- 1College of Horticulture, Gansu Agricultural University, Lanzhou, China
- 2Institute of Fruit Tree Research, Guangdong Academy of Agricultural Sciences, Guangzhou, China
- 3Agro-biological Gene Research Center, Guangdong Academy of Agricultural Sciences, Guangzhou, China
- 4College of Horticulture, Qingdao Agricultural University, Qingdao, China
Fusarium wilt of banana, a destructive disease that affects banana production, is caused by Fusarium oxysporum f. sp. cubense tropical race 4 (Foc TR4). In a previous study, we confirmed the strong inhibitory effects of Chinese leek (Allium tuberosum) on the incidence of this disease. Sulfur compounds are the primary antifungal constituents of Chinese leek. Among these, dimethyl trisulfide (DT) was the most abundant and exhibited the strongest inhibition of Foc TR4 growth and development. In the present study, the global gene expression profiles of Foc TR4 isolates treated with DT at 4,000-folds dilution (concentration of 1/4,000, v/v) for 1.5, 6, and 12 h were investigated by using RNA sequencing. The expression patterns of 15 DEGs were validated based on quantitative real-time PCR (qRT-PCR) assay. Untreated sample presented 2,556, 1,691, and 1,150 differentially expressed genes (DEGs) at 1.5, 6, and 12 h after the onset of the experiment, respectively, whereas DT-treated isolates presented 2,823, 3,546, and 6,197 DEGs. Based on Gene Ontology (GO) annotation and Kyoto Encyclopedia of Genes and Genomes (KEGG) enrichment analysis, DEGs involved in endoplasmic reticulum (ER), glycosylation, and steroid biosynthesis were significantly inhibited by DT exposure. The similar expressional patterns of 15 DEGs between RNA-seq and qRT-PCR assays indicated the reliability of the RNA-seq data. In conclusion, ER stress related to glycosylation inhibition and damage to cell membrane integrity might contribute to the toxicity of DT against Foc TR4. As the results presented here evidenced changes in gene expression associated with DT exposure, which might be used to develop new approaches for controlling FWB.
Introduction
Fusarium oxysporumS has been considered one of the most prevalent fungal pathogens, having a wide host range and causing severe losses in multiple crops such as tomato, cotton, maize, and banana (Dean et al., 2012; Aiyaz et al., 2016; Nirmaladevi et al., 2016). FWB, caused by Foc, is one of the most destructive diseases affecting banana cultivation worldwide (Ploetz, 2015), and has led to considerable losses in production since it was first discovered in Austria in 1876 (Ploetz and Pegg, 1997, 2000). It is currently found in all banana-producing regions of the world, including southern Asia, Africa, and Latin America (García-Bastidas et al., 2014; Ordonez et al., 2015; Ploetz, 2015). The pathogen has divided into 4 races and 24 VCGs, based on host type and vegetative compatibility, respectively (Das et al., 2012; Fourie et al., 2009). Among these, Foc tropical race 4 (Foc TR4, VCG 01213/16) is one of the most concern owing to its wide host range and strong pathogenicity (Li et al., 2012).
Resistance breeding is traditionally regarded as the most durable, environmentally friendly, and convenient control practice (Hwang and Ko, 2004). However, owing to the long cultivation cycle of banana and the rapid evolution of Foc, few resistant cultivars have been used in the field to date (Ploetz, 2015). Considerable interest is therefore focused on the exploitation of naturally occurring organisms, such as nonpathogenic F. oxysporum, Burkholderia cenocepacia, Bacillus amyloliquefaciens, and Streptomyces albospinus for the control of crop diseases including FWB (Postma and Rattink, 1992; Raguchander et al., 1997; Butt et al., 2001; Fravel et al., 2003; Cao et al., 2005; Asha et al., 2011a,b; Wang et al., 2013; Ho et al., 2015). Furthermore, many antifungal secondary metabolites have been identified from plants and microorganism (Paiva et al., 2010; Coleman et al., 2011), and the identification of novel antifungal targets for use as control agents is currently becoming an important strategy (De Backer and Van Dijck, 2003; Walsh et al., 2010). Some of these targets include chitin, the major component of the fungal cell wall, and ergosterol, which is essential to membrane formation. These components, being absent in most mammalian and plant cells, have been considered as main targets of antifungal compounds to prevent and control fungal infections (Behr, 2011; Alcazar-Fuoli and Mellado, 2013). However, the long-term intensive use of single target inhibitors often results in the enhancement of fungal drug resistance. Therefore, it is urgent to identify alternative therapeutics for future use.
It is also crucial to investigate the mechanisms by which these compounds exert their fungicidal activity, not only for discovery of new antifungal substances and identification of their target sites, but also for risk assessment (Ma and Michailides, 2005). The emergence of high-throughput sequencing technologies and expansion of genomic information has provided new methodologies for the investigation of antifungal mechanisms and identification of potential targets (Cools and Hammond-Kosack, 2013). Numerous studies regarding the response of fungal gene expression profiles to plant essential oils have been conducted, and potential targets such as cell wall-, cell membrane- and secondary metabolism-related genes were found (Parveen et al., 2004; Yu et al., 2010). These results have supplied information that contributes to understanding the antifungal mechanisms of plant essential oils. However, systematic studies on the mechanism of toxicity of such compounds to Foc have been limited.
Recently, we demonstrated the significant inhibitory effect of the Chinese leek (Allium tuberosum) on the occurrence of FWB in the field (Huang et al., 2012). Furthermore, the strong inhibitory effects of Chinese leek root exudates and tissue extracts on Foc growth have also been confirmed using in vitro tests (Huang et al., 2012; Zuo et al., 2015), and the strong inhibitory effects of Chinese leek extracts and secondary metabolites on other pathogenic microorganisms and nematodes have been verified (Lee et al., 2004; Tsao and Yin, 2001; Irkin and Korukluoglu, 2007; Huang et al., 2016). Studies on the mechanism of toxicity of the secondary metabolites of Chinese leek revealed that they caused ROS burst and decrease of mitochondrial membrane potentials in FocTR4 cells (Zuo et al., 2015). The expression of the ergosterol biosynthesis genes CYP51-2 and CYP51-3 was reduced but that of the autophagy related genes ATG 1, ATG 8, and ATG 15 was induced at the early stage of the treatment of Foc cells with Chinese leek root exudates (Zuo et al., 2015). Sulfur and phenolic compounds were determined to be the primary antifungal compounds in Chinese leek; of these, DT was the principal component among the sulfur compounds and showed strong inhibitory effects on Foc growth and development (Zhang et al., 2013; Zuo et al., 2015).
In the present study, we firstly confirmed the toxicity of DT to Foc TR4. Further, to explore the molecular mechanism(s) underlying this toxicity and to identify the major target sites involved, global gene expression profiles of Foc TR4 at three time points with or without DT treatment were investigated using RNA-seq.
Materials and Methods
Fungal Isolates and Chemicals
Isolates of the pathogenic fungus Foc tropical race 4 (Foc TR4, ACCC 37982), which causes FWB, was obtained from diseased Cavendish ‘Brazilian’ (AAA) plants with FWB symptoms in Guangdong Province, China. Standard DT was purchased from Sigma-Aldrich Co. LLC (St. Louis, MO, United States).
Toxicity Test
The Foc TR4 isolates were cultured on potato dextrose agar for 5 days at 28°C in the dark. Conidia were then collected and transferred into PDB, and the final concentration of these suspensions was adjusted to 4 × 106 conidia/ml. For the toxicity test, DT were added into conidial suspensions, which were diluted 2,000, 4,000, 8,000, and 16,000 folds, with the final concentration were 1/2,000, 1/4,000, 1/8,000, and 1/16,000, respectively. Then the treated conidia were cultured for 6, 12, 24, or 48 h. The viability of conidia was analyzed using the FDA assay (Tolosa et al., 2015). In brief, control and treated conidia were incubated with 50 μM FDA (Life Technologies Inc., Gaithersburg, MD, United States) in the dark, for 5 min at 28°C. To remove redundant dye, conidia were washed three times in PBS (pH7.0). Qualitative analysis was performed using a multifunctional microplate reader (Varioskan Flash;Thermo Scientific, Wilmington, DE, United States) with an excitation wavelength of 488 nm and an emission wavelength of 530 nm.
