- Laboratory of Clinical Immunogenetics and Pharmacogenetics, Hirszfeld Institute of Immunology and Experimental Therapy, Polish Academy of Sciences, Wrocław, Poland
Gamma delta (γδ) T cells represent a unique and distinct population of lymphocytes that bridge the innate and adaptive immune responses. This functional duality positions them as one of the pivotal elements in the evolution and development of the human body’s defense mechanisms. This review aims to provide a comprehensive and in-depth overview of γδ T cells, covering their origins, development, classification, and functional roles in immunology. Special attention is given to their involvement in the pathogenesis of autoimmune and cancer-related diseases—areas that remain subjects of intensive research with many unanswered questions. Additionally, this article explores the therapeutic potential of γδ T cells, which hold promise as a novel approach to treating various difficult-to-manage diseases. The review also presents an analysis of the latest clinical studies utilizing γδ T cells, emphasizing their emerging role in modern medicine. The ultimate goal of this work is to offer a holistic perspective on the current state of research on γδ T cells and their prospective applications in immunotherapy and cancer treatment, highlighting their potential to become a groundbreaking tool in future medical interventions.
1 Introduction
The documented history of γδ T cells begins in the 1980s, with the observation of a novel T-cell receptor (TCR) chain (1, 2). In 1987, scientists described an associated CD3 molecule - an unknown heterodimer comprising a chain generated by γ genes and a second chain termed δ chain (3). For over three decades, γδ T cells have been the subject of investigation by numerous researchers in different contexts.
Γδ T cells represent the initial group of lymphocytes formed in the thymus during ontogeny in all vertebrate species (4). Double-negative (CD4-CD8-, DN) thymocytes, serving as progenitor cells, initiate the formation of both αβ and γδ chains during the second stage of their maturation pathway (DN2). Fully developed TCR γδ are present in the third stage of thymocyte maturation (DN3) and it is at this juncture that the differentiation occurs between the αβ and γδ T cell lineages (5, 6). Some γδ lymphocytes leaving the thymus have the ability to perform specific anti-infective defense, which allows for a quick reaction in the newborn’s first contact with pathogens when classic T lymphocytes still acquire their abilities (7). Mature γδ lymphocytes constitute up to 5% of the total T lymphocyte population in adult humans and are distributed not only in the blood and lymphoid organs but also in various tissues such as the skin, lung and intestinal epithelium, and liver (8).
The interest in γδ T cells may arise from the unique combination of characteristics associated with both innate and adaptive immunity, positioning them as an evolutionary link in the development of these two defense mechanisms (9). Moreover, in contrast to classical T cells, γδ T lymphocytes do not necessitate antigen processing by antigen-presenting cells (APCs) and exhibit the ability to recognize non-classical antigens, while they are not under the major histocompatibility complex (MHC) restriction during antigen presentation. Possibly the mere connection of the γδ TCR receptor with the recognized antigen is sufficient for activation. The specific resemblance between γδ cells and natural killer (NK) cells, both functionally and in terms of surface receptors, can be another aspect of interest. Recognizing these shared characteristics provides a substantive basis for the development of innovative therapies for highly diverse disorders targeting these specific cell subsets (10, 11). However, a gap in knowledge about γδ T cells still persists, and its closure has the potential to reshape modern immunology.
2 γδ T cells – a whole range of variability
2.1 Essential signaling in thymocyte development
The process of γδ T cell development is observed in the thymus of all jawed vertebrates during ontogeny. Initial cells in the thymus originate from the bone marrow. Under the influence of Notch 1 (N1) and Delta-like 4 (DL4) signals, they give rise to a common thymic progenitor cell line called “early thymic precursors” (ETPs), serving as the starting population for both αβ and γδ T cell lineages (12). ETPs represent the first stage of thymocyte development from a hematopoietic stem cell. They are double-negative (DN; CD4-CD8-) lineage (DN1) characterized by the absence of CD25- expression and the presence of CD44+ on their surface. Subsequent developmental stages of thymocytes DN2-DN4 are distinguished mainly based on the detection of differences in the expression of these two receptors. CD117 is considered to be a factor enabling the survival of double-negative thymocytes and the distinction of individual thymocyte stages. Especially in the case of DN2 (CD117+CD25+CD44+) and DN3 (CD117low/-CD25+CD44low) stages, the differentiation might be difficult due to their apparent homogeneity (13, 14).
A crucial moment in determining the fate of ETPs is the induction of IL2RA gene expression, which encodes for the CD25 protein located on the thymocytes surface. This process occurs during thymocytes transition from the DN1 to the DN2 stage and is associated with the initiation of TCR receptor formation and differentiation. Between the DN2 and DN3 stages, gene rearrangement encoding individual TCR chains begins, including the γδ chains as well as the conventional β and partially α chains. The choice of lymphocyte differentiation towards αβ or γδ T is not fully explained, but two theories have originally attempted to elucidate it: 1) the stochastic model and 2) the instructive model (12, 15). In the stochastic model, progenitor cells can create γδ TCR receptors by rearranging Tcrg and Tcrd or create pre-TCR by rearranging Tcrb. This model assumes that TCR is not paramount in determining cell fate, but each cell belongs to a predetermined group. The mechanisms governing this process are unknown, but cells that do not develop a TCR compatible with their fate die. The second model explaining the determination of ETPs fates involves TCR and the possibility of tightly controlled transition of pre-TCR into the αβ receptor, while the presence of γδ TCR forms γδ T cells (15). Results and observations from various scientific teams led to the proposal of another model involving signal strength (16). Specifically, the paramount signal appears to originate from the Notch receptor family while it has been demonstrated that its activity regulates the expression of genes such as Il2ra, Gata3, Bcll1b, Notch3, and Trca. However, the activity of these genes is detected at various stages of thymocyte maturation (17). On the other hand, Notch signaling in thymocyte fate determination relies on cooperation with one of its ligands from the DL or Jagged family (Figure 1). The signal from DL4 simultaneously supports the generation of both αβ and γδ chains. A similar effect is observed with the combination of the Jagged2 ligand and Notch1 receptor, but this signal is weaker than the Jagged2 and Notch3 combination, which, in turn, promotes the formation of γδ chains and represses β chain differentiation (18). An interesting observation is that in a mouse model, the determination of T-cell fate, depending on Notch activity and signaling strength, is opposite to that in humans (19).
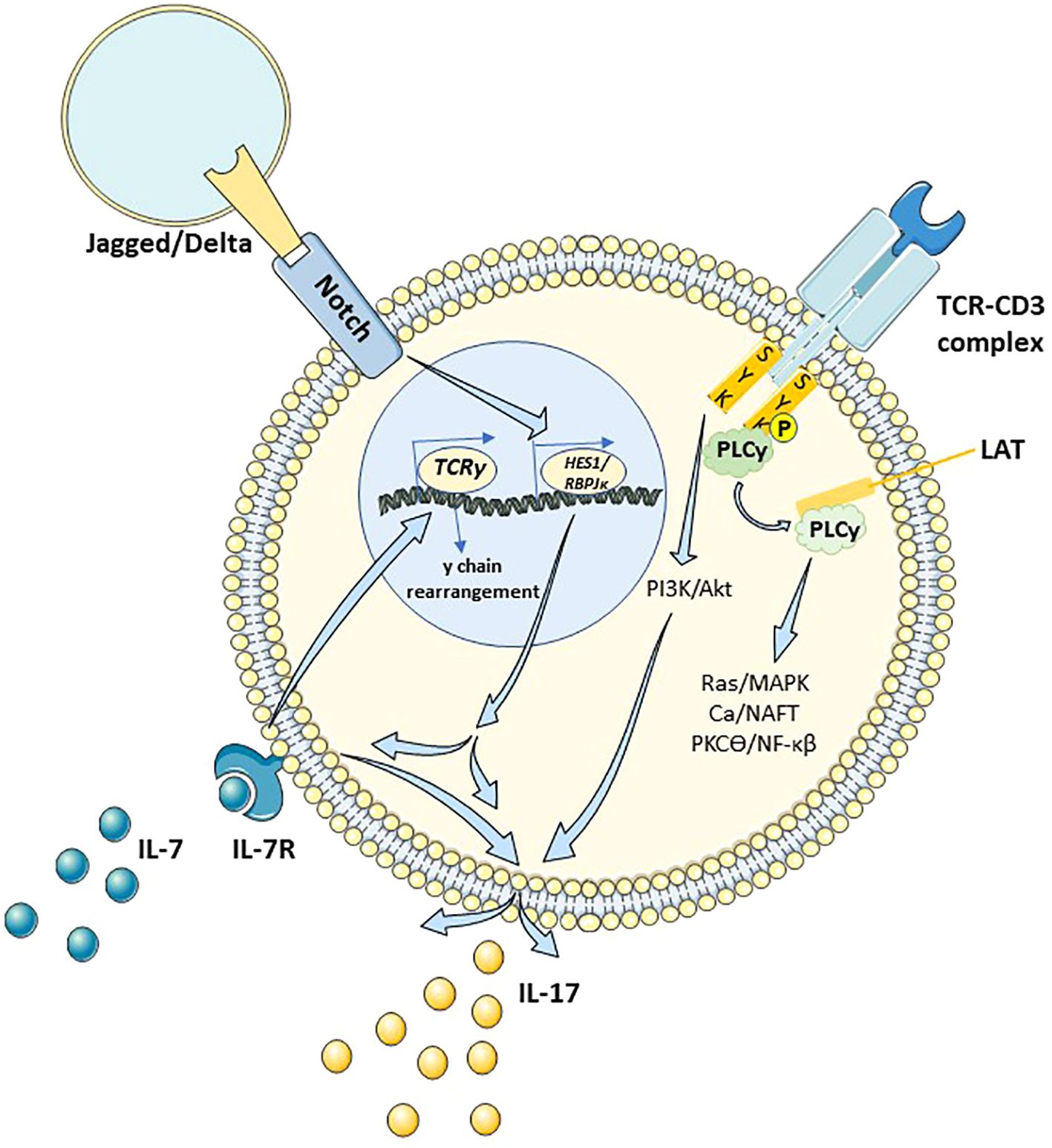
Figure 1. Schematic representation of γδ T cell and possible mechanisms involved in the regulation of its activity via engagement of TCR and Notch signaling pathways as well as IL-7 and IL-17 production. More detailed description is given within the manuscript text. TCRγ, T cell receptor gene γ chain; HES1, hairy and enhancer of split-1; RBPJκ, recombination signal-binding protein of immunoglobulin Jκ; Syk, spleen tyrosine kinase; PLCγ, phospholipase C-γ; LAT, linker for activation of T cells; MAPK, mitogen-activated protein kinases; NFAT, nuclear factor of activated T-cells; PKCθ, protein kinase C theta; NF-κB ,nuclear factor kappa B; IL-, interleukin 7; IL-7R, interleukin 7 receptor; IL-17, interleukin 17.
Furthermore, studies on mice have shown that the transition between successive stages of thymocyte development (from DN2 to DN3) requires not only Notch signals but also the involvement of interleukin 7 (IL-7) (20) (Figure 1). IL-7 and its receptor IL-7R are crucial for the development of γδ T cells and other lymphocyte lineages (21). Mice lacking IL-7R experienced a halt in the rearrangement of γ chain V-J segments, leading to the absence of γδ T cells (22, 23). Additionally, high levels of IL-7Rα and IL-7 contributes to the growth of the γδ T cell subpopulation capable of producing IL-17 (γδ17). Referring to the γδ17 T cell subpopulation again, it is worth mentioning the Notch signaling and its action through the hairy and enhancer of split-1 (HES1) transcription factor (24) or recombination signal-binding protein of immunoglobulin Jκ (RBPJκ) (25). Both pathways are independent of each other, and the inhibition of one pathway does not affect the intrathymic differentiation of γδ17 T cells by the other pathway (7). However, it is not the only signaling pathway supporting the generation of γδ17 T cells. Another pathway involves the spleen tyrosine kinase (Syk) receptor. On one hand, the signalosome called the Linker for activation of T cells (Lat), the main target for Syk in γδ cells, leads to the activation of the mitogen-activated protein kinases (MAP) cascade, as well as pathways involving nuclear factor of activated T-cells (NFAT) and the nuclear factor kappa B (NF-κB), influencing the induction of γδ T cells (26). On the other hand, there is a Lat-independent pathway where Syk directly interacts with the regulatory subunit of Phosphoinositide 3-kinase (PI3K), activating the PI3K/Akt axis (26) (Figure 1). Signals of moderate strength from this pathway are also a crucial element in the differentiation and maintenance of activity in γδ T cells producing IL-17 through the maintenance of the expression of key regulators such as retinoid-related orphan receptor (RORγt) and musculoaponeurotic fibrosarcoma oncogene homolog (c-Maf) (27, 28). It has been shown that both overactivation and downregulation of the PI3K/Akt axis reduce the development of γδ17 T cells (28) (Figure 1).
The process of ETPs fate determination in γδ T cells is highly complex, and despite numerous studies, there are still unresolved gaps in the signaling network, leaving a wide field for further research.
2.2 Creation waves
In the human fetal life, the second site for γδ T lymphocyte generation is the liver. This subset of cells generated in the fetal liver constitutes approximately 30% of all CD3+ lymphocytes. These cells are described as CD4+ and simultaneously CD8-, which is atypical for cells originating from blood and thymus. Studies in mice have shown that γδ cells in the fetal liver can undergo their own thymus-independent developmental cycle. It has been found that murine liver hematopoietic progenitor cells, described as Lin-negative and Sca-1 and Mac-1 positive (LSM), differentiate into precursors of γδ T cells or γδ TCR cells under experimental conditions. Interestingly, these cells are not capable of producing IL-17 but produce IFN-γ (29).Additionally, a consistent motif Vγ9 has been observed in human liver γδ cells along with a preferred chain of Vδ2 and Vδ3. The Vγ9Vδ2 subset was detected in the fetal liver as early as 5-6 weeks of gestation, becoming the dominant subpopulation around 20-30 weeks (8, 30).
Γδ T lymphocytes are produced in distinct waves during murine fetal life. The model of waves is based on observable changes in the functions of γδ cells, such as cytokine secretion or TCR γδ characteristics. The model established for murine development has been attempted to be applied to human fetal development (31). The first wave in humans is considered to be the pre-thymic subpopulation Vγ9Vδ2 formed in the fetal liver (5-6 weeks of gestation) (30). This assumption is supported by the fact that human thymus organogenesis begins around 6-7 weeks of gestation, and the first γδ thymocytes were detected around 10-11 weeks of gestation, indicating the pre-thymic origin of the first wave. Fetal γδ T cells of thymic origin arise in a slightly shifted wave compared to the liver wave. Fetal thymic γδ T cells mainly contain the Vδ2 chain with low variability. Additionally, fetal thymic cells, both Vδ2 and present in small amounts Vδ1, are effector-programmed and possess characteristic fetal CD3γ and CD3δ sequences, absent in postnatal γδ cell waves. While Vγ9-Vδ2+ cells dominate in the fetal thymus, the Vγ9Vδ2 cell group is the predominant subpopulation in fetal peripheral blood. However, it is gradually replaced by the subsequent wave of Vδ1 cells, which peak in umbilical cord blood at birth (32).
2.3 Rearrangement of TCR γδ, mandatory classification and γδ selection
The rearrangement of the TCR γδ initiates in the thymus at the DN2 stage of thymocytes, marked by the presence of CD44 and CD25, likely occurring in parallel with Tcrb rearrangement but preceding the rearrangement of the α chain of the classical TCR (33). The primary objective of all TCR receptor groups is the capacity for efficient antigen recognition, particularly those potentially harmful to the body. This necessitates a broad repertoire of receptor sequences capable of antigen recognition. The prevailing opinion is that, at any given time, the human body harbors a greater diversity of TCRs than the quantity of circulating lymphocytes (34). Several mechanisms ensure such diversity. Firstly, all genes encoding TCR receptor chains have a segmental structure, consisting of variable (V), diversity (D), joining (J), and constant (C) regions, with Tcra and Tcrg genes lacking D segments. The segments are randomly combined by excising the DNA fragment between the joined segments. According to the IMGT/GeneInfo database, humans possess 6 functional Vγ segments and 3 mere Vδ segments, as well as 5 shared with Vα locus (35–37). There are more V regions in the Tcra and Tcrb genes, allowing for the generation of a larger number of classical TCR combinations. However, mechanisms that significantly increase the diversity of TCR γδ include the presence of additional D regions in Tcrd, random deletions and insertions of nucleotides (named N), or the insertion of palindromic sequences (named P) during the V(D)J region joining (38). Nucleotides are added through terminal deoxynucleotidyl transferase (TdT) (39), generating variability at the junctions. One of the crucial components interacting with the antigen is complementary determinant regions (CDRs), responsible for the specificity of the V region. Among them, the CDR3, arising from the combination of V, (D), and J segments, exhibits the greatest variability and is considered a key element in the diversity of TCR repertoire (38). The CDR3 in the δ chain is longer and more diverse compared to the classical β chain, exhibiting greater sequence variability, which enables γδ TCR to recognize both protein and non-protein antigens (40).
