- Hospital of Chengdu University of Traditional Chinese Medicine, Chengdu, China
Sepsis is a life-threatening condition caused by a dysregulated host response to infection, remaining a major global health challenge despite clinical advances. Therapeutic challenges arise from antibiotic misuse, incomplete understanding of its complex pathophysiology, and the unresolved interplay of immune dysregulation and microbiota disruption. Investigating microbial homeostasis in the shift from cytokine storm to immunosuppression may elucidate the interplay between microbial metabolites, immune dysfunction, and organ injury, providing a foundation for targeted therapies and drug development. Traditional Chinese Medicine (TCM) has demonstrated significant advantages in mitigating sepsis-associated cytokine storms and modulating gut microbiota homeostasis, offering a promising strategy for developing highly effective and less toxic targeted monomeric compounds. Elucidating the interactions within the immune-inflammation-microbiota network in sepsis paves the way for biomarker-driven personalized therapeutic approaches.
1 Introduction
Sepsis is a systemic inflammatory response syndrome (SIRS) triggered by infection, fundamentally characterized by an uncontrolled immune response to pathogens, resulting in immune dysregulation, multiple organ dysfunction, and potentially life-threatening conditions (1). According to the 2016 Sepsis-3.0 consensus definition (2, 3), sepsis is defined as life-threatening organ dysfunction caused by a dysregulated host response to infection (4), typically quantified by an increase in the Sequential Organ Failure Assessment (SOFA) score of ≥2 points (5). Sepsis imposes a substantial global health burden, affecting approximately 48.9 million individuals and accounting for 20% of all global deaths in 2017 (6).
The mortality rate of non-shock sepsis ranges from 25% to 30%, while septic shock, characterized by profound circulatory and metabolic abnormalities, has a mortality rate of approximately 42.3% (7–9). The prevention, management, and prognosis of sepsis are influenced by regional economic status and healthcare resources (10). Patients in high-income countries (HICs) generally have better outcomes, whereas those in low- and middle-income countries (LMICs) face higher rates of misdiagnosis and adverse outcomes due to resource limitations and reduced efficiency in sepsis recognition and management (6). Moreover, non-infectious inflammatory conditions, such as trauma and pancreatitis, can mimic sepsis symptoms, leading to misdiagnosis rates of 15–40% (11, 12). Therefore, the development of more effective treatment strategies and the improvement of sepsis diagnostic accuracy are critical approaches to reducing sepsis-related mortality.
The key to improving diagnostic accuracy lies in elucidating the specific pathological mechanisms and etiologies of sepsis. A multidimensional integrated therapeutic strategy based on the immune-inflammatory-microbial regulatory network, including targeting cytokine storms, modulating immune cell dysfunction, and restoring microbial homeostasis, serves as the critical scientific foundation for mitigating sepsis-associated tissue damage and improving patient outcomes.
During the progression of sepsis, pathogenic microorganisms interact with host pattern recognition receptors (PRRs) (13), through their pathogen-associated molecular patterns (PAMPs) (14). activating signaling pathways such as nuclear factor-kappa B (NF-κB), mitogen-activated protein kinase (MAPK), and JAK-STAT, thereby triggering acute inflammatory responses (15), Immune cells, including macrophages, neutrophils, and dendritic cells, secrete cytokines and chemokines such as TNF-α, IL-1β, and IL-6, which amplify the immune response (16).
As sepsis progresses, the excessive activation of the immune-inflammatory response not only exacerbates the cytokine storm but also triggers complex feedback mechanisms within the immune system (17), with immunosuppression emerging as the most common pathological process in the later stages of sepsis (18). Elucidating the key mechanisms underlying immunosuppression is critical for the development of targeted therapies and the implementation of precision medicine. During the pathophysiological process of sepsis, the interaction between pathogenic microorganisms and host immune cells forms a dynamic and tightly interconnected microbiota-immune network (19). Microbiota dysbiosis stimulates inflammatory responses through the Toll-like receptor 4 (TLR4)/myeloid differentiation primary response 88 (MyD88) signaling pathway (20) and impairs intestinal barrier function via the phosphoinositide 3-kinase (PI3K)/Akt pathway (21), facilitating the translocation of pathogenic bacteria into the bloodstream and exacerbating systemic inflammation (22, 23). The dynamic interplay between inflammation and immunosuppression plays a pivotal role in the progression of sepsis (24), not only determining the pathological trajectory but also directly influencing clinical outcomes. Therefore, a comprehensive understanding of the immune-inflammatory-microbiota network (25), particularly the interactions among signaling pathways and regulatory factors, provides a critical foundation for the development of effective therapeutic strategies.
This review summarizes the dysregulation of the immune-inflammatory-microbiota network and its key mechanisms in the progression of sepsis. It analyzes the interactions among immune responses, inflammatory reactions, and microbiota dysbiosis, and explores how these mechanisms offer new therapeutic targets and potential strategies for sepsis treatment. By delving into the underlying mechanisms of this network, the review provides new perspectives for precision therapeutic approaches in sepsis, aiming to advance treatment strategies and improve clinical outcomes.
2 Review methodology
A review was conducted on the regulatory role of the immune-inflammation-microbiota network and its mechanisms in sepsis treatment based on the guidelines of the Society of Critical Care Medicine (SCCM) and the European Society of Intensive Care Medicine (ESICM). Data were collected from research articles published before March 2020 in Web of Science, PubMed, ScienceDirect, and CNKI. This information was utilized to summarize the critical roles of immunity, inflammation, and microbiota in sepsis progression, as well as the activators and inhibitors of key targets, with a particular emphasis on the regulatory effects of traditional Chinese medicine monomers on sepsis. The inclusion criteria were as follows: (a) clinical studies; (b) reviews discussing sepsis-related mechanisms, including inflammation, immunity, and microbiota. The exclusion criteria included: (a) mini-reports; (b) studies without comparisons with models; (c) duplicate articles. The review focused on keywords present in the title, abstract, or full text to ensure research reliability. A total of 157 articles were included for review (Figure 1).
3 Pathophysiological mechanisms of sepsis
3.1 Early cytokine storm and immune homeostasis imbalance in sepsis
Early biomarkers of sepsis include C-reactive protein (CRP) and procalcitonin (PCT) (26), which help distinguish bacterial infections from sterile inflammation. Soluble triggering receptor expressed on myeloid cells-1 (sTREM-1) serves as an indicator of innate immune activation and is significantly elevated in bacterial sepsis (27). Additionally, CD64 expression levels reflect neutrophil activation (28), providing potential value for early sepsis diagnosis. The early stage of systemic inflammatory response syndrome (SIRS) is marked by a robust cytokine storm, which can subsequently evolve into compensatory anti-inflammatory response syndrome (CARS) or persistent inflammation (29), immunosuppression, and catabolism syndrome (PICS) (30). Throughout this pathological progression, maintaining the dynamic equilibrium of the immune regulatory network is critical. Achieving immune homeostasis necessitates the precise spatiotemporal modulation of pro-inflammatory mediators (IL-1β, TNF-α, and IL-6) alongside anti-inflammatory mediators (IL-10 and TGF-β) (18).
The biological foundation of this therapeutic approach relies on an in-depth understanding of the functional interplay within the network of innate immune cells, including macrophages (31), neutrophils (32), and dendritic cells (33). In sepsis, microbiota dysbiosis exacerbates the aberrant activation of these cells through the gut-immune axis (34). The disruption of this balance impairs the normal functioning of the immune response, leading to an immunosuppressive state that further exacerbates immune dysfunction in sepsis.
3.2 Mechanisms of macrophage pro-inflammatory to immune suppressive transition in sepsis
Macrophages exhibit a dynamic dual regulatory role in the pathophysiology of sepsis. During the early stages of infection, macrophages recognize PAMPs (35), such as LPS from Gram-negative bacteria, through Toll-like receptors (TLRs), thereby initiating the immune response (36).
This process involves the activation of both MyD88-dependent and TRIF-dependent signaling pathways (37). Upon immune activation, the nuclear translocation of NF-κB and interferon regulatory factors (IRFs) occurs (38), promoting the transcription and secretion of pro-inflammatory cytokines such as TNF-α, IL-1β, and IL-6. Additionally, chemokines mediate the recruitment of immune cells, including neutrophils and monocytes, to the site of infection, thereby initiating a local inflammatory response to eliminate the pathogens (31). As sepsis progresses, macrophages transition from classically activated M1 macrophages to alternatively activated M2 macrophages (16). This shift is accompanied by the negative regulation of NF-κB and MAPK signaling pathways (39), resulting in a decrease in pro-inflammatory cytokine production and an upregulation of immunosuppressive mediators, such as interleukin-10 (IL-10) and TGF-β. Concurrently, immune checkpoint molecules (ICMs) such as programmed cell death protein-1 (PD-1) (40) and T-cell immunoglobulin and mucin domain-containing molecule-3 (TIM-3) are significantly upregulated on the surface of macrophages (41). These molecules transmit inhibitory signals that further impair the antigen-presenting capacity of macrophages, contributing to the suppression of the immune response.
The establishment of this immunotolerant state is closely linked to epigenetic modifications, such as histone deacetylation, and metabolic reprogramming, such as the shift toward aerobic glycolysis. These processes ultimately lead to immunoparalysis (42), which increases the risk of secondary infections and promotes the onset and progression of multiple organ dysfunction syndrome (MODS) (43).
3.3 Dual role of neutrophils in immune defense and tissue injury in sepsis
Neutrophils play a dual role in the immune pathological process of sepsis, acting both as key effector cells of innate immune defense and as important participants in inflammation-mediated tissue damage (32). As the first immune effector cells recruited to the site of infection, neutrophils recognize PAMPs via TLRs and other PRRs, initiating intracellular signaling cascades (44). By activating the NF-κB and MAPK pathways, they release pro-inflammatory cytokines TNF-α, IL-1β, chemokine CXCL8, and antimicrobial peptides such as cathelicidins (45), thereby forming a local inflammatory microenvironment to eliminate pathogens.
