- 1School of Biotechnology and Biomolecular Sciences, University of New South Wales, Sydney, NSW, Australia
- 2Department of Molecular Biology and Genetics, Istanbul University, Istanbul, Turkey
- 3Department of Biotechnology, Mannheim University of Applied Sciences, Mannheim, Germany
Organohalides are recalcitrant pollutants that have been responsible for substantial contamination of soils and groundwater. Organohalide-respiring bacteria (ORB) provide a potential solution to remediate contaminated sites, through their ability to use organohalides as terminal electron acceptors to yield energy for growth (i.e., organohalide respiration). Ideally, this process results in non- or lesser-halogenated compounds that are mostly less toxic to the environment or more easily degraded. At the heart of these processes are reductive dehalogenases (RDases), which are membrane bound enzymes coupled with other components that facilitate dehalogenation of organohalides to generate cellular energy. This review focuses on RDases, concentrating on those which have been purified (partially or wholly) and functionally characterized. Further, the paper reviews the major bacteria involved in organohalide breakdown and the evidence for microbial evolution of RDases. Finally, the capacity for using ORB in a bioremediation and bioaugmentation capacity are discussed.
Chlorinated Substances as Environmental Pollutants
Organohalides are recalcitrant environmental pollutants contaminating soil and groundwater. Across the atmosphere, pedosphere and the oceans there are over 2000 recognized chlorinated organic natural products with over 1500 produced biologically and the remainder formed through abiotic processes (Gribble, 2003, 2010). Organohalide contamination of land and water which is of anthropogenic origin (e.g., arising from the manufacture and use of pesticides, dry cleaning solvents, ozone-depleting refrigerants, industrial degreasers) is widespread and poses significant potential danger related to their adverse impacts on health and effects on ecosystems. Among the commonly reported anthropogenic organohalides are hexachlorobenzene (HCB), trichloromethane (TCM), polychlorinated biphenyls (PCBs), perchloroethene (PCE), trichloroethene (TCE), trichloroethanes (TCA), dichloroethanes (DCA), polybrominated diphenyl ethers (PBDEs), chlorinated/brominated phenols, and dioxins. Pollution of terrestrial and aquatic systems caused by the excessive release of the anthropogenic organohalides, has led researchers to search for strategies to remediate contaminated sites using microbiota which includes a diverse range of mostly anaerobic bacteria.
Polychlorinated ethenes and ethanes are major members of organohalide pollutants and their degradation can occur via anaerobic biotic and abiotic pathways (Figure 1). Chlorinated ethenes, mainly PCE and TCE, used as a dry-cleaning solvent and an industrial degreaser respectively, are among the most abundant pollutants. The products of dechlorination of PCE and TCE are cis-dichloroethene (cis-DCE), vinyl chloride (VC), and ethene, and many ORB have been found to catalyze their dechlorination partially or completely (Middeldorp et al., 1999). However, only a limited number of RDases that can dechlorinate PCE (PceA), TCE (TceA), and VC (VcrA) have been biochemically characterized (Table 1). Another group of organohalide pollutants are chlorinated ethanes, such as tetrachloroethane (TeCA), trichloroethane (TCA), and dichloroethane (DCA). In particular, 1,1,1-TCA (methyl chloroform), used as a solvent and in many consumer products, is one of the most predominant environmental pollutants and has the potential to cause serious health issues (Padilla-Crespo et al., 2014). 1,1-DCA, 1,2-DCA, and VC are also common groundwater contaminants and are classified by the US Environmental Protection Agency as a possible human carcinogen (Group C), a probable human carcinogen (Group B2) and human carcinogen (Group A), respectively (accessed Dec 2015; http://www.epa.gov/). Chlorinated methane pollutants, for example, trichloromethane (TCM), are highly hydrophobic and volatile. They are manufactured as industrial solvents for organic materials and also as intermediates for the production of polytetrafluoroethylene and the refrigerant monochlorodifluoromethane (HCFC-22; Justicia-Leon et al., 2014). Due to the poor solubility of TCM in water (<8 g/L, 20°C), high density (ρ = 1.48 g/cm3), and long half-life (3500 years), this highly recalcitrant organohalide sinks and stays as solvent pools within subsurface water systems, prompting urgent, and efficient remediation measures (Mabey and Mill, 1978; Lee et al., 2015). Fortunately, some ORB have been identified that can utilize these organohalides as terminal electron acceptors, and some RDases responsible for their reductive dehalogenation have been reported.
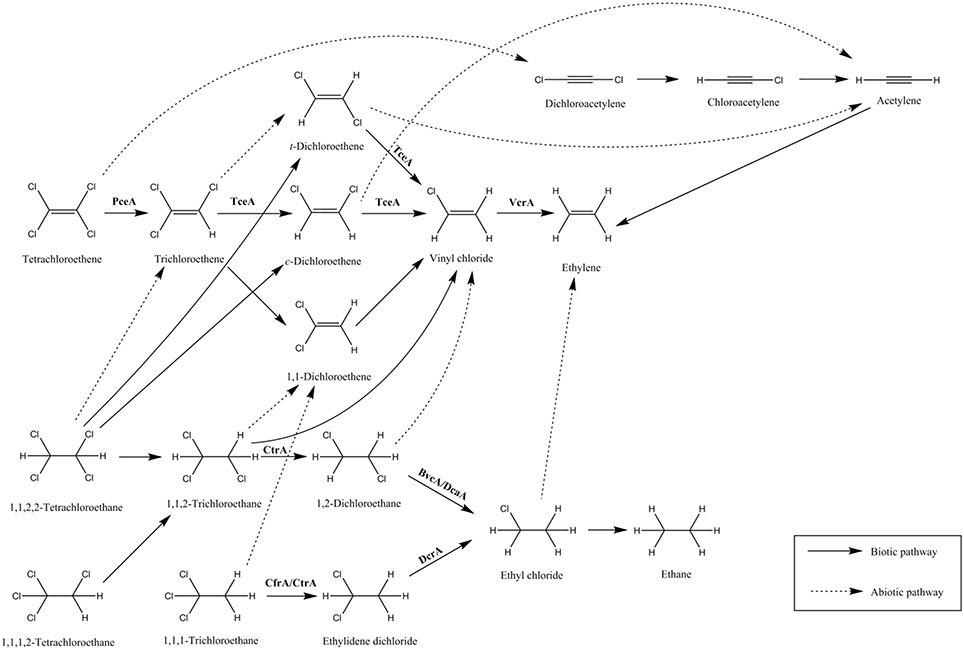
Figure 1. Dechlorination of chlorinated ethenes and ethanes via anaerobic biotic and abiotic pathways. Examples of enzymes catalyzing the biotic reactions are given: PceA (Miller et al., 1998), TceA (Magnuson et al., 2000; Fung et al., 2007), VcrA (Parthasarathy et al., 2015), CtrA (Zhao et al., 2015), BvcA (Tang et al., 2013), DcaA (Marzorati et al., 2007), CfrA (Tang and Edwards, 2013), CtrA (Ding et al., 2014), and DcrA (Tang and Edwards, 2013).
Reductive Dehalogenases in Organohalide Respiration
The discovery made three decades ago that certain anaerobic bacteria could derive their energy by reducing organohalides, has motivated an intensive research effort in this field (Shelton and Tiedje, 1984). These ORB are equipped with concerted membrane associated proteins that drive the organohalide respiration process. The ultimate goal of this respiratory process is to synthesize ATP driven by a proton motive force (PMF) established across the cytoplasmic membrane (CM). A chemiosmotic coupling between reductive dehalogenation and ATP synthesis has been proposed, as uncouplers significantly reduce the ATP pool relative to the reductive dechlorination rate (Louie and Mohn, 1999).
