- Department of Surgery, University of Maryland, Baltimore, MA, United States
Lung transplantation (LTx) offers a last resort for patients battling end-stage lung disease. Even though short-term survival has improved, these patients still face several long-term challenges, such as chronic rejection and ischemic bronchial anastomosis. In lung transplant recipients, the bronchial anastomosis is prone to complications—such as poor wound healing, necrosis, stenosis, and dehiscence—due to the marginal blood supply at this site. During peri-LTx, hypoxia and ischemia stimulate fibrotic and inflammatory cytokines at anastomotic sites, leading to abnormal collagen production and excessive granulation, which impair wound healing. Despite meticulous techniques, bronchial anastomosis remains a major cause of morbidity and mortality among lung transplant recipients. After LTx, most bronchial complications are attributed to ischemic insult since normal bronchial blood flow is disrupted, and bronchial revascularization usually takes two to four weeks, making the anastomotic bronchial vessels dependent on pulmonary artery circulation. It is clear that hypoxia, inflammation, oxidative stress, and extracellular matrix remodeling play critical roles in bronchial complications, but there is no small animal model to study them. In the context of LTx, mouse tracheal models are essential tools for studying bronchial complications, particularly ischemia, fibrosis, and stenosis, as well as evaluating potential therapeutic interventions. A well-established mouse model of orthotopic tracheal transplantation (OTT) mimics the anastomosis of the bronchi and the subsequent microvascular injury, providing a pathological correlation with anastomotic complications. A series of previous studies using the OTT model explored the microvascularization, ischemia-reperfusion, airway epithelial injury, and fibrotic remodeling effects after airway anastomosis. This review describes OTT as a model of airway anastomotic complications, which is crucial for understanding the immunological and molecular pathways as seen in clinical bronchial anastomoses, as well as improving anastomotic healing and reducing complications through targeted therapeutic strategies.
Introduction
Lung graft rejection commonly presents as acute cellular rejection with symptoms like shortness of breath, cough, fever, or asymptomatic pulmonary functions test (PFT) decline; chronic rejection such as bronchiolitis obliterans syndrome (BOS) leads to irreversible airflow obstruction and progressive respiratory decline; and antibody-mediated rejection involves donor-specific antibodies, capillaritis, and respiratory distress—all contributing to increased infection risk, reduced graft function, and complications from immunosuppression (1). Bronchial anastomotic complications after lung transplantation (LTx), can significantly impact graft function and patient outcomes, necessitating prompt diagnosis and intervention (2). The bronchial anastomosis has been clinically recognized as the most vulnerable site for operative complications and is defined as a lack of vascular perfusion, poor wound healing, stenosis, necrosis, and dehiscence of the bronchus of the donor lung and recipient (3–10). At bronchial anastomotic sites, hypoxia and ischemia stimulate profibrotic and inflammatory cytokines, impairing wound healing through abnormal collagen production and excessive granulation. During anastomosis, the recipient’s main bronchi are supplied by native bronchial arteries, while the donor’s bronchi are supplied by collateral circulation, and the revascularization of the donor by the recipient may take up to four weeks, which may leave the airways vulnerable to ischemic damage (9). Bronchial anastomotic complications after LTx can be caused by a number of factors, including bronchial ischemia, infection, immunosuppression, surgical technique, donor and recipient factors and organ preservation (11). It is believed that ischemic insults are the most common cause of airway complications because normal blood flow supply is interrupted, leaving the bronchial vessels at the anastomotic site dependent on arterial circulation following lung transplantation (9, 12). As a result of hypoxia and ischemia, major inflammatory and profibrotic cytokines are released, causing an imbalance in collagen synthesis, degradation, and excessive proliferation of granulation tissue (6, 13–15). Bronchial anastomotic complications are a major complication that can contribute to morbidity and mortality, and occur within the first year of transplantation (16). The incidence of lethal and nonlethal airway complications has decreased since the early experiences; however, it results in considerable morbidity, decreased quality of life, increased cost, and account for a mortality of 2% - 4% (3, 3, 16–20). Bronchial anastomotic complications can be treated with a multispecialty team approach, including medical management and interventional bronchoscopic procedures (21). A variety of surgical techniques have been used to overcome complications associated with bronchial anastomoses (22, 23). Despite advances in lung transplantation, airway complications after LTx remain unchanged in terms of their risk factors, incidence, and lethality. Complications related to airway grafts, an infected environment, and prolonged mechanical ventilation remain obstacles (4, 19, 24, 25). Although only a limited number of therapeutic options have been used to promote bronchial anastomotic healing, especially platelet rich plasma or platelet-derived growth factor (PDGF) therapy, their effectiveness has been limited (26, 27). The occurrence of bronchial anastomotic complications is associated with a number of factors, including primary graft dysfunction, acute rejection (AR), preoperative and postoperative pulmonary infections, prolonged mechanical ventilation, single lung transplantation, and Aspergillus fumigatus colonization (28). AR is associated with severe inflammation through perivascular, subendothelial and mononuclear infiltrates, submucosal edema, reduced graft perfusion, and poor wound healing, which are crucial risk factors for airway complications (4, 12, 19, 20, 22, 27, 29). The delay or reversal of AR with immunotherapy is highly dependent on the presence of a functional microcirculation in the graft (30). Therefore, therapies that specifically preserve the microcirculation could prevent allograft rejection and potentially subsequent fibrotic remodeling. Studies have shown that alloimmune inflammation adversely affects the microvascular connections between recipients and donors of rejecting allografts compared with non-rejecting syngrafts (31–34) (Table 1; Figure 1).
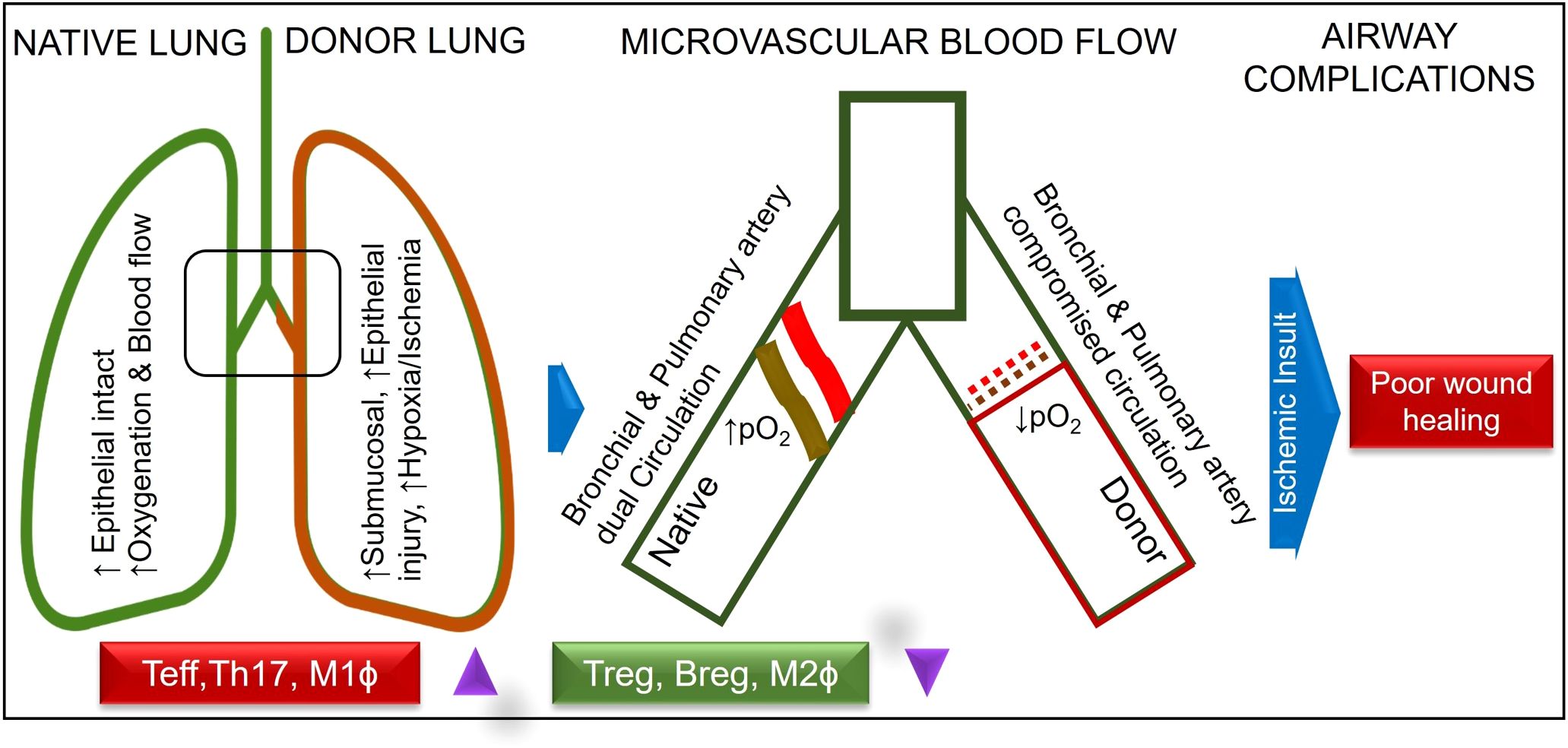
Figure 1. Normal and compromised dual circulation in native and donor lung post lung-transplantation.
Bronchial anastomosis can be replicated in small animals as a microvascular disruption between donor to recipient airway grafts
A severe hypoxic state and impaired blood flow due to massive intragraft infiltration of inflammatory cells are believed to be the primary causes of poor wound healing and associated complications in the bronchus after LTx (5, 10, 32, 35–38). Orthotopic tracheal transplantation (OTT) in mice is a well-established model that mimics bronchial anastomosis and subsequent microvascular injury post-tracheal transplantation (39). It has been demonstrated in OTT model that microvascular supplies between donors and recipients begin at day (d) 4, remain connected until day 8, and start disappearing by day 10 of tracheal transplantation in rejecting allografts, which coincides with a decline in tissue oxygenation and microvascular blood flow as compared to the non-rejecting syngrafts that are constantly oxygenated (31, 32). Additionally, pathological examinations revealed that rejecting allografts showed denuded graft epithelium during acute rejection followed by this massive deposition of subepithelial collagen and airway narrowing post-transplantation. There has been clinical evidence that adequate microvascular supply and oxygen saturation in the airway tissue are vital for lung transplantation, which was later validated in preclinical OTT studies (5, 6, 39, 40). The involvement of transforming growth factor-beta (TGF-β) and inflammatory cytokines such as interleukin-6 (IL-6) and tumor necrosis factor-alpha (TNF-α) mirrors the molecular changes seen in human patients (41). In addition, a variety of animal and in-vitro models have been developed for examining lung transplant complications, though each has some limitations (42). In particular, mouse trachea transplant models are widely used to study airway stenosis, replicating the pathological conditions observed in human patients with post-surgical airway narrowing, fibrosis, and inflammation (39, 42, 43). These preclinical studies exhibit key pathological features of clinical airway stenosis, including epithelial denudation, fibroblast proliferation, extracellular matrix remodeling, and excessive collagen deposition (42, 44). These tracheal models are essential for testing anti-fibrotic drugs, gene therapies, and regenerative approaches like stem cell therapy. In addition, these models also allow for the investigation of various bronchial complication, providing valuable insights into potential therapeutic interventions for various pathological issues. Their small size, genetic manipulability, and cost-effectiveness make them a powerful tool for studying airway stenosis and developing novel treatments to improve airway healing and function (Figure 2).