RNA Isolation and RNA-seq Library Preparation and Sequencing
To further understand the molecular mechanism of the potential antifungal action, the global gene expression profiles at 1.5, 6, and 12 h of conidia treated with DT at 1/4000 (treated group; 1.5-T, 6-T, and 12-T) and untreated conidia (control group; 1.5-C, 6-C, and 12-C) were investigated by using RNA-seq. Conidia in the control group at 0 h (0-C) were used as the control. All conidia were collected by centrifugation, and total RNA was isolated by using the Fungi RNAout kit (60305-50, TiandzInc., Beijing, China). The quality and quantity of RNA was determined using 1.5% denatured agarose gel electrophoresis and a NanoDrop 2000 Spectrophotometer (Thermo Scientific), and an Agilent 2100 Bioanalyzer (Agilent Technologies, Palo Alto, CA, United States), respectively. Poly(A) mRNA was isolated by using oligo (dT) magnetic beads and then fragmented into short segments. First-strand cDNA was then synthesized by using a random hexamer-primer, whereas second strand cDNA was synthesized by using DNA polymerase I and RNase H. Double-strand cDNA was purified by using magnetic beads. End-repair and 3’-end single nucleotide A (adenine) addition were performed using T4 DNA polymerase and Klenow 3’- to 5’- exo-polymerase, respectively. Sequencing adaptors were ligated to the fragments using T4 quick ligase; the fragments were then enriched by using PCR amplification. The quality of the sample library and its quantification were obtained using the Agilent 2100 Bioanaylzer and an ABI StepOnePlus Real-Time PCR System (ABI, CA, United States). The cDNA library were sequenced via Illumina HiSeqTM 2000.
Assembly Screening for DEGs, GO Annotation, and KEGG Enrichment
We use SOAPnuke v1.5.2 and ‘-l 5 -q 0.3 -n 0.1’ for quality control and reads filtering, respectively. After that, clean reads were mapped to the genome sequence of Foc II5 (Fusarium Comparative Sequencing Project, Broad Institute of MIT and Harvard1). Gene expression levels were quantified based on the RSEM v1.2.20 with bam file alignment by Bowtie2 v2.2.3 and default RSEM parameters (RNASeq by Expectation Maximization) (Li and Dewey, 2011). Gene identification (gene ID), length, log2 ratio, false discovery rate (FDR), GO classification, and KEGG orthology were obtained. The DEGs were screened based on the Poisson distribution method (Audic and Claverie, 1997). We used a FDR ≤ 0.01 and an absolute value of log2 ratio ≥ 2 as thresholds judge the significance of differences in gene expression. The GO classification of DEGs and the distribution analysis of gene function in species at the macro level were conducted using WEGO software (Ye et al., 2006), whereas pathway enrichment analysis was obtained from KEGG (Kanehisa et al., 2008). GO categories with a level equal to “5” and “FDR < 0.05” in each sample were screened while six most enriched pathways were selected for further investigation.
Quantitative Real-Time PCR
Fifteen DEGs were further confirmed by using qRT-PCR. In brief, the total RNA from each sample was purified and converted into cDNA using the PrimeScriptTM RT reagent Kit with gDNA Eraser (Code No. RR047A, TAKARA Biotechnology CO., LTD., Dalian, China). Primer pairs were designed for each DEG by using the online software program Primer 32 (primer sequences are listed in Supplementary Table S1). The qRT-PCR was conducted on a CFX96 TouchTM Real-Time PCR Detection System (Bio-Rad, United States) by using SYBR®Premix ExTaqTM II (Tli Rnase H Plus; TAKARA Biotechnology CO., LTD.). The specificity of primers was assessed by using the dissociation curve method. Relative gene expression was normalized to that of actin (López-Berges et al., 2010) and calculated by the comparative –ΔΔCt method. Data from toxicity tests and qRT-PCR analysis were expressed as means ± SD of three independent replicates.
Results
Inhibition of Foc TR4 Growth by DT
To determine the inhibitory effects of DT on the growth of Foc TR4, the proliferation of conidia in control and treated groups was tested at various time points (Figure 1). In the untreated group (without DT), conidial viability increased to 1.78-, 5.67-, 16.44-, and 39.26-fold that of the control (0 h), after being cultured for 6, 12, 24, and 36 h, respectively. The growth ratio was significantly inhibited by DT at concentrations up to 1/16,000 (v/v). At this concentration, viability increased to 1.12-, 1.49-, 2.85-, and 8.9-fold compared to the control at 6, 12, 24, and 36 h of culture, respectively, which were much lower than that of the untreated group at the same time point. Inhibitory effect was correlated with the concentrations of DT. The growth of the Foc TR4 were almost entirely inhibited by 1/4,000 DT treated for 24 h and viability was 0.78-, 0.86-, and 1.02-fold that of the untreated group at 6, 12, and 24 h, respectively. In addition, with 1/2,000 DT, the viability of Foc TR4 conidia were decreased to 4% that of the untreated conidia at 6 h, and no conidia survived after 12 h incubation at this concentration.
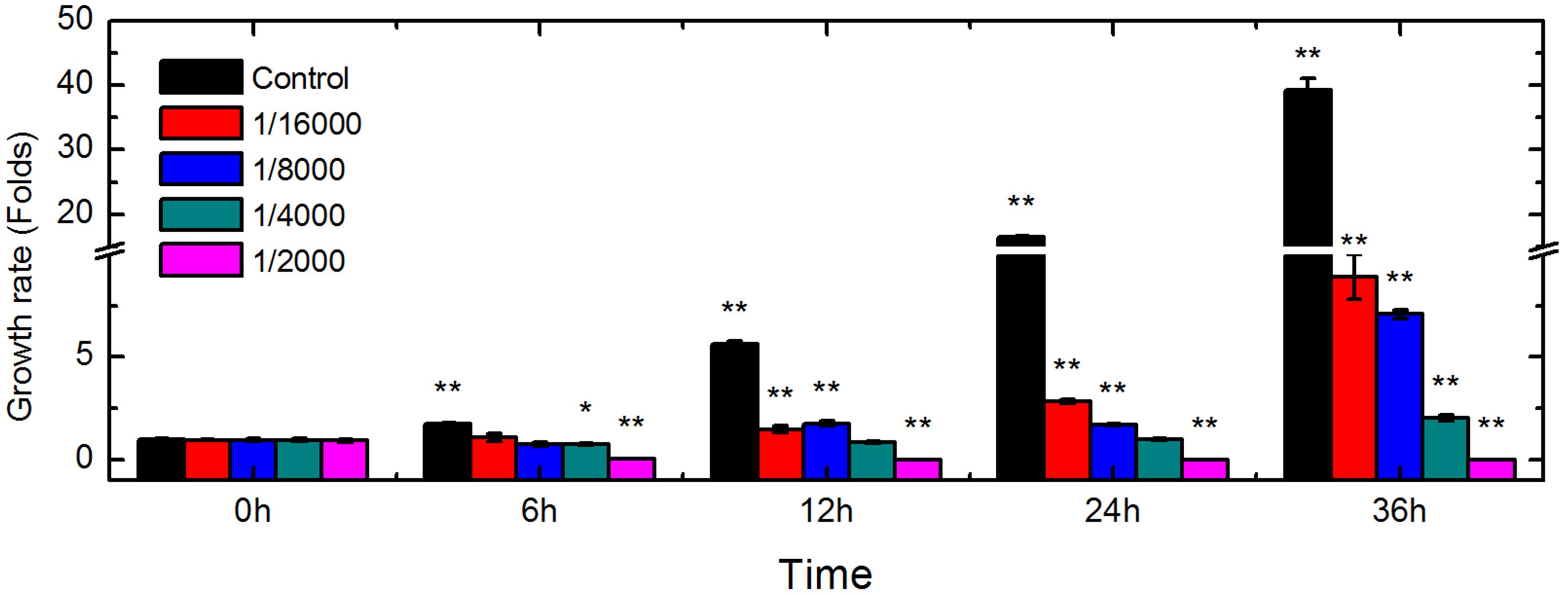
FIGURE 1. Changes in Foc TR4 viability induced by DT exposure. Conidia from control and treated isolates were incubated with FDA dye and their viability was assessed using a multifunctional microplate reader. Asterisks indicate a significant difference from the control, at ∗P < 0.05, and ∗∗P < 0.01.