The reductive dehalogenation reaction is highly exergonic, as demonstrated for most organohalides with the free energy (ΔG°′) of dechlorination with hydrogen as the electron donor in a range of between −131 and −192 kJ/mol. Furthermore, the organohalides are thermodynamically favorable as electron acceptors under anaerobic conditions, as their standard redox potential (E°′) lies between approximately +250 and +600 mV (El Fantroussi et al., 1998; Holliger et al., 1998). Although such an energy yield could potentially result in 2.5–2.7 ATP molecules per molecule of chloride ion released, considering 70 kJ is required to make one mole of ATP in a living cell (Schink and Friedrich, 1994), biomass yields per mole of chloride released for ORB are generally low. This possibly stems from the fact that only two mole H+ per mole H2 oxidized are released and three moles of H+ are required to generate sufficient proton-motive force to produce one mole of ATP (Schumacher and Holliger, 1996).
Reductive dehalogenases are central to this process. The catalytic subunit, reductive dehalogenase homologous A subunit (RdhA) of the enzyme harbors a cobalamin (vitamin B12) cofactor and two Fe-S clusters (Figure 2). It had been debated whether RdhA “faces” the cytoplasm or periplasm, and whether it is monomeric or dimeric. A recent structural study demonstrated attachment to the periplasmic side of the CM in a dimeric form (Bommer et al., 2014). In Figure 2, a putative electron transfer chain is depicted based on the biochemical pathway found in Sulfurospirillum multivorans (Bommer et al., 2014; Goris et al., 2014). RdhA is anchored to the CM via its membrane anchor protein (RdhB). Reductive dehalogenation occurs at the catalytic site where reduced cobalamin cofactor (CoI, II) attacks the halogen atom of an organohalide to cleave a carbon-halogen bond, leading to sequential removal of the halogen substituents from the organic backbone. The cleavage of the carbon-halogen bond was proposed to occur either heterolytically or homolytically (Jugder et al., 2015b). The electrons required for the reduction of a halogen ion are transferred to the Co ions through distal and proximal Fe-S clusters.
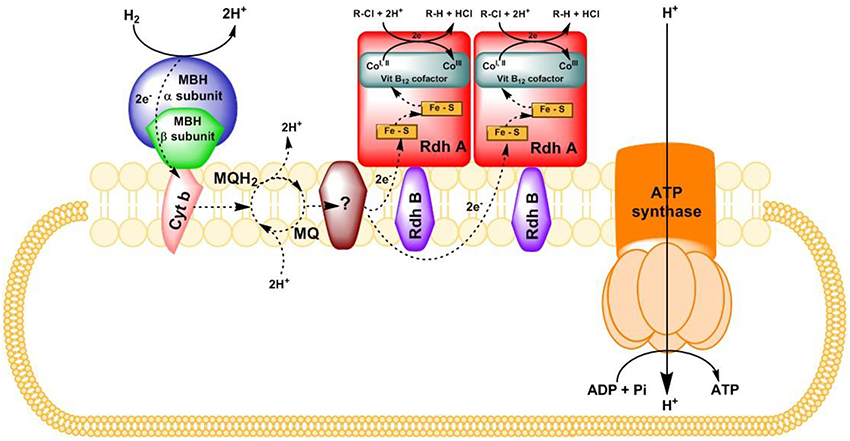
Figure 2. Putative representation of electron transfer chain with H2 as electron donor, and organohalide as electron acceptor in S. multivorans (derived from Bommer et al., 2014 and Goris et al., 2014). Rdh A, reductive dehalogenase catalytic subunit; Rdh B, reductive dehalogenase membrane anchor protein; MBH, membrane-bound uptake hydrogenase; Cyt b, cytochrome b subunit of the MBH; MQ, menaquinone; MQH2, dihydromenaquinone; R-Cl, organohalide.
The coupling between oxidation of the electron donor (in most cases, hydrogen or formate) and reductive dechlorination of electron acceptors (organohalides) is believed to drive electron transport phosphorylation, where membrane associated oxidoreductases are involved. Membrane-bound hydrogenases (MBH) are the initial oxidizers to take up the electrons released from molecular hydrogen (Jugder et al., 2013, 2015a). Both uptake (Hyd or Hup type) and energy-conserving hydrogenases (Hyc or Hym type) have been proposed to play a role in organohalide respiration (Morris et al., 2006; Rupakula et al., 2013; Kruse et al., 2015). Elevated expression of periplasmic formate dehydrogenase at transcriptional and translational levels strongly suggested its role in organohalide respiration where formate is used as an electron donor (Kruse et al., 2015). Surprisingly, the same pattern was also observed in Dehalococcoides cells where formate cannot be used as the electron donor. In this organism, serine substitution at the critical site was revealed in the formate dehydrogenases found via in-depth phylogenetic analysis (Morris et al., 2006). However, the potential role of the periplasmic formate dehydrogenase in organohalide respiration is unresolved, requiring further biochemical investigations.
For subsequent transfer across the membrane, the electrons are taken up by electron carrier(s), such as menaquinone that could possibly function as proton translocating coenzymes to release the protons on the cytoplasmic side of the membrane during the redox reaction in Dehalobacter (Schumacher and Holliger, 1996) and Desulfitobacterium (Kruse et al., 2015) species. However, the involvement of menaquinone in electron transfer in S. multivorans was questioned due to its high redox potential (−74 mV) resulting in its unlikely role in direct electron delivery to RDases (Miller et al., 1996). Thus, the involvement of additional unknown electron carriers to subsequently generate the PMF has to be envisaged. Recent studies have proposed a putative membrane bound quinol dehydrogenase as a potential candidate linking menaquinone and PceA in S. multivorans (Goris et al., 2014) or CprA in D. dehalogenans (Kruse et al., 2015). Moreover, an extracellular flavoprotein was postulated to act as an electron shuttle between the quinol dehydrogenase and the CprA (Kruse et al., 2015). Despite these findings, there is no real consensus on the exact mechanism or mechanisms of the cellular respiratory pathway or on the involvement of various membrane associated components.
Microorganisms Producing Reductive Dehalogenases
Most ORB are strict anaerobes, characterized by slow growth, light and pH sensitivity, and dependency on external supply of corrinoid cofactors. ORB are known to thrive within mutualistic anaerobic microbial communities, rather than in pure culture (Maphosa et al., 2012). Since the isolation of the first ORB, Desulfmonile tiedjei (DeWeerd et al., 1990), multiple genomes and metagenomes of ORB have been reported. The average genome size ranges from 2.6 to 3.1 Mb with an average GC content of about 44–45% (Richardson, 2013). Beyond detoxification, ORB are also an integral part of the global biogeochemical chlorine cycle between the oceans and the atmosphere (Krzmarzick et al., 2012).
RDases from Dehalococcoides, Dehalobacter, and Desulfitobacterium strains are amongst the most extensively studied of these enzymes. Due to their degree of dependency on organohalide respiration, ORB are classified as either obligate organohalide respirers that are highly specialized with very restricted metabolism or non-obligate organohalide respirers that are characterized by their capacity to use a broader range of electron acceptors and donors (Maphosa et al., 2010).
The growth profiles of Dehalobacter and Dehalococcoides, the main niche specialists from Firmicutes and Chloroflexi, respectively, are strictly dependent on organohalide respiration with mostly polychlorinated benzenes or chlorinated aliphatic hydrocarbons as electron acceptors and H2 as the sole electron donor. Although pure cultures of these obligate species can be onerous to cultivate due to their slow growth rate and fastidious nutritional requirements, the cultures used in bioremediation for bioaugmentation are typically mixed enrichment cultures for which nutritional requirements are satisfied with a mineral medium containing typically a fermentable substrate and the chlorinated electron acceptor. There have been several recent studies with enrichment cultures relevant for bioremediation showing progress on growth methods to yield high concentrations of these microbes and doubling times for D. mccartyi of 17 h (Vainberg et al., 2009; Delgado et al., 2014). Recently discovered Dehalobacter and Desulfitobacterium strains have been revealed to use TCM for their reductive respiratory process, and some of their respective RDases have also been reported (Tang et al., 2012; Tang and Edwards, 2013; Ding et al., 2014). In our lab, we have identified a mixed community containing Dehalobacter that completely transformed TCM to acetate and hydrogen (Lee et al., 2012), and subsequently a novel TCM-respiring Dehalobacter sp. strain UNSWDHB was isolated from the mixed community and genome sequenced (Deshpande et al., 2013). In contrast, Desulfitobacterium species are metabolic generalists within the Firmicutes. They are relatively easy to culture with regard to their capacity to utilize a range of electron acceptors, allowing extensive studies on these organisms and their respective enzymes.