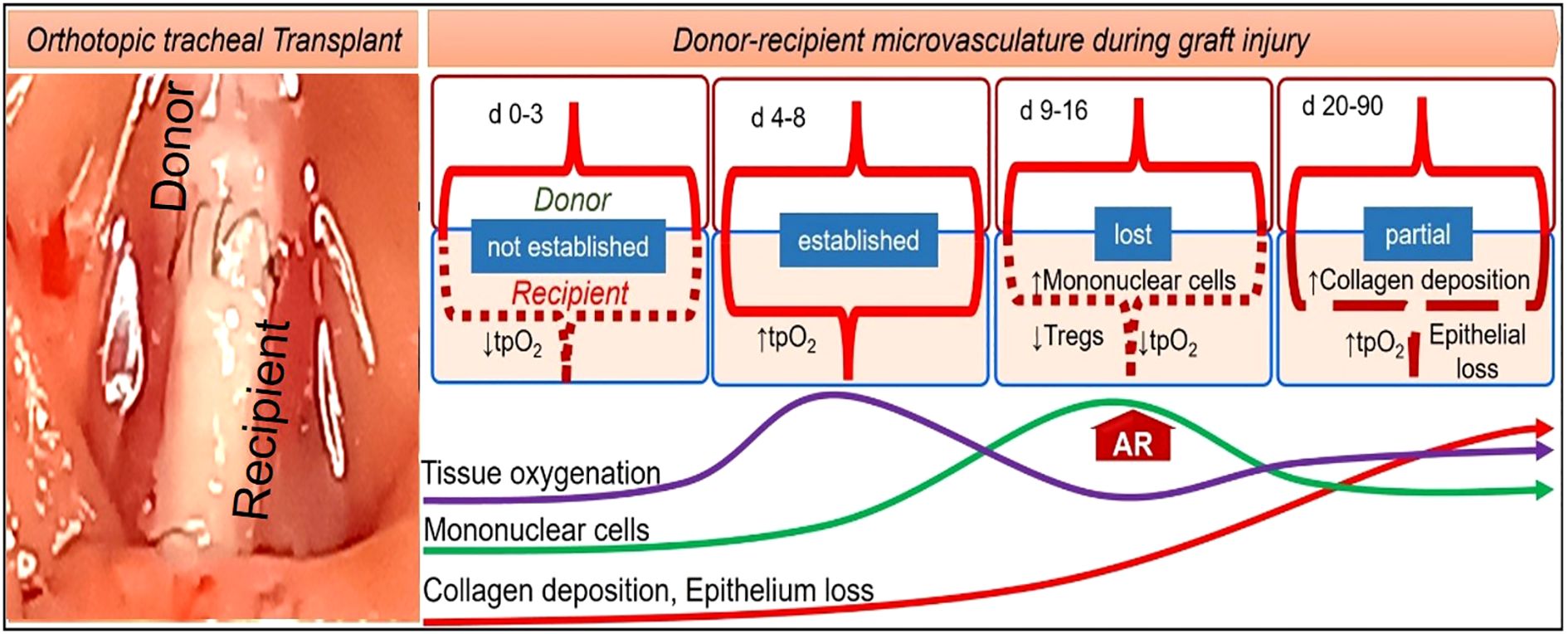
Figure 2. A mouse model of orthotopic tracheal transplantation, which demonstrates donor-recipient microvascular connections, tissue oxygenation, inflammation, collagen, and epithelial loss post-tracheal transplantation.
Immune response during airway anastomosis
CD4+ T cells play an important role during alloimmune inflammation, while Tregs and associated mediators regulate immune responses to prevent ongoing tissue damage (33, 36, 37, 45–52). In organ transplantation, inflammatory cytokines such as TNF-α, IL-2, IL-1β, and IL-6 also contribute to the immune-mediated destruction of the graft’s airway epithelium, vascular endothelium, and cilia during wound healing (53, 54). Inflammatory cytokines such as TNF-α and interleukins (IL-6, IL-8) contribute to local tissue damage and fibrosis, exacerbating bronchial stenosis (55), however, oxidative stress, driven by reactive oxygen species (ROS), further damages cellular components, impairing healing processes (56).In contrast, Tregs, M2 macrophages, and regulatory mediator s (IL-33, TGF-β, PDGF, and TSG-6) promote the repair of both airway epithelium and vascular endothelium (57–67). As a consequence of alloimmune inflammation (T cells, B cells, macrophages, active complement factors, and immunoglobulins), microvascular loss and associated tissue injuries lead to tissue remodeling, the closure of small airways, and the subsequent decline of respiratory function (5, 6, 30, 40, 68). There is, however, an association between inflammation-induced microvascular loss and airway epithelial injury, which is a major pathological reason for graft malfunction, airway narrowing and subepithelial fibrosis in allograft rejection (30). Consequently, based on the previous research therapies targeting various regulatory cells/mediators could control alloimmune inflammation, thereby promoting graft microvascular repair, airway narrowing and suppressing fibrosis (31, 69–72) (Figure 3).
Preclinical studies in OTT model have shown significant increases in CD4+ and CD8+ T cells in peripheral blood and tracheal graft from allografts compared to syngrafts (32, 35). In addition, syngrafts showed significantly elevated levels of FOXP3+ Tregs and IL-10 as compared to rejecting allografts. These findings indicated that functional microvascular loss during rejection is associated with the upregulation of both peripheral and graft infiltration of CD4+, CD8+ T cells, IL-2, TNF-α and STAT3 while a downregulation of FOXP3+ Tregs and IL-10 post-transplantation (60, 61, 73, 74). In addition, studies in OTT and other transplantation have demonstrated that alloimmune inflammation augments increase in inflammatory cell phenotypes and associated mediators, while downregulated FOXP3+ Tregs, and IL-10 in the graft, which triggers progression of airway epithelial injuries, and thus wound healing after transplantation (32, 35, 74). Various therapeutic approaches have been adapted preserve microvasculature through different regulatory mediators including regulatory cells/stem cells, IL-10, C5a blockade and CTLA-4Ig blockade in tracheal transplantation (44, 60, 61, 75). In a therapeutic approach with mesenchymal stem cells-mediated (MSCs) or regulatory T cells adoptive transfer, we successfully restored immunetolerance, through the upregulation of FOXP3+ Tregs, TSG-6 and IL-10 in the grafts (75–81). While C5a blocking significantly leads to enhanced presence of Tregs in the allograft, reinstates donor–recipient functional microvasculature, improves tissue oxygenation, microvascular blood flow, and epithelial repair, followed by an upregulation of IL-5, TGF-β, IL-10 vascular endothelial growth factor, and ANGPT1 gene expression, while it maintained a healthy epithelium and prevented subepithelial collagen deposition after 4-weeks of airway transplantation (35, 37, 51, 73). Together, C5a signaling has potential to preserve microvasculature and rescue allograft from a sustained hypoxic/ischemic phase, limits airway tissue remodeling through the induction of Treg-mediated immune tolerance. In another approach, CTLA4-Ig mediated immunosuppression significantly resulted in late increases in both peripheral CD4+/CD8+ FOXP3+ Tregs and serum IL-10, but prevents the microvascular deposition of IgG, complement factor C3d, and epithelial C4d respectively, which proportionally improved blood flow and tissue oxygenation in the graft and, thus, promotes graft repair. Also, it restored the airway lumen, epithelium, and prevented the progression of subepithelial collagen deposition up to 90 days after transplantation. This study demonstrated that CTLA4-Ig-mediated immunosuppression potentially modulates both effector response and a late surge of regulatory activity to preserve graft microvasculature and rescue allograft from sustained hypoxia and ischemia and thereby limits subepithelial fibrosis (44). All these therapeutic approaches highlighted that IL-10 mediated immunosuppression established the immune tolerance phase and thereby modulated both microvascular and epithelial integrity, which affected inflammation-associated graft malfunctioning and sub-epithelial fibrosis in rejecting allografts. Further studies investigated the reparative effects of IL-10 on microvasculature and epithelium in a mouse model of airway transplantation. To investigate the IL-10 mediated microvascular and epithelial repair, we depleted and reconstituted IL-10, and monitored graft microvasculature, airway epithelium, and associated repair proteins. Our data demonstrated that both untreated control allografts and IL-10 (−) allografts showed a significant early increase in microvascular leakiness, drop-in tissue oxygenation, blood perfusion, and denuded airway epithelium, which is associated with loss of adhesion protein Fascin-1 and β-catenin on vascular endothelial cells post-transplantation. However, IL-10 (+) promotes early microvascular and airway epithelial repair, and a proportional increase in endothelial Fascin-1, and β-catenin post-transplantation. Moreover, airway epithelial cells also express a significantly higher expression of FOXJ1 and β-catenin in syngrafts and IL-10 (+) allografts as compared to IL-10 (−) and untreated controls post-transplantation. Collectively, these findings demonstrated that IL-10 mediated microvascular and epithelial changes are associated with the expression of FOXJ1, β-catenin, and Fascin-1 proteins on the airway epithelial and vascular endothelial cells, respectively. These findings established a potential reparative modulation of IL-10 associated microvascular and epithelial repair, which could provide a vital therapeutic strategy to facilitate bronchial anastomotic graft repair in clinical settings (13, 44, 60, 61, 73–75).