The nomenclature used in describing γδ T cell subgroups is based on the presence of specific V segments in γ and δ chains. Initially, the 14 loci for Vγ genes and pseudogenes allowed classification into 6 major cell subpopulations. The largest group was VγI, encompassing the majority of functional genes (V2, V3, V4, V5, V8). The V9 loci formed a distinct VγII group (41). Currently, the prevailing nomenclature does not consider such divisions and relies on names derived from the variants of V segments in combined chains. Nevertheless, TCRs possessing Vγ9 are still referred to as VγII or Vγ2 in some works, which can be misleading. Nowadays γδ T cells are divided according to the V segments in the δ chain into Vδ1 and Vδ2 as well as Vδ3 group. The combination of selected γ and δ chains is characteristic for specific tissues (42) and the updated nomenclature follows a system more in line with the naming proposed by Heilig and Tonegawa, without subdividing the groups as in Lefranc and Rabbitts’ nomenclature. This approach has been adopted in the present work (41). According to this, the Vδ2 chain is most commonly associated with Vγ9 (Vγ9Vδ2) and constitutes the main subset of circulating γδ cells in healthy human peripheral blood (43). For comparison, the Vδ1 chain is associated with a greater number of γ chain variants and represents the main population inhabiting the liver (Vγ4), skin (Vγ4, γ5, γ6), spleen (Vγ4), or epithelium (Vγ7) (42). Additionally, the ratio between Vδ1 and Vδ2 cells in the blood varies not only in infectious and cancer diseases (44, 45) but also in human individual development. Starting from the embryonic stage, Vδ2 cell production dominates, then, at birth, there is a drastic decrease in the production of all γδ T lymphocyte groups. Around the age of 30, there is a significant dominance of Vδ2 cells over Vδ1, followed by a levelling off around the age of 45, with the Vδ1 subpopulation dominating in the proportions in the later years of adult life (45, 46). In tissues, this ratio changes even earlier, as the Vδ1 population already dominates at birth (47).
2.4 Untypical way of antigen recognition and co-stimulatory molecules
T lymphocytes bearing the classical TCR αβ receptor recognize foreign antigens of bacteria and viruses located both extracellularly and intracellularly after the incorporation of the foreign antigen into the membrane of the infected cell. This recognition is facilitated by antigen-presenting MHC molecules, a name retained to underscore that these were the first known group of proteins crucial in the graft acceptance process by the recipient’s body. An additional element enabling antigen recognition is provided by co-receptor proteins CD4 and CD8 on the surface of T lymphocytes αβ, allowing for the recognition of different classes of the MHC complex (class II and class I, respectively). As this information is considered fundamental for immunology understanding, it may come as a surprise that it does not apply to T γδ lymphocytes, which have developed their own rather sophisticated recognition system. Among The groups taking part in antigens recognition, and stimulation of γδ T cells are MHC, MHC-like molecules, the B7-like molecule family, Ig-like molecules, as well as NK cells-characterized receptors.
2.4.1 MHC molecules
MHC molecules relatively rarely serve as direct ligands for γδ TCR. However, they have been described in both mice (IEk and I-Ad) (48) and in humans, where in some circumstances the ability to recognize classical HLA molecules such as HLA-A24 (49), -B27 (50), -A2 (51), as well as non-classical HLA-E (52) has been defined. On the other hand, the lack of requirement of MHC restriction was demonstrated through studies in mice with a knockout for β2-microglobulin (β2-M), an integral part of the HLA molecule that is essential for revealing the specificity of MHC antigens. In this mice model, there was a minimal presence of T αβ lymphocytes due to an inhibited positive selection process in the thymus. On the other hand, it was shown that the lack of β2-M practically does not alter the parameters concerning T γδ cells (53, 54). Despite those results, it is hypothesized that a small subset of T γδ lymphocytes depends on MHC restriction. However, their overall abundance is low enough that the elimination of these cells in the β2-M knockout model falls below the detection threshold in the T cells’ entire population (55).
2.4.2 MHC-like molecules
Other studies in mice have identified proteins referred to as MHC class I-like, capable of stimulating T γδ lymphocytes. The most well-characterized protein in this group is polyphemusin II peptide (T22). Structurally, this peptide partially resembles MHC (56), yet it lacks the ability to bind the presented antigen, thereby activating up to 1% of T γδ lymphocytes in normal mice (57, 58). This process is primarily achievable due to the presence of a conserved fragment containing the sequence W….EGYEL in the loop determining the complementarity of the CDR3δ of the TCR γδ receptor. The linkage of aminoacids between tryptophan (W) and the EGYEL motive is variable in length and chemical nature, which may affect the binding of TCR with T22. Nevertheless, Sandstrom et al. have identified similarities in the binding of these molecules to the classical MHC and TCR αβ molecule binding scheme (58). Initial studies aiming to establish the importance of T22 in T cell development yielded conflicting results (59–61). However, the creation of a mouse model with a specific knockout for T10 and T22 demonstrated that while T22 is a key factor in TCR γδ development, it is possible to generate a small number of slightly impaired cells, yet still reactive to T22 without this antigen stimulation (62).
In humans, γδ T cells are capable of recognizing MHC-like molecules from the MIC family (MICA and MICB). These molecules are highly polymorphic and serve as stress-induced ligands for immune cell activation. Importantly, γδ T cells can recognize MIC molecules independently of the present allele, and in a relatively direct manner, as MIC molecules do not function like classical MHC since they do not present peptides to T cells. It must be mentioned that the recognition of MIC molecules does not involve the CDR3 fragment of the TCR chain (63). In this case, the role of a specific co-receptor is significant, with NKG2D serving as the receptor that recognizes these molecules. NKG2D is characteristic for several types of cells such as NK cells or CD8+ αβ T cells, but also present in abundance on the surface of γδ T cells (64).
2.4.3 B7-like molecules
Molecules related to the B7 family including butyrophilins (BTN) can also act as ligands for γδ TCR. It has been demonstrated that butyrophilin 3 A1 (BTN3A1) plays a crucial role in recognizing phosphoantigen by TCR Vγ9Vδ2 (65), especially in the context of cancer cell recognition, where, in some but not all cases, cell-to-cell contact is required (66–68). This suggests that BTN3A1 acts as an antigen-presenting molecule. While the intracellular B30.2 domain of BTN3A1 has been previously recognized for its significant role, the mechanism of cooperation between BTN3A1 and the TCR γδ was elucidated by Sandstrom’s team in 2014 (69). The current theory explaining the involvement of BTN3A1 in the activation of γδ T cells suggests binding of the phosphoantigen by the intracellular B30.2 domain and the formation of a complex with BTN2A1, causing structural changes in the molecule and transmitting the signal to the TCR γδ. Interestingly, this process involves two distant binding sites on the TCR γδ. The BTN2A1 molecule binds to the Vγ9 region, and possible binding sites for BTN3A1 are located in the CDR2 and CDR3 loops (70). Numerous supporting proteins are involved in this process (55). Nevertheless, the precise course of the interaction between BTN3A1, the antigen, and TCR γδ remains a subject of research and requires further exploration (71). However, there is already discussion about the role of butyrophilin in Vγ9Vδ2 T cell-targeted immunotherapy (72).
Recent studies highlight the intriguing role of BTNs and butyrophilin-like (BTNL) proteins in the selection and maintenance of γδ T cells. In the murine intestine, Btnl1 and Btnl6 heterocomplexes, expressed on the surface of enterocytes, regulate the maturation and expansion of Vγ7 T cells, promoting their phenotypic transition into mature γδ T cells (73). Similarly, in the human intestine, BTNL3 and BTNL8 complexes, expressed by intestinal epithelial cells, shape the organ-specific repertoire of Vγ4 T cells, influencing their selection and function (74). Disruption of functional BTNL3/BTNL8 complexes impairs the selection of Vγ4 T cells expressing CD103, a key marker of intraepithelial lymphocytes (IELs). Notably, inflammatory bowel diseases (IBD) have been associated with an overall reduction in γδ T cells, with a disproportionate decrease in the cytotoxic Vγ4 CD103+ subset. These cells exhibit high expression of activation receptors such as NCR1 and NKG2C (75), suggesting their potential therapeutic relevance. Moreover, enterocyte-expressed BTNL molecules support the long-term survival of γδ T cells in the intestine, independent of microbiota or peripheral lymphoid organs (76).
The effect of B7 family members in the activation of γδ lymphocytes also occurs through surface receptors known as Ig-like receptors. An example of such co-signaling is the interaction of the B7.1 and B7.2 proteins (commonly referred to as CD80 and CD86, respectively) located on APCs with the Ig-like receptor on γδ lymphocytes, in this case, the CD28 protein. Despite previous conflicting results regarding changes in the expression of the CD28 receptor on γδ cells (77, 78), Ribot and colleagues demonstrated a significant role of this receptor in the co-activation of γδ cells. They showed that the signal from CD28 influences the production of IL-2 and as a result of these events, human γδ cells, as well as those in the murine model, exhibit increased proliferation and survival capacity (79).
2.4.4 Ig-like co-receptors
Ig-like receptors are also involved in the recognition of cancer cells. An example of such action is demonstrated by DNAX accessory molecule-1 (DNAM-1). Phosphorylation of Ser329, one of the three possible phosphorylation sites on the intracellular domain of this protein, activates a cascade of kinases, leading to signal transmission. Studies have shown that blocking the activity of DNAM-1 results in a lack of cytotoxic response against cancer cells (80, 81).
2.4.5 NK-specific receptors
The last mentioned group of receptors are primarily associated with NK cells. This category includes receptors from the natural killer group 2 (NKG2), natural cytotoxicity receptors (NCR), and killer cell immunoglobulin-like receptors (KIR) groups. These are also abundant in γδ T cells and participate in the recognition of infectious antigens and tumor-transformed cells, as well as in the generation of cytolytic reactions against them (82, 83).
Among the NKG2 molecules, the best described in the context of γδ T cells appears to be the activating receptor NKG2D. Among the γδ cell population residing in human blood, the expression of NKG2D is approximately 10 times lower compared to NK cells (84). Stimulation of γδ cells through NKG2D occurs independently of the TCR and resembles the process that occurs in NK cells upon receptor binding to ligands such as MHC-like molecules: MICA/B. Most likely, the signaling pathway in γδ cells proceeds through the adaptor protein DAP10 and then engages the kinase PI3K (85, 86). It has been shown that in mice, signaling from this pathway is sufficient to induce cytolytic reactions originating from dendritic epidermal T cells (DETCs), which constitute a subpopulation of γδ cells in the epidermis (87). Another interesting aspect is Ca2+ ions, which are considered essential for the effective proliferation and activity of cells in the immune system, thus serving as informational and activating elements for the cell (88). It has been demonstrated that simultaneous activation of γδ TCR and NKG2D causes a sudden and short-term influx of Ca2+. This is a characteristic model for effector memory T cells. The authors of the mentioned studies suggest that the course of cell stimulation influences the effector functions of Vγ9Vδ2 subpopulations (89). The phenomenon of Ca2+ ion influx in response to NKG2D activation may be additionally inhibited by the protein kinase C theta (PKCθ) inhibitor, indicating that it is a signal supporting the action of NKG2D (90). In light of these events, it is apparent that stimulation by the NKG2D receptor enhances the function of γδ T cells and boosts the cytotoxic response against foreign cells.
NCRs receptors are not constitutively present on γδ T cells but rather they are expressed in special environments, after TCR stimulation (90). Among the NCR receptors, the activating NCR2 (NKp44) has been most closely associated with γδ T cells. The presence of NCR2 on this non-classical cell subset was identified at the beginning of the 21st century (91, 92). Furthermore, it has been demonstrated that blocking NCR2 limits cytotoxic capabilities against myeloma cells (92). NCR3 (NKp30) has also been detected on the Vδ1 subset, while NCR1 (NKp46) is found on the cytolytic intraepithelial Vδ1 T cell subset residing in the human intestines (93). Although the expression of these receptors is detected on specific γδ cell clones, the presence of each receptor is associated with the cytolytic abilities of the cells (94, 95).
There is very little literature on KIR receptors present on γδ T cells. However, it is known that similar to NK cells, KIR receptors on γδ T cells serve an inhibitory function. KIR receptors are present on Vγ9Vδ2 T cells capable of cytolytic reactions, whereas they are not detected in non-cytolytic cells. The Vγ9Vδ2 T cells subset with KIR receptors inhibits the lysis of tumor B cells possessing MHC class I (83). Additionally, blocking the interaction between KIR and MHC molecules activated the ability to recognize self-markers of γδ T cells and induced auto-reactivity (83). This is certainly an intriguing and poorly understood group of receptors located on γδ T cells, which requires investigation due to its therapeutic potential in combating both cancerous and autoimmune diseases.
In addition to the receptor groups outlined above, γδ T lymphocytes possess a wide repertoire of receptors that enable the characteristic integration of innate and adaptive immune response functions. This characteristic trait also renders γδ T cells with dual roles in immunological diseases: beneficial and adverse.
3 Dual role of γδ T cells in immune response
It is believed that γδ T cells combine characteristics of cells involved in both innate and adaptive immune protection (96). Additionally, they possess receptors on their surface that can both inhibit and activate their functional activity. Consequently, γδ T cells can be expected to participate in pathological conditions. Research supports this notion by indicating the involvement of γδ T cells in both defensive and reparative processes of the body as well as in promoting pathological states. Activated γδ T cells are capable of producing chemokines, growth factors, and cytokines characteristic for different Th lymphocyte subpopulations (97, 98), while they also possess cytotoxic abilities (92), and play a helper role for B lymphocytes (99).
Γδ T cells effectively regulate homeostasis in the tissues and organs where they reside, however, when extensively or inadequately stimulated they can turn against the host itself. Examples include pulmonary epithelial γδ T cells, intraepithelial lymphocytes (IELs) and γδ T cells in the epidermis of the intestine (named dendritic epidermal T cells - DETCs). These cells naturally participate in promoting the production of keratinocytes and growth factors for wound healing and maintaining the integrity and immunity of mucous membranes (100–102). Studies in mice have shown that overproduction of the transcription factor early growth response 3 (Egr3) stimulates the secretion of pro-inflammatory IL-17 by γδ T cells in the lungs, leading to pulmonary fibrosis (103). On the other hand, a deficiency of IL-17 in the lungs of the mouse model caused by limited stimulation from tumor necrosis factor α (TNF-α) weakens the inflammatory response, posing a danger in infectious and pathological processes (104). In the mouse intestine, TNF-α can disrupt the migration of IELs, potentially resulting in acute inflammation (100). Conversely, mice lacking IELs develop shorter intestinal crypts and poorer mucosa, resulting in less effective mucosal defense (105). Similarly, in mice with skin burn wounds, DETCs have been observed to regulate the influx of pro-inflammatory molecules to the injured site (106).
The dual nature of γδ T cells underscores their pivotal role in immune regulation, encompassing both protective and pathological processes. Understanding the complex internal and environmental mechanisms that govern their function is essential to harness their benefits while minimizing adverse effects.
4 Autoimmune diseases
One of the first publications pointed to the important role of γδ T cells in autoimmunity comes from the early nineties (107). Currently, it is known that γδ T cells play a significant role in the pathogenesis of autoimmune diseases, particularly in those affecting connective tissue. In connective tissue diseases such as rheumatoid arthritis (RA) and systemic lupus erythematosus (SLE), γδ T cells contribute to both protective and pathological processes. Their ability to produce a wide range of cytokines and chemokines positions them as key players in the inflammatory cascade, influencing the recruitment and activation of other immune cells. Understanding the mechanisms by which γδ T cells influence autoimmune responses is crucial for the development of targeted therapies aiming mitigation of γδ T cells’ detrimental effects while enhancing their protective roles. This chapter delves into the complex functions of γδ T cells in autoimmune diseases focusing on connective tissue disorders.
4.1 Rheumatoid arthritis
Initial reports on the role of γδ T cells in RA date back to the late 1980s and early 1990s (108). These studies indicated alterations in the levels of γδ T cells in the blood, synovial fluid, and synovial tissue of RA patients compared to healthy individuals (109). Additionally, variations in cell levels were observed in relation to C-reactive protein (CRP) concentrations in patients (110), as well as when dividing patients according to their age (111, 112). It was noted that RA patients with disease onset before the age of 45 exhibited a greater increase in γδ T cell numbers than older patients. This was attributed to the still undetermined repertoire of these cells in younger individuals. Hassan et al. hypothesized that antigenic stimulation during RA development leads to a sudden clonal expansion and a predisposition to a more aggressive disease course in younger patients, while older individuals with a defined repertoire of γδ T cell clonotypes do not respond as vigorously to RA-associated antigens (111).
Furthermore, the description of changes in HLA-DR expression on γδ T cells appears significant in the context of RA pathogenesis, with an increase in the percentage of HLA-DR+ γδ T cells in the peripheral blood of patients with active disease (113). It is now known that HLA-DR, particularly the HLA-DRB1*04 variant, plays a crucial role in RA pathogenesis by presenting extracellular antigens (e.g., bacterial or viral protein fragments) to helper T (CD4+) cells. The so-called shared epitope (SE) present in HLA-DR molecules predisposes to RA development by preferentially binding autoantigens, thereby leading to the activation of autoreactive CD4+ T cells and the initiation of an autoimmune response (114). In light of these facts, it can be inferred that γδ T cells significantly contribute to autoimmune response in RA (115).
Additionally, it has been demonstrated that immunization with mycobacterial heat shock protein 65 kDa (HSP65) causes an increase in the number of HLA-DR+ γδ T cells in the synovial fluid of RA patients (116). Stimulation of Vγ9Vδ2 T cells by isopentenyl pyrophosphate induces, among other effects, the production of IL-17 by these lymphocytes (117).