During the acute phase of sepsis, neutrophil functions such as phagocytosis, degranulation, and respiratory burst activity are enhanced, processes that play a critical role in pathogen clearance. As the disease progresses, neutrophil dysfunction occurs, leading to an overactivation of the immune response accompanied by persistent inflammation (46). This abnormal state results in the release of large amounts of reactive oxygen species (ROS) and proteolytic enzymes (such as elastase and matrix metalloproteinases, MMPs) (47), which directly damage endothelial cells through oxidative stress and proteolysis, compromising the integrity of the endothelial barrier. Concurrently, microvascular permeability increases, and extracellular matrix (ECM) components are degraded (48), exacerbating the SIRS and multiple organ dysfunction (MOD). Neutrophils participate in the pathological progression of sepsis through the formation of neutrophil extracellular traps (NETs) (49). The formation of NETs relies on histone citrullination and the release of granzyme, with their structure composed of chromatin DNA, histones, and granule proteins. Although NETs exert antibacterial effects by capturing pathogens, their excessive release can lead to microvascular thrombosis and tissue ischemia-reperfusion injury (50). Additionally, NETs activate the complement system and TLR signaling pathways, promoting the cascade release of pro-inflammatory cytokines. This forms a positive feedback loop, further exacerbating immune imbalance and tissue damage. NETs-associated molecules, such as cfDNA and MPO-DNA complexes, can also serve as damage-associated molecular patterns (DAMPs), which exacerbate the inflammatory response by activating pathways like the NLRP3 inflammasome (51). Therefore, neutrophil dysregulation and abnormal activation play a key role in sepsis and are important driving factors of sepsis-associated multiple organ failure (MOF). Targeting the regulation of neutrophil activation, NET formation, and the inflammation-mediated damage they induce may offer new therapeutic intervention targets for sepsis.
3.4 Dual role of dendritic cells in immune activation and tolerance in sepsis
Dendritic cells (DCs), as key antigen-presenting cells (APCs), play a crucial role in the pathological progression of sepsis by recognizing PAMPs via PRRs (52). This recognition triggers antigen processing and presentation, thereby activating specific immune responses. In the early stages of sepsis, dendritic cells (DCs) promote Th1-type immune polarization through the expression of TLRs and C-type lectin receptors (CLRs) on their surface (53). They secrete pro-inflammatory cytokines, such as IL-12 and IL-6, enhancing the host’s response to pathogens.
However, as sepsis progresses to its late stages, dendritic cells (DCs) undergo a functional phenotypic shift from an immune activation state to an immune tolerance state. This process is closely related to the negative feedback regulation induced by the excessive activation of TLR signaling pathways. Additionally, DCs are regulated by immune-suppressive mediators secreted by myeloid-derived suppressor cells (MDSCs) (54) and regulatory T cells (Tregs), such as transforming growth factor-β (TGF-β) and IL-10 (55). Refer to Table 1 for information about immune factors that play a key role in sepsis. The dysfunction of DCs not only weakens pathogen clearance efficiency but also promotes the expression of immune checkpoint molecules (such as PD-1 and CTLA-4) (56), inhibiting T cell activation and leading to acquired immune deficiency.
Moreover, tolerant DCs further reinforce the immunosuppressive microenvironment by secreting immune-suppressive cytokines, such as IL-10 and TGF-β, and inducing the expansion of Tregs, thus forming a positive feedback loop (33). The persistence of this immune tolerance state promotes the chronic progression of sepsis, increasing the risk of secondary infections and accelerating the development of multiple organ dysfunction syndrome (MODS) (57). Therefore, DCs play a dual role in the immune regulatory network of sepsis, acting as a bridge between innate and adaptive immunity, while also serving as central regulators of the immunosuppressive state. Three cell types play critical roles in the progression of sepsis. Their specific mechanisms and interactions are illustrated in Figure 2.
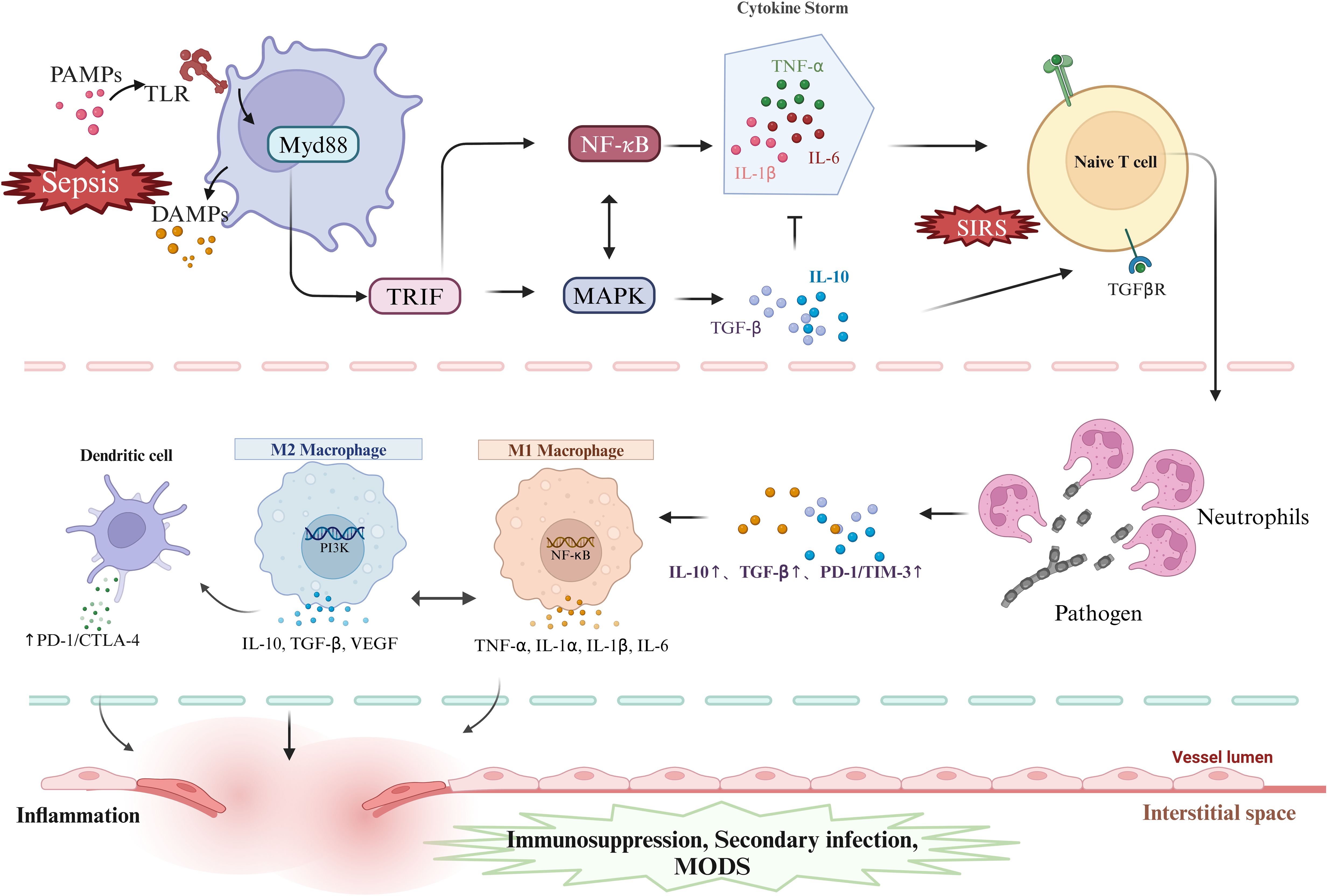
Figure 2. DAMPs and PAMPs activate PRRs, triggering MyD88- and TRIF-dependent pathways, which in turn activate NF-κB and MAPK signaling, driving the release of pro- and anti-inflammatory cytokines and promoting T cell activation. Neutrophils regulate inflammation by sensing cytokine shifts and enhancing anti-inflammatory responses. In sepsis, macrophages shift from M1 to M2 phenotypes, balancing inflammation and repair but risking immunosuppression and secondary infections. PAMPs, pathogen-associated molecular patterns; TLR, Toll-like receptors; Myd88, myeloid differentiation factor 88;TRIF,Toll-like receptor interleukin-1 receptor domain-containing adaptor inducing interferon-β; MAPK, mitogen-activated protein kinase; NF-κB, nuclear transcription factor-kB; TNF-α, tumor necrosis factor-alpha; IL-6,interleukin-6; IL-1α,interleukin-1α; IL-1β, interleukin-1β; IL-10, interleukin-10; TGF-β, transforming growth factor-β; TGF-βR, transforming growth factor-β receptor; SIRS, Systemic Inflammatory Response Syndrome; PD-1, programmed cell death protein 1; CTLA-4, Cytotoxic T Lymphocyte-Associated Antigen-4; TIM-3, T cell immunoglobulin and mucin domain-containing protein 3; MODS, Multiple Organ Dysfunction Syndrome; PI3K, Phosphatidylinositol 3-kinase. Created in https://BioRender.com.
