- 1Department of Microbiology and Immunology, Medical School of Southeast University, Nanjing, China
- 2Blood Group Reference Laboratory, Nanjing Red Cross Blood Center, Nanjing, China
- 3State Key Laboratory of Pathogen and Biosecurity, Academy of Military Medical Sciences, Beijing, China
- 4Laboratory of Advanced Biotechnology, Academy of Military Medical Sciences, Beijing, China
Since the outbreak of novel coronavirus pneumonia (COVID-19), numerous T-cell epitopes in severe acute respiratory syndrome coronavirus 2 (SARS-CoV-2) proteome have been reported. However, most of the identified CD8+ T-cell epitopes have been restricted primarily by HLA-A allotypes. The epitopes restricted by HLA-B and HLA-C allotypes are limited. This study focuses on the screening of T-cell epitopes restricted by 13 prevalent HLA-B and 13 prevalent HLA-C allotypes, which cover over 70% and 90% of the Chinese and Northeast Asian populations, respectively. Totally, 67 HLA-B restricted and 53 HLA-C restricted epitopes were validated as immunogenic epitopes with a herd predominance rate by peptide-PBMCs ex vivo coculture experiments using the PBMCs from convalescent Chinese cohort. In addition, 26 transfected cell lines expressing indicated HLA-B or HLA-C allotype were established, and used in the competitive peptide binding assays to define the affinities and cross-restriction of each validated epitope binding to HLA-B or HLA-C allotypes. These data will facilitate the design of T-cell-directed vaccines and SARS-CoV-2-specific T-cell detection tools tailored to the Northeast Asian population. The herd test of functionally validated T-cell epitopes, and the competitive peptide binding assay onto cell line array expressing prevalent HLA allotypes may serve as an additional criterion for selecting T-cell epitopes used in vaccine.
Introduction
During the coronavirus disease 2019 (COVID-19) pandemic, extensive research has highlighted the significant role of T cells in combating severe acute respiratory syndrome coronavirus 2 (SARS-CoV-2) infections (1, 2). Current vaccines mostly target the spike protein or the RBD region of the spike protein, aiming to generate neutralizing antibodies that prevent viral entry into host cells. However, the rapid decline of neutralizing antibodies (3–5) and constant emergence of viral variants have significantly reduced the effectiveness of current vaccines (6–10). The widely administered vaccines include inactivated virus vaccines (CoronaVac and BBIBP-CorV), recombinant protein vaccines (NVX-CoV2373), adenovirus vector vaccines (ChAdOx1nCoV-19 and Ad26.COV2.S), and mRNA vaccines (BNT162b2 and mRNA-1273) (11, 12). Among these, only adenovirus vector and mRNA vaccines can elicit efficient T-cell immunity compared to other forms (13, 14). Enormous studies have further demonstrated that mRNA vaccines offer the highest and most stable protection rates (11, 15), which indicates the necessity for designing an ideal vaccine to induce both humoral and cellular immunity, thereby providing a more robust antiviral response. However, the T-cell epitopes derived from mRNA or adenovirus vector vaccines are limited to the spike protein, an area highly susceptible to mutations; this may reduce protective efficacy against viral variants. Multipeptide vaccines based on T-cell epitopes are powerful tools to elicit cellular immunity and generate long-lasting memory T cells. However, due to the herd polymorphism of HLA and the limited number of peptides encompassed in a multipeptide vaccine, it is essential to select epitope peptides that possess strong immunogenicity and a large herd coverage. For the Northeast Asian population, the broad-spectrum and predominant T-cell epitopes of SARS-CoV-2 remain limited.
In our previous work, a repertoire consisting of 120 CD8+ T-cell epitopes restricted by 13 predominant HLA-A allotypes in China and Northeast Asia has been defined by cellular functional experiments (16). This study focuses on the screening of T-cell epitopes restricted by 13 predominant HLA-B and 13 prevalent HLA-C allotypes, which cover over 70% and 90% of the Chinese and Northeast Asian populations, respectively. Candidate epitopes were selected by in silico prediction and then cocultured ex vivo with the peripheral blood mononuclear cells (PBMCs) from convalescent Chinese cohort to identify the immunogenic epitopes, which activate epitope-specific memory CD8+ T cells in PBMCs. Finally, 67 HLA-B restricted and 53 HLA-C restricted epitopes were validated with a herd predominance rate. Among the 67 HLA-B restricted epitopes, 26, 36, and 5 ones had herd positive rate of 20%–36.7%, 10%–19%, and <10%, respectively. Of the 53 HLA-C restricted epitopes, 15, 27, and 11 ones had herd positive rate of 20%–27%, 10%–19%, and <10%, respectively. Furthermore, the binding affinities of each validated epitope to HLA-B or C allotypes were assessed by using an array of transfected cell lines expressing indicated HLA-B or HLA-C molecule and competitive peptide binding assay.
Materials and methods
Ethical approval, PBMC preparation, and HLA genotyping
The blood samples of convalescents after SARS-CoV-2 infection were collected in the Blood Component Preparation Section of Nanjing Red Cross Blood Center in the form of white blood cell filter trays after red blood cell filtering from March 2022 to March 2024 (the late stage of SARS-CoV-2 outbreak). These blood donors were SARS-CoV-2 negative as confirmed by PCR test upon blood donation but have been defined infection history of SARS-CoV-2. For each donor, a written informed consent was obtained upon blood donation at Nanjing Red Cross Blood Center and informed that their blood samples would be used for patient treatment and scientific research. The collection and use of human blood samples were approved by the Clinical Ethics Committee of Nanjing Red Cross Blood Center (ref: 2022-015-01).
PBMCs were freshly isolated from the white blood cell filter tray and then immediately separated using Ficoll–Paque density-gradient centrifugation. Fresh PBMCs were either used directly or cryopreserved at −80°C for further testing. Genomic DNA was extracted from each PBMCs sample using the Blood DNA Extraction kit from Tiangen Biotech Co., Ltd (Beijing, China) and followed by PCR-sequencing-based typing on HLA-B or HLA-C loci (exon 2 and 3) using the primers recommended by the International HLA working group (IHWG). Sequence alignment was carried out using the SOAPTying software.
In silico prediction of T-cell epitopes and peptide synthesis
T-cell epitopes spanning the spike, envelope, membrane, nucleocapsid, and RNA-dependent RNA polymerase (S, E, M, N, RdRP) proteins of SARS-CoV-2 (Wuhan strain) and presenting different HLA-B or HLA-C allotypes were predicted using five online epitope prediction tools (NetMHCpan 4.1 EL, NetMHCpan 4.1 BA, SMMPMSEC, Consensus, and SYFPEITHI). For each HLA-B or HLA-C allotype and each protein, up to 20 peptides (9 or 10 amino acids/peptide) presenting high scores (highest affinity) in at least two prediction tools were selected as candidate epitopes for further validation. The peptides of candidate epitopes were synthesized by Nanjing GenScript Biotech Co., Ltd. (Nanjing, China) and exhibited a purity >95% as confirmed by HPLC and mass spectrometry analysis. The lyophilized peptides were reconstituted in a DMSO-PBS solution to prepare stock solutions at a concentration of 2 mg/mL for subsequent cellular functional experiments.
Cell lines and culture
The 293T and HMy2.CIR cell lines were cultured in DMEM and RPMI1640 medium containing 10% fetal calf serum (FBS) and 1% penicillin/streptomycin, respectively. HMy2.CIR cells expressing indicated HLA-B or HLA-C molecule were cultured in RPMI1640 medium containing 10% fetal calf serum at the presence of low concentration of puromycin (1 μg/mL). All cell lines were identified by single nucleotide polymorphism (SNP) testing and incubated at 37°C in an incubator with 5% CO2.
