- 1Center for Post-Doctoral Studies, Shandong University of Traditional Chinese Medicine, Jinan, China
- 2Clinical Medical Laboratory Center, Jining No.1 People’s Hospital, Jining, China
- 3Jining No.1 People’s Hospital, Shandong First Medical University, Jining, China
- 4Cheeloo College of Medicine, Shandong University, Jinan, China
- 5Second College of Clinical Medicine, Shandong University of Traditional Chinese Medicine, Jinan, China
- 6College of First Clinical Medicine, Shandong University of Traditional Chinese Medicine, Jinan, China
The tumor microenvironment (TME) is characterized by distinct metabolic adaptations that not only drive tumor progression but also profoundly influence immune responses. Among these adaptations, lactate, a key metabolic byproduct of aerobic glycolysis, accumulates in the TME and plays a pivotal role in regulating cellular metabolism and immune cell function. Tumor-associated macrophages (TAMs), known for their remarkable functional plasticity, serve as critical regulators of the immune microenvironment and tumor progression. Lactate modulates TAM polarization by influencing the M1/M2 phenotypic balance through diverse signaling pathways, while simultaneously driving metabolic reprogramming. Furthermore, lactate-mediated histone and protein lactylation reshapes TAM gene expression, reinforcing their immunosuppressive properties. From a therapeutic perspective, targeting lactate metabolism has shown promise in reprogramming TAMs and enhancing anti-tumor immunity. Combining these metabolic interventions with immunotherapies may further augment treatment efficacy. This review underscores the crucial role of lactate in TAM regulation and tumor progression, highlighting its potential as a promising therapeutic target in cancer treatment.
1 Introduction
The tumor microenvironment (TME) is a dynamic and metabolically distinct ecosystem comprising cancer cells, stromal cells, immune cells, extracellular matrix (ECM), and signaling molecules that collectively drive tumor progression and therapy resistance (1). Hypoxia, a hallmark of the TME, stabilizes hypoxia-inducible factors (HIFs), promoting angiogenesis and metabolic reprogramming to sustain tumor growth (2, 3). These metabolic adaptations are further complemented by immune evasion strategies, including the suppression of cytotoxic T cell activity and the polarization of tumor-associated macrophages (TAMs) toward an immunosuppressive phenotype (4). The metabolic mechanisms and functions of immune cells are illustrated in Figure 1. Meanwhile, cancer-associated fibroblasts (CAFs) drive ECM remodeling, promoting tumor invasion and metastasis by interacting with cellular metabolism (5, 6).
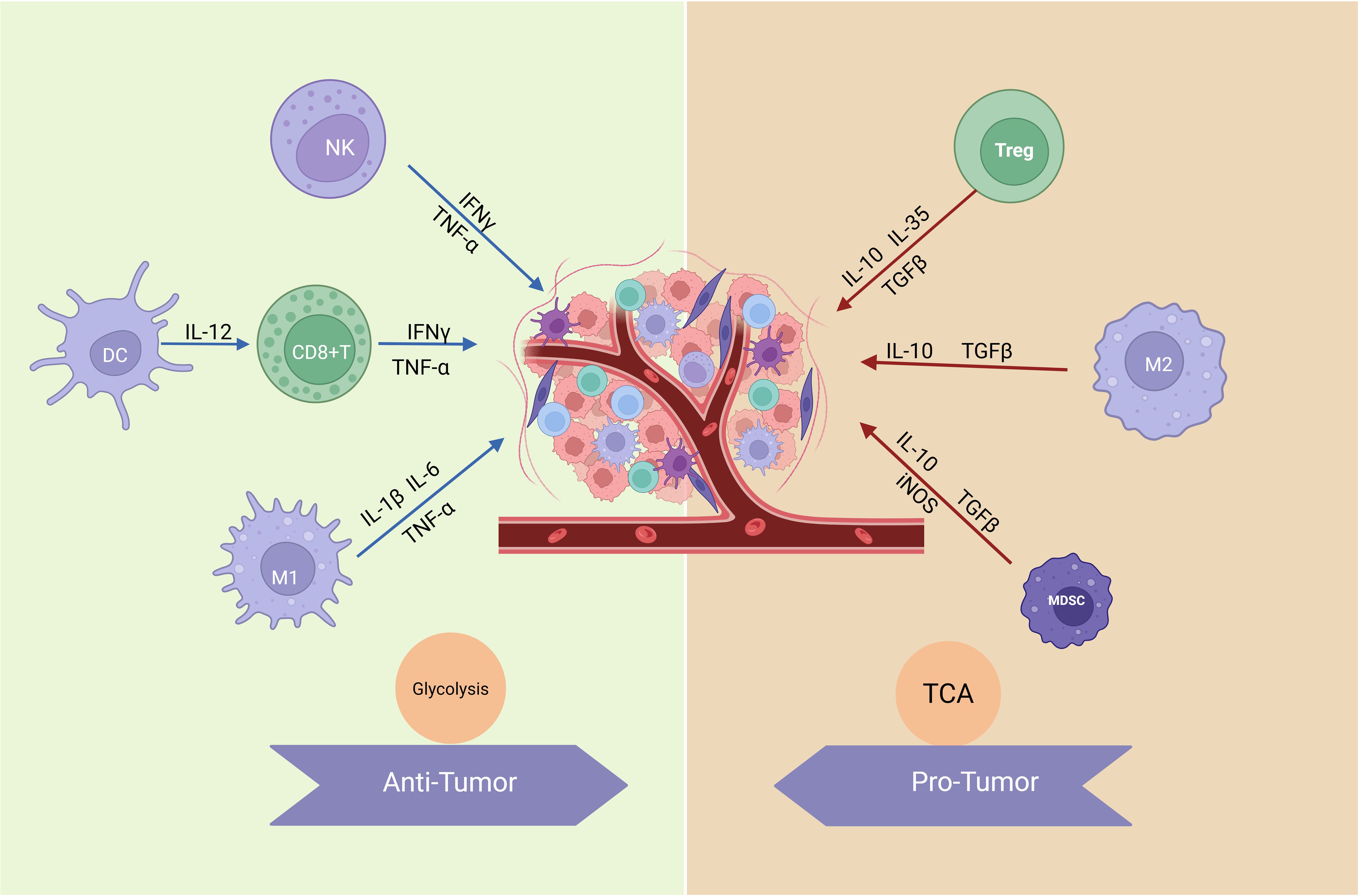
Figure 1. Metabolic mechanisms and functions of immune cells. Anti-tumor immune cells, such as effector T cells (including CD8+ T cells), NK cells, and M1-like macrophages, primarily rely on glycolysis for rapid energy supply to meet their high activity and quick response demands. Glycolysis not only supports ATP production but also provides intermediates for biosynthesis, enabling the secretion of pro-inflammatory cytokines like IFN-γ, IL-1β, and IL-6, which are crucial for tumor elimination and immune activation. In contrast, pro-tumor immune cells, including Tregs and M2-like macrophages, utilize OXPHOS as their dominant metabolic pathway. OXPHOS efficiently generates energy to sustain long-term immunosuppressive functions, facilitating the production of anti-inflammatory cytokines such as IL-10, TGF-β, and IL-35, which promote tumor progression and immune evasion. These metabolic adaptations reflect the functional specialization of immune cells within the TME. (Created with BioRender.com).
Central to these processes is the metabolic byproduct lactate, which accumulates in the TME due to the elevated glycolytic activity of cancer cells—a hallmark of the Warburg effect (7, 8). Beyond serving as a metabolic substrate, lactate acts as a potent signaling molecule, activating pathways such as HIF-1α, TGF-β, and NF-κB to promote angiogenesis, immune evasion, and ECM remodeling (9–11). The acidic microenvironment created by lactate accumulation inhibits the function of cytotoxic T cells and natural killer cells, thereby reinforcing immune suppression (12, 13). Simultaneously, lactate reprograms TAMs, shifting their balance from a pro-inflammatory M1 phenotype to an immunosuppressive, tumor-supportive M2 phenotype (14).
Macrophages are pivotal regulators within the TME due to their functional plasticity. Rather than being strictly categorized as M1 or M2, macrophages exist along a functional spectrum, with TAMs predominantly skewed toward an M2-like phenotype. These TAMs drive tumor progression through angiogenesis, immune suppression, and ECM remodeling (15–17). This reprogramming is mediated by Th2-skewing cytokines (e.g., IL-4, TGF-β1) and tumor-derived growth factors (e.g., CSF1, GM-CSF), fostering a microenvironment that supports tumor growth and metastasis (18). Moreover, TAMs suppress T cell activity by recruiting regulatory T cells via chemokines, inducing Foxp3+ iTreg cells through IL-10 and TGF-β, and depleting L-arginine via arginase I, which impairs TCR signaling (18). At one end of the spectrum, M1-like macrophages exhibit anti-tumor activity through mechanisms such as reactive oxygen species (ROS) and nitric oxide (NO) production or antibody-dependent cell-mediated cytotoxicity (ADCC) (19). However, during tumor progression, macrophages are dynamically reprogrammed by various factors in the TME, gradually shifting toward an M2-like phenotype, which perpetuates an immunosuppressive cycle and accelerates tumor development (20).
Lactate’s role in TAM reprogramming extends beyond its metabolic effects. Lactate drives histone and protein lactylation, an emerging post-translational modification that epigenetically reshapes TAM gene expression, enhancing their pro-tumoral and immunosuppressive functions (21, 22). For instance, lactate-induced lactylation of histones specifically activates genes associated with tissue repair and immunosuppression, reinforcing the M2-like phenotype (22). Concurrently, metabolic reprogramming of TAMs, orchestrated by lactate, promotes reliance on oxidative phosphorylation (OXPHOS) and the tricarboxylic acid (TCA) cycle, hallmarks of M2-like macrophage metabolism (9, 14, 23, 24). Given its multifaceted role in the TME, lactate represents an attractive therapeutic target for cancer treatment. Inhibitors targeting lactate dehydrogenase A (LDHA), a key enzyme in lactate production, and monocarboxylate transporters (MCTs), which mediate lactate transport, have shown promise in shifting TAMs along the functional spectrum from an immunosuppressive M2-like state toward a more pro-inflammatory M1-like state (25, 26). This reprogramming restores anti-tumor immunity and disrupts the immunosuppressive environment of the TME. Furthermore, combining lactate-targeting strategies with immunotherapies, such as PD-1/PD-L1 inhibitors, has demonstrated synergistic potential in preclinical studies by enhancing T cell infiltration and activity (27, 28).
This review explores the critical role of lactate in TAM polarization and tumor progression, highlighting its central position in the metabolic and immune landscape of the TME. Targeting lactate metabolism, particularly in conjunction with established immunotherapies, represents a promising strategy to overcome immune suppression, improve therapeutic outcomes, and ultimately reshape the TME for effective cancer treatment.
2 Lactate metabolism in the tumor microenvironment
2.1 Lactate production metabolism
Lactate production in the TME is primarily driven by two metabolic pathways, glycolysis and glutaminolysis, which are critical for sustaining tumor growth and progression (29). Tumor cells predominantly rely on aerobic glycolysis, known as the “Warburg effect,” converting glucose to lactate via LDHA, even in normoxic conditions (29). This process regenerates NAD+;, maintaining glycolytic flux and supporting rapid proliferation (30). The rate of glycolysis is tightly regulated by glycolytic enzymes through allosteric modulation, oncogenic signaling-driven expression changes, and post-translational modifications affecting activity, localization, and stability (31). HIF-1α plays a central role in regulating glycolysis and lactate production under hypoxic conditions by upregulating glycolytic genes such as Glut1, HK2, LDHA, and PKM2 (32). Hypoxia further enhances lactate production; however, knockout of HIF-1α significantly reduces extracellular acidification and abolishes hypoxia-induced lactate accumulation, highlighting its critical role in metabolic adaptation (30, 32).
Glutamine serves as a critical energy source in tumor cells, fueling metabolic pathways that contribute to lactate production. In parallel, glutaminolysis acts as an alternative pathway, where glutamine is converted to glutamate by glutaminase (GLS) and subsequently transformed into α-ketoglutarate (α-KG), which feeds into the TCA cycle. Through a series of enzymatic reactions, malate is generated within the cycle, exits the mitochondria, and is converted into pyruvate by malic enzyme. Pyruvate is subsequently converted into lactate, underscoring the metabolic flexibility of tumor cells and their dependence on glutamine for energy production and biosynthetic precursors. This pathway highlights the central role of glutamine metabolism in supporting tumor cell growth, survival, and adaptation under nutrient-limiting conditions (33–35). The molecular mechanisms underlying intracellular lactate production and export are depicted in Figure 2.