4 Immune balance in sepsis
4.1 Immune imbalance and regulation via PAMPs and PRRs
The inflammatory response in sepsis is triggered by the interaction between PAMPs and host PRRs. PAMPs, such as bacterial LPS, viral single-stranded RNA, and fungal β-glucans, bind to PRRs (70), thereby activating the host immune response and initiating a systemic inflammatory reaction. TLR4, as a key receptor, mediates the effects of LPS by activating the MyD88- dependent signaling pathway (71). This activation subsequently triggers extracellular signal-regulated kinases (ERK1/2), p38, c-Jun N-terminal kinase (JNK), and NF-κB, leading to the synthesis and secretion of pro-inflammatory cytokines such as IL-1β, TNF-α, and IL-6 (72). These pro-inflammatory cytokines circulate to tissues and organs, activating immune cells and amplifying inflammation. Endogenous HMGB1 is released extracellularly during cell injury or death, activating TLRs and RAGE receptors and regulating NF-κB and MAPK pathways to trigger inflammation (73). HMGB1 plays a key role in inflammasome activation, autophagy, cell survival, coagulation, and immune regulation. Studies have shown that macrophage activation under LPS stimulation is closely associated with HMGB1 release, in which oxidative stress and calcium signaling play critical roles (74). Post-translational modifications of HMGB1 not only contribute to inflammasome activation but also regulate cell death pathways such as pyroptosis (75). These mechanisms interact synergistically, exacerbating inflammation and immune dysregulation in sepsis.
Different types of PAMPs and DAMPs activate multiple signaling pathways through PRRs, collectively driving the pathogenesis of sepsis and promoting the interplay between systemic inflammatory responses and immunosuppressive states. The key information regarding DAMPs, PAMPs, and immune regulatory factors is summarized in Table 2.
4.2 Core mechanisms of immune imbalance and multi-organ failure in sepsis
Cytokine-induced cytokine storms are a key feature in the progression of sepsis. In the early stages of sepsis, the binding of PAMPs to PRRs leads to excessive activation of immune cells (94). A large accumulation of inflammatory factors such as IL-1β, TNF-α, IL-6, and IL-8 occurs in tissues, with the activation of signaling pathways like NF-κB, MAPK, and JAK/STAT, enhancing cellular immune responses (95). When the immune system is excessively activated, it leads to a systemic inflammatory response, triggering multiple organ dysfunction syndrome (MODS) and microcirculatory disturbances. IL-6, as a central cytokine in the early inflammatory response of sepsis, exerts its effects through the JAK/STAT3 signaling pathway. Upon binding to its receptor, IL-6 activates JAK kinases and promotes the phosphorylation of the STAT3 transcription factor, which subsequently induces the secretion of CRP and PCT (26). The dramatic increase in these acute-phase proteins can serve as biomarkers for the diagnosis of sepsis. Within the threshold of homeostatic balance, these key factors enhance the immune response to combat infection. However, when this balance is disrupted, the excessive release of inflammatory cytokines such as TNF-α, IL-1β, and IL-6 leads to endothelial cell damage, increased vascular permeability, leukocyte infiltration, and the formation of a negative feedback loop that exacerbates the systemic inflammatory response in sepsis.
Within the threshold of homeostatic balance, these key factors enhance the immune response to combat infection. However, when this balance is disrupted, the excessive release of inflammatory cytokines such as TNF-α, IL-1β, and IL-6 leads to endothelial cell damage (96), increased vascular permeability, leukocyte infiltration, and the formation of a negative feedback loop that exacerbates the systemic inflammatory response in sepsis. Therefore, the cytokine storm and immune dysregulation are crucial interactive components in the pathological process of sepsis. Excessive release of pro-inflammatory cytokines disrupts immune balance, while immune suppression in the later stages of sepsis leads to the loss of the body’s defense mechanisms. Maintaining the balance of cytokine levels and the immune system may be key to reducing sepsis mortality in the future.
4.3 Inflammation-driven immune suppression in sepsis
The dynamic balance between immune function and inflammation is crucial, with immune suppression being key to improving patient prognosis. Understanding the specific mechanisms of excessive immune activation and immune suppression is fundamental for future research and clinical diagnosis. Immune suppression primarily occurs in the later stages of sepsis, characterized by a significant upregulation of PD-1 and cytotoxic T lymphocyte antigen 4 (CTLA-4), which suppresses T cell activation and proliferation, weakening the intensity of the immune response (40). At this stage, tissues counteract inflammation by promoting the expression of IL-10 and transforming growth factor-beta (TGF-β) (64), reducing the immune system’s response to infection. IL-10, as a key factor, inhibits the NF-κB and Janus kinase/signal transducer and activator of transcription (JAK/STAT) pathways, playing a crucial role during the immune suppression phase. TGF-β reduces the intensity of the immune response by inhibiting T cell activation and regulating the Th1/Th2 balance (97).
In addition to cytokines, macrophage polarization plays a crucial role in the interplay between immune suppression and inflammation. During sepsis, macrophages transition from the M1 phenotype (pro-inflammatory) to the M2 phenotype (immunosuppressive) (16), with M2 macrophages subsequently secreting immune-regulatory factors such as IL-10 and TGF-β to suppress inflammation and promote immune tolerance. When this balance is disrupted, the activity of T cells, B cells, and natural killer (NK) cells significantly declines, leading to a compromised inflammatory sensing system and an increased risk of secondary infections (79). Current clinical interventions for sepsis primarily focus on anti-inflammatory and immune-regulatory restoration. Studies have shown that TNF-α-targeting monoclonal antibodies such as Infliximab and Adalimumab can significantly reduce the excessive activation of the inflammatory response (98). However, these drugs often have immunosuppressive side effects, potentially increasing the risk of infections.
Monoclonal antibodies targeting PD-1 and CTLA-4 can reduce the risk of excessive immune activation in the early stages of immune suppression. While these strategies offer new treatment possibilities, their use in sepsis remains limited (99). Understanding the mechanisms of immune suppression-inflammation interplay is crucial for future research and clinical applications. Balancing the inhibition of excessive inflammation with the restoration of immune defense will be a key challenge in sepsis treatment.
5 Regulatory roles of the microbial network in sepsis
5.1 The critical role of gut microbiota in sepsis
In the immune dysregulation process of sepsis, the gut microbiota plays a key regulatory role in immune responses through its metabolic products, particularly short-chain fatty acids (SCFAs). SCFAs (100), including acetate, propionate, and butyrate, are produced by gut bacterial fermentation and play a crucial role in regulating immune cell functions, maintaining immune tolerance, and controlling systemic inflammation. SCFAs activate immune cells by binding to G-protein-coupled receptors GPR41 and GPR43. Studies have shown that acetate can promote the differentiation of T regulatory cells (Treg) through the GPR43 receptor, enhancing immune tolerance and suppressing pro-inflammatory responses by reducing the number of Th17 cells (101). In the context of sepsis, dysbiosis of the gut microbiota leads to further disruption of inflammation and immune responses, accompanied by a decrease in beneficial bacteria and excessive proliferation of pathogens, which in turn suppresses SCFA production and reduces the body’s ability to combat cachexia (101).
The primary mechanism of intestinal barrier damage involves the binding of LPS to the TLR4 receptor on cell surfaces during sepsis, which activates the downstream adaptor molecule MyD88 (81). This adaptor molecule recruits IRAK and TBK1, leading to the activation of the IκB kinase (IKK) complex. IKK promotes the phosphorylation and subsequent degradation of IκB, releasing NF-κB (38), which enhances the transcription of myosin light-chain kinase (MLCK). Increased phosphorylation of myosin light chain (MLC) promotes the contraction of actin-myosin filaments in the intestine, exacerbating intestinal permeability (102). This allows more bacteria, endotoxins, and PAMPs to infiltrate tissues, further triggering systemic inflammatory responses. Various cells and microorganisms are essential for intestinal function and health.
5.2 Restoring microbial homeostasis to regulate inflammation in sepsis
In sepsis, the gut microbiota plays a dual role: under homeostatic conditions, the microbiota maintains intestinal epithelial barrier integrity and regulates local immune homeostasis through competitive colonization, secretion of antimicrobial peptides (AMPs), and the production of metabolic products such as short-chain fatty acids (SCFAs) (103). During sepsis treatment, the overuse of antibiotics, nutritional support, and other clinical interventions often lead to dysbiosis. This promotes the overgrowth of pathogenic bacteria, such as Enterococcus spp, Klebsiella pneumoniae, and Escherichia coli, exacerbating systemic inflammatory responses and immune dysregulation (104).
Current therapeutic strategies for microbial disruption caused by sepsis include fecal microbiota transplantation (FMT), as well as supplementation with probiotics and prebiotics, aiming to restore intestinal microbial homeostasis (105, Figure 3). Studies have indicated that FMT can restore the relative abundance of Firmicutes and Bacteroidetes phyla in some septic patients, increase SCFA levels, and subsequently reduce serum levels of inflammatory markers, such as C-reactive protein and procalcitonin, as well as pro-inflammatory cytokines (105). The development of novel drug targets for microbiome-immune network regulation is currently a major research focus. Studies have revealed that PAMPs (such as LPS, bacterial RNA, and fungal β-glucan) and DAMPs (14), including HMGB1, ATP, and uric acid, regulate inflammation and immune cells through PRRs like TLR4, receptor for advanced glycation end products (RAGE), and TLR9. These interactions modulate key signaling pathways such as NF-κB (49). Gut homeostasis is influenced by Methanobrevibacter smithii (106), whose overgrowth during sepsis disrupts microbial balance and promotes bacterial translocation, ultimately compromising intestinal health. Desulfovibrio spp produces hydrogen sulfide (H2S) (107), which impairs the intestinal barrier and induces oxidative stress and inflammation in the septic environment. In summary, treatment strategies based on the immune-inflammation-microbiome network regulation, aimed at restoring a healthy microbial ecosystem and targeting key signaling pathways, are among the promising approaches to improve sepsis treatment efficacy. We have compiled key information on probiotics used in sepsis therapy, as shown in Table 3. Further mechanistic research and large-scale clinical trials will provide more evidence in this area and promote the clinical application of novel immunoregulatory therapies.
