- 1Department of Geriatrics, Guangzhou First People’s Hospital, Guangzhou Medical University, Guangzhou, China
- 2Department of Gastroenterology and Hepatology, Guangzhou First People’s Hospital, Guangzhou Medical University, Guangzhou, China
- 3Department of Gastroenterology, The First People’s Hospital of Foshan, Foshan, China
Inorganic dietary nanoparticles (IDNPs) are frequently utilized as food additives and in packaging, resulting in their exposure becoming a substantial yet often overlooked concern for patients with inflammatory bowel disease (IBD). Considering that impaired intestinal barrier function plays a central role in the pathogenesis of IBD, this review concentrates on the roles and mechanisms of IDNPs in the intestinal barrier (physical, chemical, biological, and immune barriers) of IBD patients. Previous studies have shown that different types of nanoparticles have varying effects on animals in diverse states. In this context, factors such as the source, size, shape, dosage, and duration of action of the nanoparticles, as well as the species, gender, dietary habits, and age of the animals, significantly influence research outcomes. Future studies should undertake more comprehensive explorations into the effects and mechanisms of IDNPs with diverse sources and properties in IBD patients.
1 Introduction
Inorganic dietary nanoparticles (IDNPs) are defined as inorganic materials with dimensions typically ranging from 1 to 100 nanometers in food, exhibiting distinct physicochemical properties compared to their bulk counterparts. The IDNPs can be broadly classified into metal nanoparticles (e.g., gold, silver, zinc), metal oxide nanoparticles (e.g., titanium dioxide, iron oxide, zinc oxide), and composite nanoparticles (NPs) that combine organic and inorganic materials (1, 2). Their nanoscale dimension endows them with high surface area-to-volume ratios, which enhance their reactivity and interaction with biological molecules (3). Additionally, their size, shape, and surface properties can be tailored to influence their absorption, distribution, and bioavailability within the body (4). IDNPs are now widely utilized in various scenarios such as food additives and food packaging, and their safety and environmental toxicity are garnering increasing attention from the public.
Inflammatory bowel disease (IBD) is a chronic inflammatory disease primarily affecting the gastrointestinal tract, mainly encompassing Crohn’s disease (CD) and ulcerative colitis (UC). CD can manifest anywhere from the mouth to the anus, featuring transmural inflammation that may lead to complications such as strictures and fistulas (5). In contrast, UC is confined to the colonic mucosa and is characterized by continuous inflammation extending proximally from the rectum (5, 6). Clinically, IBD presents with diverse gastrointestinal symptoms, including abdominal pain, diarrhea, and weight loss, along with extraintestinal manifestations like arthritis and skin lesions (5). IBD is characterized by periods of exacerbation and remission, significantly impacting the morbidity and quality of life of patients. The management of IBD involves medication intervention, dietary adjustments, and surgical choices, but there is no single method that can completely cure patients (7). The etiology of IBD is multifactorial, involving genetic predisposition, environmental triggers, and deregulated immune responses to gut microbiota (8). As a type of environmental factor, the conclusions drawn from previous studies on the role of IDNPs in IBD are inconsistent (9, 10). Therefore, we have reviewed the mechanism of function of IDNPs in the intestinal barrier of IBD in order to provide insights for the future diagnosis, treatment, and prevention of the disease.
2 Inorganic dietary nanoparticles
2.1 The source of IDNPs
In modern life, IDNPs are widely utilized across various fields. Titanium dioxide nanoparticles (TiO2 NPs) are commonly used as a white pigment and brightening agent in refined foods, including confectionery items, white sauces, and icings (11–13). It is also found in toothpaste and nondairy creamers (14). Silicon dioxide nanoparticles (SiO2 NPs) are often added to powdered foods as an anticaking agent, including salt, icing sugar, spices, dried milk, and dry mixes (15, 16). Silver nanoparticles (Ag NPs) are used as a coloring agent for products like cakes, ice creams, frozen desserts, chocolates, and antibacterial food packaging (17). Iron oxide nanoparticles (Fe2O3 NPs) function as a food colorant as well as an ingredient in cosmetics and pharmaceutical coatings (18). Zinc oxide nanoparticles (ZnO NPs) can be found in dietary supplements and functional foods (19, 20). Moreover, TiO2 is used for pill coatings in the pharmaceutical industry, while AlSi is applied in paper manufacturing and powder fabrication.
2.2 The exposure levels of different IDNPs
In recent years, the exposure levels of TiO2 have been widely studied, and there are significant differences in exposure levels among different age groups in different countries due to varying dietary habits and the content of IDNPs in food. In China, children aged 2-5 are exposed to 0.337 mg TiO2/kgbw/day, whereas elderly individuals (aged 70 and above) are exposed to 0.061 mg TiO2/kgbw/day (21). In the Netherlands, the average exposure level among the population (aged 2 and above) ranges from 0.06 to 0.67 mg TiO2/kgbw/day (22). In the United States and the United Kingdom, children under 10 years old are exposed to 1-2 and 2-3 mg TiO2/kgbw/day, respectively; while the exposure levels for other age groups are 0.2-0.7 mg and 1 mg TiO2/kgbw/day, respectively (11). In Germany, children under 10 years old exhibit higher exposure levels compared to other countries, with those aged 3-9 exposed to 3.3 mg TiO2/kgbw/day (23). Research on the exposure levels of other IDNPs is relatively scarce. According to the European Food Safety Authority, infants are exposed to a daily dose of food-grade silica (E551) ranging from 0.8 to 74.2 mg/kgbw, children from 2.7 to 31.2 mg/kgbw, and adults from 0.9 to 13.2 mg/kgbw (24). The daily exposure level of silver (E174) for individuals ranging from children to adults is 0.03-2.6 mg/kgbw (24).
2.3 Intestinal absorption mode of IDNPs
IDNPs enter the digestive tract through the mouth along with food and, propelled by the peristalsis of the gastrointestinal tract, reach the small intestine. Some of them are absorbed into the system via the intestine, while the vast majority are excreted in the feces (25, 26). In experiments involving human volunteers, it was discovered that the blood TiO2 level began to rise 2 hours after oral ingestion, peaking between 8-12 hours later (27, 28). Furthermore, the presence of TiO2 nanoparticles was detected in the liver and spleen of both mice and humans, suggesting that IDNPs can accumulate within the body (29–31). The mechanisms by which IDNPs are absorbed into the systemic circulation from the intestine primarily encompass three categories: 1) trans-intestinal epithelial cell pathway; 2) transport via tight junctions adjacent to cells; 3) transport through transcytosis across M cells in Peyer’s patches (32, 33). The ability of IDNPs to penetrate the intestinal epithelium is dependent on their diameter (34). Specifically, IDNPs with diameters less than 150 µm are capable of penetrating the intestinal epithelium, whereas those with diameters under 2.5 µm are absorbed by M cells in Peyer’s patches (34, 35).
2.4 Factors affecting the function of IDNPs
The inherent characteristics of IDNPs, encompassing their composition, size, shape, surface properties, and aggregation state, can influence their functionality. IDNPs can consist of various inorganic substances, including titanium dioxide, silicon dioxide, zinc oxide, iron oxide, and more. Their size, ranging from a few nanometers to several hundred nanometers, and shape—whether sheet-like, spherical, cylindrical, or rectangular—are determined by their manufacturing method. Furthermore, their surface properties are dependent on the types of molecules present on their surface (36–38). Nevertheless, upon entering the digestive tract, the properties of these nanoparticles may undergo significant changes due to interactions with food ingredients or substances naturally present in the gastrointestinal tract (39).
After interacting with food ingredients or naturally biological substances in the gastrointestinal tract (proteins, lipids, carbohydrates, etc.), biological corona can form around the IDNPs (40, 41). The corona not only alter the structure and function of the ingredients themselves but also influence the absorption, accumulation, and toxicity of IDNPs (42, 43) (Figure 1). First, the adsorption of substances onto IDNPs can modify the composition, thickness, and charge of the surface layer, thereby affecting its aggregation state (44, 45). For instance, albumin can facilitate the aggregation of SiO2 NPs, possibly due to charge neutralization and bridging effects (40). Conversely, adding proteins to TiO2 and ZnO NPs can enhance their dispersion, possibly due to the formation of protein corona that increase the repulsion between particles (41). Additionally, the formation of biological corona can also impact the absorption of nanoparticles. Research has revealed that in the presence of proteins, the absorption of Fe3O4 NPs by Caco-2 cell monolayers increases, possibly due to the protein corona promoting the dispersion of nanoparticles (46). Finally, the formation of protein corona may alter the biocompatibility of nanoparticles, thereby affecting the toxicity of IDNPs. Study has found that the surface adsorption of bovine lactoferrin on silver nanoparticles reduces its cytotoxicity towards THP-1 cell lines (42). Therefore, the function of IDNPs in the body is a complex state that varies depending on the type of food consumed, the location in the intestine, and the disease status. Conversely, nanoparticles can also influence the activity of intrinsic biological proteins in the gastrointestinal tract while being influenced by the properties of food proteins. For example, TiO2 and SiO2 NPs can interact with trypsin and reduce its activity (47). Additionally, Ag NPs have been shown to diminish the activity of gastric protease due to surface denaturation caused by the adsorption of digestive enzymes onto the nanoparticles’ surface (48).