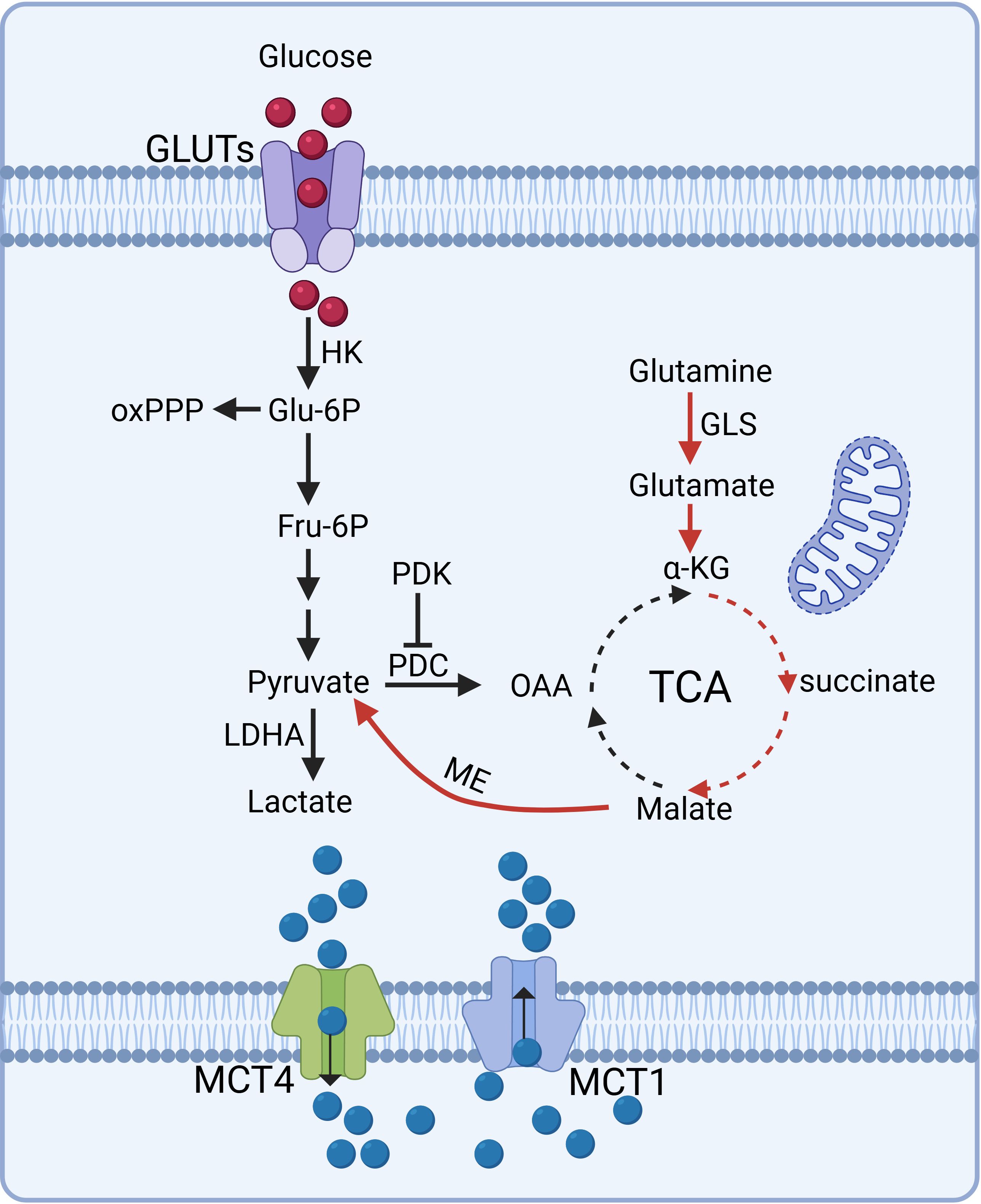
Figure 2. Molecular mechanisms of intracellular lactate production and export. Tumor cells predominantly rely on aerobic glycolysis, converting glucose into lactate via LDHA even in normoxic conditions, sustaining glycolytic flux and rapid proliferation. Glutaminolysis serves as an alternative pathway, where glutamine is metabolized into α-KG, feeding the TCA cycle and contributing to lactate production. Lactate transport is mediated by MCTs: MCT1 facilitates lactate influx or efflux depending on tissue type, while MCT4 predominantly drives lactate efflux in glycolytic or hypoxic cells. These pathways underscore tumor cells' metabolic flexibility and reliance on lactate dynamics for energy and biosynthesis. (Created with BioRender.com).
Besides tumor cells, CAFs and immune cells also contribute to lactate production within the TME. Metabolically reprogrammed CAFs exhibit enhanced glycolysis, generating lactate to support tumor growth (29). Similarly, immune cells such as macrophages and T cells undergo glycolytic reprogramming in the TME, further exacerbating lactate accumulation and shaping the metabolic landscape of the TME (14, 36–38).
2.2 Lactate secretion mechanism
In tumor cells, lactate transport is predominantly regulated by monocarboxylate transporters (MCTs), which are encoded by the SLC16 gene family (39). MCT1 is ubiquitously expressed, supporting lactate influx in oxidative tissues (e.g., heart, red muscle) and efflux in glycolytic cells. MCT2 is tissue-specific, found in liver, kidney, and neurons, facilitating lactate-driven gluconeogenesis and mitochondrial respiration. MCT3 is exclusively expressed in the eye, regulating subretinal pH. MCT4, with the lowest lactate affinity, primarily enables lactate efflux in glycolytic or hypoxic cells (e.g., white muscle, astrocytes), supporting metabolic exchanges between lactate-producing and -consuming cells, such as in skeletal muscles and the central nervous system (40). Among the MCT family, MCT1 and MCT4 (encoded by SLC16A1 and SLC16A3, respectively) are most relevant in cancer, facilitating lactate metabolism and pH homeostasis. Both are frequently upregulated in tumors such as brain, breast, cervix, and colorectal cancers, promoting progression (39–41). MCT1 is also essential for macrophage polarization and function. Knockdown of MCT1 significantly diminishes M2 macrophage polarization markers (42). MCTs facilitate the export of lactate and protons from glycolytic tumor cells and the import of lactate into oxidative tissues, supporting metabolic adaptation in tumors (41, 43).
MCT4 is predominantly upregulated under hypoxic conditions via HIF-1α, while MCT1 expression is driven by MYC, Nrf2 and epigenetic modifications (41, 43). These transporters enable lactate efflux, which creates an acidic microenvironment and promotes metabolic reprogramming essential for tumor growth and survival. Targeting MCT1 and MCT4 with small-molecule inhibitors has shown promise in disrupting tumor metabolism, though dual inhibition is required to effectively block lactate-driven tumor progression in hypoxic conditions (41, 44).
The functionality of MCT1 and MCT4 depends on their interaction with CD147 (Basigin), a transmembrane glycoprotein critical for MCT membrane localization (41, 45). CD147 binds MCT monomers through its transmembrane domain, with Glu218 serving as a key binding site (46, 47). This interaction is essential for glycolysis, ATP production, and tumor cell proliferation (45, 46). In CD147-deficient mice, the absence of MCT expression in retinal cells disrupts lactate transport, leading to energy depletion and visual impairment (45, 48). The interplay between MCTs and CD147 is fundamental to lactate metabolism and tumor adaptation, emphasizing the significance of lactate transport in cancer progression.
3 Functional polarization and metabolic reprogramming of macrophages within the tumor microenvironment
Macrophages exhibit a dynamic spectrum of phenotypes, ranging from M1 macrophages, to anti-inflammatory M2 macrophages, including subtypes M2a, M2b, and M2c. Intermediate phenotypes, such as the transitional M0 state, further enrich this continuum (49). Microenvironmental signals dynamically regulate macrophage polarization, driving gradual transitions across this functional and metabolic continuum (50). Classically activated M1 macrophages are skewed toward anti-tumor immune responses. Induced by lipopolysaccharide (LPS) and interferon-gamma (IFN-γ), M1 macrophages recognize tumor cells through surface antigens and execute their functions by producing reactive oxygen species (ROS), inducible nitric oxide synthase (iNOS) coupled with the production of pro-inflammatory cytokines (51–53). They secrete key inflammatory mediators such as interleukin-1β (IL-1β), interleukin-6 (IL-6), TNF and interleukin-12 (IL-12), driving strong pro-inflammatory responses to combat tumors (53). In contrast, alternatively activated M2 macrophages, stimulated by IL-4, IL-10, or IL-13, perform immunosuppressive and tissue-repair roles (53, 54). M2 macrophages are anti-inflammatory, characterized by low antigen-presentation capability, reduced IL-12 secretion, and high production of IL-10, IL-4, and arginase-1 (Arg-1), which suppress inflammation and promote tissue remodeling (53, 54). Their functional diversity is reflected in subtypes including M2a, M2b, and M2c, which contribute to immune regulation and homeostasis (52, 55). M1 and M2 macrophages contribute complementarily to immune balance and the response to pathological conditions. Notably, these macrophage states form a functional continuum rather than distinct, opposing categories.
The functional divergence between M1 and M2 macrophages is intricately linked to their metabolic reprogramming. Typically, M1 macrophages undergo a metabolic transition from oxidative phosphorylation (OXPHOS) to glycolysis, accompanied by an upregulated pentose phosphate pathway (56). This metabolic reprogramming leads to the accumulation of key metabolites, including succinate, citrate, and itaconate, while simultaneously disrupting the TCA cycle (57). Succinate stabilizes HIF-1α, promoting glycolytic gene transcription, while citrate and itaconate contribute to the production of inflammatory mediators such as IL-1β, nitric oxide, and prostaglandins (53, 58). The pentose phosphate pathway (PPP), plays a crucial role in cytokine production, redox homeostasis, and biosynthesis, supporting M1-driven inflammation (57, 59). Conversely, M2 macrophages rely on an intact TCA cycle to meet ATP demands for UDP-GlcNAc-dependent glycosylation, which is critical for lectin and mannose receptor functions (56, 57). Their metabolism is dominated by OXPHOS and FAO, with the TCA cycle and glutaminolysis providing substrates for the electron transport chain and sustaining oxygen consumption (57). Glutamine metabolism is essential in M2 macrophages, as it replenishes TCA cycle intermediates and provides nitrogen for UDP-GlcNAc synthesis (56). Glycolipid metabolism further regulates M2 polarization, while mitochondrial biogenesis, marked by increased expression of mitochondrial transcription factor A (TFAM) and cytochrome c oxidase subunit 1 (Cox-1), enhances their ability to mediate anti-inflammatory responses and tissue repair (53, 58). This metabolic flexibility highlights the continuous spectrum of macrophage polarization, driven by intrinsic and extrinsic signals, including lactate and tumor-derived metabolites. Lactate plays a central role in shaping macrophage phenotypes by modulating metabolic pathways and cellular signaling, thereby influencing immune responses. Understanding these mechanisms provides critical insights into immune regulation and offers promising opportunities for therapeutic interventions, particularly in cancer treatment.
Given the distinct metabolic requirements of M1 and M2 macrophages, targeting their metabolism offers a promising strategy to regulate polarization. Inhibiting glycolysis, either by activating pyruvate kinase M2 (PKM2) or suppressing pyruvate dehydrogenase kinase 1 (PDK1), has been shown to impair M1 polarization (58, 60, 61). Similarly, silencing CXCR4 inhibits glycolysis and facilitates M2 to M1 transition, highlighting a potential metabolic switch (62). In tumor-bearing mice, combining the glycolysis inhibitor 2-DG with radiation therapy restored M1 phenotypes and reduced tumor burden, demonstrating the therapeutic potential of metabolic reprogramming in the TME (63).
4 Effects of lactate on macrophage metabolic reprogramming
Lactate plays a dynamic and context-dependent role in macrophage polarization, influencing the balance along the functional spectrum between pro-inflammatory M1 and anti-inflammatory M2 phenotypes (25, 64). The effects and mechanisms of lactate on macrophage polarization are illustrated in Figure 3. High concentrations of lactate in macrophages treated with LPS induce a time- and dose-dependent shift from M1 to M2 polarization, as indicated by elongated cell morphology, increased Arg1 expression, and reduced CD86 and iNOS expression (65). Overexpression of LDHA or supplementation with exogenous lactate further promotes M2 polarization by upregulating markers like IL-10 and Arg1 while suppressing M1 markers such as TNF and IFN-γ (25). Conversely, deleting LDHA in macrophages drives M1 polarization, suppresses VEGF expression and angiogenesis, and enhances effector CD8+ T-cell activity (66). Targeting lactate metabolism through the inhibition of lactate efflux reduces M2 macrophage populations, increases CD8+ T cell infiltration, and improves the efficacy of anti-PD1 therapy in pancreatic tumors (67). Interestingly, in bone marrow-derived macrophages (BMDMs) treated with IL-4 or IFN-γ/LPS, lactate suppresses M2-associated genes (Arg1, Cd163, Cd206, Il-10) and markers (e.g., Arg1, IL-10), while enhancing M1-associated genes (Nos, TNF, IL-12) and markers (e.g., TNF, iNOS) (24). This switch decreases CD206+; F4/80+; M2 macrophages while increasing CD86+; F4/80+; M1 macrophages, accompanied by distinct morphological changes (24).