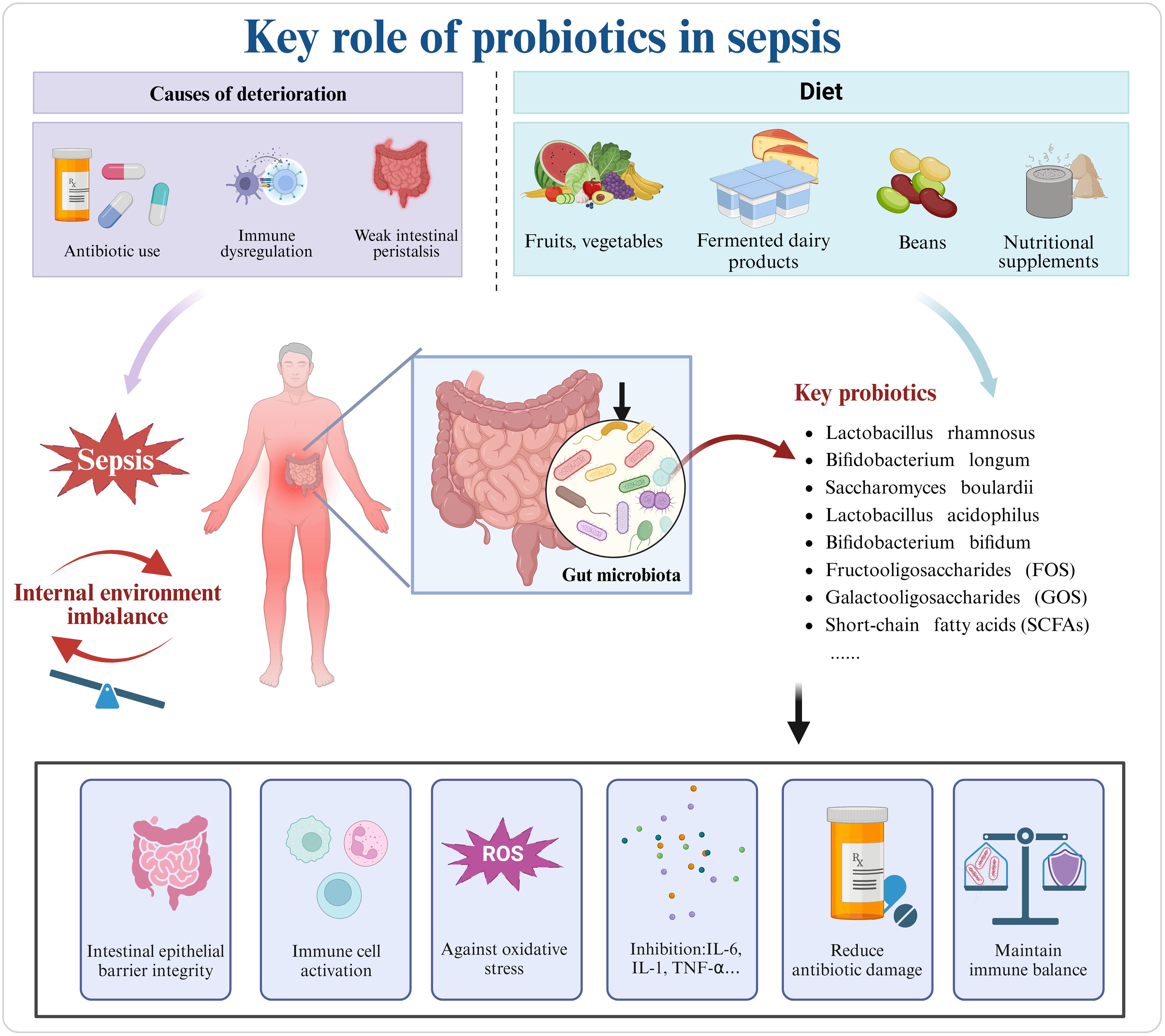
Figure 3. In sepsis, the misuse of antibiotics, immune dysregulation, and gastrointestinal motility issues exacerbate disease progression. Key probiotics, commonly supplemented through diet, can be obtained from vegetables, fruits, dairy products, legumes, and supplements. Probiotics can mitigate sepsis-induced damage by reconstructing the intestinal barrier, regulating immune cells, and combating oxidative stress. Created in https://BioRender.com.
6 TCM in gut microbiota and inflammation regulation in sepsis
Antibiotic therapy is a primary treatment for sepsis, but it has the side effect of disrupting the gut microbiota, potentially exacerbating the disease. In contrast, TCM has advantages in modulating microbial balance while addressing inflammation and immune responses. Xuebijing injection (XBJ), a Chinese herbal remedy, has been clinically approved for sepsis treatment. It primarily works by inhibiting NF-κB and GSK-3β signaling pathways (117), reducing pro-inflammatory cytokine release, and regulating the differentiation of Treg/Th17 cells in the treatment of sepsis.
Research has indicated that traditional Chinese medicine formulas, such as Dachengqi Decoction and Huanglian Jiedu Decoction (118), can improve intestinal barrier function and systemic immune response by repairing capillary permeability and regulating the TLR4/NF-κB and Akt/HO-1 signaling pathways. The combination of Huanglian can inhibit the inflammatory cascade induced by sepsis (119).
The use of individual herbs is also common, with species like Cordyceps sinensis, Rhizoma Coptidis (120), and Borneol improving LPS-induced brain injury following sepsis by regulating the HO-1, NOS2, and NF-κB/MAPK pathways (121). Well-known active compounds from Chinese herbal medicine, such as Pristimerin, Danshensu IIA, Cinnamyl alcohol, and Ginsenoside Rg3, have shown excellent anti-inflammatory effects. The information on other key individual active compounds from traditional Chinese medicine can be found in Table 4.
Traditional Chinese medicine compounds not only have the advantage of fewer side effects, but also interact directly with the gut microbiota after oral administration. With further research into the identification and pharmacological effects of these compounds, different key individual active ingredients with significant effects are becoming crucial strategies for the future treatment of sepsis.
7 Discussion
The core challenge in sepsis treatment lies in balancing immune mechanisms and inflammatory responses, particularly with the widespread use of antibiotics, which often disrupt the gut microbiota and further complicate and imbalance immune responses. This review systematically integrates the latest advancements in the regulation mechanisms of immunity, inflammation, and microbiota in sepsis treatment, emphasizing the pivotal role of the immune-inflammation-microbiota network in effective sepsis management. Unlike previous reviews that focus on individual mechanisms, we provide a holistic explanation of the dynamic balance between immune activation, inflammatory storms, and immune suppression triggered by infection. We present the dynamic process of functional phenotype transitions in immune cells (such as macrophages, neutrophils, and dendritic cells) and their impact on disease outcomes. Additionally, we explore the bidirectional effects of gut microbiota dysbiosis on systemic inflammation and immune dysfunction.
From a systemic perspective, the dynamic interplay between immune cell phenotype transitions, inflammatory storms, and immune suppression plays a key role in the progression of sepsis. Furthermore, the critical role of the microbiota and its metabolites, especially short-chain fatty acids, in immune regulation requires more attention. Although antibiotics are an important tool in the treatment of sepsis, their disruption of the gut microbiota can exacerbate endotoxin translocation and inflammatory responses, leading to multi-organ dysfunction. Unlike single-target therapies, traditional Chinese medicine enhances the multifaceted regulation of the immune system.
Traditional Chinese medicine offers the unique advantage of direct interaction with the gut microbiota through oral administration. Its components, such as flavonoids, polysaccharides, and saponins, not only exert significant anti-inflammatory, antioxidant, and immune-regulating effects but also promote the production of short-chain fatty acids (SCFAs), thereby restoring gut barrier function. Chinese patent medicines, herbal formulas, compound prescriptions, single herbs, and active ingredients (such as Pristimerin, tanshinone IIA, cinnamaldehyde, ginsenoside Rg3, etc.) play a role in regulating key signaling pathways like NF-κB, MAPK, JAK/STAT, PI3K/Akt, AMPK, and GSK-3β. These mechanisms present potential strategies to improve cure rates and patient prognosis. The multi-target combination therapy based on the immune-inflammation-microbiota network offers a new approach to addressing antibiotic resistance, uncontrolled inflammation, and immune suppression in sepsis treatment. It also opens new directions for the precision treatment of sepsis using traditional Chinese medicine. Personalized immunotherapy is a key focus in future sepsis research. Identifying critical targets through biomarker detection, tracking targeted modulation, and dynamically monitoring immune responses are essential for elucidating sepsis pathogenesis and guiding precise interventions.
Future research should further clarify the molecular mechanisms and key targets of the immune-inflammatory-microbial network regulatory system, aiming to improve the clinical outcomes of sepsis patients through multi-level interventions. In summary, this review integrates the latest research findings on immune, inflammatory, and microbial network regulation, not only enhancing the understanding of comprehensive treatment strategies for sepsis but also providing new theoretical foundations for future research and clinical applications.
8 Conclusion
Effective sepsis treatment relies on maintaining the balance between immunity, inflammation, and microbiota, as well as ensuring inter-organ coordination and homeostasis. Adopting a holistic network perspective of organ interactions and developing targeted therapies based on the immune-inflammation-microbiota network are critical strategies for enhancing treatment efficacy and improving patient outcomes.
Author contributions
YX: Writing – original draft, Writing – review & editing. JW: Writing – original draft. RY: Supervision, Writing – review & editing. ZQ: Supervision, Writing – review & editing. KL: Supervision, Writing – review & editing. PG: Writing – review & editing.
Funding
The author(s) declare that financial support was received for the research and/or publication of this article. Sichuan Provincial Administration of Traditional Chinese Medicine: Clinical Efficacy Evaluation Study of Wenshen Yangxin Mixture in the Treatment of Sepsis-Induced Cardiomyopathy (SIC) (Project Number: 2023zd018).
Conflict of interest
The authors declare that the research was conducted in the absence of any commercial or financial relationships that could be construed as a potential conflict of interest.
Generative AI statement
The author(s) declare that no Generative AI was used in the creation of this manuscript.
Publisher’s note
All claims expressed in this article are solely those of the authors and do not necessarily represent those of their affiliated organizations, or those of the publisher, the editors and the reviewers. Any product that may be evaluated in this article, or claim that may be made by its manufacturer, is not guaranteed or endorsed by the publisher.
