Z)) and phosphatidylcholine (PC)(18:0/0:0) and was negatively correlated with Cinnamic acid and 2-Hydroxycinnamic acid. The relative abundance of Desulfobacterota exhibited a positive correlation with 3-O-Acetylepisamarcandin and Triethylamine.
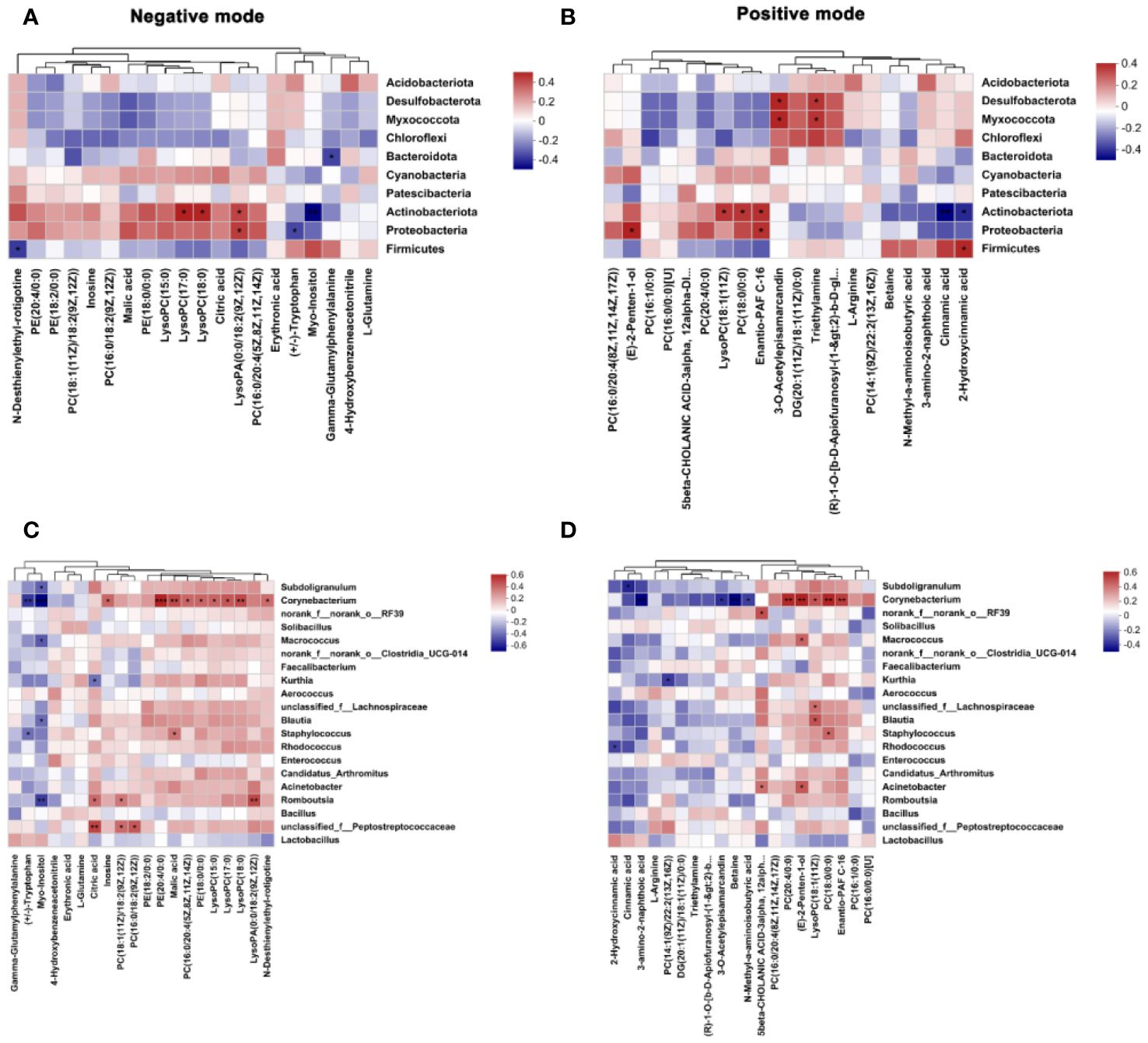
Figure 8 Spearman correlation analysis of gut microbiota and plasma metabolites in broiler chickens. (A, B) correlation between the top 10 phyla of ileal microbiota and the top 20 differential plasma metabolites in the negative and positive modes. (C, D) correlation between the top 20 genera of ileal microbiota and top 20 differential plasma metabolites in the negative and positive modes. CON group, broilers received a basal diet without a LPS challenge; MCE group, broilers received a basal diet supplemented with 400 mg/kg MCE without a LPS challenge; LPS group, broilers received a basal diet and were subjected to a LPS challenge; MCE+LPS group, broilers received a basal diet supplemented with 400 mg/kg MCE and were subjected to a LPS challenge. Significance was set at P < 0.05. *P < 0.05, **P < 0.01, ***P < 0.001. N = 8 in each group.