Hypoxia and ischemia
In lung transplantation, hypoxia and ischemia during bronchial anastomosis are critical concerns that can significantly impact the success of the procedure (10, 82). Both hypoxia and ischemia result in impaired cellular function and reduced wound healing, which can lead to complications like anastomotic dehiscence, infection, and scarring (6, 83, 84). The immune response triggers the release of inflammatory mediators that further impair vascular function and increase the risk of ischemic damage (6, 10, 83, 85, 86). These conditions can impact the healing of the bronchial anastomosis, leading to complications such as anastomotic leak, airway stenosis, or graft dysfunction (16). Hypoxia-inducible factor-1 alpha (HIF-1α) plays a key role in cellular adaptation to hypoxic conditions at the anastomotic site (87). Under oxygen deprivation, HIF-1α activation promotes angiogenesis via vascular endothelial growth factor (VEGF), but excessive hypoxia can lead to inadequate revascularization, increasing the risk of ischemia-related complications (88). Chronic ischemia can result in fibrosis and scarring of the bronchial walls, leading to bronchial stenosis, which can cause airflow obstruction (89). Ischemia refers to a reduced blood supply to the grafted lung tissue, which can occur during the surgical procedure, especially when there is tension at the bronchial anastomosis or if the vascular supply is compromised (6). Ischemia can also occur postoperatively if there is inadequate perfusion, leading to necrosis of the bronchial tissues (90). In addition, ischemia can lead to delayed healing and increase the risk of airway complications such as stenosis or bronchomalacia, as the prolonged lack of oxygen and nutrients to the bronchial tissues impair the cellular repair processes, causing fibrosis or weakening of the anastomosis (11). The mechanisms of hypoxia and ischemia during bronchial anastomosis after LTx are closely tied to the disruption of normal blood flow to the bronchial tissues and the unique challenges faced during this delicate surgical procedure (5). When performing the bronchial anastomosis, it’s critical that blood flow to the bronchial tissue is maintained or restored, and If there is poor vascularization, it leads to insufficient oxygen delivery to the anastomotic site, which results in hypoxia (10). The donor lung’s bronchial arteries are often different from those of the recipient, and in many cases, these donor vessels might not have robust anastomotic branches with the recipient’s vascular system, leading to poor perfusion (19). During the bronchial anastomoses, the bronchus may undergo handling that causes damage to microvessels in the area around the anastomosis, which disrupts the local blood flow, contributing to ischemia and hypoxia (7, 30). Furthermore, tension at the anastomotic site, such as when the bronchial tubes are not aligned properly or when excessive tension is placed on the sutures, can lead to vessel constriction or occlusion, further impeding blood flow (9). After the anastomosis, oxygen must diffuse from the surrounding tissues to the bronchial walls, and in the absence of an optimal blood supply, this diffusion is impaired, which leads to hypoxic conditions in the bronchial tissue (9, 91). Ischemia occurs when there is insufficient blood supply to a tissue, leading to a shortage of oxygen and nutrients required for cell survival and tissue function (15, 83). Several mechanisms contribute to ischemia during bronchial anastomosis, and one of the primary causes of ischemia after lung transplantation is the interruption of normal blood flow when the donor lung is surgically connected to the recipient’s vasculature (10, 82). If the bronchial arteries are not adequately reconnected or if the blood vessels are not aligned properly, the blood flow to the bronchus can be limited or obstructed (6, 92). In some cases, especially in older transplant techniques, the bronchial arteries of the donor may be ligated or not re-anastomosed, relying on collateral circulation, which may not be sufficient for proper oxygenation of the bronchial tissues (93). During the dissection and implantation process, there is a risk of damaging the small vessels that supply the bronchial tree, and if these vessels are compromised, blood flow can be reduced or completely interrupted, leading to ischemic injury (9). The endothelial lining of the blood vessels can also become dysfunctional due to ischemic injury, further impairing the ability of vessels to dilate and increase blood flow to the area (86). There are factors that contribute to both Hypoxia and Ischemia which include Inadequate anastomotic techniques and infection (94). Infection or inflammation at the site of the bronchial anastomosis can lead to local vasculitis, which can further compromise blood supply and exacerbate ischemic damage (95). A poorly executed bronchial anastomosis may not only lead to hypoxia but also contribute to ischemia if the blood vessels are pinched, compressed, or disconnected inappropriately during suturing, which involves carefully placing stitches that minimize tension and avoid damaging the vasculature (95). Rejection responses in the early post-transplant period can lead to vascular changes (e.g., endothelial injury, vasculitis), reducing blood flow to the bronchial tissues and contributing to ischemia and hypoxia. Ischemia is usually monitored using advanced imaging and monitoring techniques during surgery to ensure that the blood flow to the transplanted bronchus is adequate, which may include the use of Doppler ultrasound or other intraoperative assessments to evaluate vascular patency (89). In addition, careful attention to suturing techniques to minimize tension on the bronchus and to ensure proper alignment and blood supply to the anastomosis, ensuring an adequate vascular pedicle for the transplanted lung can help reduce ischemia. Early detection of complications through close monitoring of the patient’s respiratory status, chest imaging, and bronchoscopy to assess the integrity of the bronchial anastomosis and identify any signs of hypoxia, ischemia, or airway obstruction (96, 97). Regular follow-up with imaging and pulmonary function tests is critical to monitor for these complications, however, even after the anastomosis heals, chronic ischemia or hypoxia could result in long-term issues such as airway stenosis or bronchiectasis, which can impact the patient’s lung function over time.
Hypoxia triggers several keys signaling pathways in cells to adapt to the reduced oxygen supply, and these pathways are critical for cellular survival, angiogenesis and metabolic adaptation. The main signaling pathway triggered by hypoxia is the Hypoxia-Inducible Factor (HIF) pathway, though other pathways also play important roles in mediating cellular responses to hypoxia which include AMP-Activated Protein Kinase (AMPK) Pathway, Mitogen-Activated Protein Kinase (MAPK) Pathway, Nitric Oxide (NO) Pathway, Toll-Like Receptor (TLR) Pathway, Sirtuin Pathway, Hypoxia-Activated Signaling through the PI3K/Akt Pathway, and JAK/STAT Pathway (13, 98–100). The HIF pathway is the central molecular response to hypoxia, and it involves a family of transcription factors, primarily HIF-1 and HIF-2, which regulate the expression of genes involved in adaptation to low oxygen levels (101). In normoxic conditions, HIF-1α (a subunit of HIF-1) is hydroxylated by prolyl hydroxylase enzymes in the presence of oxygen, and this hydroxylation marks HIF-1α for degradation by the proteasome. HIF-1β (the other subunit of HIF-1) remains stable and forms a dimer with HIF-1α when it is available. However, without the accumulation of HIF-1α, the complex does not translocate to the nucleus. Under hypoxic conditions, the activity of prolyl hydroxylases is inhibited due to the lack of oxygen, preventing the hydroxylation and degradation of HIF-1α. As a result, HIF-1α accumulates, translocate to the nucleus, and dimerizes with HIF-1β. The HIF complex then binds to specific DNA sequences (hypoxia-responsive elements or HREs) in the promoters of target genes. These genes take active part in various process like angiogenesis where it promotes the expression of genes like VEGF (vascular endothelial growth factor), which stimulates the formation of new blood vessels to improve oxygen delivery. HIF also activates genes involved in anaerobic metabolism, such as GLUT1 (glucose transporter 1) and LDHA (lactate dehydrogenase), which enhance glucose uptake and lactic acid production, allowing the cell to produce energy in the absence of oxygen (102). HIF stimulates the production of erythropoietin (EPO), which promotes red blood cell production to increase oxygen-carrying capacity (103, 104) (Figure 4).
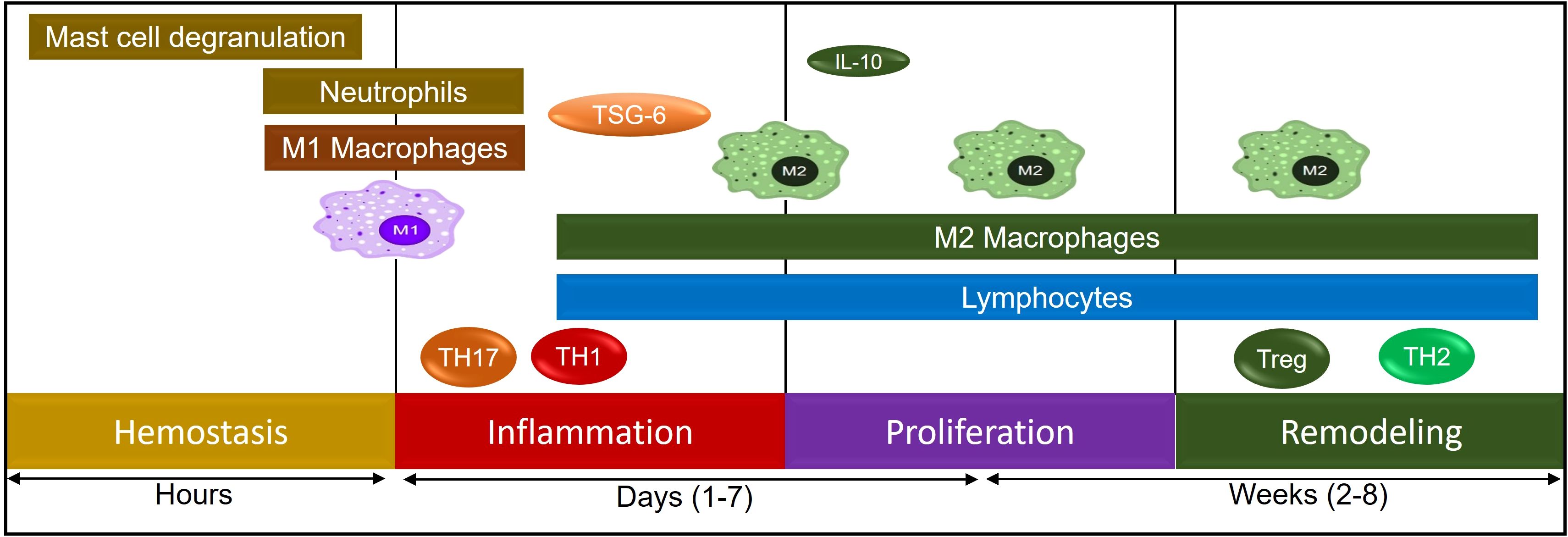
Figure 4. A schematic representation of various immune cells during the process of tissue remodeling.