References
1. Fleischmann C, Scherag A, Adhikari NK, Hartog CS, Tsaganos T, Schlattmann P, et al. Assessment of global incidence and mortality of hospital-treated sepsis. Current estimates and limitations. Am J Respir Crit Care Med. (2016) 193:259–72. doi: 10.1164/rccm.201504-0781OC
2. Zhu Y, Zhang R, Ye X, Liu H, Wei J. SAPS III is superior to SOFA for predicting 28-day mortality in sepsis patients based on Sepsis 3.0 criteria. Int J Infect diseases: IJID. (2022) 114:135–41. doi: 10.1016/j.ijid.2021.11.015
3. Wang N, Wu R, Comish PB, Kang R, Tang D. Pharmacological modulation of BET family in sepsis. Front Pharmacol. (2021) 12:642294. doi: 10.3389/fphar.2021.642294
4. van der Poll T, van de Veerdonk FL, Scicluna BP, Netea MG. The immunopathology of sepsis and potential therapeutic targets. Nature reviews. Immunology. (2017) 17:407–20. doi: 10.1038/nri.2017.36
5. Bauer M, Gerlach H, Vogelmann T, Preissing F, Stiefel J, Adam D. Mortality in sepsis and septic shock in Europe, North America and Australia between 2009 and 2019- results from a systematic review and meta-analysis. Crit Care (London England). (2020) 24:239. doi: 10.1186/s13054-020-02950-2
6. Cecconi M, Evans L, Levy M, Rhodes A. Sepsis and septic shock. Lancet (London England). (2018) 392:75–87. doi: 10.1016/s0140-6736(18)30696-2
7. Singer M, Deutschman CS, Seymour CW, Shankar-Hari M, Annane D, Bauer M, et al. The third international consensus definitions for sepsis and septic shock (Sepsis-3). JAMA. (2016) 315:801–10. doi: 10.1001/jama.2016.0287
8. Evans L, Rhodes A, Alhazzani W, Antonelli M, Coopersmith CM, French C, et al. Surviving sepsis campaign: international guidelines for management of sepsis and septic shock 2021. Intensive Care Med. (2021) 47:1181–247. doi: 10.1007/s00134-021-06506-y
9. Lei S, Li X, Zhao H, Xie Y, Li J. Prevalence of sepsis among adults in China: A systematic review and meta-analysis. Front Public Health. (2022) 10:977094. doi: 10.3389/fpubh.2022.977094
10. Font MD, Thyagarajan B, Khanna AK. Sepsis and Septic Shock - Basics of diagnosis, pathophysiology and clinical decision making. Med Clinics North America. (2020) 104:573–85. doi: 10.1016/j.mcna.2020.02.011
11. Wacker C, Prkno A, Brunkhorst FM, Schlattmann P. Procalcitonin as a diagnostic marker for sepsis: a systematic review and meta-analysis. Lancet Infect Dis. (2013) 13:426–35. doi: 10.1016/s1473-3099(12)70323-7
12. Bosmann M, Ward PA. The inflammatory response in sepsis. Trends Immunol. (2013) 34:129–36. doi: 10.1016/j.it.2012.09.004
13. Shomali N, Mahmoodpoor A, Abbas Abad AN, Marofi F, Akbari M, Xu H, et al. The relationship between extracellular/intracellular microRNAs and TLRs may be used as a diagnostic and therapeutic approach in sepsis. Immunol Invest. (2022) 51:154–69. doi: 10.1080/08820139.2020.1817067
14. Cicchinelli S, Pignataro G, Gemma S, Piccioni A, Picozzi D, Ojetti V, et al. PAMPs and DAMPs in sepsis: A review of their molecular features and potential clinical implications. Int J Mol Sci. (2024) 25:962. doi: 10.3390/ijms25020962
15. Xu J, Zhang X, Zhou M, Lu P, Xu Y, Wu L, et al. Bioactive compound C498-0670 alleviates LPS-induced sepsis via JAK/STAT and NFκB signaling pathways. Front Immunol. (2023) 14:1132265. doi: 10.3389/fimmu.2023.1132265
16. Jiao Y, Zhang T, Zhang C, Ji H, Tong X, Xia R, et al. Exosomal miR-30d-5p of neutrophils induces M1 macrophage polarization and primes macrophage pyroptosis in sepsis-related acute lung injury. Crit Care (London England). (2021) 25:356. doi: 10.1186/s13054-021-03775-3
17. Venet F, Monneret G. Advances in the understanding and treatment of sepsis-induced immunosuppression. Nat Rev Nephrol. (2018) 14:121–37. doi: 10.1038/nrneph.2017.165
18. Liu D, Huang SY, Sun JH, Zhang HC, Cai QL, Gao C, et al. Sepsis-induced immunosuppression: mechanisms, diagnosis and current treatment options. Mil Med Res. (2022) 9:56. doi: 10.1186/s40779-022-00422-y
19. Zhang YY, Ning BT. Signaling pathways and intervention therapies in sepsis. Signal Transduct Target Ther. (2021) 6:407. doi: 10.1038/s41392-021-00816-9
20. Izadparast F, Riahi-Zajani B, Yarmohammadi F, Hayes AW, Karimi G. Protective effect of berberine against LPS-induced injury in the intestine: a review. Cell Cycle (Georgetown Tex.). (2022) 21:2365–78. doi: 10.1080/15384101.2022.2100682
21. Gay NJ, Symmons MF, Gangloff M, Bryant CE. Assembly and localization of Toll-like receptor signalling complexes. Nature reviews. Immunology. (2014) 14:546–58. doi: 10.1038/nri3713
22. Assimakopoulos SF, Eleftheriotis G, Lagadinou M, Karamouzos V, Dousdampanis P, Siakallis G, et al. SARS coV-2-induced viral sepsis: the role of gut barrier dysfunction. Microorganisms. (2022) 10:1050. doi: 10.3390/microorganisms10051050
23. Rowlands BJ, Soong CV, Gardiner KR. The gastrointestinal tract as a barrier in sepsis. Br Med Bull. (1999) 55:196–211. doi: 10.1258/0007142991902213
24. Barichello T, Generoso JS, Singer M, Dal-Pizzol F. Biomarkers for sepsis: more than just fever and leukocytosis-a narrative review. Crit Care (London England). (2022) 26:14. doi: 10.1186/s13054-021-03862-5
25. Adelman MW, Woodworth MH, Langelier C, Busch LM, Kempker JA, Kraft CS, et al. The gut microbiome’s role in the development, maintenance, and outcomes of sepsis. Crit Care (London England). (2020) 24:278. doi: 10.1186/s13054-020-02989-1
26. Schuetz P. How to best use procalcitonin to diagnose infections and manage antibiotic treatment. Clin Chem Lab Med. (2023) 61:822–8. doi: 10.1515/cclm-2022-1072
27. Llitjos JF, Carrol ED, Osuchowski MF, Bonneville M, Scicluna BP, Payen D, et al. Enhancing sepsis biomarker development: key considerations from public and private perspectives. Crit Care (London England). (2024) 28:238. doi: 10.1186/s13054-024-05032-9
28. Hoffmann JJ. Neutrophil CD64: a diagnostic marker for infection and sepsis. Clin Chem Lab Med. (2009) 47:903–16. doi: 10.1515/cclm.2009.224
29. Clere-Jehl R, Mariotte A, Meziani F, Bahram S, Georgel P, Helms J. JAK-STAT targeting offers novel therapeutic opportunities in sepsis. Trends Mol Med. (2020) 26:987–1002. doi: 10.1016/j.molmed.2020.06.007
30. Rosenthal MD, Vanzant EL, Moore FA. Chronic critical illness and PICS nutritional strategies. J Clin Med. (2021) 10:2294. doi: 10.3390/jcm10112294
31. Qiu P, Liu Y, Zhang J. Review: the role and mechanisms of macrophage autophagy in sepsis. Inflammation. (2019) 42:6–19. doi: 10.1007/s10753-018-0890-8
32. Zhang H, Wang Y, Qu M, Li W, Wu D, Cata JP, et al. Neutrophil, neutrophil extracellular traps and endothelial cell dysfunction in sepsis. Clin Trans Med. (2023) 13:e1170. doi: 10.1002/ctm2.1170