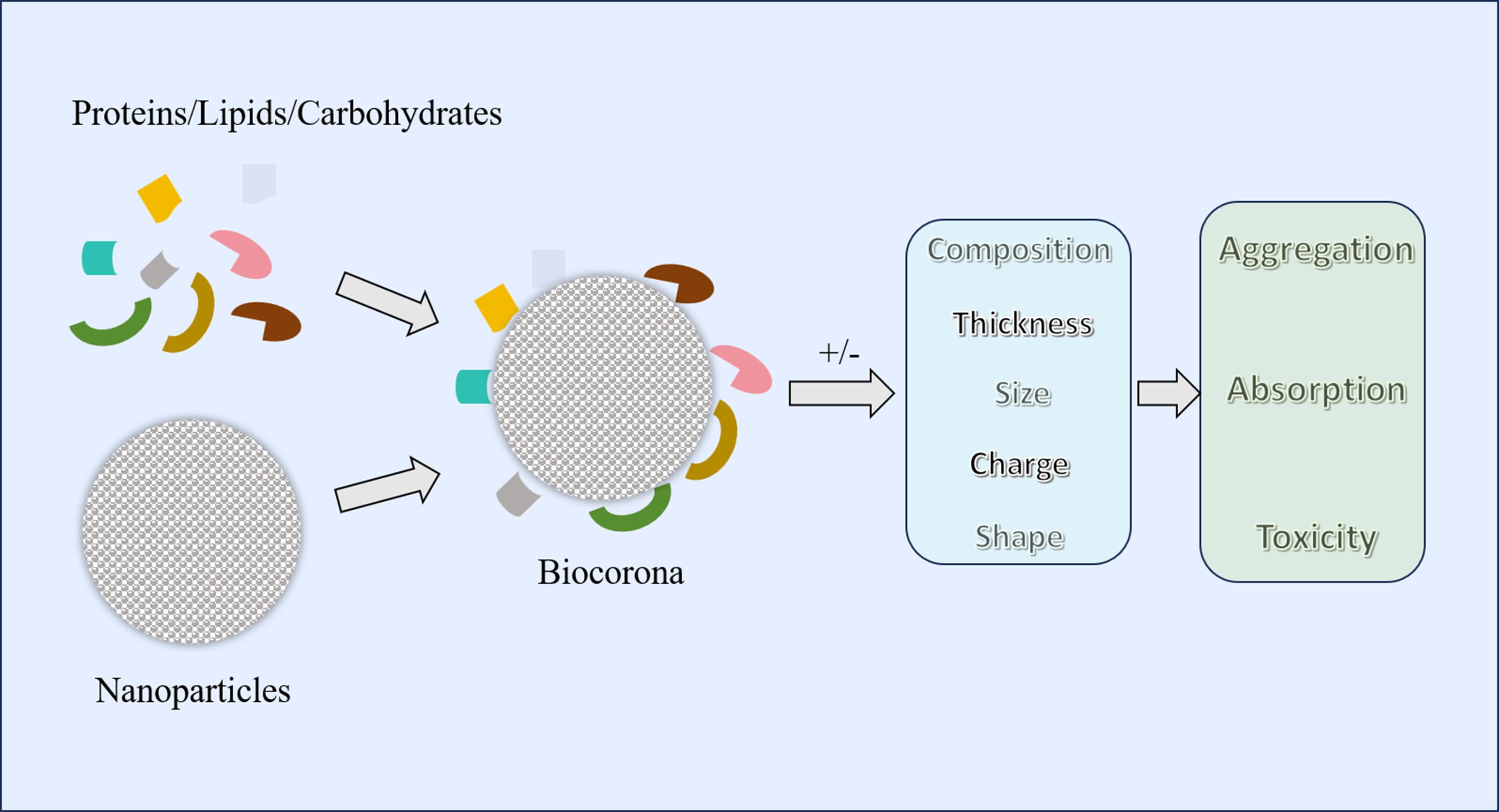
Figure 1. Formation of biocorona and its impact on different aspects of nanoparticles. Different food ingredients or inherent components of the gastrointestinal tract (proteins, lipids, carbohydrates, etc.) can form a biocorona with nanoparticles. The formation of a biocorona may alter the composition, size, thickness, charge, and shape of nanoparticles, thereby changing their aggregation, absorption, and toxicity.
3 The pathogenesis of IBD
The pathogenesis of IBD is multifactorial, involving environmental factors, dysregulation of the immune response, alterations in the gut microbiota, and genetic predispositions (49). The intestinal barrier is a complex and dynamic structure that mainly includes physical barrier, biological barrier, chemical barrier, and immune barrier, playing a crucial role in maintaining homeostasis within the gastrointestinal tract (Figure 2). Intestinal barrier serves as the first line of defense against luminal antigens, pathogens, and toxins. Previous studies have shown that damage to the intestinal barrier structure and function plays an important role in the pathogenesis of IBD (50–52).
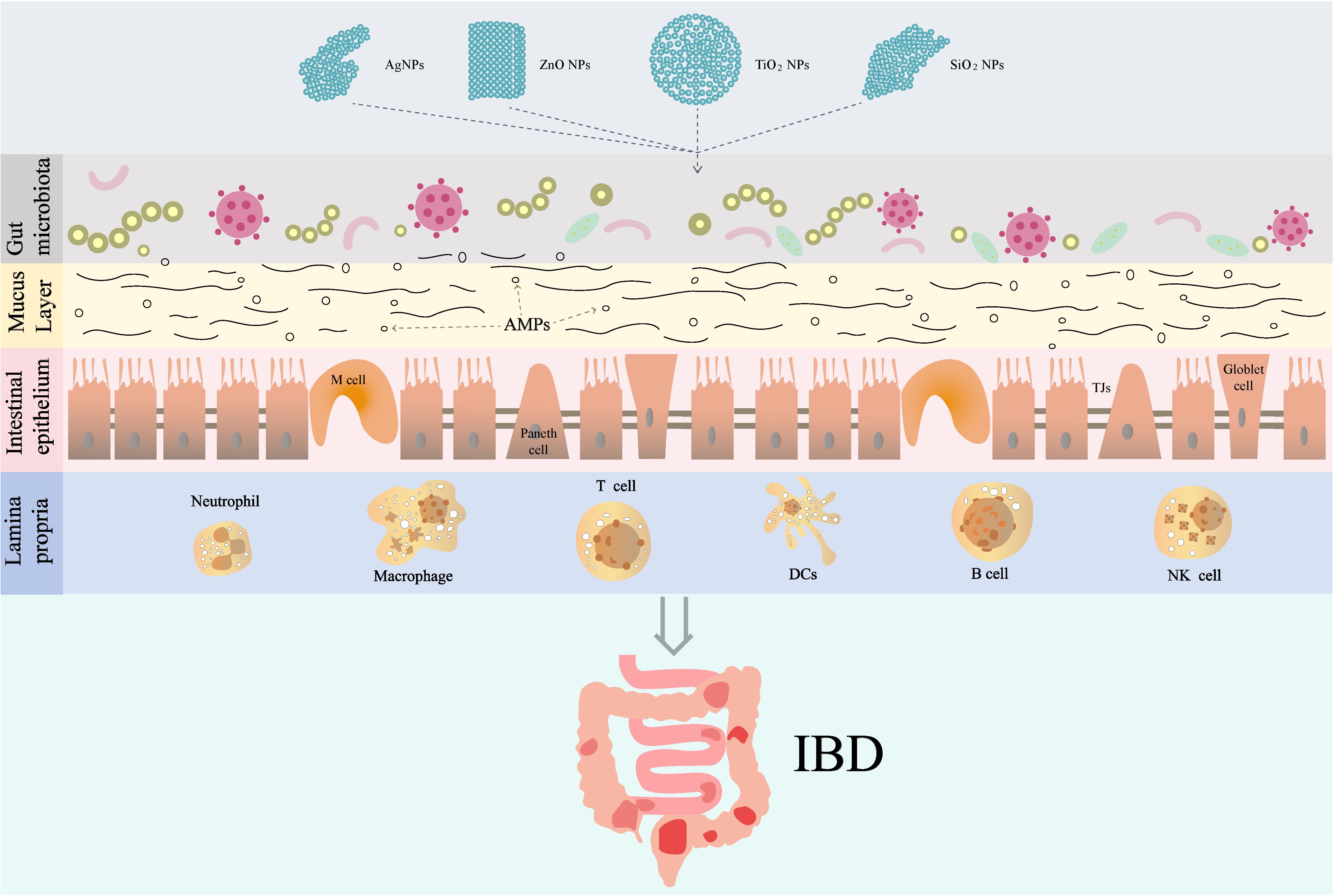
Figure 2. The role and mechanism of IDNPs in IBD. TJs, Tight junctions; AMPs, antimicrobial peptides; DCs, dendritic cells.
3.1 Physical barrier in IBD
Intestinal physical barrier is maintained by a complex interplay of epithelial cells, and tight junctions, which collectively regulate permeability and immune responses. Intestinal epithelial cells (IECs) are single-layer columnar cells that cover the surface of the intestine, mainly including absorptive epithelial cells, goblet cells, endocrine cells, and Paneth cells (53). Normal IECs are in a continuously renewing state, with intestinal stem cells generating new epithelial cells daily to replace those that shed. The dynamic equilibrium established by this turnover ensures the overall number of IECs remains constant. In diseased states, an abnormal increase in IEC mortality disrupts the structure and function of the intestinal mucosa. Gut microbiota can then exacerbate intestinal inflammation through this compromised mucosa. Conversely, abnormal inflammation can trigger the death of IECs, amplifying the inflammatory response and thus initiating a vicious cycle, from intestinal mucosal damage to severe intestinal inflammation (54, 55). Moreover, the loss of specific epithelial cell types, such as Paneth cells and goblet cells, has been associated with increased susceptibility to inflammation and apoptosis in IBD. Paneth cells play a crucial role in maintaining gut homeostasis by secreting antimicrobial peptides, and their depletion has been linked to dysbiosis and inflammation (56). Similarly, goblet cells are essential for mucus production, which protects the epithelial barrier; their loss can lead to increased epithelial permeability and inflammation (57, 58).
Tight junctions (TJs) are specialized structures formed by a complex of proteins, including claudins, occludin, and zonula occludens (ZO) proteins, which regulate paracellular permeability and maintain epithelial integrity (59, 60). Human studies have demonstrated that claudin-2 is upregulated in active Crohn’s disease, leading to increased paracellular permeability, while claudin-5 and claudin-8 are downregulated, contributing to barrier dysfunction (61, 62). In addition, other studies have reported downregulation of claudin-1, claudin-3, and claudin-4 in inflamed tissues of UC patients, which correlates with increased permeability and barrier dysfunction (63). Previous animal studies have shown that in animal models of dextran sulfate sodium (DSS)-induced colitis, there is a significant reduction in the expression of tight junction proteins such as ZO-1 and claudins, which correlates with increased intestinal permeability (64, 65). This dysregulation is not merely a consequence of inflammation but appears to precede the onset of severe bowel inflammation, indicating a potential early therapeutic target for intervention in IBD (64, 66). Furthermore, the inflammatory cytokines, particularly tumor necrosis factor-alpha (TNF-α) and interferon-gamma (IFN-γ), have been shown to disrupt tight junction integrity by altering the expression and localization of these proteins, thereby contributing to the progression of IBD (67, 68).
3.2 Biological barrier in IBD
The intestinal biological barrier, also known as the microecological barrier, refers to the normal microbiota in the intestine. The gut microbiota is a complex and dynamic ecosystem comprising trillions of microorganisms, including bacteria, fungi, viruses, and archaea. Gut microbiota is involved in synthesizing essential vitamins and metabolites, modulating the immune system, and protecting the host against pathogens by outcompeting harmful bacteria (69). In a healthy individual, the gut microbiota consists of a diverse array of microbial species that coexist in a balanced state, typically dominated by four main phyla: Firmicutes, Bacteroidetes, Actinobacteriota, and Proteobacteria. This diversity is crucial as it contributes to the overall stability and resilience of the microbiome, allowing it to perform essential functions such as digestion, metabolism, and immune modulation. The composition of gut microbiota can vary significantly between individuals due to factors such as genetics, diet, age, and environmental exposures. Studies have shown that a higher diversity of gut microbiota is generally associated with better health outcomes, while a decrease in diversity can lead to dysbiosis, which is linked to various health conditions, including IBD, obesity, and metabolic syndrome (70, 71). For instance, a reduction in the abundance of Firmicutes, particularly Faecalibacterium prausnitzii, has been linked to increased disease severity in IBD patients, while an overgrowth of Proteobacteria correlates with inflammation and disease flares (72, 73). This dysbiotic state not only exacerbates the local immune response but also disrupts the gut barrier function, contributing to the pathophysiology of IBD and increasing the risk of complications such as colonic cancer (74).