Transcriptomic Profiles of Foc TR4 Influenced by DT
The global gene expression profile of Foc TR4 treated or untreated with DT for three different periods (1.5, 6, and 12 h) were examined using RNA-seq. The raw reads have been deposited at the Sequence Read Archive (SRA) dababase of NCBI (SRR5724804 to SRR5724810). After filtering, about 10 million clean reads were obtained from each sample, of which nearly 90% were mapped to the reference genome (Table 1). Gene mapping rate and number of expressed genes ranged from 43.03 to 73.12% and from 11,137 to 13,305, respectively (Table 1). At 1.5, 6, and 12 h, 2,556, 1,691, and 1,150 DEGs were found in the control group and 2,823, 3,546, and 6,197 DEGs were found in the treated group (FDR ≤ 0.01; log2 ratio ≥ 2 or ≤ -2). Most DEGs were down-regulated in both control and treated groups. The DEGs identified within each sample was used for functional classification and annotation (Figure 2). The detail of all DEGs were supplied at Supplementary Table S2.
GO Analysis: Functional Categorization of Identified DEGs
In total, 12, 6, and 7 GO terms were distributed into BP, MF, and CC, respectively (Figure 3). A large number DEGs (3,546) were found from 6-T, indicated the multiple responses was induced. However, enriched GO term was yet to be discovered. To understand the molecular mechanism underlying DT toxicity, the early responses of Foc TR4 to this compound were first investigated. At an early stage (1.5 h), the categories “small molecule catabolic process (GO: 0044282)”, “single-organism carbohydrate process (GO: 0044724)”, “organic acid catabolic process (GO: 0016054)”, “cellular amino acid catabolic process (GO: 0009063)”, and “oxacid metabolic process (GO: 0043436)” belonging to BP were clearly enriched in the control group (1.5-C). However, the categories “glycosylation (GO: 0070085)” and “glycoprotein metabolic process (GO: 0009100)” belonging to BP, “FMN binding (GO: 0010181)” and “transferase activity, transferring bexosyl groups (GO: 0016758)” belonging to MF, and “ER (GO: 0005783)” belonging to CC were considerably affected in the treated group (1.5-T). At later stages, the categories “ribosome biogenesis (GO: 0042254)” within BP, and “preribosome, large-subunit precursor (GO: 0030687)” and “nucleolus (GO: 0005730)” within CC were enriched in the control group (6-C and 12-C). In contrast, multiple categories such as “vesicle-mediated transport (GO: 0016192)” and “mitochondrion (GO: 0005739)” within CC were significantly enriched 12 h after DT treatment.
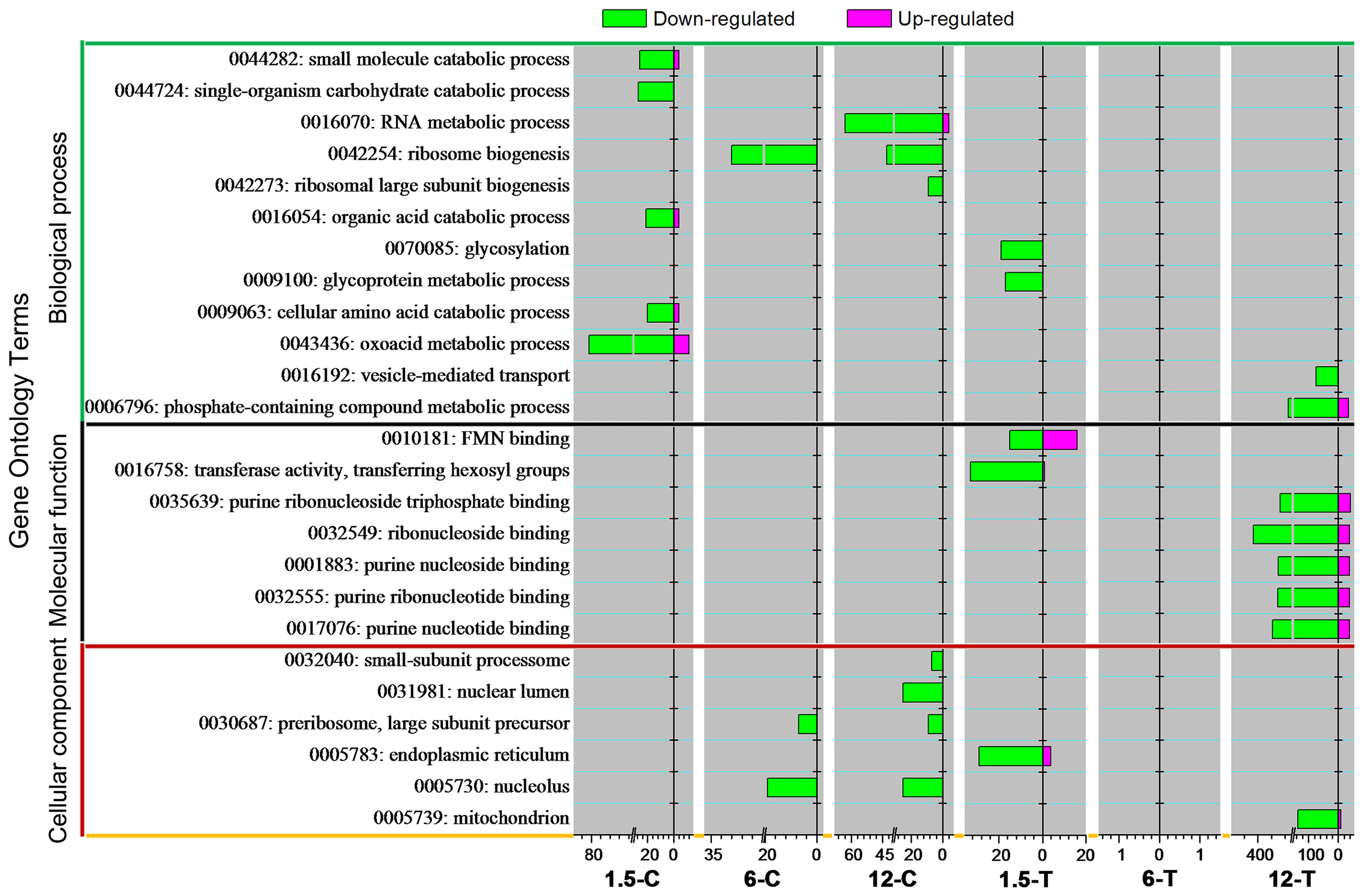
FIGURE 3. Gene Ontology classification analysis of DEGs. GO terms for which the false discovery rate (FDR) value was lower than 0.05 are listed.
Pathway Enrichment Analysis of DEGs
To investigate the biological pathways involved in the mechanism of DT toxicity to Foc TR4, the six most enriched DEGs pathways from each sample were examined (Figure 4). At 1.5 h, the DEGs involved in “RNA degradation (ko03018)”, “carbon metabolism (ko01200)”, “metabolic pathways (ko01100)”, and “tyrosine metabolism (ko00350)” were specifically enriched in the control group, while “cell cycle-yeast (ko04111)”, “mRNA surveillance pathway (ko03015)”, “glycerophospholipid metabolism (ko02564)”, and “other types of O-glycan biosynthesis (ko00514)” were specifically increased in the DT-treated group. At 12 h, multiple pathways were modified in both the control and treated groups. However, “ribosome biogenesis in eukaryotes (ko03008)” was significantly enriched in the control group, while pathways such as “endocytosis (ko04144)”, “cell cycle-yeast (ko04111)”, “RNA degradation (ko03018)”, “aminocyl-tRNA biosynthesis (ko00970)”, and “oxidative phosphorylation (ko00190)” were enriched in the DT-treated group. Moreover, “ribosome biogenesis in eukaryotes”, “tyrosine metabolism”, and “steroid biosynthesis” were specifically enriched at two time points in the control group. Cell cycle-yeast (ko04111) was discovered from top six enriched pathways in 1.5-T and 12-T but not in 6-T. However, a large number of DEGs (97) involved in this pathway were detected from 6-T (data not shown). That indicate genes involved in cell cycle-yeast of Foc TR4 were strongly influenced by DT exposure.