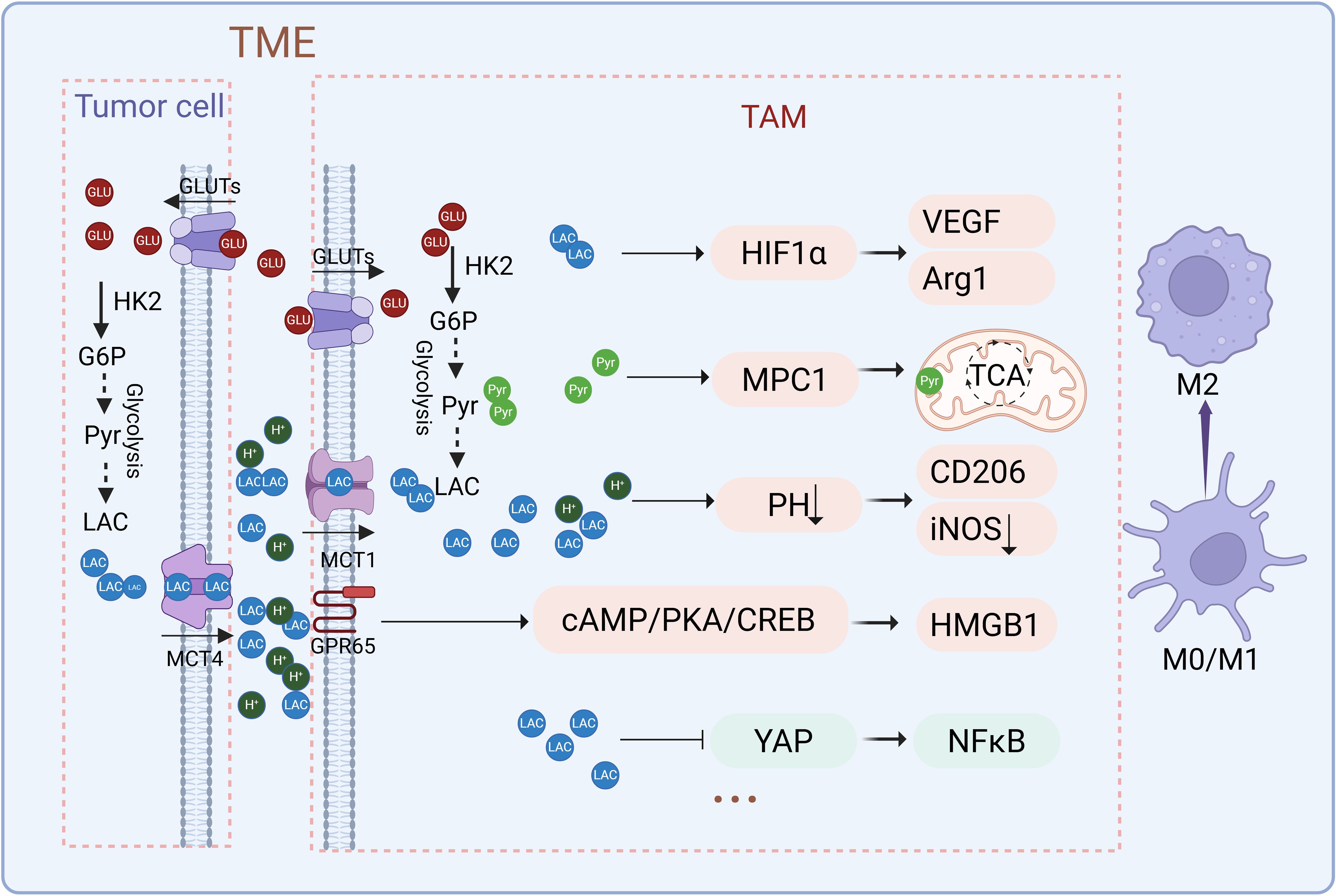
Figure 3. Effects and mechanisms of lactate on macrophage polarization. Lactate regulates macrophage polarization through multiple mechanisms, including the stabilization of HIF-1α, modulation of NF-κB signaling, activation of lactate receptors GPR65, and pH-dependent effects. These pathways converge to upregulate key factors such as Arg1, VEGF, and CD206, collectively driving the transition toward an immunosuppressive M2 phenotype. (Created with BioRender.com).
Lactate export from tumor cells is proton-coupled, leading to its accumulation alongside intratumoral acidification. This acidification alters the microenvironment’s pH, favoring distinct macrophage phenotypes: a neutral pH (7.4) tends to influence macrophages toward a more pro-inflammatory, M1-like state with elevated iNOS expression, while an acidic pH (6.7) favors an anti-inflammatory, M2-like functional state, characterized by increased CD206 expression and reduced iNOS levels (64). TAMs sense tumor-derived lactate and microenvironment acidification through GPR132 and GPR65 in a non-redundant manner, driving M2 polarization (68). Lactate activates GPR65 on TAMs, triggering the cAMP-PKA-CREB signaling cascade to promote HMGB1 secretion, thereby facilitating glioma progression (69). Melanomas, with heightened glycolytic activity, produce excessive lactate, leading to significant TME acidification. This acidic environment predominantly drives TAMs toward M2-like polarization via GPR65, upregulating key genes such as Arg1 and VEGF. Notably, knocking out GPR65 abolishes this effect, highlighting its critical role in macrophage regulation (70). Additionally, studies have shown that an acidic pH (6.8), even independent of lactate, can induce anti-inflammatory macrophage polarization by upregulating markers like Arg1 and CD206 while suppressing pro-inflammatory markers such as NOS2 and IL-6. Neutralizing tumor acidity has been demonstrated to mitigate the pro-tumor phenotypes of TAMs, emphasizing the pivotal role of pH in macrophage polarization and tumor progression (71).
Lactate exerts its regulatory effects on macrophages through distinct signaling pathways, including HIF-1α and NF-κB (72, 73). Tumor-derived lactate, absorbed by macrophages through monocarboxylate transporters (MCT1–4), activates HIF-1α, which subsequently upregulates the expression of vascular endothelial growth factor (VEGF) and arginase-1 (Arg1) (73). This activation promotes an immunosuppressive, M2-like phenotype that supports tumor progression. Inhibiting MCTs blocks lactate uptake and downstream signaling, while genetic deletion of HIF-1α abolishes lactate-induced polarization, underscoring HIF-1α’s central role in lactate-driven metabolic reprogramming (73). In addition to HIF-1α, lactate regulates macrophage polarization through the NF-κB pathway. NF-κB plays a crucial role in M1 polarization by promoting the synthesis of pro-inflammatory cytokines (72). LPS stimulation enhances NF-κB activation via interactions with Yes-associated protein (YAP) and the NF-κB subunit p65, amplifying inflammatory responses (72). However, lactate suppresses this interaction by inhibiting YAP activation, reducing NF-κB nuclear translocation, and lowering pro-inflammatory cytokine production. This inhibition dampens M1 polarization while promoting an immunosuppressive environment (72). Elevated lactate levels also enhance macrophage polarization by activating NF-κB, leading to increased PD-L1 expression, which facilitates immune evasion and tumor progression (74).
Lactate supports mitochondrial oxidative metabolism in IL-4-induced M2 macrophages by serving as a substrate for the TCA cycle (23). Lactate, absorbed by macrophages and converted to pyruvate, enters the TCA cycle through mitochondrial pyruvate carrier 1 (MPC1) (23). Blocking MPC1 significantly reduces IL-4-induced M2 polarization and associated gene expression, highlighting the dependence of M2 macrophages on mitochondrial metabolism (23). Both glucose- and lactate-derived mitochondrial pyruvate are critical for M2 polarization and their immunosuppressive functions, including suppressing CD8+; T cell proliferation and IFN-γ production (23). TCA cycle intermediates also influence macrophage polarization. Succinate, elevated in macrophages upon LPS stimulation, stabilizes HIF-1α and promotes IL-1β production by inhibiting prolyl hydroxylase (PHD). This stabilization enhances M1 polarization and inflammatory responses, contrasting with lactate’s effects on M2 polarization (57, 74).
In summary, lactate has a complex role in macrophage polarization, influencing processes such as altering microenvironmental pH, activating key signaling pathways like HIF-1α and NF-κB, and supporting mitochondrial metabolism in M2 macrophages. It primarily fosters an immunosuppressive phenotype, which in turn promotes tumor progression. However, its effects are dynamic and context-dependent. As a crucial factor in the TME, further investigation into lactate’s mechanisms could provide valuable insights and reveal new therapeutic targets for cancer treatment.
5 Lactate regulates macrophage polarization through lactylation modification
5.1 Histone lactylation
Histone modifications are critical regulators of chromatin structure, gene expression, DNA replication, and repair, thereby maintaining cellular homeostasis and genomic stability (21). Among these, histone lactylation (Kla) has emerged as a novel and significant epigenetic modification influencing macrophage polarization and immune responses. In 2019, Zhao et al. discovered lysine lactylation (Kla) as a distinct histone modification originating from lactate. Through HPLC-MS/MS, synthetic peptides, pan anti-Kla antibodies, and isotopic labeling, they validated the lactate-derived nature of Kla and identified numerous Kla sites in human MCF-7 cells and mouse macrophages (22). During M1 macrophage polarization, elevated lactate production via aerobic glycolysis correlates with increased histone Kla levels and decreased histone acetylation (Kac). Notably, histone Kla specifically promotes the late-phase expression of M2-like homeostatic genes, such as Arg1, without affecting early pro-inflammatory gene expression. This epigenetic regulation is mediated by p300, which acts as a potential Kla writer (22). Additionally, MCT4 deficiency enhances H3K18la lactylation at reparative gene loci (e.g., IL-10, PDHA1), promoting their transcription. Unlike other modifications such as methylation or acetylation, lactylation dominates during the transition to M2 macrophages, linking metabolic shifts to epigenetic remodeling and macrophage function (75). Furthermore, lactate enhances CCL18 expression in macrophages through GPR132-mediated H3K18 lactylation, selectively inducing the transcriptional activation of the CCL18 promoter, thereby linking histone lactylation to macrophage-mediated immune responses (76).
Therapeutically, targeting histone lactylation has shown promise in modulating macrophage polarization. For instance, VB124 and MD-43, inhibitors of monocarboxylate transporter 4 (MCT4), enhance H3K18la levels through p300, driving macrophages toward a reparative phenotype characterized by increased anti-inflammatory and TCA cycle gene expression and reduced pro-inflammatory and glycolysis gene expression (75). These compounds effectively decrease macrophage content in plaques and slow atherosclerosis progression in high-fat diet-fed ApoeKO mice. MD-43, in particular, selectively degrades MCT4 via the UPS pathway, further amplifying H3K18la enrichment at reparative gene promoters, highlighting its therapeutic potential (75). Additionally, mitochondrial fragmentation in macrophages during inflammatory activation enhances lactate production and histone lactylation, driven by decreased PDH expression and increased pyruvate-to-lactate conversion. This metabolic shift promotes the expression of reparative genes like Arg1 via histone lactylation, linking mitochondrial dynamics to macrophage polarization and tissue repair (77). Dysregulated glycolysis and MCT1-mediated lactate transport further regulate histone lactylation through IL-1β-dependent recruitment of GCN5, enhancing the transcription of reparative genes such as Lrg1, Vegf-a, and IL-10, which foster anti-inflammatory responses and pro-angiogenic activities (78).
5.2 Non-histone lactylation
Besides histones, lactylation also occurs on non-histone proteins, underscoring its broader regulatory roles in cellular functions such as gene expression, DNA repair, cell cycle regulation, signaling, and metabolism (21). Recent studies have uncovered its critical roles in diverse biological processes, including macrophage polarization and protozoan metabolism. For example, exercise-induced lactylation of methyl-CpG-binding protein 2 (MeCP2) at K271 promotes M2 macrophage polarization, enhances plaque stability, and suppresses RUNX1 transcription, showcasing the therapeutic potential of lactylation in treating atherosclerosis (79). Similarly, in Trypanosoma brucei, lysine lactylation extends beyond histones, with 387 sites identified in 257 non-histone proteins. Regulated by glucose metabolism through a unique lactate pathway, non-histone lactylation in T. brucei plays essential roles in energy metabolism and gene expression, uncovering novel regulatory mechanisms in early-branching eukaryotes (80).
Lactylation also regulates key metabolic enzymes. Lactate promotes the K62 lactylation of pyruvate kinase M2 (PKM2), enhancing its activity and driving the transition of LPS-induced macrophages from a pro-inflammatory state to a reparative phenotype. The K62R mutant of PKM2 abolishes lactate’s regulatory effects on both PKM2 activity and macrophage phenotype transition, underscoring the essential role of K62 lactylation in these processes (81). Similarly, inhibition of mitochondrial pyruvate carrier 1 (MPC1) prevents pyruvate entry into mitochondria, leading to elevated lactate production and increased FASN K673 lactylation. This modification reduces lipid accumulation in hepatocytes, suppresses inflammatory cytokine expression, and facilitates M2 macrophage polarization, fostering an anti-inflammatory environment (82). Figure 4 illustrates how lactate regulates macrophage polarization through lactylation modification, highlighting a key regulatory mechanism.