Th17 cells are the primary source of IL-17, a key pro-inflammatory cytokine in the pathogenesis of rheumatoid arthritis, while γδ T cells, differentiated into the γδ17 T subpopulation, play a secondary but still significant role in its production. Γδ T cells can produce IL-17 following stimulation by other molecules, such as IL-23 or IL-1β (118) (Figure 2). Classically, IL-17 contributes to the propagation of inflammation by stimulating various cell types, such as fibroblasts, macrophages, and chondrocytes, to produce other pro-inflammatory cytokines and chemokines. Those act as chemoattractants, recruiting neutrophils and macrophages to the site of inflammation, which is in most cases the joint. Moreover, IL-17 significantly contributes to the degradation of bone tissue by enhancing the expression of the Receptor Activator for Nuclear Factor κB (RANK) and its ligand (RANKL) and by activating osteoclasts (119) (Figure 2).
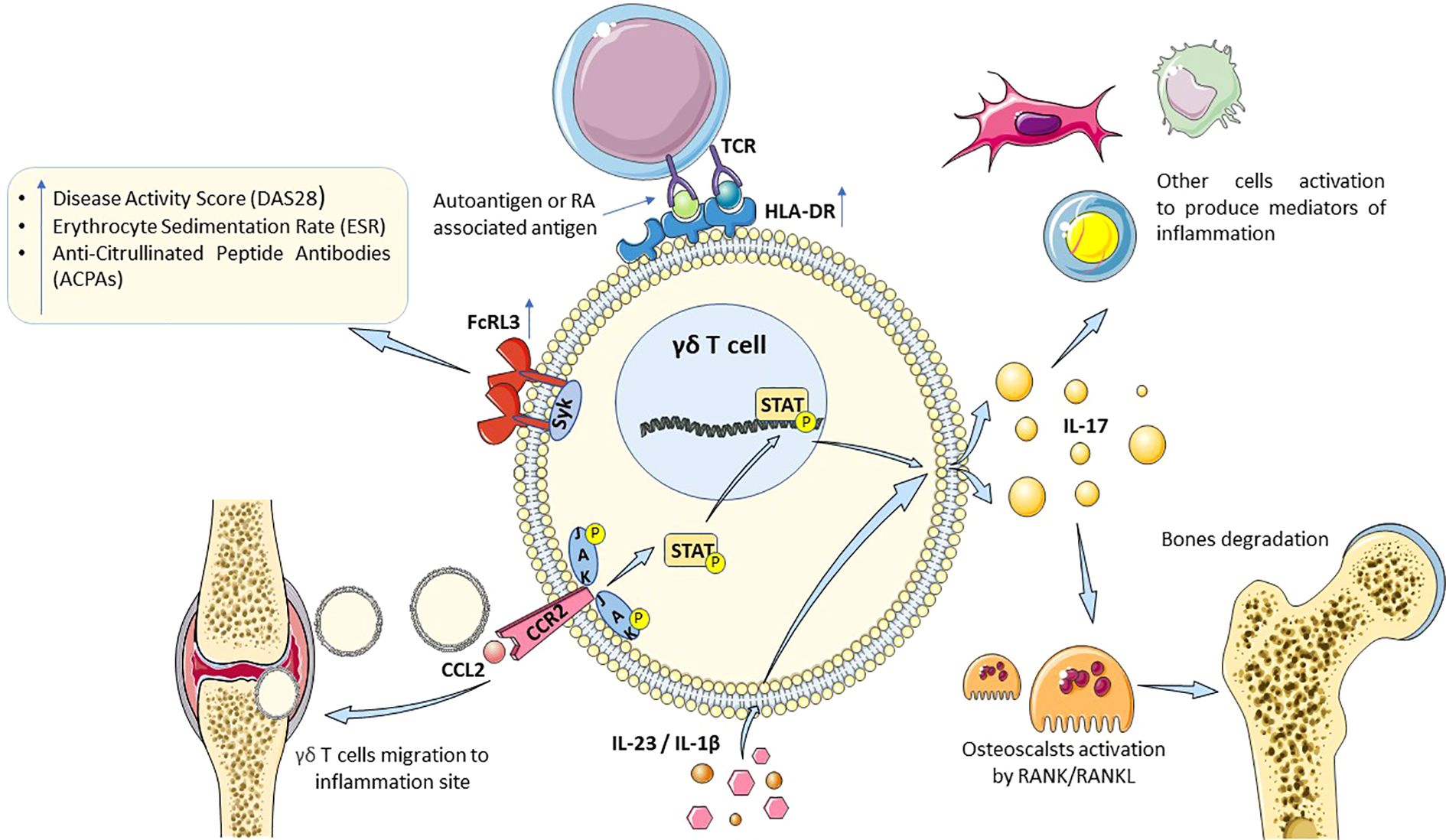
Figure 2. A proposed mechanism contributing to the pathogenesis of RA (rheumatoid arthritis), incorporating elements present in γδT cells and with proven pathogenic effects in T lymphocytes: T cell activation (HLA-DR expression), FcRL3 expression and involvement of Syk pathway, proinflammatory cytokine production including IL-17 leading to osteoclast activation and chemokine secretion facilitating γδ T cell migration to RA inflamed joints. More detailed description is given in the text. RA, rheumatoid arthritis; HLA-DR, human leukocyte antigen, DR isotype; IL, interleukin; RANK/RANKL, activator for nuclear factor κB (ligand); CCL2, chemokine (C-C motif) ligand 2; CCR2, C-C chemokine receptor 2; JAK, none-receptor tyrosine Janus kinase; STAT, signal transducer and activator of transcription protein; Syk, spleen tyrosine kinase; FcRL3, Fc receptor-like protein 3.
In the context of local inflammation occurring in the joints of RA patients, the migration of γδ17 T cells to the diseased site is crucial. During differentiation, γδ T cells exhibit increased expression of molecules CCR6, CCR2, and CXCR6 (120). CCR6 is present on classical Th17 lymphocytes and interacts with CCL20, causing Th17 migration to the site of inflammation (121) (Figure 2). For γδ T17 cells, the CCL2-CCR2 axis appears to be similarly important, facilitating their attraction to the inflamed joints (122, 123). The binding of CCL2 to its receptor can initiate, among other things, the JAK/STAT signaling pathway, the inhibition of which is targeted by biological therapies in the treatment of RA (124). In a mouse model of RA, treatment with Tofacitinib, which inhibits JAK1 and JAK3, and to a lesser extent JAK2 and TYK2, altered the levels of IL-17 and γδ17 T cells while promoting the presence of γδ T regulatory cells (125). Another mice study indicated that administration of ES-62 (a phosphorylcholine derivative molecule) lowered IL-17 levels in both joints and bones, whereas diseased mice showed an increased percentage of γδ17 T cells. In vitro studies demonstrated that ES-62 inhibits both γδ T lymphocytes and the dendritic cells (DCs) essential for modulating their both γδ T cells and DCs functionality (126).
Another potential mechanism determining the pathological processes in RA via γδ T cells is the high expression of the Fc receptor-like protein 3 (FcRL3) on their surface in RA patients. The increased expression of this receptor correlates with the Disease Activity Score (DAS28) and Erythrocyte Sedimentation Rate (ESR) (127). Furthermore, FcRs, and likely FcRL due to their significant homology, interact with Syk via its immunoreceptor tyrosine-based activation motif (ITAM) domain (127, 128), as well as its close homolog Zeta-chain-associated protein kinase 70 (ZAP-70) (127). Syk is essential for the development of the γδ T cell population. Elevated levels of Syk have been observed in RA patients and have been associated with higher titers of anti-citrullinated peptide antibodies (ACPAs) (129) (Figure 2). Mice deficient in both FcR and Syk do not develop collagen-induced arthritis (130), indicating the critical role of this pathway in the pathogenesis of RA (131).
In summary, γδ T cells contribute to the pathogenesis and progression of RA through both direct actions, such as the production of inflammatory mediators, and indirect mechanisms, including the activation of intracellular processes that create a pro-inflammatory environment conducive to joint inflammation and tissue destruction. However, further research is required to elucidate the precise role of these lymphocytes in RA (Figure 2).
4.2 Psoriasis
Psoriasis is a chronic autoimmune inflammatory skin disease characterized by red, scaly patches on the surface of the body. The etiology of psoriasis is complex, involving both genetic and immunological factors. Current research suggests that the dysregulation of T cells, including γδ T cells, plays a crucial role in the development and maintenance of inflammation in psoriasis. Studies on the role of γδ T cells in human psoriasis have revealed changes in the proportion of these cell subpopulations. Specifically, Vγ9Vδ2 T cells were found to be more abundant in the skin of affected individuals, while less frequent in the blood compared to healthy controls. This suggests a possible migration of these cells and their potential association with the disease (132). However, it is worth noting that role of γδ T cells in psoriasis are largely based on mouse models, which are extensively described in this chapter.
Numerous studies have shown that among the subpopulations of murine γδ T cells, the Vγ4 subset residing in the dermis is most closely associated with psoriasis, constituting approximately 20% of all γδ T cells in this tissue (133). Dermal γδ T cells differ somewhat from the epidermal DETC subpopulation, particularly in the structure of their TCR receptors. While epidermal T cells express the Vγ5 chain, this chain is rarely found in dermal T cells (134). Dermal γδ T cells express the CCR6 receptor, whose ligand, CCL20, is primarily located on keratinocytes and endothelial cells. The CCR6-CCL20 axis facilitates migration to the site of inflammation, suggesting a role for Vγ4 lymphocytes in this process (135). Similarly, human Vγ2Vγ9 cells have been observed to produce inflammatory mediators such as IFN-γ, IL-17A, TNF-α, CCL3, -4, -5, -6, which are present in psoriasis and aim to recruit immune cells to the skin and stimulate keratinocytes (132). Additionally, these cells are characterized by a high number of scavenger receptors Scart1 and Scart2 on their Surface (133). Scart1 and Scart2 are essential for the function of γδ T cells, especially in skin immunology, allowing these cells to recognize and respond to various ligands, which is crucial for initiating and regulating immune responses and maintaining tissue homeostasis. Moreover, a population of γδ T lymphocytes with high SCART2 expression and low CD5 and CD45RB expression has been identified in the dermis and skin lymph nodes. This group of cells can intensively produce IL-17, which described as a central player in the development of inflammation in psoriasis (136). Approximately 90% of IL-17-producing cells in the dermis are TCR γδ+ cells (137). In a mouse model of psoriasis induced by the Toll-like receptor 7 (TLR7) agonist named imiquimod (IMQ), it was shown that IL-17A-producing Vγ4Vδ4 T cells exhibit similarities, suggesting oligoclonal expansion or a common fetal origin of these cells (138, 139). This may confirm the involvement of SOX (SRY-related HMG-box) transcription factors, which are engaged in embryonic development, in the presence of Vγ4 cells capable of IL-17 production in the dermis (140). However, IL-17 production by γδ T cells requires stimulation via IL-23, which is produced by dermal myeloid dendritic cells and macrophages (137, 141). The IL-23/IL-17 axis exacerbates psoriasis-like conditions, and manipulating IL-23 availability to stimulated cells results in altered IL-17 production (137). Notably, in mice lacking the TCR γδ receptor (TCRδ -/-), IMQ stimulation resulted in significantly attenuated psoriasis-like symptoms, as it limited IL-17 production by dermal cells, rendering IL-17 levels insufficient to initiate acute inflammation (137). In addition to IL-23, IL-1β also influences γδ T cell stimulation. Both IL-1β and IL-23, through IL-1βR and IL-23R receptors on dermal γδ17 T cells, activate intracellular signaling pathways, namely mammalian target of rapamycin (mTOR) and STAT3, respectively. mTOR is a serine/threonine kinase consisting of two complexes, mTORC1 and mTORC2, essential for the survival and proliferation of γδ T lymphocytes, with mTORC2 appearing particularly crucial for dermal γδ17 T cells. The STAT3 pathway, activated via IL-23, is extremely important for the effector functions of murine dermal Vγ4 lymphocytes, while interestingly, Vγ6 cells are STAT3-independent. The mTOR and STAT3 pathways are linked by the transcription factor interferon regulatory factor 4 (IRF4), which regulates il17 gene expression (142). Various studies indicate that the mTOR and STAT3 pathways are significant for psoriasis development; however, in mouse models, mTORC2, not STAT3, deletion results in a mitigated psoriasis profile and changes in the γδ17 T cells profile (142, 143).
The literature has identified numerous factors controlling the activity of murine dermal γδ T cells and the IL-23/IL-17 axis, influencing the activity and severity of psoriasis. These include the presence of the V-domain immunoglobulin suppressor of T cell activation (VISTA) receptor, Bruton tyrosine kinase (BTK), pro-inflammatory monocytes, IL-36, microRNA molecules such as miR-20 and miR-92b, and complement system molecules (144–149). However, the role of γδ T cells in psoriasis development linked to genes affecting circadian rhythm disruption is of particular interest. Studies by Ando et al. showed that mice with deletions of CLOCK (CLCK; a core circadian gene) and PERIOD2 (PER2; an inhibitor of CLOCK) exhibit mitigated and exacerbated psoriasis symptoms, respectively, after IMQ induction. Importantly, γδ T cells isolated from CLOCK-deficient mice display altered cytokine profiles upon Il-23 stimulation. Moreover, splenic γδ T cells have lower Il-23R -expression since CLOCK binds to the E-box promoter region of the il23R gene. Loss of PER2 function yields the exact opposite effects as CLOCK mutation (150). Furthermore, other studies have shown that nuclear receptors Rev-Erb, also involved in circadian regulation, influence the ability of γδ T cells to produce IL-17, thereby mediating psoriasis symptoms (151). These findings suggest a crucial regulatory role of circadian genes in controlling the functionality of γδ T cells in psoriasis.
Evidence indicates that IL-17 produced by dermal γδ T cells is central to the inflammatory response in psoriasis. However, the multitude of factors regulating this process complicates the possibility of control. Therefore, this issue requires continuous research to uncover the functional mechanism and exploit the therapeutic potential of dermal γδ T cells.
4.3 Systemic lupus erythematosus
SLE is a chronic autoimmune disease that can affect various organs and tissues in the body, causing a wide range of symptoms such as fatigue, joint pain, skin rashes, and kidney problems. The incidence rate is approximately 40 cases per 100,000 people, with higher prevalence in African American and Hispanic and Caucasian populations (152, 153). The causes of SLE are complex, involving genetic, hormonal, and environmental factors, but this area still requires extensive research (154). Nonetheless, γδ T cells have a complex role in the pathogenesis and regulation of SLE.
Firstly, compared to the control group, the γδ T cell repertoire in SLE patients appears to be quite restricted, primarily involving the Vδ1 and Vδ2 chains, whereas the control group exhibited six active Vδ genes. Additionally, the Vγ9 cells in SLE patients differed from the control group by lacking the EVQEL motif in their junctional sequences and showing limited junctional diversity. Nevertheless, each patient exhibited unique oligoclonal transcripts, with variations in the CDR3 region length, suggesting that γδ T cells undergo peripheral oligoclonal expansion in SLE (155, 156).
Γδ T cells can regulate the humoral response, but they must first be activated through the recognition of autoantigens, such as the chaperonin-containing T-complex protein 1 subunit ζ (CCT6A), whose concentration significantly increases in SLE (156). Then they can be involved in the antibody production process by B cells. Studies on mice have shown that the simultaneous removal of αβ and γδ lymphocytes prevents class-switch recombination and the formation of autoantibodies that exacerbate the disease state in SLE models. It is well known that antibody production is associated with the formation of germinal centers (GCs), where immunoglobulin class switching occurs. This process requires T cells (157), yet evidence from murine studies suggests that γδ T cells play also a significant role in supporting the action of conventional αβ T cells (158). Another study demonstrated that γδ T cells expressing CXCR5 activate CD4+ T cells, which, upon releasing Wnt factors, begin to differentiate into various subtypes, such as T follicular helper (Tfh) cells. Activated CD4+ Tfh cells then migrate to lymphoid follicles in the lymph nodes and spleen, where they encounter naïve B cells and provide the necessary helper signals for GC formation and the initiation of antibody production and class switching (159). TCRγδ-deficient mice show impaired Tfh cell differentiation and GC formation, resulting in lower antibody levels and milder disease symptoms in SLE models (159). When αβ T cells are removed, T-cell-dependent autoantibody production still occurs, suggesting that αβ T cells are not the primary regulators in this process (158, 159).
In the peripheral blood of SLE patients, a decrease in the number of γδ T cells compared to the control group has been observed (160–162) and this decrease was more pronounced in SLE patients with anti-SSB/La antibodies, which occur in about 10-20% of patients (160–163). The level of γδ T cells also inversely correlated with disease activity markers such as SLEDAI, ESR, CRP, and anti-ds-DNA antibodies, which are key diagnostic markers for SLE (160, 161, 164). Additionally, an inverse relationship was found between the level of γδ T cells and the number of circulating plasmablasts, which may contribute to the formation of autoantibodies (164). The decrease in γδ T cells in SLE, even though they seem to be a key regulator of B cells, may be explained by increased apoptosis and reduced proliferation capacity of these cells.
Changes in the cytokine profile produced by peripheral γδ T lymphocytes, which can significantly contribute to the worsening of the patient’s condition, have also been observed (161). As in other autoimmune diseases, IL-17 appears to be a key factor, with its levels markedly increased in SLE patients’ serum (165). Du et al. identified the calcium/calmodulin dependent protein kinase IV (CaMK4) gene, whose expression changes can regulate γδ17 T cell activity in SLE. CaMK4 is a kinase that contributes to the excessive production of IL-17 in lymphatic tissues and kidneys, leading to the mediation of pathological T cell activity and the development of lupus nephritis (LN), which is one of the most common clinical forms of SLE. That suggests possibly a new therapeutic avenue (166).