Generation of HMy2.CIR cell lines expressing the indicated HLA-B or HLA-C molecule
The lentiviral vectors pLenti-EF1a-Puro-T2A-GFP expressing the indicated HLA-B or HLA-C molecules were generated in Sangon Biotech Co., Ltd (Shanghai, China) and then were transfected into 293T cells, along with packaging plasmids (pMD2.g and PAX2), using Lipofectamine 3000 (Thermo Fisher Scientific, Waltham, MA, USA). Lentiviral particles were concentrated through ultracentrifugation and stored at −80°C. All plasmids were stored and verified in the Department of Microbiology and Immunology at Medical School of Southeast University.
HMy2.CIR cell line was purchased (Zhongqiao Xinzhou Biotech, Shanghai, China) and maintained in complete IMDM medium with 10% FCS and 1% penicillin/streptomycin in T25 flasks for 48 h, after which the medium was replaced with fresh medium containing lentiviral particles and polybrene (8 µg/mL, Obio Technology, Shanghai, China) for another 24-h culture. Following this, the CIR cells stably expressing HLA-B or HLA-C molecules were selected using puromycin (2 µg/mL, Sigma-Aldrich, St. Louis, MO, USA) and were then stained with APC-conjugated anti-human HLA-A/B/C antibodies (W6/32 clone, BioLegend, San Diego, CA, USA) followed by flow cytometry using Guava® easyCyte™ HT (Luminex Corporation, Austin, TX). The CIR cells transduced with exogenous HLA-B or HLA-C molecular exhibited a significantly higher mean fluorescence intensity (MFI) compared to those transduced with the empty vector.
Peptide-PBMCs coculture experiment using convalescents’ PBMCs and intracellular IFN-γ staining
Fresh PBMCs (5×105) from each convalescent individual were stimulated with single candidate epitope peptide (20 μg/mL) or without peptide (negative control) for 1 h. Subsequently, a mixture of Brefeldin A and Monensin (BioLegend) was added to the cells for an additional 6-h coculture. After that, the cells were harvested, washed, and blocked with human Fc receptor-blocking reagent (Miltenyi Biotec) for 20 min at 4°C. Then, surface markers were stained with antibodies including FITC-conjugated anti-human CD3 (UCHT1 clone, BioLegend) and APC-conjugated anti-human CD8 (SK1 clone, BioLegend) at pre-titrated concentrations for 30 min at 4°C. After washing, the cells were fixed, permeabilized using Fix&Perm kit (Multi Sciences Co., Ltd, Shanghai, China), and then incubated with PE-conjugated anti-human IFN-γ antibody (B27 clone, BD Bioscience, San Jose, CA, USA) for another 30 min at 4°C. After washing, the cells were harvested and analyzed by flow cytometry (Guava® easyCyte™ HT, Luminex Corporation) to determine the frequencies of IFN-γ+ cells in CD3+/CD8+ populations.
Competitive peptide binding assay using transfected HMy2.CIR cell lines
The competitive peptide binding assay was used to evaluate the affinity of epitope peptide binding to HLA-B or HLA-C molecule as described in detail in our previous work (16). Briefly, the HMy2.CIR cells constantly expressing indicated HLA-B or HLA-C molecule were incubated with Cy5-labeled reference peptide (defined in published papers or in house) and peptide to be tested. In parallel, the max control well (CIR cells and Cy5-reference peptide) and back group well (CIR cells alone) were performed. After a defined incubation period, the unbound peptides were removed, and the relative binding affinity of the test peptide to the HLA molecule was quantified by the declined MFI of reference peptide binding to the CIR cell surface. The Cy5-labeled reference peptides for each HLA-B or HLA-C molecule are listed in following Table 1. The relative binding affinity for given peptide were calculated by the formula: competitive binding (%) = [1 − (MFIsample − MFIbackground)/(MFImax − MFIbackground)] × 100%. The IC50 is the concentration of unlabeled competitor peptide required to inhibit the binding of the Cy5-labeled reference peptide by 50%, which was calculated from the competitive binding inhibition (%) of the sample at 5 and 15 μM. The binding affinity of each unlabeled peptide with the indicated HLA-B or HLA-C molecule was assessed according to the IC50 value. IC50 < 5 μM (5 μM inhibition >50%) means high binding affinity, 5 μM< IC50 < 15 μM (5 μM inhibition <50% but 15 μM inhibition > 50%) means intermediate binding affinity, IC50 > 15 μM means low or no binding affinity (5 μM inhibition 20%–50% or 15 μM inhibition 30%–50% means low binding affinity; 5 μM inhibition < 20% or 15 μM inhibition < 30% means no binding affinity).
Results
A total of 272 HLA-B restricted and 407 HLA-C restricted CD8+ T-cell epitopes were selected as candidate epitopes by in silico prediction
A bunch of 9- or 10-mer epitopes restricted by 13 prevalent HLA-B allotypes (HLA-B4601, B4001, B5801, B1502, B5101, B1301, B1302, B1501, B3501, B4403, B5201, BB4801, and B0702, with a gene frequency of >1% for each allotype and a total gene frequency of >70% in Northeast Asia) and 13 prevalent HLA-C allotypes (HLA-C0102, C0602, C0702, C0801, C0304, C0302, C0303, C0401, C1402, C1202, C1502, C1203, and C0701, with a gene frequency of >1% for each allotype and a total gene frequency of >90% in Northeast Asia) were predicted using five advanced epitope prediction tools, following a series of epitope screening principles as previously described (16). Based on the length of each protein and the gene frequency of each HLA-B or HLA-C allotype, 272 HLA-B restricted and 407 HLA-C restricted epitopes as predicted were finally selected as candidate epitopes, of which 171 HLA-B restricted epitopes and 316 HLA-C restricted epitopes were cross-restricted by several HLA-B allotypes and HLA-C allotypes, respectively. Since the cross-restricted candidate epitopes do not need to be synthesized repeatedly, only 101 HLA-B restricted epitopes and 91 HLA-C restricted epitopes were synthesized as peptides for further validation. Among these, 32, 4, 17, 22, and 26 HLA-B restricted epitopes (Supplementary Figure 1A, Supplementary Table S1) and 29, 5, 16, 18, and 23 HLA-C restricted epitopes (Supplementary Figure 1B, Supplementary Table S2) harbor in S, E, M, N, and RdRp proteins, respectively.
Totally, 67 HLA-B restricted and 53 HLA-C restricted epitopes were validated as immunogenic epitopes by cellular functional experiments
To define the immunogenicity of these candidate epitopes, peptide-PBMCs ex vivo coculture experiment followed by intracellular IFN-γ staining was used to detect whether the PBMCs from convalescent individuals contained the epitope-specific memory CD8+ T cells. When the frequency of IFN-γ+/CD8+ T cells in the coculture well was twofold greater than those in the negative control well (PBMCs alone), the peptide cocultured with PBMCs was defined as real-world immunogenic epitope, implying the activation of peptide-specific memory CD8+ T-cell clones in convalescent peripheral blood.
Totally, each candidate epitope was cocultured with the PBMCs from 29 to 32 convalescents. For 101 HLA-B restricted candidate epitopes and 91 HLA-C restricted candidate epitopes, 32 and 30 convalescents’ PBMCs displayed positive CD8+ T-cell responses in the coculture experiments, respectively. Finally, 67 HLA-B restricted candidate epitopes and 53 HLA-C restricted candidate epitopes were validated as immunogenic epitopes, and each validated epitope (VEP) elicited CD8+ T-cell activation in one, two, or more convalescents’ samples (Tables 2, 3). The fold changes in IFN-γ+/CD8+ T-cell frequency in each peptide-PBMCs coculture comparing to its negative control well (PBMCs alone) are presented in Figure 1. The positive rate of each VEP inducing CD8+ T-cell activation in 29–32 random convalescents’ PBMCs is displayed in Figure 2. Most of the in-house validated CD8+ T-cell epitopes have a high herd predominance rate in Chinese cohort (3%–36.7%). Of the 67 HLA-B restricted VEPs, 26, 36, and 5 presented 20%–36.7%, 10%–19%, and <10% of herd positive rate, respectively. Of the 53 HLA-C restricted VEPs, 15, 27, and 11 presented 20%–27%, 10%–19%, and <10% of herd positive rate, respectively. In addition, the numbers of HLA-B restricted VEPs derived from S, E, M, N, and RdRp were 21, 3, 10, 16, and 17, respectively, while 19, 4, 11, 9, and 10 for HLA-C restricted VEPs (Figure 2). The flow cytometric dot plots of intracellular IFN-γ staining in representative peptide-PBMCs cocultures are displayed in Figure 3. The IFN-γ intracellular staining data for all PBMCs samples are presented in Supplementary Figures S2, S3.