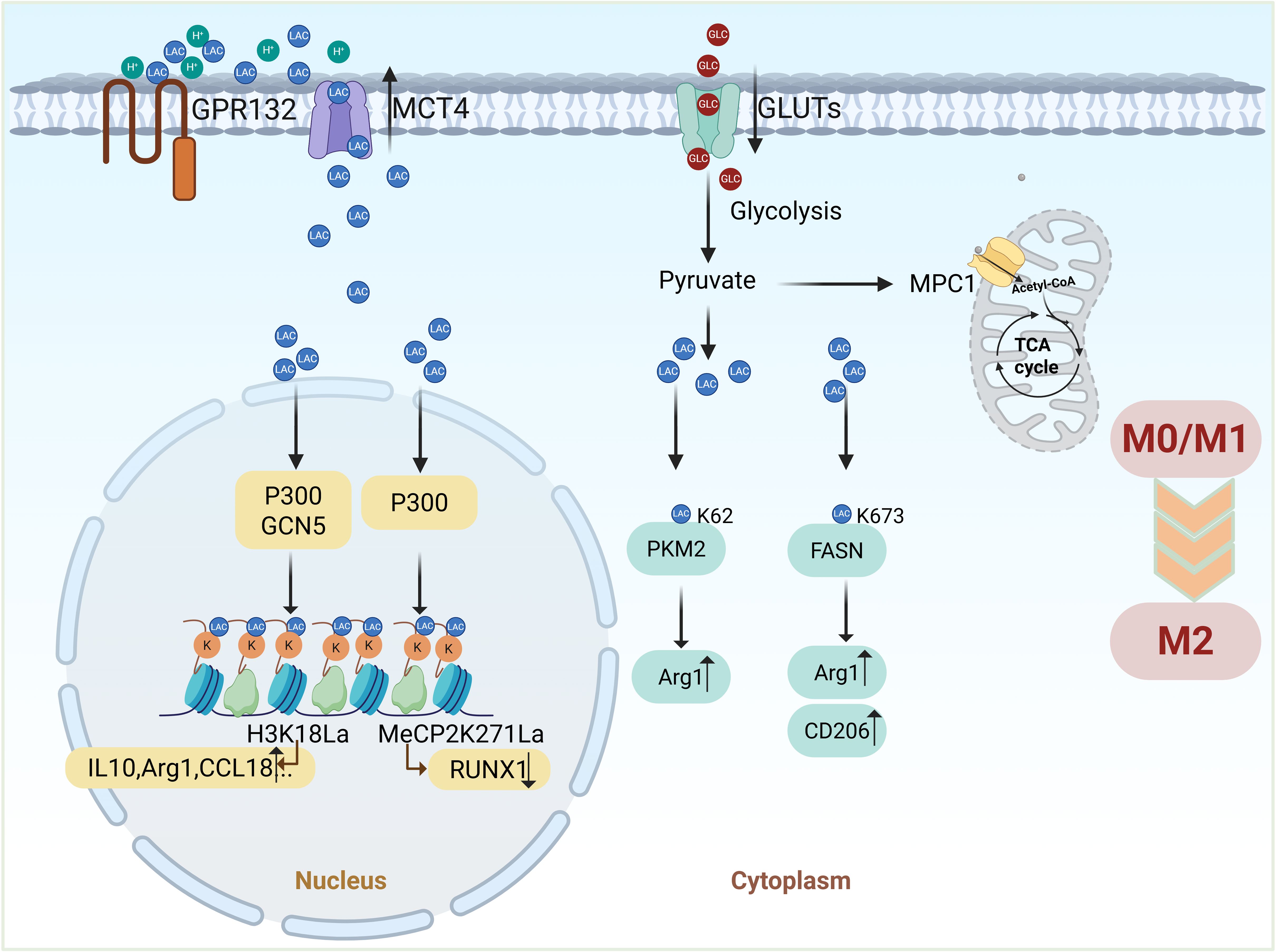
Figure 4. Lactate regulates macrophage polarization through lactylation modification. Lactate-driven lactylation connects metabolism to macrophage polarization by modifying histones, non-histone proteins, and metabolic enzymes. Histone lactylation (Kla) promotes the expression of M2-related genes (e.g., Arg1), while non-histone lactylation (e.g., MeCP2) and modifications of metabolic enzymes (e.g., PKM2, FASN) further facilitate the transition to an anti-inflammatory M2 phenotype. (Created with BioRender.com).
6 Targeting lactate and lactylation in macrophage metabolic reprogramming for cancer therapy
6.1 Therapeutic potential of targeting lactate
Lactate is crucial in driving tumor progression by promoting immune suppression and reshaping the TME. Tumor-derived lactate, transported into macrophages via MCT1, not only stabilizes HIF-1α but also induces histone lactylation, facilitating macrophage polarization independently of endogenous lactate or MPC-mediated metabolism (83). Beyond macrophage polarization, lactate suppresses T cell proliferation, cytokine production, and cytotoxicity, while inducing apoptosis and inhibiting NK cell and type 2 innate lymphoid cells (ILC2) activity through intracellular acidification (36, 83). Moreover, histone lactylation caused by lactate recruits CAFs, TIMs, and CSCs, further remodeling the TME to support tumor progression and metastasis (84). In prostate cancer, lactate activates KIAA1199 via HIF-1α-mediated lactylation, driving angiogenesis, vasculogenic mimicry, and hyaluronic acid depolymerization, ultimately accelerating tumor progression (85). Importantly, lactate-induced macrophage polarization and dynamic changes in the TME are interdependent, forming a positive feedback loop that synergistically fosters immune suppression and tumor progression. These multifaceted roles highlight lactate’s therapeutic potential. By targeting key metabolic enzymes (e.g., LDHA, HK2) or transporters (e.g., MCTs, GLUTs), lactate production and transport can be disrupted, reprogramming macrophages and alleviating immune suppression to enhance antitumor immunity. Table 1 provides a comprehensive summary of lactate-associated therapeutic targets and their corresponding inhibitors in cancer therapy.
6.1.1 Hexokinase 2
HK2 catalyzes the first committed step of glycolysis, phosphorylating glucose to glucose-6-phosphate (86). In cancer cells, HK2 is often overexpressed, driving the Warburg effect by promoting elevated glycolytic flux and lactate production even under aerobic conditions (86). This metabolic reprogramming supports tumor growth by providing energy, biosynthetic precursors, and an acidic microenvironment that suppresses immune responses. Targeting HK2 has shown significant promise in disrupting cancer metabolism. HK2 inhibitors reduce glycolysis, lower lactate production, and normalize the TME by decreasing acidity and promoting immune activation (86, 87). Preclinical studies report that HK2 inhibition not only impairs tumor growth but also enhances TAM polarization toward the M1 phenotype, boosting anti-tumor immunity. Furthermore, combining HK2 inhibitors with other therapies, such as immune checkpoint inhibitors, offers synergistic benefits, amplifying therapeutic efficacy by exploiting cancer-specific metabolic vulnerabilities (87).
6.1.2 Lactate dehydrogenase A
LDHA, a central enzyme in lactate metabolism, facilitates the conversion of pyruvate to lactate while regenerating NAD+;, a crucial cofactor for glycolysis (88). Although LDHA activity is typically elevated under hypoxic conditions, cancer cells exhibit aberrant overexpression of LDHA even in normoxic environments (89). This metabolic shift promotes excessive lactate accumulation, which acidifies the TME, suppresses anti-tumor immune responses, and fosters angiogenesis, ECM remodeling, and metastasis (88, 90, 91). Importantly, LDHA activity directly influences TAM polarization within the TME. High lactate levels favor M2-like polarization, linking LDHA overexpression to both metabolic and immune suppression in cancer. Clinically, LDHA represents a compelling therapeutic target. LDHA inhibition reduces lactate production, alleviates TME acidification, and reprograms TAMs toward a pro-inflammatory M1 phenotype. This shift boosts anti-tumor immunity and suppresses tumor progression (25). Preclinical studies have demonstrated that LDHA inhibitors effectively reduce tumor growth, metastasis, and therapy resistance, making LDHA a central nexus in cancer metabolism and immune modulation (12, 92–94).
6.1.3 Monocarboxylate transporters
MCTs, particularly MCT1 and MCT4, are integral to lactate transport in cancer cells. MCT1 facilitates lactate and pyruvate uptake, while MCT4 mediates lactate export, maintaining intracellular pH homeostasis and sustaining glycolytic flux (95). In the TME, these transporters enable cancer cells to efficiently shuttle lactate, creating an acidic microenvironment that promotes immune evasion, angiogenesis, and tumor progression.
Targeting MCTs represents a promising strategy to disrupt lactate dynamics in tumors. Inhibitors such as AZD3965 (MCT1-specific) and syrosingopine (dual MCT1/MCT4) block lactate transport, inducing metabolic stress and enhancing the efficacy of immunotherapies (44, 95, 96). Preclinical studies have demonstrated the potential of MCT inhibitors in reducing tumor growth and sensitizing cancer cells to existing treatments (96). However, challenges like tumor heterogeneity and compensatory metabolic pathways emphasize the need for combination therapies to maximize the therapeutic impact of MCT inhibition.
6.1.4 Glucose Transporters
GLUTs, especially GLUT1, are crucial regulators of glucose uptake in cancer cells, fueling glycolysis and subsequent lactate production (97). Overexpression of GLUT1 and other isoforms, such as GLUT3, is a common feature in tumors, supporting elevated glycolytic flux to provide ATP and biosynthetic precursors for rapid cell proliferation (97, 98). Lactate accumulation driven by GLUT-mediated glycolysis further contributes to immune evasion and metastatic potential by creating an acidic TME. Therapeutically, GLUT inhibition offers a viable approach to target tumor metabolism. Small-molecule inhibitors such as WZB117 and BAY-876 effectively reduce glucose uptake, suppress glycolysis, and impair tumor growth in preclinical models (98–100). Moreover, GLUT inhibition has been shown to enhance the efficacy of chemotherapy, radiotherapy, and immunotherapy by disrupting cancer cell metabolic flexibility (100, 101). These findings indicate the potential of GLUT inhibitors as a complementary strategy in cancer treatment.
6.1.5 Pyruvate dehydrogenase kinases
Pyruvate dehydrogenase kinases (PDKs) are key regulators of the conversion of pyruvate to acetyl-CoA by modulating the activity of the pyruvate dehydrogenase complex (PDC), thereby governing its entry into the TCA cycle (102, 103). By phosphorylating and inactivating PDC, PDKs shift metabolism toward glycolysis. The overexpression of PDKs, especially PDK1 and PDK3, has been linked to unfavorable clinical outcomes and resistance to therapeutic interventions across multiple cancer types. PDKs also play a significant role in macrophage metabolism (61, 104). M1 macrophages exhibit glycolytic reprogramming similar to the Warburg effect, with PDK1 essential for the expression of M1-specific markers (22, 105). Pharmacological inhibition of PDKs, such as with dichloroacetate (DCA), restores PDC activity, redirects pyruvate metabolism toward oxidative phosphorylation, and reduces glycolytic flux. This metabolic reprogramming triggers pyroptosis in tumor cells and potentiates the effectiveness of immunotherapeutic approaches (106, 107). These findings position PDK inhibitors as promising agents to modulate both tumor metabolism and immune cell function.
6.2 Therapeutic potential of targeting lactylation
In the process of macrophage polarization, lactylation shows the ability to balance inflammatory reactions and tissue regeneration, exhibiting dual functions. In M1 macrophages, increased histone lactylation during the late polarization phase activates homeostatic genes essential for wound healing, contributing to immune homeostasis and tissue regeneration (22). This dual regulatory function highlights the therapeutic potential of targeting lactylation to modulate macrophage activity, offering promising avenues for treating inflammatory diseases and cancer.
In the context of cancer, lactylation has been implicated in therapy resistance by regulating DNA repair mechanisms. MRE11, a key protein in homologous recombination (HR), undergoes lactylation at lysine 673 (K673), a modification mediated by CBP acetyltransferase and regulated by ATM. Lactylation enhances MRE11’s DNA-binding ability, facilitating HR-mediated DNA repair. Inhibiting CBP or LDH reduces MRE11 lactylation, impairs HR activity, and sensitizes tumor cells to cisplatin and PARP inhibitors, demonstrating the therapeutic relevance of targeting lactylation in cancer treatment (108). Similarly, in glioblastoma stem cells, ALDH1A3-driven pyruvate kinase activation promotes XRCC1 lactylation-mediated DNA repair and resistance to chemoradiotherapy. Targeting this pathway with the ALDH1A3 inhibitor D34–919 reverses resistance, underscoring the potential of lactylation-related interventions in glioblastoma therapy (109).
The discovery of lactylation-regulating enzymes, including P300, AARS1, CBP, and YiaC, has deepened our understanding of the functional roles of lactylation (21, 110–112). In macrophages, lactylation influences the expression of profibrotic genes through histone modifications, as evidenced by reduced gene expression following P300 knockdown (113). Beyond macrophages, lactylation can impair tumor suppressor activity, such as the lactylation of p53 mediated by tumor-derived L-lactate via AARS1. This modification weakens p53’s DNA-binding ability and tumor-suppressive functions, while β-alanine prevents p53 lactylation, enhancing chemotherapy efficacy (110). These findings highlight the therapeutic potential of targeting lactylation-regulating enzymes to modulate immune responses and overcome cancer therapy resistance.