As presented in Figure 8C, at the genus level, in the negative mode, the relative abundance of Peptostreptococcaceae was positively correlated with PC(18:1(11Z)/18:2(9Z,12Z)) and PC(16:0/18:2(9Z,12Z)). The relative abundance of Romboutsia showed a positive correlation with Citric acid. The relative abundance of Corynebacterium was positively correlated with inosine, phosphatidylethanolamine (PE)(20:4/0:0), PC(16:0/20:4(5Z,8Z,11Z,14Z)), PE(18:0/0:0), and LysoPC(15:0) and was negatively correlated with (+/-)-Tryptophan and Myo-Inositol. As shown in Figure 8D, in the positive mode, the relative abundance of Rhodococcus was negatively correlated with 2-Hydroxycinnamic acid. A positive correlation was observed between the relative abundance of Staphylococcus and PC (18:0/0:0). The relative abundance of Kurthia diaplayed a negative correlation with PC(14:1(9Z)/22:2(13Z,16Z)). The relative abundance of Corynebacterium was positively correlated with PC(20:4/0:0), LysoPC(18:1(11Z)) and PC(18:0/0:0), and was negatively correlated with Betaine.
Discussion
In this study, MCE supplementation significantly increased the ADFI during days 0-14, and, the ADG during days 15-21 was higher in the MCE+LPS group than in the LPS group. The results are in agreement with previous studies. Dietary supplementation of 350 mg/kg MCE effectively alleviated the necrotic enteritis-induced reduction of growth performance in broiler chickens (19). Diet with 0.6 mg/kg of MCE containing protopine and allotypotopine increased the final body weight and ADG and decreased F/G of broiler chickens from d 0 to 42 (28). It was reported that dietary supplementation of 50 mg/kg MCE could enhance the growth performance and intestinal morphology of early-weaned piglets (13). Sanguinarine and chelerythrine have similar structures to aromatic amino acids, which can irreversibly inhibit the activity of intestinal aromatic amino acid decarboxylase (29). Sanguinarine has been proved to promote protein retention by lowering intestinal decarboxylation of aromatic amino acids and increases feed intake by regulating the Trp-serotonin pathway, thus improving animal growth (17, 30). These responses may explain the improved growth performance of broiler chickens by MCE supplementation in this study.
T-AOC represents the capacity of the enzymatic and non-enzymatic antioxidant system in the body (31). CAT, GSH-Px and SOD are important antioxidant enzymes involved in eliminating superoxide anions and hydrogen peroxide during oxidative damage (31, 32). The level of MDA reflects the degree of lipid peroxidation in the body and is an indicator of initial cellular membrane damage (33). Our study revealed that dietary MCE supplementation increased the T-AOC and CAT activity while decreasing MDA levels in the jejunum. Similarly, dietary supplementation of 200 mg/kg MCE observably alleviated oxidative stress induced by enterotoxigenic Escherichia coli in mice, as illustrated by lower levels of MDA and increased activities of CAT and GSH-Px (18). It has been reported that sanguinarine not only decreased the ROS level induced by LPS, but also increased the activity of antioxidant enzymes by activating the Nrf2 signaling pathway in mouse mammary epithelial cells (34). Moreover, sanguinarine can inhibit the activities of nicotinamide adenine dinucleotide phosphate oxidase 2, thereby inhibiting the conversion of NADPH to ROS and improving the antioxidant capacity (35).
The intestinal morphology is a crucial indicator for gut health and intestinal absorption capacity. This study demonstrated that diets supplemented with MCE could effectively increase the ileal villus height and VCR in broiler chickens. Similarly, Song et al. (19) has discovered that the diet supplemented with 350 mg/kg MCE significantly improved the growth performance, increased the jejunal villus height and VCR and decreased the intestinal lesion score in broiler chickens with necrotic enteritis. Dietary sanguinarine supplementation at 0.7 mg/kg improved growth performance and increased the villus height and VCR of the duodenum, jejunum and ileum in yellow feathered broiler chickens at 28 days of age (17). Piglets fed a basal diet supplemented with 50 mg/kg MCE exhibited a lower crypt depth of the jejunum, higher villus height of the ileum, and higher VCR of the jejunum and ileum than the animals fed the basal diet (13). According to the findings of this study, MCE may enhance growth performance by improving intestinal morphology, thus facilitating efficient nutrient absorption.
IL-1β is the main pro-inflammatory mediator of systemic inflammatory responses (36). IL-6 is synthesized in the early period of inflammation (37). IL-8 can cause mucosal damage via the release of ROS, metalloproteinases and cytokines (38). IL-17 plays a pro-inflammatory role by promoting the secretion of chemokines (39). This study showed that dietary MCE supplementation decreased the mRNA expression of pro-inflammatory factors IL-1β, IL-8 and IL-17 in LPS-challenged broiler chickens, which indicated that MCE effectively suppressed the intestinal inflammatory responses induced by LPS challenge. Similar study has reported that pretreatment with MCE dramatically decreased the elevated mRNA expression of pro-inflammatory cytokines, such as IL-1α, IL-1β and IL-8 in porcine alveolar macrophages induced by Glaesserella parasuis (15). Previous research also found that sanguinarine and chelerythrine are responsible for the anti-microbial, anti-inflammatory and immunomodulatory properties of Macleaya cordata (40). Sanguinarine and chelerythrine displayed anti-inflammatory activity by promoting nuclear translocation of the glucocorticoid receptor and inhibiting activation of NF-κB (14, 15).
Staphylococcus can secrete various toxins and pro-inflammatory factors, alter the junctions between epithelial cells, disrupt the intestinal barrier, and increase intestinal permeability (41, 42). Moreover, Staphylococcus has strong drug resistance and can survive inside a variety of immune cells and enter the blood, causing infections of the gut, lung, and other tissues (43–45). Actinobacteriota are Gram-positive pathogens with high base pair of G+C, such as Corynebacterium and Nocardia (46). Streptococcus can produce streptolysin O, a pore-forming toxin that can influence the intestinal health (47). The LefSe results demonstrated that Staphylococcus, Actinobacteriota and Streptococcus were the taxonomic biomarkers in broiler chickens challenged with LPS, suggesting that the stimulation of LPS can lead to an increase in the relative abundance of potentially harmful bacteria in the intestine of broiler chickens. Blautia can use glucose to produce acetic acid, succinic acid, and lactic acid, and increase the content of volatile fatty acids (48). Besides, it can use carbon dioxide, carbon monoxide, hydrogen, and cellulose that cannot be used by the host as an energy source to regulate intestinal pH and maintain intestinal homeostasis (49). The LefSe results showed that birds in the MCE group has higher relative abundance of Blautia compared with other other groups, which may be related to the alleviation of intestinal inflammation and improvement of intestinal morphology.