Bronchial fibrosis and stenosis
The phase of hypoxia and ischemia peri lung transplantation LTx stimulates the production of inflammatory and profibrotic cytokines, leading to an imbalance in collagen synthesis and excessive proliferation of granulation tissue, which contributes to slow wound healing and multiple anastomotic issues that impair patient outcome (10, 90, 105). Clinical studies confirm that changes in airway oxygenation and perfusion during the development and resolution of airway obstructions are relatively understudied aspects of pulmonary physiology (5, 39, 89, 106). There has been evidence in clinical studies that ischemic injury is manifested by endothelial dysfunction resulting in increased vascular permeability and proinflammatory cytokines, which negatively affect transplantation outcomes (15). Bronchial fibrosis and bronchial stenosis are both conditions that can affect the bronchial tubes, which are muscular structures that branch off the trachea into the lungs (16). Bronchial fibrosis can cause bronchial stenosis due to external luminal constriction, which is an abnormal narrowing of the bronchial tubes that can be caused by a number of factors, including. Fibrosis is a type of bronchial anastomotic complication that can occur after lung transplantation, which can result in shortness of breath, bronchial stenosis, and anastomosis dehiscence. Airway fibrosis after lung transplantation can occur due to a number of factors, including bronchial artery injury, poor graft preservation, debridement of the peri-bronchial tissue during anastomosis can increase ischemic injury. The molecular events that contribute to fibrosis and stenosis include Cell proliferation, Inflammation, ECM degradation, MicroRNA expression, Connective tissue stiffening, and Immune responses (107). Various proinflammatory cytokines have been associated with fibrosis, which include IL-4, IL-13, IL-1, TGF-b1, IL-17-A, while IL-10 has been widely recognized as antifibrotic (108). The relationship between inflammation and fibrogenesis has led to IL-10 being identified as a potential antifibrotic target as well as a gatekeeper of fibrotic/antifibrotic signaling, so immune and cell-based therapies aiming to capitalize on IL-10 as a target could be effective in treating lung transplanted patients suffering from delayed would healing. The mechanisms that cause airway fibrosis are complex and not fully understood. However, some of the factors that may be involved include epithelial and endothelial injury, Inflammation, Oxidative stress, TGF-β, reduced autophagy in lung epithelial cells and fibroblasts can aggravate inflammation and fibrosis (109). TGF-β: This is a profibrotic mediator that can promote epithelial-mesenchymal transition (EMT), fibroblast activation. During fibrosis, activated fibroblasts synergize with TGF-β1 to promote extracellular matrix and collagen deposition (109). Matrix metalloproteinases (MMPs) are endopeptidases that contribute to the restoration of tissue architecture by eliminating damaged components of the extracellular matrix (ECM). MMP activation in the setting of a stable airway is due to alloantigen presentation, and a direct contact between T lymphocytes and monocytes has been described as a major pathway for MMP expression (110). Activated T cells induced MMP-9 from macrophages and fibroblasts, elevated levels of MMP, particularly MMP-8, MMP-9 and TIMP-1 were found in BAL fluid in transplant patients (111). Endothelial cells are critical in synthesizing growth factors such as vascular endothelial growth factor (VEGF), platelet derived growth factor (PDGF), TGFβ1 and endothelin-1(ET-1) (112). Instances where microvascular lumen is occluded by a thick collagen layer, growth factor pathways appear to be dysregulated, and this pathway therefore further adds to the scar burden in the development of bronchial stenosis (11). This pathological alteration is a common airway complication after lung transplantation that can cause a number of problems, including dyspnea, cough, wheezing, declining flow rates on spirometry, and recurring post-obstructive pneumonia (11, 19, 113). Occurrence of fibrosis a crucial factor during bronchial anastomotic complications, and extent has been extensively studied in mouse model of orthotopic tracheal transplantation, which replicated bronchial anastomoses. In tracheal transplantation model, it has been shown that progression of subepithelial collagen deposition starts soon after graft microvascular disruption, which further leads to tracheal narrowing (32, 35, 44, 60, 61, 74, 75).
Anastomotic stenosis occurs at the surgical site due to insufficient blood supply to the donor-tracheobronchial tree, which can lead to ischemia, anastomotic dehiscence, malacia and stenosis (114, 115). Bronchial stenoses are challenging complications after lung transplantation and are associated with high rates of morbidity and mortality (113, 114). While in clinical lung transplantation, airway stenosis and fibrosis are common complications after lung transplantation and can significantly impact a patient’s long-term prognosis. Studies have reported that up to 40% of lung transplant patients experience perianastomotic stenosis, and up to 4% experience non-anastomotic distal bronchial stenoses. Angiogenesis is another mechanism which can add insult to already ischemic bronchus. In murine orthotopic trachea transplant (OTT) models repair of airway microvasculature is repaired by recipient derived precursor cells via HIF-1α pathway. HIF-1α expression was also linked to microvascular integrity and Aspergillus fumigatus host-pathogen interaction in murine OTT model. Angiogenesis and extracellular matrix (ECM) deposition increases at the donor-recipient interface via the usual sequential phases of hemostasis via fibrin clot, inflammatory phase of neutrophil mediated microbe/foreign body destruction, proliferative phase of granulation tissue deposition and finally the maturation phase of tissue remodeling. After lung transplant, re-establishment of this capillary network is done predominantly by recipient derived Tie2+ (tunica intima endothelial tyrosine kinase 2) positive endothelial cells. This capillary restoration is complicated by the fact that microvasculature of airways subjected to alloimmune rejection and are unstable with small caliber arterioles, venules, have relatively poor pericyte coverage and are less efficient in delivery of nutrients and oxygen to injured airways. Angiogenesis is either driven by hypoxia (8) or occurs downstream to deposition of fibrin clot and subsequent inflammatory secretion of growth factors such as (be more specific) vascular endothelial growth factor (VEGF), fibroblast growth factor (FGF) and transforming growth factor beta (TGF-β). Hypoxia mediated cellular response is driven primarily by the hypoxia-inducible-factor (HIF) pathway. Since HIF-dependent changes in metabolism affect the phenotype and function of immune cells, the two pathways leading to angiogenesis i.e. hypoxia and inflammation are interconnected. In addition, failure of angiogenesis at the donor-recipient interface and resultant necrosis leads to 1-10% reported incidences of dehiscence (116). Another airway complications, bronchomalacia, is caused by atrophy of longitudinal elastic fibers and replacement of cartilaginous bronchial rings by fibrous collagen (24). Diffuse bronchomalacia involving the entire bronchus also has been described in the era of tracheal anastomosis but no case reports of diffuse bronchomalacia were found in the current literature that involves sequential double lung transplant with bronchial anastomosis.
Wound healing
In wound healing, several immune cells participate in the process, including platelets, neutrophils, macrophages, fibroblasts, lymphocytes, epithelial and endothelial cells. Wound healing is a complex process that goes through fibrotic remodeling, angiogenesis, cell migration and proliferation, and a discrete equilibrium of these stages to facilitate effective wound healing. This balance, however, is disrupted in chronic non-healing wounds, wherein the impaired microvascular blood supply and resulting ischemia cripple cellular functions and make it difficult to deliver the basic signaling molecules. However, Tregs, as well as their associated regulatory mediators, help to protect the tissue from inflammation (76, 117–120). During hemostasis, platelets release TGF-β1, PDGF, FGF-2, and VEGF to recruit neutrophils and macrophages, while neutrophils release ROS, NO, proteases, VEGF, and IL-17 to destroy pathogens (121–123). Besides, NK cells secrete IFN-γ, TNF-α and also release perforins and granzymes that are cytotoxic to infected cells (124). Moreover, neutrophils release TNF-α, IL-1β, IL-6, and MCP-1, which attract monocytes and dendritic cells and activate T cells that cause Th1 pro-inflammatory responses (125). In the inflammatory phase of acute wound healing, macrophages secrete IL-1, VEGF, FGF-2, TNF- α, IL-6, IFN-γ, TGF-β, and PDGF, which promote the proliferation of fibroblasts, keratinocytes, and epithelial cells, whereas in the remodeling phase IL-4, IL-10, and IL-13 induce the transition of M1 to M2 macrophages (122, 125). Besides, other cells, such as MSCs and fibroblasts, secrete TSG-6, which promotes wound healing by limiting macrophage activation, inflammation, and fibrosis (126, 127). M2 macrophages generally inhibit inflammation and promote tissue repair through IL-10 and TGF-β, which stimulate ECM synthesis, angiogenesis, and fibroblast proliferation (117). During inflammation, lymphocytes are also recruited to the wound and release IFN- γ, TGF-β, IL-10, IL-2, IL-17, and IL-22 (128). Later, angiogenesis replaces damaged vessels with granulation tissue, in which epidermal cells, fibroblasts, vascular endothelial cells, and macrophages produce β-FGF, TGF- β, and VEGF to bolster angiogenesis. VEGF induces angiogenesis through adenosine, which in turn stimulates hypoxia-induced proliferation, therefore A2A receptors, is now considered a potent regulator of the early stages of wound healing (129, 130). Transitioning from a pro-inflammatory M1 macrophage-dominant wound to an anti-inflammatory M2 macrophage-dominant milieu is essential in resolving inflammation and preparing the wound for effective repair, which is further supported through the Tregs and associated mediators (63, 117, 120, 122, 131). IL-10, produced mainly by Tregs, monocytes, Th2 cells, subsets of activated T cells, and B cells, collectively restrains inflammation and immune responsiveness by regulating cell proliferation, and differentiation (132–134). IL-10 is mostly secreted by Tregs to combat inflammation, tissue repair, and antifibrotic events; however, IL-10 levels also regulate Treg expression of FOXP3 and suppressive activities (31, 70, 73, 74, 80, 132, 133, 135–146). Furthermore, Tregs promote tissue repair through various regulatory cytokines, which include IL-10, TGF-β, IL-33 and IL-35 (147, 148). In addition to limiting collateral tissue damage caused by uncontrolled immune responses, IL-10 helps maintain the regulatory microenvironment by upregulating TSG-6, M2 macrophages, and, tolerogenic dendritic cells (DC-10), antigen-specific T regulatory type 1 (Tr1), while suppressing Th1/Th17 effector immunity (60, 78, 80, 149–152). IL-10 is a potent antifibrotic, reparative, as well as vasculo-protective cytokine that assists in the repair of tissue following a sporadic alloimmune response during transplantation (60, 61, 76, 78, 80, 151, 153, 154). The anti-inflammatory properties of IL-10 help to suppress the production of pro-inflammatory cytokines such as IFN- γ, IL-2, IL-3, and TNF- α by Th1 cells, mast cells, NK cells, endothelial cells, eosinophils, and macrophages (79, 79, 132, 150, 155–158). Through the surface expression of TSG-6, FOXJ1, Fascin-1, and β-catenin proteins, IL-10 enhances microvascular supply, tissue oxygenation, and airway epithelium regeneration in allografts, further supporting the therapeutic benefits during wound healing and tissue repair (60, 61, 76, 159) (Figure 5).
Conclusion
We conclude that a molecular pathway and associated alloimmune response and microvascular disruption during airway anastomosis play a crucial role in the development of airway complication as replicated in a mouse model of orthotopic tracheal transplantation model, which replicates airway complications as seen in clinical conditions. Understanding these molecular pathways aids in developing targeted therapeutic strategies, such as antioxidant therapies, angiogenic growth factors, and immunomodulation, to improve anastomotic healing and reduce complications.
Author contributions
MK: Conceptualization, Data curation, Formal analysis, Funding acquisition, Investigation, Methodology, Project administration, Resources, Software, Supervision, Validation, Visualization, Writing – original draft, Writing – review & editing. SB: Writing – review & editing. CL: Writing – review & editing. SK: Writing – review & editing.
Funding
The author(s) declare that financial support was received for the research and/or publication of this article. The authors declare that this study received funding from Chuck and Mary Meyers and Richard and Eibhlin Henggeler. The funders were not involved in the study design, collection, analysis, interpretation of data, the writing of this article, or the decision to submit it for publication. ASK also supported by P01 AI116501, R01AI145108-01, R01HL166402, I01 BX002299-05.