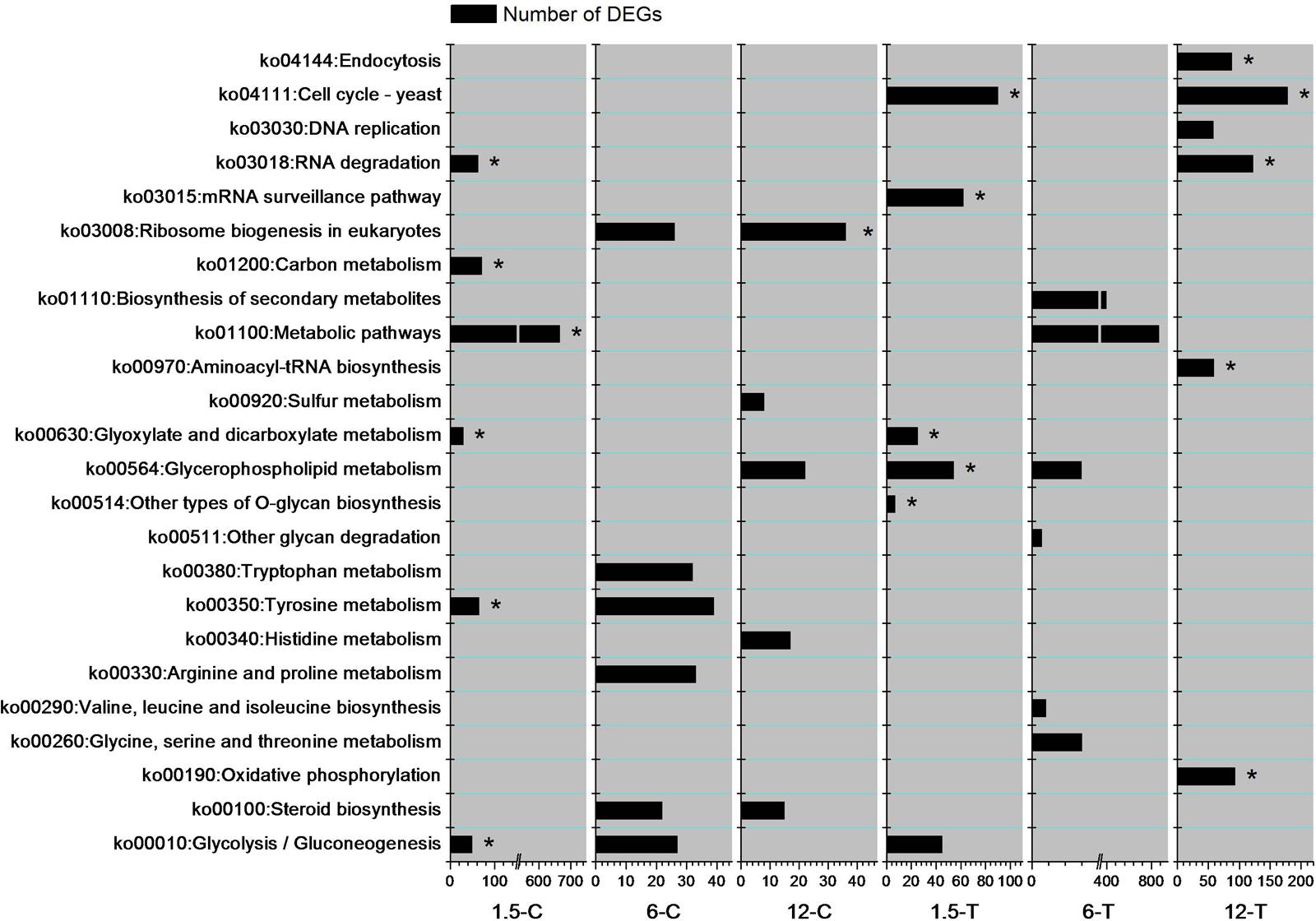
FIGURE 4. The six most enriched DEGs pathways in each sample. Asterisks indicate the pathways for which the FDR value was lower than 0.05.
Down-Regulation of DEGs Related to ER Following DT Exposure
A comprehensive illustration of DEGs related to the ER in Foc TR4, which were influenced by DT exposure, is presented in Figure 5. In total, 69 genes were differentially expressed in at least one of the samples. In the control groups, 2 out of 10, 9 out of 11, and 4 out of 5 genes were up-regulated in 1.5-C, 6-C, and 12-C, respectively, whereas 34, 23, and 49 DEGs were identified in1.5-T, 6-T, and 12-T treated groups, respectively, most of them down-regulated. Notably, a large number of DEGs found in treated groups were obviously down-regulated at the early stage of DT exposure. These included 3-ketoacyl-CoA reductase (FOIG_04985), Acetyl-CoA carboxylase (FOIG_05398), C-5 sterol desaturase (FOIG_08856), protein glycosyltransferase (FOIG_11050 and FOIG_02987), farnesyl pyrophosphate synthase (FOIG_05767), GPI mannosyltransferase (FOIG_06138 and FOIG_05129), increased recombination centers protein 22-2 (FOIG_02722), L-asparaginase (FOIG_09461), microsomal signal peptidase subunit 3 (FOIG_05783), NADPH-ferrihemoprotein reductase (FOIG_16063), oligosaccharyl transferase complex subunit OST4 (FOIG_01070), signal peptidase complex (FOIG_03977 and FOIG_05064), phosphotransferase (FOIG_05811), and vacuolar transporter chaperone 1 (FOIG_07033) (Figure 5).
Repression of DEGs Involved in Steroid Biosynthesis Following DT Exposure
The expression of the genes involved in steroid biosynthesis was considerably repressed following DT exposure (Figure 6). These genes encoded several enzymes, including EC 1.14.13.132 (FOIG_08157), CYP51G1 or EC 1.14.13.70 (FOIG_04112, FOIG_00890, FOIG_01800 and FOIG_12542), and EC 1.1.1.170 (FOIG_06501 and FOIG_02633). Moreover, the expression of genes encoding enzymes involved in ergosterol biosynthesis, such as ERG 6 (FOIG_12358, FOIG_07540), ERG 2 (FOIG_02847), ERG 3 (FOIG_8856), and ERG 5 (FOIG_13497), was clearly reduced following DT exposure.
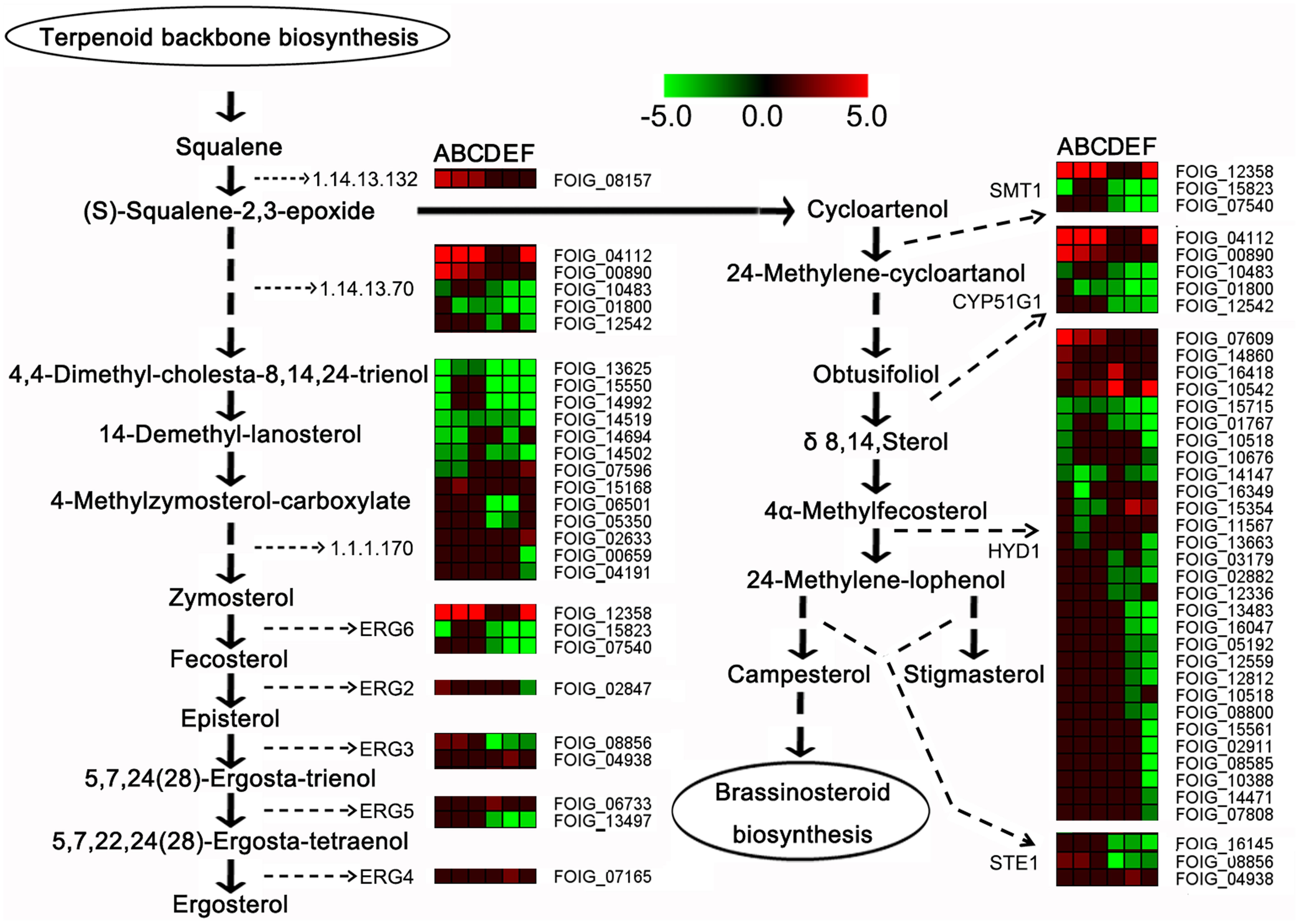
FIGURE 6. Expression profiles of steroid biosynthesis-related genes. In the heatmap, (A–C) represent 1.5-C, 6-C, and 12-C (controls after 1.5, 6, and 12 h of incubation), and (D–F) represent 1.5-T, 6-T, and 12-T (treated samples after 1.5, 6, and 12 h of DT exposure), respectively.