33. Zheng LY, Duan Y, He PY, Wu MY, Wei ST, Du XH, et al. Dysregulated dendritic cells in sepsis: functional impairment and regulated cell death. Cell Mol Biol Lett. (2024) 29:81. doi: 10.1186/s11658-024-00602-9
34. Xie B, Wang M, Zhang X, Zhang Y, Qi H, Liu H, et al. Gut-derived memory γδ T17 cells exacerbate sepsis-induced acute lung injury in mice. Nat Commun. (2024) 15:6737. doi: 10.1038/s41467-024-51209-9
35. Wu R, Wang N, Comish PB, Tang D, Kang R. Inflammasome-dependent coagulation activation in sepsis. Front Immunol. (2021) 12:641750. doi: 10.3389/fimmu.2021.641750
36. Murao A, Jha A, Aziz M, Wang P. Transcriptomic profiling of immune cells in murine polymicrobial sepsis. Front Immunol. (2024) 15:1347453. doi: 10.3389/fimmu.2024.1347453
37. Muendlein HI, Connolly WM, Cameron J, Jetton D, Magri Z, Smirnova I, et al. Neutrophils and macrophages drive TNF-induced lethality via TRIF/CD14-mediated responses. Sci Immunol. (2022) 7:eadd0665. doi: 10.1126/sciimmunol.add0665
38. Bergstrøm B, Aune MH, Awuh JA, Kojen JF, Blix KJ, Ryan L, et al. TLR8 Senses Staphylococcus aureus RNA in Human Primary Monocytes and Macrophages and Induces IFN-β Production via a TAK1-IKKβ-IRF5 Signaling Pathway. J Immunol (Baltimore Md.: 1950). (2015) 195:1100–11. doi: 10.4049/jimmunol.1403176
39. Wang Z, Wang Z. The role of macrophages polarization in sepsis-induced acute lung injury. Front Immunol. (2023) 14:1209438. doi: 10.3389/fimmu.2023.1209438
40. Chen R, Zhou L. PD-1 signaling pathway in sepsis: Does it have a future? Clin Immunol (Orlando Fla.). (2021) 229:108742. doi: 10.1016/j.clim.2021.108742
41. Luo J, Zhang C, Chen D, Chang T, Chen S, Lin Z, et al. Tim-3 pathway dysregulation and targeting in sepsis-induced immunosuppression. Eur J Med Res. (2024) 29:583. doi: 10.1186/s40001-024-02203-w
42. Silva EE, Skon-Hegg C, Badovinac VP, Griffith TS. The calm after the storm: implications of sepsis immunoparalysis on host immunity. J Immunol (Baltimore Md.: 1950). (2023) 211:711–9. doi: 10.4049/jimmunol.2300171
43. Schlapbach LJ, Watson RS, Sorce LR, Argent AC, Menon K, Hall MW, et al. International consensus criteria for pediatric sepsis and septic shock. JAMA. (2024) 331:665–74. doi: 10.1001/jama.2024.0179
44. Zhang Q, Raoof M, Chen Y, Sumi Y, Sursal T, Junger W, et al. Circulating mitochondrial DAMPs cause inflammatory responses to injury. Nature. (2010) 464:104–7. doi: 10.1038/nature08780
45. Nagaoka I, Tamura H, Reich J. Therapeutic potential of cathelicidin peptide LL-37, an antimicrobial agent, in a murine sepsis model. Int J Mol Sci. (2020) 21:5973. doi: 10.3390/ijms21175973
46. Kwok AJ, Allcock A, Ferreira RC, Cano-Gamez E, Smee M, Burnham KL, et al. Neutrophils and emergency granulopoiesis drive immune suppression and an extreme response endotype during sepsis. Nat Immunol. (2023) 24:767–79. doi: 10.1038/s41590-023-01490-5
47. Dong J, Wang S, Hu Z, Gong L. Extracellular proteins as potential biomarkers in Sepsis-related cerebral injury. Front Immunol. (2023) 14:1128476. doi: 10.3389/fimmu.2023.1128476
48. Li J, Xiao F, Lin B, Huang Z, Wu M, Ma H, et al. Ferrostatin-1 improves acute sepsis-induced cardiomyopathy via inhibiting neutrophil infiltration through impaired chemokine axis. Front Cell Dev Biol. (2024) 12:1510232. doi: 10.3389/fcell.2024.1510232
49. Denning NL, Aziz M, Gurien SD, Wang P. DAMPs and NETs in sepsis. Front Immunol. (2019) 10:2536. doi: 10.3389/fimmu.2019.02536
50. Chen Z, Zhang H, Qu M, Nan K, Cao H, Cata JP, et al. Review: the emerging role of neutrophil extracellular traps in sepsis and sepsis-associated thrombosis. Front Cell Infection Microbiol. (2021) 11:653228. doi: 10.3389/fcimb.2021.653228
51. Huang G, Bao J, Shao X, Zhou W, Wu B, Ni Z, et al. Inhibiting pannexin-1 alleviates sepsis-induced acute kidney injury via decreasing NLRP3 inflammasome activation and cell apoptosis. Life Sci. (2020) 254:117791. doi: 10.1016/j.lfs.2020.117791
52. Wu DD, Li T, Ji XY. Dendritic cells in sepsis: pathological alterations and therapeutic implications. J Immunol Res. (2017) 2017:3591248. doi: 10.1155/2017/3591248
53. Kumar V. Dendritic cells in sepsis: Potential immunoregulatory cells with therapeutic potential. Mol Immunol. (2018) 101:615–26. doi: 10.1016/j.molimm.2018.07.007
54. Zhang W, Fang X, Gao C, Song C, He Y, Zhou T, et al. MDSCs in sepsis-induced immunosuppression and its potential therapeutic targets. Cytokine Growth Factor Rev. (2023) 69:90–103. doi: 10.1016/j.cytogfr.2022.07.007
55. Padovani CM, Yin K. Immunosuppression in sepsis: biomarkers and specialized pro-resolving mediators. Biomedicines. (2024) 12:175. doi: 10.3390/biomedicines12010175
56. Wakeley ME, Gray CC, Monaghan SF, Heffernan DS, Ayala A. Check point inhibitors and their role in immunosuppression in sepsis. Crit Care Clinics. (2020) 36:69–88. doi: 10.1016/j.ccc.2019.08.006
57. Zhu CL, Wang Y, Liu Q, Li HR, Yu CM, Li P, et al. Dysregulation of neutrophil death in sepsis. Front Immunol. (2022) 13:963955. doi: 10.3389/fimmu.2022.963955
58. Zhao W, Wang H, Zhang X, Zhang L, Pu W, Ma Y, et al. Effects of IFN-γ on the immunological microenvironment and TAM polarity in stage IA non-small cell lung cancer and its mechanisms. BMC Pulm Med. (2024) 24:46. doi: 10.1186/s12890-023-02809-6
59. Kwek SS, Kahn J, Greaney SK, Lewis J, Cha E, Zhang L, et al. GM-CSF and ipilimumab therapy in metastatic melanoma: Clinical outcomes and immunologic responses. Oncoimmunology. (2016) 5:e1101204. doi: 10.1080/2162402x.2015.1101204
60. Johnson SE, Shah N, Panoskaltsis-Mortari A, LeBien TW. Murine and human IL-7 activate STAT5 and induce proliferation of normal human pro-B cells. J Immunol (Baltimore Md.: 1950). (2005) 175:7325–31. doi: 10.4049/jimmunol.175.11.7325
61. Lee HW, Lee JI, Um SH, Ahn SH, Chang HY, Park YK, et al. Combination therapy of thymosin alpha-1 and lamivudine for HBeAg positive chronic hepatitis B: A prospective randomized, comparative pilot study. J Gastroenterol Hepatol. (2008) 23:729–35. doi: 10.1111/j.1440-1746.2008.05387.x
62. Yan L, Li J, Zhang C. The role of MSCs and CAR-MSCs in cellular immunotherapy. Cell Comm Signal: CCS. (2023) 21:187. doi: 10.1186/s12964-023-01191-4
63. Sharma P, Goswami S, Raychaudhuri D, Siddiqui BA, Singh P, Nagarajan A, et al. Immune checkpoint therapy-current perspectives and future directions. Cell. (2023) 186:1652–69. doi: 10.1016/j.cell.2023.03.006
64. Deng Z, Fan T, Xiao C, Tian H, Zheng Y, Li C, et al. TGF-β signaling in health, disease, and therapeutics. Signal Transduct Target Ther. (2024) 9:61. doi: 10.1038/s41392-024-01764-w
65. Characiejus D, Pasukoniene V, Jacobs JJ, Eidukevicius R, Jankevicius F, Dobrovolskiene N, et al. Prognostic significance of peripheral blood CD8highCD57+ lymphocytes in bladder carcinoma patients after intravesical IL-2. Anticancer Res. (2011) 31:699–703.
