- Co-Innovation Center for Sustainable Forestry in Southern China, Nanjing Forestry University, Nanjing, China
Potassium has an important role to play in multiple cellular processes. In Saccharomyces cerevisiae, the serine/threonine (S/T) kinase Sat4/Hal4 is required for potassium accumulation, and thus, regulates the resistance to sodium salts and helps in the stabilization of other plasma membrane transporters. However, the functions of Sat4 in filamentous phytopathogenic fungi are largely unknown. In this study, ChSat4, the yeast Sat4p homolog in Colletotrichum higginsianum, has been identified. Target deletion of ChSAT4 resulted in defects in mycelial growth and sporulation. Intracellular K+ accumulation was significantly decreased in the ChSAT4 deletion mutant. Additionally, the ΔChsat4 mutant showed defects in cell wall integrity, hyperoxide stress response, and pathogenicity. Localization pattern analysis indicated ChSat4 was localized in the cytoplasm. Furthermore, ChSat4 showed high functional conservation with the homolog FgSat4 in Fusarium graminearum. Taken together, our data indicated that ChSat4 was important for intracellular K+ accumulation and infection morphogenesis in C. higginsianum.
Introduction
Potassium is indispensable for multiple cellular processes, wherein it has important roles in the maintenance of the membrane potential and intracellular pH, and regulation of cellular enzyme activity (Kahm et al., 2012). Potassium is observed to be accumulated against its electrochemical gradient at a higher concentration intracellularly than that in the extracellular environment. In Saccharomyces cerevisiae, the uptake of potassium across the plasma membrane is driven by the membrane potential, which is generated by proton pumping via H+-ATPase (Rodriguez-Navarro, 2000; Buch-Pedersen et al., 2006). Genetic analyses revealed that potassium transport in S. cerevisiae is regulated by several proteins, including the high-affinity transporter proteins Trk1 and Trk2; the protein kinases Hal4, Hal5, and Sky1; the protein phosphatase Ppz1, Ppz2, and calcineurin; the G protein Arl1; and a protein of unknown function, Hal1 (Rodriguez-Navarro, 2000; Rotin et al., 2000; Wang et al., 2005; Pérez-Valle et al., 2007). In S. cerevisiae, SAT4 encodes a serine/threonine kinase, which positively regulates potassium uptake through a Trk1p-dependent manner (Mulet et al., 1999). This protein also contributes to the stabilization of the plasma membrane transporters, the control of carbon and nitrogen metabolism, and the regulation of the transcriptional activator Gln3 (Mulet et al., 1999; Pérez-Valle et al., 2010; Hirasaki et al., 2011). The deletion of SAT4 resulted in a remarkably lower cellular K+ concentration. Consequently, a sat4 mutant exhibited hypersensitivity to Na+, Li+, and Ca2+ (Mulet et al., 1999). In the filamentous fungus Fusarium graminearum, the deletion of FgSAT4 inhibited hyphal growth and sporulation, altered the normal conidial morphology, increased the sensitivity to NaCl, and decreased the pathogenicity to the host plant (Wang et al., 2011; Zheng et al., 2012). These observations indicated that SAT4 and its orthologs may share different functions and regulatory mechanisms in S. cerevisiae and phytopathogenic fungi.
Anthracnose caused by Colletotrichum spp. affects a wide range of commercial crops and plants worldwide (Waller, 1992; Nakamura et al., 2018). Colletotrichum higginsianum causes anthracnose and blight on several members of Brassicaceae, and causes significant economic losses (O’Connell et al., 2004). C. higginsianum employs a hemibiotrophic infection process, which initially establishes infection through a conidium that germinates and produces a germ tube that further develops into an appressorium, which ruptures the host cuticle, produces specialized primary biotrophic hyphae to invade the host cells, and finally differentiates into necrotrophic hyphae that destroy and kill the host tissues (O’Connell et al., 2004, 2012; Plaumann et al., 2018). Sat4 is involved in the regulation of potassium accumulation and stress resistance in S. cerevisiae. Although filamentous fungi have different lifestyles from that of yeast, the functions of SAT4 orthologs in phytopathogenic fungi are largely unknown.
In this study, a yeast S/T kinase Sat4 homolog ChSat4 was identified and characterized in C. higginsianum. We show that ChSat4 is not only involved in K+ accumulation, but is also important for hyphal growth, sporulation, cell wall integrity, and pathogenicity.
Materials and Methods
Fungal Strains and Culture
Colletotrichum higginsianum strain IMI349063 was used as the wild type for transformation. All strains were cultured in an incubator at 25°C. Modified Mathur’s medium, oat meal agar (OMA), minimal medium (MM), and potato dextrose agar (PDA) were used to analyze the vegetative growth of the fungal strains (Qi et al., 2012). Liquid complete medium (CM) was used to culture and harvest the fungal mycelia for genomic DNA extraction, and the protoplasts were prepared as described (Sweigard et al., 1992).
Nucleic Acid Manipulations, Southern Blotting, and Semiquantitative Reverse Transcription-Polymerase Chain Reaction (RT-PCR)
Fungal genomic DNA was extracted as described by Damm et al. (2008). Southern blotting was preformed according to the manufacturer’s instructions of a DIG High Prime DNA Labeling and Detection Starter Kit (Roche Applied Science, Penzberg, Germany). Total RNA was isolated using an RNA extraction kit (Invitrogen, Carlsbad, CA, United States). Semiquantitative RT-PCR was performed as described by Huang et al. (2017).
Targeted Gene Deletion and Complementation
The ChSAT4 gene replacement construct was generated using the standard one-step gene replacement strategy (Tang et al., 2015). The upstream and downstream flanking sequences of ChSAT4 were amplified using primer sets Ch10150_U_F/Ch10150_U_R and Ch10150_D_F/Ch10150_D_R, respectively (Supplementary Table S1). The resulting PCR products were digested with restriction endonucleases and ligated to the hygromycin phosphotransferase (HPH) cassette released from the pCX62 vector. After ligation, a 3.4-kb gene replacement fragment was amplified with a primer set Ch10150_U_F/Ch10150_D_R and transformed into the protoplasts of C. higginsianum wild type IMI349063 using the method of protoplasts preparation and transformation employed in F. graminearum as described by Li et al. (2018).
For complementation assays, a 2.2-kb fragment containing the whole ChSAT4 gene and its native promoter region was amplified with primers Ch10150_COM_F/R (Supplementary Table S1) and inserted into the vector pYF11 (Zhao et al., 2004). The resulting construct ChSAT4-GFP was verified by sequencing and transformed into the ΔChsat4 mutant.
To assay the functional conservation of Sat4 between C. higginsianum and F. graminearum, the construct ChSAT4-GFP was transformed into the ΔFgsat4 mutant to generate the ΔFgsat4/ChSAT4 strain. First, the ΔFgsat4 mutant was generated using the split marker approach with the primers FGSG_06939_F1/R2, HYGF/R, and FGSG_06939_F3/R4. After transformation of the wild type PH-1, hygromycin-resistant transformants were screened using PCR with primers sets FGSG_06939_InF/R and FGSG_06939_OuF/HPHCON_R2 (Supplementary Table S1). Second, the construct ChSAT4-GFP was transformed into protoplasts of the ΔFgsat4 mutant to generate the ΔFgsat4/ChSAT4 strains. The transformants resistant to zeomycin were screened using PCR with primer set FGSG_06939_InF/R and GFP signal examination.
Vegetative Growth, Sporulation, Stress Resistance Assays
Mycelial blocks of IMI349063, the ΔChsat4 mutant, and the complemented strain were inoculated onto CM, Mathur’s, PDA, and MM media in the dark at 25°C (Araújo et al., 2014). The diameters of the fungal colonies were measured after incubation for 5 days. The conidia of the wild type IMI349063, the ΔChsat4 mutant, and the complemented strain were induced in the CMC liquid as described by Araújo et al. (2014).
For stress resistance assays, mycelial blocks (5 × 5 mm) were inoculated onto Mathur’s agar plates containing H2O2 (5, 7.5, and 10 mM), NaCl (0.7 M), sodium dodecyl sulfate (SDS) (0.005%), Calcofluor white (CFW) (50 and 100 μg mL-1), and Congo red CR (200, 400, and 600 μg mL-1), respectively, and cultured at 25°C in the dark for 5 days (Guo et al., 2010). All experiments were carried out three times with three replicates.
Determination of the K+ Concentration in the Mycelia
The wild type strain IMI349063 and the ΔChsat4 strain were cultured in liquid CM and Mathur’s medium for 2 days, respectively. Mycelia were harvested and dried using a freeze drier and digested with a solution containing 98% H2SO4, and 30% H2O2 was added to restore the colorless, mycelial digestion solution. Next, the digestions were measured using a flame spectrophotometer (Almeida et al., 2015). The experiments were carried out three times with three replicates.
Plant Infection Assays
For pathogenicity assays, fresh mycelial mats of the wild type IMI349063, the ΔChsat4 mutant, and the complemented strain were inoculated onto unwounded leaves and petioles of Brassica rapa subsp. campestris (Lyu et al., 2016). The penetration abilities of the wild type IMI349063, the ΔChsat4 mutant, and the complemented strain were examined using the cellophane membrane technique (He et al., 2017). To quantify fungal biomass in planta, DNA was isolated from infected Brassica chinensis leaves inoculated by the wild type and the ΔChsat4 mutant at 3 days postinoculation (dpi), respectively. The quantitative PCR (qPCR) was used to examine the relative fungal biomass as described by Plaumann et al. (2018). These experiments were performed three times, with three replicates for each treatment.
For the pathogenicity assays of F. graminearum wild type PH-1, the ΔFgsat4 mutant, and the ΔFgsat4/ChSAT4 strain, blocks of PH-1, ΔFgsat4, and ΔFgsat4/ChSAT4 were inoculated into the liquid CMC medium to induce sporulation for 3 days. Then, conidia were collected and suspended in ddH2O at a concentration of 106 conidia/ml. Ten milliliters of conidial suspension were inoculated into tomato (Lycopersicon esculentum) fruits and incubated in a chamber at 25°C (Gao et al., 2016). In order to quantify fungal biomass during infection, DNA was isolated from infected tomato fruit inoculated by the wild type PH-1, the ΔFgsat4 mutant, and the transformant ΔFgsat4/ChSAT4 at 4 dpi. Relative fungal biomass was monitored using qPCR as described by Plaumann et al. (2018). These experiments were carried out three times with three replicates.