Acknowledgments
The author would like to thank the University of Maryland Information Technology section for all the software support they provided.
Conflict of interest
The authors declare that the research was conducted in the absence of any commercial or financial relationships that could be construed as a potential conflict of interest.
Generative AI statement
The author(s) declare that no Generative AI was used in the creation of this manuscript.
Publisher’s note
All claims expressed in this article are solely those of the authors and do not necessarily represent those of their affiliated organizations, or those of the publisher, the editors and the reviewers. Any product that may be evaluated in this article, or claim that may be made by its manufacturer, is not guaranteed or endorsed by the publisher.
References
1. Parulekar AD, Kao CC. Detection, classification, and management of rejection after lung transplantation. J Thorac Dis. (2019) 11:S1732–S9. doi: 10.21037/jtd.2019.03.83
2. Thabut G, Mal H. Outcomes after lung transplantation. J Thorac Dis. (2017) 9:2684–91. doi: 10.21037/jtd.2017.07.85
3. Anile M, Diso D, Rendina EA, Venuta F. Airway anastomosis for lung transplantation. J Thorac Dis. (2016) 8:S197–203. doi: 10.3978/j.issn.2072-1439.2016.01.67
4. Simonova MS, Rusakov MA, Parshin VD. Airway complications after lung transplantation. Khirurgiia (Mosk). (2021) 7):77–83. doi: 10.17116/hirurgia202107177
5. Luckraz H, Goddard M, McNeil K, Atkinson C, Charman SC, Stewart S, et al. Microvascular changes in small airways predispose to obliterative bronchiolitis after lung transplantation. J Heart Lung Transplant. (2004) 23:527–31. doi: 10.1016/j.healun.2003.07.003
6. Dhillon GS, Zamora MR, Roos JE, Sheahan D, Sista RR, van der Starre P, et al. Lung transplant airway hypoxia: A diathesis to fibrosis? Am J Respir Crit Care Med. (2010) 182:230–6. doi: 10.1164/rccm.200910-1573OC
7. Nicolls MR, Hsu JL, Jiang X. Microvascular injury after lung transplantation. Curr Opin Organ Transplant. (2016) 21:279–84. doi: 10.1097/MOT.0000000000000307
8. Nicolls MR, Voelkel NF. Hypoxia and the lung: beyond hypoxic vasoconstriction. Antioxid Redox Signal. (2007) 9:741–3. doi: 10.1089/ars.2007.1574
9. Nicolls MR, Zamora MR. Bronchial Blood Supply after Lung Transplantation without Bronchial Artery Revascularization. Curr Opin Organ Transplant. (2010) 15:563–7. doi: 10.1097/MOT.0b013e32833deca9
10. Pasnupneti S, Nicolls MR. Airway hypoxia in lung transplantation. Curr Opin Physiol. (2019) 7:21–6. doi: 10.1016/j.cophys.2018.12.002
11. Jindal A, Avasaral S, Grewal H, Mehta A. Airway complications following lung transplantation. Indian J Thoracic Cardiovasc Surg. (2022) 38:326–34. doi: 10.1007/s12055-022-01376-5
12. Yserbyt J, Dooms C, Vos R, Dupont LJ, Van Raemdonck DE, Verleden GM. Anastomotic airway complications after lung transplantation: risk factors, treatment modalities and outcome-a single-centre experience. Eur J Cardiothorac Surg. (2016) 49:e1–8. doi: 10.1093/ejcts/ezv363
13. Jiang X, Khan MA, Tian W, Beilke J, Natarajan R, Kosek J, et al. Adenovirus-mediated hif-1alpha gene transfer promotes repair of mouse airway allograft microvasculature and attenuates chronic rejection. J Clin Invest. (2011) 121:2336–49. doi: 10.1172/JCI46192
14. Wilkes DS. Chronic lung allograft rejection and airway microvasculature: is hif-1 the missing link? J Clin Invest. (2011) 121:2155–7. doi: 10.1172/JCI58329
15. den Hengst WA, Gielis JF, Lin JY, Van Schil PE, De Windt LJ, Moens AL. Lung ischemia-reperfusion injury: A molecular and clinical view on a complex pathophysiological process. Am J Physiol Heart Circ Physiol. (2010) 299:H1283–99. doi: 10.1152/ajpheart.00251.2010
16. Frye L, Machuzak M. Airway complications after lung transplantation. Clinics Chest Med. (2017) 38:693–706. doi: 10.1016/j.ccm.2017.07.010
17. Groetzner J, Kur F, Spelsberg F, Behr J, Frey L, Bittmann I, et al. Airway anastomosis complications in de novo lung transplantation with sirolimus-based immunosuppression. J Heart Lung Transplant. (2004) 23:632–8. doi: 10.1016/S1053-2498(03)00309-7
18. Van De Wauwer C, Van Raemdonck D, Verleden GM, Dupont L, De Leyn P, Coosemans W, et al. Risk Factors for Airway Complications within the First Year after Lung Transplantation. Eur J Cardiothorac Surg. (2007) 31:703–10. doi: 10.1016/j.ejcts.2007.01.025
19. Crespo MM. Airway complications in lung transplantation. J Thorac Dis. (2021) 13:6717–24. doi: 10.21037/jtd-20-2696
20. Awori Hayanga JW, Aboagye JK, Shigemura N, Hayanga HK, Murphy E, Khaghani A, et al. Airway complications after lung transplantation: contemporary survival and outcomes. J Heart Lung Transplant. (2016) 35:1206–11. doi: 10.1016/j.healun.2016.04.019
21. Chhajed PN, Malouf MA, Tamm M, Spratt P, Glanville AR. Interventional bronchoscopy for the management of airway complications following lung transplantation. Chest. (2001) 120:1894–9. doi: 10.1378/chest.120.6.1894
22. Weder W, Inci I, Korom S, Kestenholz PB, Hillinger S, Eich C, et al. Airway complications after lung transplantation: risk factors, prevention and outcome. Eur J Cardiothorac Surg. (2009) 35:293–8; discussion 8. doi: 10.1016/j.ejcts.2008.09.035
23. Schweiger T, Nenekidis I, Stadler JE, Schwarz S, Benazzo A, Jaksch P, et al. Single running suture technique is associated with low rate of bronchial complications after lung transplantation. J Thoracic Cardiovasc Surg. (2020) 160:1099–108.e3. doi: 10.1016/j.jtcvs.2019.12.119
24. Muñoz-Fos A, Moreno P, González FJ, Ruiz E, Vaquero JM, Baamonde C, et al. Airway complications after lung transplantation—a contemporary series of 400 bronchial anastomoses from a single center. J Clin Med. (2023) 12:3061. doi: 10.3390/jcm12093061
25. Kim HH, Jo KW, Shim TS, Ji W, Ahn JH, Oh DK, et al. Incidence, risk factors, and clinical characteristics of airway complications after lung transplantation. Sci Rep. (2023) 13:667. doi: 10.1038/s41598-023-27864-1
26. Siddique A, Sabbah BN, Arabi T, Shakir IM, Abdulqawi R, AlKattan K, et al. Treatment of bronchial anastomotic fistula using autologous platelet-rich plasma post lung transplantation. J Cardiothorac Surg. (2022) 17:204. doi: 10.1186/s13019-022-01965-w
27. Fernandez-Bussy S, Majid A, Caviedes I, Akindipe O, Baz M, Jantz M. Treatment of airway complications following lung transplantation. Arch Bronconeumol. (2011) 47:128–33. doi: 10.1016/j.arbres.2010.10.011
28. Garrido G, Dhillon GS. Medical course and complications after lung transplantation. Psychosoc Care End-Stage Organ Dis Transplant Patients. (2018), 279–88. doi: 10.1007/978-3-319-94914-7_26
29. Morrison MI, Pither TL, Fisher AJ. Pathophysiology and classification of primary graft dysfunction after lung transplantation. J Thorac Dis. (2017) 9:4084–97. doi: 10.21037/jtd.2017.09.09
30. Babu AN, Murakawa T, Thurman JM, Miller EJ, Henson PM, Zamora MR, et al. Microvascular destruction identifies murine allografts that cannot be rescued from airway fibrosis. J Clin Invest. (2007) 117:3774–85. doi: 10.1172/JCI32311
31. Khan MA, Hsu JL, Assiri AM, Broering DC. Targeted complement inhibition and microvasculature in transplants: A therapeutic perspective. Clin Exp Immunol. (2015) 183. doi: 10.1111/cei.12713
32. Khan MA, Jiang X, Dhillon G, Beilke J, Holers VM, Atkinson C, et al. Cd4+ T cells and complement independently mediate graft ischemia in the rejection of mouse orthotopic tracheal transplants. Circ Res. (2011) 109:1290–301. doi: 10.1161/CIRCRESAHA.111.250167
33. Bluestone JA. Foxp3, the transcription factor at the heart of the rebirth of immune tolerance. J Immunol. (2017) 198:979–80. doi: 10.4049/jimmunol.1602060
34. Sakaguchi S. Naturally arising cd4+ Regulatory T cells for immunologic self-tolerance and negative control of immune responses. Annu Rev Immunol. (2004) 22:531–62. doi: 10.1146/annurev.immunol.21.120601.141122
35. Khan MA, Maasch C, Vater A, Klussmann S, Morser J, Leung LL, et al. Targeting complement component 5a promotes vascular integrity and limits airway remodeling. Proc Natl Acad Sci U S A. (2013) 110:6061–6. doi: 10.1073/pnas.1217991110
36. Khan MA, Nicolls MR. Complement-mediated microvascular injury leads to chronic rejection. Adv Exp Med Biol. (2013) 734:233–46. doi: 10.1007/978-1-4614-4118-2_16
37. Khan MA, Shamma T. Complement factor and T-cell interactions during alloimmune inflammation in transplantation. J Leukoc Biol. (2019) 105:681–94. doi: 10.1002/JLB.5RU0718-288R
38. Khan MA, Shamma T, Kazmi S, Altuhami A, Ahmed HA, Assiri AM, et al. Hypoxia-induced complement dysregulation is associated with microvascular impairments in mouse tracheal transplants. J Transl Med. (2020) 18:147. doi: 10.1186/s12967-020-02305-z
39. Khan MA, Dhillon G, Jiang X, Lin YC, Nicolls MR. New methods for monitoring dynamic airway tissue oxygenation and perfusion in experimental and clinical transplantation. Am J Physiol Lung Cell Mol Physiol. (2012) 303. doi: 10.1152/ajplung.00162.2012
40. Luckraz H, Goddard M, McNeil K, Atkinson C, Sharples LD, Wallwork J. Is obliterative bronchiolitis in lung transplantation associated with microvascular damage to small airways? Ann Thorac Surg. (2006) 82:1212–8. doi: 10.1016/j.athoracsur.2006.03.070
41. Sullivan DE, Ferris M, Nguyen H, Abboud E, Brody AR. Tnf-alpha induces tgf-beta1 expression in lung fibroblasts at the transcriptional level via ap-1 activation. J Cell Mol Med. (2009) 13:1866–76. doi: 10.1111/j.1582-4934.2009.00647.x
42. Lama VN, Belperio JA, Christie JD, El-Chemaly S, Fishbein MC, Gelman AE, et al. Models of lung transplant research: A consensus statement from the national heart, lung, and blood institute workshop. JCI Insight. (2017) 2:1–14. doi: 10.1172/jci.insight.93121
43. Hua X, Deuse T, Tang-Quan KR, Robbins RC, Reichenspurner H, Schrepfer S. Heterotopic and orthotopic tracheal transplantation in mice used as models to study the development of obliterative airway disease. J Vis Exp. (2010) 35:1–4. doi: 10.3791/1437
44. Khan MA, Shamma T, Altuhami A, Ahmed HA, Assiri AM, Broering DC. Ctla4-ig mediated immunosuppression favors immunotolerance and restores graft in mouse airway transplants. Pharmacol Res. (2022) 178, 106147. doi: 10.1016/j.phrs.2022.106147