The bacterial metabolites short-chain fatty acids (SCFAs), primarily produced by the fermentation of dietary fibers by gut microbiota, play a pivotal role in maintaining gut health and modulating inflammation, particularly in the context of IBD. Research indicates that SCFAs, such as butyrate, propionate, and acetate, exert protective effects by enhancing the integrity of the intestinal barrier, modulating immune responses, and exhibiting anti-inflammatory properties. For instance, butyrate has been shown to promote the differentiation of regulatory T cells, which are crucial for maintaining immune homeostasis and preventing excessive inflammatory responses in the gut (75). Furthermore, SCFAs can inhibit histone deacetylases, leading to the upregulation of anti-inflammatory genes and the downregulation of pro-inflammatory cytokines, thus alleviating the inflammatory processes associated with IBD (76). Additionally, studies have demonstrated that SCFAs can enhance mucus production and strengthen tight junctions between epithelial cells, thereby improving gut barrier function and reducing permeability, which is often compromised in IBD patients (77). The therapeutic potential of SCFAs is being explored, with dietary interventions aimed at increasing SCFAs production showing promise in managing IBD symptoms and promoting remission (78).
3.3 Chemical barrier in IBD
The intestinal chemical barrier comprises various components, including the mucus layer, and antimicrobial peptides (AMPs) that work together to prevent harmful substances from penetrating the intestinal epithelium. The mucus layer is primarily composed of mucins, and glycoproteins secreted by goblet cells, which play a vital role in maintaining intestinal homeostasis and immune function (79–81). Goblet cells are crucial for the production of mucins, particularly MUC2, which forms the gel-like structure of the mucus layer. In IBD, the number and function of goblet cells are often impaired, leading to reduced mucin production and a thinner mucus layer (82–84). This reduction in mucus thickness allows for closer contact between luminal bacteria and the intestinal epithelium, potentially leading to increased inflammation and further mucosal damage (85, 86). Cytokines such as TNF-α and IL-6 have been implicated in the downregulation of mucin production, contributing to the deterioration of the mucus layer (87, 88).
AMPs are essential components of the intestinal chemical barrier, exhibiting broad-spectrum antimicrobial activity against bacteria, viruses, and fungi. These peptides are produced by various cells in the gut, including Paneth cells and epithelial cells, and are crucial for maintaining gut homeostasis. AMPs can disrupt microbial membranes, inhibit cell wall synthesis, and modulate immune responses, making them vital for the innate immune defense (6, 7). Different types of AMPs, such as defensins and cathelicidins, have been identified, each with unique mechanisms of action. The production of AMPs can be influenced by various factors, including microbial composition and inflammatory signals, highlighting their role in the gut’s response to pathogenic challenges (8, 9). In IBD, a decrease in the number of Paneth cells may lead to a reduction in the production of AMPs, resulting in dysbiosis of the gut microbiota and promotion of intestinal inflammation (56).
3.4 Immune barrier in IBD
The gut immune barrier is a complex and dynamic network that comprises various immune cells, including T cells, B cells, macrophages, NK cells, neutrophil, and dendritic cells, which are strategically located within the mucosa-associated lymphoid tissue (MALT). The MALT includes structures such as Peyer’s patches, isolated lymphoid follicles, and mesenteric lymph nodes, all of which are essential for the initiation and regulation of immune responses (89). Peyer’s patches, located in the ileum, are particularly important for sampling luminal antigens and facilitating the activation of B and T cells. They contain specialized epithelial cells called M cells that transport antigens from the gut lumen to underlying immune cells, thereby initiating immune responses (90). The presence of MALT in the gut allows for a rapid and robust immune response to pathogens while also promoting tolerance to harmless antigens, such as food proteins and commensal bacteria. Moreover, the role of MALT extends beyond the gut; it is also involved in the systemic immune response. The lymphocytes activated in the gut can migrate to other tissues, contributing to the overall immune surveillance of the body (91). Immune cells in the MALT, particularly T cells, B cells, and innate lymphoid cells, secrete a variety of cytokines that play critical roles in the pathogenesis of IBD. These cytokines mediate cell-cell communication and orchestrate the immune response. For instance, pro-inflammatory cytokines such as IL-17 and TNF-α are often elevated in IBD patients and contribute to the inflammatory processes that characterize the disease (92, 93). On the other hand, regulatory T cells (Tregs) produce anti-inflammatory cytokines like IL-10, which help to maintain immune tolerance and prevent excessive inflammation (94). The balance between pro-inflammatory and anti-inflammatory cytokines is crucial for the maintenance of intestinal homeostasis. Dysregulation of this balance can lead to chronic inflammation and tissue damage, contributing to the progression of IBD (95, 96).
4 The role and possible mechanisms of IDNPs in IBD
The effect of IDNPs on IBD has been studied in recent years. Riuz et al. have found that patients with active UC exhibit elevated levels of titanium in their blood compared to healthy controls and patients in remission from UC (10). And they found that TiO2 NPs treatment promotes intestinal inflammation in DSS-induced colitis mice via activation of NLRP3 inflammasome. However, other researchers have found that TiO2 NPs can alleviate 2,4,6-trinitrobenzenesulfonic acid (TNBS)-induced colitis in mice (9). Similarly, the effect of Ag NPs on IBD is also contradictory. For instance, Chen et al. have shown that Ag NPs induce colitis-like symptoms in the mucosa of the small intestine (97). Conversely, Siczek et al. have reported beneficial effects of Ag NPs on DSS-induced colitis (98). This may reflect the diversity of the effects of NPs on IBD, and the possible mechanisms by which IDNPs affect IBD are discussed as follows (Figure 2):
4.1 IDNPs on physical barrier injury
IDNPs mainly affect intestinal epithelial permeability by influencing the IECs and tight junctions adjacent to epithelial cells. Yan et al. indicate that exposure to TiO2 NPs leads to a significant downregulation of tight junction proteins such as occludin, and ZO-1 in the intestinal tract of juvenile mice (99). However, the expression levels of ZO-1 and claudin-2 proteins were not affected by exposure of Caco-2 cells, a widely used model for human intestinal epithelium, to SiO2 NPs (100). Besides, tight junction (Cldn1, Cldn5, Cldn6, Cldn10 and Pecam1) genes were all upregulated significantly in the ileum of female rats treated with 10 nm Ag NPs (101). In addition, Li et al. found that ZnO NPs increased the level of the ZO-1 and Claudin genes, and decreased expression of the Cyt-c and Caspase-3 levels in bovine intestinal epithelial cells (102). Interestingly, other studies have shown that exposure to Ag and ZnO NPs can display cytotoxicity, as evidenced by decreased levels of cell viability of Caco-2 cell (103, 104). This may be due to the inconsistent dosage and size of nanoparticles used in different research. (Table 1)
4.2 IDNPs on gut microbiota
4.2.1 TiO2 NPs
Some studies suggest that TiO2 NPs have minimal impact on gut microbiota at low concentrations. For instance, one study utilizing a defined model intestinal bacterial community found only minor reductions in Bacteroides ovatus and an increase in Clostridium cocleatum following exposure to food-grade TiO2 NPs at doses relevant to humans, such as after consuming one to two pieces of gum or candy (105). Similarly, another study exposing mice to 2.5 mg/kg body weight/day of TiO2 NPs for 7 days found no changes in the composition of fecal microbiota (97). These findings indicate that, under certain conditions, TiO2 NPs may not significantly disturb gut microbial balance. Conversely, other studies have reported more pronounced effects. A study utilizing a model microbial community within a model colon observed alterations in the microbial community’ s phenotype, including significant changes in bacterial metabolites such as SCFAs, after administering 3 mg/L TiO2 for 5 days (106). Another investigation found that the rutile form of TiO2 NPs increased the abundance of Proteobacteria, while the anatase form did not; both forms significantly decreased the genus Prevotella (107). Additionally, rutile NPs increased levels of Rhodococcus, whereas anatase NPs raised levels of Bacteroides (107). These findings suggest that the chemical and physical properties of TiO2 NPs, including their form and coating, influence their impact on gut microbiota. Besides, considering the long-term exposure of modern humans to IDNPs, the duration of exposure seems to be a key factor in the diversity of results across different studies. The mechanisms by which TiO2 NPs alter gut microbiota are not fully understood but may involve several factors. The small size and large surface area of TiO2 NPs facilitate interaction with bacterial cells, potentially damaging cell membranes or interfering with bacterial metabolism. Additionally, TiO2 NPs may indirectly affect gut microbiota by altering the gut environment, such as by reducing pH or affecting nutrient availability. Chronic exposure to TiO2 NPs may also lead to cumulative effects on gut microbiota, exacerbating physiological alterations induced by other factors, such as an unbalanced diet.
4.2.2 SiO2 NPs
Chen et al. have observed an increase in microbial diversity and richness, accompanied by an enrichment of Firmicutes and Proteobacteria, while populations of Bacteroidetes and Lactobacillus decreased in mice exposed to SiO2 NPs at a dose relevant to human consumption for 1 week (97). This unexpected effect underscores the need for thorough risk assessment, particularly considering the low absorption rate of SiO2 in the human gastrointestinal tract, which may lead to accumulation in the gut lumen and prolonged exposure to the microbiota. Besides, another study has found that a decreased relative abundance of Actinobacteria in SiO2 exposed mice (108).
4.2.3 ZnO NPs
Several studies have investigated the effects of ZnO NPs on gut microbiota, reporting varied outcomes based on the animal model, dosage, and duration of exposure. For instance, in piglets, exposure to ZnO NPs at 600 mg/kg for 14 days increased bacterial richness and diversity in the ileum, while these parameters decreased in the cecum and colon (109). The ileum specifically exhibited an increased abundance of Streptococcus and a decreased proportion of Lactobacillus. Conversely, in the colon, Lactobacillus abundance increased, while the populations of Oscillospira and Prevotella decreased (109). These findings suggest that ZnO NPs exert differential effects on microbiota composition along the gastrointestinal tract. Similar trends were observed in hens, where a dose-dependent decrease in bacterial community richness was noted in the ileal microbiota following exposure to ZnO NPs at doses ranging from 25 to 100 mg/kg for nine weeks (110). This decrease was accompanied by an increase in populations of Bacteroidetes, Fusobacteria, and Bacilli, along with a decrease in Proteobacteria and Lactobacillus (110). Besides, studies using human microbiota from healthy donors have shown that ZnO NPs can impair the production capacity of SCFAs, suggesting alterations in the metabolic activity of the gut microbiota (106). These changes in SCFAs production are significant, as SCFAs play crucial roles in maintaining gut health and providing energy to colonocytes. The mechanisms underlying the interactions between ZnO NPs and gut microbiota are not fully understood but likely involve multiple factors. The antimicrobial activity of ZnO NPs is well-documented and is thought to contribute to the observed changes in microbiota composition (106). Additionally, factors such as particle size, shape, coating, and dosage of ZnO NPs may influence their reactivity with gut bacteria and, therefore, their impact on microbiota. Therefore, ZnO NPs have the potential to cause significant alterations in both the composition and metabolic activity of the intestinal microbiota. These changes depend on various factors, including dosage, duration of exposure, and the specific region of the gastrointestinal tract.