In a word, lactylation-regulated macrophage polarization represents a critical mechanism at the intersection of immune modulation and cancer therapy. By influencing macrophage function and mediating tumor resistance, lactylation offers a unique therapeutic target for addressing both tumor progression and treatment challenges. Future efforts should focus on uncovering the molecular mechanisms of lactylation and developing specific inhibitors or activators to exploit its therapeutic potential. Lactylation-based strategies could form the foundation of next-generation cancer therapies, addressing immune dysregulation and overcoming therapy resistance in tandem.
6.3 Enhancing immunotherapy via lactate modulation
Lactate metabolism modulation presents a promising strategy to enhance the efficacy of immunotherapy by overcoming therapeutic resistance. By reducing lactate accumulation or inhibiting lactate-mediated signaling pathways, macrophage polarization can be reprogrammed, facilitating the transition from M2-like to M1-like state (64, 65, 72, 73). This shift not only rebalances the TME but also creates a more immunostimulatory milieu that enhances the effectiveness of various immunotherapies, including immune checkpoint inhibitors, such as anti-PD-1 or anti-PD-L1 antibodies (27, 28), CAR-T cell therapy, and cancer vaccines (114). In the TME, tumor-derived exosomes promote the polarization of TAMs towards the M2 phenotype, which is a key mediator of resistance to anti-PD-1 therapy (115). M2 TAMs contribute to immunosuppression and tumor progression mainly through the secretion of cytokines (e.g., TGF-β, PGE2), expression of immune checkpoint ligands (e.g., PD-L1, VISTA), and the release of exosomal microRNAs (e.g., miR-21, miR-155-5p), all of which inhibit the activity of CD8+; T cells and limit the anti-tumor immune response (115, 116). These findings suggest the potential of targeting TAMs as a therapeutic strategy to overcome resistance to immunotherapy and improve treatment outcomes. Recent studies demonstrate that combining conventional chemotherapies, such as oxaliplatin and cyclophosphamide, with lactate modulation can further reprogram macrophages, driving M1 polarization and early chemokine upregulation. This reprogramming enhances the inflammatory response and recruits immune cells to the tumor site (114). Additionally, CAR-T cell-derived IFNγ further activates M1-like macrophages expressing iNOS, which produce chemokines such as Cxcl9 and Cxcl10. These chemokines promote CXCR3-dependent immune cell recruitment, establishing a positive feedback loop that amplifies CAR-T cell tumor infiltration and enhances the overall anti-tumor immune response (114).
7 Discussion and perspectives
Tumor cells and their microenvironment exhibit profound heterogeneity, a key factor that influences macrophage polarization and contributes to therapeutic resistance across various cancer types. In prostate cancer, docetaxel-treated cells secrete CSF-1 to recruit TAMs, which then activate CXCR4 signaling via CXCL12 secretion, promoting tumor cell survival and resistance to chemotherapy (117). In breast cancer, chemotherapy-induced tumor debris triggers HO-1 upregulation in TAMs, suppressing M1 polarization and contributing to an immunosuppressive microenvironment (118). In lung cancer, Oct4 expression enhances M-CSF secretion, driving TAMs toward an M2 phenotype that supports tumor progression (119). These findings highlight the significant heterogeneity of TAMs in shaping the TME and mediating therapeutic resistance across multiple malignancies. This underscores the necessity of investigating key regulatory factors, such as lactate, which profoundly influence macrophage polarization and immune modulation, thereby playing a pivotal role in tumor progression and therapy resistance.
Lactate, once considered a mere byproduct of anaerobic metabolism, has emerged as a key regulator in tumor metabolism and immune modulation, particularly in TAMs. Lactate-driven metabolic reprogramming influences macrophage polarization, shaping the TME and impacting tumor progression, immune evasion, and therapeutic resistance (9–11, 120). This has established lactate as a promising therapeutic target in cancer treatment.
TAMs exhibit dynamic polarization along a functional spectrum, shaped by metabolic signals from the TME, ranging from pro-inflammatory (M1-like) to anti-inflammatory (M2-like) phenotypes. Within tumors, elevated lactate levels drive macrophages toward an M2-like phenotype, characterized by enhanced immune suppression and tissue remodeling, while preserving their ability to adapt to environmental changes. This M2-like polarization is associated with cytokine secretion (e.g., TGF-β, IL-10) that fosters immune evasion and promotes tumor growth (121). In contrast, M1-like macrophages are associated with anti-tumor immunity, partly through the secretion of pro-inflammatory cytokines like TNF, while their functional state remains influenced by dynamic environmental signals (122). Lactate modulates this polarization shift by influencing not only metabolic processes but also epigenetic mechanisms, particularly histone lactylation. This lactate-induced epigenetic modification plays a key role in macrophage polarization by activating the expression of genes linked to immune suppression and tissue repair (22). Lactate-induced histone modification enhances the expression of M2-associated genes like Arg1, promoting immune tolerance and tissue regeneration (22). Non-histone lactylation also influences key proteins involved in metabolism, linking metabolic shifts to broader cellular functions (82). Lactylation thus emerges as a crucial mechanism by which lactate regulates macrophage behavior and immune responses within the TME.
Given lactate’s profound influence on macrophage polarization and tumor progression, targeting lactate metabolism offers a promising strategy for cancer therapy. Enzymes like LDHA and HK2, crucial for lactate production, are overexpressed in many cancers. Inhibiting LDHA reduces lactate levels, alleviates TME acidification, and reprograms TAMs toward a pro-inflammatory M1-like phenotype, thereby enhancing anti-tumor immunity (25). Similarly, HK2 inhibition disrupts glycolysis, normalizes the TME, and boosts immune activation (87). These findings underscore LDHA and HK2 as promising therapeutic targets. MCTs, especially MCT1 and MCT4, regulate lactate uptake and export in tumor cells and macrophages (26, 95). MCT inhibition blocks lactate transport, inducing metabolic stress in tumor cells and enhancing immune responses. Preclinical studies have demonstrated the potential of MCT inhibitors, such as AZD3965 (MCT1-specific) and syrosingopine (dual MCT1/MCT4), to reduce tumor growth and improve immunotherapy efficacy (44, 95, 96). However, the heterogeneity of tumors and compensatory metabolic pathways pose challenges, highlighting the need for combination therapies.
Beyond macrophage polarization, lactylation has been linked to cancer therapy resistance, particularly in DNA repair. Proteins like MRE11 and XRCC1, involved in homologous recombination, undergo lactylation, which enhances DNA repair and confers resistance to chemotherapy (108, 109). Inhibiting lactylation-regulating enzymes, such as CBP or LDHA, sensitizes tumors to cisplatin and PARP inhibitors, presenting lactylation as a potential target for overcoming therapy resistance (108, 109). This has been particularly demonstrated in glioblastoma, where lactylation-related pathways reverse chemoradiotherapy resistance (109). Notably, lactate modulation has the potential to improve the effectiveness of immunotherapies. Tumor-derived lactate drives TAMs toward an immunosuppressive M2 phenotype, which contributes to resistance against ICIs and CAR-T cell therapies (28, 114–116). By targeting lactate production or lactylation, it is possible to reprogram TAMs to an M1 phenotype, creating a more immunostimulatory environment. Combining lactate-modulating agents with ICIs or CAR-T cell therapies has shown promise in preclinical studies, amplifying anti-tumor immunity and overcoming resistance (28). Despite the therapeutic potential of lactate targeting, several challenges remain, including tumor heterogeneity, metabolic plasticity, and compensatory mechanisms. Moreover, while lactate and lactylation offer novel targets, further research is needed to elucidate the molecular mechanisms underlying their effects on immune modulation and therapy resistance. Identifying biomarkers to predict responses to lactate-targeting therapies and optimizing combination strategies will be essential for enhancing therapeutic efficacy.
In conclusion, lactate-mediated metabolic reprogramming of TAMs is a critical mechanism that drives tumor progression, immune evasion, and therapeutic resistance. By influencing macrophage polarization and modulating immune responses through lactate and lactylation, tumors create an environment conducive to their growth and survival. Targeting lactate metabolism and lactylation offers a promising therapeutic strategy to reprogram the immune landscape, overcome therapy resistance, and enhance the efficacy of cancer treatments. Continued research into the molecular mechanisms of lactate and lactylation will be crucial for developing novel cancer therapies aimed at reshaping the TME and improving treatment outcomes.
Author contributions
XJ: Conceptualization, Data curation, Formal analysis, Investigation, Visualization, Writing – original draft, Writing – review & editing. NZ: Data curation, Investigation, Validation, Writing – review & editing. TY: Investigation, Validation, Writing – original draft. JW: Investigation, Validation, Writing – original draft. LH: Investigation, Validation, Writing – original draft. CS: Formal analysis, Supervision, Writing – original draft. HZ: Conceptualization, Formal analysis, Funding acquisition, Supervision, Writing – original draft, Writing – review & editing. SJ: Conceptualization, Formal analysis, Funding acquisition, Methodology, Resources, Supervision, Writing – original draft, Writing – review & editing.
Funding
The author(s) declare that financial support was received for the research and/or publication of this article. This study was supported by National Natural Science Foundation of China (grant no. 82074360), Project of National Administration of Traditional Chinese Medicine (no. GZY-KJS-SD-2023-026), Natural Science Foundation of Shandong Province (grant no. ZR2022MH319 and ZR2022LZY027), and Key Research and Development Program of Jining City (grant no. 2024YXNS246).
Acknowledgments
Thanks to the editors and reviewers for their hard work and important comments.
Conflict of interest
The authors declare that the research was conducted in the absence of any commercial or financial relationships that could be construed as a potential conflict of interest.
Generative AI statement
The author(s) declare that no Generative AI was used in the creation of this manuscript.
Publisher’s note
All claims expressed in this article are solely those of the authors and do not necessarily represent those of their affiliated organizations, or those of the publisher, the editors and the reviewers. Any product that may be evaluated in this article, or claim that may be made by its manufacturer, is not guaranteed or endorsed by the publisher.
