- Center for Vaccine Development and Global Health, University of Maryland School of Medicine, Baltimore, MD, United States
Adjuvants are crucial in vaccines and cancer therapies, enhancing therapeutic efficacy through diverse mechanisms. In vaccines, adjuvants are traditionally valued for amplifying immune responses, ensuring robust and long-lasting protection against pathogens. In cancer treatments, adjuvants can boost the effectiveness of chemotherapy or immunotherapy by targeting tumor antigens, rendering cancer cells more vulnerable to treatment. Recent research has uncovered new molecular-level effects of the adjuvants, mainly through epigenetic mechanisms. Epigenetics encompasses heritable modifications in gene expression that do not alter the DNA sequence, impacting processes such as DNA methylation, histone modification, and non-coding RNA expression. These epigenetic changes play a pivotal role in regulating gene activity, influencing immune pathways, and modulating the strength and duration of immune responses. Whether in vaccines or cancer treatments, understanding how adjuvants interact with epigenetic regulators offers significant potential for developing more precise, cell-targeted therapies across various medical fields. This review delves into the evolving role of adjuvants and their interactions with epigenetic mechanisms. It also examines the potential of harnessing epigenetic changes to enhance adjuvant efficacy and explores the novel use of epigenetic inhibitors as adjuvants in therapeutic settings.
1 Adjuvants in vaccines
Several types of adjuvants are currently employed to enhance vaccine efficacy through diverse mechanisms and approaches. Historically, aluminum salts, such as aluminum hydroxide and aluminum phosphate, were the most widely used adjuvants. These adjuvants boost the immune response by forming depots at the injection site (i.e., depot effect), thereby prolonging antigen exposure. Additionally, they activate the innate immune system by triggering the NOD-like receptor protein 3 (NLRP3) inflammasome pathway, resulting in the production of pro-inflammatory cytokines (1). Though, recent research has shifted toward new compounds with adjuvant properties, including nano- and micro-particles (e.g., polymers like poly(lactic-co-glycolic) acid (PLGA) or liposomes), emulsions (e.g., MF59 or AS03), immune potentiators (e.g., cytokines, Toll-like receptors (TLR) agonists), combination adjuvants (e.g., alum with immune potentiators), and mucosal adjuvants to target nasal or oral routes (2). For example, oil-in-water emulsions like MF59 and AS03 enhance local immune activation by promoting antigen uptake by antigen-presenting cells (APCs) and activating the nuclear factor kappa-B (NF-κB) (3). Although traditionally viewed as a pro-inflammatory pathway, recent research has revealed its complexity, suggesting that NF-κB may mediate both pro- and anti-inflammatory responses (4). TLR agonists include a range of molecules such as polyinosinic acid (Poly I) – TLR3 agonist (e.g., Investigational use in vaccines for influenza, HIV, and certain cancers) (5), monophosphoryl Lipid A (MPLA) – TLR4 agonist (e.g., Cervarix (HPV vaccine) (6, 7), and CpG Oligodeoxynucleotides (CpG-ODN) – TLR9 agonist (e.g., Heplisav-B (hepatitis B vaccine) (8, 9). These adjuvants can promote either Th1 immune responses and facilitate the development of CD4+ and CD8+ T cells. Additionally, TLR agonists play a crucial role in modulating Th2 responses by activating B cells and enhancing antibody production against weakly immunogenic antigens (10), thereby improving both the quality and quantity of antigen-specific antibodies.
2 Adjuvants in cancer immunotherapies
Beyond traditional vaccines, adjuvants are becoming increasingly important in cancer vaccines and immunotherapies, where they are used to boost the immune system’s ability to recognize and attack tumor cells. Unlike traditional vaccines, which target pathogens, cancer vaccines and immunotherapies must overcome the immune system’s tolerance to self-antigens and its suppression by the tumor microenvironment (TME) (11). One class of adjuvants utilized in cancer vaccines is the stimulator of interferon genes protein (STING) agonists, a class of molecules that activate the STING pathway, a key mediator of inflammation (12). They promote the recruitment of effector immune cells and enhance the priming of tumor-specific CD8+ T cells (13). For example, cyclic GMP-AMP synthase (cGAS)-STING agonists, which activate the cGAS-STING pathway, are linked to the activation of Interferon Regulatory Factor 3 (IRF3) and NF-κB signaling pathways. These agonists promote the secretion of type I interferons, making them promising adjuvants for developing effective subunit vaccines (14). They also detect self-DNA released from tumors or dying cells (15). Suppression of STING signaling through epigenetic silencing impedes DNA damage (16). While commonly used to enhance immune responses in vaccines, saponin-based adjuvants (SBAs), such as QS-21, are also being investigated for cancer immunotherapy. Derived from the bark of the Quillaja saponaria tree, SBAs form immune-stimulating complexes that boost antigen uptake by APCs, including dendritic cells (DCs) (17). A recent study examining DC subset responses to SBAs found that the CD163+ CD14+ DC subset is the primary responder to this adjuvant in humans (18). Another class of adjuvants explored for cancer vaccines is the non-nucleoside DNA methyltransferase inhibitor (DNMTi) MC3343, a quinoline-based analog with potential adjuvanticity in osteosarcoma therapy (19). MC3343 reactivates a series of regulatory genes linked to osteoblastic differentiation, which are aberrantly silenced in osteosarcoma, thus helping to restore the balance between cell proliferation and differentiation. Furthermore, MC3343 sensitizes tumor cells to chemotherapy by enhancing the efficacy of doxorubicin and cisplatin. It achieves this by inducing chromatin decondensation, which facilitates drug binding to DNA, leading to increased DNA damage and apoptosis (19). These advancements highlight the role of epigenetic regulation in boosting immune responses.