Production, Purification, and Characterization of RDases
Between the late 1990s and the early 2000s, several efforts to obtain pure native RDases were reported (Table 1). Since RDases are membrane-associated, the use of ultracentrifugation for membrane fractionation followed by different solubilization techniques was commonly used as initial purification steps. Commonly this was followed by chromatographic separation utilizing ion exchange, hydrophobic interaction, and size exclusion matrices. For the most part, this resulted in partially purified proteins with varied specific activity. The PceA from S. multivorans (Neumann et al., 1996) represents the highest specific activity reported (158 μmol/min/mg), however, the range of activities reported are broad, with activities <1 μmol/min/mg described. Indeed, due to the difficulties with producing and purifying functional native enzymes, efforts to express these enzymes heterologously have arisen, with recent successes in generating functional enzymes (Jugder et al., 2015b) described (summarized in Table 2). Early efforts to express recombinant PceAs in E. coli failed to obtain functionally active enzymes (Neumann et al., 1998; Suyama et al., 2002; Kimoto et al., 2010; Sjuts et al., 2012), however, recently three research teams successfully developed expression and purification strategies to obtain catalytically active RDases in different heterologous hosts (Table 2). Specific activities reported for these enzymes are at the lower end of the range reported for native enzymes (2–10 μmol/min/mg). The demonstrated success with purifying a number of the native enzymes suggests that this approach cannot be ignored for generating enzymes for biochemical and structural characterization, however, early promise shown in recent heterologous expression of RDases will encourage further developments in recombinant expression strategies.
Determination of Reductive Dehalogenase Substrate Specificity
Given that ORB harbor multiple reductive dehalogenase genes, the prediction of substrate specificity based on sequence information is difficult (Hug et al., 2013). Buttet et al discussed sequence-substrate relationships within their work and concluded that sequence similarity and substrate specificity are generally not correlated (Buttet et al., 2013). Although RDases contain highly conserved domains, the authors emphasized that even minimal variations in the sequence are responsible for different substrate ranges. Functional assays require the isolation and purification of the native biocatalyst and thereby remain a bottleneck to assess substrate specificity in many cases (Richardson, 2013).
Given these issues, we performed a maximum likelihood-based phylogenetic analysis based on the amino acid sequence of the only RDases characterized to date. This analysis reveals two main clusters with each having different clades arranged according to their substrate specificity (Figure 3). Most of these RDases are grouped within the first cluster (cluster I), with their functional similarity against certain substrates, such as chlorinated ethenes, chlorinated propanes, chlorinated benzenes, chlorinated ethanes and ethenes, chlorinated methanes and ethanes, and meta/para-chlorophenols. The RDases studied in all Dehalococcoides members are also included in this cluster and they form a separate clade, with the exception of CbrA from D. mccartyi CBDB1. All trichloromethane and trichloroethane-reductases (TmrA, CtrA, and CfrA), sharing high sequence homology, are also grouped into the same clade. The second main group (cluster II) contains aliphatic organohalide-reducing enzymes, such as ortho-bromophenol and ortho-chlorophenol reductases. Two main clades included here represent a distinct class of RDases typically identified in aerobic microbes (BhbA and NpRdhA) and all CprAs found in Desulfitobacterium species, both of which catalyze ortho-halogenated phenols. There are however, RDases with similar function, such as PceA from S. multivorans, PceA from S. sediminis and all other PceAs that are located in different branches. Another interesting observation is that RDases with different functionality, such as DcpA from Dehalogenimonas lykanthroporepellens BL-DC-9 and CbrA from D. mccartyi CBDB1, group into the same clade, it can be speculated that these enzymes share a similar substrate specificity that is yet unknown. We contend that this phylogenetic clustering based on RDase amino acid sequences is potentially a useful tool for predicting functional specificity of these enzymes and this information could also be exploited for further specific genetic tools to study homologous proteins as putative RDases.
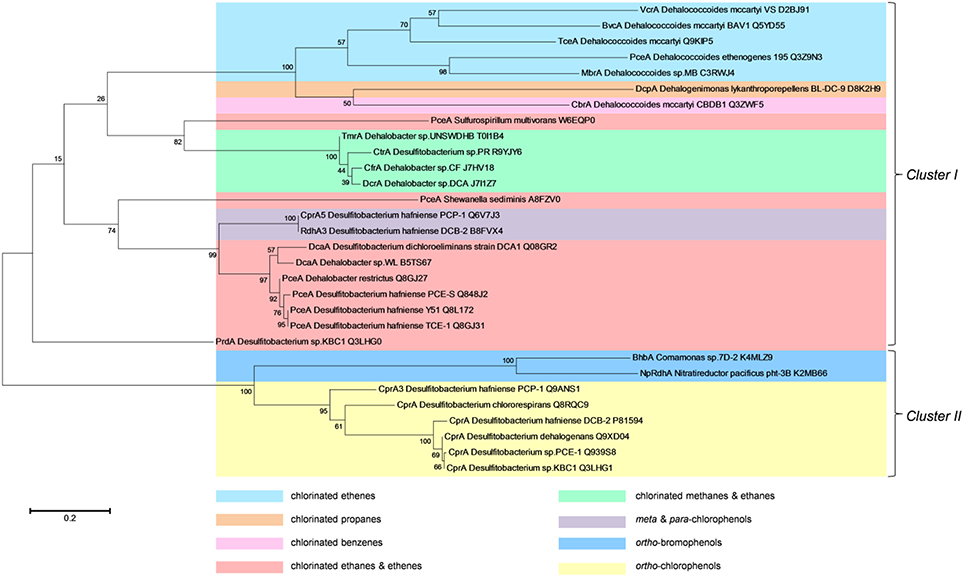
Figure 3. Maximum Likelihood phylogenetic analysis of reductive dehalogenases characterized to date. The evolutionary history was inferred by using the Maximum Likelihood method based on the JTT matrix-based model (Jones et al., 1992). The tree with the highest log likelihood (−7407.3363) is shown. The percentage of trees in which the associated taxa clustered together is shown next to the branches. Initial tree(s) for the heuristic search were obtained by applying the Neighbor-Joining method to a matrix of pairwise distances estimated using a JTT model. The tree is drawn to scale, with branch lengths measured in the number of substitutions per site. The analysis involved 30 amino acid sequences. All positions containing gaps and missing data were eliminated. There were a total of 201 positions in the final dataset. Evolutionary analyses were conducted in MEGA6 (Tamura et al., 2013).
A broad range of substrate specificity has been reported among ORB. Different substrates for the TceA reductive dehalogenase from Dehalospirillum multivorans were evaluated by offering different electron donors and acceptors to the native enzyme and the reductive dechlorination of chlorinated propenes in addition to chlorinated ethenes were reported (Neumann et al., 2002). The PceA of Desulfitobacterium sp. Y51 was found to dechlorinate not only PCE and TCE but also various chloroethanes, especially those with a high number of chloride substituents (Suyama et al., 2002). TceA from Dehalococcoides ethenogenes also catalyzes the dehalogenation of various three to five carbon atom containing haloalkanes and haloalkenes besides its more favored substrate TCE, albeit at lower rates (Magnuson et al., 2000).
Evolutionary Aspects of ORB and their RDases
Horizontal gene transfer (HGT) is a characteristic evolutionary mechanism that is widely reported among various genera of ORB (Liang et al., 2012). This is supported by the discovery of various mobile genetic elements, including insertion sequences (IS), transposases (TnpA), genomic islands (GIs), prophages, and recombinases, which have been revealed to be adjacent to the partial or complete set of the pceABCT cluster.
A composite circularized transposon, Tn-Dha1, that contains two identical ISs (ISDha1) surrounding the pceABCT gene cluster was identified in D. hafniense strain TCE1 (Maillard et al., 2005), but in the absence of PCE, Tn-Dha1 was rapidly lost (Banerjee and Ragsdale, 2003). In recent genomic studies of Dehalobacter strains, transposition also appears to be an important horizontal transfer mechanism for the reductive dehalogenase genes (rdh genes). However, further validation of this mechanism is required (Tang et al., 2012; Deshpande et al., 2013; Kruse et al., 2013).