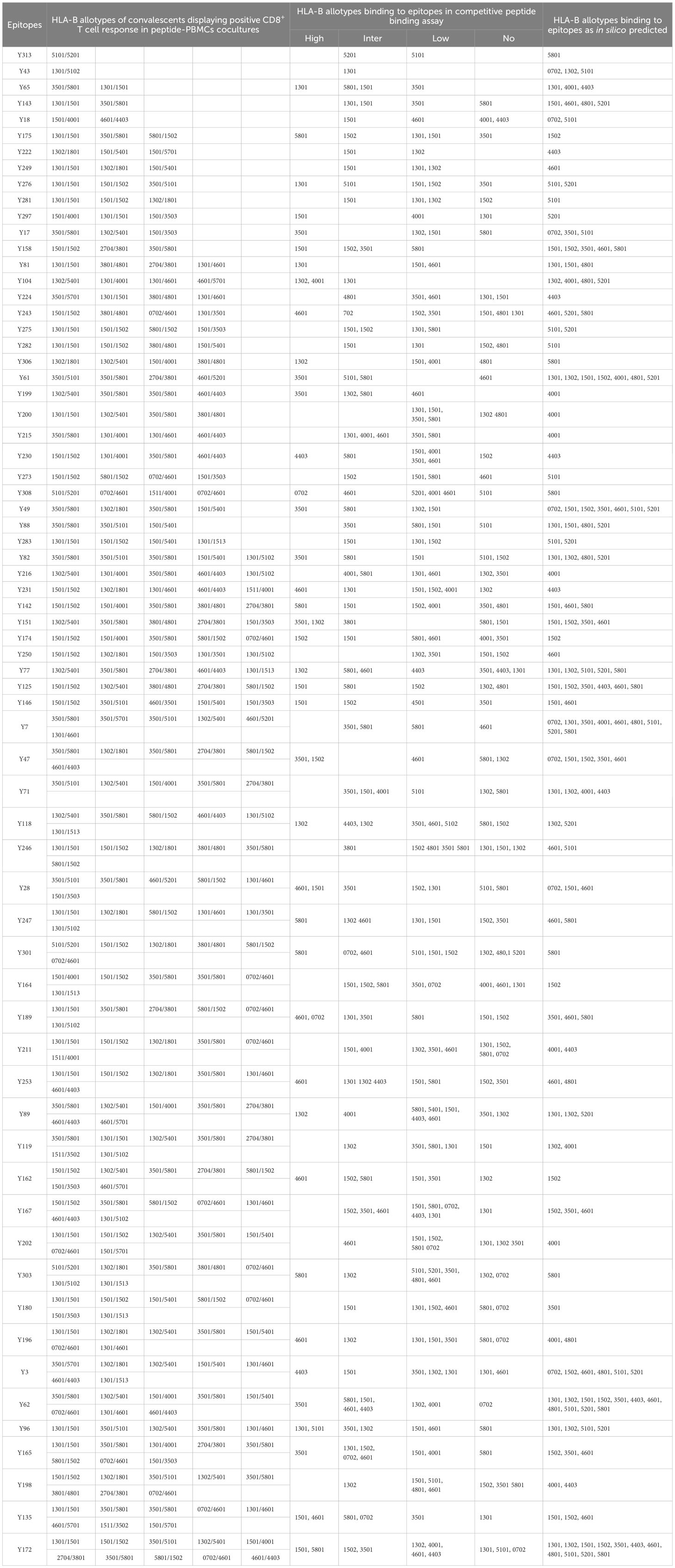
Table 2. HLA-B cross-restriction of 67 CD8+ T-cell epitopes validated by peptide-PBMCs coculture experiments.
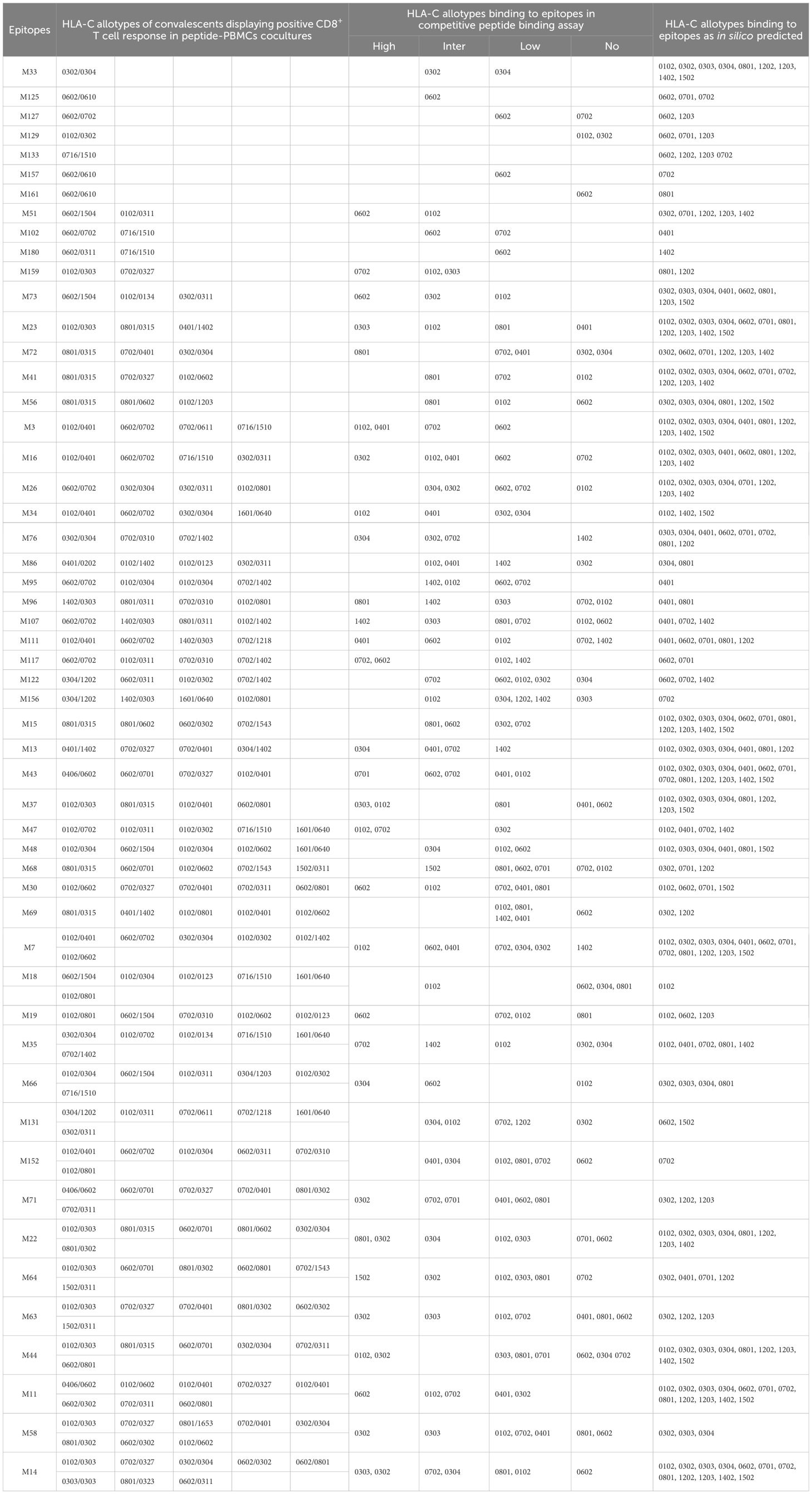
Table 3. HLA-C cross-restriction of 53 CD8+ T-cell epitopes validated by peptide-PBMCs coculture experiments.