45. Marek-Trzonkowska N, Mysliwiec M, Dobyszuk A, Grabowska M, Techmanska I, Juscinska J, et al. Administration of cd4+Cd25highcd127- regulatory T cells preserves beta-cell function in type 1 diabetes in children. Diabetes Care. (2012) 35:1817–20. doi: 10.2337/dc12-0038
46. Abdel-Gadir A, Massoud AH, Chatila TA. Antigen-specific treg cells in immunological tolerance: implications for allergic diseases. F1000Research. (2018) 7:38–. doi: 10.12688/f1000research.12650.1
47. Lu L, Barbi J, Pan F. The regulation of immune tolerance by foxp3. Nat Rev Immunol. (2017) 17:703–17. doi: 10.1038/nri.2017.75
48. Pellerin L, Jenks JA, Bégin P, Bacchetta R, Nadeau KC. Regulatory T cells and their roles in immune dysregulation and allergy. Immunol Res. (2014) 58:358–68. doi: 10.1007/s12026-014-8512-5
49. Okubo Y, Torrey H, Butterworth J, Zheng H, Faustman DL. Treg activation defect in type 1 diabetes: correction with tnfr2 agonism. Clin Trans Immunol. (2016) 5:e56–e. doi: 10.1038/cti.2015.43
50. Wang HX, Kang X, Chu S, Li H, Li X, Yin X, et al. Dysregulated icos(+) proinflammatory and suppressive regulatory T cells in patients with rheumatoid arthritis. Exp Ther Med. (2018) 16:3728–34. doi: 10.3892/etm.2018.6657
51. Khan MA, Assiri AM, Broering DC. Complement and macrophage crosstalk during process of angiogenesis in tumor progression. J BioMed Sci. (2015) 22:58. doi: 10.1186/s12929-015-0151-1
52. Khan MA, Assiri AM, Broering DC. Complement mediators: key regulators of airway tissue remodeling in asthma. J Transl Med. (2015) 13:272. doi: 10.1186/s12967-015-0565-2
53. Fogal B, Yi T, Wang C, Rao DA, Lebastchi A, Kulkarni S, et al. Neutralizing il-6 reduces human arterial allograft rejection by allowing emergence of cd161+ Cd4+ Regulatory T cells. J Immunol. (2011) 187:6268–80. doi: 10.4049/jimmunol.1003774
54. Ioannidou E, Kao D, Chang N, Burleson J, Dongari-Bagtzoglou A. Elevated serum interleukin-6 (Il-6) in solid-organ transplant recipients is positively associated with tissue destruction and il-6 gene expression in the periodontium. J Periodontol. (2006) 77:1871–8. doi: 10.1902/jop.2006.060014
55. Cabrini G, Rimessi A, Borgatti M, Lampronti I, Finotti A, Pinton P, et al. Role of cystic fibrosis bronchial epithelium in neutrophil chemotaxis. Front Immunol. (2020) 11:1438. doi: 10.3389/fimmu.2020.01438
56. Hunt M, Torres M, Bachar-Wikstrom E, Wikstrom JD. Cellular and molecular roles of reactive oxygen species in wound healing. Commun Biol. (2024) 7:1534. doi: 10.1038/s42003-024-07219-w
57. Wagner MJ, Khan M, Mohsin S. Healing the broken heart; the immunomodulatory effects of stem cell therapy. Front Immunol. (2020) 11:639. doi: 10.3389/fimmu.2020.00639
58. Song T, Eirin A, Zhu X, Zhao Y, Krier JD, Tang H, et al. Mesenchymal stem cell-derived extracellular vesicles induce regulatory T cells to ameliorate chronic kidney injury. Hypertension. (2020) 75:1223–32. doi: 10.1161/hypertensionaha.119.14546
59. Day AJ, Milner CM. Tsg-6: A multifunctional protein with anti-inflammatory and tissue-protective properties. Matrix Biol. (2019) 78-79:60–83. doi: 10.1016/j.matbio.2018.01.011
60. Kazmi S, Khan MA, Shamma T, Altuhami A, Ahmed HA, Mohammed Assiri A, et al. Targeting interleukin-10 restores graft microvascular supply and airway epithelium in rejecting allografts. Int. J. Mol. Sci. (2022) 23:1269. doi: 10.3390/ijms23031269
61. Khan MA, Ashoor GA, Shamma T, Alanazi F, Altuhami A, Kazmi S, et al. Il-10 mediated immunomodulation limits subepithelial fibrosis and repairs airway epithelium in rejecting airway allografts. Cells. (2021) 10:1248. doi: 10.3390/cells10051248
62. McIntyre LL, Greilach SA, Othy S, Sears-Kraxberger I, Wi B, Ayala-Angulo J, et al. Regulatory T cells promote remyelination in the murine experimental autoimmune encephalomyelitis model of multiple sclerosis following human neural stem cell transplant. Neurobiol Dis. (2020) 140:104868. doi: 10.1016/j.nbd.2020.104868
63. Croasdell Lucchini A, Gachanja NN, Rossi AG, Dorward DA, Lucas CD. Epithelial cells and inflammation in pulmonary wound repair. Cells. (2021) 10:1–17. doi: 10.3390/cells10020339
64. Crosby LM, Waters CM. Epithelial repair mechanisms in the lung. Am J Physiol Lung Cell Mol Physiol. (2010) 298:L715–31. doi: 10.1152/ajplung.00361.2009
65. Lamalice L, Le Boeuf F, Huot J. Endothelial cell migration during angiogenesis. Circ Res. (2007) 100:782–94. doi: 10.1161/01.RES.0000259593.07661.1e
66. de Perrot M, Imai Y, Volgyesi GA, Waddell TK, Liu M, Mullen JB, et al. Effect of ventilator-induced lung injury on the development of reperfusion injury in a rat lung transplant model. J Thorac Cardiovasc Surg. (2002) 124:1137–44. doi: 10.1067/mtc.2002.125056
67. Verleden SE, Von der Thusen J, Roux A, Brouwers ES, Braubach P, Kuehnel M, et al. When tissue is the issue: A histological review of chronic lung allograft dysfunction. Am J Transplant. (2020) 20:2644–51. doi: 10.1111/ajt.15864
68. Jiang X, Sung YK, Tian W, Qian J, Semenza GL, Nicolls MR. Graft microvascular disease in solid organ transplantation. J Mol Med (Berl). (2014) 92:797–810. doi: 10.1007/s00109-014-1173-y
69. Khan MA, Nicolls MR, Surguladze B, Saadoun I. Complement components as potential therapeutic targets for asthma treatment. Respir Med. (2014) 108:543–9. doi: 10.1016/j.rmed.2014.01.005
70. Khan MA. T regulatory cell mediated immunotherapy for solid organ transplantation: A clinical perspective. Mol Med. (2016) 22:892–904. doi: 10.2119/molmed.2016.00050
71. Knoedler L, Dean J, Diatta F, Thompson N, Knoedler S, Rhys R, et al. Immune modulation in transplant medicine: A comprehensive review of cell therapy applications and future directions. Front Immunol. (2024) 15:1372862. doi: 10.3389/fimmu.2024.1372862
72. Khan MA, Lau CL, Krupnick AS. Monitoring regulatory T cells as a prognostic marker in lung transplantation. Front Immunol. (2023) 14:1235889. doi: 10.3389/fimmu.2023.1235889
73. Khan MA, Alanazi F, Ahmed HA, Vater A, Assiri AM, Broering DC. C5a blockade increases regulatory T cell numbers and protects against microvascular loss and epithelial damage in mouse airway allografts. Front Immunol. (2018) 9:1010. doi: 10.3389/fimmu.2018.01010
74. Khan MA, Alanazi F, Ahmed HA, Al-Mohanna FH, Assiri AM, Broering DC. Foxp3+ Regulatory T cell ameliorates microvasculature in the rejection of mouse orthotopic tracheal transplants. Clin Immunol. (2017) 174:84–98. doi: 10.1016/j.clim.2016.11.011
75. Khan MA, Alanazi F, Ahmed HA, Shamma T, Kelly K, Hammad MA, et al. Ipsc-derived msc therapy induces immune tolerance and supports long-term graft survival in mouse orthotopic tracheal transplants. Stem Cell Res Ther. (2019) 10:290. doi: 10.1186/s13287-019-1397-4
76. King A, Balaji S, Le LD, Crombleholme TM, Keswani SG. Regenerative wound healing: the role of interleukin-10. Adv Wound Care. (2014) 3:315–23. doi: 10.1089/wound.2013.0461
77. Singampalli KL, Balaji S, Wang X, Parikh UM, Kaul A, Gilley J, et al. The role of an il-10/hyaluronan axis in dermal wound healing. Front Cell Dev Biol. (2020) 8:636. doi: 10.3389/fcell.2020.00636
78. Steen EH, Wang X, Balaji S, Butte MJ, Bollyky PL, Keswani SG. The role of the anti-inflammatory cytokine interleukin-10 in tissue fibrosis. Adv Wound Care (New Rochelle). (2020) 9:184–98. doi: 10.1089/wound.2019.1032
79. Hara M, Kingsley CI, Niimi M, Read S, Turvey SE, Bushell AR, et al. Il-10 is required for regulatory T cells to mediate tolerance to alloantigens in vivo. J Immunol. (2001) 166:3789–96. doi: 10.4049/jimmunol.166.6.3789
80. Hsu P, Santner-Nanan B, Hu M, Skarratt K, Lee CH, Stormon M, et al. Il-10 potentiates differentiation of human induced regulatory T cells via stat3 and foxo1. J Immunol. (2015) 195:3665–74. doi: 10.4049/jimmunol.1402898
81. Kassan M, Galan M, Partyka M, Trebak M, Matrougui K. Interleukin-10 released by cd4(+)Cd25(+) natural regulatory T cells improves microvascular endothelial function through inhibition of nadph oxidase activity in hypertensive mice. Arterioscler Thromb Vasc Biol. (2011) 31:2534–42. doi: 10.1161/ATVBAHA.111.233262
82. Wilkes DS. Airway hypoxia, bronchiolar artery revascularization, and obliterative bronchiolitis/bronchiolitis obliterans syndrome: are we there yet? Am J Respir Crit Care Med. (2010) 182:136–7. doi: 10.1164/rccm.201004-0508ED
83. Kraft BD, Suliman HB, Colman EC, Mahmood K, Hartwig MG, Piantadosi CA, et al. Hypoxic gene expression of donor bronchi linked to airway complications after lung transplantation. Am J Respir Crit Care Med. (2016) 193:552–60. doi: 10.1164/rccm.201508-1634OC
84. Guo S, Dipietro LA. Factors affecting wound healing. J Dent Res. (2010) 89:219–29. doi: 10.1177/0022034509359125
85. Delbove A, Senage T, Gazengel P, Tissot A, Lacoste P, Cellerin L, et al. Incidence and risk factors of anastomotic complications after lung transplantation. Ther Adv Respir Dis. (2022) 16:17534666221110354. doi: 10.1177/17534666221110354
86. Ta HQ, Kuppusamy M, Sonkusare SK, Roeser ME, Laubach VE. The endothelium: gatekeeper to lung ischemia-reperfusion injury. Respir Res. (2024) 25:172. doi: 10.1186/s12931-024-02776-4
87. Suresh MV, Balijepalli S, Solanki S, Aktay S, Choudhary K, Shah YM, et al. Hypoxia-inducible factor 1alpha and its role in lung injury: adaptive or maladaptive. Inflammation. (2023) 46:491–508. doi: 10.1007/s10753-022-01769-z
88. Bosch-Marce M, Okuyama H, Wesley JB, Sarkar K, Kimura H, Liu YV, et al. Effects of aging and hypoxia-inducible factor-1 activity on angiogenic cell mobilization and recovery of perfusion after limb ischemia. Circ Res. (2007) 101:1310–8. doi: 10.1161/CIRCRESAHA.107.153346
89. DeFreitas MR, McAdams HP, Azfar Ali H, Iranmanesh AM, Chalian H. Complications of lung transplantation: update on imaging manifestations and management. Radiol Cardiothorac Imaging. (2021) 3:e190252. doi: 10.1148/ryct.2021190252
90. Soetanto V, Grewal US, Mehta AC, Shah P, Varma M, Garg D, et al. Early postoperative complications in lung transplant recipients. Indian J Thorac Cardiovasc Surg. (2022) 38:260–70. doi: 10.1007/s12055-021-01178-1
91. Powers KA, Dhamoon AS. Physiology, pulmonary ventilation and perfusion. In: Statpearls. Treasure Island FL: StatPearls Publishing. (2025).