Verification of DEGs Using qRT-PCR
To confirm the accuracy of the RNA-seq results, the expression patterns of 15 representative DEGs involved in enriched GO terms or pathways were verified by qRT-PCR assay (Figure 7). These included DEGs involved in steroid biosynthesis (FOIG_07540, FOIG_08157, FOIG_12358, and FOIG_15550), and related to the ER (FOIG_05398, FOIG_08856, FOIG_14848, and FOIG_16063). The changes observed in trends were similar between RNA-seq and qRT-PCR assays, although several genes presented different expression at the same time point. This indicated the reliability of the RNA-seq data.
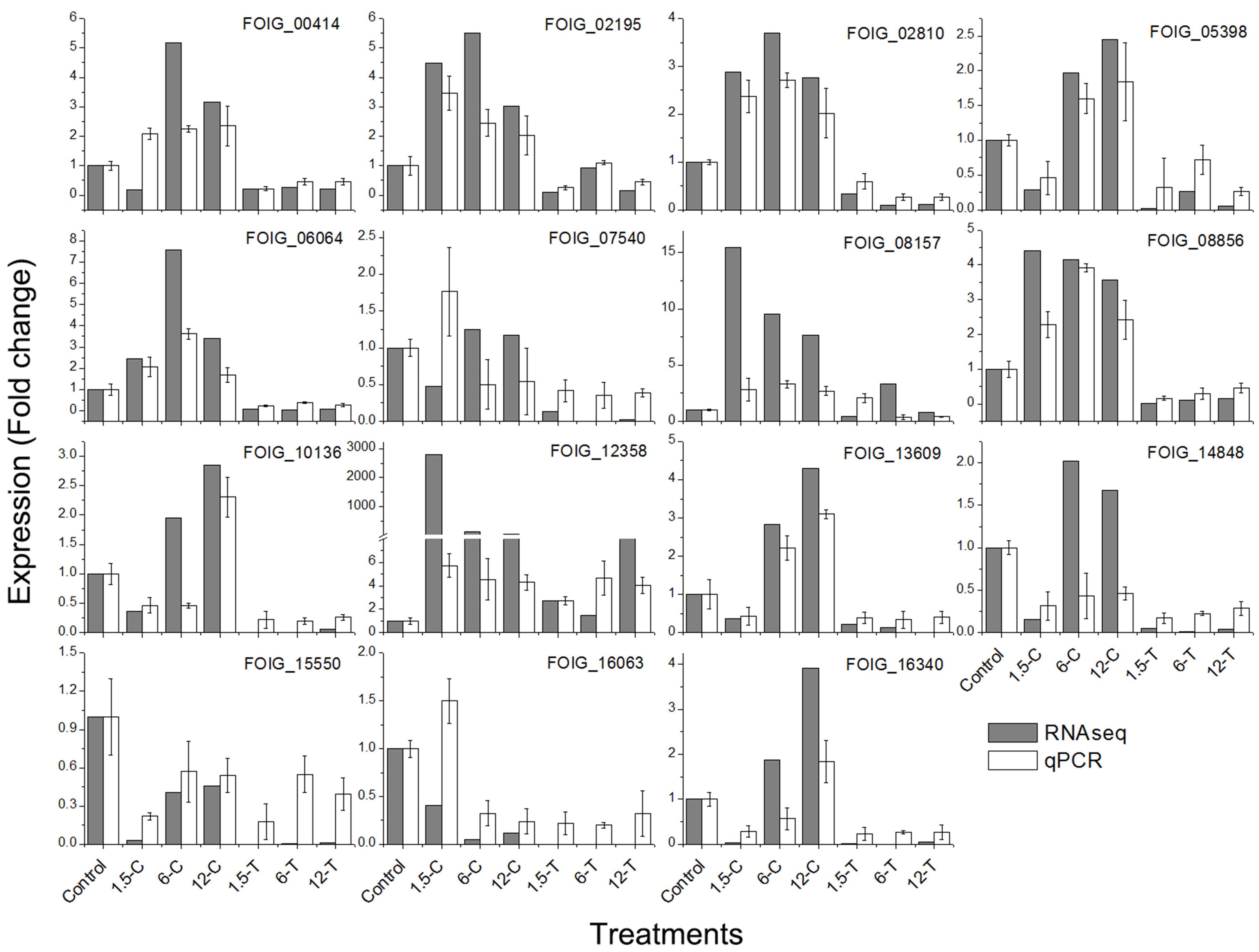
FIGURE 7. Quantitative real-time PCR validation of candidate genes in enriched GO terms and pathways. Genes involved in: endocytosis, FOIG_02195, FOIG_00414, FOIG_13609, and FOIG_02810; oxidative phosphorylation, FOIG_06064; steroid biosynthesis, FOIG_07540, FOIG_08157, FOIG_12358, andFOIG_15550; and ER, FOIG_05398, FOIG_08856, FOIG_14848, and FOIG_16063.
Discussion
The aim of this study was to examine the toxicity of DT against Foc TR4 and the molecular mechanisms underlying it; our results confirmed that the antifungal activity of DT against this pathogen and showed the dynamic gene expression profiles of the Foc TR4 response to DT. The GO and KEGG analysis indicated that the DEGs involved in ER and steroid biosynthesis were significantly enriched following DT exposure. At a later stage of DT exposure, DEGs associated with preribosome, endocytosis, RNA degradation, and oxidative phosphorylation were also notably influenced by DT. To the best of our knowledge, this is the first investigation of global gene expressions of Foc TR4 in response to DT exposure.
ER Stress Was Induced in Foc TR4 at an Early Stage of DT Exposure
The ER is not only responsible for the synthesis and folding of most secretory and transmembrane proteins, but also perceives and responds to cellular biotic and abiotic stresses, i.e., to ER stress (Zhang and Kaufman, 2008). In multiple fungal and mammalian cells, ER stress is induced by several compounds, such as cisplatin and cadmium, and could be considered as a target for the toxicity of those compounds (Mandic et al., 2003; Gardarin et al., 2010). In the present study, the DEGs involved in ER were significantly enriched from 1.5-T onward, and most of these were down-regulated (Figures 3, 5). The expression of four genes, namely FOIG_05398, FOIG_08856, FOIG_14848, and FOIG_16063, was also validated by qRT-PCR (Figure 7), suggesting that ER stress is induced in Foc TR4 at an early stage of DT exposure.
In general, ER stress is characterized by an accumulation of unfolded proteins in the ER, which results in the unfold protein response (UPR) (Zhang and Kaufman, 2008). This can be induced by the inhibition of glycosylation, rapid generation of ROS and perturbation of calcium metabolism (Yokouchi et al., 2008; Gardarin et al., 2010). Glycosylation, a post-translational modification of proteins on the ER or ribosome, plays important roles in many cellular events, both structural and signal recognition-related (Hart, 1992). In fungi, glycosylation is involved in the synthesis of the cell wall, cryptococcal capsule, glycoproteins, and glycolipids (Klutts et al., 2006). The expression of Foc TR4 genes involved in glycosylation and in glycoproteins metabolic processes was significantly reduced following DT treatment (Figure 3, and Supplementary Table S3). A rapid ROS burst was also reported from Foc TR4 treated with Chinese leek root exudates (Zuo et al., 2015). However, a reduction in the expression of genes related to calcium influx, such as calnexin like protein (FOIG_01078), were enriched in at the later stage of DT exposure (Figure 5). Therefore, ER stress in Foc TR4 resulting from DT exposure might be related to glycosylation inhibition and ROS accumulation, but it is likely not involve the perturbation of calcium influx homeostasis.