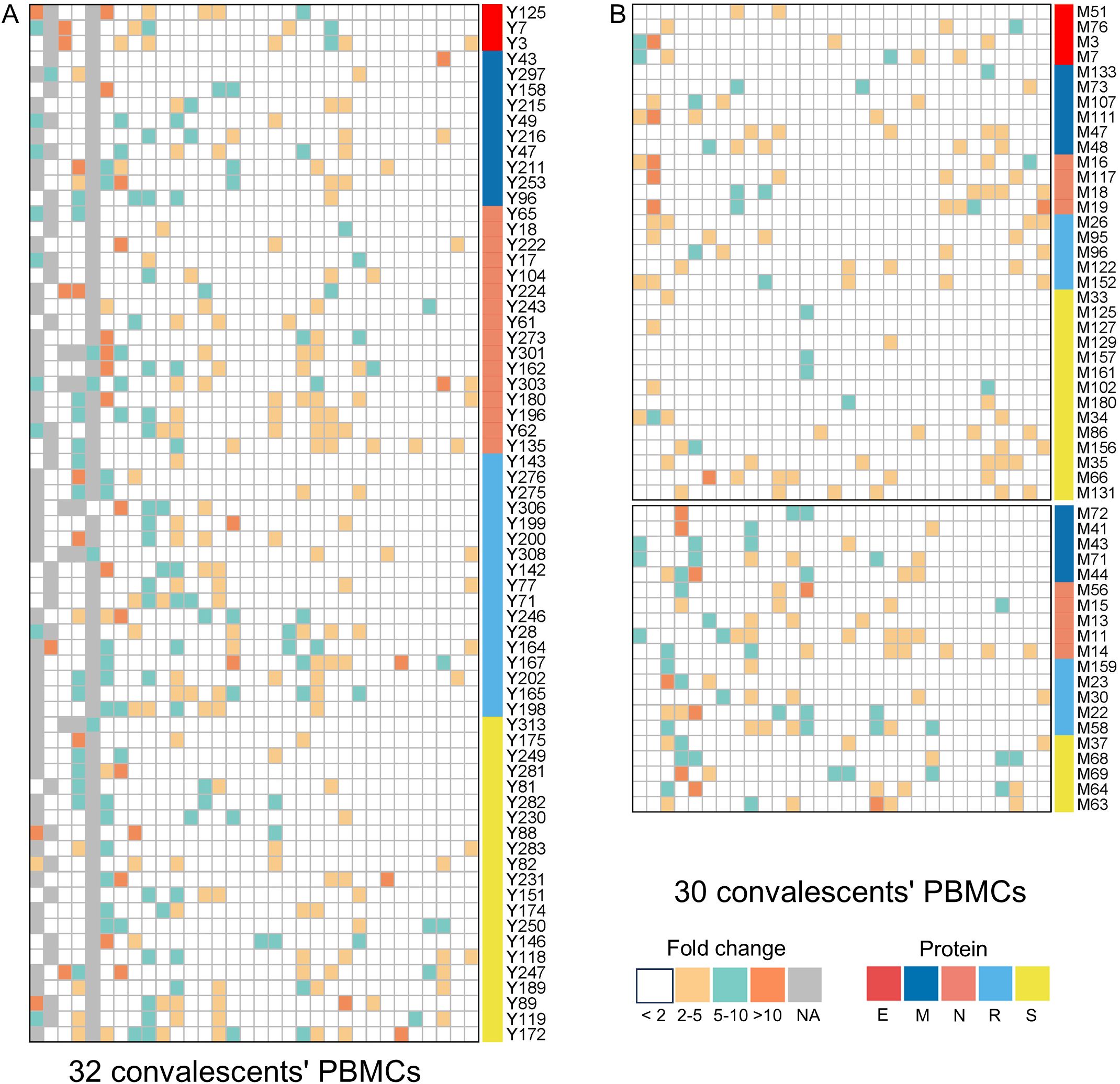
Figure 1. The epitopes inducing CD8+ T-cell activation in peptides-PBMCs ex vivo cocultures. The heatmap illustrates the results of peptide-PBMCs coculture experiments. Each column represents a convalescent PBMCs sample, and each row represents an indicated epitope peptide. Each color element within the heatmap denote the fold changes of IFN-γ+/CD8+ T-cell frequency in the peptide-PBMCs coculture relative to its negative control well (PBMCs alone) (white: < 2; yellow: 2–5; cyan: 5–10; orange: >10). Elements in gray indicate no coculture experiment. (A) The cocultures of HLA-B restricted epitopes with convalescents’ PBMCs samples. (B) The cocultures of HLA-C restricted epitopes with convalescents’ PBMCs samples.
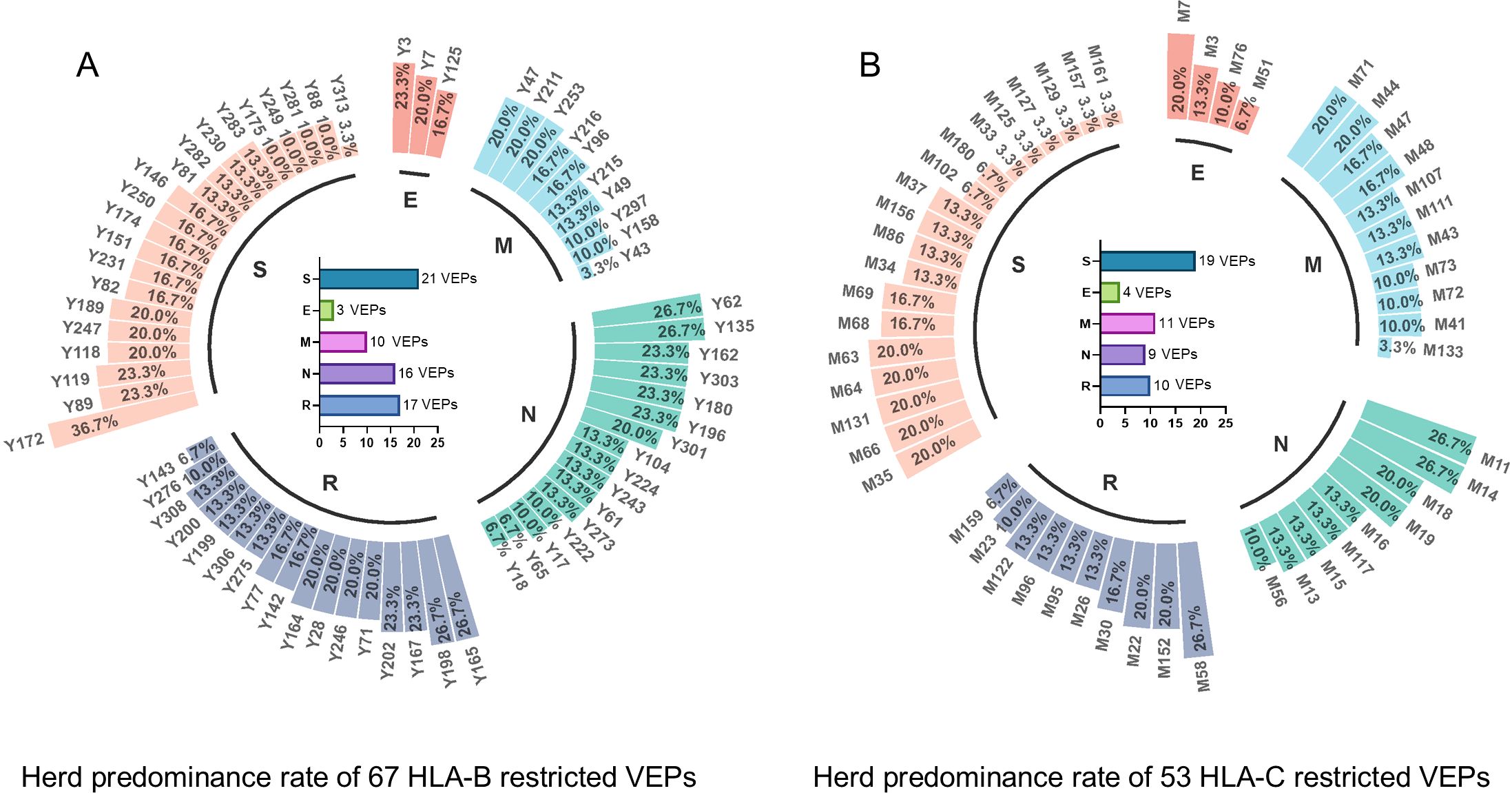
Figure 2. Herd predominance rate of 67 HLA-B restricted VEPs and 53 HLA-C restricted VEPs. Circular histogram displayed the positive rate of each VEP inducing CD8+ T-cell activation in the cocultures with 29–32 random convalescents’ PBMCs. (A) positive rate of each HLA-B restricted VEP as tested using 32 convalescent samples. (B) Positive rate of each HLA-C restricted VEPs as tested using 30 convalescent samples. Internal histogram exhibited the numbers of VEPs derived from different proteins.