92. Nicolls MR, Dhillon GS, Daddi N. A critical role for airway microvessels in lung transplantation. Am J Respir Crit Care Med. (2016) 193:479–81. doi: 10.1164/rccm.201511-2117ED
93. Yun JJ, Unai S, Pettersson G. Lung transplant with bronchial arterial revascularization: review of surgical technique and clinical outcomes. J Thorac Dis. (2019) 11:S1821–S8. doi: 10.21037/jtd.2019.09.09
94. Kafi A, Taylor C, Cheng G. Airway complications related to lung transplantation. Curr Pulmonol Rep. (2024) 13:38–46. doi: 10.1007/s13665-024-00340-5
95. FitzSullivan E, Gries CJ, Phelan P, Farjah F, Gilbert E, Keech JC, et al. Reduction in airway complications after lung transplantation with novel anastomotic technique. Ann Thorac Surg. (2011) 92:309–15. doi: 10.1016/j.athoracsur.2011.01.077
96. Yamamoto T, Yoshida S, Nakajima T, Fujiwara T, Suzuki H, Iwata T, et al. Bronchoscopic assessment of bronchial anastomosis by visualizing local circulation status-index of hemoglobin (Ihb) imaging. J Thorac Dis. (2018) 10:2196–205. doi: 10.21037/jtd.2018.03.160
97. Ugur Chousein EG, Turan D, Vayvada M, Tanriverdi E, Tasci AE, Ozgul MA, et al. Management of airway complications following lung transplantation: first interventional bronchoscopy report from turkiye. Turk J Med Sci. (2024) 54:615–22. doi: 10.55730/1300-0144.5830
98. Flores K, Siques P, Brito J, Arribas SM. Ampk and the challenge of treating hypoxic pulmonary hypertension. Int J Mol Sci. (2022) 23:1–25. doi: 10.3390/ijms23116205
99. Guo M, Zhang M, Cao X, Fang X, Li K, Qin L, et al. Notch4 mediates vascular remodeling via erk/jnk/P38 mapk signaling pathways in hypoxic pulmonary hypertension. Respir Res. (2022) 23:6. doi: 10.1186/s12931-022-01927-9
100. Hu X, Li J, Fu M, Zhao X, Wang W. The jak/stat signaling pathway: from bench to clinic. Signal Transduct Target Ther. (2021) 6:402. doi: 10.1038/s41392-021-00791-1
101. Bravo-Reyna C, Zentella A, Ventura-Gallegos J, Torres-Villalobos G, Miranda-Galvan V, Alanis-Mendizabal J, et al. Experimental lung transplantation related with hif-1, vegf, ros. Assessment of hif-1alpha, vegf, and reactive oxygen species after competitive blockade of chetomin for lung transplantation in rats. Physiol Res. (2024) 73:809–17. doi: 10.33549/physiolres.935385
102. Kierans SJ, Taylor CT. Regulation of glycolysis by the hypoxia-inducible factor (Hif): implications for cellular physiology. J Physiol. (2021) 599:23–37. doi: 10.1113/JP280572
103. Kirito K. Regulation of erythropoiesis by hypoxia inducible factors (Hifs). Rinsho Ketsueki. (2011) 52:368–75. doi: 10.1016/j.blre.2012.12.003
104. Haase VH. Regulation of erythropoiesis by hypoxia-inducible factors. Blood Rev. (2013) 27:41–53. doi: 10.1016/j.blre.2012.12.003
105. Bos S, De Sadeleer LJ, Vanstapel A, Beeckmans H, Sacreas A, Yserbyt J, et al. Antifibrotic drugs in lung transplantation and chronic lung allograft dysfunction: A review. Eur Respir Rev. (2021) 30:338–56. doi: 10.1183/16000617.0050-2021
106. Park MS. Medical complications of lung transplantation. J Chest Surg. (2022) 55:338–56. doi: 10.5090/jcs.22.066
107. Harrell CR, Volarevic A, Djonov V, Arsenijevic A, Volarevic V. The role of micrornas in mesenchymal stem cell-based modulation of pulmonary fibrosis. Cell Transplant. (2024) 33:9636897241281026. doi: 10.1177/09636897241281026
108. Sziksz E, Pap D, Lippai R, Beres NJ, Fekete A, Szabo AJ, et al. Fibrosis related inflammatory mediators: role of the il-10 cytokine family. Mediators Inflammation. (2015) 2015:764641. doi: 10.1155/2015/764641
109. Frangogiannis N. Transforming growth factor-beta in tissue fibrosis. J Exp Med. (2020) 217:e20190103. doi: 10.1084/jem.20190103
110. Lacraz S, Isler P, Vey E, Welgus HG, Dayer JM. Direct contact between T lymphocytes and monocytes is a major pathway for induction of metalloproteinase expression. J Biol Chem. (1994) 269:22027–33. doi: 10.1016/S0021-9258(17)31750-7
111. Smith GN Jr., Mickler EA, Payne KK, Lee J, Duncan M, Reynolds J, et al. Lung transplant metalloproteinase levels are elevated prior to bronchiolitis obliterans syndrome. Am J Transplant. (2007) 7:1856–61. doi: 10.1111/j.1600-6143.2007.01850.x
112. Goumans MJ, Liu Z, ten Dijke P. Tgf-beta signaling in vascular biology and dysfunction. Cell Res. (2009) 19:116–27. doi: 10.1038/cr.2008.326
113. Weber J, Martins RS, Muslim Z, Baig MZ, Poulikidis K, Al Shetawi AH, et al. Anastomotic stenosis of bioengineered trachea grafts is driven by transforming growth factor B1-induced signaling, proinflammatory macrophages, and delayed epithelialization. JTCVS Open. (2023) 15:489–96. doi: 10.1016/j.xjon.2023.07.016
114. Santacruz JF, Mehta AC. Airway complications and management after lung transplantation: ischemia, dehiscence, and stenosis. Proc Am Thoracic Soc. (2009) 6:79–93. doi: 10.1513/pats.200808-094GO
115. Mora-Cuesta VM, Tello-Mena S, Izquierdo-Cuervo S, Iturbe-Fernández D, Sánchez-Moreno L, Ballesteros MA, et al. Bronchial stenosis after lung transplantation from cdcd donors using simultaneous abdominal normothermic regional perfusion: A single-center experience. Transplantation. (2023) 107:2415. doi: 10.1097/TP.0000000000004698
116. Bottero S, Meucci D, Trozzi M, Carotti A. Dehiscence of bronchial anastomosis after lung transplantation: A successful unconventional treatment. Ann Thorac Surg. (2018) 106:e81–e3. doi: 10.1016/j.athoracsur.2018.02.058
117. Brancato SK, Albina JE. Wound macrophages as key regulators of repair: origin, phenotype, and function. Am J Pathol. (2011) 178:19–25. doi: 10.1016/j.ajpath.2010.08.003
118. Chung AS, Ferrara N. Developmental and pathological angiogenesis. Annu Rev Cell Dev Biol. (2011) 27:563–84. doi: 10.1146/annurev-cellbio-092910-154002
119. Stellos K, Kopf S, Paul A, Marquardt JU, Gawaz M, Huard J, et al. Platelets in regeneration. Semin Thromb Hemost. (2010) 36:175–84. doi: 10.1055/s-0030-1251502
120. Nosbaum A, Prevel N, Truong HA, Mehta P, Ettinger M, Scharschmidt TC, et al. Cutting edge: regulatory T cells facilitate cutaneous wound healing. J Immunol. (2016) 196:2010–4. doi: 10.4049/jimmunol.1502139
121. Wilgus TA. Immune cells in the healing skin wound: influential players at each stage of repair. Pharmacol Res. (2008) 58:112–6. doi: 10.1016/j.phrs.2008.07.009
122. Larouche J, Sheoran S, Maruyama K, Martino MM. Immune regulation of skin wound healing: mechanisms and novel therapeutic targets. Adv Wound Care (New Rochelle). (2018) 7:209–31. doi: 10.1089/wound.2017.0761
123. MacLeod AS, Mansbridge JN. The innate immune system in acute and chronic wounds. Adv Wound Care (New Rochelle). (2016) 5:65–78. doi: 10.1089/wound.2014.0608
124. Tosello-Trampont A, Surette FA, Ewald SE, Hahn YS. Immunoregulatory role of nk cells in tissue inflammation and regeneration. Front Immunol. (2017) 8:301. doi: 10.3389/fimmu.2017.00301
125. Chen L, Deng H, Cui H, Fang J, Zuo Z, Deng J, et al. Inflammatory responses and inflammation-associated diseases in organs. Oncotarget. (2018) 9:7204–18. doi: 10.18632/oncotarget.23208
126. Kota DJ, Wiggins LL, Yoon N, Lee RH. Tsg-6 produced by hmscs delays the onset of autoimmune diabetes by suppressing th1 development and enhancing tolerogenicity. Diabetes. (2013) 62:2048–58. doi: 10.2337/db12-0931
127. Lee RH, Pulin AA, Seo MJ, Kota DJ, Ylostalo J, Larson BL, et al. Intravenous hmscs improve myocardial infarction in mice because cells embolized in lung are activated to secrete the anti-inflammatory protein tsg-6. Cell Stem Cell. (2009) 5:54–63. doi: 10.1016/j.stem.2009.05.003
128. Strbo N, Yin N, Stojadinovic O. Innate and adaptive immune responses in wound epithelialization. Adv Wound Care (New Rochelle). (2014) 3:492–501. doi: 10.1089/wound.2012.0435