Light Microscopy and Data Analysis
The chitin deposited in the cell wall was observed using CFW (Sigma, St. Louis, MO, United States) staining as described by Huang et al. (2017). Photographs were taken using a Zeiss M2 microscope (Carl Zeiss, Germany). Statistical analyses were performed with the SPSS 19.0 software program (SPSS Inc., Chicago, IL, United States) using a one-way analysis of variance (ANOVA) (p < 0.01).
Results
Identification and Deletion of ChSAT4 in Colletotrichum higginsianum
Using the S. cerevisiae SAT4 sequence as the reference to search the C. higginsianum genome database, the CH063_10150 genetic loci encoding the SAT4 homolog ChSAT4 was identified. ChSat4 contains 490 amino acids sharing 52% sequence identity with Sat4. Phylogenetic analysis showed that the Sat4 proteins in filamentous fungi have diverged from those of unicellular yeasts, and ChSat4 of C. higginsianum and other Sat4 proteins from Colletotrichum formed a monophyletic lineage (Supplementary Figure S1A). This result indicates that the Sat4 proteins are conserved in fungi.
In order to investigate the function of ChSat4 in C. higginsianum, a gene deletion mutant ΔChsat4 was generated by replacing the ChSAT4 coding region with the hygromycin phosphotransferase resistance (HPH) gene. Results showed that the transcript of ChSAT4 was not detected in the ΔChsat4 mutant (Supplementary Figure S1B). Southern blotting analysis further confirmed that the target gene ChSAT4 was deleted and replaced by HPH gene in the ΔChsat4 mutant (Supplementary Figure S1C). For mutant complementation, ChSAT4-GFP fusion construct was introduced into the ΔChsat4 mutant to obtain the complementation strain ΔChsat4/SAT4 (Supplementary Figure S1B).
Roles of ChSat4 in Vegetative Growth and Asexual Development
To determine the role of ChSat4 in vegetative growth, the wild type IMI349063, the ΔChsat4 mutant, and the complemented strain ΔChsat4/SAT4 were cultured on CM, Mathur’s, PDA, and MM media plates. The ΔChsat4 mutant produced smaller colonies compared to the wild type and complemented strains (Figures 1A,B). In addition, the mycelial dry weight of the ΔChsat4 mutant was reduced compared to that of the wild type and the complemented strains (Figure 1C).
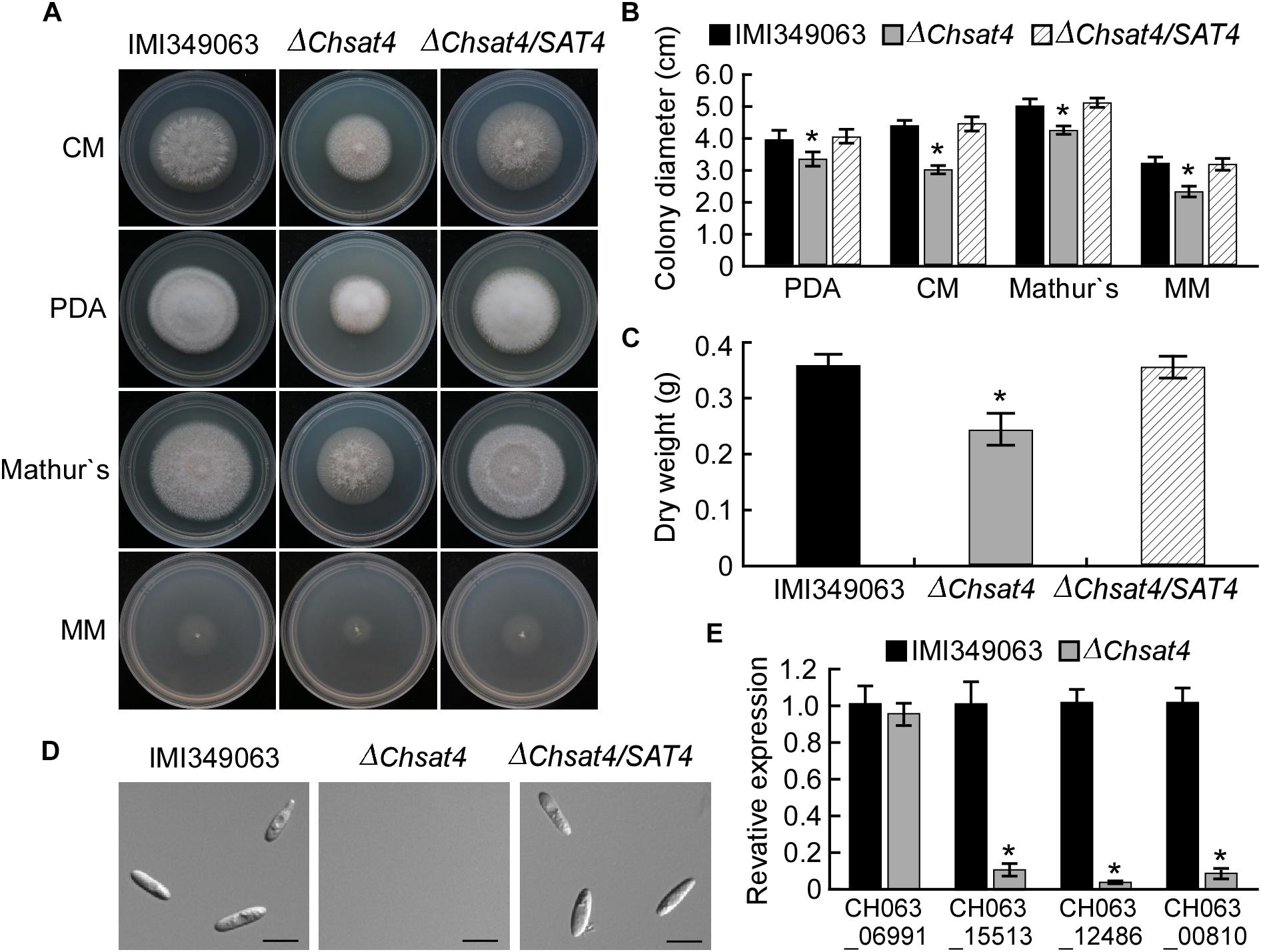
FIGURE 1. Effects of ChSat4 on vegetative mycelial growth and conidiation. (A) Colony morphology of the wild type IMI340963, ΔChsat4 mutant, and the complemented strain grown on CM, PDA, Mathur’s, and MM media plates for 5 days. (B) Colony diameter of IMI340963, ΔChsat4 mutant, and the complemented strain grown on CM, PDA, Mathur’s, and MM media plates for 5 days. (C) Dry weight of the mycelia from the wild type IMI340963, ΔChsat4 mutant, and the complemented strain. Error bars represent the SD from the means, and asterisks indicate statistically significant differences (p < 0.01). (D) Sporulation of IMI340963, ΔChsat4 mutant, and the complemented strain in CMC medium. Bars = 10 μm. (E) The expression levels of conidiation-related genes. The qRT-PCR was performed with the RNA extracted from mycelia of the wild type and ΔChsat4 mutant cultured in CM liquid medium for 2 days. Error bars represent the SD, and t-test analysis was shown with ∗p < 0.01 versus the wild type.
Asexual spores are important for the disease cycle of C. higginsianum. The sporulation abilities of ΔChsat4 mutant, the wild type IMI349063, and the complemented strain were assayed. The ΔChsat4 mutant did not produce any conidia in the CMC medium, while the wild type and complemented strains produced abundant conidia (Figure 1D). In Magnaporthe oryzae, the sporulation is regulated by some genes such as MoCON7, MoCOM1, MoAPS2 and MoACR1 (Kim and Lee, 2012; Huang et al., 2017). In order to further analyze the effect of deletion of ChSAT4 on the expression of conidiation-related genes in C. higginsianum, the expression level of homologous of these genes (CON7: CH063_06991; COM1: CH063_15513; APS2: CH063_12486; ACR1: CH063_00810) was examined using qRT-PCR. Results showed that the expression levels of these conidiation-related genes was significantly decreased except for the CON7 in the ΔChsat4 mutant compared to the wild type (Figure 1E). These results indicated that the ChSat4 was involved in regulating the vegetative growth and sporulation in C. higginsianum.
Contribution of ChSat4 to K+ Accumulation and NaCl Stress Resistance
Saccharomyces cerevisiae Sat4 was involved in the cellular K+ accumulation (Kahm et al., 2012). To investigate whether ChSat4 exhibits a similar function, the concentration of K+ in the mycelia of the wild type and the ΔChsat4 mutant were examined. In both media of CM and Mathur’s, the K+ concentration of the ΔChsat4 mutant was significantly decreased compared to that of the wild type (Figure 2A). The K+ accumulation may lead to a relatively low osmotic potential, which could cause sensitivity to extracellular osmotic stress. To confirm this hypothesis, the ΔChsat4 mutant was exposed to NaCl and the growth inhibition rates were calculated. The results showed that the growth of the ΔChsat4 mutant was significantly inhibited compared to that of the wild type and complemented strains (Figure 2B). These results indicated that ChSat4 was involved in the cellular K+ accumulation and osmotic stress resistance.