3 Epigenetics
Epigenetics involves inheritable changes in gene expression that occur without alterations to the DNA sequence itself. These modifications can include DNA methylation, histone modification, and non-coding RNA regulation, which collectively influences gene activity and cellular function. Understanding epigenetic mechanisms is crucial for developing better and more effective treatments in the realm of immunotherapies, both for cancer and infectious diseases. DNA methylation was the first epigenetic mechanism recognized. It involves the covalent transfer of a methyl group to the C-5 position of the cytosine ring of DNA by DNA methyltransferases (20). Histones are proteins around which DNA is wrapped, and their chemical modifications can affect how tightly or loosely DNA is packaged. Common histone modifications include acetylation, methylation, phosphorylation, and ubiquitination (21). These modifications can specifically influence the expression of immune-related genes in distinct immune cell subsets (22, 23). In previous studies, mucosal-associated invariant T (MAIT) cells exhibiting distinct cytokine profiles, which were associated with protection against typhoid fever (24), showed infection-induced changes in chromatin marks following Salmonella enterica serovar Typhi (S. Typhi) exposure, with these changes being dependent on specific cell subsets (23). Additionally, cross-talk between intestinal epithelial cells and innate lymphocytes, such as natural killer (NK) cells and MAIT cells, played a crucial role in triggering these chromatin modifications within innate lymphocytes (22). Since previous studies have shown that intestinal epithelial cells can differentially recognize closely related strains (25–27), a fundamental question arises: would these epithelial cells induce the same or distinct sets of chromatin changes in response to closely related strains? Non-coding RNAs, including microRNAs (miRNAs) and long-coding RNAs (lncRNAs), can also modulate immune responses by regulating the expression of key immune genes (28). MiRNAs can bind to messenger RNAs (mRNAs) and inhibit their translation or promote their degradation. lncRNAs can interact with chromatin, transcription factors, or other RNAs to regulate gene expression (29). For instance, specific miRNAs have been shown to regulate the differentiation and function of DC and control many aspects of inflammatory processes (30). Exploration of epigenetics pathways in vaccines and immunotherapies allows researchers to design adjuvants that activate immune cells and modify the epigenetic landscape to restore or improve immune function.
4 How adjuvants trigger epigenetic changes
The signaling pathways activated by adjuvants influence epigenetic changes within immune cells. The elicited modifications lead to alterations in gene expression and immune cell functionality (Figure 1). Recent data highlights adjuvants’ ability to reprogram the innate immune system to give rise to heightened resistance against pathogens by training the innate immune system (31). A recent study explored the use of AS03 and its role in epigenetic changes that increased antiviral defenses. Researchers found that including AS03 in the vaccine prompted chemical changes in innate immune cells, leading to increased expression of antiviral genes and resistance to Zika and dengue viruses (32). In contrast, vaccines administered without AS03 failed to induce these epigenetic changes (32). Specifically, AS03 increased chromatin accessibility at loci associated with Interferon Regulatory Factor (IRF) and Signal Transducer and Activator of Transcription (STAT) in monocytes and DCs (33). This improved accessibility facilitated stronger activation of antiviral pathways, whereas non-adjuvanted vaccines resulted in only temporary epigenetic effects. These insights emphasize the dual role of adjuvants in enhancing immediate immune response and in epigenetic reprogramming by altering chromatin accessibility and transcription factor (TF) dynamics to support long-term adaptive immunity. Clinical trials of adjuvants like AS01 and AS03 have shown their ability to enhance adaptive immune responses by activating the IFN-signaling pathway and engaging innate immune cells, particularly innate-like T cells, which undergo epigenetic modifications post-immunization, a phenomenon known as trained immunity (34). Also, yeast-derived adjuvants such as zymosan and β-glucans can stimulate innate immunity and promote trained immunity (35–37). Histone modifications with chromatin reconfiguration have proven to be a central process for trained immunity (38). Novakovic and colleagues showed that β-glucan affects the H3K27ac and H3K4me3 marks in monocytes by reprogramming gene expression patterns during training and upon re-stimulation (36).