Firmicutes are the dominant phylum of bacteria in broiler chickens. It has been reported that mice colonized with microbiota abundant in Firmicutes from healthy human fecal samples exhibited downregulation of the TH17 pathway and colonic inflammation (50). In this study, MCE supplementation increased the relative abundance of Firmicutes, which was similar to the studies of Song et al. (19) in a necrotic enteritis model and Wang et al. (51) in a chronic heat stress model. Lactobacillus can inhibit the activity of pathogenic bacteria (such as Escherichia coli and Staphylococcus aureus) and regulate the activity of immune cells and epithelial cells, thereby improving host immunity and intestinal barrier function (52). The present study showed that MCE supplementation increased the relative abundance of Lactobacillus. This was in agreement with the findings observed in mice, where MCE administration alleviated the decreased Lactobacillus population induced by heat stress (53). Meanwhile, dietary MCE supplementation can increase the abundance of Lactobacillus and adjust the intestinal pH, which is beneficial to the intestinal luminal environment in early-weaned piglets (13). Actinobacteriota, Peptostretococcaceae and Rhodococcus can promote inflammation by releasing pathogenic toxins such as diphtheria toxin (54). It has been reported that Actinobacteriota is highly associated with ulcerative colitis, Crohn’s disease, immunodeficiency, and other diseases (55). Our study showed that MCE could alleviate the increase in the relative abundance of Actinobacteriota, Peptostretococcaceae and Rhodococcus caused by LPS, thereby suppressing intestinal inflammation.
The results of differential metabolite analysis and KEGG enrichment analysis between the MCE+LPS and LPS groups showed that the different metabolites were mainly enriched in Lipid metabolism, Nucleotide metabolism, Amino acid metabolism, and Membrane transport. In Amino acid metabolism pathway, the MCE+LPS group significantly increased the levels of L-aspartic acid, L-glutamate, L-serine, β-alanine, and 5-Hydroxy-L-tryptophan compared with the LPS group. L-aspartic acid can inhibit the formation of cell membranes in Staphylococcus aureus, and regulate leukocyte phagocytosis and the immune response (56, 57). In addition, it can promote the entry of argininosuccinic acid into the tricarboxylic acid cycle and enhance cell survival (58). L-glutamate is the precursor of glutathione and plays an antioxidant role. It is also the precursor of γ-aminobutyric acid (GABA), which acts as a signaling molecule in brain cells, islet cells and intestinal cells. Moreover, glutamate is the only amino acid that can stimulate afferent gastric vagal nerves (59). L-serine can bind to pyruvate kinase to produce pyruvate and affect glucose metabolism, and it is a necessary amino acid for ceramide synthesis (60). In addition, 5-Hydroxy-L-tryptophan can enhance glucose and lipid metabolism and stimulate the proliferation of T cells and B lymphocytes through the synthesis of 5-hydroxytryptamine (61–64). β-alanine is the rate-limiting precursor for the synthesis of carnosine synthesis, and it can exert antioxidant and neuroprotective effects by regulating carnosine synthesis (65–67). These results suggest that MCE supplementation can regulate the levels of related metabolites and alter amino acid metabolism.
In the present study, hypoxanthine, uracil and deoxyguanosine are enriched in the nucleotide metabolism pathway. Hypoxanthine produces ROS during metabolism, which may cause oxidative stress, trigger caspase-3-induced apoptosis, and increase inflammation (68). Uracil is formed by mismatch due to spontaneous deamination of cytosine (69). Deoxyguanosine and pseudouridine are the biomarkers for oxidative DNA damage and prostate cancer, respectively (70). In this study, we found that dietary MCE supplementation could decrease the levels of these metabolites and regulate nucleotide metabolism. In addition, our study showed that 13-(S)-HODPE, PC and PE were key metabolites in lipid metabolism. It is reported that 13-(S)-HODPE can increase the production of ROS and promote the expression of apoptotic genes such as caspase-3 and p21, thus promoting cell apoptosis (71). The present study showed that MCE decreased the level of 13-(S)-HODPE, suggesting that MCE may inhibit oxidant stress by reducing the 13-(S)-HODPE level. The present study demonstrated that MCE supplementation significantly reduced the level of 13-(S)-HODPE. PC and PE are the most abundant phospholipids in all mammalian cell membranes, and the ratio of PC to PE is a key factor for low-density lipoprotein metabolism and energy metabolism in cellular organelles, and it plays an important role in gut and liver health (72). This study showed that MCE supplementation decreased the levels of both PC and PE, suggesting that MCE supplementation could regulate the lipid metabolic pathway and participate in the regulation of gut inflammation.