In summary, γδ T cells play a complex and multifaceted role in the pathogenesis and regulation of SLE, influencing various stages of the immune response and inflammatory process, with a particularly crucial involvement in B cell differentiation and autoantibody production.
4.4 Systemic sclerosis
Systemic sclerosis (SSc) is a chronic autoimmune disease characterized by widespread vascular abnormalities and fibrosis of the skin and internal organs. It predominantly affects women and can lead to severe complications involving the lungs, heart, kidneys, and gastrointestinal tract. The pathogenesis of SSc involves a complex interplay between genetic predisposition, environmental factors, and dysregulated immune responses. Understanding the cellular and molecular mechanisms underlying SSc is crucial for developing effective therapeutic strategies and improving patient outcomes (167).
Γδ T cells play a significant role in the pathogenesis of SSc, with their functions and numbers varying depending on the disease stage and their location in the body. In SSc, γδ T cells exhibit limited variability in junctional sequence length regardless of tissue origin, suggesting oligoclonal expression in response to a restricted antigen pool (168). Research indicates a decrease in the total number of γδ T cells in the peripheral blood of SSc patients, especially in the early stages of the disease and in the presence of anti-topoisomerase I antibodies (anti-Scl-70) belonging to anti-nuclear antibodies (ANA) (169, 170). Conversely, there has been a confirmed increase in the Vδ1+ subpopulation in bronchoalveolar lavage fluid (171, 172). In the early phase of SSc, γδ T cells, mainly Vδ1+, also accumulate in perivascular areas of the skin, potentially due to the expression of CD49d (90% of γδ T cells), a ligand for vascular cell adhesion protein (VCAM) involved in lymphocyte migration to tissues. CD49d+γδ T cells were not found in the skin of healthy individuals (172, 173). However, the expression of another cell migration protein, CD62L, is reduced in SSc patients (174).
Γδ T cells also exhibit increased numbers of activating receptors and molecules (HLA-DR, CD69) and cytotoxic mediators (CD8, CD16), potentially exacerbating inflammation and disease severity (172). Additionally, those cells expressing the CD27 receptor show a higher percentage of cytotoxic mediators granzyme B and perforin, indicating their activity and involvement in the pathological state (169). Interestingly, Ueda-Hayakawa reported excessive production of collagen type I alpha 2 chain (COL1A2) mRNA in fibroblasts cultured with γδ T cells (174). However, another study found no differences in the percentage of Vγ9 cells between SSc patients and controls, with the cells maintaining their cytotoxic ability and regulating fibroblast growth through apoptosis induction (175). The discrepancy between these studies may be due to the direct contact between lymphocytes and fibroblasts, leading to different fibroblast response patterns (174, 175).
In terms of cytokine production γδ T cells in SSc exhibit a Th1 polarization, such as IFN-γ production, which contrasts with the Th2 polarization seen in the decidua during pregnancy, where Vδ1 cells inhibit the immune response against the developing fetus (172, 176) This difference suggests the diverse functions of γδ T cells in two distinct conditions, despite the suggestion that γδ T cells interacting with persisting fetal cells post-pregnancy might influence SSc development. These cells can recognize trophoblast antigens, such as Hsp60, and modulate the immune response (176).
The role of γδ T cells in SSc is poorly understood, but existing research suggests it is significant, necessitating further studies.
5 Other dysfunctional conditions
5.1 Tumor changes
Although tumors are not classified as an immunological disease, they are inextricably linked to the immune system. Due to their unique properties and therapeutic potential, the role of γδ T lymphocytes in tumorigenesis is the subject of intensive research. Γδ T cells have the ability to directly recognize and eliminate cancer cells, making them a key element of the body’s defense against tumor development. Their activity includes cytotoxic mechanisms, interaction with other immune cells, and the production of cytokines that support the immune response and influence the tumor microenvironment. Understanding the role of γδ cells in tumorigenesis may lead to the exploration of novel therapies that will harness these cells against tumor development.
The antitumor activity of γδ T cells begins with the recognition of cancerous cells. The γδ TCR is polyclonal; however, its diversity is lower than that of the αβ TCR. This is due to the limited number of V-segment genes and a more restricted clone repertoire in specific tissues. However, this constrained polyclonality, combined with the presence of a longer CDR3 region in the δ chain, allows the γδ TCR to recognize both peptide and non-peptide antigens in an MHC-independent manner. This unique feature is being utilized in the development of novel targeted therapies (177, 178).It is known that the ability of γδ T cells to recognize tumors and initiate direct cytotoxicity against them depends on various receptors and the tumor microenvironment (Figure 3). Upon interaction with cancer cells, γδ T cells can express Fas ligand (FASL) and TNF-related apoptosis-inducing ligand (TRAIL), which, upon binding to their respective receptors, Fas (CD95) and TRAIL-R1/R2, activate death pathways in target cells (179, 180). Additionally, FASL induction can be regulated by the activation of NKG2D (181, 182) (Figure 3). NKG2D is the best described receptor responsible for cytotoxic activation and recognizes ligands such as MICA, MICB, and ULBP, which are expressed on cancer cell surfaces (183) (Figure 3). These ligands are usually absent in healthy tissues, but their expression increases under cellular stress, such as during cancer transformation (184). The TCR γδ also plays a role in tumor recognition. The Vγ9Vδ2 subpopulation can identify phosphoantigens (pAg) such as isopentenyl pyrophosphate (IPP), which are overproduced due to dysregulated mevalonate pathways in cancerous cells (185). Butyrophilin 2A1 (BTN2A1) has emerged as a key molecule in this reaction. BTN2A1, together with BTN3A1, is present on the surface of antigen-presenting cells and cancer cells, and after the internal domain of BTN3A1 binds phosphoantigens, conformational changes in both molecules occur, allowing BTN2A1 to bind to the Vγ9 region of the TCR γδ (186) (Figure 3). Studies have shown that BTN2A1 cannot be replaced by any other butyrophilin family molecule, as this leads to the loss of γδ T cell stimulation, while BTN3A1 is essential for pAg recognition (65, 70).
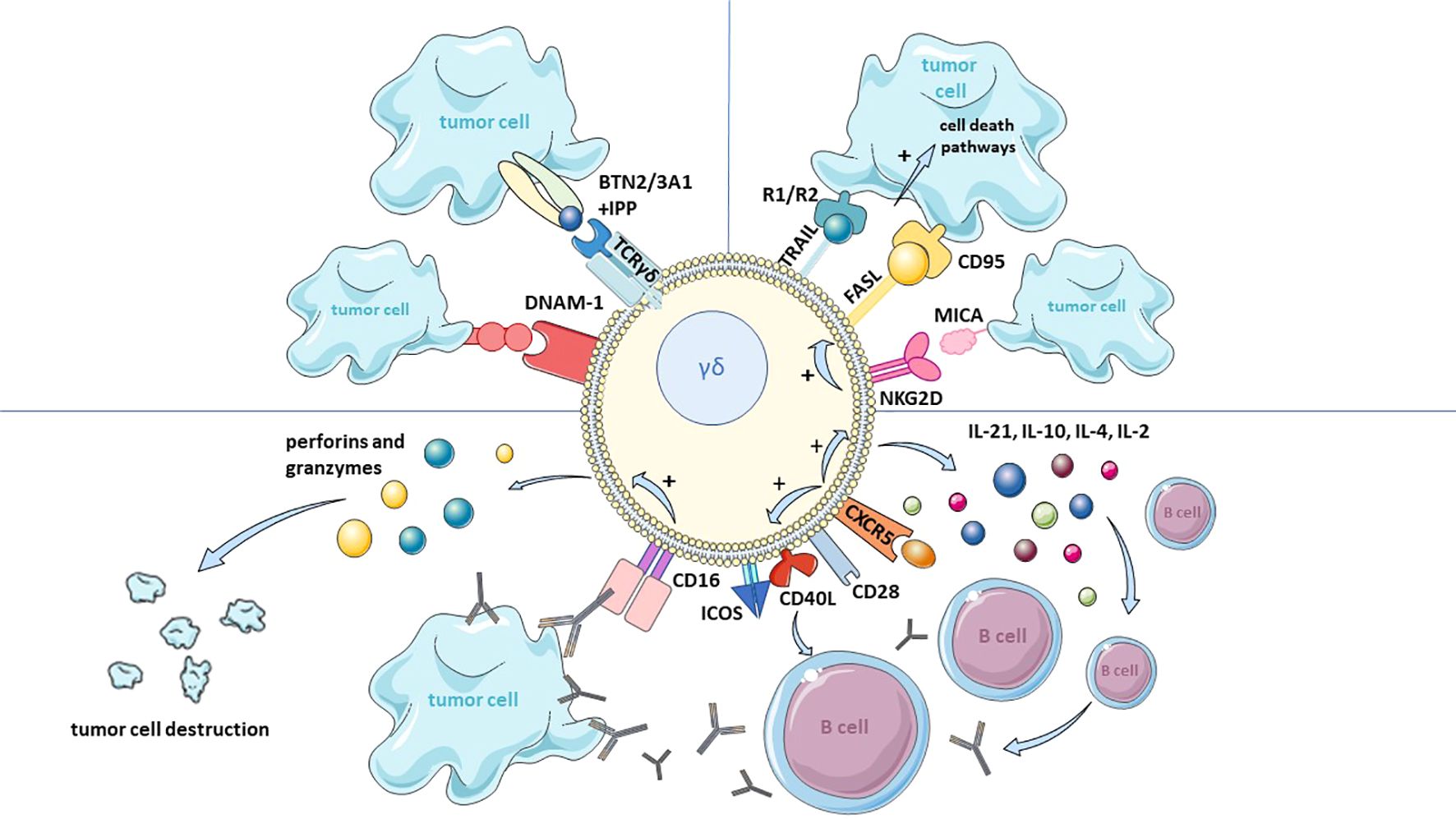
Figure 3. Scheme of γδ T cells interaction with tumor cells aiming their destruction. More detailed description is given in the text. TRAIL, TNF-related apoptosis-inducing ligand; R1/R2, TNF-related apoptosis-inducing receptor; FASL, Fas ligand; CD95, Fas receptor; MICA, MHC class I polypeptide–related sequence A; NKG2D, receptor from the natural killer group 2 D; IL, interleukin; CXCR5, C-X-C motif chemokine receptor; CD28, cluster of differentiation 28, Tp44; CD40L, cluster of differentiation 40, p50; ICOS, inducible T cell costimulatory; CD16, cluster of differentiation, FcγRIII; DNAM-1, DNAX Accessory Molecule-1; BTN2/3A1, butyrophilins 2/3 A1; IPP, isopentenyl pyrophosphate.
The signal from TCR γδ stimulation is not always sufficient, and in some cases, γδ T cells may require additional receptors, such as CD226 (DNAM-1) or NKp30, to effectively kill cancer cells (187, 188). These diverse receptors allow γδ T cells to flexibly respond to different types of tumors, making them attractive targets in cancer therapy. To date, γδ T cell activity against various cancers, including leukemia, B-cell lymphoma, prostate cancer, melanoma, and mesenchymal glioma, has been described (90, 189–193).
Another mechanism of γδ T cell antitumor activity is antibody-dependent cellular cytotoxicity (ADCC). In this process, γδ T cells participate in recognizing and eliminating tumor cells by binding to antibodies specific for tumor antigens. These antibodies coat the cancer cells, and the Fc fragment of the antibodies binds to the FcγRIII (CD16) receptor on γδ T cells, leading to their activation (194) (Figure 3). Upon activation, γδ T cells destroy the coated cancer cells by releasing cytotoxic proteins, such as perforin and granzymes (195). Perforin facilitates the access of proteolytic granzymes to the interior of the target cell, and disruption of these proteins’ functions may result in the sudden onset of cancer (196). Moreover, γδ T cells can enhance ADCC by regulating the maturation and function of B lymphocytes (197). This occurs through a cascade of events, starting with the presence of CXC chemokine receptor type 5 (CXCR5) on γδ T cells, whose stimulation increases the expression of co-stimulatory receptors such as Inducible T-cell costimulator (ICOS), CD40L, and CD28 on Vγ9Vδ2 cells, as well as the production of cytokines IL-21, IL-10, IL-4, and IL-2 (198) (Figure 3). The interaction of these receptors with their ligands on B cells (ICOSL, CD40, and CD86) and the action of the produced cytokines stimulate B cells to produce antibodies (195) (Figure 3).
Γδ T cells also interact with other cell types, including DCs, which play an essential role in the immune response to tumor formation due to their ability to present tumor antigens (199). DCs stimulation occurs through cytokine signaling as well as direct interactions between the two cell types. Similar to interactions with B cells, CD40, CD80, and CD86 molecules on DCs are involved, and HLA-DR production is stimulated, which is necessary for antigen presentation to T cells (200). Furthermore, γδ T cells can influence the activity of dendritic cells (DCs) through interactions between Toll-like receptors (TLRs) and their ligands on DC’s surface (201). This combined stimulation of γδ T cells and TLRs leads to increased production of pro-inflammatory cytokines, such as IL-12, which promotes Th1-type responses, as demonstrated in co-cultures of the two cell types (202). The effect of DC activation by γδ T cells can be further enhanced by cytokines produced by γδ T cells, particularly IFN-γ, TNF, and IL-6. Unfortunately, γδ T cell cytokine production can also have negative effects in the context of tumorigenesis. The γδ17 T cells subset, capable of producing IL-17, can alter the tumor microenvironment by supporting angiogenesis and the formation of vascular connections that nourish the tumor, a state which is associated with poorer patient outcomes (203, 204).
Research on the role of γδ T lymphocytes in tumorigenesis reveals their crucial role in the immunological control of malignancies. Interactions between cancer cells and the immune system are fundamental for understanding and developing effective therapies. Due to their unique properties, γδ T cells represent a promising direction in cancer immunotherapy. Future studies on their mechanisms of action and potential clinical applications may lead to groundbreaking discoveries that could revolutionize cancer treatment approaches and improve patient outcomes. Thus, γδ T cells are becoming not only the subject of scientific research but also a beacon of hope for new, more effective therapeutic methods in oncology.
6 Promising future of therapies based on γδ T cells
With the implementation of Regulation (EU) 536/2014, the regulations governing the registration of trials in Europe have been tightened to ensure transparency at all stages of research (205). It is important to note that as of January 31, 2022, this regulation replaced previous directives to enhance participant protection and facilitate cross-border collaboration in research on new drugs. The Clinical Trials Information System (CTIS) portal and database have been introduced, providing a single location for submitting applications for clinical trial authorization across the European Union (206). Instead of submitting trials in each country individually, researchers can now do so simultaneously for multiple EU member states. All studies had to be transferred to the CTIS system by January 31, 2025 (207).
In the context of clinical research, therapies based on γδ T cells are becoming increasingly important, although they are currently mostly in the research phase and have not yet been fully approved by regulatory authorities such as the FDA in the United States or the EMA in Europe. While they have not reached the status of standard oncological treatment, these therapies are available through clinical trials and orphan drug programs in the United States and many European countries (208, 209).
A search for “gamma delta T cells” in ClinicalTrials.gov (210) yields 41 records of all registered clinical trials under this term. The oldest, registered under NCT01404702, commenced in 2011 and investigated the stimulating effect of zoledronic acid (ZOL) therapy combined with interleukin-2 (IL-2) on γδ T cells capable of killing neuroblastoma cells in a pediatric group. Unfortunately, this study did not yield the expected therapeutic results (211). However, the potential implementation of γδ T cells in this context has not been abandoned. Currently, a phase I study (NCT05400603) is underway in Atlanta (Georgia, United States), aimed to determine the maximum tolerated dose (MTD) and recommended phase II dose (RP2D) of allogeneic expanded γδ T cells in combination therapy for children with refractory or relapsed neuroblastoma and refractory/relapsed osteosarcoma, as well as defining the toxicity of these cells. The sponsor underscores the significance of this research, noting that over half of children suffering from these diseases do not survive or experience treatment-related toxicity. On the other hand, there are reports suggesting potential adverse effects following zoledronic acid treatment, which may lead to the development or recurrence of autoimmune or malignant diseases, possibly involving activated Vγ9δ2 T cells in this process (212). However, further research and observation are needed to confirm these findings.
Current research on γδ T cells focuses on three main categories of therapy: γδ T cell-stimulating therapies, combination therapies with other immune components, and cell engineering therapies utilizing genetically modified γδ T cells. Although these treatment methods have not yet gained full acceptance, they represent a promising direction primarily in oncology (213).
Γδ T cell-stimulating therapies aim to activate, expand, and enhance the natural cytotoxic properties of γδ T cells to improve their ability to recognize and destroy cancer cells. Rather than directly modifying cells, these therapies support their natural activity by stimulating relevant receptors and signaling pathways. This group includes immunotherapy using bisphosphonates, NKG2D agonists, and stimulation with cytokines such as IL-2 and IL-15 (95, 214, 215).
Combination therapies involving γδ T cells with other components of the immune system aim to enhance therapeutic efficacy. The integration of multiple mechanisms enables a more comprehensive and effective immune response. Examples include therapies based on monoclonal antibodies, where γδ T cells cooperate with antibodies such as rituximab to strengthen the ADCC response; combinations with TLR modulators that influence γδ T cell activity towards DCs, as well as combination therapies with checkpoint inhibitors, including blocking PD-1 and CTLA-4 receptors (216–219).