4.2.4 Ag NPs
Various studies have explored the impact of Ag NPs on the intestinal microbiota, yielding contrasting results. For example, some studies have reported non-significant changes in the cecal microbiota composition of mice and rats following oral exposure to Ag NPs, regardless of particle size (111, 112). In contrast, other studies have documented shifts in microbial populations, characterized by increased proportions of Bacteroidetes and pathogenic gram-negative bacteria, alongside decreased proportions of Firmicutes, Lactobacillus, and Bifidobacterium (113). These alterations were sex-dependent, with males generally exhibiting more prominent changes in Lactobacillus and Bacteroidetes, while females showed a higher increase in Enterobacteria (113). This sex-dependency could be attributed to physiological differences in the gut microbiome between males and females, as well as variations in NPs biodistribution and toxicity. Additionally, the size of Ag NPs has been implicated as an influencing factor, albeit with heterogeneous results that do not conclusively determine a definitive size or dose effect (97, 111–114). Despite the abundance of rodent studies, data on the effects of Ag NPs on human gut microbiota are limited. One in vitro study determined the short-term impacts of Ag NPs on a defined human bacterial community, observing alterations in bacterial composition characterized by decreased abundances of beneficial bacteria and increased proportions of pathogenic species (115). These findings suggest that Ag NPs may similarly disrupt the balance of human gut microbiota, albeit the extent and consequences of these alterations require further investigation.
Mechanistically, Ag NPs exert their antimicrobial effects through various pathways. One proposed mechanism involves the release of toxic Ag ions, which are responsible for the antimicrobial activity of Ag NPs. However, sulfidation of Ag NPs, which can occur in the presence of sulfur-containing food matrices, limits the release of these ions and may contribute to the discrepancies observed in rodent studies (116). Additionally, the thinner cell membranes of gram-negative bacteria render them more vulnerable to Ag NPs toxicity, as evidenced by reduced abundances of gram-negative anaerobes such as Bacteroides ovatus after Ag NPs treatment (114). Besides, studies in vitro have shown that Ag NPs can alter the metabolic activity of microbes, resulting in reduced gas production and changes in fatty acid profiles (114, 115). These alterations suggest that Ag NPs impact the functional aspects of the gut microbiota, potentially affecting nutrient metabolism and energy homeostasis. Therefore, Ag NPs exhibit complex and multifaceted effects on the gut microbiota, influenced by factors such as dose, size, duration of exposure, and sex of the host. While some studies report profound alterations in microbial populations, others suggest minimal impact. The mechanisms underlying these effects are not fully understood but likely involve the release of toxic ions, disruption of microbial metabolism, and sex-dependent differences in NPs biodistribution and toxicity.
4.3 IDNPs on chemical barrier
To date, no studies have investigated the effects of IDNPs on antimicrobial peptides. Various IDNPs have effects on the intestinal mucus layer. Limage et al. demonstrated that exposure to TiO2 NPs resulted in changes to the mucus layer’s thickness and composition, which could have profound implications for gut health and microbiota interactions (117). Besides, Jeong et al. found that rats exposed to Ag NPs (60 nm) for 28 days exhibited increased mucus secretion, and the levels of neutral and acidic mucins in the goblet cells of the ileum, colon, or rectum were significantly decreased (118). Among the acidic mucins, the proportion of sulfated mucins declined, while the proportion of sialylated mucins increased (118). Van den Brule et al. reported that goblet cells in the ileum of Ag NPs-treated mice were not significantly affected, and the integrity of the glycocalyx was maintained (116). Williams et al. found that the administration of Ag NPs to male and female rats had little effect on MUC2 expression but induced a decrease in MUC3 expression in the ileum. The reduction of MUC3 in female rats was more significant compared to male rats (113). Although there are no studies on the effects of ZnO NPs on the intestinal mucus layer, previous research has shown that zinc deficiency can disrupt mucus production (119). Given the complex interactions between nanoparticles and the intestinal mucus layer, further experiments are needed to verify the impact of ZnO NPs.
4.4 IDNPs on immune barrier
4.4.1 TiO2 NPs
Previous study has demonstrated that TiO2 NPs can traverse the ileum epithelium and Peyer’ s patches, leading to epithelial impairment and chronic damage (32). This initial interaction paves the way for subsequent immune disturbances. Upon entering the intestinal mucosa, TiO2 NPs disrupt tight junctions between intestinal epithelial cells, compromising the intestinal barrier function (99). This disruption not only facilitates the translocation of additional particles and microbial components but also initiates an inflammatory cascade (99). In vitro study further corroborate these findings, revealing that TiO2 NPs induce the expression of pro-inflammatory cytokines such as TNF-α, IFN-γ, and IL-12, which are hallmarks of a Th1-mediated immune response (120). In vivo studies using animal models have also highlighted the immune-modulatory effects of TiO2 NPs. Exposure to TiO2 NPs has been associated with increased production of both Th1 and Th2 cytokines in the mucosa of the small intestine, indicating a complex interplay between different immune pathways (121, 122). Notably, these immune disturbances coincide with microbiota dysbiosis, further perpetuating the inflammatory state (121). The mechanisms underlying these immune effects are multifaceted. TiO2 NPs have been shown to prime macrophages with an abnormal activation state, characterized by an excessive pro-inflammatory phenotype and suppressed innate immune function (123). Additionally, TiO2 NPs induce mitochondrial dysfunction and oxidative stress, which can exacerbate inflammation and impair immune cell function (123). Of particular concern is the potential for TiO2 NPs to worsen pre-existing intestinal inflammation, such as that observed in IBD (10). Studies in animal models of IBD have demonstrated that oral administration of TiO2 NPs exacerbates intestinal inflammation through the activation of the NLRP3 inflammasome, resulting in the release of pro-inflammatory cytokines IL-1β and IL-18 (10). These findings align with clinical observations of increased blood titanium levels and TiO2 particle accumulation in the Peyer’ s patches of IBD patients (10). Moreover, TiO2 NPs have been implicated in the development of colitis-associated cancer (CAC). Chronic inflammatory states, such as those induced by TiO2 NPs, predispose individuals to colorectal cancer (CRC) (24, 124). By disrupting the intestinal barrier and promoting a pro-inflammatory microenvironment, TiO2 NPs may facilitate the progression from inflammation to neoplasia (24, 124). Therefore, TiO2 NPs exert profound effects on the intestinal immune system through multiple mechanisms, including disruption of the intestinal barrier, induction of pro-inflammatory cytokines, and modulation of macrophage function. These immune disturbances are accompanied by microbiota dysbiosis, further perpetuating the inflammatory state and potentially increasing the risk of chronic diseases such as IBD and CRC.
4.4.2 SiO2 NPs
The impact of SiO2 NPs on intestinal immunity is multifaceted. A study has demonstrated that SiO2 NPs can elicit a pro-inflammatory response in the gut. For instance, oral administration of SiO2 NPs to mice has been shown to increase the production of pro-inflammatory cytokines in the small intestine and colon, accompanied by histological evidence of epithelial damage and crypt loss (125). This finding suggests that SiO2 NPs may disrupt the intestinal barrier, leading to the translocation of bacteria and other harmful substances and thereby triggering an immune response. However, not all studies have reported adverse effects of SiO2 NPs on gut immunity. Other research has found no significant changes in hematological, histopathological, or biochemical properties in rats and mice orally administered SiO2 NPs of varying sizes and surface properties (126). These studies suggest that the toxicity of SiO2 NPs may be influenced by particle characteristics such as size and surface coating, as well as by the experimental conditions and animal models employed. The mechanisms underlying the immune effects of SiO2 NPs are not fully understood but likely involve interactions with the gut microbiota and immune cells. SiO2 NPs may disrupt the balance of gut microbiota, leading to dysbiosis and an altered immune response. Additionally, these nanoparticles may directly interact with immune cells, such as macrophages and dendritic cells, modulating their function and affecting the production of cytokines and other immune mediators. Therefore, the effects of SiO2 NPs on gut immunity are complex and contingent on multiple factors. While some studies have reported adverse effects, such as pro-inflammatory responses and epithelial damage, others have found no significant changes.
4.4.3 ZnO NPs
Existing research indicates that zinc oxide nanoparticles (ZnO-NPs) have a protect effects on intestinal immunity. For instance, in piglets, ZnO-NPs have been shown to enhance the expression of antioxidant enzymes and tight junction proteins, both of which are crucial for maintaining intestinal barrier integrity (109). These effects are believed to reduce stress associated with weaning in piglets by mitigating oxidative damage and improving gut barrier function (109). Furthermore, ZnO-NPs have been reported to promote proliferation and inhibit apoptosis in enterocytes, indicating a potential role in intestinal tissue repair and regeneration. These findings are supported by studies demonstrating that ZnO-NPs derived from plant extracts exhibit antioxidant and anti-inflammatory properties, suggesting their potential as therapeutic agents for mitigating gut inflammation (127). The mechanisms underlying the effects of ZnO-NPs on intestinal immunity are multifaceted. ZnO-NPs appear to modulate the production of cytokines, which are critical for regulating immune responses. For instance, ZnO-NPs have been reported to decrease the expression of pro-inflammatory cytokines in rat models of colitis, suggesting an anti-inflammatory effect (109, 128). Xia et al. found that the expression levels of pro-inflammatory cytokines IFN - γ, IL-1 β, TNF - α, and NF - κ B were reduced in the ileum after treatment with ZnO-NPs (109). Similarly, Li et al. found that ZnO-NPs can reduce the expression of pro-inflammatory cytokines IL-1 β and TNF - α in DSS treated mice, which may be related to the activation of the Nrf2 pathway (128).