References
1. Elhanani O, Ben-Uri R, Keren L. Spatial profiling technologies illuminate the tumor microenvironment. Cancer Cell. (2023) 41:404–20. doi: 10.1016/j.ccell.2023.01.010
2. Aziguli T, Xiao SY, Yang Y, Mutailifu M, Li XQ, Yin SQ, et al. ENO1 promotes PDAC progression by inhibiting CD8(+) T cell infiltration through upregulating PD-L1 expression via HIF-1α signaling. Trans Oncol. (2025) 52:102261. doi: 10.1016/j.tranon.2024.102261
3. Hou W, Xiao C, Zhou R, Yao X, Chen Q, Xu T, et al. Inhibiting autophagy selectively prunes dysfunctional tumor vessels and optimizes the tumor immune microenvironment. Theranostics. (2025) 15:258–76. doi: 10.7150/thno.98285
4. Wang P, Qiu J, Fang Y, Li S, Liu K, Cao Y, et al. SENP3 inhibition suppresses hepatocellular carcinoma progression and improves the efficacy of anti-PD-1 immunotherapy. Cell Death differentiation. (2025). doi: 10.1038/s41418-024-01437-9
5. Cao L, Li B, Zheng S, Zhang Q, Qian Y, Ren Y, et al. Reprogramming of fibroblasts into cancer-associated fibroblasts via IGF2-mediated autophagy promotes metastasis of lung cancer cells. iScience. (2024) 27:111269. doi: 10.1016/j.isci.2024.111269
6. Lee JH, Sánchez-Rivera FJ, He L, Basnet H, Chen FX, Spina E, et al. TGF-β and RAS jointly unmask primed enhancers to drive metastasis. Cell. (2024) 187:6182–6199.e6129. doi: 10.1016/j.cell.2024.08.014
7. Gan M, Liu N, Li W, Chen M, Bai Z, Liu D, et al. Metabolic targeting of regulatory T cells in oral squamous cell carcinoma: new horizons in immunotherapy. Mol Cancer. (2024) 23:273. doi: 10.1186/s12943-024-02193-7
8. Jiang M, Fang H, Tian H. Metabolism of cancer cells and immune cells in the initiation, progression, and metastasis of cancer. Theranostics. (2025) 15:155–88. doi: 10.7150/thno.103376
9. Brown TP, Ganapathy V. Lactate/GPR81 signaling and proton motive force in cancer: Role in angiogenesis, immune escape, nutrition, and Warburg phenomenon. Pharmacol Ther. (2020) 206:107451. doi: 10.1016/j.pharmthera.2019.107451
10. Gu J, Zhou J, Chen Q, Xu X, Gao J, Li X, et al. Tumor metabolite lactate promotes tumorigenesis by modulating MOESIN lactylation and enhancing TGF-β signaling in regulatory T cells. Cell Rep. (2022) 39:110986. doi: 10.1016/j.celrep.2022.110986
11. Yan P, Liu J, Li Z, Wang J, Zhu Z, Wang L, et al. Glycolysis reprogramming in idiopathic pulmonary fibrosis: unveiling the mystery of lactate in the lung. Int J Mol Sci. (2023) 25:315. doi: 10.3390/ijms25010315
12. Brand A, Singer K, Koehl GE, Kolitzus M, Schoenhammer G, Thiel A, et al. LDHA-associated lactic acid production blunts tumor immunosurveillance by T and NK cells. Cell Metab. (2016) 24:657–71. doi: 10.1016/j.cmet.2016.08.011
13. Peralta RM, Xie B, Lontos K, Nieves-Rosado H, Spahr K, Joshi S, et al. Dysfunction of exhausted T cells is enforced by MCT11-mediated lactate metabolism. Nat Immunol. (2024) 25:2297–307. doi: 10.1038/s41590-024-01999-3
14. Liu B, Xian Y, Chen X, Shi Y, Dong J, Yang L, et al. Inflammatory fibroblast-like synoviocyte-derived exosomes aggravate osteoarthritis via enhancing macrophage glycolysis. Advanced Sci (Weinheim Baden-Wurttemberg Germany). (2024) 11:e2307338. doi: 10.1002/advs.202307338
15. Qiu S, Xie L, Lu C, Gu C, Xia Y, Lv J, et al. Gastric cancer-derived exosomal miR-519a-3p promotes liver metastasis by inducing intrahepatic M2-like macrophage-mediated angiogenesis. J Exp Clin Cancer research: CR. (2022) 41:296. doi: 10.1186/s13046-022-02499-8
16. Gao J, Liang Y, Wang L. Shaping polarization of tumor-associated macrophages in cancer immunotherapy. Front Immunol. (2022) 13:888713. doi: 10.3389/fimmu.2022.888713
17. Han S, Wang W, Wang S, Yang T, Zhang G, Wang D, et al. Tumor microenvironment remodeling and tumor therapy based on M2-like tumor associated macrophage-targeting nano-complexes. Theranostics. (2021) 11:2892–916. doi: 10.7150/thno.50928
18. Noy R, Pollard JW. Tumor-associated macrophages: from mechanisms to therapy. Immunity. (2014) 41:49–61. doi: 10.1016/j.immuni.2014.06.010
19. Pan Y, Yu Y, Wang X, Zhang T. Tumor-associated macrophages in tumor immunity. Front Immunol. (2020) 11:583084. doi: 10.3389/fimmu.2020.583084
20. Zhang Q, Sioud M. Tumor-associated macrophage subsets: shaping polarization and targeting. Int J Mol Sci. (2023) 24:7493. doi: 10.3390/ijms24087493
21. Hu Y, He Z, Li Z, Wang Y, Wu N, Sun H, et al. Lactylation: the novel histone modification influence on gene expression, protein function, and disease. Clin Epigenet. (2024) 16:72. doi: 10.1186/s13148-024-01682-2
22. Zhang D, Tang Z, Huang H, Zhou G, Cui C, Weng Y, et al. Perez-Neut M et al: Metabolic regulation of gene expression by histone lactylation. Nature. (2019) 574:575–80. doi: 10.1038/s41586-019-1678-1
23. Noe JT, Rendon BE, Geller AE, Conroy LR, Morrissey SM, Young LEA, et al. Lactate supports a metabolic-epigenetic link in macrophage polarization. Sci Adv. (2021) 7:eabi8602. doi: 10.1126/sciadv.abi8602
24. Han S, Bao X, Zou Y, Wang L, Li Y, Yang L, et al. d-lactate modulates M2 tumor-associated macrophages and remodels immunosuppressive tumor microenvironment for hepatocellular carcinoma. Sci Adv. (2023) 9:eadg2697. doi: 10.1126/sciadv.adg2697
25. Wang F, Liao W, Li C, Zhu L. Silencing BMAL1 promotes M1/M2 polarization through the LDHA/lactate axis to promote GBM sensitivity to bevacizumab. Int Immunopharmacol. (2024) 134:112187. doi: 10.1016/j.intimp.2024.112187
26. Weng YS, Tseng HY, Chen YA, Shen PC, Al Haq AT, Chen LM, et al. MCT-1/miR-34a/IL-6/IL-6R signaling axis promotes EMT progression, cancer stemness and M2 macrophage polarization in triple-negative breast cancer. Mol Cancer. (2019) 18:42. doi: 10.1186/s12943-019-0988-0
27. Zhang H, Liu L, Liu J, Dang P, Hu S, Yuan W, et al. Roles of tumor-associated macrophages in anti-PD-1/PD-L1 immunotherapy for solid cancers. Mol Cancer. (2023) 22:58. doi: 10.1186/s12943-023-01725-x
28. Cai H, Zhang Y, Wang J, Gu J. Defects in macrophage reprogramming in cancer therapy: the negative impact of PD-L1/PD-1. Front Immunol. (2021) 12:690869. doi: 10.3389/fimmu.2021.690869
29. Dai E, Wang W, Li Y, Ye D, Li Y. Lactate and lactylation: Behind the development of tumors. Cancer Lett. (2024) 591:216896. doi: 10.1016/j.canlet.2024.216896
30. Sharma D, Singh M, Rani R. Role of LDH in tumor glycolysis: Regulation of LDHA by small molecules for cancer therapeutics. Semin Cancer Biol. (2022) 87:184–95. doi: 10.1016/j.semcancer.2022.11.007
31. Paul S, Ghosh S, Kumar S. Tumor glycolysis, an essential sweet tooth of tumor cells. Semin Cancer Biol. (2022) 86:1216–30. doi: 10.1016/j.semcancer.2022.09.007
32. Yang Z, Su W, Wei X, Qu S, Zhao D, Zhou J, et al. HIF-1α drives resistance to ferroptosis in solid tumors by promoting lactate production and activating SLC1A1. Cell Rep. (2023) 42:112945. doi: 10.1016/j.celrep.2023.112945
33. Damiani C, Colombo R, Gaglio D, Mastroianni F, Pescini D, Westerhoff HV, et al. A metabolic core model elucidates how enhanced utilization of glucose and glutamine, with enhanced glutamine-dependent lactate production, promotes cancer cell growth: The WarburQ effect. PloS Comput Biol. (2017) 13:e1005758. doi: 10.1371/journal.pcbi.1005758
34. Miao Y, Zheng Y, Geng Y, Yang L, Cao N, Dai Y, et al. he role of GLS1-mediated glutaminolysis/2-HG/H3K4me3 and GSH/ROS signals in Th17 responses counteracted by PPARγ agonists. Theranostics. (2021) 11:4531–48. doi: 10.7150/thno.54803
35. Chinopoulos C. From glucose to lactate and transiting intermediates through mitochondria, bypassing pyruvate kinase: considerations for cells exhibiting dimeric PKM2 or otherwise inhibited kinase activity. Front Physiol. (2020) 11:543564. doi: 10.3389/fphys.2020.543564
36. Chen L, Huang L, Gu Y, Cang W, Sun P, Xiang Y. Lactate-lactylation hands between metabolic reprogramming and immunosuppression. Int J Mol Sci. (2022) 23:11943. doi: 10.3390/ijms231911943
37. Linares JF, Cid-Diaz T, Duran A, Osrodek M, Martinez-Ordoñez A, Reina-Campos M, et al. The lactate-NAD(+) axis activates cancer-associated fibroblasts by downregulating p62. Cell Rep. (2022) 39:110792. doi: 10.1016/j.celrep.2022.110792
38. Spiljar M, Kuchroo VK. Metabolic regulation and function of T helper cells in neuroinflammation. Semin immunopathol. (2022) 44:581–98. doi: 10.1007/s00281-022-00959-z
39. Benítez-Muñoz JA, Cupeiro R, Rubio-Arias J, Amigo T, González-Lamuño D. Exercise influence on monocarboxylate transporter 1 (MCT1) and 4 (MCT4) in the skeletal muscle: A systematic review. Acta Physiol (Oxford England). (2024) 240:e14083. doi: 10.1111/apha.14083
40. Payen VL, Mina E, Van Hée VF, Porporato PE, Sonveaux P. Monocarboxylate transporters in cancer. Mol Metab. (2020) 33:48–66. doi: 10.1016/j.molmet.2019.07.006
41. Doherty JR, Cleveland JL. Targeting lactate metabolism for cancer therapeutics. J Clin Invest. (2013) 123:3685–92. doi: 10.1172/jci69741
42. Fan Z, Yang G, Luo R, Qu X, Ye X, Wang J, et al. Sublethal heat treatment enhances lactic acid uptake in macrophages via MCT1, leading to reduced paraspeckle formation and a subsequent decrease in macrophage pyroptosis. Front Immunol. (2023) 14:1290185. doi: 10.3389/fimmu.2023.1290185
43. Felmlee MA, Jones RS, Rodriguez-Cruz V, Follman KE, Morris ME. Monocarboxylate transporters (SLC16), function, regulation, and role in health and disease. Pharmacol Rev. (2020) 72:466–85. doi: 10.1124/pr.119.018762
44. Benjamin D, Robay D, Hindupur SK, Pohlmann J, Colombi M, El-Shemerly MY, et al. Dual inhibition of the lactate transporters MCT1 and MCT4 is synthetic lethal with metformin due to NAD+ Depletion in cancer cells. Cell Rep. (2018) 25:3047–3058.e3044. doi: 10.1016/j.celrep.2018.11.043
45. Kanekura T. CD147/basigin is involved in the development of Malignant tumors and T-cell-mediated immunological disorders via regulation of glycolysis. Int J Mol Sci. (2023) 24:17344. doi: 10.3390/ijms242417344
46. Kirk P, Wilson MC, Heddle C, Brown MH, Barclay AN, Halestrap AP. CD147 is tightly associated with lactate transporters MCT1 and MCT4 and facilitates their cell surface expression. EMBO J. (2000) 19:3896–904. doi: 10.1093/emboj/19.15.3896
47. Köpnick AL, Jansen A, Geistlinger K, Epalle NH, Beitz E. Basigin drives intracellular accumulation of l-lactate by harvesting protons and substrate anions. PLoS One. (2021) 16:e0249110. doi: 10.1371/journal.pone.0249110
48. Philp NJ, Ochrietor JD, Rudoy C, Muramatsu T, Linser PJ. Loss of MCT1, MCT3, and MCT4 expression in the retinal pigment epithelium and neural retina of the 5A11/basigin-null mouse. Invest Ophthalmol Visual Sci. (2003) 44:1305–11. doi: 10.1167/iovs.02-0552
49. Mantovani A, Sica A, Sozzani S, Allavena P, Vecchi A, Locati M. The chemokine system in diverse forms of macrophage activation and polarization. Trends Immunol. (2004) 25:677–86. doi: 10.1016/j.it.2004.09.015
50. Avila-Ponce de León U, Vázquez-Jiménez A, Padilla-Longoria P, Resendis-Antonio O. Uncoding the interdependency of tumor microenvironment and macrophage polarization: insights from a continuous network approach. Front Immunol. (2023) 14:1150890. doi: 10.3389/fimmu.2023.1150890
51. Ghafouri-Fard S, Abak A, Tavakkoli Avval S, Shoorei H, Taheri M, Samadian M. The impact of non-coding RNAs on macrophage polarization. BiomedPharmacother. (2021) 142:112112. doi: 10.1016/j.biopha.2021.112112
52. Wang S, Liu G, Li Y, Pan Y. Metabolic reprogramming induces macrophage polarization in the tumor microenvironment. Front Immunol. (2022) 13:840029. doi: 10.3389/fimmu.2022.840029