Comparative genomic analyses of Dehalococcoides sp. strains VS and BAV1 as well as other enrichment cultures (ANAS and KB-1) revealed horizontal transfer of the VC reductase encoding genes vcrABC and bvcAB that were embedded in GIs specifically integrated at the single-copy gene, ssrA, encoding a structural RNA (McMurdie et al., 2009, 2011). Also, microarray transcriptomic studies proved the up-regulation of bacteriophage and transposase genes was observed in D. ethenogenes strain 195 and a mixed microbial consortium (KB-1) in response to starvation (Grostern et al., 2010).
Interestingly, most of the rdhAB operons are not passengers of HGT through recently acquired GIs; instead, they are inherited vertically, as only a few of these operons are located in the GIs. Of a total of 19 putative rdhAB operons in D. ethenogenes 195 only three of them are found in GIs. Similarly, only the cfrAB operon (out of 17 rdhAB operons) in the Dehalobacter CF was found within a GI. For Desulfitobacterium dehalogenans, none of its six rdhAB operons are found in a GI. Nevertheless, it is obvious that ORB genomes are dynamic, harboring many GIs. Also, diversity is observable in rdhAB regions. One possible explanation for this is the evidence of various mobile genetic elements (MGEs) in the close proximity of rdhAB operons, and many rdhAB related genes (e.g., PceC, PceT, CRP etc.) are seriously disrupted by MGE insertion. In case of Desulfitobacterium hafniense Y51 and TCE-1, they also have catabolic transposons harboring rdhAB operons. This type of transposon is functional and easily travels among bacteria.
HGT is important for microorganisms exposed to environmental stress caused by toxic polychlorinated pollutants to develop acquired catabolic pathways from even phylogenetically distinct bacteria in order to adapt to contaminated ecosystems and compete in the microbial community. The cells equipped with reductive dehalogenation capacity via HGT of rdh genes from other ORB can become able to use organohalides either in their respiratory process as the terminal electron acceptors or co-metabolically to permit tolerance to high levels of organochlorines (Liang et al., 2012). This may also lead to formation of a large proportion of the microbial community that is best equipped for given organochlorine degradation and further facilitate a HGT-mediated in situ bioremediation approach.
Role and Significance of ORB and RDases in Bioremediation
Anaerobic reductive dehalogenation can be a critical initial step in the bioremediation of many organohalides (for example, PCE/TCE to cis-DCE); however, complete detoxification usually requires further aerobic oxidation of the dechlorination products, such as cis-DCE and VC (Coleman et al., 2002). It should however, be noted that certain ORB-containing enrichment cultures, such as Dehalococcoides-containing KB-1 culture and Dehalobacter-rich AusCF culture, have been found to completely dechlorinate PCE to ethene (Major et al., 2002) and TCM to acetate and hydrogen (Lee et al., 2012), respectively. Although such complete reductive dehalogenation under anaerobic conditions is feasible, great attention should be paid to the risk associated with the accumulation of similarly toxic cis-DCE and VC, which may require further biostimulation with excess electron donors. Such additional stimulation can cause further competing processes such as nitrate and sulfate reduction and methanogenesis (Sun and Cupples, 2012; Révész et al., 2014). Hence, development of processes based on sequential stimulation of anaerobic-aerobic biodegradation has attracted significant attention and has been demonstrated (Thullner et al., 2012; Matturro et al., 2013) followed by further electro-bioremediation approaches (Rossetti et al., 2008; Matturro et al., 2012). Alternatively, the complete dechlorination of TCE to ethene of the Dehalococcoides mixed culture, which already had TceA and VcrA, was further enhanced by additional inoculation of Dehalococcoides strain BAV1, which contains BvcA enzyme for dehalogenation of cis-DCE and VC to ethene, using anaerobic biotrickling filters (Futagami et al., 2011).
Numerous technical aspects must be taken into account for subsurface bioremediation technologies. A critical step is site assessment and process monitoring based on data obtained from site samples using chemical (such as contaminant identification and characterization, electron acceptor alternatives, local nutrients, redox potential, pH, and the presence of potential inhibitors of reductive dehalogenation) and microbiological analyses (molecular biological tools for microbial community diversity and activity analyses). Recent rapid advances in molecular biological tools have had a profound effect on the understanding of bioremediation processes in the field biological remedial processes and the most widely used tool is qPCR for the 16S rRNA gene for bacterial identification (Smits et al., 2004; Chen et al., 2014). It was recently demonstrated that TaqMan chemistry (the fluorogenic 5′ nuclease) is recommended for enumeration of Dehalococcoides 16S rRNA biomarker genes over SYBR Green I detection chemistry, where nonspecific amplification observed in groundwater sample assessment (Hatt and Löffler, 2012). Moreover, qPCR targeted to rdhA genes using degenerate and gene-specific primers provides more accurate information with regard to characterization of dechlorination activity of the microbial community (Regeard et al., 2004; Lee et al., 2006; Ritalahti et al., 2006; Cupples, 2008). The applicability of qPCR in the near term has further been strengthened by its extension to mRNA-based qPCR, which offer more direct assessment of dechlorination activity. Furthermore, a microfluidics-based, nanoliter qPCR platform has recently been designed for the quantification of rdh gene repertoires and applied to on-site quantitative analysis of microbial diversity (Mayer-Blackwell et al., 2014). Among other tools reported are denaturing gradient gel electrophoresis (DGGE; Grostern and Edwards, 2006; Futagami et al., 2011), terminal restriction fragment length polymorphism (T-RFLP; Major et al., 2002), fluorescence in situ hybridization (FISH; Rossetti et al., 2008; Matturro et al., 2012, 2013), and DNA-stable isotope probing (SIP) or compound specific stable isotope analysis (CSIA; Sun and Cupples, 2012; Thullner et al., 2012; Révész et al., 2014). The long-term need for molecular biology tools for bioremediation research and implementation has to some degree been met by recent advances in the “omics” (Regeard et al., 2004; Smits et al., 2004; Ritalahti et al., 2006; Hatt and Löffler, 2012) and array approaches; however, their full scale on-site practicability is yet to be established. On-site monitoring of genetic biomarkers is desirable and this was recently demonstrated by the design of a hand-held device (termed Gene-Z), which allows real-time and DNA extraction-free loop mediated isothermal amplification using primers targeted at Dehalococcoides spp. specific 16S rRNA and vcrA genes (Grostern and Edwards, 2006).
Biostimulation approaches can facilitate the implementation of a successful bioremedy via adjustment of pH and redox potential with addition of base or external reducing equivalents (Dybas et al., 2002). A critical issue for successful in situ anaerobic bioremediation is the delivery of organic substrates or donors with sufficient loading rates and uniform distribution into the subsurface. Failure to do this may potentially lead to accumulation of regulated intermediate degradation products, such as cis-DCE and VC. Lactate, molasses, vegetable, or emulsified vegetable oil, hydrogen release compound (HRC®), mulch and compost are common electron donors but variable approaches have to be designed for different substrates, as each have differing physio-chemical properties (Henry, 2010). Microbiological bottlenecks, in particular the absence or insufficient quantity of ORB with desired dechlorination activity, can also be addressed via bioaugmentation approaches. Notable pioneering efforts were made on PCE detoxification by Dehalococcoides augmentation (Major et al., 2002; Lee et al., 2006) that paved a way to more recent field scale practices (Ritalahti et al., 2005; Cupples, 2008; Schaefer et al., 2009; Justicia-Leon et al., 2014; Révész et al., 2014). Genetic engineering of environmental microorganisms for bioremediation purposes could be a considered option, however, such genetic modification processes are scientifically challenging and are likely to be problematic in terms of regulatory policies (Snow et al., 2005; de Lorenzo, 2009).