4.4.4 Ag NPs
Numerous studies have investigated the interaction of Ag NPs with the gastrointestinal tract, revealing their potential to modulate both innate and adaptive immune responses. For instance, a study has shown that Ag NPs induce colitis-like symptoms characterized by increased intestinal epithelial microvilli disruption, elevated histological scores, and the production of pro-inflammatory cytokines such as TNF-α, IFN-γ, and IL-4 in the mucosa of the small intestine (97). This finding indicates that a pro-inflammatory mechanism by which Ag NPs may exacerbate intestinal inflammation and disrupt immune homeostasis (97). Conversely, another research has reported beneficial effects of Ag NPs on gastrointestinal tract health. For example, in a mouse model of DSS-induced colitis, Ag NPs significantly decreased the macroscopic score and effectively attenuated colonic damage (98). These results indicate that Ag NPs may exhibit anti-inflammatory properties under certain conditions, which could be harnessed for therapeutic purposes in the treatment of IBD. The contrasting effects of Ag NPs on gut immunity may stem from differences in particle characteristics, such as size, shape, surface coating, and aggregation state, all of which can influence biodistribution, cellular uptake, and toxicity. Additionally, gut microbiota plays a crucial role in modulating the immune response to Ag NPs, with variations in microbiota composition among individuals potentially contributing to divergent responses (111, 112). Therefore, the effects of Ag NPs on gut immunity are multifaceted and depend on various factors.
5 Current challenges and dilemmas
The Western diet, characterized by high fat, low fiber, and high sugar intake, has been implicated in the rising incidence of IBD. And Western diet is accompanied by the consumption of various food additives, which contains different IDNPs. In recent years, numerous studies have examined the role and mechanisms of IDNPs in IBD; however, a definitive conclusion has not yet been reached. The primary challenges we currently face include the following: First, due to variations in nanoparticles and animal models, conclusions drawn from different studies are often inconsistent or even contradictory. This inconsistency may arise from differences in the source, size, shape, and dosage of nanoparticles used across research institutions, as well as variations in the type, sex, age and dietary habits (including proportions of protein, lipid, and carbohydrate) of the animals. These factors influence the absorption, function, and metabolism of nanoparticles in the body, ultimately leading to deviations in research outcomes. Given that the human body is a complex biological system, with variations in diet, pre-existing conditions, medication use, and disease activity among different patients with IBD, the impact of INDPs on individuals may be more intricate. Second, previous studies in animals and humans often focus on the short-term effects of IDNPs exposure, while research on chronic toxicity resulting from long-term exposure in vivo remains limited. Since the impact of IDNPs on populations is likely to be prolonged and continuous, this issue warrants greater attention. Third, the consumption levels of IDNPs can vary significantly among individuals of differing cultural backgrounds, dietary habits, and ages; however, there is currently no reliable and accurate method for calculating intake. This presents a challenge for animal research, as imprecise dosage calculations can lead to inaccuracies when extrapolating results to human populations. Forth, considering that food packaging does not directly come into contact with the human body in practical scenarios (humans do not consume the outer packaging), there is currently no relevant research on the exposure levels and effects of IDNPs in food packaging. However, this often-overlooked issue may also impact IBD, and future research should focus on this area. Finally, a unified standard for the toxicity monitoring of IDNPs remains lacking, representing a significant gap in disease controlling. Given the widespread use of food additives, this presents a serious challenge for human health.
6 Directions for future exploration
To address the aforementioned challenges, further research is needed to investigate the pathophysiological changes associated with nanoparticles from different sources in various human environments. For patients with IBD, the intake of IDNPs should be approached with caution, taking into account individual characteristics. After resolving these issues, IDNPs could play a more significant role in the diagnosis and treatment of IBD. For example, an IBD disease activity risk model or prognosis model could be established based on varying blood concentrations of IDNPs, enabling more accurate predictions for disease diagnosis and prognosis. There should also be some clinical studies on the effects of IDNPs in food additives on the disease activity of IBD to obtain more accurate conclusions. In terms of treatment, consuming foods containing IDNPs that have therapeutic effects may benefit patients’ conditions. Additionally, modifying IDNPs and researching drug-loaded IDNPs may represent future directions for IBD treatment.
7 Conclusion
IDNPs commonly used in food additives and packaging, pose a significant yet often neglected risk for patients with IBD. This review examines their impact and mechanisms on the intestinal barrier in IBD. Previous studies have demonstrated that different types of nanoparticles exert varying effects on animals in different states. In this context, factors such as the NPs’ source, size, shape, dosage, and duration of action, as well as the animal’ s species, gender, dietary habits, and age, significantly influence the research results. Future research should explore the effects and mechanisms of diverse IDNPs on patients with IBD in greater depth.
Author contributions
DL: Data curation, Formal Analysis, Funding acquisition, Investigation, Methodology, Resources, Validation, Writing – original draft, Writing – review & editing. GL: Conceptualization, Data curation, Investigation, Methodology, Project administration, Resources, Visualization, Writing – original draft. HX: Data curation, Investigation, Methodology, Project administration, Resources, Visualization, Writing – review & editing. KL: Conceptualization, Data curation, Investigation, Methodology, Project administration, Resources, Supervision, Validation, Visualization, Writing – review & editing. ZL: Conceptualization, Funding acquisition, Investigation, Methodology, Resources, Supervision, Validation, Visualization, Writing – original draft, Writing – review & editing. CZ: Conceptualization, Data curation, Investigation, Methodology, Project administration, Resources, Supervision, Validation, Visualization, Writing – original draft, Writing – review & editing.
Funding
The author(s) declare that financial support was received for the research and/or publication of this article. This work was supported by the GuangDong Basic and Applied Basic Research Foundation (No. 2023A1515140118), the Foshan 14th-fifth high-level key specialty construction project (No. FSGSP145001), and Guangzhou Health Science and Technology Project (No. 20241A011008).
Conflict of interest
The authors declare that the research was conducted in the absence of any commercial or financial relationships that could be construed as a potential conflict of interest.
Generative AI statement
The author(s) declare that no Generative AI was used in the creation of this manuscript.
Publisher’s note
All claims expressed in this article are solely those of the authors and do not necessarily represent those of their affiliated organizations, or those of the publisher, the editors and the reviewers. Any product that may be evaluated in this article, or claim that may be made by its manufacturer, is not guaranteed or endorsed by the publisher.
References
1. Kandasamy G. Inorganic nanocarriers for siRNA delivery for cancer treatments. Biomed Mater. (2024) 19:022001. doi: 10.1088/1748-605X/ad1baf
2. Ferrisse TM, Dias LM, Oliveira A, Jordão CC, Ewerton Garcia de Oliveira M, Pavarina AC. Efficacy of antimicrobial photodynamic therapy mediated by photosensitizers conjugated with inorganic nanoparticles: systematic review and meta-analysis. Pharmaceutics. (2022) 14:2050. doi: 10.3390/pharmaceutics14102050
3. Damasco JA, Ravi S, Perez JD, Hagaman DE, Melancon MP. Understanding nanoparticle toxicity to direct a safe-by-design approach in cancer nanomedicine. Nanomater (Basel). (2020) 10:2186. doi: 10.3390/nano10112186
4. Au ALS, Mojadadi A, Shao J-Y, Ahmad G, Witting PK. Physiological benefits of novel selenium delivery via nanoparticles. Int J Mol Sci. (2023) 24:6068. doi: 10.3390/ijms24076068
5. Shen Y, Shi L, Zhang J, Zhu H, Yao Y, Liu Z, et al. Thromboelastography in patients with inflammatory bowel disease. Gastroenterol Res Pract. (2020) 2020:1–5. doi: 10.1155/2020/3245657
6. Liu F, Lee SA, Riordan SM, Zhang L, Zhu L. Global studies of using fecal biomarkers in predicting relapse in inflammatory bowel disease. Front Med. (2020) 7. doi: 10.3389/fmed.2020.580803
7. Zhang W, Zhong G, Ren X, Li M. Research progress of Ustekinumab in the treatment of inflammatory bowel disease. Front Immunol. (2024) 15:1322054. doi: 10.3389/fimmu.2024.1322054
8. Furfaro F, Ragaini E, Peyrin–Biroulet L, Danese S. Novel therapies and approaches to inflammatory bowel disease (IBD). J Clin Med. (2022) 11:4374. doi: 10.3390/jcm11154374
9. Gao Y, Li T, Duan S, Lyu L, Li Y, Xu L, et al. Impact of titanium dioxide nanoparticles on intestinal community in 2,4,6-trinitrobenzenesulfonic acid (TNBS)-induced acute colitis mice and the intervention effect of vitamin E. Nanoscale. (2021) 13:1842–62. doi: 10.1039/D0NR08106J
10. Ruiz PA, Moron B, Becker HM, Lang S, Atrott K, Spalinger MR, et al. Titanium dioxide nanoparticles exacerbate DSS-induced colitis: role of the NLRP3 inflammasome. Gut. (2017) 66:1216–24. doi: 10.1136/gutjnl-2015-310297
11. Weir A, Westerhoff P, Fabricius L, Hristovski K, von Goetz N. Titanium dioxide nanoparticles in food and personal care products. Environ Sci Technol. (2012) 46:2242–50. doi: 10.1021/es204168d
12. Winkler HC, Notter T, Meyer U, Naegeli H. Critical review of the safety assessment of titanium dioxide additives in food. J Nanobiotechnol. (2018) 16:51. doi: 10.1186/s12951-018-0376-8
13. Dudefoi W, Terrisse H, Richard-Plouet M, Gautron E, Popa F, Humbert B, et al. Criteria to define a more relevant reference sample of titanium dioxide in the context of food: a multiscale approach. Food Addit Contam Part A Chem Anal Control Expo Risk Assess. (2017) 34:653–65. doi: 10.1080/19440049.2017.1284346
14. Lomer MC, Thompson RP, Powell JJ. Fine and ultrafine particles of the diet: influence on the mucosal immune response and association with Crohn’s disease. Proc Nutr Soc. (2002) 61:123–30. doi: 10.1079/PNS2001134
15. Contado C, Mejia J, Lozano Garcia O, Piret JP, Dumortier E, Toussaint O, et al. Physicochemical and toxicological evaluation of silica nanoparticles suitable for food and consumer products collected by following the EC recommendation. Anal Bioanal Chem. (2016) 408:271–86. doi: 10.1007/s00216-015-9101-8
16. Chen J, Guo Y, Zhang X, Liu J, Gong P, Su Z, et al. Emerging nanoparticles in food: sources, application, and safety. J Agric Food Chem. (2023) 71:3564–82. doi: 10.1021/acs.jafc.2c06740
17. Minemura M, Shimizu Y. Gut microbiota and liver diseases. World J Gastroenterol. (2015) 21:1691–702. doi: 10.3748/wjg.v21.i6.1691
18. Askri D, Ouni S, Galai S, Chovelon B, Arnaud J, Sturm N, et al. Nanoparticles in foods? A multiscale physiopathological investigation of iron oxide nanoparticle effects on rats after an acute oral exposure: Trace element biodistribution and cognitive capacities. Food Chem Toxicol. (2019) 127:173–81. doi: 10.1016/j.fct.2019.03.006
19. Thomas S, Patel D, Bittel B, Wolski K, Wang Q, Kumar A, et al. Effect of high-dose zinc and ascorbic acid supplementation vs usual care on symptom length and reduction among ambulatory patients with SARS-coV-2 infection: the COVID A to Z randomized clinical trial. JAMA Netw Open. (2021) 4:e210369. doi: 10.1001/jamanetworkopen.2021.0369
20. Wang Y, Yuan L, Yao C, Ding L, Li C, Fang J, et al. A combined toxicity study of zinc oxide nanoparticles and vitamin C in food additives. Nanoscale. (2014) 6:15333–42. doi: 10.1039/C4NR05480F
21. He L, Wang H, Duan S, Gao Y, Lyu L, Ou X, et al. Characterization of titanium dioxide nanoparticles in confectionary products and estimation of dietary exposure level among the Chinese population. NanoImpact. (2022) 28:100435. doi: 10.1016/j.impact.2022.100435
22. Rompelberg C, Heringa MB, van Donkersgoed G, Drijvers J, Roos A, Westenbrink S, et al. Oral intake of added titanium dioxide and its nanofraction from food products, food supplements and toothpaste by the Dutch population. Nanotoxicology. (2016) 10:1404–14. doi: 10.1080/17435390.2016.1222457
23. Bachler G, von Goetz N, Hungerbuhler K. Using physiologically based pharmacokinetic (PBPK) modeling for dietary risk assessment of titanium dioxide (TiO2) nanoparticles. Nanotoxicology. (2015) 9:373–80. doi: 10.3109/17435390.2014.940404
24. Lamas B, Martins Breyner N, Houdeau E. Impacts of foodborne inorganic nanoparticles on the gut microbiota-immune axis: potential consequences for host health. Part Fibre Toxicol. (2020) 17:19. doi: 10.1186/s12989-020-00349-z
25. Warheit DB, Donner EM. Risk assessment strategies for nanoscale and fine-sized titanium dioxide particles: Recognizing hazard and exposure issues. Food Chem Toxicol. (2015) 85:138–47. doi: 10.1016/j.fct.2015.07.001
26. Geraets L, Oomen AG, Krystek P, Jacobsen NR, Wallin H, Laurentie M, et al. Tissue distribution and elimination after oral and intravenous administration of different titanium dioxide nanoparticles in rats. Part Fibre Toxicol. (2014) 11:30. doi: 10.1186/1743-8977-11-30
27. Jones K, Morton J, Smith I, Jurkschat K, Harding AH, Evans G. Human in vivo and in vitro studies on gastrointestinal absorption of titanium dioxide nanoparticles. Toxicol Lett. (2015) 233:95–101. doi: 10.1016/j.toxlet.2014.12.005
28. Bockmann J, Lahl H, Eckert T, Unterhalt B. Blood titanium levels before and after oral administration titanium dioxide. Pharmazie. (2000) 55:140–3.