A novel concept involves the utilization of genetic engineering methods to introduce changes into γδ T cells (Figure 4). This therapy involves genetically modifying γδ T cells equipped with specific receptors (e.g., CAR) (220) that enable more precise and effective recognition of cancer cells. As a result, γδ T cells can target tumors more accurately. The classic CAR-T therapy was approved by the FDA in 2017 for treating B-cell acute lymphoblastic leukemia and diffuse large B-cell lymphoma (DLBCL) (221). The procedure is based on the classical Adoptive Cell Therapy (ACT) method, which involves collecting T cells from the patient, expanding them in vitro, and then reinfusing them into the patient, with the added aspect of genetic modification in CAR therapy. Another option is the introduction of allogeneic modified γδ T cells from donors into the patient’s body (222).
As of the writing of this article (September 2024), three active clinical trials regarding CAR-T γδ therapies are visible in the ClinicalTrials.gov database. The oldest, which is actively recruiting patients, concerns the safety and efficacy of therapy in patients suffering from severe SLE (NCT06106893) (223). Two additional studies, registered in the second half of 2024, are also Phase I or I/II trials, concerning lupus nephritis (NCT06375993) and clear cell renal cell carcinoma (ccRCC) (NCT06480565). A notable difference in these clinical trials is the functionality of CAR-Tγδ cells, as they target various antigens characteristic of the diseases. In the study NCT06375993, the target antigen is CD20, while for NCT06480565, it is CD70 (224, 225).
In addition to registered CAR-Tγδ clinical trials, there are 10 active studies concerning other therapies using γδ T cells. Most of these involve allogeneic γδ T cells administered to patients with various types of solid tumors (NCT04765462, NCT05400603), glioblastoma multiforme (GBM) (NCT05664243), and blood cancers (NCT053588, NCT04764513). The tested methods pertain to both monotherapy and combination therapies with traditional oncological treatment methods. Furthermore, an anti-PD1 therapy using γδ T cells for solid tumors is also in the research phase (NCT06404281), along with allogeneic γδ T cells transplants (NCT06364787, NCT06364800, NCT06212388).
A particularly noteworthy investigation is the application of bispecific antibodies targeting anti-Vδ2 and anti-prostate specific membrane antigen (PSMA) for the treatment of metastatic castration resistant prostate cancer (mCRPC). This innovative therapy aims to enhance the cytotoxic response of γδ T cells against tumor cells (NCT05369000) (226). The study is set to conclude by the end of 2027, and its findings may be of value given that another drug from the same sponsor, LAVA-051, which is also a bispecific antibody, has been approved by the FDA as an orphan drug for treating chronic lymphocytic leukemia (CLL) (227). Nevertheless, according to the clinicaltrials.gov database (accessed on March 17, 2025), the study was terminated due to business reasons, not related to product safety issues.
The utilization of γδ T cell-based therapies in autoimmune diseases, including rheumatological disorders, remains in its infancy. There is still a substantial need for further research and collaboration among the scientific and medical communities to make such treatments feasible and accessible. Nonetheless, the scientific landscape is continuously evolving, providing new evidence that underscores the significance of this area of inquiry. The dualistic nature of γδ T cells presents considerable immunomodulatory and therapeutic potential, which merits further exploration and attention (228–230).
7 Conclusions
γδ T cells are becoming an increasingly intriguing area of research, particularly due to their therapeutic potential in the treatment of autoimmune diseases and cancers. With their unique properties, γδ T cells demonstrate the ability to quickly recognize and eliminate cancer cells, positioning them as promising candidates in cancer immunotherapy. Studies suggest that these cells can act as regulators of immune responses, which may be crucial in the context of autoimmune diseases where the balance between pro-inflammatory and anti-inflammatory responses is disrupted.
It is also noteworthy that γδ T cells possess the ability to support other immune cells, enhancing their therapeutic efficacy. Their dualistic action allows them to be both pro-inflammatory and anti-inflammatory, creating opportunities for developing personalized therapies tailored to the specific needs of patients. Further research into the mechanisms of γδ T cell action may reveal new treatment strategies that could benefit patients who currently lack effective therapeutic options.
Clinical trial examples involving γδ T cell therapies indicate that their potential in clinical practice is significant. While these therapies are still in the research phase, the results of previous clinical experiences suggest that γδ T cells may play a pivotal role in future immunotherapeutic approaches. Given the growing interest in new strategies for cancer and autoimmune disease treatment, it is worthwhile to continue exploring γδ T cells to fully understand their capabilities and potential clinical applications.
Author contributions
SB: Conceptualization, Visualization, Writing – original draft, Writing – review & editing. KB-K: Funding acquisition, Supervision, Writing – original draft, Writing – review & editing.
Funding
The author(s) declare that financial support was received for the research and/or publication of this article. This work was supported by the project No. 2023/49/N/NZ5/04128 from the National Science Centre, Poland.
Acknowledgments
Special thanks to Ms. Ioulia Gavriilidi, MSc, for language proofreading. Figures were created by using “Servier Medical Art”, licensed under a Creative Commons Attribution under CC BY 4.0.
Conflict of interest
The authors declare that the research was conducted in the absence of any commercial or financial relationships that could be construed as a potential conflict of interest.
The author(s) declared that they were an editorial board member of Frontiers, at the time of submission. This had no impact on the peer review process and the final decision.
Generative AI statement
The author(s) declare that no Generative AI was used in the creation of this manuscript.
Publisher’s note
All claims expressed in this article are solely those of the authors and do not necessarily represent those of their affiliated organizations, or those of the publisher, the editors and the reviewers. Any product that may be evaluated in this article, or claim that may be made by its manufacturer, is not guaranteed or endorsed by the publisher.
References
1. Brenner MB, McLean J, Dialynas DP, Strominger JL, Smith JA, Owen FL, et al. Identification of a putative second T-cell receptor. Nature. (1986) 322:145–9. doi: 10.1038/322145a0
2. Bank I, DePinho RA, Brenner MB, Cassimeris J, Alt FW, Chess L. A functional T3 molecule associated with a novel heterodimer on the surface of immature human thymocytes. Nature. (1986) 322:179–81. doi: 10.1038/322179a0
3. Born W, Miles C, White J, O'Brien R, Freed JH, Marrack P, et al. Peptide sequences of T-cell receptor delta and gamma chains are identical to predicted X and gamma proteins. Nature. (1987) 330:572–4. doi: 10.1038/330572a0
4. Hayday AC. (gamma)(delta) cells: a right time and a right place for a conserved third way of protection. Annu Rev Immunol. (2000) 18:975–1026. doi: 10.1146/annurev.immunol.18.1.975
5. Kreslavsky T, Garbe AI, Krueger A, von Boehmer H. T cell receptor-instructed alphabeta versus gammadelta lineage commitment revealed by single-cell analysis. J Exp Med. (2008) 205:1173–86. doi: 10.1084/jem.20072425
6. Garbe AI, von Boehmer. H. TCR and Notch synergize in alphabeta versus gammadelta lineage choice. Trends Immunol. (2007) 28:124–31. doi: 10.1016/j.it.2007.01.004
7. Parker ME, Ciofani M. Regulation of γδ T cell effector diversification in the thymus. Front Immunol. (2020) 11:42. doi: 10.3389/fimmu.2020.00042
8. Qu G, Wang S, Zhou Z, Jiang D, Liao A, Luo J. Comparing mouse and human tissue-resident γδ T cells. Front Immunol. (2022) 13:891687. doi: 10.3389/fimmu.2022.891687
9. Chien YH, Meyer C, Bonneville M. γδ T cells: first line of defense and beyond. Annu Rev Immunol. (2014) 32:121–55. doi: 10.1146/annurev-immunol-032713-120216
10. Pistoia V, Tumino N, Vacca P, Veneziani I, Moretta A, Locatelli F, et al. Human γδ T-cells: from surface receptors to the therapy of high-risk leukemias. Front Immunol. (2018) 9:984. doi: 10.3389/fimmu.2018.00984
11. Morandi F, Yazdanifar M, Cocco C, Bertaina A, Airoldi I. Engineering the bridge between innate and adaptive immunity for cancer immunotherapy: focus on γδ T and NK cells. Cells. (2020) 9:1757. doi: 10.3390/cells9081757
12. Giri S, Girdhari L. Differentiation and functional plasticity of gamma-delta (γδ) T cells under homeostatic and disease conditions. Mol Immunol. (2021) 136:138–49. doi: 10.1016/j.molimm.2021.06.006
13. Porritt HE, Rumfelt LL, Tabrizifard S, Schmitt TM, Zúñiga-Pflücker JC, Petrie HT. Heterogeneity among DN1 prothymocytes reveals multiple progenitors with different capacities to generate T cell and non-T cell lineages. Immunity. (2004) 20:735–45. doi: 10.1016/j.immuni.2004.05.004
14. Hernandez JB, Newton RH, Walsh CM. Life and death in the thymus–cell death signaling during T cell development. Curr Opin Cell Biol. (2010) 22:865–71. doi: 10.1016/j.ceb.2010.08.003
15. Hayes SM, Li L, Love PE. TCR signal strength influences alphabeta/gammadelta lineage fate. Immunity. (2005) 22:583–93. doi: 10.1016/j.immuni.2005.03.014
16. Fahl SP, Coffey F, Wiest DL. Origins of γδ T cell effector subsets: a riddle wrapped in an enigma. J Immunol (Baltimore Md: 1950). (2014) 193:4289–94. doi: 10.4049/jimmunol.1401813
17. Hosokawa H, Rothenberg EV. How transcription factors drive choice of the T cell fate. Nat Rev Immunol. (2021) 21:162–76. doi: 10.1038/s41577-020-00426-6
18. Van de Walle I, Waegemans E, De Medts J, De Smet G, De Smedt M, Snauwaert S, et al. Specific Notch receptor-ligand interactions control human TCR-αβ/γδ development by inducing differential Notch signal strength. J Exp Med. (2013) 210:683–97. doi: 10.1084/jem.20121798
19. Van de Walle I, De Smet G, De Smedt M, Vandekerckhove B, Leclercq G, Plum J, et al. An early decrease in Notch activation is required for human TCR-alphabeta lineage differentiation at the expense of TCR-gammadelta T cells. Blood. (2009) 113:2988–98. doi: 10.1182/blood-2008-06-164871
20. Balciunaite G, Ceredig R, Fehling HJ, Zúñiga-Pflücker JC, Rolink AG. The role of Notch and IL-7 signaling in early thymocyte proliferation and differentiation. Eur J Immunol. (2005) 35:1292–300. doi: 10.1002/eji.200425822
21. Kang J, Coles M. IL-7: the global builder of the innate lymphoid network and beyond, one niche at a time. Semin Immunol. (2012) 24:190–7. doi: 10.1016/j.smim.2012.02.003
22. Maki K, Sunaga S, Ikuta K. The V-J recombination of T cell receptor-gamma genes is blocked in interleukin-7 receptor-deficient mice. J Exp Med. (1996) 184:2423–7. doi: 10.1084/jem.184.6.2423
23. He YW, Malek TR. Interleukin-7 receptor alpha is essential for the development of gamma delta + T cells, but not natural killer cells. J Exp Med. (1996) 184:289–93. doi: 10.1084/jem.184.1.289
24. Shibata K, Yamada H, Sato T, Dejima T, Nakamura M, Ikawa T, et al. Notch-Hes1 pathway is required for the development of IL-17-producing γδ T cells. Blood. (2011) 118:586–93. doi: 10.1182/blood-2011-02-334995
25. Nakamura M, Shibata K, Hatano S, Sato T, Ohkawa Y, Yamada H, et al. A genome-wide analysis identifies a notch-RBP-Jκ-IL-7Rα axis that controls IL-17-producing γδ T cell homeostasis in mice. J Immunol (Baltimore Md: 1950). (2015) 194:243–51. doi: 10.4049/jimmunol.1401619
26. Muro R, Nitta T, Nakano K, Okamura T, Takayanagi H, Suzuki H. γδTCR recruits the Syk/PI3K axis to drive proinflammatory differentiation program. J Clin Invest. (2018) 128:415–26. doi: 10.1172/JCI95837
27. Moon KD, Post CB, Durden DL, Zhou Q, De P, Harrison ML, et al. Molecular basis for a direct interaction between the Syk protein-tyrosine kinase and phosphoinositide 3-kinase. J Biol Chem. (2005) 280:1543–51. doi: 10.1074/jbc.M407805200
28. Sumaria N, Martin S, Pennington DJ. Constrained TCRγδ-associated Syk activity engages PI3K to facilitate thymic development of IL-17A-secreting γδ T cells. Sci Signal. (2021) 14:eabc5884. doi: 10.1126/scisignal.abc5884
29. Hu Y, Fang K, Wang Y, Lu N, Sun H, Zhang C. Single-cell analysis reveals the origins and intrahepatic development of liver-resident IFN-γ-producing γδ T cells. Cell Mol Immunol. (2021) 18:954–68. doi: 10.1038/s41423-021-00656-1
30. McVay LD, Carding SR. Extrathymic origin of human gamma delta T cells during fetal development. J Immunol (Baltimore Md: 1950). (1996) 157:2873–82.
31. Sanchez Sanchez G, Tafesse Y, Papadopoulou M, Vermijlen D. Surfing on the waves of the human γδ T cell ontogenic sea. Immunol Rev. (2023) 315:89–107. doi: 10.1111/imr.13184
32. Dimova T, Brouwer M, Gosselin F, Tassignon J, Leo O, Donner C, et al. Effector Vγ9Vδ2 T cells dominate the human fetal γδ T-cell repertoire. Proc Natl Acad Sci U.S.A. (2015) 112:E556–65. doi: 10.1073/pnas.1412058112
33. Capone M, Hockett RD Jr., Zlotnik A. Kinetics of T cell receptor beta, gamma, and delta rearrangements during adult thymic development: T cell receptor rearrangements are present in CD44(+)CD25(+) Pro-T thymocytes. Proc Natl Acad Sci U.S.A. (1998) 95:12522–7. doi: 10.1073/pnas.95.21.12522
34. Davis MM. T cell receptor gene diversity and selection. Annu Rev Biochem. (1990) 59:475–96. doi: 10.1146/annurev.bi.59.070190.002355
35. IMGT®, the international ImMunoGeneTics information system® . Available online at: https://www.imgt.org/GeneInfoServlets/htdocs/Documentation.html (Accessed September 27, 2024).
36. Baum TP, Hierle V, Pasqual N, Bellahcene F, Chaume D, Lefranc MP, et al. IMGT/GeneInfo: T cell receptor gamma TRG and delta TRD genes in database give access to all TR potential V(D)J recombinations. BMC Bioinf. (2006) 7:224. doi: 10.1186/1471-2105-7-224
37. Legut M, Cole DK, Sewell AK. The promise of γδ T cells and the γδ T cell receptor for cancer immunotherapy. Cell Mol Immunol. (2015) 12:656–68. doi: 10.1038/cmi.2015.28
38. Chen H, Zou M, Teng D, Zhang J, He W. Characterization of the diversity of T cell receptor γδ complementary determinant region 3 in human peripheral blood by Immune Repertoire Sequencing. J Immunol Methods. (2017) 443:9–17. doi: 10.1016/j.jim.2017.01.009
39. Bodger MP, Janossy G, Bollum FJ, Burford GD, Hoffbrand AV. The ontogeny of terminal deoxynucleotidyl transferase positive cells in the human fetus. Blood. (1983) 61:1125–31.
40. Rock EP, Sibbald PR, Davis MM, Chien YH. CDR3 length in antigen-specific immune receptors. J Exp Med. (1884) 179:323–8. doi: 10.1084/jem.179.1.323
41. Lefranc MP, Rabbitts TH. A nomenclature to fit the organization of the human T-cell receptor gamma and delta genes. Res Immunol. (1990) 141:615–8. doi: 10.1016/0923-2494(90)90068-a
42. Ribot JC, Lopes N, Silva-Santos B. γδ T cells in tissue physiology and surveillance. Nat Rev Immunol. (2021) 21:221–32. doi: 10.1038/s41577-020-00452-4
43. Willcox CR, Davey MS, Willcox. BE. Development and selection of the human Vγ9Vδ2+ T-cell repertoire. Front Immunol. (2018) 9:1501. doi: 10.3389/fimmu.2018.01501
44. Poggi A, Carosio R, Fenoglio D, Brenci S, Murdaca G, Setti M, et al. Migration of V delta 1 and V delta 2 T cells in response to CXCR3 and CXCR4 ligands in healthy donors and HIV-1-infected patients: competition by HIV-1 Tat. Blood. (2004) 103:2205–13. doi: 10.1182/blood-2003-08-2928
45. Hu Y, Hu Q, Li Y, Lu L, Xiang Z, Yin Z, et al. γδ T cells: origin and fate, subsets, diseases and immunotherapy. Signal Transduct Target Ther. (2023) 8:434. doi: 10.1038/s41392-023-01653-8
46. Boehme L, Roels J, Taghon T. Development of γδ T cells in the thymus - A human perspective. Semin Immunol. (2022) 61-64:101662. doi: 10.1016/j.smim.2022.101662
47. Fichtner AS, Ravens S, Prinz I. Human γδ TCR repertoires in health and disease. Cells. (2020) 9:800. doi: 10.3390/cells9040800
48. Schild H, Mavaddat N, Litzenberger C, Ehrich EW, Davis MM, Bluestone JA, et al. The nature of major histocompatibility complex recognition by gamma delta T cells. Cell. (1994) 76:29–37. doi: 10.1016/0092-8674(94)90170-8
49. Ciccone E, Viale O, Pende D, Malnati M, Battista Ferrara G, Barocci S, et al. Specificity of human T lymphocytes expressing a gamma/delta T cell antigen receptor. Recognition of a polymorphic determinant of HLA class I molecules by a gamma/delta clone. Eur J Immunol. (1989) 19:1267–71. doi: 10.1002/eji.1830190718
50. Del Porto P, D'Amato M, Fiorillo MT, Tuosto L, Piccolella E, Sorrentino R. Identification of a novel HLA-B27 subtype by restriction analysis of a cytotoxic gamma delta T cell clone. J Immunol. (1994) 153:3093–100.