Cell-free enzymatic bioremediation has been an attractive alternative to bioaugmentation, owing to its several advantages, such as growth-rate independence, potentially faster reaction rates, targeted and predictable activity, greater physiochemical tolerances, readily biodegradable, potential for improvement or modification by protein engineering, fermentation-based large-scale production and no contamination with genetic material (Sutherland et al., 2004; Russell et al., 2011). Notwithstanding these advantages, significant limitations and challenges still remain to be addressed. In view of enzyme production and their characteristics, major hurdles remain, including obtaining and maintaining RDases in a catalytically active form with sufficient yield, reconstitution of corrinoid cofactors and Fe-S clusters under anaerobic conditions, physical tolerance against extreme environmental conditions with variable pH and temperature and inexpensive production costs for pure enzymes. Additionally, negative effects of the pollutants present on the enzyme efficiency, costly purification processes of the free enzymes and enzyme instability under harsh environmental conditions may practically restrict the use of free-enzyme bioremedients. Whilst immobilized enzymes on suitable carriers may be more feasible (Gianfreda and Rao, 2004), their application in the bioremediation of organohalide-polluted sites remains some way off.
Concluding Remarks and Future Research
Recent advances in optimizing growth conditions of ORB, understanding metabolic pathways via omics-based approaches, expressing functional enzymes in recombinant forms and elucidating protein crystal structures have significantly progressed understanding of the microbial mechanisms of dehalogenation. Nevertheless, generation of a sufficient quantity of biocatalysts with high activity remains as a significant challenge for developing further understanding of structure-function relationships. The recombinant approach, whilst promising, requires significant development to produce functional holoenzymes with the correct corrinoid cofactor and Fe-S clusters. However, recombinant strategies have begun to demonstrate specific activities which are comparable to purified native enzymes and recombinant expression provides future capacity for the enhancement of the enzyme activity, tuning substrate specificity, and improving enzyme stability. Both rational (protein engineering) and irrational (directed evolution) approaches can be employed to increase recombinant enzyme diversity. Clustering of known enzymes based on amino acid sequence revealed in this study, suggests that rational approaches to engineering, (for example substrate specificity), can be informed by this type of analysis. In addition, the further exploration of novel niches, harboring microorganisms with organohalide-respiring capability via reductive dehalogenation, is expected to continue and yield new insights. More effort should be devoted to metatranscriptomic studies of microbial consortia to identify novel candidates with potential organohalide biodegradation applications. Metagenomic mining for novel rdhA genes from environmental samples further provides potential to discover new dehalogenation genes from non-culturable members of these niche environments.
Author Contributions
BJ, HE, SB, ML, CM, and MM contributed to the conception and design of the work and to the acquisition, analysis and interpretation of the data. All authors contributed to the drafting of the manuscript and approved the final version to be published.
Funding
This work was in part supported by an ARC Linkage grant (LP130100454). BJ is the recipient of a University of New South Wales postgraduate scholarship.
Conflict of Interest Statement
The authors declare that the research was conducted in the absence of any commercial or financial relationships that could be construed as a potential conflict of interest.
Acknowledgments
BJ is thankful to the University of New South Wales for provision of a University International Postgraduate Award.
References
Banerjee, R., and Ragsdale, S. W. (2003). The many faces of vitamin B12: catalysis by cobalamin-dependent enzymes. Annu. Rev. Biochem. 72, 209–247. doi: 10.1146/annurev.biochem.72.121801.161828
Bommer, M., Kunze, C., Fesseler, J., Schubert, T., Diekert, G., and Dobbek, H. (2014). Structural basis for organohalide respiration. Science 346, 455–458. doi: 10.1126/science.1258118
Boyer, A., Pagé-BéLanger, R., Saucier, M., Villemur, R., Lépine, F., Juteau, P., et al. (2003). Purification, cloning and sequencing of an enzyme mediating the reductive dechlorination of 2,4,6-trichlorophenol from Desulfitobacterium frappieri PCP-1. Biochem. J. 373, 297–303. doi: 10.1042/bj20021837
Buttet, G. F., Holliger, C., and Maillard, J. (2013). Functional genotyping of Sulfurospirillum spp. in mixed cultures allowed the identification of a new tetrachloroethene reductive dehalogenase. Appl. Environ. Microbiol. 79, 6941–6947. doi: 10.1128/AEM.02312-13
Chen, J., Bowman, K. S., Rainey, F. A., and Moe, W. M. (2014). Reassessment of PCR primers targeting 16S rRNA genes of the organohalide-respiring genus Dehalogenimonas. Biodegradation 25, 747–756. doi: 10.1007/s10532-014-9696-z
Coleman, N. V., Mattes, T. E., Gossett, J. M., and Spain, J. C. (2002). Phylogenetic and kinetic diversity of aerobic vinyl chloride-assimilating bacteria from contaminated sites. Appl. Environ. Microbiol. 68, 6162–6171. doi: 10.1128/AEM.68.12.6162-6171.2002
Cupples, A. M. (2008). Real-time PCR quantification of Dehalococcoides populations: methods and applications. J. Microbiol. Methods 72, 1–11. doi: 10.1016/j.mimet.2007.11.005
Delgado, A., Fajardo-Williams, D., Popat, S. C., Torres, C. I., and Krajmalnik-Brown, R. (2014). Successful operation of continuous reactors at short retention times results in high-density, fast-rate Dehalococcoides dechlorinating cultures. Appl. Microbiol. Biotechnol. 98, 2729–2737. doi: 10.1007/s00253-013-5263-5
de Lorenzo, V. (2009). Recombinant bacteria for environmental release: what went wrong and what we have learnt from it. Clin. Microbiol. Infect. 15(Suppl. 1), 63–65. doi: 10.1111/j.1469-0691.2008.02683.x
Deshpande, N. P., Wong, Y. K., Manefield, M., Wilkins, M. R., and Lee, M. (2013). Genome sequence of Dehalobacter UNSWDHB, a chloroform-dechlorinating bacterium. Genome Announc. 1:e00720–13. doi: 10.1128/genomeA.00720-13
DeWeerd, K., Mandelco, L., Tanner, R., Woese, C., and Suflita, J. (1990). Desulfomonile tiedjei gen. nov. and sp. nov., a novel anaerobic, dehalogenating, sulfate-reducing bacterium. Arch. Microbiol. 154, 23–30. doi: 10.1007/BF00249173
Ding, C., Zhao, S., and He, J. (2014). A Desulfitobacterium sp. strain PR reductively dechlorinates both 1,1,1-trichloroethane and chloroform. Environ. Microbiol. 16, 3387–3397. doi: 10.1111/1462-2920.12387
Dybas, M. J., Hyndman, D. W., Heine, R., Tiedje, J., Linning, K., Wiggert, D., et al. (2002). Development, operation, and long-term performance of a full-scale biocurtain utilizing bioaugmentation. Environ. Sci. Technol. 36, 3635–3644. doi: 10.1021/es0114557
El Fantroussi, S., Naveau, H., and Agathos, S. N. (1998). Anaerobic dechlorinating bacteria. Biotechnol. Prog. 14, 167–188. doi: 10.1021/bp980011k
Fung, J. M., Morris, R. M., Adrian, L., and Zinder, S. H. (2007). Expression of reductive dehalogenase genes in Dehalococcoides ethenogenes strain 195 growing on tetrachloroethene, trichloroethene, or 2,3-dichlorophenol. Appl. Environ. Microbiol. 73, 4439–4445. doi: 10.1128/AEM.00215-07
Futagami, T., Okamoto, F., Hashimoto, H., Fukuzawa, K., Higashi, K., Nazir, K. H., et al. (2011). Enrichment and characterization of a trichloroethene-dechlorinating consortium containing multiple “Dehalococcoides” strains. Biosci. Biotechnol. Biochem. 75, 1268–1274. doi: 10.1271/bbb.110028
Gianfreda, L., and Rao, M. A. (2004). Potential of extra cellular enzymes in remediation of polluted soils: a review. Enzyme Microb. Technol. 35, 339–354. doi: 10.1016/j.enzmictec.2004.05.006
Goris, T., Schubert, T., Gadkari, J., Wubet, T., Tarkka, M., Buscot, F., et al. (2014). Insights into organohalide respiration and the versatile catabolism of Sulfurospirillum multivorans gained from comparative genomics and physiological studies. Environ. Microbiol. 16, 3562–3580. doi: 10.1111/1462-2920.12589
Gribble, G. W. (2003). The diversity of naturally produced organohalogens. Chemosphere 52, 289–297. doi: 10.1016/S0045-6535(03)00207-8
Gribble, G. W. (2010). Naturally Occurring Organohalogen Compounds: A Comprehensive Update. New York, NY: Springer-Verlag Wien.