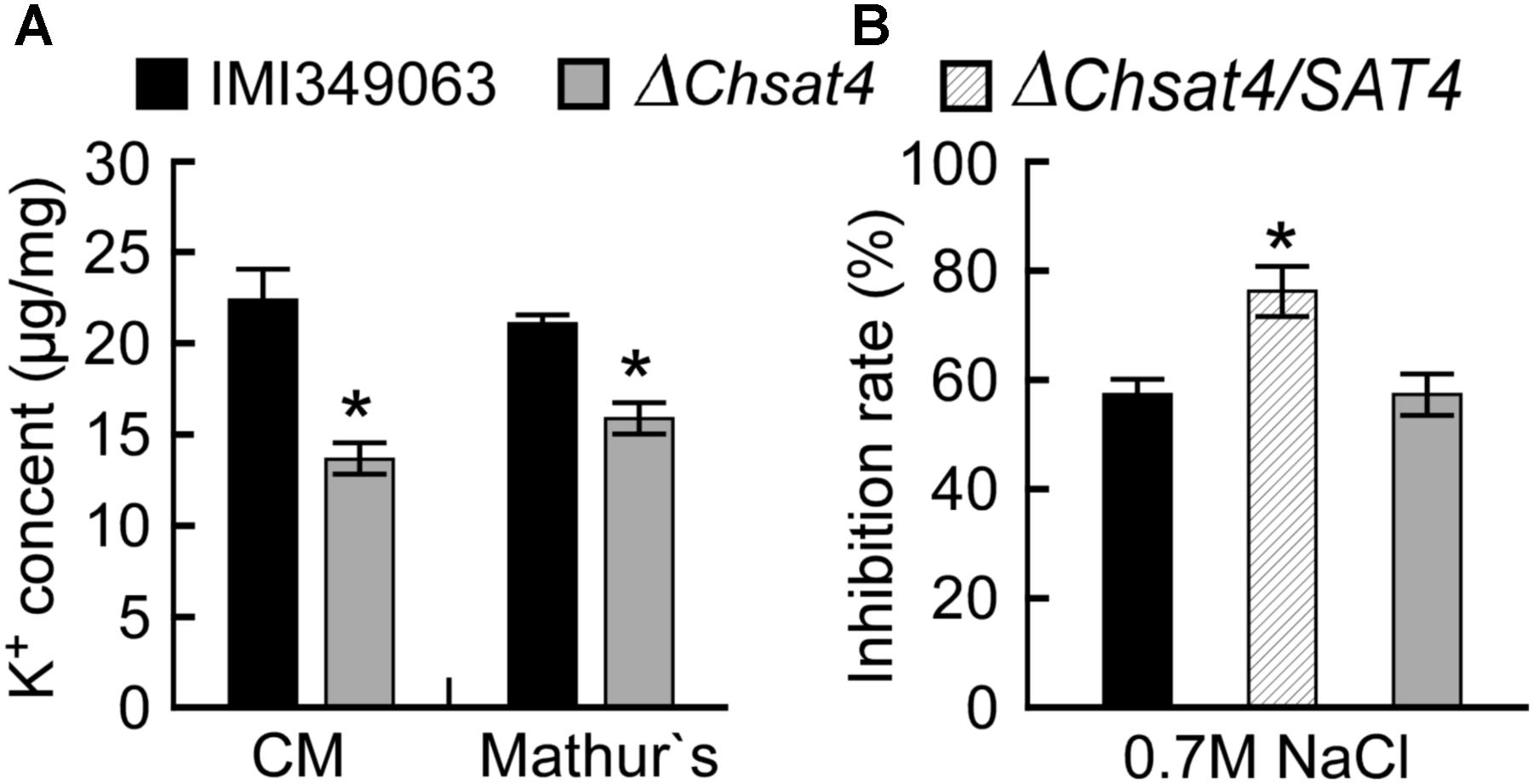
FIGURE 2. ChSAT4 deletion mutant decreasing the K+ concentration and NaCl stress resistance. (A) K+ concentration in the mycelia of C. higginsianum cultured in CM and Mathur’s media. (B) Inhibition rate of mycelial growth of IMI340963, ΔChsat4 mutant and the complemented strain grown on CM plates contain 0.7 M NaCl for 5 days. Error bars represent the SD from the means, and asterisks indicate statistically significant differences (p < 0.01).
Effect of Deletion of ChSAT4 on Cell Wall Integrity
To evaluate the effect of the deletion of ChSAT4 on the cell wall integrity in C. higginsianum, the wild type IMI349063, the ΔChsat4 mutant, and the complemented strain were inoculated onto Mathur’s plates containing the cell wall inhibitor SDS, CFW, and CR, respectively. When exposed to SDS, the ΔChsat4 mutant was more sensitive than the wild type and the complemented strain (Figure 3). However, the ΔChsat4 mutant was more tolerant to CFW and CR than the wild type and the complemented strain (Figure 3). These data indicated that the deletion of ChSAT4 altered the normal resistance to cell wall inhibitors.
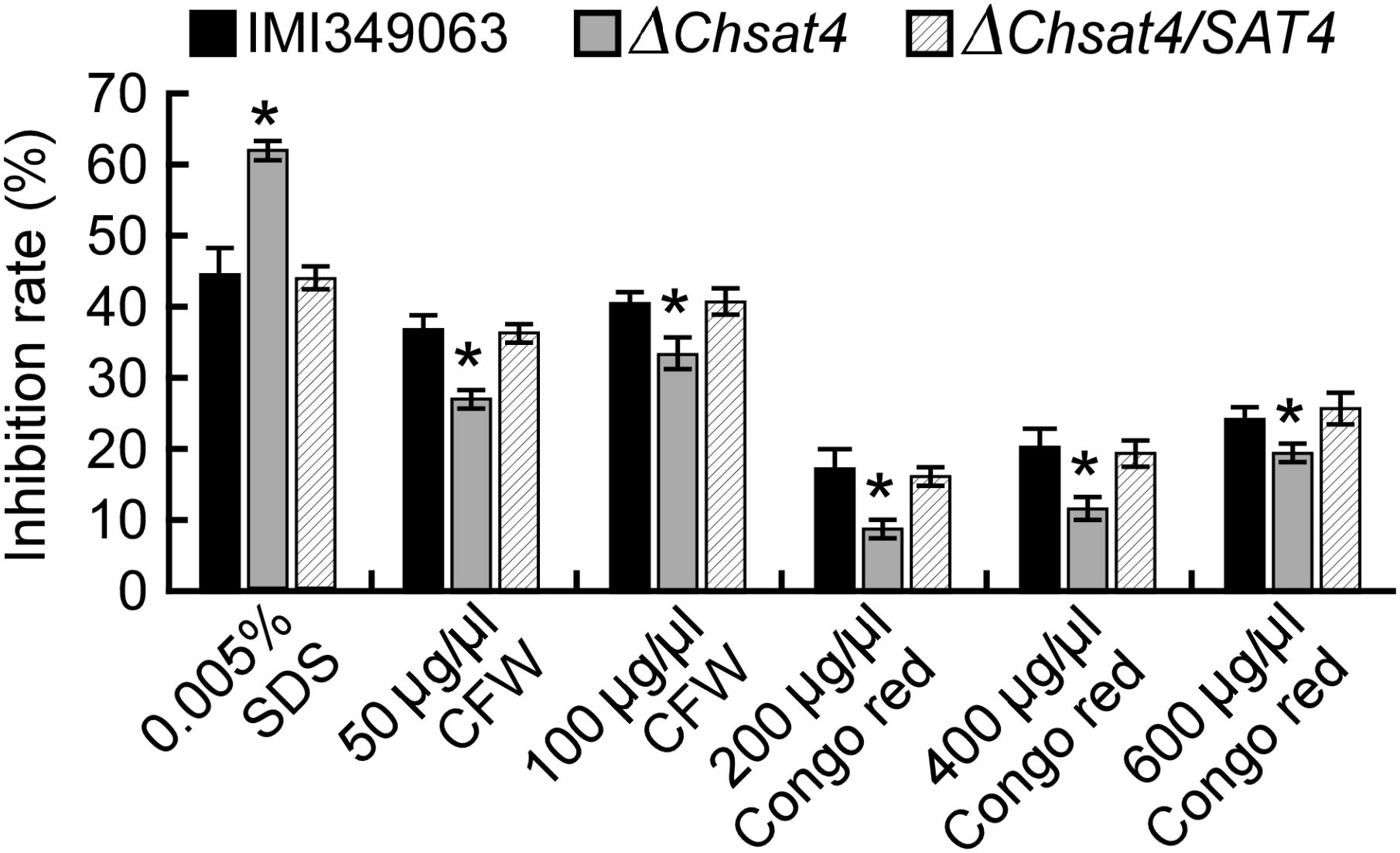
FIGURE 3. The CHSAT4 deletion mutant altering the resistance to various cell wall inhibitors. Inhibition rates of hyphal growth of IMI340963, the ΔChsat4 mutant, and the complemented strain grown on CM plates containing SDS, CFW, Congo red for 5 days.
Chitin is a major component of the cell wall of fungi, and the normal synthesis and distribution of chitin are important for polar hyphal tip growth and hyphal morphology (Huang et al., 2017). Chitin distribution was assayed using CFW staining, and results showed that chitin primarily accumulated in hyphal tips in both of the wild type and complemented strain. However, the chitin distribution was not restricted to the growing apices in the ΔChsat4 mutant (Figure 4A). To further analyze the effect of deletion of ChSAT4 on the cell wall integrity, the protoplast release assay was performed, and the results showed that the ΔChsat4 mutant released protoplasts more quickly than both of the wild type and complemented strain (Figure 4B). Additionally, the expression level of seven chitin synthases genes (CHS1: CH063_05042; CHS2: CH063_04156; CHS3: CH063_11805; CHS4: CH063_05355; CHS5: CH063_01328; CHS6: CH063_12829; CHS7: CH063_06688) was tested using qRT-PCR. The result showed that the expression levels of these seven genes were significantly lower in ΔChsat4 mutant than those of the wild type (Figure 4C). These results indicated that the ΔChsat4 mutant was defective in cell wall integrity.