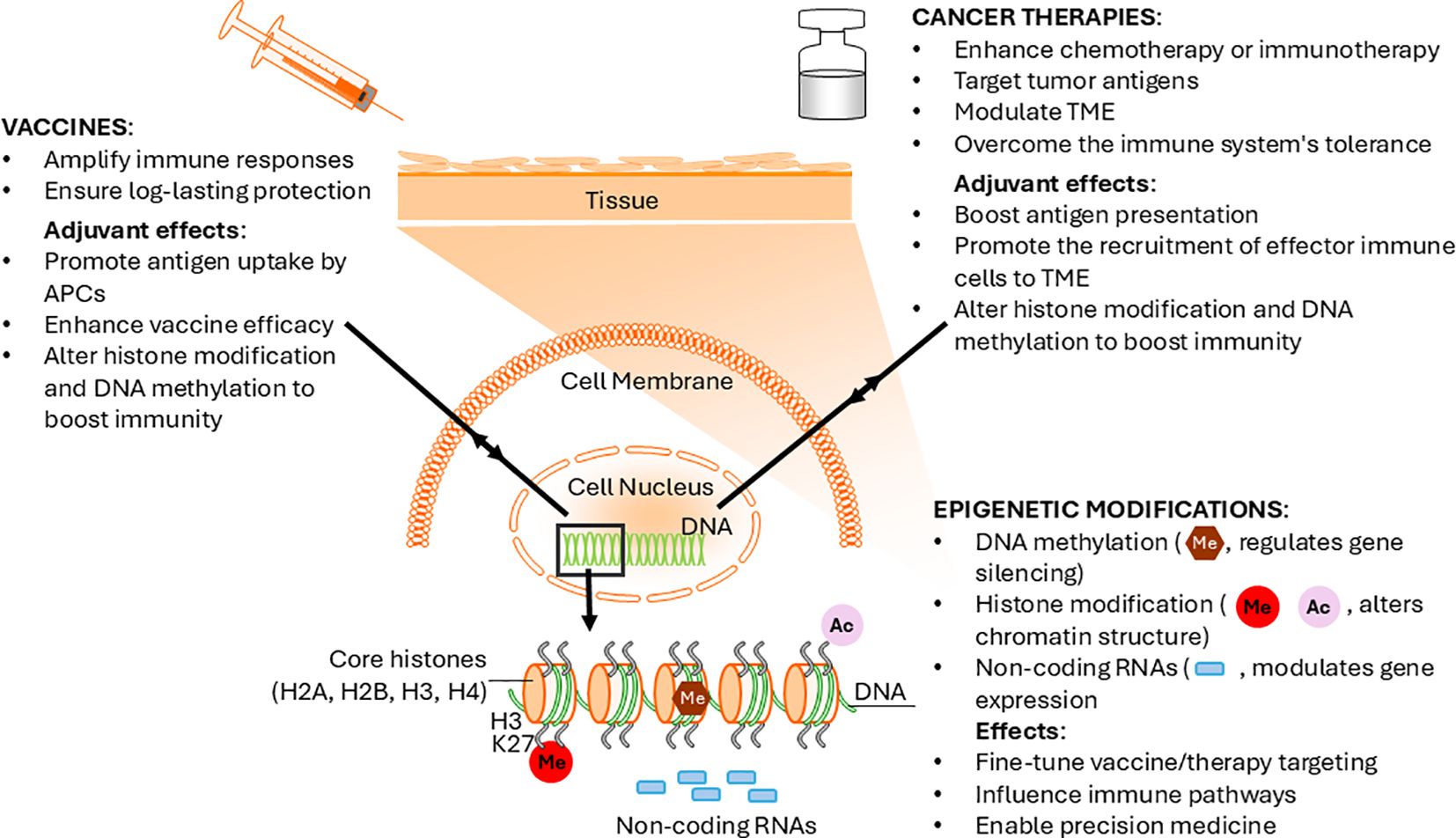
Figure 1. Bidirectional relationship between epigenetic changes and adjuvant potency. Schematic representation of adjuvant-induced epigenetic modulation enhancing immunity in vaccines and cancer therapy.
In cancer immunotherapies incorporating adjuvants, the epigenetic modifications induced by these agents are crucial, as they enhance the immune system’s capacity to overcome its inherent limitations in recognizing and eliminating tumor cells (Figure 1). The interplay between cell types directly involved in tumor lytic activities, such as NK cells, T cells, and macrophages, and the epigenetic regulators plays a crucial role in shaping the effectiveness of cancer vaccines (39, 40). The utilization of adjuvants, such as TLR agonists, induces stable epigenetic imprinting in both normal and cancer cells. There are many TLR adjuvants, each inducing distinct epigenetic changes in immune cells. Stimulation of the TLR3 receptor with Poly I promotes DNA methylation in peripheral blood mononuclear cells (41) and drives the expression of pro-inflammatory cytokines through direct epigenetic regulation at the promoter regions of their gene loci. Additionally, it reactivates silenced miRNAs in tumor cells, thereby enhancing the anti-tumor responses (42). Furthermore, TLR4 ligands can reprogram monocytes by inducing histone modifications that favor the expression of inflammatory genes. These changes have long-lasting effects on monocyte behavior, potentially sustaining an inflammatory response that is beneficial for combating tumor growth (36). While these insights enhance our understanding of the epigenetic changes triggered by adjuvants, significant gaps remain in fully elucidating their precise modes of action, underlying mechanisms, and potential applications in vaccine-based therapies.