129. Borges PA, Waclawiak I, Georgii JL, Fraga-Junior VDS, Barros JF, Lemos FS, et al. Adenosine diphosphate improves wound healing in diabetic mice through P2y12 receptor activation. Front Immunol. (2021) 12:651740. doi: 10.3389/fimmu.2021.651740
130. Perez-Aso M, Chiriboga L, Cronstein BN. Pharmacological blockade of adenosine A2a receptors diminishes scarring. FASEB J. (2012) 26:4254–63. doi: 10.1096/fj.12-209627
131. Mills CD. M1 and M2 macrophages: oracles of health and disease. Crit Rev Immunol. (2012) 32:463–88. doi: 10.1615/CritRevImmunol.v32.i6.10
132. Boehler A. The role of interleukin-10 in lung transplantation. Transplant Immunol. (2002) 9:121–4. doi: 10.1016/S0966-3274(02)00045-X
133. Chaudhry A, Samstein RM, Treuting P, Liang Y, Pils MC, Heinrich J-M, et al. Interleukin-10 signaling in regulatory T cells is required for suppression of th17 cell-mediated inflammation. Immunity. (2011) 34:566–78. doi: 10.1016/j.immuni.2011.03.018
134. Cobbold SP, Nolan KF, Graca L, Castejon R, Le Moine A, Frewin M, et al. Regulatory T cells and dendritic cells in transplantation tolerance: molecular markers and mechanisms. Immunol Rev. (2003) 196:109–24. doi: 10.1046/j.1600-065X.2003.00078.x
135. Brockmann L, Soukou S, Steglich B, Czarnewski P, Zhao L, Wende S, et al. Molecular and functional heterogeneity of il-10-producing cd4(+) T cells. Nat Commun. (2018) 9:5457–. doi: 10.1038/s41467-018-07581-4
136. Cobbold SP, Graca L, Lin CY, Adams E, Waldmann H. Regulatory T cells in the induction and maintenance of peripheral transplantation tolerance. Transpl Int. (2003) 16:66–75. doi: 10.1007/s00147-003-0545-y
137. van der Net JB, Bushell A, Wood KJ, Harden PN. Regulatory T cells: first steps of clinical application in solid organ transplantation. Transpl Int. (2016) 29:3–11. doi: 10.1111/tri.12608
138. Tang Q, Kang SM. Interpretation of transplant biopsies and immune responses following treg cell therapy. Curr Opin Organ Transplant. (2014) 19:616–20. doi: 10.1097/MOT.0000000000000132
139. Fontenot JD, Gavin MA, Rudensky AY. Foxp3 programs the development and function of cd4+Cd25+ Regulatory T cells. Nat Immunol. (2003) 4:330–6. doi: 10.1038/ni904
140. Hori S, Nomura T, Sakaguchi S. Control of regulatory T cell development by the transcription factor foxp3. Science. (2003) 299:1057–61. doi: 10.1126/science.1079490
141. Khattri R, Cox T, Yasayko SA, Ramsdell F. An essential role for scurfin in cd4+Cd25+ T regulatory cells. Nat Immunol. (2003) 4:337–42. doi: 10.1038/ni909
142. Murai M, Turovskaya O, Kim G, Madan R, Karp CL, Cheroutre H, et al. Interleukin 10 acts on regulatory T cells to maintain expression of the transcription factor foxp3 and suppressive function in mice with colitis. Nat Immunol. (2009) 10:1178–84. doi: 10.1038/ni.1791
143. Bharat A, Fields RC, Steward N, Trulock EP, Patterson GA, Mohanakumar T. Cd4 + 25+ Regulatory T cells limit th1-autoimmunity by inducing il-10 producing T cells following human lung transplantation. Am J Transplant. (2006) 6:1799–808. doi: 10.1111/j.1600-6143.2006.01383.x
144. Mohammad Afzal Khan FA, Ahmed HA, Hasan AF, Altuhami A, Assiri AM, Clemens D, et al. The therapeutic potential of treg cells in preserving microvascular health in a mouse model of orthotopic tracheal transplantation. J Clin Cell Immunol. (2016) 7:89. doi: 10.4172/2155-9899.C1.028
145. Sakaguchi S. Naturally arising foxp3-expressing cd25+Cd4+ Regulatory T cells in immunological tolerance to self and non-self. Nat Immunol. (2005) 6:345–52. doi: 10.1038/ni1178
146. Heim C, Khan MA, Motsch B, Gocht A, Ramsperger-Gleixner M, Stamminger T, et al. Microvascular integrity can be preserved by anti-platelet therapy and in combination with mtor inhibitor. J Heart Lung Transplant. (2017) 36:S376. doi: 10.1016/j.healun.2017.01.1069
147. Sakai R, Ito M, Komai K, Iizuka-Koga M, Matsuo K, Nakayama T, et al. Kidney gata3(+) regulatory T cells play roles in the convalescence stage after antibody-mediated renal injury. Cell Mol Immunol. (2021) 18:1249–61. doi: 10.1038/s41423-020-00547-x
148. Faustino LD, Griffith JW, Rahimi RA, Nepal K, Hamilos DL, Cho JL, et al. Interleukin-33 activates regulatory T cells to suppress innate gammadelta T cell responses in the lung. Nat Immunol. (2020) 21:1371–83. doi: 10.1038/s41590-020-0785-3
149. Rachmawati H, Beljaars L, Reker-Smit C, Bakker HI, Van Loenen-Weemaes AM, Lub-De Hooge MN, et al. Intravenous administration of recombinant human il-10 suppresses the development of anti-thy 1-induced glomerulosclerosis in rats. PDA J Pharm Sci Technol. (2011) 65:116–30.
150. Deng B, Wehling-Henricks M, Villalta SA, Wang Y, Tidball JG. Il-10 triggers changes in macrophage phenotype that promote muscle growth and regeneration. J Immunol. (2012) 189:3669–80. doi: 10.4049/jimmunol.1103180
151. Lopes RL, Borges TJ, Zanin RF, Bonorino C. Il-10 is required for polarization of macrophages to M2-like phenotype by mycobacterial dnak (Heat shock protein 70). Cytokine. (2016) 85:123–9. doi: 10.1016/j.cyto.2016.06.018
152. O’Garra A, Vieira PL, Vieira P, Goldfeld AE. Il-10–producing and naturally occurring cd4+ Tregs: limiting collateral damage. J Clin Invest. (2004) 114:1372–8. doi: 10.1172/JCI23215
153. Makita N, Hizukuri Y, Yamashiro K, Murakawa M, Hayashi Y. Il-10 enhances the phenotype of M2 macrophages induced by il-4 and confers the ability to increase eosinophil migration. Int Immunol. (2015) 27:131–41. doi: 10.1093/intimm/dxu090
154. Cypel M, Liu M, Rubacha M, Yeung JC, Hirayama S, Anraku M, et al. Functional repair of human donor lungs by il-10 gene therapy. Sci Trans Med. (2009) 1:4ra9–9. doi: 10.1126/scitranslmed.3000266
155. Hawrylowicz CM. Regulatory T cells and il-10 in allergic inflammation. J Exp Med. (2005) 202:1459–63. doi: 10.1084/jem.20052211
156. Tang-Feldman YJ, Lochhead GR, Lochhead SR, Yu C, Pomeroy C. Interleukin-10 repletion suppresses pro-inflammatory cytokines and decreases liver pathology without altering viral replication in murine cytomegalovirus (Mcmv)-infected il-10 knockout mice. Inflammation Res. (2011) 60:233–43. doi: 10.1007/s00011-010-0259-4
157. Niu J, Yue W, Song Y, Zhang Y, Qi X, Wang Z, et al. Prevention of acute liver allograft rejection by il-10-engineered mesenchymal stem cells. Clin Exp Immunol. (2014) 176:473–84. doi: 10.1111/cei.12283
158. Fischbein MP, Yun J, Laks H, Irie Y, Oslund-Pinderski L, Fishbein MC, et al. Regulated interleukin-10 expression prevents chronic rejection of transplanted hearts. J Thoracic Cardiovasc Surg. (2003) 126:216–23. doi: 10.1016/S0022-5223(03)00026-6
Keywords: lung transplant - ischemia-reperfusion injury, microvascular abnormalities, airway anastomosis, airway anastomotic complications, tissue repair and organ regeneration
Citation: Khan MA, Bhusal S, Lau CL and Krupnick AS (2025) Bronchial anastomotic complications as a microvascular disruption in a mouse model of airway transplantation. Front. Immunol. 16:1567657. doi: 10.3389/fimmu.2025.1567657
Received: 27 January 2025; Accepted: 23 April 2025;
Published: 14 May 2025.
Edited by:
Niv Pencovich, Sheba Medical Center, IsraelReviewed by:
Ashwin Ajith, Augusta University, United StatesThirugnanasambandan Sunder, Apollo Hospitals, India
Copyright © 2025 Khan, Bhusal, Lau and Krupnick. This is an open-access article distributed under the terms of the Creative Commons Attribution License (CC BY). The use, distribution or reproduction in other forums is permitted, provided the original author(s) and the copyright owner(s) are credited and that the original publication in this journal is cited, in accordance with accepted academic practice. No use, distribution or reproduction is permitted which does not comply with these terms.
*Correspondence: Mohammad Afzal Khan, bW9oYW1tYWQua2hhbkBzb20udW1hcnlsYW5kLmVkdQ==