Under normal conditions, ER stress triggers vital responses for cell survival, including autophagy, RNA degradation, and apoptosis (Bonilla et al., 2002; Tabas and Ron, 2011; Hetz, 2012). Cell death under ER stress depends on the core mitochondrial apoptosis pathway (Hetz, 2012). At the later stage of DT exposure, DEGs involved in endocytosis, RNA degradation, and oxidative phosphorylation were enriched (Figure 4). As evidenced by the expression profiles of these DEGs, most of them were down-regulated in 12-T but not differentially expressed in other samples (Supplementary Table S3). This is consistent with the reduction in the mitochondrial transmembrane potential of Foc TR4 induced by Chinese leek root exudates (Zuo et al., 2015). Therefore, mitochondrial damage in Foc TR4, probably resulting from ER stress, was induced at a later stage of DT exposure. These results indicate that ER stress of Foc TR4 results in the activation of multiple stress responses, such as autophagy, RNA degradation, and mitochondrial damage.
DT Might Affect Foc TR4 Cell Membrane Integrity
In addition to ER, several target sites, such as the cell wall, fungal sterols, and nucleic acids, have been proposed to account for the activity of antifungal compounds (Ghannoum and Rice, 1999). Plant essential oils or their volatile compositions have strong antifungal activity against Penicillium digitatum, P. italicum, and Galactomyces citriaurantii (Droby et al., 2008; Wuryatmo et al., 2003, 2014). The cell membrane, mitochondrion, and genetic material have also been considered major targets of volatile compound toxicity (Bakkali et al., 2008; Shao et al., 2013). Sterol and glycerophospholipids are important cellular or subcellular membrane components and are required for cell growth and proliferation, maintenance of organelle morphology, signal transduction, and lipid homeostasis (Kihara and Igarashi, 2004; Nebauer et al., 2004). Ergosterol is one of the principal sterol components in the fungal cell membrane; blocking ergosterol biosynthesis usually leads to the disruption of cell structure and function, and even to cell death (OuYang et al., 2016). Our results demonstrated that the DEGs involved in glycerophospholipids metabolism and steroid biosynthesis were highly enriched in 6-C and 12-C (Figures 4, 6). Among these, the relative expression of FOIG_07540, FOIG_08157, FOIG_12358, and FOIG_15550 was verified by qRT-PCR (Figure 7). Notably, the genes encoding several key enzymes responsible for ergosterol biosynthesis, including ERG3, ERG5, ERG2, ERG6, and CYP51G1 (ERG11) exhibited much lower expression levels in the treated group than in the control group (Figure 7). A previous study confirmed that the ergosterol biosynthesis pathway is essential for Foc TR4 conidial germination and that it could be considered an important target for FWB control (Deng et al., 2015). Reduced expression of the genes involved in ergosterol biosynthesis usually results in a decrease in ergosterol content in cells, causing osmotic disturbances and the disruption of cell growth and proliferation (Khan et al., 2010; Sun et al., 2011). Regarding the considerable influence of DT exposure on the expression of Foc TR4 genes involved in steroid and glycerophospholipids metabolism, we suggest that damage to cell membrane integrity might be a critical reason for the toxicity of DT against Foc TR4.
Conclusion
We present a possible mechanism for the toxicity of DT against Foc TR4 (Figure 8). ER stress of Foc TR4, which is related to glycosylation and ROS accumulation, was induced at the early stage of DT exposure; this also caused multiple toxic responses such as RNA degradation, autophagy, and mitochondrial damage. In addition, clear inhibition of the genes involved in steroid biosynthesis and glycerophospholipids metabolism indicated that cell membrane integrity might be disrupted in Foc TR4 in response to DT exposure. These data provide a comprehensive high-resolution analysis of the gene expression shifts associated with DT exposure and suggest a new approach for the control of FWB.
Author Contributions
YH, BC, and CZ conceived the study and participated in its design and coordination. CZ and WZ performed the experiments, collected, analyzed, and deposited the data and prepared the draft. CZ and ZC proofread the final draft and revised the manuscript. All authors have read and approved the manuscript.
Funding
This work was supported by the Natural Science Foundation of China No. 31471864, 31501728, and 31272151.
Conflict of Interest Statement
The authors declare that the research was conducted in the absence of any commercial or financial relationships that could be construed as a potential conflict of interest.
Acknowledgments
We would like to thank Dr. Lijun Bai (GeneBang Inc., Chengdu, China, www.genebang.com) for technical assistance with RNA sequencing and bioinformatic analysis.
Abbreviations
BP, Biological process; CC, Cellular component; DEGs, Differentially expressed genes; DT, Dimethyl trisulfide; ER, Endoplasmic reticulum; FDA, Fluorescein diacetate; Foc, Fusarium oxysporum f. sp. Cubense; Foc TR4, Fusarium oxysporum f. sp. cubense tropical race 4; FWB, Fusarium wilt of banana; GO, Gene ontology; KEGG, Kyoto Encyclopedia of Genes and Genomes; MF, Molecular function; PBS, phosphate buffer solution; PDB, potato dextrose broth; qRT-PCR, Quantitative real-time PCR; RNA-seq, RNA-sequencing; ROS, Reactive oxygen species; VCGs, Vegetative compatibility groups.
Supplementary Material
The Supplementary Material for this article can be found online at: http://journal.frontiersin.org/article/10.3389/fmicb.2017.01365/full#supplementary-material
TABLE S1 | Primers used for qRT-PCR.
TABLE S2 | Expression profiles of the DEGs related to oxidative phosphorylation, endocytosis, and RNA degradation.
TABLE S3 | Significantly DEGs in control and DT-treated groups.
Footnotes
References
Aiyaz, M., Thimmappa Divakara, S., Mudili, V., George Moore, G., Kumar Gupta, V., Chandra Nayaka, S., et al. (2016). Molecular diversity of seed-borne Fusarium species associated with maize in India. Curr. Genom. 17, 132–144. doi: 10.2174/1389202917666151116213056
Alcazar-Fuoli, L., and Mellado, E. (2013). Ergosterol biosynthesis in Aspergillus fumigatus: its relevance as an antifungal target and role in antifungal drug resistance. Front. Microbiol. 3:439. doi: 10.3389/fmicb.2012.00439
Asha, B. B., Nayaka, C. S., Shankar, U. A., Srinivas, C., and Niranjana, S. R. (2011a). Biological control of F. oxysporum f. sp. lycopersici causing wilt of tomato by Pseudomonas fluorescens. Int. J. Microbiol. Res. 3, 79–84. doi: 10.9735/0975-5276.3.2.79-84
Asha, B. B., Nayaka, S. C., Shankar, A. C., Srinivas, C., and Niranjana, S. R. (2011b). Selection of effective bio-antagonistic bacteria for biological control of tomato wilt caused by Fusarium oxysporum f. sp. lycopersici. Bioscan Int. Quart. J. Life Sci. 6, 239–244.
Audic, S., and Claverie, J. M. (1997). The significance of digital gene expression profiles. Genome Res. 7, 986–995. doi: 10.1101/gr.7.10.986
Bakkali, F., Averbeck, S., Averbeck, D., and Idaomar, M. (2008). Biological effects of essential oils–a review. Food Chem. Toxicol. 46, 446–475. doi: 10.1016/j.fct.2007.09.106
Behr, J. B. (2011). Chitin synthase, a fungal glycosyltransferase that is a valuable antifungal target. Chimia 65, 49–53. doi: 10.2533/chimia.2011.49
Bonilla, M., Nastase, K. K., and Cunningham, K. W. (2002). Essential role of calcineurin in response to endoplasmic reticulum stress. EMBO J. 21, 2343–2353. doi: 10.1093/emboj/21.10.2343
Butt, T. M., Jackson, C., and Magan, N. (eds) (2001). Fungi as biocontrol agents: progress problems and potential. CABI 51, 70–71. doi: 10.1017/S0269-915X(03)23217-1
Cao, L., Qiu, Z., You, J., Tan, H., and Zhou, S. (2005). Isolation and characterization of endophytic streptomycete antagonists of Fusarium wilt pathogen from surface-sterilized banana roots. FEMS Microbiol. Lett. 247, 147–152. doi: 10.1016/j.femsle.2005.05.006
Coleman, J. J., Ghosh, S., Okoli, I., and Mylonakis, E. (2011). Antifungal activity of microbial secondary metabolites. PLoS ONE 6:e25321. doi: 10.1371/journal.pone.0025321
Cools, H. J., and Hammond-Kosack, K. E. (2013). Exploitation of genomics in fungicide research: current status and future perspectives. Mol. Plant Pathol. 14, 197–210. doi: 10.1111/mpp.12001
Das, A., Venkataramana, M., Chandranayaka, S., Murali, H. S., and Batra, H. V. (2012). Molecular characterization of Fusarium oxysporum f. sp. cubense isolates from banana. Pest Manag. Hortic. Ecosyst. 18, 171–178.