5 Epigenetic changes modulating adjuvant potency
The interplay between epigenetic changes and adjuvant potency reveals a bidirectional relationship (Figure 1). On the one hand, adjuvants induce epigenetic modifications to enhance immune responses. On the other hand, these epigenetic changes modulate the adjuvants’ efficacy. Increasingly, the effectiveness of adjuvants is understood to be closely tied to epigenetic memory, a process associated with trained immunity. Tailoring the epigenetic landscape to maximize adjuvant potency offers promising avenues for developing more effective cancer combinatorial therapies. Advancements in genome-editing tools, such as CRISPR/Cas9, present opportunities for enhancing adjuvant design by precisely manipulating epigenetic marks, leading to an optimized immune response (43). A recent study examining the immune suppressive TME identified HIF1α as a key contributor to the suppressive properties of tumor-associated macrophages. Researchers employed the CRISPR/dCas9-EZH2 system to epigenetically silence HIF1α through targeted histone H3 methylation in its promoter region. This led to sustained repression of HIF α, creating a population of macrophages termed HIF α Epigenetically Repressed Macrophages. When injected into a melanoma mouse model, these macrophages reprogrammed the TME, reducing immune suppression and fostering a tumor-suppressing phenotype. This reprogramming resulted in a notable reduction in tumor burden and an extension of overall survival rates in the treated mice (44). By epigenetically reprogramming immune cells, such as macrophages, to adopt a tumor-suppressing phenotype, we can create a more favorable immune microenvironment that synergizes with adjuvant-enhanced therapeutic models to optimize immune response.
Epigenetic changes can also potentially downregulate inhibitory pathways that suppress immune responses to pathogens, thereby enhancing adjuvant potency. For example, during S. Typhi infection, human leukocyte antigen G (HLA-G) expression on infected-target cells significantly contributes to the downregulation of IFN-γ production by MAIT cells (45). This discovery highlights a promising opportunity to epigenetically target the HLA-G pathway to promote robust MAIT cell activation, leading to increased IFN-γ production and a stronger overall immune response. Epigenetic regulation of HLA-G is partly controlled by cis-acting mechanisms (46).
6 Epigenetic inhibitors functioning as adjuvants in immunotherapy
Cancer, a disease driven by the accumulation of genetic and epigenetic modifications (47), is increasingly being targeted through therapeutic strategies that modulate the epigenetic landscape and TME. Cancer’s ability to manipulate epigenetic marks allows it to evade immune surveillance and diminish the effectiveness of immune-based therapies. Addressing these cancer-driven epigenetic pathways offers dual benefits: reversing tumor progression and enhancing the efficacy of immunotherapies (48). In epithelial ovarian cancer cell lines that did not express NY-ESO-1, a highly immunogenic tumor-associated antigen capable of eliciting both humoral and cellular immune responses (49), treatment with the DNA methylation inhibitor decitabine (Dacogen®) (50) restored its expression, which, combined with a protein vaccine and chemotherapy, led to improved immune responses (51). These findings underscore the potential of epigenetic inhibitory reprogramming to enhance antigen presentation and stimulate stronger immune responses, paving the way for novel cancer immunotherapy strategies. Histone deacetylase inhibitors (HDACis) also function as potent adjuvants by modulating histone acetylation, promoting chromatin relaxation, and enhancing the transcription of immune-related genes (52). For instance, the HDACi AR-42 significantly improved the efficacy of a DNA vaccine targeting the human papillomavirus (HPV) protein E7 in a lung cancer model. This combination resulted in heightened CD8+ T-cell responses and enhanced anti-tumor effects compared to the vaccine alone (53). Epigenetic inhibitors also synergize with immune checkpoint inhibitors (ICIs), making them highly valuable tools in combination with immunotherapies. Enhancer of Zeste Homolog 2 (EZH2), a histone methyltransferase, has been implicated in modulating T-cell activity. In melanoma and bladder cancer models, combining EZH2 inhibitors with anti-cytotoxic T-lymphocyte-associated antigen 4 (CTLA-4) therapy demonstrated a direct role for EZH2-mediated T-cell reprogramming in enhancing anti-tumor immunity (54). CTLA-4 is a critical immune checkpoint that negatively regulates T-cell immune function, and its inhibition enhances immune system activation (55). These findings highlight how epigenetic modulation can act as functional adjuvants, enhancing the efficacy of checkpoint blockade therapies and rendering them effective even in previously resistant tumor types. Beyond enhancing antigen presentation and checkpoint blockade, epigenetic inhibitors broadly modulate the TME. The HDAC inhibitor, 2-hexyl-4-pentylene acid (HPTA), demonstrated significant anti-tumor effects in a rat breast cancer model by leading to the increase in CXCL9/10 mRNA expression and protein levels following treatment (56). CXCL9/10 is involved in immune cell migration, differentiation, and activation (57). This study showed that HPTA treatment enhanced the recruitment of CD4+ T cells to tumor tissues, a process mediated by increased CXCL9/10 expression. The accumulation of T cells at the tumor site boosted the immune response, underscoring the potential of epigenetic inhibitors like HPTA to function as adjuvants in cancer therapeutics.
Additionally, epigenetic modulation has been explored to combat bacterial pathogens, enhancing the body’s natural defenses against infection—an increasingly critical need in light of the global antimicrobial resistance crisis. Drugs targeting epigenetic modifiers of bacterial pathogens, such as methyltransferase inhibitors, hold promising therapeutic potential. For example, current research has focused on Legionella pneumophila, the primary causative agent of Legionnaires’ disease, a severe form of acute pneumonia (58). This Gram-negative pathogen utilizes RomA, a SET-domain methyltransferase, to manipulate the host’s epigenetic landscape. RomA facilitates the pathogen’s survival by methylating histone H3 at lysine 14 (H3K14) during infection. To counter this mechanism, researchers developed a high-content imaging screening assay to identify potential RomA inhibitors. These inhibitors offer a novel approach to combating Legionella pneumophila by preventing the pathogen from altering host epigenetics (59). Functioning similarly to traditional adjuvants, these inhibitors indirectly enhance the host’s natural defense mechanisms, presenting a promising avenue for anti-infective therapies. The key question, therefore, is: how can epigenetic adjuvants be developed to mimic the potency of live vaccines in inducing robust T-cell responses in humans, which subunit vaccines have thus far failed to achieve, even with potent adjuvants (33)?