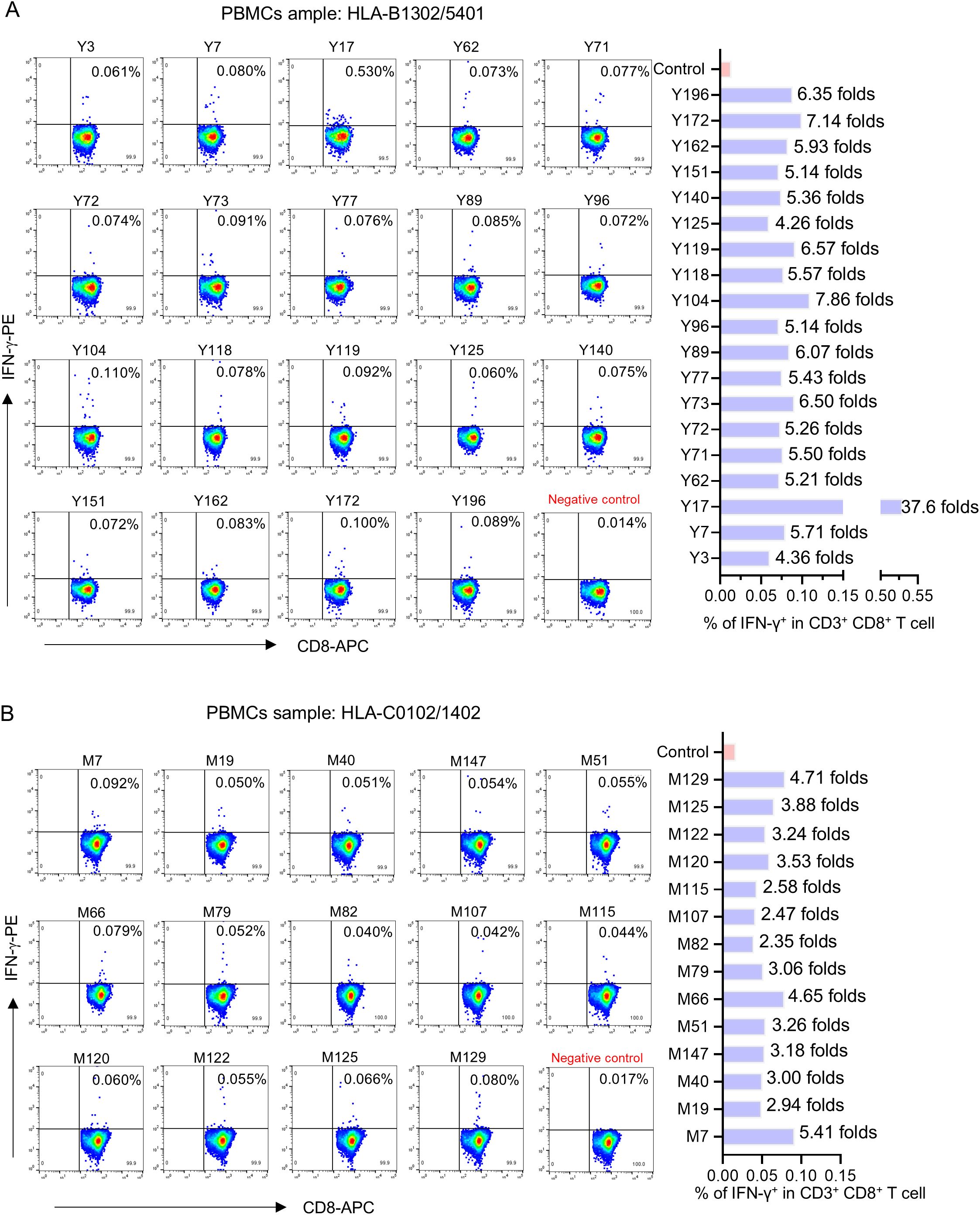
Figure 3. The flow cytometric dot plots of intracellular IFN-γ staining in representative peptide-PBMCs cocultures. After ex vivo coculture of PBMCs with candidate epitope peptide, the cells were harvested and followed by intracellular IFN-γ staining using FITC-conjugated anti-human CD3, APC-conjugated anti-human CD8, and PE-conjugated anti-human IFN-γ antibodies. After washing, the cells were harvested and analyzed by flow cytometry to determine the frequencies of IFN-γ+ cells in CD3+/CD8+ populations. Negative control means PBMCs alone well. (A, B) Representative flow cytometry plots (left) and the histogram showed the frequency of IFN-γ+/CD3+/CD8+ T cell elicited by each epitope (right).
Binding affinity and cross-binding of validated epitopes with corresponding HLA-B and C allotypes were analyzed using competitive peptide binding assay
To evaluate the affinity between VEPs and HLA-B or HLA-C allotypes, 26 transfected HMy2.CIR cell lines stably expressing the indicated HLA-B or C allotype were successfully constructed and identified, including HLA-B4601, B4001, B5801, B1502, B5101, B1301, B1302, B1501, B3501, B4403, B5201, B4801, B0702; HLA-C0102, C0602, C0702, C0801, C0304, C0302, C0303, C0401, C1402, C1202, C1502, C1203, and C0701. HMy2.CIR is a human B lymphocyte strain with HLA class I antigen deficiency, which does not express HLA-A and HLA-B molecules and only expresses trace HLA-Cw4. The CIR cells transduced with exogenous HLA-B or HLA-C gene displayed GFP fluorescence under a fluorescent microscope (Supplementary Figure S4) and exhibited a significantly higher fluorescence intensity compared to those transduced with empty vector, after being stained with APC-conjugated anti-HLA-A/B/C antibodies (Supplementary Figure S5).
Then, competitive peptide binding assays were performed. Briefly, each HMy2.CIR cell line expressing indicated HLA-B or HLA-C molecule was cocultured with the corresponding Cy5-labeled reference peptide and the no-labeled competitor VEP peptide and followed by flow cytometry analysis. The flow cytometric line diagrams showed that most of the tested VEP peptides could efficiently compete with the Cy5-labeled reference peptide and bind to the HLA-B or HLA-C molecules onto the relative CIR cell lines, leading to leftward shift of the fluorescence peak compared to the max control well (CIR cells and Cy5-reference peptide) (Figure 4). Supplementary Tables S3, S4 exhibited the binding affinity of each HLA-B and HLA-C allotype with corresponding VEPs, respectively. Most of the HLA-B and HLA-C allotypes could crossly bind to 3–24 VEPs with high or intermediate affinity, such as HLA-B1501 and HLA-C0102 efficiently bound to 24 and 17 VEPs, respectively. On the other hand, most of VEPs showed cross-binding to several HLA-B or HLA-C molecules with high- or inter-affinity. The HLA restriction of each VEP is further summarized in Tables 2, 3 by combining the data of cellular functional assay, competitive peptide binding assay, and in silico prediction. These data suggest that most of the in-house validated CD8+ T-cell epitopes have a large coverage in China and Northeast Asia. Supplementary Figures S6, S7 displayed the flow cytometric histograms of each VEP binding to multiple HLA-B or HLA-C allotypes in competitive peptide binding assays.