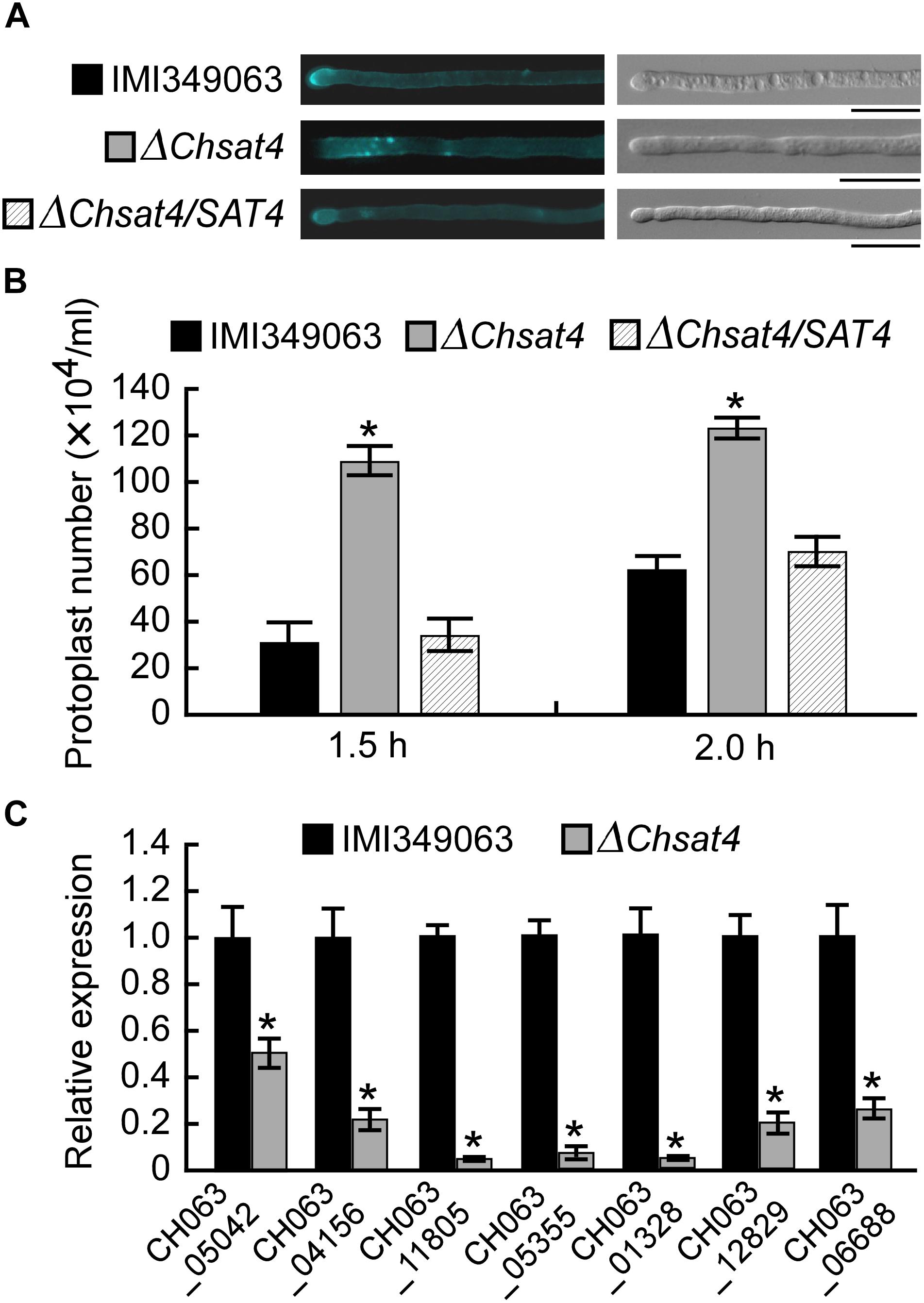
FIGURE 4. Deletion of ChSAT4 altering the cell wall integrity. (A) Disruption of ChSAT4 altered the chitin distribution on the cell wall. (B) Protoplasts of the wild type and ΔChsat4 mutant after being treated with a solution of cell wall lytic enzyme for 1.5 and 2 h. Error bars represent the SD from the means, and asterisks indicate statistically significant differences (p < 0.01). (C) The expression of seven chitin synthases in the wild type and ΔChsat4 mutant. Error bars represent the SD, and t-test analysis was shown with ∗p < 0.01 versus the wild type.
Function of ChSat4 in Responses to Hyperoxide Stress
To evaluate the effect of the ChSAT4 deletion on the resistance to oxidative stress, the wild type, the ΔChsat4 mutant, and the complemented strain were inoculated onto Mathur’s plates where they were subjected to H2O2. In the presence of H2O2, the ΔChsat4 mutant showed higher growth inhibition rates than those of the wild type and complemented strain (Figures 5A,B). The qRT-PCR analysis showed that the expression levels of catalase (CAT: CH063_11688) and peroxidase (POX: CH063_05165) were significantly decreased in the ΔChsat4 mutant (Figure 5C). These results indicated that the deletion of ChSAT4 may result in the reduced expression of the genes involved in reactive oxygen species scavenging, and lead to a greater sensitivity to H2O2.
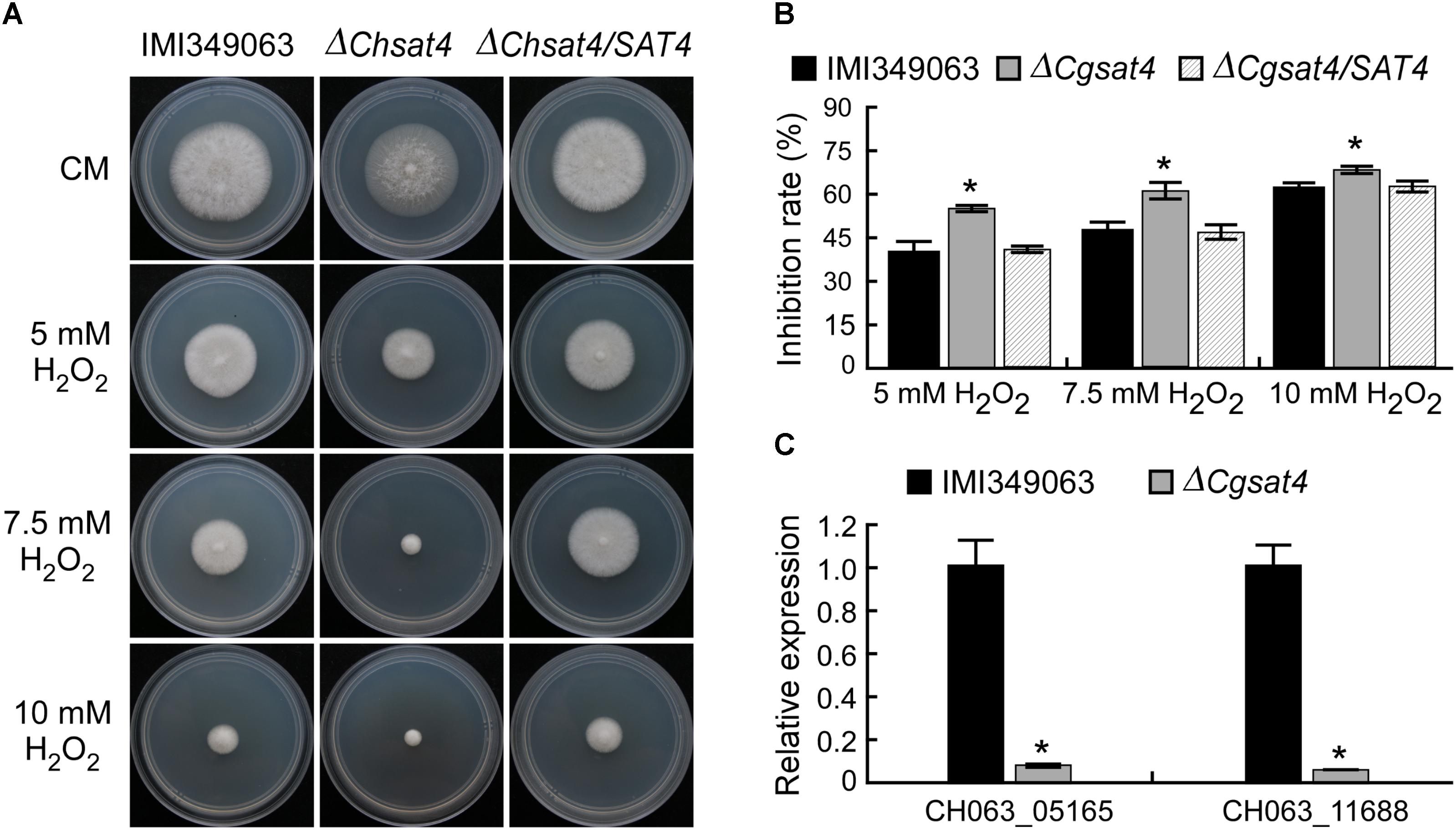
FIGURE 5. Sensitivity of the ChSAT4 deletion mutant to H2O2. (A) Colony diameter of IMI340963, ΔChsat4 mutant, and the complemented strain grown on CM plates containing different concentrations of H2O2 for 5 days. (B) Inhibition rates of mycelial growth of IMI340963, ΔChsat4 mutant, and the complemented strain exposed to H2O2. Error bars represent SD, and asterisks indicate statistically significant differences (p < 0.01). (C) The expression levels of catalase and peroxidase in the wild type and the ΔChsat4 mutant. Error bars represent the SD, and t-test analysis was shown with ∗p < 0.01 versus the wild type.
Pathogenicity of the ChSAT4 Deletion Mutant
Since the ΔChsat4 mutant was defective in sporulation, mycelial blocks of the wild type, the ΔChsat4 mutant, and the complemented strain were inoculated on detached leaves and petioles of B. rapa subsp. campestris, respectively, to evaluate their pathogenicity. At 5 dpi, typical and enlarged lesions were observed on the leaves of B. rapa subsp. campestris inoculated with the wild type and complemented strains. In contrast, there were very small and limited lesions on the leaves inoculated with the ΔChsat4 mutant. There were no lesions observed on the leaves inoculated solely with agar (Figures 6A,B). When the petioles were inoculated using the mycelial mats of the wild type, the ΔChsat4 mutant, and the complemented strain, similar results were observed (Figure 6C). In order to evaluate differences in fungal biomass between the ΔChsat4 mutant and the wild type during infection, the C. higginsianum actin DNA fragment was amplified by qPCR using the B. rapa actin gene for normalization. The result showed that a highly significant reduction in the amount of fungal actin DNA was observed at 3 dpi for the ΔChsat4 mutant compared with the wild type (Figure 6D).