The results of Spearman correlation analysis of gut microbiota and plasma metabolites showed that the relative abundance of Firmicutes was positively correlated with the level of 2-hydroxycinnamic acid, a well-known antioxidant (73, 74), suggesting that Firmicutes could exert antioxidant function by increasing the level of 2-Hydroxycinnamic acid. Corynebacterium plays a pathogenic role by secreting phospholipase D (PLD) and diphtheria toxin, and PLD can activate NF-κB in epithelial cells, release inflammatory factors such as IL-6, and increase vascular permeability (75). In addition, Corynebacterium is closely associated with inflammatory diseases, such as bacteriemia and endocarditis (76). Our results showed that the relative abundance of Corynebacterium was negatively correlated with (+/-)-tryptophan and myo-inositol and positively correlated with inosine, suggesting that Corynebacterium could alter the levels of related metabolites, thereby regulating amino acid and nucleotide metabolic pathways.
Conclusion
In conclusion, dietary MCE supplementation at 400 mg/kg benefited the growth performance and alleviated the intestinal injury in broiler chickens challenged with LPS, which might be closely related to the modulation of gut microbiota and plasma metabolites. MCE supplementation could increase the relative abundance of potentially probiotic bacteria (such as Blautia and Lactobacillus) and decrease the relative abundance of potentially pathogenic bacteria (such as Actinobacteriota, Peptostretococcaceae, and Rhodococcus) in the gut. Moreover, MCE supplementation could alter the composition of metabolites and regulate many metabolic pathways, mainly including Lipid metabolism, Amino acid metabolism, and Nucleotide metabolism in broiler chickens. This study provides a valuable reference for nutritional regulation to prevent the gut damage induced by immune stress in broiler chickens.
Data availability statement
The original contributions presented in the study are publicly available. This data can be found here: Sequence Read Archive of NCBI, accession number PRJNA1087016.
Ethics statement
The animal study was approved by Animal Care and Use Committee of Qingdao Agricultural University (No. DKY20220513). The study was conducted in accordance with the local legislation and institutional requirements.
Author contributions
XW: Data curation, Investigation, Methodology, Writing – original draft. TZ: Data curation, Investigation, Methodology, Writing – review & editing. WL: Project administration, Supervision, Writing – review & editing. MZ: Project administration, Supervision, Writing – review & editing. LZ: Methodology, Software, Writing – review & editing. NW: Methodology, Software, Writing – review & editing. XZ: Methodology, Software, Writing – review & editing. BZ: Funding acquisition, Investigation, Project administration, Supervision, Writing – review & editing.
Funding
The author(s) declare financial support was received for the research, authorship, and/or publication of this article. This project was supported by the National Natural Science Foundation of China (32102580), the Natural Science Foundation of Shandong Province (ZR2020QC181), the Young Innovation Team Development Plan of Higher Education of Shandong Province (2023KJ166), the Doctoral Science Research Startup Funding of Qingdao Agricultural University (663/1120008), and the Open Project of State Key Laboratory of Animal Nutrition.
Conflict of interest
The authors declare that the research was conducted in the absence of any commercial or financial relationships that could be construed as a potential conflict of interest.
Publisher’s note
All claims expressed in this article are solely those of the authors and do not necessarily represent those of their affiliated organizations, or those of the publisher, the editors and the reviewers. Any product that may be evaluated in this article, or claim that may be made by its manufacturer, is not guaranteed or endorsed by the publisher.
Abbreviations
MCE, Macleaya cordata extract; LPS, lipopolysaccharide; ADG, average daily gain; ADFI, average daily feed intake; F/G, the ratio of feed to gain; T-AOC, total antioxidant capacity; CAT, catalase; GSH-Px, glutathione peroxidase; MDA, malondialdehyde; SOD, superoxide dismutase; VCR, villus height-to-crypt depth ratio; IL, interleukin; TNF-α, tumor necrosis factor alpha; IFN-γ, interferon γ; iNOS, inducible nitric oxide synthase; GAPDH, glyceraldehyde-3-phosphate dehydrogenase; SEM, standard error of the mean; LDA, linear discriminant analysis; LEfSe, linear discriminant analysis effect size; QC, quality control; PCA, principal component analysis; PLS-DA, partial least squares-discriminant analysis; TXB2, thromboxane B2; ROS, reactive oxygen species; NF-κB, nuclear factor kappa B; PLD, phospholipase D; GABA, precursor of γ-aminobutyric acid; PC, phosphatidylcholine; PE, phosphatidylethanolamine; M/Z, mass to charge ratio; FC, fold change; VIP, variable important in projection; 20-Hydroxy-PGF2a, 20-hydroxy Prostaglandin F2a.