De Backer, M. D., and Van Dijck, P. (2003). Progress in functional genomics approaches to antifungal drug target discovery. Trends Microbiol. 11, 470–478. doi: 10.1016/j.tim.2003.08.008
Dean, R., Van Kan, J. A., Pretorius, Z. A., Hammond-Kosack, K. E., Di Pietro, A., Spanu, P. D., et al. (2012). The top 10 fungal pathogens in molecular plant pathology. Mol. Plant Pathol. 13, 414–430. doi: 10.1111/j.1364-3703.2011.00783.x
Deng, G. M., Yang, Q. S., He, W. D., Li, C. Y., Yang, J., Zuo, C. W., et al. (2015). Proteomic analysis of conidia germination in Fusarium oxysporum f. sp. cubense tropical race 4 reveals new targets in ergosterol biosynthesis pathway for controlling Fusarium wilt of banana. Appl. Microbiol. Biotechnol. 99, 7189–7207. doi: 10.1007/s00253-015-6768-x
Droby, S., Eick, A., Macarisin, D., Cohen, L., Rafael, G., Stange, R., et al. (2008). Role of citrus volatiles in host recognition, germination and growth of Penicillium digitatum and Penicillium italicum. Postharvest Biol. Technol. 49, 386–396. doi: 10.1016/j.postharvbio.2008.01.016
Fourie, G., Steenkamp, E. T., Gordon, T. R., and Viljoen, A. (2009). Evolutionary relationships among the Fusarium oxysporum f. sp. cubense vegetative compatibility groups. Appl. Environ. Microbiol. 75, 4770–4781. doi: 10.1128/AEM.00370-09
Fravel, D., Olivain, C., and Alabouvette, C. (2003). Fusarium oxysporum and its biocontrol. New Phytol. 157, 493–502. doi: 10.1046/j.1469-8137.2003.00700.x
García-Bastidas, F., Ordóñez, N., Konkol, J., Al-Qasim, M., Naser, Z., Abdelwali, M., et al. (2014). First report of Fusarium oxysporum f. sp. cubense tropical race 4 associated with Panama disease of banana outside Southeast Asia. Plant Dis. 98, 694–694. doi: 10.1094/PDIS-09-13-0954-PDN
Gardarin, A., Chédin, S., Lagniel, G., Aude, J. C., Godat, E., Catty, P., et al. (2010). Endoplasmic reticulum is a major target of cadmium toxicity in yeast. Mol. Microbiol. 76, 1034–1048. doi: 10.1111/j.1365-2958.2010.07166.x
Ghannoum, M. A., and Rice, L. B. (1999). Antifungal agents: mode of action, mechanisms of resistance, and correlation of these mechanisms with bacterial resistance. Clin. Microbiol. Rev. 12, 501–517.
Hart, G. W. (1992). Glycosylation. Curr. Opin. Cell Biol. 4, 1017–1023. doi: 10.1016/0955-0674(92)90134-X
Hetz, C. (2012). The unfolded protein response: controlling cell fate decisions under ER stress and beyond. Nat. Rev. Mol. Cell Biol. 13, 89–102. doi: 10.1038/nrm3270
Ho, Y. N., Chiang, H. M., Chao, C. P., Su, C. C., Hsu, H. F., Guo, C. T., et al. (2015). In planta biocontrol of soilborne Fusarium wilt of banana through a plant endophytic bacterium, Burkholderia cenocepacia 869T2. Plant Soil 387, 295–306. doi: 10.1007/s11104-014-2297-0
Huang, Y. H., Mao, Z. C., and Xie, B. Y. (2016). Chinese leek (Allium tuberosum Rottler ex Sprengel) reduced disease symptom caused by root-knot nematode. J. Integr. Agric. 15, 364–372. doi: 10.1016/S2095-3119(15)61032-2
Huang, Y. H., Wang, R. C., Li, C. H., Zuo, C. W., Wei, Y. R., Zhang, L., et al. (2012). Control of Fusarium wilt in banana with Chinese leek. Eur. J. Plant Pathol. 134, 87–95. doi: 10.1007/s10658-012-0024-3
Hwang, S. C., and Ko, W. H. (2004). Cavendish banana cultivars resistant to Fusarium wilt acquired through somaclonal variation in Taiwan. Plant Dis. 88, 580–588. doi: 10.1094/PDIS.2004.88.6.580
Irkin, R., and Korukluoglu, M. (2007). Control of Aspergillus niger with garlic, onion and leek extracts. Afr. J. Biotechnol. 6, 384–387. doi: 10.5897/AJB2007.000-2018
Kanehisa, M., Araki, M., Goto, S., Hattori, M., Hirakawa, M., Itoh, M., et al. (2008). KEGG for linking genomes to life and the environment. Nucleic Acids Res. 36(Suppl. 1), D480–D484. doi: 10.1093/nar/gkm882
Khan, A., Ahmad, A., Akhtar, F., Yousuf, S., Xess, I., Khan, L. A., et al. (2010). Ocimum sanctum essential oil and its active principles exert their antifungal activity by disrupting ergosterol biosynthesis and membrane integrity. Res. Microbiol. 161, 816–823. doi: 10.1016/j.resmic.2010.09.008
Kihara, A., and Igarashi, Y. (2004). Cross talk between sphingolipids and glycerophospholipids in the establishment of plasma membrane asymmetry. Mol. Biol. Cell 15, 4949–4959. doi: 10.1091/mbc.E04-06-0458
Klutts, J. S., Yoneda, A., Reilly, M. C., Bose, I., and Doering, T. L. (2006). Glycosyltransferases and their products: cryptococcal variations on fungal themes. FEMS Yeast Res. 6, 499–512. doi: 10.1111/j.1567-1364.2006.00054.x
Lee, C. F., Han, C. K., and Tsau, J. L. (2004). In vitro inhibitory activity of Chinese leek extract against Campylobacter species. Int. J. Food Microbiol. 94, 169–174. doi: 10.1016/j.ijfoodmicro.2004.01.009
Li, B., and Dewey, C. N. (2011). RSEM: accurate transcript quantification from RNA-Seq data with or without a reference genome. BMC Bioinformatics 12:323. doi: 10.1186/1471-2105-12-323
Li, C. Y., Deng, G. M., Yang, J., Viljoen, A., Jin, Y., Kuang, R. B., et al. (2012). Transcriptome profiling of resistant and susceptible Cavendish banana roots following inoculation with Fusarium oxysporum f. sp. cubense tropical race 4. BMC Genomics 13:374. doi: 10.1186/1471-2164-13-374
López-Berges, M. S., Rispail, N., Prados-Rosales, R. C., and Di Pietro, A. (2010). A nitrogen response pathway regulates virulence functions in Fusarium oxysporum via the protein kinase TOR and the bZIP protein MeaB. Plant Cell 22, 2459–2475. doi: 10.1105/tpc.110.075937
Ma, Z., and Michailides, T. J. (2005). Advances in understanding molecular mechanisms of fungicide resistance and molecular detection of resistant genotypes in phytopathogenic fungi. Crop Prot. 24, 853–863. doi: 10.1016/j.cropro.2005.01.011
Mandic, A., Hansson, J., Linder, S., and Shoshan, M. C. (2003). Cisplatin induces endoplasmic reticulum stress and nucleus-independent apoptotic signaling. J. Biol. Chem. 278, 9100–9106. doi: 10.1074/jbc.M210284200
Nebauer, R., Birner-Grünberger, R., and Daum, G. (2004). “3 Biogenesis and cellular dynamics of glycerophospholipids in the yeast Saccharomyces cerevisiae,” in Lipid Metabolism and Membrane Biogenesis. Topics in Current Genetics, Vol. 6, ed. G. Daum (Berlin: Springer), 125–168. doi: 10.1007/978-3-540-40999-1_4
Nirmaladevi, D., Venkataramana, M., Srivastava, R. K., Uppalapati, S. R., Gupta, V. K., Yli-Mattila, T., et al. (2016). Molecular phylogeny, pathogenicity and toxigenicity of Fusarium oxysporum f. sp. lycopersici. Sci. Rep. 6:21367. doi: 10.1038/srep21367
Ordonez, N., García-Bastidas, F., Laghari, H. B., Akkary, M. Y., Harfouche, E. N., Al Awar, B. N., et al. (2015). First report of Fusarium oxysporum f. sp. cubense tropical race 4 causing Panama disease in Cavendish bananas in Pakistan and Lebanon. Plant Dis. 100, 209. doi: 10.1094/PDIS-12-14-1356-PDN
OuYang, Q., Tao, N., and Jing, G. (2016). Transcriptional profiling analysis of Penicillium digitatum, the causal agent of citrus green mold, unravels an inhibited ergosterol biosynthesis pathway in response to citral. BMC Genomics 17:599. doi: 10.1186/s12864-016-2943-4
Paiva, P. M. G., Gomes, F. S., Napoleão, T. H., Sá, R. A., Correia M. T. S., and Coelho, L. C. B. B. (2010). Antimicrobial activity of secondary metabolites and lectins from plants. Curr. Res. Technol. Educ. Top. Appl. Microbiol. Microb. Biotechnol. 1, 396–406.