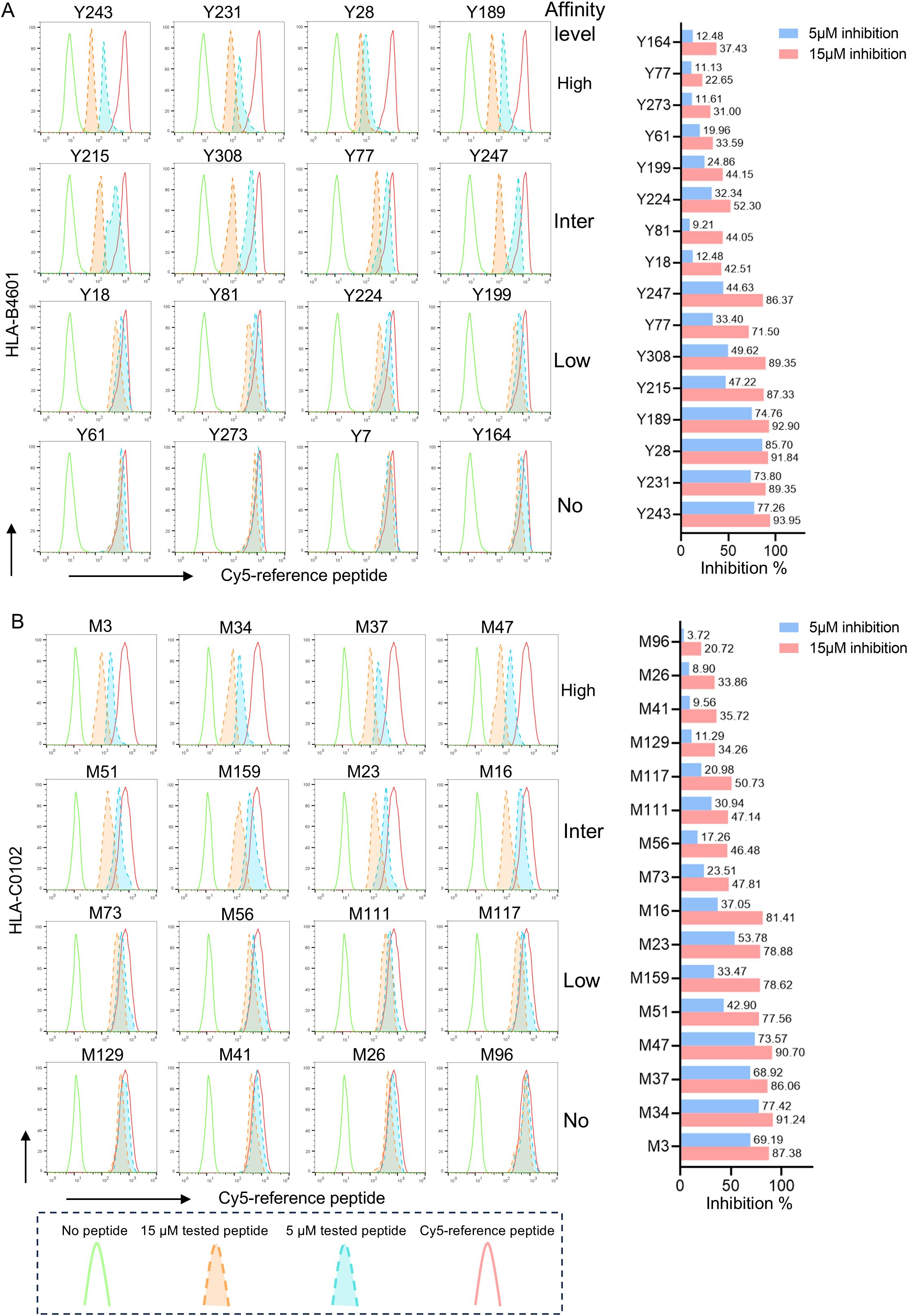
Figure 4. The representative flow cytometric histograms of VEPs binding to HLA-B and HLA-C allotypes in competitive peptide binding assays. The HMy2.CIR cells constantly expressing indicated HLA-B or HLA-C molecule were incubated with Cy5-labeled reference peptide and the no-labeled epitope peptide. After a defined incubation period, the unbound peptides were removed, and the relative binding affinity of the tested epitope peptide to the HLA-B or HLA-C molecule was quantified by the declined MFI of reference peptide binding to the CIR cell surface at different concentration of tested epitope peptide (5 and 15μm). In parallel, the max control well (CIR cells and Cy5-reference peptide; red solid-line peak in flow cytometry histograms) and background well (CIR cells alone; green solid-line peak in flow cytometry histograms) were performed. (A) Representative flow cytometry plots reflecting the affinity between epitopes and HLA-B4601 (left), the histogram showed the inhibition rates of each epitope at 5 and 10 μM concentrations against the Cy5-reference peptide (right) when incubated with CIR-B4601; (B) representative flow cytometry plots reflecting the affinity between epitopes and HLA-C0102 (left), the histogram showed the inhibition rates of each epitope at 5 and 10 μM concentrations against the Cy5-reference peptide (right) when incubated with CIR-C0102.
Discussion
Humoral immunity against SARS-CoV-2 wanes quickly and thus cannot maintain long-term antiviral immunity (3–5) and exhibits weak resistance to viral variants (9, 10). Conversely, T cells against SARS-CoV-2 showed powerful effects and long-lasting memory immunity for defending against infection (21, 22). More importantly, the T-cell epitopes possess a much larger repertoire and much higher conservatism as compared with the B-cell epitopes, implying stronger resistance to viral variants. Therefore, a lot of attention has changed to the multipeptide vaccines based on T-cell epitopes, such as CoVepiT (NCT04885361), EpiVacCorona (NCT04527575) (23), IMP CoVac-1 (containing six T-cell epitopes) (24), PepGNP-SARSCoV2 (NCT04935801, containing nine T-cell epitopes) (25), UB-612 (containing five T-cell epitopes and RBD-Fc) (26), and BNT162B4 (mRNA vaccine based on T-cell epitopes) (27). To design an effective multipeptide vaccine tailored to an indicated geographic population, the T-cell epitopes should be selected according to the following criteria: 1) broad spectrum, the epitopes can bind to various prevalent HLA allotypes with a large coverage of the indicated population; 2) high herd predominance, the epitope has a high positive rate to induce T-cell response in the indicated population; 3) conservation, the epitopes are located in the conserved protein regions and no substitution of anchor amino acids in viral evolutionary history; and 4) high affinity and strong immunogenicity, the epitope can strongly bind to HLA molecule to maintain the peptide–MHC complexes onto T-cell surface for extended duration and robust T-cell response.
Currently, numerous studies have reported at least 1,000 functionally validated CD8+ T-cell epitopes, but most ones are mainly restricted by HLA-A allotypes, particularly the common allotypes such as HLA-A2 or A24 (28). Nevertheless, HLA-B and HLA-C allotypes, as classic HLA class I molecules, can also present antigenic peptides to induce robust T-cell responses, so HLA-B and HLA-C restricted epitopes can serve as important supplements of peptide cocktail used in multipeptide vaccines, especially for the individuals carrying rare HLA-A alleles. For example, if the individual possesses rare HLA-A allotype but dominant HLA-B or C allotypes, the HLA-B or HLA-C restricted epitopes also can elicit robust T-cell immunity to defend against viral infection. This strategy, utilizing the cocktail of HLA-A, HLA-B, and HLA-C restricted T-cell epitopes in vaccine, enables a minimal number of peptides to cover a broader population. Moreover, several studies have indicated that certain HLA-B or HLA-C allotype carriers are more susceptible to SARS-CoV-2 infection and exhibited higher rates of severe disease and mortality (29–33). These findings underscore the necessity of considering the HLA-B and HLA-C genotypes of vulnerable populations when designing vaccines.