29. Heringa MB, Peters RJB, Bleys R, van der Lee MK, Tromp PC, van Kesteren PCE, et al. Detection of titanium particles in human liver and spleen and possible health implications. Part Fibre Toxicol. (2018) 15:15. doi: 10.1186/s12989-018-0251-7
30. Talamini L, Gimondi S, Violatto MB, Fiordaliso F, Pedica F, Tran NL, et al. Repeated administration of the food additive E171 to mice results in accumulation in intestine and liver and promotes an inflammatory status. Nanotoxicology. (2019) 13:1087–101. doi: 10.1080/17435390.2019.1640910
31. Lin Z, Monteiro-Riviere NA, Riviere JE. Pharmacokinetics of metallic nanoparticles. Wiley Interdiscip Rev Nanomed Nanobiotech. (2015) 7:189–217. doi: 10.1002/wnan.2015.7.issue-2
32. Brun E, Barreau F, Veronesi G, Fayard B, Sorieul S, Chaneac C, et al. Titanium dioxide nanoparticle impact and translocation through ex vivo, in vivo and in vitro gut epithelia. Part Fibre Toxicol. (2014) 11:13. doi: 10.1186/1743-8977-11-13
33. Zeman T, Loh EW, Cierny D, Sery O. Penetration, distribution and brain toxicity of titanium nanoparticles in rodents’ body: a review. IET Nanobiotech. (2018) 12:695–700. doi: 10.1049/iet-nbt.2017.0109
34. Campanale C, Massarelli C, Savino I, Locaputo V, Uricchio VF. A detailed review study on potential effects of microplastics and additives of concern on human health. Int J Environ Res Public Health. (2020) 17:1212. doi: 10.3390/ijerph17041212
35. Wright SL, Kelly FJ. Plastic and human health: A micro issue? Environ Sci Technol. (2017) 51:6634–47. doi: 10.1021/acs.est.7b00423
36. McClements DJ, DeLoid G, Pyrgiotakis G, Shatkin JA, Xiao H, Demokritou P. The Role of the Food Matrix and Gastrointestinal Tract in the assessment of biological properties of ingested engineered nanomaterials (iENMs): State of the science and knowledge gaps. NanoImpact. (2016) 3-4:47–57. doi: 10.1016/j.impact.2016.10.002
37. Zhou P, Guo M, Cui X. Effect of food on orally-ingested titanium dioxide and zinc oxide nanoparticle behaviors in simulated digestive tract. Chemosphere. (2021) 268:128843. doi: 10.1016/j.chemosphere.2020.128843
38. Zhong Q, Zhang L. Nanoparticles fabricated from bulk solid lipids: Preparation, properties, and potential food applications. Adv Colloid Interface Sci. (2019) 273:102033. doi: 10.1016/j.cis.2019.102033
39. Dehkordi NH, Tajik H, Moradi M, Kousheh SA, Molaei R. Antibacterial interactions of colloid nanosilver with eugenol and food ingredients. J Food Prot. (2019) 82:1783–92. doi: 10.4315/0362-028X.JFP-19-174
40. Lee JA, Kim MK, Song JH, Jo MR, Yu J, Kim KM, et al. Biokinetics of food additive silica nanoparticles and their interactions with food components. Colloids Surf B Biointerf. (2017) 150:384–92. doi: 10.1016/j.colsurfb.2016.11.001
41. Zhou H, Dai T, Liu J, Tan Y, Bai L, Rojas OJ, et al. Chitin nanocrystals reduce lipid digestion and β-carotene bioaccessibility: An in-vitro INFOGEST gastrointestinal study. Food Hydrocolloids. (2021) 113:106494. doi: 10.1016/j.foodhyd.2020.106494
42. Nayak PS, Borah SM, Gogoi H, Asthana S, Bhatnagar R, Jha AN, et al. Lactoferrin adsorption onto silver nanoparticle interface: Implications of corona on protein conformation, nanoparticle cytotoxicity and the formulation adjuvanticity. Chem Eng J. (2019) 361:470–84. doi: 10.1016/j.cej.2018.12.084
43. Bing J, Xiao X, McClements DJ, Biao Y, Chongjiang C. Protein corona formation around inorganic nanoparticles: Food plant proteins-TiO2 nanoparticle interactions. Food Hydrocolloids. (2021) 115:106594. doi: 10.1016/j.foodhyd.2021.106594
44. Abarca-Cabrera L, Fraga-Garcia P, Berensmeier S. Bio-nano interactions: binding proteins, polysaccharides, lipids and nucleic acids onto magnetic nanoparticles. Biomater Res. (2021) 25:12. doi: 10.1186/s40824-021-00212-y
45. Franco-Ulloa S, Tatulli G, Bore SL, Moglianetti M, Pompa PP, Cascella M, et al. Dispersion state phase diagram of citrate-coated metallic nanoparticles in saline solutions. Nat Commun. (2020) 11:5422. doi: 10.1038/s41467-020-19164-3
46. Di Silvio D, Rigby N, Bajka B, Mackie A, Baldelli Bombelli F. Effect of protein corona magnetite nanoparticles derived from bread in vitro digestion on Caco-2 cells morphology and uptake. Int J Biochem Cell Biol. (2016) 75:212–22. doi: 10.1016/j.biocel.2015.10.019
47. Phue WH, Liu M, Xu K, Srinivasan D, Ismail A, George S. A comparative analysis of different grades of silica particles and temperature variants of food-grade silica nanoparticles for their physicochemical properties and effect on trypsin. J Agric Food Chem. (2019) 67:12264–72. doi: 10.1021/acs.jafc.9b03638
48. Ault AP, Stark DI, Axson JL, Keeney JN, Maynard AD, Bergin IL, et al. Protein corona-induced modification of silver nanoparticle aggregation in simulated gastric fluid. Environ Sci Nano. (2016) 3:1510–20. doi: 10.1039/C6EN00278A
49. Zhang Y, Li Y. Inflammatory bowel disease: pathogenesis. World J Gastroenterol. (2014) 20:91. doi: 10.3748/wjg.v20.i1.91
50. Salim SY, Soderholm JD. Importance of disrupted intestinal barrier in inflammatory bowel diseases. Inflammation Bowel Dis. (2011) 17:362–81. doi: 10.1002/ibd.21403
51. Scalavino V, Piccinno E, Bianco G, Schena N, Armentano R, Giannelli G, et al. The increase of miR-195-5p reduces intestinal permeability in ulcerative colitis, modulating tight junctions’ Expression. Int J Mol Sci. (2022) 23:5840. doi: 10.3390/ijms23105840
52. Zhao H, Zhou Y, Xu J, Zhang Y, Wang H, Zhao C, et al. Short-chain fatty acid-producing bacterial strains attenuate experimental ulcerative colitis by promoting M2 macrophage polarization via JAK/STAT3/FOXO3 axis inactivation. J Transl Med. (2024) 22:369. doi: 10.1186/s12967-024-05122-w
53. Newberry RD, Hogan SP. Intestinal epithelial cells in tolerance and allergy to dietary antigens. J Allergy Clin Immunol. (2021) 147:45–8. doi: 10.1016/j.jaci.2020.10.030
54. Patankar JV, Becker C. Cell death in the gut epithelium and implications for chronic inflammation. Nat Rev Gastroenterol Hepatol. (2020) 17:543–56. doi: 10.1038/s41575-020-0326-4
55. Garcia-Carbonell R, Wong J, Kim JY, Close LA, Boland BS, Wong TL, et al. Elevated A20 promotes TNF-induced and RIPK1-dependent intestinal epithelial cell death. Proc Natl Acad Sci U S A. (2018) 115:E9192–E200. doi: 10.1073/pnas.1810584115
56. Adolph TE, Tomczak M, Niederreiter L, Ko HJ, Böck J, Martínez-Naves E, et al. Paneth cells as a site of origin for intestinal inflammation. Nature. (2013) 503:272–6. doi: 10.1038/nature12599
57. Vereecke L, Vieira-Silva S, Billiet T, Es J, Guire CM, Slowicka K, et al. A20 controls intestinal homeostasis through cell-specific activities. Nat Commun. (2014) 5:5103. doi: 10.1038/ncomms6103
58. Jozawa H, Inoue-Yamauchi A, Arimura S, Yamanashi Y. Loss of C/EBPδ Enhances apoptosis of intestinal epithelial cells and exacerbates experimental colitis in mice. Genes to Cells. (2019) 24:619–26. doi: 10.1111/gtc.12711
59. Pang L, Huynh J, Alorro M, Li X, Ernst M, Chand AL. STAT3 signalling via the IL-6ST/gp130 cytokine receptor promotes epithelial integrity and intestinal barrier function during DSS-induced colitis. Biomedicines. (2021) 9:187. doi: 10.3390/biomedicines9020187
60. Zhu L, Han J, Li L, Wang Y, Liu Y, Zhang S. Claudin family participates in the pathogenesis of inflammatory bowel diseases and colitis-associated colorectal cancer. Front Immunol. (2019) 10. doi: 10.3389/fimmu.2019.01441
61. Zeißig S, Bürgel N, Günzel D, Richter JF, Mankertz J, Wahnschaffe U, et al. Changes in expression and distribution of claudin 2, 5 and 8 lead to discontinuous tight junctions and barrier dysfunction in active Crohn’s disease. Gut. (2007) 56:61–72. doi: 10.1136/gut.2006.094375
62. Amasheh S, Milatz S, Krug SM, Markov AG, Günzel D, Amasheh M, et al. Tight junction proteins as channel formers and barrier builders. Ann New York Acad Sci. (2009) 1165:211–9. doi: 10.1111/j.1749-6632.2009.04439.x
63. Oshima T, Miwa H, Joh T. Changes in the expression of claudins in active ulcerative colitis. J Gastroenterol Hepatol. (2008) 23:S146–150. doi: 10.1111/j.1440-1746.2008.05405.x