53. Sha W, Zhao B, Wei H, Yang Y, Yin H, Gao J, et al. Astragalus polysaccharide ameliorates vascular endothelial dysfunction by stimulating macrophage M2 polarization via potentiating Nrf2/HO-1 signaling pathway. Phytomedicine. (2023) 112:154667. doi: 10.1016/j.phymed.2023.154667
54. Boutilier AJ, Elsawa SF. Macrophage polarization states in the tumor microenvironment. Int J Mol Sci. (2021) 22:6995. doi: 10.3390/ijms22136995
55. Abdelaziz MH, Abdelwahab SF, Wan J, Cai W, Huixuan W, Jianjun C, et al. Alternatively activated macrophages; a double-edged sword in allergic asthma. J Trans Med. (2020) 18:58. doi: 10.1186/s12967-020-02251-w
56. Jha AK, Huang SC, Sergushichev A, Lampropoulou V, Ivanova Y, Loginicheva E, et al. Network integration of parallel metabolic and transcriptional data reveals metabolic modules that regulate macrophage polarization. Immunity. (2015) 42:419–30. doi: 10.1016/j.immuni.2015.02.005
57. Viola A, Munari F, Sánchez-Rodríguez R, Scolaro T, Castegna A. The metabolic signature of macrophage responses. Front Immunol. (2019) 10:1462. doi: 10.3389/fimmu.2019.01462
58. Mehla K, Singh PK. Metabolic regulation of macrophage polarization in cancer. Trends Cancer. (2019) 5:822–34. doi: 10.1016/j.trecan.2019.10.007
59. Jeroundi N, Roy C, Basset L, Pignon P, Preisser L, Blanchard S, et al. Glycogenesis and glyconeogenesis from glutamine, lactate and glycerol support human macrophage functions. EMBO Rep. (2024) 25:5383–407. doi: 10.1038/s44319-024-00278-4
60. Palsson-McDermott EM, Curtis AM, Goel G, Lauterbach MA, Sheedy FJ, Gleeson LE, et al. Pyruvate kinase M2 regulates Hif-1α activity and IL-1β induction and is a critical determinant of the warburg effect in LPS-activated macrophages. Cell Metab. (2015) 21:65–80. doi: 10.1016/j.cmet.2014.12.005
61. Tan Z, Xie N, Cui H, Moellering DR, Abraham E, Thannickal VJ, et al. Pyruvate dehydrogenase kinase 1 participates in macrophage polarization via regulating glucose metabolism. J Immunol (Baltimore Md: 1950). (2015) 194:6082–9. doi: 10.4049/jimmunol.1402469
62. Zhang Y, Zhang C, Feng R, Meng T, Peng W, Song J, et al. CXCR4 regulates macrophage M1 polarization by altering glycolysis to promote prostate fibrosis. Cell Commun Signal. (2024) 22:456. doi: 10.1186/s12964-024-01828-y
63. Farooque A, Afrin F, Adhikari JS, Dwarakanath BS. Polarization of macrophages towards M1 phenotype by a combination of 2-deoxy-d-glucose and radiation: Implications for tumor therapy. Immunobiology. (2016) 221:269–81. doi: 10.1016/j.imbio.2015.10.009
64. Liao ZX, Fa YC, Kempson IM, Tseng SJ. Repolarization of M2 to M1 macrophages triggered by lactate oxidase released from methylcellulose hydrogel. Bioconjugate Chem. (2019) 30:2697–702. doi: 10.1021/acs.bioconjchem.9b00618
65. Zhou HC, Yu WW, Yan XY, Liang XQ, Ma XF, Long JP, et al. Lactate-driven macrophage polarization in the inflammatory microenvironment alleviates intestinal inflammation. Front Immunol. (2022) 13:1013686. doi: 10.3389/fimmu.2022.1013686
66. Seth P, Csizmadia E, Hedblom A, Vuerich M, Xie H, Li M, et al. Deletion of lactate dehydrogenase-A in myeloid cells triggers antitumor immunity. Cancer Res. (2017) 77:3632–43. doi: 10.1158/0008-5472.Can-16-2938
67. Kesh K, Garrido VT, Dosch A, Durden B, Gupta VK, Sharma NS, et al. Stroma secreted IL6 selects for “stem-like” population and alters pancreatic tumor microenvironment by reprogramming metabolic pathways. Cell Death Dis. (2020) 11:967. doi: 10.1038/s41419-020-03168-4
68. Ye L, Jiang Y, Zhang M. Crosstalk between glucose metabolism, lactate production and immune response modulation. Cytokine Growth Factor Rev. (2022) 68:81–92. doi: 10.1016/j.cytogfr.2022.11.001
69. Yan C, Yang Z, Chen P, Yeh Y, Sun C, Xie T, et al. GPR65 sensing tumor-derived lactate induces HMGB1 release from TAM via the cAMP/PKA/CREB pathway to promote glioma progression. J Exp Clin Cancer Res. (2024) 43:105. doi: 10.1186/s13046-024-03025-8
70. Bohn T, Rapp S, Luther N, Klein M, Bruehl TJ, Kojima N, et al. Tumor immunoevasion via acidosis-dependent induction of regulatory tumor-associated macrophages. Nat Immunol. (2018) 19:1319–29. doi: 10.1038/s41590-018-0226-8
71. El-Kenawi A, Gatenbee C, Robertson-Tessi M, Bravo R, Dhillon J, Balagurunathan Y, et al. Acidity promotes tumour progression by altering macrophage phenotype in prostate cancer. Br J Cancer. (2019) 121:556–66. doi: 10.1038/s41416-019-0542-2
72. Yang K, Xu J, Fan M, Tu F, Wang X, Ha T, et al. Lactate suppresses macrophage pro-inflammatory response to LPS stimulation by inhibition of YAP and NF-κB activation via GPR81-mediated signaling. Front Immunol. (2020) 11:587913. doi: 10.3389/fimmu.2020.587913
73. Colegio OR, Chu NQ, Szabo AL, Chu T, Rhebergen AM, Jairam V, et al. Phillips GM et al: Functional polarization of tumour-associated macrophages by tumour-derived lactic acid. Nature. (2014) 513:559–63. doi: 10.1038/nature13490
74. Li M, Yang Y, Xiong L, Jiang P, Wang J, Li C. Metabolism, metabolites, and macrophages in cancer. J Hematol Oncol. (2023) 16:80. doi: 10.1186/s13045-023-01478-6
75. Zhang Y, Jiang H, Dong M, Min J, He X, Tan Y, et al. Macrophage MCT4 inhibition activates reparative genes and protects from atherosclerosis by histone H3 lysine 18 lactylation. Cell Rep. (2024) 43:114884. doi: 10.1016/j.celrep.2024.114884
76. Sun J, Feng Q, He Y, Wang M, Wu Y. Lactate activates CCL18 expression via H3K18 lactylation in macrophages to promote tumorigenesis of ovarian cancer. Acta Biochim Biophys Sin. (2024) 56:1373–86. doi: 10.3724/abbs.2024111
77. Susser LI, Nguyen MA, Geoffrion M, Emerton C, Ouimet M, Khacho M, et al. Mitochondrial fragmentation promotes inflammation resolution responses in macrophages via histone lactylation. Mol Cell Biol. (2023) 43:531–46. doi: 10.1080/10985549.2023.2253131
78. Wang N, Wang W, Wang X, Mang G, Chen J, Yan X, et al. Histone lactylation boosts reparative gene activation post-myocardial infarction. Circ Res. (2022) 131:893–908. doi: 10.1161/circresaha.122.320488
79. Chen L, Zhang M, Yang X, Wang Y, Huang T, Li X, et al. Methyl-CpG-binding 2 K271 lactylation-mediated M2 macrophage polarization inhibits atherosclerosis. Theranostics. (2024) 14:4256–77. doi: 10.7150/thno.94738
80. Zhang N, Jiang N, Yu L, Guan T, Sang X, Feng Y, et al. Protein lactylation critically regulates energy metabolism in the protozoan parasite trypanosoma brucei. Front Cell Dev Biol. (2021) 9:719720. doi: 10.3389/fcell.2021.719720
81. Wang J, Yang P, Yu T, Gao M, Liu D, Zhang J, et al. Lactylation of PKM2 suppresses inflammatory metabolic adaptation in pro-inflammatory macrophages. Int J Biol Sci. (2022) 18:6210–25. doi: 10.7150/ijbs.75434
82. Gao R, Li Y, Xu Z, Zhang F, Xu J, Hu Y, et al. Mitochondrial pyruvate carrier 1 regulates fatty acid synthase lactylation and mediates treatment of nonalcoholic fatty liver disease. Hepatology (Baltimore Md). (2023) 78:1800–15. doi: 10.1097/hep.0000000000000279
83. Fang X, Zhao P, Gao S, Liu D, Zhang S, Shan M, et al. Lactate induces tumor-associated macrophage polarization independent of mitochondrial pyruvate carrier-mediated metabolism. Int J Biol macromolecules. (2023) 237:123810. doi: 10.1016/j.ijbiomac.2023.123810
84. Wang T, Ye Z, Li Z, Jing DS, Fan GX, Liu MQ, et al. Lactate-induced protein lactylation: A bridge between epigenetics and metabolic reprogramming in cancer. Cell proliferation. (2023) 56:e13478. doi: 10.1111/cpr.13478
85. Luo Y, Yang Z, Yu Y, Zhang P. HIF1α lactylation enhances KIAA1199 transcription to promote angiogenesis and vasculogenic mimicry in prostate cancer. Int J Biol Macromol. (2022) 222:2225–43. doi: 10.1016/j.ijbiomac.2022.10.014
86. Rho H, Terry AR, Chronis C, Hay N. Hexokinase 2-mediated gene expression via histone lactylation is required for hepatic stellate cell activation and liver fibrosis. Cell Metab. (2023) 35:1406–1423.e1408. doi: 10.1016/j.cmet.2023.06.013
87. Yang Y, Liu Q, Wang M, Li L, Yu Y, Pan M, et al. Genetically programmable cell membrane-camouflaged nanoparticles for targeted combination therapy of colorectal cancer. Signal Transduct target Ther. (2024) 9:158. doi: 10.1038/s41392-024-01859-4
88. Certo M, Tsai CH, Pucino V, Ho PC, Mauro C. Lactate modulation of immune responses in inflammatory versus tumour microenvironments. Nat Rev Immunol. (2021) 21:151–61. doi: 10.1038/s41577-020-0406-2
89. Liu J, Zhang C, Zhang T, Chang CY, Wang J, Bazile L, et al. Metabolic enzyme LDHA activates Rac1 GTPase as a noncanonical mechanism to promote cancer. Nat Metab. (2022) 4:1830–46. doi: 10.1038/s42255-022-00708-4
90. Végran F, Boidot R, Michiels C, Sonveaux P, Feron O. Lactate influx through the endothelial cell monocarboxylate transporter MCT1 supports an NF-κB/IL-8 pathway that drives tumor angiogenesis. Cancer Res. (2011) 71:2550–60. doi: 10.1158/0008-5472.Can-10-2828
91. Baumann F, Leukel P, Doerfelt A, Beier CP, Dettmer K, Oefner PJ, et al. Lactate promotes glioma migration by TGF-beta2-dependent regulation of matrix metalloproteinase-2. Neuro-oncology. (2009) 11:368–80. doi: 10.1215/15228517-2008-106
92. Chen H, Li Y, Li H, Chen X, Fu H, Mao D, et al. Hu K et al: NBS1 lactylation is required for efficient DNA repair and chemotherapy resistance. Nature. (2024) 631:663–9. doi: 10.1038/s41586-024-07620-9
93. Jiang Y, Li F, Gao B, Ma M, Chen M, Wu Y, et al. KDM6B-mediated histone demethylation of LDHA promotes lung metastasis of osteosarcoma. Theranostics. (2021) 11:3868–81. doi: 10.7150/thno.53347
94. Khan F, Lin Y, Ali H, Pang L, Dunterman M, Hsu WH, et al. Lactate dehydrogenase A regulates tumor-macrophage symbiosis to promote glioblastoma progression. Nat Commun. (2024) 15:1987. doi: 10.1038/s41467-024-46193-z
95. Fang Y, Liu W, Tang Z, Ji X, Zhou Y, Song S, et al. Monocarboxylate transporter 4 inhibition potentiates hepatocellular carcinoma immunotherapy through enhancing T cell infiltration and immune attack. Hepatology (Baltimore Md). (2023) 77:109–23. doi: 10.1002/hep.32348
96. Babl N, Decking SM, Voll F, Althammer M, Sala-Hojman A, Ferretti R, et al. MCT4 blockade increases the efficacy of immune checkpoint blockade. J immunotherapy Cancer. (2023) 11:e007349. doi: 10.1136/jitc-2023-007349
97. Barron CC, Bilan PJ, Tsakiridis T, Tsiani E. Facilitative glucose transporters: Implications for cancer detection, prognosis and treatment. Metabolism. (2016) 65:124–39. doi: 10.1016/j.metabol.2015.10.007
98. Yadav D, Yadav A, Bhattacharya S, Dagar A, Kumar V, Rani R. GLUT and HK: Two primary and essential key players in tumor glycolysis. Semin Cancer Biol. (2024) 100:17–27. doi: 10.1016/j.semcancer.2024.03.001
99. Li YL, Weng HC, Hsu JL, Lin SW, Guh JH, Hsu LC. The combination of MK-2206 and WZB117 exerts a synergistic cytotoxic effect against breast cancer cells. Front Pharmacol. (2019) 10:1311. doi: 10.3389/fphar.2019.01311
100. Miller ZA, Muthuswami S, Mueller A, Ma RZ, Sywanycz SM, Naik A, et al. GLUT1 inhibitor BAY-876 induces apoptosis and enhances anti-cancer effects of bitter receptor agonists in head and neck squamous carcinoma cells. Cell Death Discov. (2024) 10:339. doi: 10.1038/s41420-024-02106-z
101. Zhao F, Ming J, Zhou Y, Fan L. Inhibition of Glut1 by WZB117 sensitizes radioresistant breast cancer cells to irradiation. Cancer Chemother Pharmacol. (2016) 77:963–72. doi: 10.1007/s00280-016-3007-9