Different from the screening of T-cell epitopes from other researchers, this study aims to provide a pool of broad spectrum and herd predominant CD8+ T-cell epitopes, which are derived from various SARS-CoV-2 proteins, tailored to the polymorphism of HLA-B and HLA-C allotypes in Northeast Asia, and has a high herd predominance rate in Chinese cohort. More importantly, the epitopes were validated by using convalescents’ fresh PBMCs and peptide-PBMCs ex vivo cocultures, which are cellular functional experiments to determine the epitope-specific memory CD8+ T-cell clones in convalescent peripheral blood. All enrolled convalescents had recovered from SARS-CoV-2 infection at least 2 years prior, which ensured that the T-cell responses elicited by epitopes were from memory T cells. In addition, the HLA restriction of T-cell epitope is difficult to determine because there is no standard method for now. To solve this issue, this study constructed a series of transfected HyM2.CIR cell lines, which constantly express indicated HLA-B or HLA-C molecules, and followed by an abundance of competitive peptide binding assay, and finally defined the affinities and cross-restriction of each validated epitope with associated HLA-B or HLA-C allotypes depending on the relatively objective data.
In summary, this study provided a verified repertoire of T-cell epitopes, which derived from SARS-CoV-2 main proteins and restricted by predominant HLA-B and HLA-C allotypes covering most of China and Northeast Asia population. These data will facilitate the design and development of SARS-CoV-2 peptide vaccines and specific T-cell detection systems for Northeast Asia. The herd test of functionally validated T-cell epitopes and the competitive peptide binding assay onto cell line array expressing prevalent HLA allotypes may serve as an additional criterion for selecting T-cell epitopes used in vaccine.
Data availability statement
The original contributions presented in the study are included in the article/Supplementary Material. Further inquiries can be directed to the corresponding authors.
Ethics statement
The studies involving humans were approved by Clinical Ethics Committee of Nanjing Red Cross Blood Center. The studies were conducted in accordance with the local legislation and institutional requirements. Written informed consent for participation in this study was provided by the participants’ legal guardians/next of kin. The manuscript presents research on animals that do not require ethical approval for their study.
Author contributions
YZ: Writing – original draft, Conceptualization, Data curation, Investigation, Methodology. MP: Investigation, Methodology, Writing – original draft. CH: Resources, Writing – original draft. ML: Validation, Writing – original draft. XH: Data curation, Writing – original draft. QF: Resources, Writing – original draft. YW: Investigation, Methodology, Writing – original draft. FY: Investigation, Methodology, Writing – original draft. CY: Conceptualization, Validation, Writing – original draft. GZ: Conceptualization, Supervision, Writing – review & editing. CS: Writing – review & editing, Funding acquisition.
Funding
The author(s) declare that financial support was received for the research and/or publication of this article. This work was funded by the National Key Research and Development Program of China (2022YFC2304103). The sponsors had no role in study design, data collection and analysis, preparation of the manuscript, or decision to submit the article for publication.
Conflict of interest
The authors declare that the research was conducted in the absence of any commercial or financial relationships that could be construed as a potential conflict of interest.
Generative AI statement
The author(s) declare that no Generative AI was used in the creation of this manuscript.
Publisher’s note
All claims expressed in this article are solely those of the authors and do not necessarily represent those of their affiliated organizations, or those of the publisher, the editors and the reviewers. Any product that may be evaluated in this article, or claim that may be made by its manufacturer, is not guaranteed or endorsed by the publisher.
Supplementary material
The Supplementary Material for this article can be found online at: https://www.frontiersin.org/articles/10.3389/fimmu.2025.1545510/full#supplementary-material
Abbreviations
COVID-19, coronavirus disease 2019; SARS-CoV-2, severe acute respiratory syndrome coronavirus-2; RBD, receptor-binding domain; HLA, human leukocyte antigens; PBMCs, peripheral blood mononuclear cells; RdRp, RNA-dependent RNA polymerase; MFI, mean fluorescence intensity; PBLs, peripheral blood lymphocyte; ICS, intracellular cytokine staining; VEPs, validated epitope peptides; PHA, phytohemagglutinin.
References
1. Sette A and Crotty S. Adaptive immunity to SARS-coV-2 and COVID-19. Cell. (2021) 184:861–80. doi: 10.1016/j.cell.2021.01.007
2. Sette A, Sidney J, and Crotty S. T cell responses to SARS-coV-2. Annu Rev Immunol. (2023) 41:343–73. doi: 10.1146/annurev-immunol-101721-061120
3. Patel MM, Thornburg NJ, Stubblefield WB, Talbot HK, Coughlin MM, Feldstein LR, et al. Change in antibodies to SARS-coV-2 over 60 days among health care personnel in nashville, tennessee. JAMA. (2020) 324:1781–2. doi: 10.1001/jama.2020.18796
4. Guo L, Wang G, Wang Y, Zhang Q, Ren L, Gu X, et al. SARS-CoV-2-specific antibody and T-cell responses 1 year after infection in people recovered from COVID-19: a longitudinal cohort study. Lancet Microbe. (2022) 3:e348–e56. doi: 10.1016/S2666-5247(22)00036-2
5. Naaber P, Tserel L, Kangro K, Sepp E, Jurjenson V, Adamson A, et al. Dynamics of antibody response to BNT162b2 vaccine after six months: a longitudinal prospective study. Lancet Reg Health Eur. (2021) 10:100208. doi: 10.1016/j.lanepe.2021.100208
6. Hachmann NP, Miller J, Collier AY, Ventura JD, Yu J, Rowe M, et al. Neutralization escape by SARS-coV-2 omicron subvariants BA.2.12.1, BA.4, and BA.5. N Engl J Med. (2022) 387:86–8. doi: 10.1056/NEJMc2206576
7. Qu P, Faraone J, Evans JP, Zou X, Zheng YM, Carlin C, et al. Neutralization of the SARS-coV-2 omicron BA.4/5 and BA.2.12.1 subvariants. N Engl J Med. (2022) 386:2526–8. doi: 10.1056/NEJMc2206725
8. Altarawneh HN, Chemaitelly H, Ayoub HH, Hasan MR, Coyle P, Yassine HM, et al. Protective effect of previous SARS-coV-2 infection against omicron BA.4 and BA.5 subvariants. N Engl J Med. (2022) 387:1620–2. doi: 10.1056/NEJMc2209306
9. Sanyaolu A, Marinkovic A, Prakash S, Haider N, Williams M, Okorie C, et al. SARS-CoV-2 Omicron variant (B.1.1.529): A concern with immune escape. World J Virol. (2022) 11:137–43. doi: 10.5501/wjv.v11.i3.137
10. Tulimilli SV, Dallavalasa S, Basavaraju CG, Kumar Rao V, Chikkahonnaiah P, Madhunapantula SV, et al. Variants of severe acute respiratory syndrome coronavirus 2 (SARS-coV-2) and vaccine effectiveness. Vaccines (Basel). (2022) 10(10):1751. doi: 10.3390/vaccines10101751