64. Liu G-H, Liu H-M, Chen Y-S, Lee T-Y. Effect of electroacupuncture in mice with dextran sulfate sodium-induced colitis and the influence of gut microbiota. Evidence-Based Complement Altern Med. (2020) 2020:2087903. doi: 10.1155/2020/2087903
65. Kim MW, Choi S, Kim SY, Yoon YS, Kang JH, Oh SH. Allyl isothiocyanate ameliorates dextran sodium sulfate-induced colitis in mouse by enhancing tight junction and mucin expression. Int J Mol Sci. (2018) 19:2025. doi: 10.3390/ijms19072025
66. Woo JK, Choi S, Kang JH, Kim DE, Hurh B-S, Jeon JY, et al. Fermented barley and soybean (BS) mixture enhances intestinal barrier function in dextran sulfate sodium (DSS)-induced colitis mouse model. BMC Complement Altern Med. (2016) 16:498. doi: 10.1186/s12906-016-1479-0
67. Salimi A. The anti-inflammatory effect of a probiotic cocktail in human feces induced-mouse model. Inflammation. (2023) 46:2178–92. doi: 10.1007/s10753-023-01870-x
68. Su L, Shen L, Clayburgh D, Nalle SC, Sullivan EA, Meddings J, et al. Targeted epithelial tight junction dysfunction causes immune activation and contributes to development of experimental colitis. Gastroenterology. (2009) 136:551–63. doi: 10.1053/j.gastro.2008.10.081
69. Alves JLB, Costa P, Sales LCS, Silva Luis CC, Bezerra TPT, Souza MLA, et al. Shedding light on the impacts of Spirulina platensis on gut microbiota and related health benefits. Crit Rev Food Sci Nutr. (2024) 2024:1–14. doi: 10.1080/10408398.2024.2323112
70. Krishna V, Kumar N, Banerjee S. Gut microbiota and inflammatory disorders. Curr Drug Targets. (2022) 23:156–69. doi: 10.2174/1389450122666210623125603
71. De-Sales-Millan A, Aguirre-Garrido JF, Gonzalez-Cervantes RM, Velazquez-Aragon JA. Microbiome-gut-mucosal-immune-brain axis and autism spectrum disorder (ASD): A novel proposal of the role of the gut microbiome in ASD aetiology. Behav Sci (Basel). (2023) 13:548. doi: 10.3390/bs13070548
72. Bajaj A, Markandey M, Kedia S, Ahuja V. Gut bacteriome in inflammatory bowel disease: An update on recent advances. Indian J Gastroenterol. (2024) 43:103–11. doi: 10.1007/s12664-024-01541-1
73. Lal S, Kandiyal B, Ahuja V, Takeda K, Das B. Gut microbiome dysbiosis in inflammatory bowel disease. Prog Mol Biol Transl Sci. (2022) 192:179–204. doi: 10.1016/bs.pmbts.2022.09.003
74. Upadhyay KG, Desai DC, Ashavaid TF, Dherai AJ. Microbiome and metabolome in inflammatory bowel disease. J Gastroenterol Hepatol. (2023) 38:34–43. doi: 10.1111/jgh.16043
75. Dong LN, Wang M, Guo J, Wang JP. Role of intestinal microbiota and metabolites in inflammatory bowel disease. Chin Med J (Engl). (2019) 132:1610–4. doi: 10.1097/CM9.0000000000000290
76. Lavelle A, Sokol H. Gut microbiota-derived metabolites as key actors in inflammatory bowel disease. Nat Rev Gastroenterol Hepatol. (2020) 17:223–37. doi: 10.1038/s41575-019-0258-z
77. Scott SA, Fu J, Chang PV. Microbial tryptophan metabolites regulate gut barrier function via the aryl hydrocarbon receptor. Proc Natl Acad Sci U S A. (2020) 117:19376–87. doi: 10.1073/pnas.2000047117
78. Wang C, Gu Y, Chu Q, Wang X, Ding Y, Qin X, et al. Gut microbiota and metabolites as predictors of biologics response in inflammatory bowel disease: A comprehensive systematic review. Microbiol Res. (2024) 282:127660. doi: 10.1016/j.micres.2024.127660
79. Wlodarska M, Luo C, Kolde R, d’Hennezel E, Annand JW, Heim CE, et al. Indoleacrylic acid produced by commensal peptostreptococcus species suppresses inflammation. Cell Host Microbe. (2017) 22:25–37.e6. doi: 10.1016/j.chom.2017.06.007
80. Chen T, Wang R, Duan Z, Yuan X, Ding Y, Feng Z, et al. Akkermansia muciniphila protects against psychological disorder-induced gut microbiota-mediated colonic mucosal barrier damage and aggravation of colitis. Front Cell Infect Microbiol. (2021) 11. doi: 10.3389/fcimb.2021.723856
81. Chi H, Wang D, Chen M, Lin J, Zhang S, Yu F, et al. Shaoyao decoction inhibits inflammation and improves intestinal barrier function in mice with dextran sulfate sodium-induced colitis. Front Pharmacol. (2021) 12. doi: 10.3389/fphar.2021.524287
82. Placet M, Molle CM, Arguin G, Geha S, Gendron FP. The expression of P2Y6 receptor promotes the quality of mucus in colitic mice. FEBS J. (2021) 288:5459–73. doi: 10.1111/febs.v288.18
83. Schwerbrock NM, Makkink MK, Sluis M, Büller HA, Einerhand AWC, Sartor RB, et al. Interleukin 10-deficient mice exhibit defective colonic muc2 synthesis before and after induction of colitis by commensal bacteria. Inflamm Bowel Dis. (2004) 10:811–23. doi: 10.1097/00054725-200411000-00016
84. Leoncini G. Mucin expression profiles in ulcerative colitis: new insights on the histological mucosal healing. Int J Mol Sci. (2024) 25:1858. doi: 10.3390/ijms25031858
85. Fyderek K, Strus M, Kowalska-Duplaga K, Gosiewski T, Wedrychowicz A, Jedynak-Wasowicz U, et al. Mucosal bacterial microflora and mucus layer thickness in adolescents with inflammatory bowel disease. World J Gastroenterol. (2009) 15:5287–94. doi: 10.3748/wjg.15.5287
86. Lock JY, Carlson TL, Wang CM, Chen A, Carrier RL. Acute exposure to commonly ingested emulsifiers alters intestinal mucus structure and transport properties. Sci Rep. (2018) 8:10008. doi: 10.1038/s41598-018-27957-2
87. Dorofeyev AE, Василенко ИВ, Rassokhina OA, Kondratiuk RB. Mucosal barrier in ulcerative colitis and Crohn’s disease. Gastroenterol Res Pract. (2013) 2013:1–9. doi: 10.1155/2013/431231
88. Peng K, Shao B-Z, Xu Z, Chen X, Liu C. Intestinal autophagy and its pharmacological control in inflammatory bowel disease. Front Immunol. (2017) 7. doi: 10.3389/fimmu.2016.00695
89. An J, Liu Y, Wang Y, Fan R, Hu X, Zhang F, et al. The role of intestinal mucosal barrier in autoimmune disease: A potential target. Front Immunol. (2022) 13:871713. doi: 10.3389/fimmu.2022.871713
90. Ren Z, Guo C, Yu S, Zhu L, Wang Y, Hu H, et al. Progress in mycotoxins affecting intestinal mucosal barrier function. Int J Mol Sci. (2019) 20:2777. doi: 10.3390/ijms20112777
91. Chen S, He R, He B, Xu L, Zhang S. Potential roles of exosomal lncRNAs in the intestinal mucosal immune barrier. J Immunol Res. (2021) 2021:7183136. doi: 10.1155/2021/7183136
92. Frigerio S, Lartey DA, D’Haens GR, Grootjans J. The role of the immune system in IBD-associated colorectal cancer: from pro to anti-tumorigenic mechanisms. Int J Mol Sci. (2021) 22:12739. doi: 10.3390/ijms222312739
93. Chen Y, Cui W, Li X, Yang H. Interaction between commensal bacteria, immune response and the intestinal barrier in inflammatory bowel disease. Front Immunol. (2021) 12:761981. doi: 10.3389/fimmu.2021.761981
94. Negi S, Saini S, Tandel N, Sahu K, Mishra RPN, Tyagi RK. Translating treg therapy for inflammatory bowel disease in humanized mice. Cells. (2021) 10:1847. doi: 10.3390/cells10081847
95. Lu Q, Yang MF, Liang YJ, Xu J, Xu HM, Nie YQ, et al. Immunology of inflammatory bowel disease: molecular mechanisms and therapeutics. J Inflammation Res. (2022) 15:1825–44. doi: 10.2147/JIR.S353038
96. Kureshi CT, Dougan SK. Cytokines in cancer. Cancer Cell. (2024) 43:15–35. doi: 10.1016/j.ccell.2024.11.011
97. Chen H, Zhao R, Wang B, Cai C, Zheng L, Wang H, et al. The effects of orally administered Ag, TiO2 and SiO2 nanoparticles on gut microbiota composition and colitis induction in mice. NanoImpact. (2017) 8:80–8. doi: 10.1016/j.impact.2017.07.005
98. Siczek K, Zatorski H, Chmielowiec-Korzeniowska A, Pulit-Prociak J, Smiech M, Kordek R, et al. Synthesis and evaluation of anti-inflammatory properties of silver nanoparticle suspensions in experimental colitis in mice. Chem Biol Drug Des. (2017) 89:538–47. doi: 10.1111/cbdd.12876
99. Yan J, Chen Q, Tian L, Li K, Lai W, Bian L, et al. Intestinal toxicity of micro- and nano-particles of foodborne titanium dioxide in juvenile mice: Disorders of gut microbiota-host co-metabolites and intestinal barrier damage. Sci Total Environ. (2022) 15:821:153279. doi: 10.1016/j.scitotenv.2022.153279
100. Cornu R, Chretien C, Pellequer Y, Martin H, Beduneau A. Small silica nanoparticles transiently modulate the intestinal permeability by actin cytoskeleton disruption in both Caco-2 and Caco-2/HT29-MTX models. Arch Toxicol. (2020) 94:1191–202. doi: 10.1007/s00204-020-02694-6