51. Spits H, Paliard X, Engelhard VH, de Vries JE. Cytotoxic activity and lymphokine production of T cell receptor (TCR)-alpha beta+ and TCR-gamma delta+ cytotoxic T lymphocyte (CTL) clones recognizing HLA-A2 and HLA-A2 mutants. Recognition of TCR-gamma delta+ CTL clones is affected by mutations at positions 152 and 156. J Immunol. (1990) 144:4156–62.
52. Barakonyi A, Kovacs KT, Miko E, Szereday L, Varga P, Szekeres-Bartho J. Recognition of nonclassical HLA class I antigens by gamma delta T cells during pregnancy. J Immunol. (2002) 168:2683–8. doi: 10.4049/jimmunol.168.6.2683
53. Correa I, Bix M, Liao NS, Zijlstra M, Jaenisch R, Raulet D. Most gamma delta T cells develop normally in beta 2-microglobulin-deficient mice. Proc Natl Acad Sci U.S.A. (1992) 89:653–7. doi: 10.1073/pnas.89.2.653
54. Bigby M, Markowitz JS, Bleicher PA, Grusby MJ, Simha S, Siebrechtet M, et al. Most gamma delta T cells develop normally in the absence of MHC class II molecules. J Immunol. (1993) 151:4465–75. doi: 10.4049/jimmunol.151.9.4465
55. Deseke M, Prinz I. Ligand recognition by the γδ TCR and discrimination between homeostasis and stress conditions. Cell Mol Immunol. (2020) 17:914–24. doi: 10.1038/s41423-020-0503-y
56. Wingren C, Crowley MP, Degano M, Chien Y, Wilson IA. Crystal structure of a gammadelta T cell receptor ligand T22: a truncated MHC-like fold. Science. (2000) 287:310–4. doi: 10.1126/science.287.5451.310
57. Crowley MP, Fahrer AM, Baumgarth N, Hampl J, Gutgemann I, Teyton L, et al. A population of murine gammadelta T cells that recognize an inducible MHC class Ib molecule. Science. (2000) 287:314–6. doi: 10.1126/science.287.5451.314
58. Sandstrom A, Scharf L, McRae G, Hawk AJ, Meredith SC, Adams EJ. γδ T cell receptors recognize the non-classical major histocompatibility complex (MHC) molecule T22 via conserved anchor residues in a MHC peptide-like fashion. J Biol Chem. (2012) 287:6035–43. doi: 10.1074/jbc.M111.333153
59. Schweighoffer E, Fowlkes BJ. Positive selection is not required for thymic maturation of transgenic gamma delta T cells. J Exp Med. (1996) 183:2033–41. doi: 10.1084/jem.183.5.2033
60. Pereira P, Zijlstra M, McMaster J, Loring JM, Jaenisch R, Tonegawa S. Blockade of transgenic gamma delta T cell development in beta 2-microglobulin deficient mice. EMBO J. (1992) 11:25–31. doi: 10.1002/j.1460-2075.1992.tb05023.x
61. Wells FB, Gahm SJ, Hedrick SM, Bluestone JA, Dent A, Matis LA. Requirement for positive selection of gamma delta receptor-bearing T cells. Science. (1991) 253:903–5. doi: 10.1126/science.1831565
62. Fahl SP, Coffey F, Kain L, Zarin P, Dunbrack R, Teyton L, et al. Role of a selecting ligand in shaping the murine γδ-TCR repertoire. Proc Natl Acad Sci U.S.A. (2018) 115:1889–94. doi: 10.1073/pnas.1718328115
63. Champagne E. γδ T cell receptor ligands and modes of antigen recognition. Arch Immunol Ther Exp. (2011) 59:117–37. doi: 10.1007/s00005-011-0118-1
64. Rincon-Orozco B, Kunzmann V, Wrobel P, Kabelitz D, Steinle A, Herrmann T. Activation of V gamma 9V delta 2 T cells by NKG2D. J Immunol. (2005) 175:2144–51. doi: 10.4049/jimmunol.175.4.2144
65. Harly C, Guillaume Y, Nedellec S, Peigné CM, Mönkkönen H, Mönkkönen J, et al. Key implication of CD277/butyrophilin-3 (BTN3A) in cellular stress sensing by a major human γδ T-cell subset. Blood. (2012) 120:2269–79. doi: 10.1182/blood-2012-05-430470
66. Chan KF, Duarte JDG, Ostrouska S, Behren A. γδ T cells in the tumor microenvironment-interactions with other immune cells. Front Immunol. (2022) 13:894315. doi: 10.3389/fimmu.2022.894315
67. Lang F, Peyrat MA, Constant P, Davodeau F, David-Ameline J, Poquet Y, et al. Early activation of human V gamma 9V delta 2 T cell broad cytotoxicity and TNF production by nonpeptidic mycobacterial ligands. J Immunol. (1995) 154:5986–94. doi: 10.4049/jimmunol.154.11.5986
68. Morita CT, Beckman EM, Bukowski JF, Tanaka Y, Band H, Bloom BR, et al. Direct presentation of nonpeptide prenyl pyrophosphate antigens to human gamma delta T cells. Immunity. (1995) 3:495–507. doi: 10.1016/1074-7613(95)90178-7
69. Sandstrom A, Peigné CM, Léger A, Crooks JE, Konczak F, Gesnel MC, et al. The intracellular B30.2 domain of butyrophilin 3A1 binds phosphoantigens to mediate activation of human Vγ9Vδ2 T cells. Immunity. (2014) 40:490–500. doi: 10.1016/j.immuni.2014.03.003
70. Rigau M, Ostrouska S, Fulford TS, Johnson DN, Woods K, Ruan Z, et al. Butyrophilin 2A1 is essential for phosphoantigen reactivity by γδ T cells. Science. (2020) 367:eaay5516. doi: 10.1126/science.aay5516
71. Herrmann T, Karunakaran MM. Butyrophilins: γδ T cell receptor ligands, immunomodulators and more. Front Immunol. (2022) 13:876493. doi: 10.3389/fimmu.2022.876493
72. Rigau M, Uldrich AP, Behren A. Targeting butyrophilins for cancer immunotherapy. Trends Immunol. (2021) 42:670–80. doi: 10.1016/j.it.2021.06.002
73. Jandke A, Melandri D, Monin L, Ushakov DS, Laing AG, Vantourout P, et al. Butyrophilin-like proteins display combinatorial diversity in selecting and maintaining signature intraepithelial γδ T cell compartments. Nat Commun. (2020) 11:3769. doi: 10.1038/s41467-020-17557-y
74. Di Marco Barros R, Roberts NA, Dart RJ, Vantourout P, Jandke A, Nussbaumer O, et al. Epithelia use butyrophilin-like molecules to shape organ-specific γδ T cell compartments. Cell. (2016) 167:203–218.e17. doi: 10.1016/j.cell.2016.08.030
75. Dart RJ, Zlatareva I, Vantourout P, Theodoridis E, Amar A, Kannambath S, et al. Conserved γδ T cell selection by BTNL proteins limits progression of human inflammatory bowel disease. Sci (New York N.Y.). (2023) 381:eadh0301. doi: 10.1126/science.adh0301
76. Suzuki T, Kilbey A, Casa-Rodríguez N, Lawlor A, Georgakopoulou A, Hayman H, et al. β-catenin drives butyrophilin-like molecule loss and γδ T-cell exclusion in colon cancer. Cancer Immunol Res. (2023) 11:1137–55. doi: 10.1158/2326-6066.CIR-22-0644
77. Sperling AI, Linsley PS, Barrett TA, Bluestone JA. CD28-mediated costimulation is necessary for the activation of T cell receptor-gamma delta+ T lymphocytes. J Immunol. (1993) 151:6043–50. doi: 10.4049/jimmunol.151.11.6043
78. De Rosa SC, Mitra DK, Watanabe N, Herzenberg LA, Herzenberg LA, Roederer M. Vdelta1 and Vdelta2 gammadelta T cells express distinct surface markers and might be developmentally distinct lineages. J Leukoc Biol. (2001) 70:518–26. doi: 10.1189/jlb.70.4.518
79. Ribot JC, Debarros A, Mancio-Silva L, Pamplona A, Silva-Santos B. B7-CD28 costimulatory signals control the survival and proliferation of murine and human γδ T cells via IL-2 production. J Immunol. (2012) 189:1202–8. doi: 10.4049/jimmunol.1200268
80. Toutirais O, Cabillic F, Le Friec G, Salot S, Loyer P, Le Gallo M, et al. DNAX accessory molecule-1 (CD226) promotes human hepatocellular carcinoma cell lysis by Vgamma9Vdelta2 T cells. Eur J Immunol. (2009) 39:1361–8. doi: 10.1002/eji.200838409
81. Wang X, Mou W, Han W, Xi Y, Chen X, Zhang H, et al. Diminished cytolytic activity of γδ T cells with reduced DNAM-1 expression in neuroblastoma patients. Clin Immunol. (2019) 203:63–71. doi: 10.1016/j.clim.2019.04.006
82. Cazzetta V, Bruni E, Terzoli S, Carenza C, Franzese S, Piazza R, et al. NKG2A expression identifies a subset of human Vδ2 T cells exerting the highest antitumor effector functions. Cell Rep. (2021) 37:109871. doi: 10.1016/j.celrep.2021.109871
83. Halary F, Peyrat MA, Champagne E, Lopez-Botet M, Moretta A, Moretta L, et al. Control of self-reactive cytotoxic T lymphocytes expressing gamma delta T cell receptors by natural killer inhibitory receptors. Eur J Immunol. (1997) 27:2812–21. doi: 10.1002/eji.1830271111
84. Bauer S, Groh V, Wu J, Steinle A, Phillips JH, Lanier LL, et al. Activation of NK cells and T cells by NKG2D, a receptor for stress-inducible MICA. Science. (1999) 285:727–9. doi: 10.1126/science.285.5428.727
85. Gilfillan S, Ho EL, Cella M, Yokoyama WM, Colonna M. NKG2D recruits two distinct adapters to trigger NK cell activation and costimulation. Nat Immunol. (2002) 3:1150–5. doi: 10.1038/ni857
86. Billadeau DD, Upshaw JL, Schoon RA, Dick CJ, Leibson PJ. NKG2D-DAP10 triggers human NK cell-mediated killing via a Syk-independent regulatory pathway. Nat Immunol. (2003) 4:557–64. doi: 10.1038/ni929
87. Ibusuki A, Kawai K, Yoshida S, Uchida Y, Nitahara-Takeuchi A, Kuroki K, et al. NKG2D triggers cytotoxicity in murine epidermal γδ T cells via PI3K-dependent, Syk/ZAP70-independent signaling pathway. J Invest Dermatol. (2014) 134:396–404. doi: 10.1038/jid.2013.353
88. Feske S. Calcium signalling in lymphocyte activation and disease. Nat Rev Immunol. (2007) 7:690–702. doi: 10.1038/nri2152
89. Nedellec S, Sabourin C, Bonneville M, Scotet E. NKG2D costimulates human V gamma 9V delta 2 T cell antitumor cytotoxicity through protein kinase C theta-dependent modulation of early TCR-induced calcium and transduction signals. J Immunol. (2010) 185:55–63. doi: 10.4049/jimmunol.1000373
90. Correia DV, Fogli M, Hudspeth K, da Silva MG, Mavilio D, Silva-Santos B. Differentiation of human peripheral blood Vδ1+ T cells expressing the natural cytotoxicity receptor NKp30 for recognition of lymphoid leukemia cells. Blood. (2011) 118:992–1001. doi: 10.1182/blood-2011-02-339135
91. Cantoni C, Bottino C, Vitale M, Pessino A, Augugliaro R, Malaspina A, et al. NKp44, a triggering receptor involved in tumor cell lysis by activated human natural killer cells, is a novel member of the immunoglobulin superfamily. J Exp Med. (1999) 189:787–96. doi: 10.1084/jem.189.5.787
92. von-Lilienfeld-Toal M, Nattermann J, Feldmann G, Sievers E, Frank S, Strehl J, et al. Activated gammadelta T cells express the natural cytotoxicity receptor natural killer p 44 and show cytotoxic activity against myeloma cells. Clin Exp Immunol. (2006) 144:528–33. doi: 10.1111/j.1365-2249.2006.03078.x
93. Mikulak J, Oriolo F, Bruni E, Roberto A, Colombo FS, Villa A, et al. NKp46-expressing human gut-resident intraepithelial Vδ1 T cell subpopulation exhibits high antitumor activity against colorectal cancer. JCI Insight. (2019) 4:e125884. doi: 10.1172/jci.insight.125884
94. Zarobkiewicz MK, Bojarska-Junak AA. The mysterious actor-γδ T lymphocytes in chronic lymphocytic leukaemia (CLL). Cells. (2022) 11:661. doi: 10.3390/cells11040661
95. Almeida AR, Correia DV, Fernandes-Platzgummer A, da Silva CL, da Silva MG, Anjos DR, et al. Delta one T cells for immunotherapy of chronic lymphocytic leukemia: clinical-grade expansion/differentiation and preclinical proof of concept. Clin Cancer Res. (2016) 22:5795–804. doi: 10.1158/1078-0432.CCR-16-0597
96. Paul S, Singh AK, Shilpi, Lal G. Phenotypic and functional plasticity of gamma-delta (γδ) T cells in inflammation and tolerance. Int Rev Immunol. (2014) 33:537–58. doi: 10.3109/08830185.2013.863306
97. Rei M, Pennington DJ, Silva-Santos B. The emerging Protumor role of γδ T lymphocytes: implications for cancer immunotherapy. Cancer Res. (2015) 75:798–802. doi: 10.1158/0008-5472.CAN-14-3228
98. Ferrick DA, Schrenzel MD, Mulvania T, Hsieh B, Ferlin WG, Lepper H. Differential production of interferon-gamma and interleukin-4 in response to Th1- and Th2-stimulating pathogens by gamma delta T cells in vivo. Nature. (1995) 373:255–7. doi: 10.1038/373255a0
99. Pao W, Wen L, Smith AL, Gulbranson-Judge A, Zheng B, Kelsoe G, et al. Gamma delta T cell help of B cells is induced by repeated parasitic infection, in the absence of other T cells. Curr Biol. (1996) 6:1317–25. doi: 10.1016/s0960-9822(02)70718-5
100. Edelblum KL, Shen L, Weber CR, Marchiando AM, Clay BS, Wang Y, et al. Dynamic migration of γδ intraepithelial lymphocytes requires occludin. Proc Natl Acad Sci U.S.A. (2012) 109:7097–102. doi: 10.1073/pnas.1112519109
101. Jameson J, Ugarte K, Chen N, Yachi P, Fuchs E, Boismenu R, et al. A role for skin gammadelta T cells in wound repair. Science. (2002) 296:747–9. doi: 10.1126/science.1069639
102. Jameson JM, Cauvi G, Witherden DA, Havran WL. A keratinocyte-responsive gamma delta TCR is necessary for dendritic epidermal T cell activation by damaged keratinocytes and maintenance in the epidermis. J Immunol. (2004) 172:3573–9. doi: 10.4049/jimmunol.172.6.3573
103. Parkinson RM, Collins SL, Horton MR, Powell JD. Egr3 induces a Th17 response by promoting the development of γδ T cells. PloS One. (2014) 9:e87265. doi: 10.1371/journal.pone.0087265
104. Mathews JA, Williams AS, Brand JD, Wurmbrand AP, Chen L, Ninin FM, et al. γδ T cells are required for pulmonary IL-17A expression after ozone exposure in mice: role of TNFα. PloS One. (2014) 9:e97707. doi: 10.1371/journal.pone.0097707
105. Kober OI, Ahl D, Pin C, Holm L, Carding SR, Juge N. γδ T-cell-deficient mice show alterations in mucin expression, glycosylation, and goblet cells but maintain an intact mucus layer. Am J Physiol Gastrointest Liver Physiol. (2014) 306:G582–93:7. doi: 10.1152/ajpgi.00218.2013
106. Rani M, Zhang Q, Schwacha MG. Burn wound γδ T-cells support a Th2 and Th17 immune response. J Burn Care Res. (2014) 35:46–53. doi: 10.1097/01.bcr.0000440705.91099.cc
107. Bröker BM, Smith MD, Yoshino S, Lydyard PM, Emmrich F. Do gamma delta T cells play an important role in autoimmune disease? Behring Inst Mitt. (1991) 88:43–51.
108. Brennan F, Plater-Zyberk C, Maini RN, Feldmann M. Coordinate expansion of 'fetal type' lymphocytes (TCR gamma delta+T and CD5+B) in rheumatoid arthritis and primary Sjögren's syndrome. Clin Exp Immunol. (1989) 77:175–8.