7 Future directions and conclusions
The dynamic interplay between adjuvants and epigenetic mechanisms holds tremendous promise for paving the path for personalized immunotherapies that lead to better patient outcomes. Many research efforts are underway to explore the potential of these novel tools in immunotherapies. However, significant gaps remain in our understanding of how specific adjuvants influence epigenetic modifications, how epigenetic tools can be used to enhance adjuvant potency, and how these changes, in turn, impact immune responses. Addressing these gaps is crucial for harnessing the full potential of adjuvants in clinical settings. Future studies should focus on elucidating the precise mechanisms by which adjuvants induce their epigenetic changes in immune cells, the duration and stability of these modifications, and their implications for immune memory and response efficacy. By advancing our knowledge in these areas, we can optimize the design of adjuvants and develop more effective, tailored immunotherapy strategies that enhance patient outcomes and combat a wide range of diseases.
Author contributions
YM: Writing – original draft, Writing – review & editing. RS: Conceptualization, Funding acquisition, Writing – original draft, Writing – review & editing.
Funding
The author(s) declare financial support was received for the research, authorship, and/or publication of this article. This work was supported, in part, by NIAID, NIH, grant U19-AI181108 (Cooperative Center for Human Immunology (CCHI)) to RSG (Research Project PI).
Acknowledgments
We recognize the generous financial assistance provided by the National Institute of Allergy and Infectious Diseases at the National Institutes of Health (NIH).
Conflict of interest
The authors declare that the research was conducted in the absence of any commercial or financial relationships that could be construed as a potential conflict of interest.
The author(s) declared that they were an editorial board member of Frontiers, at the time of submission. This had no impact on the peer review process and the final decision.
Generative AI statement
The author(s) declare that no Generative AI was used in the creation of this manuscript.
Publisher’s note
All claims expressed in this article are solely those of the authors and do not necessarily represent those of their affiliated organizations, or those of the publisher, the editors and the reviewers. Any product that may be evaluated in this article, or claim that may be made by its manufacturer, is not guaranteed or endorsed by the publisher.
Author disclaimer
The content is solely the authors’ responsibility and does not necessarily represent the official views of the National Institute of Allergy and Infectious Diseases, the National Institutes of Health, the National Health Service, the National Institute for Health Research (NIHR).
Abbreviations
NLRP3, NOD-like receptor protein 3; PLGA, polymers like poly(lactic-co-glycolic) acid; NF-κB, Nuclear factor kappa-B; TLR, Toll-like receptors; IRF, Interferon regulatory factor; DC, dendritic cell; TF, Transcription factor; STAT, Signal transducer and activator of transcription; MAIT, mucosal-associated invariant T cells; HLA-G, human leukocyte antigen G; HIF α, Hypoxia-inducible factor 1-alpha; TME, tumor microenvironment; DNMTi, DNA methyltransferase inhibitors; HDACi, Histone deacetylase inhibitors; MDS, myelodysplastic syndrome; NY-ESO-1, New York esophageal cancer; HPV, human papillomavirus; EZH2, Enhancers of Zeste Homolog 2; Tregs, regulatory T cells; CTLA-4, cytotoxic T-lymphocyte-associated antigen 4; HPTA, 2-hexyl-4-pentylene acid.