Grostern, A., Duhamel, M., Dworatzek, S., and Edwards, E. A. (2010). Chloroform respiration to dichloromethane by a Dehalobacter population. Environ. Microbiol. 12, 1053–1060. doi: 10.1111/j.1462-2920.2009.02150.x
Grostern, A., and Edwards, E. A. (2006). Growth of Dehalobacter and Dehalococcoides spp. during degradation of chlorinated ethanes. Appl. Environ. Microbiol. 72, 428–436. doi: 10.1128/AEM.72.1.428-436.2006
Hatt, J. K., and Löffler, F. E. (2012). Quantitative real-time PCR (qPCR) detection chemistries affect enumeration of the Dehalococcoides 16S rRNA gene in groundwater. J. Microbiol. Methods 88, 263–270. doi: 10.1016/j.mimet.2011.12.005
Henry, B. (2010). Loading Rates and Impacts of Substrate Delivery for Enhanced Anaerobic Bioremediation. Arlington, VA: DTIC Document.
Holliger, C., Wohlfarth, G., and Diekert, G. (1998). Reductive dechlorination in the energy metabolism of anaerobic bacteria. FEMS Microbiol. Rev. 22, 383–398. doi: 10.1111/j.1574-6976.1998.tb00377.x
Hug, L. A., Maphosa, F., Leys, D., Löffler, F. E., Smidt, H., Edwards, E. A., et al. (2013). Overview of organohalide-respiring bacteria and a proposal for a classification system for reductive dehalogenases. Philos. Trans. R. Soc. Lond. B Biol. Sci. 368, 20120322. doi: 10.1098/rstb.2012.0322
Jones, D. T., Taylor, W. R., and Thornton, J. M. (1992). The rapid generation of mutation data matrices from protein sequences. Comput. Appl. Biosci. 8, 275–282. doi: 10.1093/bioinformatics/8.3.275
Jugder, B. E., Chen, Z., Ping, D. T., Lebhar, H., Welch, J., and Marquis, C. P. (2015a). An analysis of the changes in soluble hydrogenase and global gene expression in Cupriavidus necator (Ralstonia eutropha) H16 grown in heterotrophic diauxic batch culture. Microb. Cell Fact. 14, 42. doi: 10.1186/s12934-015-0226-4
Jugder, B. E., Ertan, H., Lee, M., Manefield, M., and Marquis, C. P. (2015b). Reductive dehalogenases come of age in biological destruction of organohalides. Trends Biotechnol. 33, 595–610. doi: 10.1016/j.tibtech.2015.07.004
Jugder, B. E., Welch, J., Aguey-Zinsou, K. F., and Marquis, C. P. (2013). Fundamentals and electrochemical applications of [Ni-Fe]-uptake hydrogenases. RSC Adv. 3, 8142–8159. doi: 10.1039/c3ra22668a
Justicia-Leon, S. D., Higgins, S., Mack, E. E., Griffiths, D. R., Tang, S., Edwards, E. A., et al. (2014). Bioaugmentation with distinct Dehalobacter strains achieves chloroform detoxification in microcosms. Environ. Sci. Technol. 48, 1851–1858. doi: 10.1021/es403582f
Kimoto, H., Suye, S., Makishima, H., Arai, J., Yamaguchi, S., Fujii, Y., et al. (2010). Cloning of a novel dehalogenase from environmental DNA. Biosci. Biotechnol. Biochem. 74, 1290–1292. doi: 10.1271/bbb.100027
Kruse, T., Maillard, J., Goodwin, L., Woyke, T., Teshima, H., Bruce, D., et al. (2013). Complete genome sequence of Dehalobacter restrictus PER-K23(T). Stand. Genomic Sci. 8, 375–388.
Kruse, T., van de Pas, B. A., Atteia, A., Krab, K., Hagen, W. R., Goodwin, L., et al. (2015). Genomic, proteomic, and biochemical analysis of the organohalide respiratory pathway in Desulfitobacterium dehalogenans. J. Bacteriol. 197, 893–904. doi: 10.1128/JB.02370-14
Krzmarzick, M. J., Crary, B. B., Harding, J. J., Oyerinde, O. O., Leri, A. C., Myneni, S. C., et al. (2012). Natural niche for organohalide-respiring Chloroflexi. Appl. Environ. Microbiol. 78, 393–401. doi: 10.1128/AEM.06510-11
Lee, M., Low, A., Zemb, O., Koenig, J., Michaelsen, A., and Manefield, M. (2012). Complete chloroform dechlorination by organochlorine respiration and fermentation. Environ. Microbiol. 14, 883–894. doi: 10.1111/j.1462-2920.2011.02656.x
Lee, M., Marquis, C., Judger, B.-E., and Manefield, M. (2015). Anaerobic microorganisms and bioremediation of organohalide pollution. Microbiol. Aust. 36, 125–128. doi: 10.1071/ma15044
Lee, P. K. H., Johnson, D. R., Holmes, V. F., He, J. Z., and Alvarez-Cohen, L. (2006). Reductive dehalogenase gene expression as a biomarker for physiological activity of Dehalococcoides spp. Appl. Environ. Microbiol. 72, 6161–6168. doi: 10.1128/AEM.01070-06
Liang, B., Jiang, J., Zhang, J., Zhao, Y., and Li, S. (2012). Horizontal transfer of dehalogenase genes involved in the catalysis of chlorinated compounds: evidence and ecological role. Crit. Rev. Microbiol. 38, 95–110. doi: 10.3109/1040841X.2011.618114
Louie, T. M., and Mohn, W. W. (1999). Evidence for a chemiosmotic model of dehalorespiration in Desulfomonile tiedjei DCB-1. J. Bacteriol. 181, 40–46.
Mabey, W., and Mill, T. (1978). Critical review of hydrolysis of organic compounds in water under environmental conditions. J. Phys. Chem. Ref. Data 7, 383–415. doi: 10.1063/1.555572
Mac Nelly, A., Kai, M., Svatoš, A., Diekert, G., and Schubert, T. (2014). Functional heterologous production of reductive dehalogenases from Desulfitobacterium hafniense strains. Appl. Environ. Microbiol. 80, 4313–4322. doi: 10.1128/AEM.00881-14
Magnuson, J. K., Romine, M. F., Burris, D. R., and Kingsley, M. T. (2000). Trichloroethene reductive Dehalogenase from Dehalococcoides ethenogenes: sequence of tceA and substrate range characterization. Appl. Environ. Microbiol. 66, 5141–5147. doi: 10.1128/AEM.66.12.5141-5147.2000
Magnuson, J. K., Stern, R. V., Gossett, J. M., Zinder, S. H., and Burris, D. R. (1998). Reductive dechlorination of tetrachloroethene to ethene by a two-component enzyme pathway. Appl. Environ. Microbiol. 64, 1270–1275.