109. Jacobs MR, Haynes BF. Increase in TCR gamma delta T lymphocytes in synovia from rheumatoid arthritis patients with active synovitis. J Clin Immunol. (1992) 12:130–8. doi: 10.1007/BF00918143
110. Mitogawa T, Nishiya K, Ota Z. Frequency of gamma delta T cells in peripheral blood, synovial fluid, synovial membrane and lungs from patients with rheumatoid arthritis. Acta Med Okayama. (1992) 46:371–9. doi: 10.18926/AMO/32664
111. Hassan J, Yanni G, Hegarty V, Feighery C, Bresnihan B, Whelan A. Increased numbers of CD5+ B cells and T cell receptor (TCR) gamma delta+ T cells are associated with younger age of onset in rheumatoid arthritis (RA). Clin Exp Immunol. (1996) 103:353–6. doi: 10.1111/j.1365-2249.1996.tb08287.x
112. Schwaneck EC, Renner R, Junker L, Tony HP, Kleinert S, Gernert M, et al. T cells, natural killer cells, and γδT cells in a large patient cohort with rheumatoid arthritis: influence of age and anti-rheumatic therapy. Scand J Rheumatol. (2020) 49:8–12. doi: 10.1080/03009742.2019.1634755
113. Lamour A, Jouen-Beades F, Lees O, Gilbert D, Le Loet X, Tron F. Analysis of T cell receptors in rheumatoid arthritis: the increased expression of HLA-DR antigen on circulating gamma delta+ T cells is correlated with disease activity. Clin Exp Immunol. (1992) 89:217–22. doi: 10.1111/j.1365-2249.1992.tb06935.x
114. Wysocki T, Olesińska M, Paradowska-Gorycka A. Current understanding of an emerging role of HLA-DRB1 gene in rheumatoid arthritis-from research to clinical practice. Cells. (2020) 9:1127. doi: 10.3390/cells9051127
115. Bank I. The role of gamma delta T cells in autoimmune rheumatic diseases. Cells. (2020) 9:462. doi: 10.3390/cells9020462
116. Kogure A, Miyata M, Nishimaki T, Kasukawa R. Proliferative response of synovial fluid mononuclear cells of patients with rheumatoid arthritis to mycobacterial 65 kDa heat shock protein and its association with HLA-DR+.gamma delta+ T cells. J Rheumatol. (1994) 21:1403–8.
117. Hu C, Qian L, Miao Y, Huang Q, Miao P, Wang P, et al. Antigen-presenting effects of effector memory Vγ9Vδ2 T cells in rheumatoid arthritis. Cell Mol Immunol. (2012) 9:245–54. doi: 10.1038/cmi.2011.50
118. Sutton CE, Lalor SJ, Sweeney CM, Brereton CF, Lavelle EC, Mills KH. Interleukin-1 and IL-23 induce innate IL-17 production from gammadelta T cells, amplifying Th17 responses and autoimmunity. Immunity. (2009) 31:331–41. doi: 10.1016/j.immuni.2009.08.001
119. Lee Y. The role of interleukin-17 in bone metabolism and inflammatory skeletal diseases. BMB Rep. (2013) 46:479–83. doi: 10.5483/bmbrep.2013.46.10.141
120. Narayan K, Sylvia KE, Malhotra N, Yin CC, Martens G, Vallerskog T, et al. Intrathymic programming of effector fates in three molecularly distinct γδ T cell subtypes. Nat Immunol. (2012) 13:511–8. doi: 10.1038/ni.2247
121. Dohlman TH, Chauhan SK, Kodati S, Hua J, Chen Y, Omoto M. The CCR6/CCL20 axis mediates Th17 cell migration to the ocular surface in dry eye disease. Invest Ophthalmol Vis Sci. (2013) 54:4081–91. doi: 10.1167/iovs.12-11216
122. Ramírez-Valle F, Gray EE, Cyster JG. Inflammation induces dermal Vγ4+ γδT17 memory-like cells that travel to distant skin and accelerate secondary IL-17-driven responses. Proc Natl Acad Sci U.S.A. (2015) 112:8046–51. doi: 10.1073/pnas.1508990112
123. Akitsu A, Ishigame H, Kakuta S, Chung SH, Ikeda S, Shimizu K, et al. IL-1 receptor antagonist-deficient mice develop autoimmune arthritis due to intrinsic activation of IL-17-producing CCR2(+)Vγ6(+)γδ T cells. Nat Commun. (2015) 6:7464. doi: 10.1038/ncomms8464
124. Moadab F, Khorramdelazad H, Abbasifard M. Role of CCL2/CCR2 axis in the immunopathogenesis of rheumatoid arthritis: Latest evidence and therapeutic approaches. Life Sci. (2021) 269:119034. doi: 10.1016/j.lfs.2021.119034
125. Yang X, Zhan N, Jin Y, Ling H, Xiao C, Xie Z, et al. Tofacitinib restores the balance of γδTreg/γδT17 cells in rheumatoid arthritis by inhibiting the NLRP3 inflammasome. Theranostics. (2021) 11:1446–57. doi: 10.7150/thno.47860
126. Pineda MA, McGrath MA, Smith PC, Al-Riyami L, Rzepecka J, Gracie JA, et al. The parasitic helminth product ES-62 suppresses pathogenesis in collagen-induced arthritis by targeting the interleukin-17-producing cellular network at multiple sites. Arthritis Rheum. (2012) 64:3168–78. doi: 10.1002/art.34581
127. Bajpai UD, Swainson LA, Mold JE, Graf JD, Imboden JB, McCune JM. A functional variant in FCRL3 is associated with higher Fc receptor-like 3 expression on T cell subsets and rheumatoid arthritis disease activity. Arthritis Rheum. (2012) 64:2451–9. doi: 10.1002/art.34457
128. Sánchez-Mejorada G, Rosales C. Signal transduction by immunoglobulin Fc receptors. J Leukoc Biol. (1998) 63:521–33. doi: 10.1002/jlb.63.5.521
129. Iwata S, Nakayamada S, Fukuyo S, Kubo S, Yunoue N, Wang SP. Activation of Syk in peripheral blood B cells in patients with rheumatoid arthritis: a potential target for abatacept therapy. Arthritis Rheumatol. (2015) 67:63–73. doi: 10.1002/art.38895
130. Jakus Z, Simon E, Balázs B, Mócsai A. Genetic deficiency of Syk protects mice from autoantibody-induced arthritis. Arthritis Rheum. (2010) 62:1899–910. doi: 10.1002/art.27438
131. Deng GM, Kyttaris VC, Tsokos GC. Targeting syk in autoimmune rheumatic diseases. Front Immunol. (2016) 7:78. doi: 10.3389/fimmu.2016.00078
132. Laggner U, Di Meglio P, Perera GK, Hundhausen C, Lacy KE, Ali N, et al. Identification of a novel proinflammatory human skin-homing Vγ9Vδ2 T cell subset with a potential role in psoriasis. J Immunol. (2011) 187:2783–93. doi: 10.4049/jimmunol.1100804
133. O'Brien RL, Born WK. Dermal γδ T cells–What have we learned? Cell Immunol. (2015) 296:62–9. doi: 10.1016/j.cellimm.2015.01.011
134. Gray EE, Suzuki K, Cyster JG. Cutting edge: Identification of a motile IL-17-producing gammadelta T cell population in the dermis. J Immunol. (2011) 186:6091–5. doi: 10.4049/jimmunol.1100427
135. Paradis TJ, Cole SH, Nelson RT, Gladue RP. Essential role of CCR6 in directing activated T cells to the skin during contact hypersensitivity. J Invest Dermatol. (2008) 128:628–33. doi: 10.1038/sj.jid.5701055
136. Kisielow J, Kopf M, Karjalainen K. SCART scavenger receptors identify a novel subset of adult gammadelta T cells. J Immunol. (2008) 181:1710–6. doi: 10.4049/jimmunol.181.3.1710
137. Cai Y, Shen X, Ding C, Qi C, Li K, Li X, et al. Pivotal role of dermal IL-17-producing γδ T cells in skin inflammation. Immunity. (2011) 35:596–610. doi: 10.1016/j.immuni.2011.08.001
138. Roark CL, Huang Y, Jin N, Aydintug MK, Casper T, Sun D, et al. A canonical Vγ4Vδ4+ γδ T cell population with distinct stimulation requirements which promotes the Th17 response. Immunol Res. (2013) 55:217–30. doi: 10.1007/s12026-012-8364-9
139. Roark CL, French JD, Taylor MA, Bendele AM, Born WK, O'Brien RL. Exacerbation of collagen-induced arthritis by oligoclonal, IL-17-producing gamma delta T cells. J Immunol. (2007) 179:5576–83. doi: 10.4049/jimmunol.179.8.5576
140. Malhotra N, Narayan K, Cho OH, Sylvia KE, Yin C, Melichar H, et al. A network of high-mobility group box transcription factors programs innate interleukin-17 production. Immunity. (2013) 38:681–93. doi: 10.1016/j.immuni.2013.01.010
141. Byamba D, Kim DY, Kim DS, Kim TG, Jee H, Kim SH, et al. Skin-penetrating methotrexate alleviates imiquimod-induced psoriasiform dermatitis via decreasing IL-17-producing gamma delta T cells. Exp Dermatol. (2014) 23:492–6. doi: 10.1111/exd.12448
142. Cai Y, Xue F, Qin H, Chen X, Liu N, Fleming C, et al. Differential roles of the mTOR-STAT3 signaling in dermal γδ T cell effector function in skin inflammation. Cell Rep. (2019) 27:3034–3048.e5. doi: 10.1016/j.celrep.2019.05.019
143. Agerholm R, Rizk J, Viñals MT, Bekiaris V. STAT3 but not STAT4 is critical for γδT17 cell responses and skin inflammation. EMBO Rep. (2019) 20:e48647. doi: 10.15252/embr.201948647
144. Li N, Xu W, Yuan Y, Ayithan N, Imai Y, Wu X, et al. Immune-checkpoint protein VISTA critically regulates the IL-23/IL-17 inflammatory axis. Sci Rep. (2017) 7:1485. doi: 10.1038/s41598-017-01411-1
145. Nadeem A, Ahmad SF, Al-Harbi NO, El-Sherbeeny AM, Alasmari AF, Alanazi WA, et al. Bruton's tyrosine kinase inhibitor suppresses imiquimod-induced psoriasis-like inflammation in mice through regulation of IL-23/IL-17A in innate immune cells. Int Immunopharmacol. (2020) 80:106215. doi: 10.1016/j.intimp.2020.106215
146. Pinget GV, Tan J, Niewold P, Mazur E, Angelatos AS, King NJC, et al. Immune modulation of monocytes dampens the IL-17+ γδ T cell response and associated psoriasis pathology in mice. J Invest Dermatol. (2020) 140:2398–2407.e1. doi: 10.1016/j.jid.2020.03.973
147. Hernández-Santana YE, Leon G, St Leger D, Fallon PG, Walsh PT. Keratinocyte interleukin-36 receptor expression orchestrates psoriasiform inflammation in mice. Life Sci Alliance. (2020) 3:e201900586. doi: 10.26508/lsa.201900586
148. Tokić S, Jirouš M, Plužarić V, Mihalj M, Šola M, Tolušić Levak M, et al. The miR-20a/miR-92b profile is associated with circulating γδ T-cell perturbations in mild psoriasis. Int J Mol Sci. (2023) 24:4323. doi: 10.3390/ijms24054323
149. Zheng QY, Xu F, Yang Y, Sun DD, Zhong Y, Wu S, et al. C5a/C5aR1 mediates IMQ-induced psoriasiform skin inflammation by promoting IL-17A production from γδ-T cells. FASEB J. (2020) 34:10590–604. doi: 10.1096/fj.202000384R
150. Ando N, Nakamura Y, Aoki R, Ishimaru K, Ogawa H, Okumura K, et al. Circadian gene clock regulates psoriasis-like skin inflammation in mice. J Invest Dermatol. (2015) 135:3001–8. doi: 10.1038/jid.2015.316
151. Wang S, Kozai M, Mita H, Cai Z, Masum MA, Ichii O, et al. REV-ERB agonist suppresses IL-17 production in γδT cells and improves psoriatic dermatitis in a mouse model. BioMed Pharmacother. (2021) 144:112283. doi: 10.1016/j.biopha.2021.112283
152. Tian J, Zhang D, Yao X, Huang Y, Lu Q. Global epidemiology of systemic lupus erythematosus: a comprehensive systematic analysis and modelling study. Ann Rheum Dis. (2023) 82:351–6. doi: 10.1136/ard-2022-223035
153. Stojan G, Petri M. Epidemiology of systemic lupus erythematosus: an update. Curr Opin Rheumatol. (2018) 30:144–50. doi: 10.1097/BOR.0000000000000480
154. Accapezzato D, Caccavale R, Paroli MP, Gioia C, Nguyen BL, Spadea L, et al. Advances in the pathogenesis and treatment of systemic lupus erythematosus. Int J Mol Sci. (2023) 24:6578. doi: 10.3390/ijms24076578
155. Olive C, Gatenby PA, Serjeantson SW. Restricted junctional diversity of T cell receptor delta gene rearrangements expressed in systemic lupus erythematosus (SLE) patients. Clin Exp Immunol. (1994) 97:430–8. doi: 10.1111/j.1365-2249.1994.tb06106.x
156. Chen H, You H, Wang L, Zhang X, Zhang J, He W. Chaperonin-containing T-complex protein 1 subunit ζ Serves as an autoantigen recognized by human Vδ2 γδ T cells in autoimmune diseases. J Biol Chem. (2016) 291:19985–93. doi: 10.1074/jbc.M115.700070
157. Wen L, Pao W, Wong FS, Peng Q, Craft J, Zheng B, et al. Germinal center formation, immunoglobulin class switching, and autoantibody production driven by "non alpha/beta" T cells. J Exp Med. (1996) 183:2271–82. doi: 10.1084/jem.183.5.2271
158. Peng SL, Craft J. T cells in murine lupus: propagation and regulation of disease. Mol Biol Rep. (1996) 23:247–51. doi: 10.1007/BF00351176
159. Rezende RM, Lanser AJ, Rubino S, Kuhn C, Skillin N, Moreira TG, et al. γδ T cells control humoral immune response by inducing T follicular helper cell differentiation. Nat Commun. (2018) 9:3151. doi: 10.1038/s41467-018-05487-9
160. Riccieri V, Spadaro A, Parisi G, Taccari E, Moretti T, Bernardini G, et al. Down-regulation of natural killer cells and of gamma/delta T cells in systemic lupus erythematosus. Does it correlate to autoimmunity and to laboratory indices of disease activity? Lupus. (2000) 9:333–7. doi: 10.1191/096120300678828460
161. Lu Z, Su D, Wang D, Li X, Feng X, Sun L. Elevated apoptosis and impaired proliferation contribute to downregulated peripheral γ δ T cells in patients with systemic lupus erythematosus. Clin Dev Immunol. (2013) 2013(2013):405395. doi: 10.1155/2013/405395
162. Yuan S, Wang C, Zeng Y, Li J, Li W, He Z, et al. Aberrant phenotypes of circulating γδ-T cells may be involved in the onset of systemic lupus erythematosus. Lupus. (2024) 33:587–97. doi: 10.1177/09612033241240864
163. Koga T, Ichinose K, Tsokos GC. T cells and IL-17 in lupus nephritis. Clin Immunol. (2017) 185:95–9. doi: 10.1016/j.clim.2016.04.010
164. Koga T, Endo Y, Umeda M, Sato T, Mizunoo Y, Furukawa K, et al. Reduction in the percentage of circulating variable delta 2 T cells in systemic lupus erythematosus. Clin Immunol. (2020) 220:108577. doi: 10.1016/j.clim.2020.108577
165. Du B, Fan X, Lei F, Zhang S, Li G, Xi X. Comparative transcriptome analysis reveals a potential role for CaMK4 in γδT17 cells from systemic lupus erythematosus patients with lupus nephritis. Int Immunopharmacol. (2020) 80:106139. doi: 10.1016/j.intimp.2019.106139
166. Pattanaik D, Brown M, Postlethwaite BC, Postlethwaite AE. Pathogenesis of systemic sclerosis. Front Immunol. (2015) 6:272. doi: 10.3389/fimmu.2015.00272
167. White B, Yurovsky VV. Oligoclonal expansion of V delta 1+ gamma/delta T-cells in systemic sclerosis patients. Ann N Y Acad Sci. (1995) 756:382–91. doi: 10.1111/j.1749-6632.1995.tb44542.x
168. Henriques A, Silva C, Santiago M, Henriques MJ, Martinho A, Trindade H, et al. Subset-specific alterations in frequencies and functional signatures of γδ T cells in systemic sclerosis patients. Inflammation Res. (2016) 65:985–94. doi: 10.1007/s00011-016-0982-6
169. Holcombe RF, Baethge BA, Wolf RE, Betzing KW, Stewart RM. Natural killer cells and gamma delta T cells in scleroderma: relationship to disease duration and anti-Scl-70 antibodies. Ann Rheum Dis. (1995) 54:69–72. doi: 10.1136/ard.54.1.69
170. White B. Immunopathogenesis of systemic sclerosis. Rheum Dis Clin North Am. (1996) 22:695–708. doi: 10.1016/s0889-857x(05)70296-9
171. Giacomelli R, Matucci-Cerinic M, Cipriani P, Ghersetich I, Lattanzio R, Pavan A, et al. Circulating Vdelta1+ T cells are activated and accumulate in the skin of systemic sclerosis patients. Arthritis Rheum. (1998) 41:327–34. doi: 10.1002/1529-0131(199802)41:2<327::AID-ART17>3.0.CO;2-S
172. Cook-Mills JM. VCAM-1 signals during lymphocyte migration: role of reactive oxygen species. Mol Immunol. (2002) 39:499–508. doi: 10.1016/s0161-5890(02)00206-7
173. Ueda-Hayakawa I, Hasegawa M, Hamaguchi Y, Takehara K, Fujimoto M. Circulating γ/δ T cells in systemic sclerosis exhibit activated phenotype and enhance gene expression of proalpha2(I) collagen of fibroblasts. J Dermatol Sci. (2013) 69:54–60. doi: 10.1016/j.jdermsci.2012.10.003
174. Bendersky A, Markovits N, Bank I. Vgamma9+ gammadelta T cells in systemic sclerosis patients are numerically and functionally preserved and induce fibroblast apoptosis. Immunobiology. (2010) 215:380–94. doi: 10.1016/j.imbio.2009.05.012
175. Giacomelli R, Cipriani P, Fulminis A, Nelson JL, Matucci-Cerinic M. Gamma/delta T cells in placenta and skin: their different functions may support the paradigm of microchimerism in systemic sclerosis. Clin Exp Rheumatol. (2004) 22:S28–30.