References
1. Wyns H, Plessers E, De Backer P, Meyer E, Croubels S. In vivo porcine lipopolysaccharide inflammation models to study immunomodulation of drugs. Vet Immunol Immunopathol. (2015) 166:58–69. doi: 10.1016/j.vetimm.2015.06.001
2. Mani V, Weber TE, Baumgard LH, Gabler NK. Growth and development symposium: endotoxin, inflammation, and intestinal function in livestock. J Anim Sci. (2012) 90:1452–65. doi: 10.2527/jas.2011-4627
3. Wu XX, Huang X, Chen RR, Li T, Ye HJ, Xie W, et al. Paeoniflorin prevents intestinal barrier disruption and inhibits lipopolysaccharide (LPS)-induced inflammation in Caco-2 cell monolayers. Inflammation. (2019) 42:2215–25. doi: 10.1007/s10753-019-01085-z
4. Niu XT, Sun C, Zhao L, Chen XM, Wang GQ, Li MY. The major role of glucocorticoid receptor (GR) in astaxanthin alleviates immune stress in Channa argus lymphocyte. Aquaculture. (2024) 584:740637. doi: 10.1016/j.aquaculture.2024.740637
5. Puris E, Kouřil Š, Najdekr L, Auriola S, Loppi S, Korhonen P, et al. Metabolomic, lipidomic and proteomic characterisation of lipopolysaccharide-induced inflammation mouse model. Neuroscience. (2022) 496:165–78. doi: 10.1016/j.neuroscience.2022.05.030
6. Lv Z, Fan H, Gao M, Zhang X, Li G, Fan Y, et al. The accessible chromatin landscape of lipopolysaccharide-induced systemic inflammatory response identifying epigenome signatures and transcription regulatory networks in chickens. Int J Biol Macromol. (2024) 266:131136. doi: 10.1016/j.ijbiomac.2024.131136
7. Yu Y, Li Q, Zeng X, Xu Y, Jin K, Liu J, et al. Effects of probiotics on the growth performance, antioxidant functions, immune responses, and caecal microbiota of broilers challenged by lipopolysaccharide. Front Vet Sci. (2022) 9:846649. doi: 10.3389/fvets.2022.846649
8. Yu Z, Zhao L, Zhao JL, Xu W, Guo Z, Zhang AZ, et al. Dietary Taraxacum mongolicum polysaccharide ameliorates the growth, immune response, and antioxidant status in association with NF-κB, Nrf2 and TOR in Jian carp (Cyprinus carpio var. Jian). Aquaculture. (2022) 547:737522. doi: 10.1016/j.aquaculture.2021.737522
9. Yang L, Liu G, Liang X, Wang M, Zhu X, Luo Y, et al. Effects of berberine on the growth performance, antioxidative capacity and immune response to lipopolysaccharide challenge in broilers. Anim Sci J. (2019) 90:1229–38. doi: 10.1111/asj.13255
10. Li B, Zhang JQ, Han XG, Wang ZL, Xu YY, Miao JF. Macleaya cordata helps improve the growth-promoting effect of chlortetracycline on broiler chickens. J Zhejiang Univ Sci B. (2018) 19:776–84. doi: 10.1631/jzus.B1700435
11. Lei F, Liu X, Huang H, Fu S, Zou K, Zhang S, et al. The macleaya cordata symbiont: revealing the effects of plant niches and alkaloids on the bacterial community. Front Microbiol. (2021) 12:681210. doi: 10.3389/fmicb.2021.681210
12. Lee KW, Kim JS, Oh ST, Kang CW, An BK. Effects of dietary sanguinarine on growth performance, relative organ weight, cecal microflora, serum cholesterol level and meat quality in broiler chickens. J Poult. Sci. (2015) 52:15–22. doi: 10.2141/jpsa.0140073
13. Chen J, Kang B, Zhao Y, Yao K, Fu C. Effects of natural dietary supplementation with Macleaya cordata extract containing sanguinarine on growth performance and gut health of early-weaned piglets. J Anim Physiol Anim Nutr (Berl). (2018) 102:1666–74. doi: 10.1111/jpn.12976
14. Dvorák Z, Vrzal R, Maurel P, Ulrichová J. Differential effects of selected natural compounds with anti-inflammatory activity on the glucocorticoid receptor and NF-kappaB in HeLa cells. Chem Biol Interact. (2006) 159:117–28. doi: 10.1016/j.cbi.2005.10.105
15. Zeng Z, Zhang H, Gui G, Luo J, Liu S. Macleaya cordata extract modulates inflammation via inhibition of the NF-κB and MAPK signaling pathways in porcine alveolar macrophages induced by Glaesserella parasuis. Can J Vet Res. (2022) 86:254–60.
16. Lin L, Liu YC, Huang JL, Liu XB, Qing ZX, Zeng JG, et al. Medicinal plants of the genus Macleaya (Macleaya cordata, Macleaya microcarpa): A review of their phytochemistry, pharmacology, and toxicology. Phytother Res. (2018) 32:19–48. doi: 10.1002/ptr.5952
17. Liu ZY, Wang XL, Ou SQ, Hou DX, He JH. Sanguinarine modulate gut microbiome and intestinal morphology to enhance growth performance in broilers. PloS One. (2020) 15:e0234920. doi: 10.1371/journal.pone.0234920
18. Guan G, Ding S, Yin Y, Duraipandiyan V, Al-Dhabi NA, Liu G. Macleaya cordata extract alleviated oxidative stress and altered innate immune response in mice challenged with enterotoxigenic Escherichia coli. Sci China Life Sci. (2019) 62:1019–27. doi: 10.1007/s11427-018-9494-6