Maillard, J., Regeard, C., and Holliger, C. (2005). Isolation and characterization of Tn-Dha1, a transposon containing the tetrachloroethene reductive dehalogenase of Desulfitobacterium hafniense strain TCE1. Environ. Microbiol. 7, 107–117. doi: 10.1111/j.1462-2920.2004.00671.x
Major, D. W., McMaster, M. L., Cox, E. E., Edwards, E. A., Dworatzek, S. M., Hendrickson, E. R., et al. (2002). Field demonstration of successful bioaugmentation to achieve dechlorination of tetrachloroethene to ethene. Environ. Sci. Technol. 36, 5106–5116. doi: 10.1021/es0255711
Maphosa, F., de Vos, W. M., and Smidt, H. (2010). Exploiting the ecogenomics toolbox for environmental diagnostics of organohalide-respiring bacteria. Trends Biotechnol. 28, 308–316. doi: 10.1016/j.tibtech.2010.03.005
Maphosa, F., Lieten, S. H., Dinkla, I., Stams, A. J., Smidt, H., and Fennell, D. E. (2012). Ecogenomics of microbial communities in bioremediation of chlorinated contaminated sites. Front. Microbiol. 3:351. doi: 10.3389/fmicb.2012.00351
Marzorati, M., de Ferra, F., Van Raemdonck, H., Borin, S., Allifranchini, E., Carpani, G., et al. (2007). A novel reductive dehalogenase, identified in a contaminated groundwater enrichment culture and in Desulfitobacterium dichloroeliminans strain DCA1, is linked to dehalogenation of 1,2-dichloroethane. Appl. Environ. Microbiol. 73, 2990–2999. doi: 10.1128/AEM.02748-06
Matturro, B., Aulenta, F., Majone, M., Papini, M. P., Tandoi, V., and Rossetti, S. (2012). Field distribution and activity of chlorinated solvents degrading bacteria by combining CARD-FISH and real time PCR. N. Biotechnol. 30, 23–32. doi: 10.1016/j.nbt.2012.07.006
Matturro, B., Heavner, G. L., Richardson, R. E., and Rossetti, S. (2013). Quantitative estimation of Dehalococcoides mccartyi at laboratory and field scale: comparative study between CARD-FISH and real time PCR. J. Microbiol. Methods 93, 127–133. doi: 10.1016/j.mimet.2013.02.011
Mayer-Blackwell, K., Azizian, M. F., Machak, C., Vitale, E., Carpani, G., de Ferra, F., et al. (2014). Nanoliter qPCR platform for highly parallel, quantitative assessment of reductive dehalogenase genes and populations of dehalogenating microorganisms in complex environments. Environ. Sci. Technol. 48, 9659–9667. doi: 10.1021/es500918w
McMurdie, P. J., Behrens, S. F., Müller, J. A., Göke, J., Ritalahti, K. M., Wagner, R., et al. (2009). Localized plasticity in the streamlined genomes of vinyl chloride respiring Dehalococcoides. PLoS Genet. 5:e1000714. doi: 10.1371/journal.pgen.1000714
McMurdie, P. J., Hug, L. A., Edwards, E. A., Holmes, S., and Spormann, A. M. (2011). Site-specific mobilization of vinyl chloride respiration islands by a mechanism common in Dehalococcoides. BMC Genomics 12:287. doi: 10.1186/1471-2164-12-287
Middeldorp, P. J. M., Luijten, M. L. G. C., van de Pas, B. A., van Eekert, M. H. A., Kengen, S. W. M., Schraa, G., et al. (1999). Anaerobic microbial reductive dehalogenation of chlorinated ethenes. Bioremediat. J. 3, 151–169. doi: 10.1080/10889869991219280
Miller, E., Wohlfarth, G., and Diekert, G. (1996). Studies on tetrachloroethene respiration in Dehalospirillum multivorans. Arch. Microbiol. 166, 379–387. doi: 10.1007/BF01682983
Miller, E., Wohlfarth, G., and Diekert, G. (1998). Purification and characterization of the tetrachloroethene reductive dehalogenase of strain PCE-S. Arch. Microbiol. 169, 497–502. doi: 10.1007/s002030050602
Morris, R. M., Sowell, S., Barofsky, D., Zinder, S., and Richardson, R. (2006). Transcription and mass-spectroscopic proteomic studies of electron transport oxidoreductases in Dehalococcoides ethenogenes. Environ. Microbiol. 8, 1499–1509. doi: 10.1111/j.1462-2920.2006.01090.x
Müller, J. A., Rosner, B. M., Von Abendroth, G., Meshulam-Simon, G., McCarty, P. L., and Spormann, A. M. (2004). Molecular identification of the catabolic vinyl chloride reductase from Dehalococcoides sp. strain VS and its environmental distribution. Appl. Environ. Microbiol. 70, 4880–4888. doi: 10.1128/AEM.70.8.4880-4888.2004
Neumann, A., Seibert, A., Trescher, T., Reinhardt, S., Wohlfarth, G., and Diekert, G. (2002). Tetrachloroethene reductive dehalogenase of Dehalospirillum multivorans: substrate specificity of the native enzyme and its corrinoid cofactor. Arch. Microbiol. 177, 420–426. doi: 10.1007/s00203-002-0409-3
Neumann, A., Wohlfarth, G., and Diekert, G. (1996). Purification and characterization of tetrachloroethene reductive dehalogenase from Dehalospirillum multivorans. J. Biol. Chem. 271, 16515–16519. doi: 10.1074/jbc.271.28.16515
Neumann, A., Wohlfarth, G., and Diekert, G. (1998). Tetrachloroethene dehalogenase from Dehalospirillum multivorans: cloning, sequencing of the encoding genes, and expression of the pceA gene in Escherichia coli. J. Bacteriol. 180, 4140–4145.
Ni, S., Fredrickson, J. K., and Xun, L. (1995). Purification and characterization of a novel 3-chlorobenzoate-reductive dehalogenase from the cytoplasmic membrane of Desulfomonile tiedjei DCB-1. J. Bacteriol. 177, 5135–5139.
Padilla-Crespo, E., Yan, J., Swift, C., Wagner, D. D., Chourey, K., Hettich, R. L., et al. (2014). Identification and environmental distribution of dcpA, which encodes the reductive dehalogenase catalyzing the dichloroelimination of 1,2-dichloropropane to propene in organohalide-respiring Chloroflexi. Appl. Environ. Microbiol. 80, 808–818. doi: 10.1128/AEM.02927-13
Parthasarathy, A., Stich, T. A., Lohner, S. T., Lesnefsky, A., Britt, R. D., and Spormann, A. M. (2015). Biochemical and EPR-spectroscopic investigation into heterologously expressed vinyl chloride reductive dehalogenase (VcrA) from Dehalococcoides mccartyi strain VS. J. Am. Chem. Soc. 137, 3525–3532. doi: 10.1021/ja511653d
Payne, K. A., Quezada, C. P., Fisher, K., Dunstan, M. S., Collins, F. A., Sjuts, H., et al. (2014). Reductive dehalogenase structure suggests a mechanism for B12-dependent dehalogenation. Nature 517, 513–516. doi: 10.1038/nature13901
Regeard, C., Maillard, J., and Holliger, C. (2004). Development of degenerate and specific PCR primers for the detection and isolation of known and putative chloroethene reductive dehalogenase genes. J. Microbiol. Methods 56, 107–118. doi: 10.1016/j.mimet.2003.09.019
Révész, K. M., Lollar, B. S., Kirshtein, J. D., Tiedeman, C. R., Imbrigiotta, T. E., Goode, D. J., et al. (2014). Integration of stable carbon isotope, microbial community, dissolved hydrogen gas, and 2HH2O tracer data to assess bioaugmentation for chlorinated ethene degradation in fractured rocks. J. Contam. Hydrol. 156, 62–77. doi: 10.1016/j.jconhyd.2013.10.004
Richardson, R. E. (2013). Genomic insights into organohalide respiration. Curr. Opin. Biotechnol. 24, 498–505. doi: 10.1016/j.copbio.2013.02.014
Ritalahti, K. M., Amos, B. K., Sung, Y., Wu, Q. Z., Koenigsberg, S. S., and Löffler, F. E. (2006). Quantitative PCR targeting 16S rRNA and reductive dehalogenase genes simultaneously monitors multiple Dehalococcoides strains. Appl. Environ. Microbiol. 72, 2765–2774. doi: 10.1128/AEM.72.4.2765-2774.2006
Ritalahti, K. M., Loffler, F. E., Rasch, E. E., and Koenigsberg, S. S. (2005). Bioaugmentation for chlorinated ethene detoxification: bioaugmentation and molecular diagnostics in the bioremediation of chlorinated ethene-contaminated sites. Ind. Biotechnol. 1, 114–118. doi: 10.1089/ind.2005.1.114
Rossetti, S., Aulenta, F., Majone, M., Crocetti, G., and Tandoi, V. (2008). Structure analysis and performance of a microbial community from a contaminated aquifer involved in the complete reductive dechlorination of 1,1,2,2-tetrachloroethane to ethene. Biotechnol. Bioeng. 100, 240–249. doi: 10.1002/bit.21776
Rupakula, A., Kruse, T., Boeren, S., Holliger, C., Smidt, H., and Maillard, J. (2013). The restricted metabolism of the obligate organohalide respiring bacterium Dehalobacter restrictus: lessons from tiered functional genomics. Philos. Trans. R. Soc. Lond. B Biol. Sci. 368, 20120325. doi: 10.1098/rstb.2012.0325
Russell, R. J., Scott, C., Jackson, C. J., Pandey, R., Pandey, G., Taylor, M. C., et al. (2011). The evolution of new enzyme function: lessons from xenobiotic metabolizing bacteria versus insecticide-resistant insects. Evol. Appl. 4, 225–248. doi: 10.1111/j.1752-4571.2010.00175.x
Schaefer, C. E., Condee, C. W., Vainberg, S., and Steffan, R. J. (2009). Bioaugmentation for chlorinated ethenes using Dehalococcoides sp.: comparison between batch and column experiments. Chemosphere 75, 141–148. doi: 10.1016/j.chemosphere.2008.12.041
Schink, B., and Friedrich, M. (1994). Energetics of syntrophic fatty-acid oxidation. FEMS Microbiol. Rev. 15, 85–94. doi: 10.1111/j.1574-6976.1994.tb00127.x
Schumacher, W., and Holliger, C. (1996). The proton/electron ratio of the menaquinone-dependent electron transport from dihydrogen to tetrachloroethene in "Dehalobacter restrictus". J. Bacteriol. 178, 2328–2333.