101. Orr SE, Gokulan K, Boudreau M, Cerniglia CE, Khare S. Alteration in the mRNA expression of genes associated with gastrointestinal permeability and ileal TNF-alpha secretion due to the exposure of silver nanoparticles in Sprague-Dawley rats. J Nanobiotechnol. (2019) 17:63. doi: 10.1186/s12951-019-0499-6
102. Li Y, Pan M, Meng S, Xu W, Wang S, Dou M, et al. The effects of zinc oxide nanoparticles on antioxidation, inflammation, tight junction integrity, and apoptosis in heat-stressed bovine intestinal epithelial cells in vitro. Biol Trace Elem Res. (2024) 202:2042–51. doi: 10.1007/s12011-023-03826-6
103. Carrillo-Romero J, Mentxaka G, Garcia-Salvador A, Katsumiti A, Carregal-Romero S, Goni-de-Cerio F. Assessing the toxicity of metal- and carbon-based nanomaterials in vitro: impact on respiratory, intestinal, skin, and immune cell lines. Int J Mol Sci. (2024) 25:10910. doi: 10.3390/ijms252010910
104. Gillois K, Stoffels C, Leveque M, Fourquaux I, Blesson J, Mils V, et al. Repeated exposure of Caco-2 versus Caco-2/HT29-MTX intestinal cell models to (nano)silver in vitro: Comparison of two commercially available colloidal silver products. Sci Total Environ. (2021) 754:142324. doi: 10.1016/j.scitotenv.2020.142324
105. Dudefoi W, Moniz K, Allen-Vercoe E, Ropers M-H, Walker VK. Impact of food grade and nano-TiO2 particles on a human intestinal community. Food Chem Toxicol. (2017) 106:242–9. doi: 10.1016/j.fct.2017.05.050
106. Taylor AA, Marcus IM, Guysi RL, Walker SL. Metal oxide nanoparticles induce minimal phenotypic changes in a model colon gut microbiota. Environ Eng Sci. (2015) 32:602–12. doi: 10.1089/ees.2014.0518
107. Li J, Yang S, Lei R, Gu W, Qin Y, Ma S, et al. Oral administration of rutile and anatase TiO(2) nanoparticles shifts mouse gut microbiota structure. Nanoscale. (2018) 10:7736–45. doi: 10.1039/C8NR00386F
108. Bredeck G, Kampfer AAM, Sofranko A, Wahle T, Lison D, Ambroise J, et al. Effects of dietary exposure to the engineered nanomaterials CeO(2), SiO(2), Ag, and TiO(2) on the murine gut microbiome. Nanotoxicology. (2021) 15:934–50. doi: 10.1080/17435390.2021.1940339
109. Xia T, Lai W, Han M, Han M, Ma X, Zhang L. Dietary ZnO nanoparticles alters intestinal microbiota and inflammation response in weaned piglets. Oncotarget. (2017) 8:64878–91. doi: 10.18632/oncotarget.17612
110. Feng Y, Min L, Zhang W, Liu J, Hou Z, Chu M, et al. Zinc oxide nanoparticles influence microflora in ileal digesta and correlate well with blood metabolites. Front Microbiol. (2017) 8:992. doi: 10.3389/fmicb.2017.00992
111. Hadrup N, Loeschner K, Bergstrom A, Wilcks A, Gao X, Vogel U, et al. Subacute oral toxicity investigation of nanoparticulate and ionic silver in rats. Arch Toxicol. (2012) 86:543–51. doi: 10.1007/s00204-011-0759-1
112. Wilding LA, Bassis CM, Walacavage K, Hashway S, Leroueil PR, Morishita M, et al. Repeated dose (28-day) administration of silver nanoparticles of varied size and coating does not significantly alter the indigenous murine gut microbiome. Nanotoxicology. (2016) 10:513–20. doi: 10.3109/17435390.2015.1078854
113. Williams K, Milner J, Boudreau MD, Gokulan K, Cerniglia CE, Khare S. Effects of subchronic exposure of silver nanoparticles on intestinal microbiota and gut-associated immune responses in the ileum of Sprague-Dawley rats. Nanotoxicology. (2015) 9:279–89. doi: 10.3109/17435390.2014.921346
114. Javurek AB, Suresh D, Spollen WG, Hart ML, Hansen SA, Ellersieck MR, et al. Gut dysbiosis and neurobehavioral alterations in rats exposed to silver nanoparticles. Sci Rep. (2017) 7:2822. doi: 10.1038/s41598-017-02880-0
115. Das P, McDonald J, Petrof E, Allen-Vercoe E, Walker V. Nanosilver-mediated change in human intestinal microbiota. J Nanomed Nanotechnol. (2014) 5:5. doi: 10.4172/2157-7439.1000235
116. van den Brule S, Ambroise J, Lecloux H, Levard C, Soulas R, De Temmerman PJ, et al. Dietary silver nanoparticles can disturb the gut microbiota in mice. Part Fibre Toxicol. (2016) 13:38. doi: 10.1186/s12989-016-0149-1
117. Limage R, Tako E, Kolba N, Guo Z, Garcia-Rodriguez A, Marques CNH, et al. TiO(2) nanoparticles and commensal bacteria alter mucus layer thickness and composition in a gastrointestinal tract model. Small. (2020) 16:e2000601. doi: 10.1002/smll.202000601
118. Jeong GN, Jo UB, Ryu HY, Kim YS, Song KS, Yu IJ. Histochemical study of intestinal mucins after administration of silver nanoparticles in Sprague–Dawley rats. Arch Toxicol. (2010) 84:63–9. doi: 10.1007/s00204-009-0469-0
119. Maares M, Keil C, Straubing S, Robbe-Masselot C, Haase H. Zinc deficiency disturbs mucin expression, O-glycosylation and secretion by intestinal goblet cells. Int J Mol Sci. (2020) 21:6149. doi: 10.3390/ijms21176149
120. Nogueira CM, de Azevedo WM, Dagli ML, Toma SH, Leite AZ, Lordello ML, et al. Titanium dioxide induced inflammation in the small intestine. World J Gastroenterol. (2012) 18:4729–35. doi: 10.3748/wjg.v18.i34.4729
121. Yan J, Wang D, Li K, Chen Q, Lai W, Tian L, et al. Toxic effects of the food additives titanium dioxide and silica on the murine intestinal tract: Mechanisms related to intestinal barrier dysfunction involved by gut microbiota. Environ Toxicol Pharmacol. (2020) 80:103485. doi: 10.1016/j.etap.2020.103485
122. Yao L, Tang Y, Chen B, Hong W, Xu X, Liu Y, et al. Oral exposure of titanium oxide nanoparticles induce ileum physical barrier dysfunction via Th1/Th2 imbalance. Environ Toxicol. (2020) 35:982–90. doi: 10.1002/tox.22934
123. Huang C, Sun M, Yang Y, Wang F, Ma X, Li J, et al. Titanium dioxide nanoparticles prime a specific activation state of macrophages. Nanotoxicology. (2017) 11:737–50. doi: 10.1080/17435390.2017.1349202
124. Abraham BP. Cancer surveillance in ulcerative colitis and Crohn’s disease: new strategies. Curr Opin Gastroenterol. (2016) 32:32–7. doi: 10.1097/MOG.0000000000000234
125. van der Zande M, Vandebriel RJ, Groot MJ, Kramer E, Herrera Rivera ZE, Rasmussen K, et al. Sub-chronic toxicity study in rats orally exposed to nanostructured silica. Part Fibre Toxicol. (2014) 11:8. doi: 10.1186/1743-8977-11-8
126. Yoshida T, Yoshioka Y, Takahashi H, Misato K, Mori T, Hirai T, et al. Intestinal absorption and biological effects of orally administered amorphous silica particles. Nanoscale Res Lett. (2014) 9:532. doi: 10.1186/1556-276X-9-532
127. Nagajyothi PC, Cha SJ, Yang IJ, Sreekanth TV, Kim KJ, Shin HM. Antioxidant and anti-inflammatory activities of zinc oxide nanoparticles synthesized using Polygala tenuifolia root extract. J Photochem Photobiol B. (2015) 146:10–7. doi: 10.1016/j.jphotobiol.2015.02.008
Keywords: inorganic dietary nanoparticles, inflammatory bowel disease, intestinal barrier, immune barrier, biological barrier
Citation: Luo D, Luo G, Xu H, Li K, Li Z and Zhang C (2025) Inorganic dietary nanoparticles in intestinal barrier function of inflammatory bowel disease: allies or adversaries? Front. Immunol. 16:1563504. doi: 10.3389/fimmu.2025.1563504
Received: 20 January 2025; Accepted: 24 March 2025;
Published: 09 April 2025.
Edited by:
Angela Rizzi, Agostino Gemelli University Polyclinic (IRCCS), ItalyReviewed by:
Franco Scaldaferri, Catholic University of the Sacred Heart, ItalyLeopold Green, Purdue University, United States
Copyright © 2025 Luo, Luo, Xu, Li, Li and Zhang. This is an open-access article distributed under the terms of the Creative Commons Attribution License (CC BY). The use, distribution or reproduction in other forums is permitted, provided the original author(s) and the copyright owner(s) are credited and that the original publication in this journal is cited, in accordance with accepted academic practice. No use, distribution or reproduction is permitted which does not comply with these terms.
*Correspondence: Cong Zhang, emhhbmdjNTdAbWFpbDIuc3lzdS5lZHUuY24=; Zhaotao Li, MTM4Mjc3NzQ4MzNAMTM5LmNvbQ==; Kangbao Li, Y2FuYmxlbGVlQDE2My5jb20=
†These authors have contributed equally to this work