19. Song B, He J, Pan X, Kong L, Xiao C, Keerqin C, et al. Dietary Macleaya cordata extract supplementation improves the growth performance and gut health of broiler chickens with necrotic enteritis. J Anim Sci Biotechnol. (2023) 14:113. doi: 10.1186/s40104-023-00916-2
20. Guo S, Lei J, Liu L, Qu X, Li P, Liu X, et al. Effects of macleaya cordata extract on laying performance, egg quality, and serum indices in Xuefeng black-bone chicken. Poult Sci. (2021) 100:101031. doi: 10.1016/j.psj.2021.101031
21. Han D, Yang H, Li J, Zhang C, Ye L, Dong J, et al. Macleaya cordata extract improves growth performance, immune responses and anti-inflammatory capacity in neonatal piglets. Vet Microbiol. (2024) 293:110090. doi: 10.1016/j.vetmic.2024.110090
22. Takiishi T, Fenero CIM, Câmara NOS. Intestinal barrier and gut microbiota: Shaping our immune responses throughout life. Tissue Barriers. (2017) 5:e1373208. doi: 10.1080/21688370.2017.1373208
23. Etienne-Mesmin L, Chassaing B, Desvaux M, De Paepe K, Gresse R, Sauvaitre T, et al. Experimental models to study intestinal microbes-mucus interactions in health and disease. FEMS Microbiol Rev. (2019) 43:457–89. doi: 10.1093/femsre/fuz013
24. Clavijo V, Flórez MJV. The gastrointestinal microbiome and its association with the control of pathogens in broiler chicken production: A review. Poult Sci. (2018) 97:1006–21. doi: 10.3382/ps/pex359
25. Brisbin JT, Gong J, Sharif S. Interactions between commensal bacteria and the gut-associated immune system of the chicken. Anim Health Res Rev. (2008) 9:101–10. doi: 10.1017/S146625230800145X
26. Larissa P, Breno CB, Ricardo M, Jean F, Mariana C, Luiz F, et al. Effect of sanguinarine in drinking water on Salmonella control and the expression of immune cells in peripheral blood and intestinal mucosa of broilers. J Appl Poultry Res. (2013) 22:430–8. doi: 10.3382/japr.2012-00649
27. Huang P, Zhang Y, Xiao K, Jiang F, Wang H, Tang D, et al. The chicken gut metagenome and the modulatory effects of plant-derived benzylisoquinoline alkaloids. Microbiome. (2018) 6:211. doi: 10.1186/s40168-018-0590-5
28. Liu Y, Li Y, Niu J, Liu H, Jiao N, Huang L, et al. Effects of dietary macleaya cordata extract containing isoquinoline alkaloids supplementation as an alternative to antibiotics in the diets on growth performance and liver health of broiler chickens. Front Vet Sci. (2022) 9:950174. doi: 10.3389/fvets.2022.950174
29. Drsata J, Ulrichová J, Walterová D. Sanguinarine and chelerythrine as inhibitors of aromatic amino acid decarboxylase. J Enzyme Inhib. (1996) 10:231–7. doi: 10.3109/14756369609036530
31. Zhu F, Zhang B, Li J, Zhu L. Effects of fermented feed on growth performance, immune response, and antioxidant capacity in laying hen chicks and the underlying molecular mechanism involving nuclear factor-κB. Poult Sci. (2020) 99:2573–80. doi: 10.1016/j.psj.2019.12.044
32. Nandi A, Yan LJ, Jana CK, Das N. Role of catalase in oxidative stress- and age-associated degenerative diseases. Oxid Med Cell Longev. (2019) 2019:9613090. doi: 10.1155/2019/9613090
33. Du JH, Xu MY, Wang Y, Lei Z, Yu Z, Li MY. Evaluation of Taraxacum mongolicum flavonoids in diets for Channa argus based on growth performance, immune responses, apoptosis and antioxidant defense system under lipopolysaccharide stress. Fish Shellfish Immunol. (2022) 131:1224–33. doi: 10.1016/j.fsi.2022.11.034
34. Zheng Z, Zheng Y, Liang X, Xue G, Wu H. Sanguinarine enhances the integrity of the blood-milk barrier and inhibits oxidative stress in lipopolysaccharide-stimulated mastitis. Cells. (2022) 11:3588. doi: 10.3390/cells11223658
35. Liu Y, Jiao R, Ma ZG, Liu W, Wu QQ, Yang Z, et al. Sanguinarine inhibits angiotensin II-induced apoptosis in H9c2 cardiac cells via restoring reactive oxygen species-mediated decreases in the mitochondrial membrane potential. Mol Med Rep. (2015) 12:3400–8. doi: 10.3892/mmr.2015.3841
36. Hernandez-Santana YE, Giannoudaki E, Leon G, Lucitt MB, Walsh PT. Current perspectives on the interleukin-1 family as targets for inflammatory disease. Eur J Immunol. (2019) 49:1306–20. doi: 10.1002/eji.201848056
37. Tanaka T, Narazaki M, Kishimoto T. IL-6 in inflammation, immunity, and disease. Cold Spring Harb Perspect Biol. (2014) 6:a016295. doi: 10.1101/cshperspect.a016295
38. Ghasemi H, Ghazanfari T, Yaraee R, Faghihzadeh S, Hassan ZM. Roles of IL-8 in ocular inflammations: a review. Ocul Immunol Inflammation. (2011) 19:401–12. doi: 10.3109/09273948.2011.618902
39. McGeachy MJ, Cua DJ, Gaffen SL. The IL-17 family of cytokines in health and disease. Immunity. (2019) 50:892–906. doi: 10.1016/j.immuni.2019.03.021
40. Šimánek V, Vespalec R, Sedo A, Ulrichová J, Vičar JJC. Quaternary benzo[c]phenanthridine alkaloids - biological activities. Chem Probes Biol. (2003) 36:245–54.