66. Liu JQ, Zhang C, Zhang X, Yan J, Zeng C, Talebian F, et al. Intratumoral delivery of IL-12 and IL-27 mRNA using lipid nanoparticles for cancer immunotherapy. J Controlled Rel. (2022) 345:306–13. doi: 10.1016/j.jconrel.2022.03.021
67. Kurz E, Hirsch CA, Dalton T, Shadaloey SA, Khodadadi-Jamayran A, Miller G, et al. Exercise-induced engagement of the IL-15/IL-15Rα axis promotes anti-tumor immunity in pancreatic cancer. Cancer Cell. (2022) 40:720–737.e5. doi: 10.1016/j.ccell.2022.05.006
68. Kalliolias GD, Ivashkiv LB. TNF biology, pathogenic mechanisms and emerging therapeutic strategies. Nat Rev Rheumatol. (2016) 12:49–62. doi: 10.1038/nrrheum.2015.169
69. Wang Y, Wang J, Zheng W, Zhang J, Wang J, Jin T, et al. Identification of an IL-1 receptor mutation driving autoinflammation directs IL-1-targeted drug design. Immunity. (2023) 56:1485–1501.e7. doi: 10.1016/j.immuni.2023.05.014
70. Moriyama K, Nishida O. Targeting cytokines, pathogen-associated molecular patterns, and damage-associated molecular patterns in sepsis via blood purification. Int J Mol Sci. (2021) 22:8882. doi: 10.3390/ijms22168882
71. Huang L, Li Y, Cheng Z, Lv Z, Luo S, Xia Y. PCSK9 promotes endothelial dysfunction during sepsis via the TLR4/myD88/NF-κB and NLRP3 pathways. Inflammation. (2023) 46:115–28. doi: 10.1007/s10753-022-01715-z
72. Mager CE, Mormol JM, Shelton ED, Murphy PR, Bowman BA, Barley TJ, et al. p38 MAPK and MKP-1 control the glycolytic program via the bifunctional glycolysis regulator PFKFB3 during sepsis. J Biol Chem. (2023) 299:103043. doi: 10.1016/j.jbc.2023.103043
73. Deng C, Zhao L, Yang Z, Shang JJ, Wang CY, Shen MZ, et al. Targeting HMGB1 for the treatment of sepsis and sepsis-induced organ injury. Acta Pharmacol Sin. (2022) 43:520–8. doi: 10.1038/s41401-021-00676-7
74. Wang G, Jin S, Huang W, Li Y, Wang J, Ling X, et al. LPS-induced macrophage HMGB1-loaded extracellular vesicles trigger hepatocyte pyroptosis by activating the NLRP3 inflammasome. Cell Death Discovery. (2021) 7:337. doi: 10.1038/s41420-021-00729-0
75. Yang R, Zhang X. A potential new pathway for heparin treatment of sepsis-induced lung injury: inhibition of pulmonary endothelial cell pyroptosis by blocking hMGB1-LPS-induced caspase-11 activation. Front Cell Infection Microbiol. (2022) 12:984835. doi: 10.3389/fcimb.2022.984835
76. Kumar P, Schroder EA, Rajaram MVS, Harris EN, Ganesan LP. The battle of LPS clearance in host defense vs. Inflammatory signaling. Cells. (2024) 13:1590. doi: 10.3390/cells13181590
77. Chen XS, Wang SH, Liu CY, Gao YL, Meng XL, Wei W, et al. Losartan attenuates sepsis-induced cardiomyopathy by regulating macrophage polarization via TLR4-mediated NF-κB and MAPK signaling. Pharmacol Res. (2022) 185:106473. doi: 10.1016/j.phrs.2022.106473
78. Tsuji N, Tsuji T, Yamashita T, Hayase N, Hu X, Yuen PS, et al. BAM15 treats mouse sepsis and kidney injury, linking mortality, mitochondrial DNA, tubule damage, and neutrophils. J Clin Invest. (2023) 133:e152401. doi: 10.1172/jci152401
79. Kim EY, Ner-Gaon H, Varon J, Cullen AM, Guo J, Choi J, et al. Post-sepsis immunosuppression depends on NKT cell regulation of mTOR/IFN-γ in NK cells. J Clin Invest. (2020) 130:3238–52. doi: 10.1172/jci128075
80. Li B, Niu S, Geng H, Yang C, Zhao C. Berberine attenuates neonatal sepsis in mice by inhibiting FOXA1 and NF-κB signal transduction via the induction of miR-132-3p. Inflammation. (2021) 44:2395–406. doi: 10.1007/s10753-021-01510-2
81. Li X, Xu M, Shen J, Li Y, Lin S, Zhu M, et al. Sorafenib inhibits LPS-induced inflammation by regulating Lyn-MAPK-NF-kB/AP-1 pathway and TLR4 expression. Cell Death Discovery. (2022) 8:281. doi: 10.1038/s41420-022-01073-7
82. Chen Y, Cui M, Cui Y. Vagus nerve stimulation attenuates septic shock-induced cardiac injury in rats. Physiol Res. (2023) 72:731–9. doi: 10.33549/physiolres.935136
83. Hassan NF, El-Ansary MR, Selim H, Ousman MS, Khattab MS, El-Ansary MRM, et al. Alirocumab boosts antioxidant status and halts inflammation in rat model of sepsis-induced nephrotoxicity via modulation of Nrf2/HO-1, PCSK9/HMGB1/NF-κB/NLRP3 and Fractalkine/CX3CR1 hubs. Biomed Pharmacother = Biomed Pharmacother. (2024) 177:116929. doi: 10.1016/j.biopha.2024.116929
84. Yan J, Zhang J, Wang Y, Liu H, Sun X, Li A, et al. Rapidly inhibiting the inflammatory cytokine storms and restoring cellular homeostasis to alleviate sepsis by blocking pyroptosis and mitochondrial apoptosis pathways. Adv Sci (Weinheim Baden-Wurttemberg Germany). (2023) 10:e2207448. doi: 10.1002/advs.202207448
85. Pei H, Chen J, Qu J, Lu Z. S100A9 exacerbates sepsis-induced acute lung injury via the IL17-NFκB-caspase-3 signaling pathway. Biochem Biophys Res Commun. (2024) 710:149832. doi: 10.1016/j.bbrc.2024.149832
86. Huang L, Zhang L, Liu Z, Zhao S, Xu D, Li L, et al. Pentamidine protects mice from cecal ligation and puncture-induced brain damage via inhibiting S100B/RAGE/NF-κB. Biochem Biophys Res Commun. (2019) 517:221–6. doi: 10.1016/j.bbrc.2019.07.045
87. Zeng Z, Li D, Liu F, Zhou C, Shao Q, Ding C, et al. Mitochondrial DNA plays an important role in lung injury induced by sepsis. J Cell Biochem. (2019) 120:8547–60. doi: 10.1002/jcb.28142
88. Liu TF, Vachharajani V, Millet P, Bharadwaj MS, Molina AJ, McCall CE. Sequential actions of SIRT1-RELB-SIRT3 coordinate nuclear-mitochondrial communication during immunometabolic adaptation to acute inflammation and sepsis. J Biol Chem. (2015) 290:396–408. doi: 10.1074/jbc.M114.566349
89. Wu SC, Rau CS, Wu YC, Wu CJ, Tsai CW, Huang LH, et al. Circulating exosomes from septic mice activate NF-κB/MIR17HG pathway in macrophages. Biomedicines. (2024) 12:534. doi: 10.3390/biomedicines12030534
90. Xue R, Yiu WH, Chan KW, Lok SWY, Zou Y, Ma J, et al. Long non-coding RNA NEAT1, NOD-like receptor family protein 3 inflammasome, and acute kidney injury. J Am Soc Nephrol: JASN. (2024) 35:998–1015. doi: 10.1681/asn.0000000000000362
91. Jiang X, Wang Y, Qin Y, He W, Benlahrech A, Zhang Q, et al. Micheliolide provides protection of mice against Staphylococcus aureus and MRSA infection by down-regulating inflammatory response. Sci Rep. (2017) 7:41964. doi: 10.1038/srep41964
92. Sun Z, Pan Y, Qu J, Xu Y, Dou H, Hou Y. 17β-estradiol promotes trained immunity in females against sepsis via regulating nucleus translocation of relB. Front Immunol. (2020) 11:1591. doi: 10.3389/fimmu.2020.01591
93. Harriett AJ, Esher Righi S, Lilly EA, Fidel P Jr., Noverr MC. Efficacy of candida dubliniensis and fungal β-glucans in inducing trained innate immune protection against inducers of sepsis. Front Cell Infection Microbiol. (2022) 12:898030. doi: 10.3389/fcimb.2022.898030
94. Jarczak D, Nierhaus A. cytokine storm-definition, causes, and implications. Int J Mol Sci. (2022) 23:11740. doi: 10.3390/ijms231911740
95. Li Y, Zhang H, Chen C, Qiao K, Li Z, Han J, et al. Biomimetic immunosuppressive exosomes that inhibit cytokine storms contribute to the alleviation of sepsis. Adv Matls (Deerfield Beach Fla.). (2022) 34:e2108476. doi: 10.1002/adma.202108476
96. Pei F, Yao RQ, Ren C, Bahrami S, Billiar TR, Chaudry IH, et al. Expert consensus on the monitoring and treatment of sepsis-induced immunosuppression. Mil Med Res. (2022) 9:74. doi: 10.1186/s40779-022-00430-y
97. Gupta DL, Bhoi S, Mohan T, Galwnkar S, Rao DN. Coexistence of Th1/Th2 and Th17/Treg imbalances in patients with post traumatic sepsis. Cytokine. (2016) 88:214–21. doi: 10.1016/j.cyto.2016.09.010
98. Senousy SR, El-Daly M, Ibrahim ARN, Khalifa MMA, Ahmed AF. Effect of celecoxib and infliximab against multiple organ damage induced by sepsis in rats: A comparative study. Biomedicines. (2022) 10:1613. doi: 10.3390/biomedicines10071613
99. Ji HH, Tang XW, Dong Z, Song L, Jia YT. Adverse event profiles of anti-CTLA-4 and anti-PD-1 monoclonal antibodies alone or in combination: analysis of spontaneous reports submitted to FAERS. Clin Drug Invest. (2019) 39:319–30. doi: 10.1007/s40261-018-0735-0
100. He Z, Ma Y, Yang S, Zhang S, Liu S, Xiao J, et al. Gut microbiota-derived ursodeoxycholic acid from neonatal dairy calves improves intestinal homeostasis and colitis to attenuate extended-spectrum β-lactamase-producing enteroaggregative Escherichia coli infection. Microbiome. (2022) 10:79. doi: 10.1186/s40168-022-01269-0
101. Wang Y, Deng H, Xiao L, Pan Y. Escherichia coli Nissle 1917 Protects against Sepsis-Induced Intestinal Damage by Regulating the SCFA/GPRs Signaling Pathway. Microorganisms. (2024) 12:1622. doi: 10.3390/microorganisms12081622
102. Sun Y, Oami T, Liang Z, Miniet AA, Burd EM, Ford ML, et al. Membrane permeant inhibitor of myosin light chain kinase worsens survival in murine polymicrobial sepsis. Shock (Augusta Ga.). (2021) 56:621–8. doi: 10.1097/shk.0000000000001759
103. Schluter J, Djukovic A, Taylor BP, Yan J, Duan C, Hussey GA, et al. The TaxUMAP atlas: Efficient display of large clinical microbiome data reveals ecological competition in protection against bacteremia. Cell Host Microbe. (2023) 31:1126–1139.e6. doi: 10.1016/j.chom.2023.05.027