References
1. Marrack P, McKee AS, Munks MW. Towards an understanding of the adjuvant action of aluminium. Nat Rev Immunol. (2009) 9:287–93. doi: 10.1038/nri2510
2. Facciolà A, Visalli G, Laganà A, Di Pietro A. An overview of vaccine adjuvants: current evidence and future perspectives. Vaccines. (2022) 89:1–26. doi: 10.3390/vaccines10050819
3. Apostólico Jde S, Lunardelli VA, Coirada FC, Boscardin SB, Rosa DS. Adjuvants: classification, modus operandi, and licensing. J Immunol Res. (2016) 2016:1459394. doi: 10.1155/2016/1459394
4. Lawrence T. The nuclear factor NF-kappaB pathway in inflammation. Cold Spring Harbor Perspect Biol. (2009) 1:a001651. doi: 10.1101/cshperspect.a001651
5. Longhi MP, Trumpfheller C, Idoyaga J, Caskey M, Matos I, Kluger C, et al. Dendritic cells require a systemic type I interferon response to mature and induce CD4+ Th1 immunity with poly IC as adjuvant. J Exp Med. (2009) 206:1589–602. doi: 10.1084/jem.20090247
6. Didierlaurent AM, Morel S, Lockman L, Giannini SL, Bisteau M, Carlsen H, et al. AS04, an aluminum salt- and TLR4 agonist-based adjuvant system, induces a transient localized innate immune response leading to enhanced adaptive immunity. J Immunol. (2009) 183:6186–97. doi: 10.4049/jimmunol.0901474
7. Denny L, Hendricks B, Gordon C, Thomas F, Hezareh M, Dobbelaere K, et al. Safety and immunogenicity of the HPV-16/18 AS04-adjuvanted vaccine in HIV-positive women in South Africa: a partially-blind randomised placebo-controlled study. Vaccine. (2013) 31:5745–53. doi: 10.1016/j.vaccine.2013.09.032
8. Champion CR. Heplisav-B: A hepatitis B vaccine with a novel adjuvant. Ann Pharmacother. (2021) 55:783–91. doi: 10.1177/1060028020962050
9. Schillie S, Harris A, Link-Gelles R, Romero J, Ward J, Nelson N. Recommendations of the advisory committee on immunization practices for use of a hepatitis B vaccine with a novel adjuvant. MMWR. Morbidity mortality weekly Rep. (2018) 67:455–8. doi: 10.15585/mmwr.mm6715a5
10. Toussi DN, Massari P. Immune adjuvant effect of molecularly-defined toll-like receptor ligands. Vaccines. (2014) 2:323–53. doi: 10.3390/vaccines2020323
11. Paston SJ, Brentville VA, Symonds P, Durrant LG, Vaccines C. Adjuvants, and delivery systems. Front Immunol. (2021) 12:627932. doi: 10.3389/fimmu.2021.627932
12. Decout A, Katz JD, Venkatraman S, Ablasser A. The cGAS-STING pathway as a therapeutic target in inflammatory diseases. Nat Rev Immunol. (2021) 21:548–69. doi: 10.1038/s41577-021-00524-z
13. Luo K, Li N, Ye W, Gao H, Luo X, Cheng B. Activation of stimulation of interferon genes (STING) signal and cancer immunotherapy. Molecules. (2022) 4638:1–17. doi: 10.3390/molecules27144638
14. Van Herck S, Feng B, Tang L. Delivery of STING agonists for adjuvanting subunit vaccines. Advanced Drug delivery Rev. (2021) 179:114020. doi: 10.1016/j.addr.2021.114020
15. Chen Q, Sun L, Chen ZJ. Regulation and function of the cGAS-STING pathway of cytosolic DNA sensing. Nat Immunol. (2016) 17:1142–9. doi: 10.1038/ni.3558
16. Konno H, Yamauchi S, Berglund A, Putney RM, Mulé JJ, Barber GN. Suppression of STING signaling through epigenetic silencing and missense mutation impedes DNA damage mediated cytokine production. Oncogene. (2018) 37:2037–51. doi: 10.1038/s41388-017-0120-0
17. Sun HX, Xie Y, Ye YP. Advances in saponin-based adjuvants. Vaccine. (2009) 27:1787–96. doi: 10.1016/j.vaccine.2009.01.091
18. Ho NI, Huis In ‘t Veld LGM, van Eck van der Sluijs J, Heuts BMH, Looman MWG, Kers-Rebel ED, et al. Saponin-based adjuvants enhance antigen cross-presentation in human CD11c(+) CD1c(+) CD5(-) CD163(+) conventional type 2 dendritic cells. J immunotherapy Cancer. (2023) 11:1–17. doi: 10.1136/jitc-2023-007082
19. Manara MC, Valente S, Cristalli C, Nicoletti G, Landuzzi L, Zwergel C, et al. A quinoline-based DNA methyltransferase inhibitor as a possible adjuvant in osteosarcoma therapy. Mol Cancer Ther. (2018) 17:1881–92. doi: 10.1158/1535-7163.MCT-17-0818
20. Jin B, Li Y, Robertson KD. DNA methylation: superior or subordinate in the epigenetic hierarchy? Genes Cancer. (2011) 2:607–17. doi: 10.1177/1947601910393957
21. Bannister AJ, Kouzarides T. Regulation of chromatin by histone modifications. Cell Res. (2011) 21:381–95. doi: 10.1038/cr.2011.22
22. Salerno-Goncalves R, Chen H, Bafford AC, Sztein MB. Epigenetic regulation in epithelial cells and innate lymphocyte responses to S. Typhi infection: insights into IFN-γ production and intestinal immunity. Front Immunol. (2024) 15. doi: 10.3389/fimmu.2024.1448717