41. Kwak YK, Vikström E, Magnusson KE, Vécsey-Semjén B, Colque-Navarro P, Möllby R. The Staphylococcus aureus alpha-toxin perturbs the barrier function in Caco-2 epithelial cell monolayers by altering junctional integrity. Infect Immun. (2012) 80:1670–80. doi: 10.1128/IAI.00001-12
42. Kim MH, Liu W, Borjesson DL, Curry FR, Miller LS, Cheung AL, et al. Dynamics of neutrophil infiltration during cutaneous wound healing and infection using fluorescence imaging. J Invest Dermatol. (2008) 128:1812–20. doi: 10.1038/sj.jid.5701223
43. Raineri EJM, Altulea D, van Dijl JM. Staphylococcal trafficking and infection-from 'nose to gut' and back. FEMS Microbiol Rev. (2022) 46:fuab041. doi: 10.1093/femsre/fuab041
44. Horn J, Stelzner K, Rudel T, Fraunholz M. Inside job: Staphylococcus aureus host-pathogen interactions. Int J Med Microbiol. (2018) 308:607–24. doi: 10.1016/j.ijmm.2017.11.009
45. Balraadjsing PP, de Jong EC, van Wamel WJB, Zaat SAJ. Dendritic cells internalize staphylococcus aureus more efficiently than staphylococcus epidermidis, but do not differ in induction of antigen-specific T cell proliferation. Microorganisms. (2019) 8:19. doi: 10.3390/microorganisms8010019
46. Barka EA, Vatsa P, Sanchez L, Gaveau-Vaillant N, Jacquard C, Meier-Kolthoff JP, et al. Taxonomy, physiology, and natural products of actinobacteria. Microbiol Mol Biol Rev. (2016) 80:1–43. doi: 10.1128/MMBR.00019-15
47. Langshaw EL, Reynolds S, Ozberk V, Dooley J, Calcutt A, Zaman M, et al. Streptolysin O deficiency in Streptococcus pyogenes M1T1 covR/S mutant strain attenuates virulence in in vitro and in vivo infection models. mBio. (2023) 14:e0348822. doi: 10.1128/mbio.03488-22
48. Tong X, Xu J, Lian F, Yu X, Zhao Y, Xu L, et al. Structural alteration of gut microbiota during the amelioration of human type 2 diabetes with hyperlipidemia by metformin and a traditional chinese herbal formula: a multicenter, randomized, open label clinical trial. mBio. (2018) 9:e02392–17. doi: 10.1128/mBio.02392-17
49. Liu C, Finegold SM, Song Y, Lawson PA. Reclassification of Clostridium coccoides, Ruminococcus hansenii, Ruminococcus hydrogenotrophicus, Ruminococcus luti, Ruminococcus productus and Ruminococcus schinkii as Blautia coccoides gen. nov., comb. nov., Blautia hansenii comb. nov., Blautia hydrogenotrophica comb. nov., Blautia luti comb. nov., Blautia producta comb. nov., Blautia schinkii comb. nov. and description of Blautia wexlerae sp. nov., isolated from human faeces. Int J Syst Evol Microbiol. (2008) 58:1896–902. doi: 10.1099/ijs.0.65208-0
50. Natividad JM, Pinto-Sanchez MI, Galipeau HJ, Jury J, Jordana M, Reinisch W, et al. Ecobiotherapy rich in firmicutes decreases susceptibility to colitis in a humanized gnotobiotic mouse model. Inflammation Bowel Dis. (2015) 21:1883–93. doi: 10.1097/MIB.0000000000000422
51. Wang M, Zhang J, Huang X, Liu Y, Zeng J. Effects of dietary Macleaya cordata extract on growth performance, biochemical indices, and intestinal microbiota of yellow-feathered broilers subjected to chronic heat stress. Anim (Basel). (2022) 12:2197. doi: 10.3390/ani12172197
52. Scillato M, Spitale A, Mongelli G, Privitera GF, Mangano K, Cianci A, et al. Antimicrobial properties of Lactobacillus cell-free supernatants against multidrug-resistant urogenital pathogens. Microbiologyopen. (2021) 10:e1173. doi: 10.1002/mbo3.1173
53. Wang M, Huang X, Liu Y, Zeng J. Effects of Macleaya cordata extract on blood biochemical indices and intestinal flora in heat-stressed mice. Anim (Basel). (2022) 12:2589. doi: 10.3390/ani12192589
54. Hadfield TL, McEvoy P, Polotsky Y, Tzinserling VA, Yakovlev AA. The pathology of diphtheria. J Infect Dis. (2000) 181Suppl 1:S116–120. doi: 10.1086/315551
55. Matulionyte R, Rohner P, Uçkay I, Lew D, Garbino J. Secular trends of nocardia infection over 15 years in a tertiary care hospital. J Clin Pathol. (2004) 57:807–12. doi: 10.1136/jcp.2004.016923
56. Wu GY, Brosnan JT. Macrophages can convert citrulline into arginine. Biochem J. (1992) 281:45–8. doi: 10.1042/bj2810045
57. Lin L, Zhang P, Chen X, Hu W, Abdel-Samie MA, Li C, et al. Inhibition of Staphylococcus aureus biofilms by poly-L-aspartic acid nanoparticles loaded with Litsea cubeba essential oil. Int J Biol Macromol. (2023) 242:124904. doi: 10.1016/j.ijbiomac.2023.124904
58. Yang MJ, Cheng ZX, Jiang M, Zeng ZH, Peng B, Peng XX, et al. Boosted TCA cycle enhances survival of zebrafish to Vibrio alginolyticus infection. Virulence. (2018) 9:634–44. doi: 10.1080/21505594.2017.1423188
59. Brosnan JT, Brosnan ME. Glutamate: a truly functional amino acid. Amino Acids. (2013) 45:413–8. doi: 10.1007/s00726-012-1280-4
60. Maugard M, Vigneron PA, Bolaños JP, Bonvento G. L-Serine links metabolism with neurotransmission. Prog Neurobiol. (2021) 197:101896. doi: 10.1016/j.pneurobio.2020.101896