102. Jeoung NH. Pyruvate dehydrogenase kinases: therapeutic targets for diabetes and cancers. Diabetes Metab J. (2015) 39:188–97. doi: 10.4093/dmj.2015.39.3.188
103. Patel MS, Nemeria NS, Furey W, Jordan F. The pyruvate dehydrogenase complexes: structure-based function and regulation. J Biol Chem. (2014) 289:16615–23. doi: 10.1074/jbc.R114.563148
104. Anwar S, Shamsi A, Mohammad T, Islam A, Hassan MI. Targeting pyruvate dehydrogenase kinase signaling in the development of effective cancer therapy. Biochim Biophys Acta Rev Cancer. (2021) 1876:188568. doi: 10.1016/j.bbcan.2021.188568
105. O’Neill LA, Hardie DG. Metabolism of inflammation limited by AMPK and pseudo-starvation. Nature. (2013) 493:346–55. doi: 10.1038/nature11862
106. Jin J, Yuan P, Yu W, Lin J, Xu A, Xu X, et al. Mitochondria-targeting polymer micelle of dichloroacetate induced pyroptosis to enhance osteosarcoma immunotherapy. ACS nano. (2022) 16:10327–40. doi: 10.1021/acsnano.2c00192
107. Semba H, Takeda N, Isagawa T, Sugiura Y, Honda K, Wake M, et al. HIF-1α-PDK1 axis-induced active glycolysis plays an essential role in macrophage migratory capacity. Nat Commun. (2016) 7:11635. doi: 10.1038/ncomms11635
108. Chen Y, Wu J, Zhai L, Zhang T, Yin H, Gao H, et al. Metabolic regulation of homologous recombination repair by MRE11 lactylation. Cell. (2024) 187:294–311.e221. doi: 10.1016/j.cell.2023.11.022
109. Li G, Wang D, Zhai Y, Pan C, Zhang J, Wang C, et al. Glycometabolic reprogramming-induced XRCC1 lactylation confers therapeutic resistance in ALDH1A3-overexpressing glioblastoma. Cell Metab. (2024) 36:1696–1710.e1610. doi: 10.1016/j.cmet.2024.07.011
110. Zong Z, Xie F, Wang S, Wu X, Zhang Z, Yang B, et al. Alanyl-tRNA synthetase, AARS1, is a lactate sensor and lactyltransferase that lactylates p53 and contributes to tumorigenesis. Cell. (2024) 187:2375–2392.e2333. doi: 10.1016/j.cell.2024.04.002
111. Ju J, Zhang H, Lin M, Yan Z, An L, Cao Z, et al. The alanyl-tRNA synthetase AARS1 moonlights as a lactyltransferase to promote YAP signaling in gastric cancer. J Clin Invest. (2024) 134:e174587. doi: 10.1172/jci174587
112. Dong H, Zhang J, Zhang H, Han Y, Lu C, Chen C, et al. YiaC and CobB regulate lysine lactylation in Escherichia coli. Nat Commun. (2022) 13:6628. doi: 10.1038/s41467-022-34399-y
113. Cui H, Xie N, Banerjee S, Ge J, Jiang D, Dey T, et al. Lung myofibroblasts promote macrophage profibrotic activity through lactate-induced histone lactylation. Am J respiratory Cell Mol Biol. (2021) 64:115–25. doi: 10.1165/rcmb.2020-0360OC
114. Srivastava S, Furlan SN, Jaeger-Ruckstuhl CA, Sarvothama M, Berger C, Smythe KS, et al. Immunogenic chemotherapy enhances recruitment of CAR-T cells to lung tumors and improves antitumor efficacy when combined with checkpoint blockade. Cancer Cell. (2021) 39:193–208.e110. doi: 10.1016/j.ccell.2020.11.005
115. Pu Y, Ji Q. Tumor-associated macrophages regulate PD-1/PD-L1 immunosuppression. Front Immunol. (2022) 13:874589. doi: 10.3389/fimmu.2022.874589
116. Kitamura F, Semba T, Yasuda-Yoshihara N, Yamada K, Nishimura A, Yamasaki J, et al. Cancer-associated fibroblasts reuse cancer-derived lactate to maintain a fibrotic and immunosuppressive microenvironment in pancreatic cancer. JCI insight. (2023) 8:e163022. doi: 10.1172/jci.insight.163022
117. Guan W, Li F, Zhao Z, Zhang Z, Hu J, Zhang Y. Tumor-Associated Macrophage Promotes the Survival of Cancer Cells upon Docetaxel Chemotherapy via the CSF1/CSF1R-CXCL12/CXCR4 Axis in Castration-Resistant Prostate Cancer. Genes. (2021) 12:773. doi: 10.3390/genes12050773
118. Kim SH, Saeidi S, Zhong X, Gwak SY, Muna IA, Park SA, et al. Breast cancer cell debris diminishes therapeutic efficacy through heme oxygenase-1-mediated inactivation of M1-like tumor-associated macrophages. Neoplasia (New York NY). (2020) 22:606–16. doi: 10.1016/j.neo.2020.08.006
119. Lu CS, Shiau AL, Su BH, Hsu TS, Wang CT, Su YC, et al. Oct4 promotes M2 macrophage polarization through upregulation of macrophage colony-stimulating factor in lung cancer. J Hematol Oncol. (2020) 13:62. doi: 10.1186/s13045-020-00887-1
120. Rabinowitz JD, Enerbäck S. Lactate: the ugly duckling of energy metabolism. Nat Metab. (2020) 2:566–71. doi: 10.1038/s42255-020-0243-4
121. Wang H, Yung MMH, Ngan HYS, Chan KKL, Chan DW. The impact of the tumor microenvironment on macrophage polarization in cancer metastatic progression. Int J Mol Sci. (2021) 22:6560. doi: 10.3390/ijms22126560
122. Zhu X, Liang R, Lan T, Ding D, Huang S, Shao J, et al. Tumor-associated macrophage-specific CD155 contributes to M2-phenotype transition, immunosuppression, and tumor progression in colorectal cancer. J Immunother Cancer. (2022) 10:e004219. doi: 10.1136/jitc-2021-004219
123. Boufaied N, Chetta P, Hallal T, Cacciatore S, Lalli D, Luthold C, et al. Obesogenic high-fat diet and MYC cooperate to promote lactate accumulation and tumor microenvironment remodeling in prostate cancer. Cancer Res. (2024) 84:1834–55. doi: 10.1158/0008-5472.Can-23-0519
124. Chen Z, Wu H, Wang Y, Rao Y, Yan J, Ran B, et al. Enhancing melanoma therapy by modulating the immunosuppressive microenvironment with an MMP-2 sensitive and nHA/GNE co-encapsulated hydrogel. Acta Biomater. (2024) 188:79–92. doi: 10.1016/j.actbio.2024.08.055
125. Mazzio E, Mack N, Badisa RB, Soliman KFA. Triple isozyme lactic acid dehydrogenase inhibition in fully viable MDA-MB-231 cells induces cytostatic effects that are not reversed by exogenous lactic acid. Biomolecules. (2021) 11:1751. doi: 10.3390/biom11121751
126. Guo L, Yang Y, Sheng Y, Wang J, Li W, Zhou X, et al. Galloflavin relieves the Malignant behavior of colorectal cancer cells in the inflammatory tumor microenvironment. Front Pharmacol. (2021) 12:752118. doi: 10.3389/fphar.2021.752118
127. Rahnasto-Rilla M, Järvenpää J, Huovinen M, Schroderus AM, Ihantola EL, Küblbeck J, et al. Effects of galloflavin and ellagic acid on sirtuin 6 and its anti-tumorigenic activities. Biomed Pharmacother. (2020) 131:110701. doi: 10.1016/j.biopha.2020.110701
128. Han X, Sheng X, Jones HM, Jackson AL, Kilgore J, Stine JE, et al. Evaluation of the anti-tumor effects of lactate dehydrogenase inhibitor galloflavin in endometrial cancer cells. J Hematol Oncol. (2015) 8:2. doi: 10.1186/s13045-014-0097-x
129. Sun T, Liu B, Li Y, Wu J, Cao Y, Yang S, et al. Oxamate enhances the efficacy of CAR-T therapy against glioblastoma via suppressing ectonucleotidases and CCR8 lactylation. J Exp Clin Cancer Res. (2023) 42:253. doi: 10.1186/s13046-023-02815-w
130. Zhao Z, Han F, Yang S, Wu J, Zhan W. Oxamate-mediated inhibition of lactate dehydrogenase induces protective autophagy in gastric cancer cells: involvement of the Akt-mTOR signaling pathway. Cancer Lett. (2015) 358:17–26. doi: 10.1016/j.canlet.2014.11.046
131. de Mey S, Dufait I, Jiang H, Corbet C, Wang H, Van De Gucht M, et al. Dichloroacetate radiosensitizes hypoxic breast cancer cells. Int J Mol Sci. (2020) 21:9367. doi: 10.3390/ijms21249367
132. Cook KM, Shen H, McKelvey KJ, Gee HE, Hau E. Targeting glucose metabolism of cancer cells with dichloroacetate to radiosensitize high-grade gliomas. Int J Mol Sci. (2021) 22:7265. doi: 10.3390/ijms22147265
133. Zheng R, Chen X, Zhao L, Yang N, Guan R, Chen A, et al. A porphysome-based photodynamic O(2) economizer for hypoxic tumor treatment by inhibiting mitochondrial respiration. Chem Commun (Cambridge England). (2021) 57:4134–7. doi: 10.1039/d1cc00544h
134. Skwarski M, McGowan DR, Belcher E, Di Chiara F, Stavroulias D, McCole M, et al. Mitochondrial inhibitor atovaquone increases tumor oxygenation and inhibits hypoxic gene expression in patients with non-small cell lung cancer. Clin Cancer Res. (2021) 27:2459–69. doi: 10.1158/1078-0432.Ccr-20-4128
135. Bourigault P, Skwarski M, Macpherson RE, Higgins GS, McGowan DR. Timing of hypoxia PET/CT imaging after 18F-fluoromisonidazole injection in non-small cell lung cancer patients. Sci Rep. (2022) 12:21746. doi: 10.1038/s41598-022-26199-7
136. Yang T, Zhang X, Yang X, Li Y, Xiang J, Xiang C, et al. A mitochondria-targeting self-assembled carrier-free lonidamine nanodrug for redox-activated drug release to enhance cancer chemotherapy. J materials Chem B. (2023) 11:3951–7. doi: 10.1039/d2tb02728c
137. Halford S, Veal GJ, Wedge SR, Payne GS, Bacon CM, Sloan P, et al. A phase I dose-escalation study of AZD3965, an oral monocarboxylate transporter 1 inhibitor, in patients with advanced cancer. Clin Cancer Res. (2023) 29:1429–39. doi: 10.1158/1078-0432.Ccr-22-2263
138. Polański R, Hodgkinson CL, Fusi A, Nonaka D, Priest L, Kelly P, et al. Activity of the monocarboxylate transporter 1 inhibitor AZD3965 in small cell lung cancer. Clin Cancer Res. (2014) 20:926–37. doi: 10.1158/1078-0432.Ccr-13-2270
139. Beloueche-Babari M, Wantuch S, Casals Galobart T, Koniordou M, Parkes HG, Arunan V, et al. MCT1 inhibitor AZD3965 increases mitochondrial metabolism, facilitating combination therapy and noninvasive magnetic resonance spectroscopy. Cancer Res. (2017) 77:5913–24. doi: 10.1158/0008-5472.Can-16-2686
140. Corbet C, Bastien E, Draoui N, Doix B, Mignion L, Jordan BF, et al. Interruption of lactate uptake by inhibiting mitochondrial pyruvate transport unravels direct antitumor and radiosensitizing effects. Nat Commun. (2018) 9:1208. doi: 10.1038/s41467-018-03525-0
141. Sandforth L, Ammar N, Dinges LA, Röcken C, Arlt A, Sebens S, et al. Impact of the monocarboxylate transporter-1 (MCT1)-mediated cellular import of lactate on stemness properties of human pancreatic adenocarcinoma cells†. Cancers. (2020) 12:581. doi: 10.3390/cancers12030581
142. Chatterjee P, Bhowmik D, Roy SS. A systemic analysis of monocarboxylate transporters in ovarian cancer and possible therapeutic interventions. Channels (Austin Tex). (2023) 17:2273008. doi: 10.1080/19336950.2023.2273008
143. Khan A, Valli E, Lam H, Scott DA, Murray J, Hanssen KM, et al. Targeting metabolic activity in high-risk neuroblastoma through Monocarboxylate Transporter 1 (MCT1) inhibition. Oncogene. (2020) 39:3555–70. doi: 10.1038/s41388-020-1235-2
Keywords: tumor microenvironment, lactate metabolism, tumor-associated macrophages, immune regulation, cancer therapy, metabolic reprogramming
Citation: Jin X, Zhang N, Yan T, Wei J, Hao L, Sun C, Zhao H and Jiang S (2025) Lactate-mediated metabolic reprogramming of tumor-associated macrophages: implications for tumor progression and therapeutic potential. Front. Immunol. 16:1573039. doi: 10.3389/fimmu.2025.1573039
Received: 08 February 2025; Accepted: 21 April 2025;
Published: 13 May 2025.
Edited by:
Hoang V. Le, National Institute on Drug Abuse (NIH), United StatesReviewed by:
Sevasti Karaliota, National Cancer Institute at Frederick (NIH), United StatesSonja Decking, University Medical Center Regensburg, Germany
Copyright © 2025 Jin, Zhang, Yan, Wei, Hao, Sun, Zhao and Jiang. This is an open-access article distributed under the terms of the Creative Commons Attribution License (CC BY). The use, distribution or reproduction in other forums is permitted, provided the original author(s) and the copyright owner(s) are credited and that the original publication in this journal is cited, in accordance with accepted academic practice. No use, distribution or reproduction is permitted which does not comply with these terms.
*Correspondence: Shulong Jiang, am5zbGppYW5nQDE2My5jb20=; Haibo Zhao, emhieXNAc2luYS5jb20=