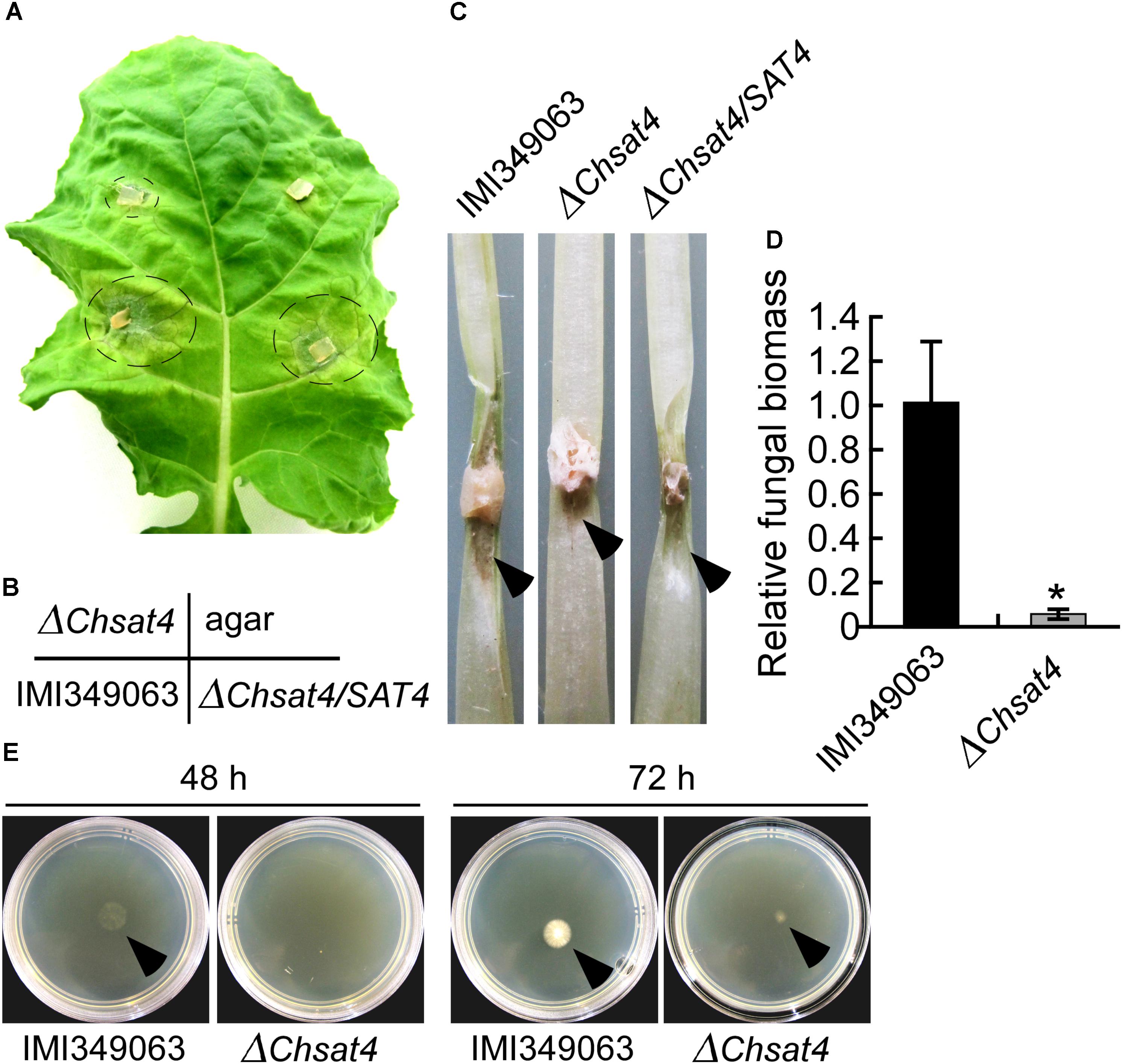
FIGURE 6. Defects of the ΔChsat4 mutant in pathogenicity to the host plant. (A) Pathogenicity assay of the wild type IMI340963, ΔChsat4 mutant, and the complemented strain on detached leaves of Brassica rapa subsp. campestris. Circles indicate the diseased areas. (B) The plug position of the wild type IMI340963, ΔChsat4 mutant, and the complemented strain, and a blank CM plug in (A) was indicated. (C) Pathogenicity assay when inoculation of wild type IMI340963, ΔChsat4 mutant, and the complemented strain on detached petioles of Brassica rapa subsp. campestris. Lesions are indicated by arrows. (D) Relative fungal biomass monitored from infected tissues of B. chinensis at 3 dpi. The qPCR was performed to amplify the C. higginsianum actin DNA gene relative to the B. chinensis actin gene. Error bars represent the SD, and t-test analysis was shown with ∗p < 0.01 versus the wild type. (E) Penetration of cellophane sheets. Colonies of the wild type IMI349063 and the ΔChsat4 mutant grown for 48 and 72 h on a CM medium plate covered by a cellophane sheet (L), and the colony in the same plate with cellophane removal and incubated for one additional day (R), respectively. Colonies are indicated by arrows.
The in vitro cellophane penetration ability has been reported to correlate significantly with in vivo pathogenicity in Fusarium oxysporum and other filamentous fungi (Rosales and Pietro, 2008; Rispail and Di Pietro, 2009; Gu et al., 2015). To further investigate the role of ChSat4 in mycelial penetration ability, the wild type and ΔChsat4 mutant were inoculated on a cellophane membrane placed on a CM plate, respectively. The ΔChsat4 mutant penetrated the cellophane and formed a colony on the medium plate at 72 h postinoculation (hpi). In contrast, the wild type was able to cross cellophane membranes at 48 hpi (Figure 6E). These results indicated that ChSat4 was required for the mycelial penetration and full virulence in C. higginsianum.
Subcellular Localization of ChSat4-GFP Fusion Protein
The ChSAT4-GFP fusion construct was introduced into the ΔChsat4 mutant, and the complementation strain ΔChsat4/SAT4 was obtained. Our data indicated that the complemented strain ΔChsat4/SAT4 completely restored the defects in the ΔChsat4 mutant as shown earlier. To further investigate the localization pattern of the ChSat4 in C. higginsianum, GFP signal of the fusion protein ChSat4-GFP expressed in the ΔChsat4/SAT4 strain was observed under an epifluorescence microscope. Results showed that a strong green fluorescence signal was observed in the hyphal cytoplasm in the ΔChsat4/SAT4 strain (Figure 7A). We also observed the same GFP localization pattern in the conidia (Figure 7B). These results suggest that ChSat4 was localized in the cytoplasm in C. higginsianum.
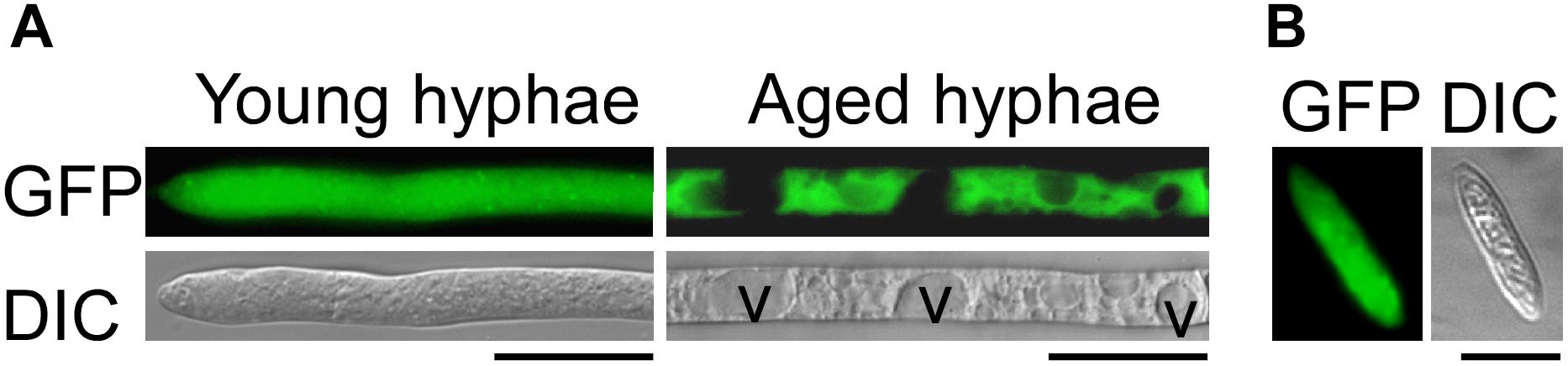
FIGURE 7. Cellular localization of ChSat4-GFP. The subcellular localization of ChSat4 detected using a ChSAT4-GFP fusion protein driven by its native promoter. (A) Cellular localization of ChSat4-GFP in vegetative hyphae. V indicates the vesicles. (B) Cellular localization of ChSat4-GFP in conidia. Bars = 10 μm.
Cross-Species Function of ChSat4 With FgSat4 in F. graminearum
Like C. higginsianum, F. graminearum belongs to hemibiotrophic phytopathogen, employing a biotrophic phase during its initial stage of infection before switching to necrotrophy and inducing cell death (Jansen et al., 2005; Huang et al., 2016). To assess the functional conservation of the Sat4 protein in the filamentous fungi C. higginsianum and F. graminearum, the ChSAT4-GFP fusion construct was introduced into the ΔFgsat4 mutant and produced the transformant ΔFgsat4/ChSAT4. The mycelial growth of the ΔFgsat4 mutant was significantly reduced on the CM plates compared to that of the wild type PH-1; however, the transformant of ΔFgsat4/ChSAT4 showed similar colony diameter to the wild type (Figure 8A). The ability to produce conidia of the ΔFgSat4 mutant was significantly decreased, but there was no significant difference between the ΔFgsat4/ChSAT4 strain and the wild type (Figure 8B). When exposed to the stressors KCl and NaCl, ΔFgsat4/ChSAT4 showed an inhibition rate similar to that of the wild type PH-1 and restored the defects in the resistance to KCl and NaCl shown in the ΔFgsat4 mutant (Figure 8C). The pathogenicity assay on tomato fruits also showed that the virulence of the ΔFgSat4 mutant significantly decreased, but the ΔFgsat4/ChSAT4 strain showed strong virulence similar to that of the wild type (Figure 8D). Fungal biomass was monitored during infection, and results showed that the amount of fungal actin gene DNA was significantly reduced in the ΔFgsat4 mutant than that in the wild type and the ΔFgsat4/ChSAT4 strain (Figure 8E). These results suggested that ChSat4 shared some cross-species function with FgSat4 in F. graminearum.