Schumacher, W., Holliger, C., Zehnder, A. J., and Hagen, W. R. (1997). Redox chemistry of cobalamin and iron-sulfur cofactors in the tetrachloroethene reductase of Dehalobacter restrictus. FEBS Lett. 409, 421–425. doi: 10.1016/S0014-5793(97)00520-6
Shelton, D. R., and Tiedje, J. M. (1984). Isolation and partial characterization of bacteria in an anaerobic consortium that mineralizes 3-chlorobenzoic acid. Appl. Environ. Microbiol. 48, 840–848.
Sjuts, H., Fisher, K., Dunstan, M. S., Rigby, S. E., and Leys, D. (2012). Heterologous expression, purification and cofactor reconstitution of the reductive dehalogenase PceA from Dehalobacter restrictus. Protein Expr. Purif. 85, 224–229. doi: 10.1016/j.pep.2012.08.007
Smits, T. H., Devenoges, C., Szynalski, K., Maillard, J., and Holliger, C. (2004). Development of a real-time PCR method for quantification of the three genera Dehalobacter, Dehalococcoides, and Desulfitobacterium in microbial communities. J. Microbiol. Methods 57, 369–378. doi: 10.1016/j.mimet.2004.02.003
Snow, A. A., Andow, D. A., Gepts, P., Hallerman, E. M., Power, A., Tiedje, J. M., et al. (2005). Genetically engineered organisms and the environment: current status and recommendations. Ecol. Appl. 15, 377–404. doi: 10.1890/04-0539
Sun, W., and Cupples, A. M. (2012). Diversity of five anaerobic toluene-degrading microbial communities investigated using stable isotope probing. Appl. Environ. Microbiol. 78, 972–980. doi: 10.1128/AEM.06770-11
Sutherland, T. D., Horne, I., Weir, K. M., Coppin, C. W., Williams, M. R., Selleck, M., et al. (2004). Enzymatic bioremediation: from enzyme discovery to applications. Clin. Exp. Pharmacol. Physiol. 31, 817–821. doi: 10.1111/j.1440-1681.2004.04088.x
Suyama, A., Yamashita, M., Yoshino, S., and Furukawa, K. (2002). Molecular characterization of the PceA reductive dehalogenase of Desulfitobacterium sp. strain Y51. J. Bacteriol. 184, 3419–3425. doi: 10.1128/JB.184.13.3419-3425.2002
Tamura, K., Stecher, G., Peterson, D., Filipski, A., and Kumar, S. (2013). MEGA6: molecular evolutionary genetics analysis version 6.0. Mol. Biol. Evol. 30, 2725–2729. doi: 10.1093/molbev/mst197
Tang, S., Chan, W. W., Fletcher, K. E., Seifert, J., Liang, X., Löffler, F. E., et al. (2013). Functional characterization of reductive dehalogenases by using blue native polyacrylamide gel electrophoresis. Appl. Environ. Microbiol. 79, 974–981. doi: 10.1128/AEM.01873-12
Tang, S., Gong, Y., and Edwards, E. A. (2012). Semi-automatic in silico gap closure enabled de novo assembly of two Dehalobacter genomes from metagenomic data. PLoS ONE 7:e52038. doi: 10.1371/journal.pone.0052038
Tang, S. Q., and Edwards, E. A. (2013). Identification of Dehalobacter reductive dehalogenases that catalyse dechlorination of chloroform, 1,1,1-trichloroethane and 1,1-dichloroethane. Philos. Trans. R. Soc. B Biol. Sci. 368:20120318. doi: 10.1098/rstb.2012.0318
Thibodeau, J., Gauthier, A., Duguay, M., Villemur, R., Lépine, F., Juteau, P., et al. (2004). Purification, cloning, and sequencing of a 3,5-dichlorophenol reductive dehalogenase from Desulfitobacterium frappieri PCP-1. Appl. Environ. Microbiol. 70, 4532–4537. doi: 10.1128/AEM.70.8.4532-4537.2004
Thullner, M., Centler, F., Richnow, H.-H., and Fischer, A. (2012). Quantification of organic pollutant degradation in contaminated aquifers using compound specific stable isotope analysis – review of recent developments. Org. Geochem. 42, 1440–1460. doi: 10.1016/j.orggeochem.2011.10.011
Vainberg, S., Condee, C. W., and Steffan, R. J. (2009). Large-scale production of bacterial consortia for remediation of chlorinated solvent-contaminated groundwater. J. Ind. Microbiol. Biotechnol. 36, 1189–1197. doi: 10.1007/s10295-009-0600-5
van de Pas, B. A., Gerritse, J., de Vos, W. M., Schraa, G., and Stams, A. J. (2001). Two distinct enzyme systems are responsible for tetrachloroethene and chlorophenol reductive dehalogenation in Desulfitobacterium strain PCE1. Arch. Microbiol. 176, 165–169. doi: 10.1007/s002030100316
van de Pas, B. A., Smidt, H., Hagen, W. R., van der Oost, J., Schraa, G., Stams, A. J., et al. (1999). Purification and molecular characterization of ortho-chlorophenol reductive dehalogenase, a key enzyme of halorespiration in Desulfitobacterium dehalogenans. J. Biol. Chem. 274, 20287–20292. doi: 10.1074/jbc.274.29.20287
Xun, L., Topp, E., and Orser, C. S. (1992). Purification and characterization of a tetrachloro-p-hydroquinone reductive dehalogenase from a Flavobacterium sp. J. Bacteriol. 174, 8003–8007.
Keywords: reductive dehalogenase, organohalide respiration, bioremediation, Dehalobacter, Dehalococcoides
Citation: Jugder B-E, Ertan H, Bohl S, Lee M, Marquis CP and Manefield M (2016) Organohalide Respiring Bacteria and Reductive Dehalogenases: Key Tools in Organohalide Bioremediation. Front. Microbiol. 7:249. doi: 10.3389/fmicb.2016.00249
Received: 09 December 2015; Accepted: 15 February 2016;
Published: 01 March 2016.
Edited by:
Gavin Collins, National University of Ireland, IrelandReviewed by:
Jun-Jie Zhang, Wuhan Institute of Virology, ChinaChristopher L. Hemme, University of Rhode Island, USA
Copyright © 2016 Jugder, Ertan, Bohl, Lee, Marquis and Manefield. This is an open-access article distributed under the terms of the Creative Commons Attribution License (CC BY). The use, distribution or reproduction in other forums is permitted, provided the original author(s) or licensor are credited and that the original publication in this journal is cited, in accordance with accepted academic practice. No use, distribution or reproduction is permitted which does not comply with these terms.
*Correspondence: Christopher P. Marquis, Yy5tYXJxdWlzQHVuc3cuZWR1LmF1