11. Sadarangani M, Marchant A, and Kollmann TR. Immunological mechanisms of vaccine-induced protection against COVID-19 in humans. Nat Rev Immunol. (2021) 21:475–84. doi: 10.1038/s41577-021-00578-z
12. Abdella WS, Selim AN, Diab S, Qobeia E, and Elgazzar AF. Vaccination and therapeutics. Adv Exp Med Biol. (2024) 1457:165–84. doi: 10.1007/978-3-031-61939-7_9
13. Zhang Z, Mateus J, Coelho CH, Dan JM, Moderbacher CR, Galvez RI, et al. Humoral and cellular immune memory to four COVID-19 vaccines. Cell. (2022) 185:2434–51 e17. doi: 10.1016/j.cell.2022.05.022
14. Hielscher F, Schmidt T, Klemis V, Wilhelm A, Marx S, Abu-Omar A, et al. NVX-CoV2373-induced cellular and humoral immunity towards parental SARS-CoV-2 and VOCs compared to BNT162b2 and mRNA-1273-regimens. J Clin Virol. (2022) 157:105321. doi: 10.1016/j.jcv.2022.105321
15. Tan CY, Chiew CJ, Lee VJ, Ong B, Lye DC, and Tan KB. Comparative effectiveness of 3 or 4 doses of mRNA and inactivated whole-virus vaccines against COVID-19 infection, hospitalization and severe outcomes among elderly in Singapore. Lancet Reg Health West Pac. (2022) 29:100654. doi: 10.1016/j.lanwpc.2022.100654
16. Jin X, Ding Y, Sun S, Wang X, Zhou Z, Liu X, et al. Screening HLA-A-restricted T cell epitopes of SARS-CoV-2 and the induction of CD8(+) T cell responses in HLA-A transgenic mice. Cell Mol Immunol. (2021) 18:2588–608. doi: 10.1038/s41423-021-00784-8
17. Gray CM, Mlotshwa M, Riou C, Mathebula T, de Assis Rosa D, Mashishi T, et al. Human immunodeficiency virus-specific gamma interferon enzyme-linked immunospot assay responses targeting specific regions of the proteome during primary subtype C infection are poor predictors of the course of viremia and set point. J Virol. (2009) 83:470–8. doi: 10.1128/JVI.01678-08
18. Pattekar A, Mayer LS, Lau CW, Liu C, Palko O, Bewtra M, et al. Norovirus-specific CD8(+) T cell responses in human blood and tissues. Cell Mol Gastroenterol Hepatol. (2021) 11:1267–89. doi: 10.1016/j.jcmgh.2020.12.012
19. Dudaniec K, Westendorf K, Nossner E, and Uckert W. Generation of epstein-barr virus antigen-specific T cell receptors recognizing immunodominant epitopes of LMP1, LMP2A, and EBNA3C for immunotherapy. Hum Gene Ther. (2021) 32:919–35. doi: 10.1089/hum.2020.283
20. Martin SD, Wick DA, Nielsen JS, Little N, Holt RA, and Nelson BH. A library-based screening method identifies neoantigen-reactive T cells in peripheral blood prior to relapse of ovarian cancer. Oncoimmunology. (2017) 7:e1371895. doi: 10.1080/2162402X.2017.1371895
21. Tan CW, Chia WN, Young BE, Zhu F, Lim BL, Sia WR, et al. Pan-sarbecovirus neutralizing antibodies in BNT162b2-immunized SARS-coV-1 survivors. N Engl J Med. (2021) 385:1401–6. doi: 10.1056/NEJMoa2108453
22. Le Bert N, Tan AT, Kunasegaran K, Tham CYL, Hafezi M, Chia A, et al. SARS-CoV-2-specific T cell immunity in cases of COVID-19 and SARS, and uninfected controls. Nature. (2020) 584:457–62. doi: 10.1038/s41586-020-2550-z
23. Ryzhikov AB, Ryzhikov EA, Bogryantseva MP, Usova SV, Nechaeva EA, Danilenko ED, et al. Assessment of safety and prophylactic efficacy of the epiVacCorona peptide vaccine for COVID-19 prevention (Phase III). Vaccines (Basel). (2023) 11(5):998. doi: 10.3390/vaccines11050998
24. Heitmann JS, Bilich T, Tandler C, Nelde A, Maringer Y, Marconato M, et al. A COVID-19 peptide vaccine for the induction of SARS-CoV-2 T cell immunity. Nature. (2022) 601:617–22. doi: 10.1038/s41586-021-04232-5
25. Miauton A, Audran R, Besson J, Maby-El Hajjami H, Karlen M, Warpelin-Decrausaz L, et al. Safety and immunogenicity of a synthetic nanoparticle-based, T cell priming peptide vaccine against dengue in healthy adults in Switzerland: a double-blind, randomized, vehicle-controlled, phase 1 study. EBioMedicine. (2024) 99:104922. doi: 10.1016/j.ebiom.2023.104922
26. Wang CY, Hwang KP, Kuo HK, Peng WJ, Shen YH, Kuo BS, et al. A multitope SARS-CoV-2 vaccine provides long-lasting B cell and T cell immunity against Delta and Omicron variants. J Clin Invest. (2022) 132(10):e157707. doi: 10.1172/JCI157707
27. Arieta CM, Xie YJ, Rothenberg DA, Diao H, Harjanto D, Meda S, et al. The T-cell-directed vaccine BNT162b4 encoding conserved non-spike antigens protects animals from severe SARS-CoV-2 infection. Cell. (2023) 186:2392–409 e21. doi: 10.1016/j.cell.2023.04.007
28. Jin X, Liu X, and Shen C. A systemic review of T-cell epitopes defined from the proteome of SARS-CoV-2. Virus Res. (2023) 324:199024. doi: 10.1016/j.virusres.2022.199024
29. Weiner J, Suwalski P, Holtgrewe M, Rakitko A, Thibeault C, Muller M, et al. Increased risk of severe clinical course of COVID-19 in carriers of HLA-C*04:01. EClinicalMedicine. (2021) 40:101099. doi: 10.1016/j.eclinm.2021.101099
30. Naidoo L, Arumugam T, and Ramsuran V. HLA-B and C expression contributes to COVID-19 disease severity within a South African cohort. Genes (Basel). (2024) 15(4):522. doi: 10.3390/genes15040522
31. Naemi FMA, Al-Adwani S, Al-Khatabi H, and Al-Nazawi A. Association between the HLA genotype and the severity of COVID-19 infection among South Asians. J Med Virol. (2021) 93:4430–7. doi: 10.1002/jmv.27003
32. Hovhannisyan A, Madelian V, Avagyan S, Nazaretyan M, Hyussyan A, Sirunyan A, et al. HLA-C*04:01 affects HLA class I heterozygosity and predicted affinity to SARS-coV-2 peptides, and in combination with age and sex of Armenian patients contributes to COVID-19 severity. Front Immunol. (2022) 13:769900. doi: 10.3389/fimmu.2022.769900
Keywords: SARS-CoV-2, HLA-B allotype, HLA-C allotype, T-cell epitope, vaccine
Citation: Zhao Y, Peng M, He C, Li M, Han X, Fu Q, Wu Y, Yue F, Yan C, Zhao G and Shen C (2025) Predominant T-cell epitopes of SARS-CoV-2 restricted by multiple prevalent HLA-B and HLA-C allotypes in Northeast Asia. Front. Immunol. 16:1545510. doi: 10.3389/fimmu.2025.1545510
Received: 15 December 2024; Accepted: 25 April 2025;
Published: 21 May 2025.
Edited by:
Diego Cantoni, MRC-University of Glasgow Centre for Virus Research (MRC), United KingdomReviewed by:
Jasdeep Singh, University of Denver, United StatesSantrupti Nerli, Genentech, United States
Chanchan Xiao, Jinan University, China
Copyright © 2025 Zhao, Peng, He, Li, Han, Fu, Wu, Yue, Yan, Zhao and Shen. This is an open-access article distributed under the terms of the Creative Commons Attribution License (CC BY). The use, distribution or reproduction in other forums is permitted, provided the original author(s) and the copyright owner(s) are credited and that the original publication in this journal is cited, in accordance with accepted academic practice. No use, distribution or reproduction is permitted which does not comply with these terms.
*Correspondence: Guangyu Zhao, Z3Vhbmd5dTA1MjVAMTYzLmNvbQ==; Chuanlai Shen, Y2h1YW5sYWlzaGVuQHNldS5lZHUuY24=
†These authors have contributed equally to this work
‡ORCID: Guangyu Zhao, orcid.org/0000-0002-0925-5216
Chuanlai Shen, orcid.org/0000-0002-3748-3742