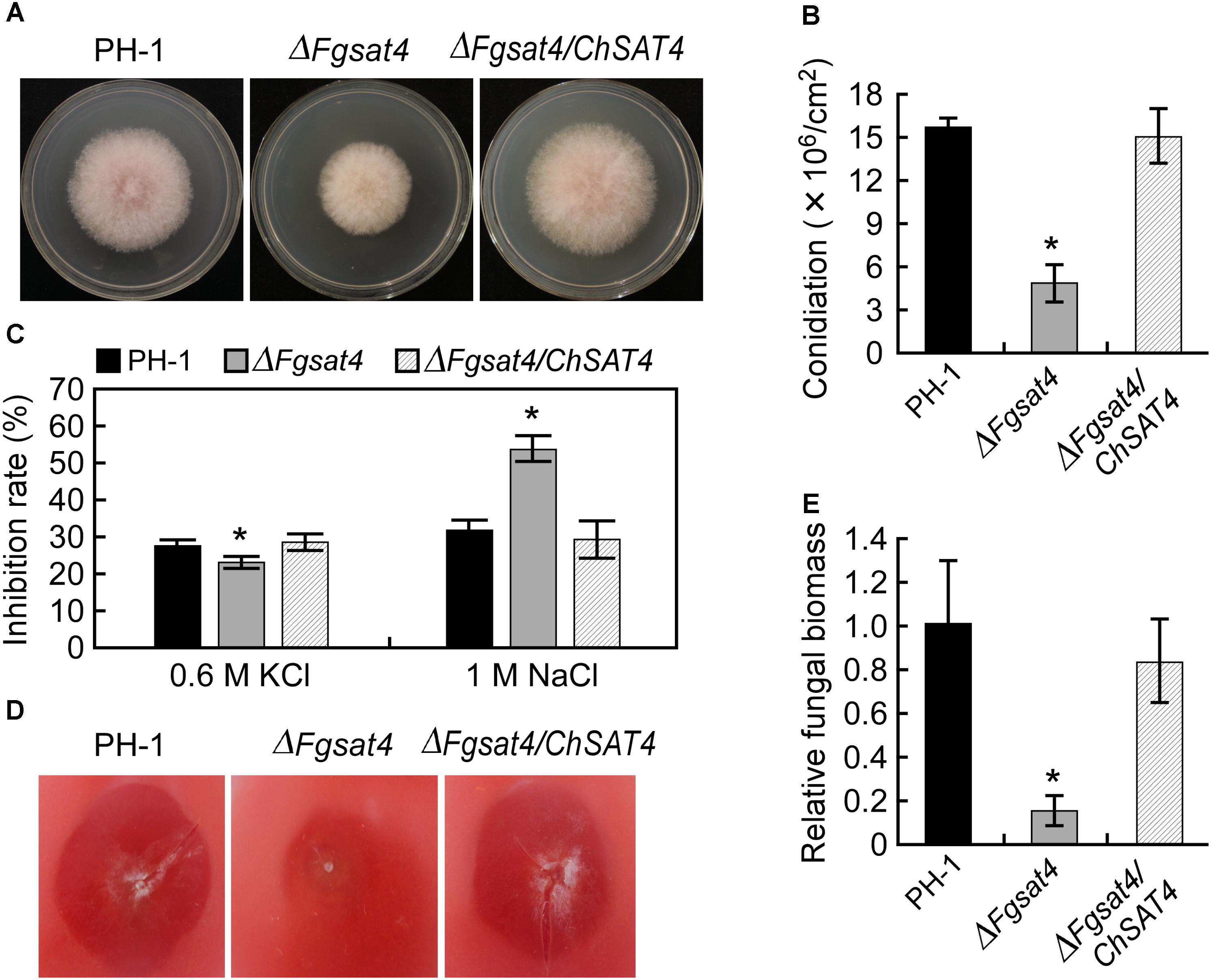
FIGURE 8. Restoration of some defects of the ΔFgsat4 mutant in Fusarium graminearum by ChSAT4. (A) Mycelial growth of the wild type PH-1, ΔFgsat4 mutant, and ΔFgsat4/ChSAT4 strain on CM medium plates. (B) The ability to produce conidia of wild type PH-1, ΔFgsat4 mutant, and ΔFgsat4/ChSAT4 strain. (C) The mycelial growth inhibition rate of the wild type PH-1, ΔFgsat4 mutant, and ΔFgsat4/ChSAT4 strain when exposed to NaCl and KCl. (D) Pathogenicity of wild type PH-1, ΔFgsat4 mutant, and ΔFgsat4/ChSAT4 strain on tomato fruit at 5 dpi. (E) Relative fungal biomass during infection monitored by qPCR. Total DNA was extracted from leaf tissues inoculated either with the wild type PH-1, ΔChsat4 mutant, and ΔFgsat4/ChSAT4 strain at 4 dpi. Error bars represent SD, and asterisks indicate statistically significant differences (p < 0.01).
Discussion
In eukaryotic organisms, reversible protein phosphorylation by protein kinases is involved in the regulation of various growth and developmental processes and responses to environmental stimuli (Serrano, 1996). Protein kinase genes, ChCBK1 (Schmidpeter et al., 2017) and ChMK1 (Wei et al., 2016), were important for plant infection in C. higginsianum. In this study, the deletion of serine/threonine kinase ChSAT4 resulted in various defects in C. higginsianum, including the reduction of vegetative growth, stress response, K+ accumulation, cell wall integrity, and pathogenicity in C. higginsianum.
The cell wall of fungi plays a key role in the exchange of material between the cell and the external environment. In plant pathogenic fungi, cell wall integrity is required for polarized hyphal growth, invasive structure development, and the infection of the plant (Samalova et al., 2017). Chitin is a major component of the filamentous fungal cell wall, and the normal synthesis and distribution of chitin are important to maintain polar hyphal tip growth and morphology. The deletion of ChSAT4 altered the resistance to the cell wall stressors SDS, CFW, and CR. The ΔChsat4 mutant was sensitive to SDS and showed stronger resistance to CFW and CR than the wild type and the complemented strain. The CFW staining also showed that the deletion of ChSAT4 altered the normal distribution of chitin in the mycelium. In addition, when the mycelia were treated with the cell wall lytic enzyme, the ΔChsat4 mutant released protoplasts more quickly than the wild type. The expression levels of chitin synthases were significantly inhibited in the ΔChSat4 mutant. These data indicated that ChSat4 was involved in the cell wall integrity in C. higginsianum by regulating the chitin synthases expression.
Monovalent cations such as protons, potassium, and sodium play multiple key roles in eukaryotic cells (Robinson et al., 1992). The regulation of cellular ion homeostasis is a basic property of living cells (Dichtl et al., 1997). The Na+ and K+ are important intracellular cations, and the concentration of these cations affects fundamental physiological progresses (Benito et al., 2011). A high concentration of cations usually leads to toxicity. However, the threshold for the toxicity of lithium and sodium is much lower than that for potassium since large amounts of sodium and lithium accumulation in the cytosol tends to destroy essential and sensitive enzymes (Pérez-Valle et al., 2007; Corratgé-Faillie et al., 2010; Hirasaki et al., 2011). In S. cerevisiae, the high-affinity potassium uptake system is encoded by the transporters Trk1 and Trk2 (Garciadeblas et al., 2007; Casado et al., 2010). The Trk1–Trk2 system is activated by two protein kinases encoded by two paralogs, Sat4/Hal4 and Hal5, to increase the influx of potassium and decrease the membrane potential toxicity (Pérez-Valle et al., 2010). The ΔScsat4 mutant was extremely sensitive to a variety of toxic cations such as lithium and sodium (Munson et al., 2004). In F. graminearum, FgSat4 was not directly involved in osmoregulation, but it may be specifically involved in the regulation of K+/Na+ transporter (Wang et al., 2011). In this study, the deletion of ChSAT4 significantly decreased the concentration of intracellular K+ in C. higginsianum. In addition, the ΔChsat4 mutant altered the resistance to KCl and NaCl in the extracellular environment. These results indicated that ChSat4 was required for the regulation of the intracellular and extracellular balance of K+, and the deletion of ChSAT4 could change the normal physiological process. Whether ChSat4 regulates the accumulation K+ to maintain the proper intracellular concentration, and avoid cation toxicity by regulating the activity of K+ transporter needs to be explored in further research.
Multifaceted signaling pathways are involved in plant-pathogen interaction (Imam et al., 2016b; Kumar et al., 2016). In plants, the rapid accumulation of ROS is considered to be the first response against invading pathogens (Shetty et al., 2007; Imam et al., 2016a). Hydrogen peroxide (H2O2), an important ROS, has been reported to inhibit biotrophic pathogens, but to benefit necrotrophic pathogens (Mellersh et al., 2002; Able, 2003). In the hemibiotrophic fungus, Septoria tritici, hyphal growth was inhibited by H2O2 during the biotrophic phase, but a large H2O2 accumulation occurs in the host during reproduction (Shetty et al., 2007). C. higginsianum develops large bulbous biotrophic hyphae in the first infected cell, and the necrotrophic secondary filamentous hyphae develop in the neighboring cells (Korn et al., 2015). During these plant infection processes, C. higginsianum needs to counteract the ROS stress and regulate gene expression in response to oxidative stress. In this study, the ΔChsat4 mutant showed a higher mycelial growth inhibition rate than the wild type and complemented strain when exposed to H2O2. Since H2O2 is involved in the host defense in the early interaction between C. higginsianum and its host (Huser et al., 2009), the defect of resistance to H2O2 may lead to a reduced ability to scavenge host-derived ROS and an attenuation of virulence.