23. Sztein MB, Bafford AC, Salerno-Goncalves R. Salmonella enterica serovar Typhi exposure elicits ex vivo cell-type-specific epigenetic changes in human gut cells. Sci Rep. (2020) 10:13581. doi: 10.1038/s41598-020-70492-2
24. Salerno-Gonçalves R, Fresnay S, Magder L, Darton TC, Waddington CS, Blohmke CJ, et al. Mucosal-Associated Invariant T cells exhibit distinct functional signatures associated with protection against typhoid fever. Cell Immunol. (2022) 378:104572. doi: 10.1016/j.cellimm.2022.104572
25. Salerno-Goncalves R, Chen H, Bafford AC, Izquierdo M, Hormazábal JC, Lagos R, et al. Early host immune responses in a human organoid-derived gallbladder monolayer to Salmonella Typhi strains from patients with acute and chronic infections: a comparative analysis. Front Immunol. (2024) 15:1334762. doi: 10.3389/fimmu.2024.1334762
26. Salerno-Goncalves R, Kayastha D, Fasano A, Levine MM, Sztein MB. Crosstalk between leukocytes triggers differential immune responses against Salmonella enterica serovars Typhi and Paratyphi. PloS Negl Trop Dis. (2019) 13:e0007650. doi: 10.1371/journal.pntd.0007650
27. Salerno-Gonçalves R, Galen JE, Levine MM, Fasano A, Sztein MB. Manipulation of salmonella typhi gene expression impacts innate cell responses in the human intestinal mucosa. Front Immunol. (2018) 9. doi: 10.3389/fimmu.2018.02543
28. Statello L, Guo CJ, Chen LL, Huarte M. Gene regulation by long non-coding RNAs and its biological functions. Nature reviews. Mol Cell Biol. (2021) 22:96–118. doi: 10.1038/s41580-020-00315-9
29. Kaikkonen MU, Lam MT, Glass CK. Non-coding RNAs as regulators of gene expression and epigenetics. Cardiovasc Res. (2011) 90:430–40. doi: 10.1093/cvr/cvr097
30. Scalavino V, Liso M, Serino G. Role of microRNAs in the regulation of dendritic cell generation and function. Int J Mol Sci. (2020) 21:1–18. doi: 10.3390/ijms21041319
31. Lee A, Wimmers F, Pulendran B. Epigenetic adjuvants: durable reprogramming of the innate immune system with adjuvants. Curr Opin Immunol. (2022) 77:102189. doi: 10.1016/j.coi.2022.102189
32. Wimmers F, Donato M, Kuo A, Ashuach T, Gupta S, Li C, et al. The single-cell epigenomic and transcriptional landscape of immunity to influenza vaccination. Cell. (2021) 184:3915–3935.e21. doi: 10.1016/j.cell.2021.05.039
33. Pulendran B, S. Arunachalam P, O’Hagan DT. Emerging concepts in the science of vaccine adjuvants. Nat Rev Drug Discovery. (2021) 20:454–75. doi: 10.1038/s41573-021-00163-y
34. Burny W, Callegaro A, Bechtold V, Clement F, Delhaye S, Fissette L, et al. Different adjuvants induce common innate pathways that are associated with enhanced adaptive responses against a model antigen in humans. Front Immunol. (2017) 8:943. doi: 10.3389/fimmu.2017.00943
35. Qi C, Cai Y, Gunn L, Ding C, Li B, Kloecker G, et al. Differential pathways regulating innate and adaptive antitumor immune responses by particulate and soluble yeast-derived β-glucans. Blood. (2011) 117:6825–36. doi: 10.1182/blood-2011-02-339812
36. Novakovic B, Habibi E, Wang SY, Arts RJW, Davar R, Megchelenbrink W, et al. beta;-glucan reverses the epigenetic state of LPS-induced immunological tolerance. Cell. (2016) 167:1354–68.e14. doi: 10.1016/j.cell.2016.09.034
37. Ciarlo E, Heinonen T, Théroude C, Asgari F, Le Roy D, Netea MG, et al. Trained immunity confers broad-spectrum protection against bacterial infections. J Infect Dis. (2020) 222:1869–81. doi: 10.1093/infdis/jiz692
38. Netea MG, Joosten LA, Latz E, Mills KH, Natoli G, Stunnenberg HG, et al. Trained immunity: A program of innate immune memory in health and disease. Science. (2016) 352:aaf1098. doi: 10.1126/science.aaf1098
39. Keshari S, Shavkunov AS, Miao Q, Saha A, Minowa T, Molgora M, et al. Comparing neoantigen cancer vaccines and immune checkpoint therapy unveils an effective vaccine and anti-TREM2 macrophage-targeting dual therapy. Cell Rep. (2024) 43:114875. doi: 10.1016/j.celrep.2024.114875
40. Tien FM, Lu HH, Lin SY, Tsai HC. Epigenetic remodeling of the immune landscape in cancer: therapeutic hurdles and opportunities. J BioMed Sci. (2023) 30:3. doi: 10.1186/s12929-022-00893-0
41. Wang H, Wang J, Ning C, Zheng X, Fu J, Wang A, et al. Genome-wide DNA methylation and transcriptome analyses reveal genes involved in immune responses of pig peripheral blood mononuclear cells to poly I:C. Sci Rep. (2017) 7:9709. doi: 10.1038/s41598-017-10648-9
42. Kartikasari AER, Prakash MD, Cox M, Wilson K, Boer JC, Cauchi JA, et al. Therapeutic cancer vaccines-T cell responses and epigenetic modulation. Front Immunol. (2018) 9:3109. doi: 10.3389/fimmu.2018.03109
43. McCutcheon SR, Rohm D, Iglesias N, Gersbach CA. Epigenome editing technologies for discovery and medicine. Nat Biotechnol. (2024) 42:1199–217. doi: 10.1038/s41587-024-02320-1