104. Lee CC, Feng Y, Yeh YM, Lien R, Chen CL, Zhou YL, et al. Gut dysbiosis, bacterial colonization and translocation, and neonatal sepsis in very-low-birth-weight preterm infants. Front Microbiol. (2021) 12:746111. doi: 10.3389/fmicb.2021.746111
105. Lou X, Xue J, Shao R, Yang Y, Ning D, Mo C, et al. Fecal microbiota transplantation and short-chain fatty acids reduce sepsis mortality by remodeling antibiotic-induced gut microbiota disturbances. Front Immunol. (2022) 13:1063543. doi: 10.3389/fimmu.2022.1063543
106. Drancourt M, Djemai K, Gouriet F, Grine G, Loukil A, Bedotto M, et al. Methanobrevibacter smithii archaemia in febrile patients with bacteremia, including those with endocarditis. Clin Infect Dis. (2021) 73:e2571–9. doi: 10.1093/cid/ciaa998
107. Hagiya H, Kimura K, Nishi I, Yamamoto N, Yoshida H, Akeda Y, et al. Desulfovibrio desulfuricans bacteremia: A case report and literature review. Anaerobe. (2018) 49:112–5. doi: 10.1016/j.anaerobe.2017.12.013
108. Marques CG, Dos Santos Quaresma MVL, França Ferracini CB, Alves Carrilho FB, Nakamoto FP, Lucin GA, et al. Effect of caloric restriction with probiotic supplementation on body composition, quality of life, and psychobiological factors of obese men: A randomized, double-blinded placebo-controlled clinical trial. Clin Nutr (Edinburgh Scotland). (2025) 45:234–49. doi: 10.1016/j.clnu.2024.12.031
109. Demers M, Dagnault A, Desjardins J. A randomized double-blind controlled trial: impact of probiotics on diarrhea in patients treated with pelvic radiation. Clin Nutr (Edinburgh Scotland). (2014) 33:761–7. doi: 10.1016/j.clnu.2013.10.015
110. Shan LS, Hou P, Wang ZJ, Liu FR, Chen N, Shu LH, et al. Prevention and treatment of diarrhoea with Saccharomyces boulardii in children with acute lower respiratory tract infections. Beneficial Microbes. (2013) 4:329–34. doi: 10.3920/bm2013.0008
111. Bleichner G, Bléhaut H, Mentec H, Moyse D. Saccharomyces boulardii prevents diarrhea in critically ill tube-fed patients. A multicenter, randomized, double-blind placebo-controlled trial. Intensive Care Med. (1997) 23:517–23. doi: 10.1007/s001340050367
112. Roberts JD, Suckling CA, Peedle GY, Murphy JA, Dawkins TG, Roberts MG. An exploratory investigation of endotoxin levels in novice long distance triathletes, and the effects of a multi-strain probiotic/prebiotic, antioxidant intervention. Nutrients. (2016) 8:733. doi: 10.3390/nu8110733
113. Manhart N, Spittler A, Bergmeister H, Mittlböck M, Roth E. Influence of fructooligosaccharides on Peyer’s patch lymphocyte numbers in healthy and endotoxemic mice. Nutr (Burbank Los Angeles County Calif.). (2003) 19:657–60. doi: 10.1016/s0899-9007(03)00059-5
114. Krumbeck JA, Rasmussen HE, Hutkins RW, Clarke J, Shawron K, Keshavarzian A, et al. Probiotic Bifidobacterium strains and galactooligosaccharides improve intestinal barrier function in obese adults but show no synergism when used together as synbiotics. Microbiome. (2018) 6:121. doi: 10.1186/s40168-018-0494-4
115. Kullar R, Goldstein EJC, Johnson S, McFarland LV. Lactobacillus bacteremia and probiotics: A review. Microorganisms. (2023) 11:896. doi: 10.3390/microorganisms11040896
116. Gözmen S, Sükran Gözmen K, Apa H, Aktürk H, Sorguç Y, Bayram N, et al. Secondary bacteremia in rotavirus gastroenteritis. Pediatr Infect Dis J. (2014) 33:775–7. doi: 10.1097/inf.0000000000000324
117. Cao L, Li Z, Ren Y, Wang M, Yang Z, Zhang W, et al. Xuebijing protects against septic acute liver injury based on regulation of GSK-3β Pathway. Front Pharmacol. (2021) 12:627716. doi: 10.3389/fphar.2021.627716
118. Pan G, Wu Y, Liu Y, Zhou F, Li S, Yang S. Dachengqi decoction ameliorates sepsis-induced liver injury by inhibiting the TGF-β1/Smad3 pathways. J Trad Comp Med. (2024) 14:256–65. doi: 10.1016/j.jtcme.2023.09.001
119. Li X, Wei S, Niu S, Ma X, Li H, Jing M, et al. Network pharmacology prediction and molecular docking-based strategy to explore the potential mechanism of Huanglian Jiedu Decoction against sepsis. Comput Biol Med. (2022) 144:105389. doi: 10.1016/j.compbiomed.2022.105389
120. Choi YY, Kim MH, Cho IH, Kim JH, Hong J, Lee TH, et al. Inhibitory effect of Coptis chinensis on inflammation in LPS-induced endotoxemia. J Ethnopharmacology. (2013) 149:506–12. doi: 10.1016/j.jep.2013.07.008
121. Saxena J, Agarwal G, Das S, Kumar A, Thakkar K, Kaushik S, et al. Immunopharmacological Insights into Cordyceps spp.: Harnessing Therapeutic Potential for Sepsis. Curr Pharm design. (2024) 31(11):823–42. doi: 10.2174/0113816128326301240920040036
122. Liu J, Wu YH, Zhang ZL, Li P. Tanshinone IIA improves sepsis-induced acute lung injury through the ROCK2/NF-κB axis. Toxicol Appl Pharmacol. (2022) 446:116021. doi: 10.1016/j.taap.2022.116021
123. Kurokawa M, Brown J, Kagawa Y, Shiraki K. Cytokine-regulatory activity and therapeutic efficacy of cinnamyl derivatives in endotoxin shock. Eur J Pharmacol. (2003) 474:283–93. doi: 10.1016/s0014-2999(03)02036-3
124. Wang WB, Li JT, Hui Y, Shi J, Wang XY, Yan SG. Combination of pseudoephedrine and emodin ameliorates LPS-induced acute lung injury by regulating macrophage M1/M2 polarization through the VIP/cAMP/PKA pathway. Chin Med. (2022) 17:19. doi: 10.1186/s13020-021-00562-8
125. Zhao H, Liu Z, Shen H, Jin S, Zhang S. Glycyrrhizic acid pretreatment prevents sepsis-induced acute kidney injury via suppressing inflammation, apoptosis and oxidative stress. Eur J Pharmacol. (2016) 781:92–9. doi: 10.1016/j.ejphar.2016.04.006
126. Kwon HS, Park JH, Kim DH, Kim YH, Park JH, Shin HK, et al. Licochalcone A isolated from licorice suppresses lipopolysaccharide-stimulated inflammatory reactions in RAW264.7 cells and endotoxin shock in mice. J Mol Med (Berlin Germany). (2008) 86:1287–95. doi: 10.1007/s00109-008-0395-2
127. Wang Y, Yu W, Shi C, Hu P. Crocetin attenuates sepsis-induced cardiac dysfunction via regulation of inflammatory response and mitochondrial function. Front Physiol. (2020) 11:514. doi: 10.3389/fphys.2020.00514
128. Xing W, Yang L, Peng Y, Wang Q, Gao M, Yang M, et al. Ginsenoside Rg3 attenuates sepsis-induced injury and mitochondrial dysfunction in liver via AMPK-mediated autophagy flux. Bioscience Rep. (2017) 37:BSR20170934. doi: 10.1042/bsr20170934
129. Lv T, Zhang C, Hu L, Wang C, Li S, Wang H, et al. Berberine in sepsis: effects, mechanisms, and therapeutic strategies. J Immunol Res. (2023) 2023:4452414. doi: 10.1155/2023/4452414
130. Vajdi M, Sefidmooye Azar P, Mahmoodpoor A, Dashti F, Sanaie S, Kiani Chalmardi F, et al. A comprehensive insight into the molecular and cellular mechanisms of action of resveratrol on complications of sepsis a systematic review. Phytother Res: PTR. (2023) 37:3780–808. doi: 10.1002/ptr.7917
131. Liu P, Xiao Z, Yan H, Lu X, Zhang X, Luo L, et al. Baicalin suppresses Th1 and Th17 responses and promotes Treg response to ameliorate sepsis-associated pancreatic injury via the RhoA-ROCK pathway. Int Immunopharmacol. (2020) 86:106685. doi: 10.1016/j.intimp.2020.106685
132. Zhang Y, Zeng Y, Huang M, Cao G, Lin L, Wang X, et al. Andrographolide attenuates sepsis-induced acute kidney injury by inhibiting ferroptosis through the Nrf2/FSP1 pathway. Free Radical Res. (2024) 58:156–69. doi: 10.1080/10715762.2024.2330413
133. He J, Qin W, Jiang S, Lin Y, Lin Y, Yang R, et al. Oxymatrine attenuates sepsis-induced inflammation and organ injury via inhibition of HMGB1/RAGE/NF-κB signaling pathway. Drug Dev Res. (2024) 85:e22219. doi: 10.1002/ddr.22219
Keywords: cytokine storm, immune balance, probiotics, treatment outcome, traditional Chinese medicine (TCM)
Citation: Xu Y, Wang J, Yuan R, Qin Z, Long K and Gao P (2025) Targeting the immuno-inflammatory-microbial network: a key strategy for sepsis treatment. Front. Immunol. 16:1575516. doi: 10.3389/fimmu.2025.1575516
Received: 12 February 2025; Accepted: 27 March 2025;
Published: 14 April 2025.
Edited by:
William D. Shipman, Skin & Beauty Center - Board Certified Dermatologist, United StatesReviewed by:
Georgia Damoraki, National and Kapodistrian University of Athens, GreeceSunil Joshi, QuantumImmunogenix-A Third Eye Network Inc., United States
Puneet Gandhi, Bhopal Memorial Hospital & Research Centre, India
Copyright © 2025 Xu, Wang, Yuan, Qin, Long and Gao. This is an open-access article distributed under the terms of the Creative Commons Attribution License (CC BY). The use, distribution or reproduction in other forums is permitted, provided the original author(s) and the copyright owner(s) are credited and that the original publication in this journal is cited, in accordance with accepted academic practice. No use, distribution or reproduction is permitted which does not comply with these terms.
*Correspondence: Yue Xu, eHV5dWVAc3R1LmNkdXRjbS5lZHUuY24=; Peiyang Gao, Z2FvcHk5MzBAMTI2LmNvbQ==