As a homolog of ChSat4, FgSat4 (Fg06939) was identified in the phytopathogenic fungus F. graminearum (Wang et al., 2011). The deletion of FgSAT4 resulted in a defect in resistance to NaCl stress (Wang et al., 2011). C. higginsianum ChSAT4 shares a 75% sequence identity with FgSAT4 in F. graminearum (Supplementary Figure S2). Sequence analysis also showed that both of FgSAT4 and ChSAT4 have a conserved STKc_HAL4_like domain (Supplementary Figure S2), which was determined to regulate potassium ion uptake and cellular resistance to other ions such as sodium in budding and fission yeast (Marchler-Bauer et al., 2017). Here, our data showed ChSat4 is required for potassium accumulation and resistance to ion stress in C. higginsianum (Figure 2). Heterologous expression of ChSAT4 in the ΔFgsat4 mutant restored its defects in stress resistance to KCl and NaCl (Figure 7). These results may indicate that Sat4 not only has a conserved function in potassium uptake and cation stress resistance in yeast, but also shares the similar roles in phytopathogenic fungi. Furthermore, the deletion of FgSAT4 significantly reduced the ability to produce conidia, and resulted in the defect in pathogenicity to the host plant in F. graminearum (Wang et al., 2011). However, in C. higginsianum, conidiation was completely abolished, and the virulence to the host was significantly reduced in the ΔChsat4 mutant (Figure 6). Interestingly, expression of ChSAT4 in the ΔFgsat4 mutant restored the ability to produce conidia and pathogenicity in the ΔFgsat4 mutant (Figure 8). These results may indicate that Sat4 shares a conserved regulation to pathogenicity in C. higginsianum and F. graminearum. Whether the kinase Sat4 is involved in regulating pathogenicity in other plant pathogenic fungi and its expression regulation model needs to be emphasized in further studies.
Conclusion
Serine/threonine kinase ChSat4 is required for vegetative growth, asexual development, stress response, K+ accumulation, cell wall integrity, and full pathogenicity in C. higginsianum.
Author Contributions
LH and J-RY conceived and designed the experiments. J-YY, Y-LF, and PW performed the experiments. LH, J-YY, and Y-LF analyzed the experiment data. LH and J-RY contributed to reagents, materials, and analysis tools. LH and J-YY wrote the paper. All authors have read and approved of the final manuscript.
Funding
This study was financially supported by the Major Project of Jiangsu Province University Natural Science Research (16KJA220002), the Fund of Independent Innovation of Agricultural Sciences of Jiangsu Province [CX(16)1005], and the Priority Academic Program Development of Jiangsu Higher Education Institutions (PAPD).
Conflict of Interest Statement
The authors declare that the research was conducted in the absence of any commercial or financial relationships that could be construed as a potential conflict of interest.
Supplementary Material
The Supplementary Material for this article can be found online at: https://www.frontiersin.org/articles/10.3389/fmicb.2018.02311/full#supplementary-material
References
Able, A. J. (2003). Role of reactive oxygen species in the response of barley to necrotrophic pathogens. Protoplasma 221, 137–143. doi: 10.1007/s00709-002-0064-1
Almeida, S. M., Umeo, S. H., Marcante, R. C., Yokota, M. E., Valle, J. S., Dragunski, D. C., et al. (2015). Iron bioaccumulation in mycelium of Pleurotus ostreatus. Braz. J. Microbiol. 46, 195–200. doi: 10.1590/S1517-838246120130695
Araújo, L., Gonçalves, A. E., and Stadnik, M. J. (2014). Ulvan effect on conidial germination and appressoria formation of Colletotrichum gloeosporioides. Phytoparasitica 42, 631–640. doi: 10.1007/s12600-014-0404-7
Benito, B., Garciadeblas, B., Fraile-Escanciano, A., and Rodriguez-Navarro, A. (2011). Potassium and sodium uptake systems in fungi. The transporter diversity of Magnaporthe oryzae. Fungal Genet. Biol. 48, 812–822. doi: 10.1016/j.fgb.2011.03.002
Buch-Pedersen, M. J., Rudashevskaya, E. L., Berner, T. S., Venema, K., and Palmgren, M. G. (2006). Potassium as an intrinsic uncoupler of the plasma membrane H+ -ATPase. J. Biol. Chem. 281, 38285–38292. doi: 10.1074/jbc.M604781200
Casado, C., Yenush, L., Melero, C., Ruiz Mdel, C., Serrano, R., Pérez-Valle, J., et al. (2010). Regulation of Trk-dependent potassium transport by the calcineurin pathway involves the Hal5 kinase. FEBS Lett. 584, 2415–2420. doi: 10.1016/j.febslet.2010.04.042
Corratgé-Faillie, C., Jabnoune, M., Zimmermann, S., Very, A. A., Fizames, C., and Sentenac, H. (2010). Potassium and sodium transport in non-animal cells: the Trk/Ktr/HKT transporter family. Cell Mol. Life Sci. 67, 2511–2532. doi: 10.1007/s00018-010-0317-7
Damm, U., Mostert, L., Crous, P. W., and Fourie, P. H. (2008). Novel Phaeoacremonium species associated with necrotic wood of Prunus trees. Persoonia 20, 87–102. doi: 10.3767/003158508X324227
Dichtl, B., Stevens, A., and Tollervey, D. (1997). Lithium toxicity in yeast is due to the inhibition of RNA processing enzymes. EMBO J. 16, 7184–7195. doi: 10.1093/emboj/16.23.7184
Gao, T., Chen, J., and Shi, Z. (2016). Fusarium graminearum pyruvate dehydrogenase kinase 1 (FgPDK1) is critical for conidiation, mycelium growth, and pathogenicity. PLoS One 11:e0158077. doi: 10.1371/journal.pone.015807
Garciadeblas, B., Barrero-Gil, J., Benito, B., and Rodriguez-Navarro, A. (2007). Potassium transport systems in the moss Physcomitrella patens: pphak1 plants reveal the complexity of potassium uptake. Plant J. 52, 1080–1093. doi: 10.1111/j.1365-313X.2007.03297.x
Gu, Q., Chen, Y., Liu, Y., Zhang, C., and Ma, Z. (2015). The transmembrane protein FgSho1 regulates fungal development and pathogenicity via the MAPK module Ste50-Ste11-Ste7 in Fusarium graminearum. New Phytol. 206, 315–328. doi: 10.1111/nph.13158
Guo, M., Guo, W., Chen, Y., Dong, S., Zhang, X., Zhang, H., et al. (2010). The basic leucine zipper transcription factor Moatf1 mediates oxidative stress responses and is necessary for full virulence of the rice blast fungus Magnaporthe oryzae. Mol. Plant Microbe Interact. 23, 1053–1068. doi: 10.1094/MPMI-23-8-1053
He, P., Wang, Y., Wang, X., Zhang, X., and Tian, C. (2017). The mitogen-activated protein kinase CgMK1 governs appressorium formation, melanin synthesis, and plant infection of Colletotrichum gloeosporioides. Front. Microbiol. 8:2216. doi: 10.3389/fmicb.2017.02216
Hirasaki, M., Horiguchi, M., Numamoto, M., Sugiyama, M., Kaneko, Y., Nogi, Y., et al. (2011). Saccharomyces cerevisiae protein phosphatase Ppz1 and protein kinases Sat4 and Hal5 are involved in the control of subcellular localization of Gln3 by likely regulating its phosphorylation state. J. Biosci. Bioeng. 111, 249–254. doi: 10.1016/j.jbiosc.2010.11.013
Huang, L., Zhang, S., Yin, Z., Liu, M., Li, B., Zhang, H., et al. (2017). MoVrp1, a putative protein verprolin protein, is required for asexual development and infection in the rice blast fungus Magnaporthe oryzae. Sci. Rep. 7:41148. doi: 10.1038/srep41148
Huang, Y., Li, L., Smith, K. P., and Muehlbauer, G. J. (2016). Differential transcriptomic responses to Fusarium graminearum infection in two barley quantitative trait loci associated with Fusarium head blight resistance. BMC Genomics 17:387. doi: 10.1186/s12864-016-2716-0
Huser, A., Takahara, H., Schmalenbach, W., and O’Connell, R. (2009). Discovery of pathogenicity genes in the crucifer anthracnose fungus Colletotrichum higginsianum, using random insertional mutagenesis. Mol. Plant Microbe Interact. 22, 143–156. doi: 10.1094/MPMI-22-2-0143
Imam, J., Mandal, N. P., Variar, M., and Shukla, P. (2016a). “Advances in molecular mechanism toward understanding plant-microbe interactions: A study of M. oryzae versus rice,” in Frontier Discoveries and Innovations in Interdisciplinary Microbiology, ed. P. Shukla (Berlin: Springer), 79–96.