176. Deniger DC, Maiti SN, Mi T, Switzer KC, Ramachandran V, Hurton LV, et al. Activating and propagating polyclonal gamma delta T cells with broad specificity for Malignancies. Clin Cancer Res. (2014) 20:5708–19. doi: 10.1158/1078-0432.CCR-13-3451
177. Yu X, Song L, Cen L, Cao B, Tao R, Shen Y, et al. Pan-cancer γδ TCR analysis uncovers clonotype diversity and prognostic potential. Cell Rep Med. (2024) 5:101764. doi: 10.1016/j.xcrm.2024.101764
178. Todaro M, D'Asaro M, Caccamo N, Iovino F, Francipane MG, Meraviglia S, et al. Efficient killing of human colon cancer stem cells by gammadelta T lymphocytes. J Immunol. (2009) 182:7287–96. doi: 10.4049/jimmunol.0804288
179. Xiang Z, Liu Y, Zheng J, Liu M, Lv A, Gao Y, et al. Targeted activation of human Vγ9Vδ2-T cells controls epstein-barr virus-induced B cell lymphoproliferative disease. Cancer cel. (2014) 26:565–76. doi: 10.1016/j.ccr.2014.07.026
180. Dokouhaki P, Schuh NW, Joe B, Allen CA, Der SD, Tsao MS, et al. NKG2D regulates production of soluble TRAIL by ex vivo expanded human γδ T cells. Eur J Immunol. (2013) 43:3175–82. doi: 10.1002/eji.201243150
181. Groh V, Smythe K, Dai Z, Spies T. Fas-ligand-mediated paracrine T cell regulation by the receptor NKG2D in tumor immunity. Nat Immunol. (2006) 7:755–62. doi: 10.1038/ni1350
182. Raulet DH, Guerra N. Oncogenic stress sensed by the immune system: role of natural killer cell receptors. Nat Rev Immunol. (2009) 9:568–80. doi: 10.1038/nri2604
183. Correia DV, Lopes A, Silva-Santos B. Tumor cell recognition by γδ T lymphocytes: T-cell receptor vs. NK-cell receptors Oncoimmunol. (2013) 2:e22892. doi: 10.4161/onci.22892
184. Zhao L, Chang WC, Xiao Y, Liu HW, Liu P. Methylerythritol phosphate pathway of isoprenoid biosynthesis. Annu Rev Biochem. (2013) 82:497–530. doi: 10.1146/annurev-biochem-052010-100934
185. Yang Y, Li L, Yuan L, Zhou X, Duan J, Xiao H, et al. A structural change in butyrophilin upon phosphoantigen binding underlies phosphoantigen-mediated Vγ9Vδ2 T cell activation. Immunity. (2019) 50:1043–1053.e5. doi: 10.1016/j.immuni.2019.02.016
186. Sun LL, Zhang ZL, Li YJ, Wang SD, Li HY, Li BH, et al. Zoledronic acid sensitizes rhabdomyosarcoma cells to cytolysis mediated by human γδ T cells. Oncol Lett. (2017) 14:5597–604. doi: 10.3892/ol.2017.6894
187. Suzuki T, Terao S, Acharya B, Naoe M, Yamamoto S, Okamura H, et al. The antitumour effect of {gamma}{delta} T-cells is enhanced by valproic acid-induced up-regulation of NKG2D ligands. Anticancer Res. (2010) 30:4509–13.
188. Lança T, Correia DV, Moita CF, Raquel H, Neves-Costa A, Ferreira C, et al. The MHC class Ib protein ULBP1 is a nonredundant determinant of leukemia/lymphoma susceptibility to gammadelta T-cell cytotoxicity. Blood. (2010) 115:2407–11. doi: 10.1182/blood-2009-08-237123
189. Street SE, Hayakawa Y, Zhan Y, Lew AM, MacGregor D, Jamieson AM, et al. Innate immune surveillance of spontaneous B cell lymphomas by natural killer cells and gammadelta T cells. J Exp Med. (2004) 199:879–84. doi: 10.1084/jem.20031981
190. Liu Z, Eltoum IE, Guo B, Beck BH, Cloud GA, Lopez RD. Protective immunosurveillance and therapeutic antitumor activity of gammadelta T cells demonstrated in a mouse model of prostate cancer. J Immunol. (2008) 180:6044–53. doi: 10.4049/jimmunol.180.9.6044
191. He W, Hao J, Dong S, Gao Y, Tao J, Chi H, et al. Naturally activated V gamma 4 gamma delta T cells play a protective role in tumor immunity through expression of eomesodermin. J Immunol. (2010) 185:126–33. doi: 10.4049/jimmunol.0903767
192. Chauvin C, Joalland N, Perroteau J, Jarry U, LaFrance L, Willem C, et al. NKG2D controls natural reactivity of Vγ9Vδ2 T lymphocytes against mesenchymal glioblastoma cells. Clin Cancer Res. (2019) 25:7218–28. doi: 10.1158/1078-0432.CCR-19-0375
193. Schönefeldt S, Wais T, Herling M, Mustjoki S, Bekiaris V, Moriggl R, et al. The diverse roles of γδ T cells in cancer: from rapid immunity to aggressive lymphoma. Cancers. (2021) 13:6212. doi: 10.3390/cancers13246212
194. Oberg HH, Kellner C, Gonnermann D, Sebens S, Bauerschlag D, Gramatzki M, et al. Tribody ((HER2)2xCD16) is more effective than trastuzumab in enhancing γδ T cell and natural killer cell cytotoxicity against HER2-expressing cancer cells. Front Immunol. (2018) 9:814. doi: 10.3389/fimmu.2018.00814
195. Cullen SP, Brunet M, Martin SJ. Granzymes in cancer and immunity. Cell Death Differ. (2010) 17:616–23. doi: 10.1038/cdd.2009.206
196. Huang Y, Getahun A, Heiser RA, Detanico TO, Aviszus K, Kirchenbaum GA, et al. γδ T cells shape preimmune peripheral B cell populations. J Immunol. (2016) 196:217–31. doi: 10.4049/jimmunol.1501064
197. Caccamo N, Battistini L, Bonneville M, Poccia F, Fournié JJ, Meraviglia S, et al. CXCR5 identifies a subset of Vgamma9Vdelta2 T cells which secrete IL-4 and IL-10 and help B cells for antibody production. J Immunol. (2006) 177:5290–5. doi: 10.4049/jimmunol.177.8.5290
198. Del Prete A, Salvi V, Soriani A, Laffranchi M, Sozio F, Bosisio D, et al. Dendritic cell subsets in cancer immunity and tumor antigen sensing. Cell Mol Immunol. (2023) 20:432–47. doi: 10.1038/s41423-023-00990-6
199. Ismaili J, Olislagers V, Poupot R, Fournié JJ, Goldman M. Human gamma delta T cells induce dendritic cell maturation. Clin Immunol. (2002) 103:296–302. doi: 10.1006/clim.2002.5218
200. Dar AA, Patil RS, Chiplunkar SV. Insights into the relationship between toll like receptors and gamma delta T cell responses. Front Immunol. (2014) 5:366. doi: 10.3389/fimmu.2014.00366
201. Petrasca A, Derek DG. Human Vδ2(+) γδ T cells differentially induce maturation, cytokine production, and alloreactive T cell stimulation by dendritic cells and B cells. Front Immunol. (2014) 5:650. doi: 10.3389/fimmu.2014.00650
202. Patil RS, Shah SU, Shrikhande SV, Goel M, Dikshit RP, Chiplunkar SV. IL17 producing γδT cells induce angiogenesis and are associated with poor survival in gallbladder cancer patients. Int J Cancer. (2016) 139:4. doi: 10.1002/ijc.30134
203. Ma S, Cheng Q, Cai Y, Gong H, Wu Y, Yu X, et al. IL-17A produced by γδ T cells promotes tumor growth in hepatocellular carcinoma. Cancer Res. (2014) 74:1969–82. doi: 10.1158/0008-5472.CAN-13-2534
204. Regulation (EU) No 536/2014 of the European Parliament and of the Council of 16 April 2014 on clinical trials on medicinal products for human use, and repealing Directive 2001/20/EC Text with EEA relevance (2014). Available online at: https://eur-lex.europa.eu/eli/reg/2014/536/oj (Accessed September 27, 2024).
205. Clinical trials' transition to new EU system . Available online at: https://euclinicaltrials.eu/about-this-website/?lang=pl (Accessed September 27 2024).
206. Scavone C, di Mauro G, Pietropaolo M, Alfano R, Berrino L, Rossi F, et al. The European clinical trials regulation (No 536/2014): changes and challenges. Expert Rev Clin Pharmacol. (2019) 12:1027–32. doi: 10.1080/17512433.2019.1680282
207. Orphan drugs database . Available online at: https://www.orpha.net/en/drug/trade_substancemenu (Accessed September 27, 2024).
208. Yan W, Dunmall LSC, Lemoine NR, Wang Y, Wang Y, Wang P. The capability of heterogeneous γδ T cells in cancer treatment. Front Immunol. (2023) 14:1285801. doi: 10.3389/fimmu.2023.1285801
209. National Library of Medicine.8600 Rockville Pike, Bethesda, MD 20894. Available online at: https://clinicaltrials.gov/ (Accessed September 27, 2024).
210. Pressey JG, Adams J, Harkins L, Kelly D, You Z, Lamb L. In vivo expansion and activation of γδ T cells as immunotherapy for refractory neuroblastoma: A phase 1 study. Medicine. (2016) 95:e4909. doi: 10.1097/MD.0000000000004909
211. Markovits N, Loebstein R, Bank I. Immune-mediated syndromes following intravenous bisphosphonate therapy. Inflammopharmacology. (2017) 25:665–71. doi: 10.1007/s10787-017-0365-9
212. Revesz IA, Joyce P, Ebert LM, Prestidge CA. Effective γδ T-cell clinical therapies: current limitations and future perspectives for cancer immunotherapy. Clin Transl Immunol. (2024) 13:e1492. doi: 10.1002/cti2.1492
213. Tanaka Y, Murata-Hirai K, Iwasaki M, Matsumoto K, Hayashi K, Kumagai A, et al. Expansion of human γδ T cells for adoptive immunotherapy using a bisphosphonate prodrug. Cancer Sci. (2018) 109:587–99. doi: 10.1111/cas.13491
214. Van Acker HH, Anguille S, Willemen Y, Van den Bergh JM, Berneman ZN, Lion E, et al. Interleukin-15 enhances the proliferation, stimulatory phenotype, and antitumor effector functions of human gamma delta T cells. J Hematol Oncol. (2016) 9:101. doi: 10.1186/s13045-016-0329-3
215. Schiller CB, Braciak TA, Fenn NC, Seidel UJ, Roskopf CC, Wildenhain S, et al. CD19-specific triplebody SPM-1 engages NK and γδ T cells for rapid and efficient lysis of Malignant B-lymphoid cells. Oncotarget. (2016) 7:83392–408. doi: 10.18632/oncotarget.13110
216. Serrano R, Wesch D, Kabelitz D. Activation of human γδ T cells: modulation by toll-like receptor 8 ligands and role of monocytes. Cells. (2020) 9:713. doi: 10.3390/cells9030713
217. de Vries NL, van de Haar J, Veninga V, Chalabi M, Ijsselsteijn ME, van der Ploeg M, et al. γδ T cells are effectors of immunotherapy in cancers with HLA class I defects. Nature. (2023) 613:743–50. doi: 10.1038/s41586-022-05593-1
218. Rancan C, Arias-Badia M, Dogra P, Chen B, Aran D, Yang H, et al. Exhausted intratumoral Vδ2- γδ T cells in human kidney cancer retain effector function. Nat Immunol. (2023) 24:612–24. doi: 10.1038/s41590-023-01448-7
219. Rozenbaum M, Meir A, Aharony Y, Itzhaki O, Schachter J, Bank I, et al. Gamma-delta CAR-T cells show CAR-directed and independent activity against leukemia. Front Immunol. (2020) 11:1347. doi: 10.3389/fimmu.2020.01347
220. U.S. Food and Drug Administration. FDA News Release, FDA approves CAR-T cell therapy to treat adults with certain types of large B-cell lymphoma (2017). Available online at: https://www.fda.gov/news-events/press-announcements/fda-approves-car-t-cell-therapy-treat-adults-certain-types-large-b-cell-lymphomahttps://clinicaltrials.gov/ (Accessed September 28, 2024).
221. Jhita N, Raikar S. Allogeneic gamma delta T cells as adoptive cellular therapy for hematologic Malignancies. Explor Immunol. (2022) 2:334–50. doi: 10.37349/ei.2022.00054
222. Guffroy A, Jacquel L, Guffroy B, Martin T. CAR-T cells for treating systemic lupus erythematosus: A promising emerging therapy. Joint Bone Spine. (2024) 91:5. doi: 10.1016/j.jbspin.2024.105702
223. Teng S, Tian Y, Luo N, Zheng Q, Shao M, Li L. Efficacy and safety of an anti-CD20 monoclonal antibody, rituximab, for lupus nephritis: A meta-analysis. Int J Rheum Dis. (2022) 25:101–9. doi: 10.1111/1756-185X.14240
224. Huang RR, Chen Z, Kroeger N, Pantuck A, Said J, Kluger HM, et al. CD70 is consistently expressed in primary and metastatic clear cell renal cell carcinoma. Clin Genitourin Cancer. (2024) 22:347–53. doi: 10.1016/j.clgc.2023.12.003
225. Mehra N, Voortman J, Wise DR, Calvo E, Piulats JM, Gonzalez-Billalabeitia E, et al. Phase I/II trial of LAVA-1207, a novel bispecific gamma-delta T-cell engager alone, or with low dose IL-2 or pembrolizumab, in metastatic castration resistant prostate cancer (mCRPC). J Clin Oncol. (2024) 14:16. doi: 10.1200/JCO.2024.42.16_suppl.TPS2672
226. LAVA therapeutics. LAVA Therapeutics Receives FDA Orphan Drug Designation for LAVA-051 for the Treatment of Chronic Lymphocytic Leukemia (2021). Available online at: https://ir.lavatherapeutics.com/node/6896/pdf (Accessed September 28, 2024).
227. Rossini M, Adami G, Viapiana O, Idolazzi L, Fassio A, Giollo A, et al. Rheumatoid arthritis, γδ T cells and bisphosphonates. Ann Rheum Dis. (2018) 77:e57. doi: 10.1136/annrheumdis-2017-212510
228. Nguyen CT, Maverakis E, Eberl M, Adamopoulos IE. γδ T cells in rheumatic diseases: from fundamental mechanisms to autoimmunity. Semin Immunopathol. (2019) 41:595–605. doi: 10.1007/s00281-019-00752-5
229. Gao Z, Bai Y, Lin A, Jiang A, Zhou C, Cheng Q, et al. Gamma delta T-cell-based immune checkpoint therapy: attractive candidate for antitumor treatment. Mol Cancer. (2023) 22:31. doi: 10.1186/s12943-023-01722-0
Keywords: γδ T cells, autoimmunity, diseases, novel therapies, treatment
Citation: Biały S and Bogunia-Kubik K (2025) Uncovering the mysteries of human gamma delta T cells: from origins to novel therapeutics. Front. Immunol. 16:1543454. doi: 10.3389/fimmu.2025.1543454
Received: 11 December 2024; Accepted: 24 March 2025;
Published: 10 April 2025.
Edited by:
Ilan Bank, Sheba Medical Center, IsraelReviewed by:
Rafael Rezende, Brigham and Women’s Hospital and Harvard Medical School, United StatesOndrej Stepanek, Institute of Molecular Genetics (ASCR), Czechia
Copyright © 2025 Biały and Bogunia-Kubik. This is an open-access article distributed under the terms of the Creative Commons Attribution License (CC BY). The use, distribution or reproduction in other forums is permitted, provided the original author(s) and the copyright owner(s) are credited and that the original publication in this journal is cited, in accordance with accepted academic practice. No use, distribution or reproduction is permitted which does not comply with these terms.
*Correspondence: Sylwia Biały, c3lsd2lhLmJpYWx5QGhpcnN6ZmVsZC5wbA==