44. Dong Y, Zhang S, Gao X, Yin D, Wang T, Li Z, et al. HIF1α epigenetically repressed macrophages via CRISPR/Cas9-EZH2 system for enhanced cancer immunotherapy. Bioact Mater. (2021) 6:2870–80. doi: 10.1016/j.bioactmat.2021.02.008
45. Salerno-Gonçalves R, Rezwan T, Luo D, Tettelin H, Sztein MB. B cells control mucosal-associated invariant T cell responses to Salmonella enterica serovar Typhi infection through the CD85j HLA-G receptor. Front Immunol. (2021). doi: 10.3389/fimmu.2021.728685
46. Mouillot G, Marcou C, Rousseau P, Rouas-Freiss N, Carosella ED, Moreau P. HLA-G gene activation in tumor cells involves cis-acting epigenetic changes. Int J Cancer. (2005) 113:928–36. doi: 10.1002/(ISSN)1097-0215
47. Takeshima H, Ushijima T. Accumulation of genetic and epigenetic alterations in normal cells and cancer risk. NPJ Precis Oncol. (2019) 3:7. doi: 10.1038/s41698-019-0079-0
48. Griffiths EA, Srivastava P, Matsuzaki J, Brumberger Z, Wang ES, Kocent J, et al. NY-ESO-1 vaccination in combination with decitabine induces antigen-specific T-lymphocyte responses in patients with myelodysplastic syndrome. Clin Cancer research: an Off J Am Assoc Cancer Res. (2018) 24:1019–29. doi: 10.1158/1078-0432.CCR-17-1792
49. Zhou H, Ma Y, Liu F, Li B, Qiao D, Ren P, et al. Current advances in cancer vaccines targeting NY-ESO-1 for solid cancer treatment. Front Immunol. (2023) 14:1255799. doi: 10.3389/fimmu.2023.1255799
50. Nie J, Liu L, Li X, Han W. Decitabine, a new star in epigenetic therapy: the clinical application and biological mechanism in solid tumors. Cancer Lett. (2014) 354:12–20. doi: 10.1016/j.canlet.2014.08.010
51. Woloszynska-Read A, James SR, Song C, Jin B, Odunsi K, Karpf AR. BORIS/CTCFL expression is insufficient for cancer-germline antigen gene expression and DNA hypomethylation in ovarian cell lines. Cancer Immun. (2010) 10:6.
52. Moran B, Davern M, Reynolds JV, Donlon NE, Lysaght J. The impact of histone deacetylase inhibitors on immune cells and implications for cancer therapy. Cancer Lett. (2023) 559:216121. doi: 10.1016/j.canlet.2023.216121
53. Lee SY, Huang Z, Kang TH, Soong RS, Knoff J, Axenfeld E, et al. Histone deacetylase inhibitor AR-42 enhances E7-specific CD8+ T cell-mediated antitumor immunity induced by therapeutic HPV DNA vaccination. J Mol Med (Berl). (2013) 91:1221–31. doi: 10.1007/s00109-013-1054-9
54. Goswami S, Apostolou I, Zhang J, Skepner J, Anandhan S, Zhang X, et al. Modulation of EZH2 expression in T cells improves efficacy of anti-CTLA-4 therapy. J Clin Invest. (2018) 128:3813–8. doi: 10.1172/JCI99760
55. Buchbinder EI, Desai A. CTLA-4 and PD-1 pathways: similarities, differences, and implications of their inhibition. Am J Clin Oncol. (2016) 39:98–106. doi: 10.1097/COC.0000000000000239
56. Chen C, Lim D, Cai Z, Zhang F, Liu G, Dong C, et al. HDAC inhibitor HPTA initiates anti-tumor response by CXCL9/10-recruited CXCR3(+)CD4(+)T cells against PAHs carcinogenicity. Food Chem toxicology: an Int J published Br Ind Biol Res Assoc. (2023) 176:113783. doi: 10.1016/j.fct.2023.113783
57. Tokunaga R, Zhang W, Naseem M, Puccini A, Berger MD, Soni S, et al. CXCL9, CXCL10, CXCL11/CXCR3 axis for immune activation - A target for novel cancer therapy. Cancer Treat Rev. (2018) 63:40–7. doi: 10.1016/j.ctrv.2017.11.007
58. Newton HJ, Ang DK, van Driel IR, Hartland EL. Molecular pathogenesis of infections caused by Legionella pneumophila. Clin Microbiol Rev. (2010) 23:274–98. doi: 10.1128/CMR.00052-09
Keywords: adjuvant, epigenetic, vaccine, cancer therapy, immunity, human
Citation: Megdiche Y and Salerno-Gonçalves R (2025) Harnessing adjuvant-induced epigenetic modulation for enhanced immunity in vaccines and cancer therapy. Front. Immunol. 16:1547213. doi: 10.3389/fimmu.2025.1547213
Received: 17 December 2024; Accepted: 05 February 2025;
Published: 18 February 2025.
Edited by:
Abel A. Ramos Vega, National Polytechnic Institute (IPN), MexicoReviewed by:
Chaitenya Verma, Sharda University, IndiaCopyright © 2025 Megdiche and Salerno-Gonçalves. This is an open-access article distributed under the terms of the Creative Commons Attribution License (CC BY). The use, distribution or reproduction in other forums is permitted, provided the original author(s) and the copyright owner(s) are credited and that the original publication in this journal is cited, in accordance with accepted academic practice. No use, distribution or reproduction is permitted which does not comply with these terms.
*Correspondence: Rosângela Salerno-Gonçalves, cm1lemdoYW5Ac29tLnVtYXJ5bGFuZC5lZHU=