Imam, J., Singh, P. K., and Shukla, P. (2016b). Plant microbe interactions in post genomic era: perspectives and applications. Front. Microbiol. 7:1488. doi: 10.3389/fmicb.2016.01488
Jansen, C., von Wettstein, D., Schäfer, W., Kogel, K., Felk, A., and Maier, F. J. (2005). Infection patterns in barley and wheat spikes inoculated with wild-type and trichodiene synthase gene disrupted Fusarium graminearum. Proc. Natl. Acad. Sci. U.S.A. 102, 16892–16897. doi: 10.1073/pnas.0508467102
Kahm, M., Navarrete, C., Llopis-Torregrosa, V., Herrera, R., Barreto, L., Yenush, L., et al. (2012). Potassium starvation in yeast: mechanisms of homeostasis revealed by mathematical modeling. PLoS Comput. Biol. 8:e1002548. doi: 10.1371/journal.pcbi.1002548
Kim, K. S., and Lee, Y. (2012). Gene expression profiling during conidation in the rice blast pathogen Magnaporthe oryzae. PLoS One 7:e43202. doi: 10.1371/journal.pone.0043202
Korn, M., Schmidpeter, J., Dahl, M., Muller, S., Voll, L. M., and Koch, C. (2015). A genetic screen for pathogenicity genes in the hemibiotrophic fungus Colletotrichum higginsianum identifies the plasma membrane proton pump Pma2 required for host penetration. PLoS One 10:e0125960. doi: 10.1371/journal.pone.0125960
Kumar, V., Baweja, M., Singh, P. K., and Shukla, P. (2016). Recent developments in systems biology and metabolic engineering of plant-microbe interactions. Front. Plant Sci. 7:1421. doi: 10.3389/fpls.2016.01421
Li, B., Dong, X., Li, X. R., Chen, H. G., Zhang, H. F., Zheng, X. B., et al. (2018). A subunit of the HOPS endocytic tethering complex, FgVps41, is important for fungal development and plant infection in Fusarium graminearum. Environ. Microbiol. 20, 1436–1451. doi: 10.1111/1462-2920.14050
Lyu, X., Shen, C., Fu, Y., Xie, J., Jiang, D., Li, G., et al. (2016). The microbial opsin homolog Sop1 is involved in Sclerotinia sclerotiorum development and environmental stress response. Front. Microbiol. 6:1504. doi: 10.3389/fmicb.2015.01504
Marchler-Bauer, A., Bo, Y., Han, L., He, J., Lanczychi, C. J., Lu, S., et al. (2017). CDD/SPARCLE: functional classification of proteins via subfamily domain architectures. Nucleic Acids Res. 45, D200–D203. doi: 10.1093/nar/gkw1129
Mellersh, D. G., Foulds, I. V., Higgins, V. J., and Heath, M. C. (2002). H2O2 plays different roles in determining penetration failure in three diverse plant-fungal interactions. Plant J. 29, 257–268. doi: 10.1046/j.0960-7412.2001.01215.x
Mulet, J. M., Leube, M. P., Kron, S. J., Rios, G., Fink, G. R., and Serrano, R. (1999). A novel mechanism of ion homeostasis and salt tolerance in yeast: the Hal4 and Hal5 protein kinases modulate the Trk1-Trk2 potassium transporter. Mol. Cell. Biol. 19, 3328–3337. doi: 10.1128/MCB.19.5.332
Munson, A. M., Haydon, D. H., Love, S. L., Fell, G. L., Palanivel, V. R., and Rosenwald, A. G. (2004). Yeast ARL1 encodes a regulator of K+ influx. J. Cell Sci. 117, 2309–2320. doi: 10.1242/jcs.01050
Nakamura, M., Fujikawa, T., Nakamori, D., and Iwai, H. (2018). Draft genome sequence of Colletotrichum sansevieriae Sa-1-2, the anthracnose pathogen of Sansevieria trifasciata. Data Brief 18, 691–695. doi: 10.1016/j.dib.2018.03.083
O’Connell, R., Herbert, C., Sreenivasaprasad, S., Khatib, M., Esquerré-Tugayé, M.-T., and Dumas, B. (2004). A novel Arabidopsis-Colletotrichum pathosystem for the molecular dissection of plant-fungal interactions. Mol. Plant Microbe Interact. 17, 272–282. doi: 10.1094/MPMI.2004.17.3.272
O’Connell, R. J., Thon, M. R., Hacquard, S., Amyotte, S. G., Kleemann, J., Torres, M. F., et al. (2012). Lifestyle transitions in plant pathogenic Colletotrichum fungi deciphered by genome and transcriptome analyses. Nat. Genet. 44, 1060–1065. doi: 10.1038/ng.2372
Pérez-Valle, J., Jenkins, H., Merchan, S., Montiel, V., Ramos, J., Sharma, S., et al. (2007). Key role for intracellular K+ and protein kinases Sat4/Hal4 and Ha15 in the plasma membrane stabilization of yeast nutrient transporters. Mol. Cell. Biol. 27, 5725–5736. doi: 10.1128/MCB.01375-06
Pérez-Valle, J., Rothe, J., Primo, C., Martínez-Pastor, M., Ariño, J., Pascual-Ahuir, A., et al. (2010). Hal4 and Hal5 protein kinases are required for general control of carbon and nitrogen uptake and metabolism. Eukaryot. Cell 9, 1881–1890. doi: 10.1128/EC.00184-10
Plaumann, P., Schmidpeter, J., Dahl, M., Taher, L., and Koch, C. (2018). A dispensable chromosome is required for virulence in the hemibiotrophic plant pathogen Colletotrichum higginsianum. Front. Microbiol. 9:1005. doi: 10.3389/fmicb.2018.01005
Qi, Z., Wang, Q., Dou, X., Wang, W., Zhao, Q., Lv, R., et al. (2012). MoSwi6, an APSES family transcription factor, interacts with MoMps1 and is required for hyphal and conidial morphogenesis, appressorial function and pathogenicity of Magnaporthe oryzae. Mol. Plant Pathol. 13, 677–689. doi: 10.1111/j.1364-3703.2011.00779.x
Rispail, N., and Di Pietro, A. (2009). Fusarium oxysporum Ste12 controls invasive growth and virulence downstream of the Fmk1 MAPK cascade. Mol. Plant Microbe Interact. 22, 830–839. doi: 10.1094/MPMI-22-7-0830
Robinson, L. C., Hubbard, E. J., Graves, P. R., DePaoli-Roach, A. A., Roach, P. J., Kung, C., et al. (1992). Yeast casein kinase I homologues: an essential gene pair. Proc. Natl. Acad. Sci. U.S.A. 89, 28–32. doi: 10.1073/pnas.89.1.28
Rodriguez-Navarro, A. (2000). Potassium transport in fungi and plants. Biochim. Biophys. Acta 1469, 1–30. doi: 10.1016/S0304-4157(99)00013-1
Rosales, R. C. P., and Pietro, A. D. (2008). Vegetative hyphal fusion is not essential for plant infection by Fusarium oxysporum. Eukaryot. Cell 7, 162–171. doi: 10.1128/EC.00258-07
Rotin, D., Staub, O., and Haguenauer-Tsapis, R. (2000). Ubiquitination and endocytosis of plasma membrane proteins: role of Nedd4/Rsp5p family of ubiquitin-protein ligases. J. Membr. Biol. 176, 1–17. doi: 10.1007/s00232001079
Samalova, M., Melida, H., Vilapana, F., Bulone, V., Soanes, D. M., Talbot, N. J., et al. (2017). The β-1,3-glucanosyltransferases (Gels) affect the structure of the rice blast fungal cell wall during appressorium-mediated plant infection. Cell. Microbiol. 19:e12659. doi: 10.1111/cmi.12659
Schmidpeter, J., Dahl, M., Hofmann, J., and Koch, C. (2017). ChMob2 binds to ChCbk1 and promotes virulence and conidiation of the fungal pathogen Colletotrichum higginsianum. BMC Microbiol. 17:22. doi: 10.1186/s12866-017-0932-7
Serrano, R. (1996). Salt tolerance in plants and microorganisms: toxicity targets and defense mechanisms. Int. Rev. Cytol. 165, 1–52. doi: 10.1016/S0074-7696(08)62219-6
Shetty, N. P., Mehrabi, R., Lütken, H., Haldrup, A., Kema, G. H. J., Collinge, D. B., et al. (2007). Role of hydrogen peroxide during the interaction between the hemibiotrophic fungal pathogen Septoria tritici and wheat. New Phytol. 174, 637–647. doi: 10.1111/j.1469-8137.2007.02026.x
Sweigard, J. A., Chumley, F. G., and Valent, B. (1992). Disruption of a Magnaporthe grisea cutinase gene. Mol. Gen. Genet. 232, 183–190.
Tang, W., Ru, Y., Hong, L., Zhu, Q., Zuo, R., Guo, X., et al. (2015). System-wide characterization of bZIP transcription factor proteins involved in infection-related morphogenesis of Magnaporthe oryzae. Environ. Microbiol. 17, 1377–1396. doi: 10.1111/1462-2920.12618
Waller, J. M. (1992). “Colletotrichum diseases of perennial and other cash crops,” in Colletotrichum: Biology, Pathology and Control, eds J. A. Bailey and M. J. Jeger (Wallingford: CAB International), 167–185.
Wang, C., Zhang, S., Hou, R., Zhao, Z., Zheng, Q., Xu, Q., et al. (2011). Functional analysis of the kinome of the wheat scab fungus Fusarium graminearum. PLoS Pathog. 7:e1002460. doi: 10.1371/journal.ppat.1002460
Wang, L. Y., Shimada, K., Morishita, M., and Shiozaki, K. (2005). Response of fission yeast to toxic cations involves cooperative action of the stress-activated protein kinase Spc1/Sty1 and the Hal4 protein kinase. Mol. Cell. Biol. 25, 3945–3955. doi: 10.1074/jbc.M204593200
Wei, W., Xiong, Y., Zhu, W., Wang, N., Yang, G., and Peng, F. (2016). Colletotrichum higginsianum mitogen-activated protein kinase ChMK1: role in growth, cell wall integrity, colony melanization, and pathogenicity. Front. Microbiol. 7:1212. doi: 10.3389/fmicb.2016.01212
Zhao, X., Xue, C., Kim, Y., and Xu, J. (2004). A ligation-PCR approach for generating gene replacement constructs in Magnaporthe grisea. Fungal Genet. Newsl. 51, 17–18. doi: 10.4148/1941-4765.1137
Keywords: Colletotrichum higginsianum, serine/threonine kinase, K+ accumulation, cell wall integrity, pathogenicity
Citation: Yang J-Y, Fang Y-L, Wang P, Ye J-R and Huang L (2018) Pleiotropic Roles of ChSat4 in Asexual Development, Cell Wall Integrity Maintenance, and Pathogenicity in Colletotrichum higginsianum. Front. Microbiol. 9:2311. doi: 10.3389/fmicb.2018.02311
Received: 16 April 2018; Accepted: 10 September 2018;
Published: 24 October 2018.
Edited by:
Dirk Albert Balmer, Syngenta, SwitzerlandReviewed by:
Pratyoosh Shukla, Maharshi Dayanand University, IndiaKei Hiruma, Nara Institute of Science and Technology (NAIST), Japan
Copyright © 2018 Yang, Fang, Wang, Ye and Huang. This is an open-access article distributed under the terms of the Creative Commons Attribution License (CC BY). The use, distribution or reproduction in other forums is permitted, provided the original author(s) and the copyright owner(s) are credited and that the original publication in this journal is cited, in accordance with accepted academic practice. No use, distribution or reproduction is permitted which does not comply with these terms.
*Correspondence: Lin Huang, bGh1YW5nQG5qZnUuZWR1LmNu