Parveen, M., Hasan, M. K., Takahashi, J., Murata, Y., Kitagawa, E., Kodama, O., et al. (2004). Response of Saccharomyces cerevisiae to a monoterpene: evaluation of antifungal potential by DNA microarray analysis. J. Antimicrob. Chemother. 54, 46–55. doi: 10.1093/jac/dkh245
Ploetz, R. C. (2015). Fusarium wilt of banana. Phytopathology 105, 1512–1521. doi: 10.1094/PHYTO-04-15-0101-RVW
Ploetz, R. C., and Pegg, K. G. (1997). Fusarium wilt of banana and Wallace’s line: was the disease originally restricted to his Indo-Malayan region? Australas. Plant Pathol. 26, 239–249. doi: 10.1071/AP97039
Ploetz, R. C., and Pegg, K. G. (2000). “Fusarium wilt,” in Diseases of Banana, Abacá and Enset, ed. D. R. Jones (Wallingford: CABI Publishing), 143–159.
Postma, J., and Rattink, H. (1992). Biological control of Fusarium wilt of carnation with a nonpathogenic isolate of Fusarium oxysporum. Can. J. Bot. 70, 1199–1205. doi: 10.1139/b92-150
Raguchander, T., Jayashree, K., and Samiyappan, R. (1997). Management of Fusarium wilt of banana using antagonistic microorganisms. J. Biol. Control 11, 101–105. doi: 10.18311/jbc/1997/7590
Shao, X., Cheng, S., Wang, H., Yu, D., and Mungai, C. (2013). The possible mechanism of antifungal action of tea tree oil on Botrytis cinerea. J. Appl. Microbiol. 114, 1642–1649. doi: 10.1111/jam.12193
Sun, X. P., Wang, J. Y., Feng, D., Ma, Z. H., and Li, H. Y. (2011). PdCYP51B, a new putative sterol 14a–demethylase gene of Penicillium digitatum involved in resistance toimazalil and other fungicides inhibiting ergosterol synthesis. Appl. Microbiol. Biotechnol. 91, 1107–1119. doi: 10.1007/s00253-011-3355-7
Tabas, I., and Ron, D. (2011). Integrating the mechanisms of apoptosis induced by endoplasmic reticulum stress. Nat. Cell Biol. 13, 184–190. doi: 10.1038/ncb0311-184
Tolosa, L., Donato, M. T., and Gómez-Lechón, M. J. (2015). “General cytotoxicity assessment by means of the MTT assay,” in Protocols in In Vitro Hepatocyte Research, eds M. Vinken and V. Rogiers (New York, NY: Springer), 333–348. doi: 10.1007/978-1-4939-2074-7_26
Tsao, S. M., and Yin, M. C. (2001). In-vitro antimicrobial activity of four diallyl sulphides occurring naturally in garlic and Chinese leek oils. J. Med. Microbiol. 50, 646–649. doi: 10.1099/0022-1317-50-7-646
Walsh, T. J., Viviani, M. A., Arathoon, E., Chiou, C., Ghannoum, M., Groll, A. H., et al. (2010). New targets and delivery systems for antifungal therapy. Med. Mycol. 38(Suppl. 1), 335–347. doi: 10.1080/mmy.38.1.335.347
Wang, B., Yuan, J., Zhang, J., Shen, Z., Zhang, M., Li, R., et al. (2013). Effects of novel bioorganic fertilizer produced by Bacillus amyloliquefaciens W19 on antagonism of Fusarium wilt of banana. Biol. Fertil. Soils 49, 435–446. doi: 10.1007/s00374-012-0739-5
Wuryatmo, E., Able, A. J., Ford, C. M., and Scott, E. S. (2014). Effect of volatile citral on the development of blue mould, green mould and sour rot on navel orange. Austral. Plant Pathol. 43, 403–411. doi: 10.1007/s13313-014-0281-z
Wuryatmo, E., Klieber, A., and Scott, E. S. (2003). Inhibition of citrus postharvest pathogens by vapor of citral and related compounds in culture. J. Agric. Food Chem. 51, 2637–2640. doi: 10.1021/jf026183l
Ye, J., Fang, L., Zheng, H., Zhang, Y., Chen, J., Zhang, Z., et al. (2006). WEGO: a web tool for plotting GO annotations. Nucleic Acids Res. 34(Suppl. 2), W293–W297. doi: 10.1093/nar/gkl031
Yokouchi, M., Hiramatsu, N., Hayakawa, K., Okamura, M., Du, S., Kasai, A., et al. (2008). Involvement of selective reactive oxygen species upstream of proapoptotic branches of unfolded protein response. J. Biol. Chem. 283, 4252–4260. doi: 10.1074/jbc.M705951200
Yu, L., Guo, N., Yang, Y., Wu, X. P., Meng, R. Z., Fan, J. W., et al. (2010). Microarray analysis of p–anisaldehyde–induced transcriptome of Saccharomyces cerevisiae. J. Ind. Microbiol. Biot. 37, 313–322. doi: 10.1007/s10295-009-0676-y
Zhang, H., Mallik, A., and Zeng, R. S. (2013). Control of Panama disease of banana by rotating and intercropping with Chinese chive (Allium tuberosum Rottler): role of plant volatiles. J. Chem. Ecol. 39, 243–252. doi: 10.1007/s10886-013-0243-x
Zhang, K., and Kaufman, R. J. (2008). From endoplasmic-reticulum stress to the inflammatory response. Nature 454, 455–462. doi: 10.1038/nature07203
Keywords: Dimethyl trisulfide (DT), Fusarium oxysporum f. sp. cubense tropical race 4 (Foc TR4), target sites, endoplasmic reticulum (ER) stress, steroid biosynthesis
Citation: Zuo C, Zhang W, Chen Z, Chen B and Huang Y (2017) RNA Sequencing Reveals that Endoplasmic Reticulum Stress and Disruption of Membrane Integrity Underlie Dimethyl Trisulfide Toxicity against Fusarium oxysporum f. sp. cubense Tropical Race 4. Front. Microbiol. 8:1365. doi: 10.3389/fmicb.2017.01365
Received: 26 April 2017; Accepted: 05 July 2017;
Published: 24 July 2017.
Edited by:
Charley Christian Staats, Universidade Federal do Rio Grande do Sul, BrazilReviewed by:
Bjorn Sandrock, Philipps University of Marburg, GermanyChandra Nayak, University of Mysore, India
Guilherme Loss, Laboratório Nacional de Computação Científica, Brazil
Copyright © 2017 Zuo, Zhang, Chen, Chen and Huang. This is an open-access article distributed under the terms of the Creative Commons Attribution License (CC BY). The use, distribution or reproduction in other forums is permitted, provided the original author(s) or licensor are credited and that the original publication in this journal is cited, in accordance with accepted academic practice. No use, distribution or reproduction is permitted which does not comply with these terms.
*Correspondence: Baihong Chen, YmhjaEBnc2F1LmVkdS5jbg== Yonghong Huang, Z3N0c2hoQDEyNi5jb20=