61. Zhang Z, Qin S, Wang Y, Liang H, Wang R, Li F. L-ascorbic acid could ameliorate the damage of myocardial microvascular endothelial cell caused by hypoxia-reoxygenation via targeting HMGB1. J Bioenerg Biomembr. (2023) 55:115–22. doi: 10.1007/s10863-023-09962-x
62. León-Ponte M, Ahern GP, O'Connell PJ. Serotonin provides an accessory signal to enhance T-cell activation by signaling through the 5-HT7 receptor. Blood. (2007) 109:3139–46. doi: 10.1182/blood-2006-10-052787
63. Hernández-Castellano LE, Özçelik R, Hernandez LL, Bruckmaier RM. Short communication: Supplementation of colostrum and milk with 5-hydroxy-l-tryptophan affects immune factors but not growth performance in newborn calves. J Dairy Sci. (2018) 101:794–800. doi: 10.3168/jds.2017-13501
64. Hernández-Castellano LE, Hernandez LL, Sauerwein H, Bruckmaier RM. Endocrine and metabolic changes in transition dairy cows are affected by prepartum infusions of a serotonin precursor. J Dairy Sci. (2017) 100:5050–7. doi: 10.3168/jds.2016-12441
65. Hoffman JR, Zuckerman A, Ram O, Sadot O, Stout JR, Ostfeld I, et al. Behavioral and inflammatory response in animals exposed to a low-pressure blast wave and supplemented with β-alanine. Amino Acids. (2017) 49:871–86. doi: 10.1007/s00726-017-2383-8
66. Hoffman JR, Varanoske A, Stout JR. Effects of β-Alanine supplementation on carnosine elevation and physiological performance. Adv Food Nutr Res. (2018) 84:183–206. doi: 10.1016/bs.afnr.2017.12.003
67. Boldyrev AA, Aldini G, Derave W. Physiology and pathophysiology of carnosine. Physiol Rev. (2013) 93:1803–45. doi: 10.1152/physrev.00039.2012
68. Zhou X, Zhang B, Zhao X, Lin Y, Wang J, Wang X, et al. Chlorogenic acid supplementation ameliorates hyperuricemia, relieves renal inflammation, and modulates intestinal homeostasis. Food Funct. (2021) 12:5637–49. doi: 10.1039/D0FO03199B
69. Kavli B, Otterlei M, Slupphaug G, Krokan HE. Uracil in DNA–general mutagen, but normal intermediate in acquired immunity. DNA Repair (Amst). (2007) 6:505–16. doi: 10.1016/j.dnarep.2006.10.014
70. Stockert JA, Gupta A, Herzog B, Yadav SS, Tewari AK, Yadav KK. Predictive value of pseudouridine in prostate cancer. Am J Clin Exp Urol. (2019) 7:262–72.
71. Biswas P, Swaroop S, Dutta N, Arya A, Ghosh S, Dhabal S, et al. IL-13 and the hydroperoxy fatty acid 13(S)HpODE play crucial role in inducing an apoptotic pathway in cancer cells involving MAO-A/ROS/p53/p21 signaling axis. Free Radic Biol Med. (2023) 195:309–28. doi: 10.1016/j.freeradbiomed.2022.12.103
72. van der Veen JN, Kennelly JP, Wan S, Vance JE, Vance DE, Jacobs RL. The critical role of phosphatidylcholine and phosphatidylethanolamine metabolism in health and disease. Biochim Biophys Acta Biomembr. (2017) 1859:1558–72. doi: 10.1016/j.bbamem.2017.04.006
73. Sharma P, Singh R. Efficacy of trans-2-hydroxycinnamic Acid against trichlorfon-induced oxidative stress in wistar rats. Toxicol Int. (2012) 19:295–300. doi: 10.4103/0971-6580.103671
74. Rivera-Antonio A, Rosales-Hernández MC, Balbuena-Rebolledo I, Santiago-Quintana JM, Mendieta-Wejebe JE, Correa-Basurto J, et al. Myeloperoxidase inhibitory and antioxidant activities of (E)-2-Hydroxy-α-aminocinnamic acids obtained through microwave-assisted synthesis. Pharm (Basel). (2021) 14:513. doi: 10.3390/ph14060513
75. Hacker E, Antunes CA, Mattos-Guaraldi AL, Burkovski A, Tauch A. Corynebacterium ulcerans, an emerging human pathogen. Future Microbiol. (2016) 11:1191–208. doi: 10.2217/fmb-2016-0085
Keywords: Macleaya cordata extract, lipopolysaccharide, intestinal injury, gut microbiota, plasma metabolites, broiler chicken
Citation: Wang X, Zhang T, Li W, Zhang M, Zhao L, Wang N, Zhang X and Zhang B (2024) Dietary supplementation with Macleaya cordata extract alleviates intestinal injury in broiler chickens challenged with lipopolysaccharide by regulating gut microbiota and plasma metabolites. Front. Immunol. 15:1414869. doi: 10.3389/fimmu.2024.1414869
Received: 09 April 2024; Accepted: 08 July 2024;
Published: 19 July 2024.
Edited by:
Yaozhong Hu, Nankai University, ChinaReviewed by:
Lei Zhao, Heilongjiang Bayi Agricultural University, ChinaGuangxin Chen, Shanxi University, China
Liping Gan, Henan University of Technology, China
Copyright © 2024 Wang, Zhang, Li, Zhang, Zhao, Wang, Zhang and Zhang. This is an open-access article distributed under the terms of the Creative Commons Attribution License (CC BY). The use, distribution or reproduction in other forums is permitted, provided the original author(s) and the copyright owner(s) are credited and that the original publication in this journal is cited, in accordance with accepted academic practice. No use, distribution or reproduction is permitted which does not comply with these terms.
*Correspondence: Beibei Zhang, emhhbmdiZWliZWlAcWF1LmVkdS5jbg==