- 1United Graduate School of Agricultural Science, Tokyo University of Agriculture and Technology, Tokyo, Japan
- 2Graduate School of Agriculture, Tokyo University of Agriculture and Technology, Tokyo, Japan
- 3Institute of Genetics, Technische Universität Dresden, Dresden, Germany
Bradyrhizobium elkanii USDA61 is incompatible with mung bean (Vigna radiata cv. KPS1) and soybean (Glycine max cv. BARC2) and unable to nodulate either plant. This incompatibility is due to the presence of a functional type III secretion system (T3SS) that translocates effector protein into host cells. We previously identified five genes in B. elkanii that are responsible for its incompatibility with KPS1 plants. Among them, a novel gene designated as innB exhibited some characteristics associated with the T3SS and was found to be responsible for the restriction of nodulation on KPS1. In the present study, we further characterized innB by analysis of gene expression, protein secretion, and symbiotic phenotypes. The innB gene was found to encode a hypothetical protein that is highly conserved among T3SS-harboring rhizobia. Similar to other rhizobial T3SS-associated genes, the expression of innB was dependent on plant flavonoids and a transcriptional regulator TtsI. The InnB protein was secreted via the T3SS and was not essential for secretion of other nodulation outer proteins. In addition, T3SS-dependent translocation of InnB into nodule cells was confirmed by an adenylate cyclase assay. According to inoculation tests using several Vigna species, InnB promoted nodulation of at least one V. mungo cultivar. These results indicate that innB encodes a novel type III effector controlling symbiosis with Vigna species.
Introduction
Symbiotic relationships between legumes and soil bacteria, collectively called rhizobia, substantially contribute to agricultural production and the nitrogen cycle on the Earth (Broughton et al., 2000; Perret et al., 2000). Rhizobia induce the formation of specialized organs, known as root nodules, and are accommodated within them. The rhizobia in these nodules terminally differentiate into bacteroids, which are capable of reducing atmospheric nitrogen to ammonium assimilated by plants. In exchange, the host plants supply carbon sources and provide an appropriate environment for rhizobia (Oldroyd et al., 2011).
The nodulation process involves a complex exchange of molecular signals between rhizobia and plants that enables the hosts to distinguish compatible rhizobia from potential pathogens. Certain flavonoids exuded by host legume roots interact specifically with the rhizobial protein NodD, which binds to specific promoter sequences (called nod boxes) and activates the transcription of nodulation (nod) genes (Cullimore et al., 2001; Radutoiu et al., 2007). The products of nod genes synthesize rhizobial signal molecules called nodulation factors (NFs). NFs are recognized by host receptors (NF receptors, NFRs) that activate the host-signaling pathway, resulting in rhizobial infection and nodule organogenesis (Cullimore et al., 2001; Radutoiu et al., 2007; Madsen et al., 2010; Miwa and Okazaki, 2017).
Flavonoids from host legumes also induce secretion of specific proteins via the bacterial type III protein secretion system (T3SS). The T3SS was originally identified as a translocation system of effector proteins that function as virulence or avirulence proteins (Hueck, 1998). A similar system has been identified in numerous rhizobia (Viprey et al., 1998; Göttfert et al., 2001; Krause et al., 2002; Krishnan et al., 2003; López-Baena et al., 2008; Okazaki et al., 2009, 2010; Sánchez et al., 2009; Pérez-Montaño et al., 2016). The gene cluster for the rhizobial T3SS (tts) consists of genes encoding a secretion apparatus, secreted proteins and a transcriptional activator TtsI that induces the expression of tts genes by binding to conserved cis-elements termed tts boxes. Rhizobial type III secreted proteins are designated as nodulation outer proteins (Nops) (Krause et al., 2002; Marie et al., 2004; López-Baena et al., 2008; Wassem et al., 2008; Okazaki et al., 2009). Rhizobial T3SSs and secreted effectors, called T3 effectors, are involved in host-range determination and nodulation efficiency (Marie et al., 2003; Deakin and Broughton, 2009). Depending on the host plant, T3 effectors can have a positive, negative or neutral effect on symbiosis (Staehelin and Krishnan, 2015; Miwa and Okazaki, 2017).
Bradyrhizobium elkanii establishes symbiosis with a wide range of legumes, including soybean (Glycine max), mung bean (Vigna radiata), groundnut (Arachis hypogaea) and Aeschynomene spp. We previously reported that the T3SS of B. elkanii promotes nodule formation on soybean cultivar Enrei and Aeschynomene spp. but restricts nodulation on soybean cultivars carrying the Rj4 allele (Faruque et al., 2015) and V. radiata cv. KPS1 (Okazaki et al., 2009). We identified five genes of USDA61 responsible for the incompatibility with V. radiata cv. KPS1 (Nguyen et al., 2017), one of which, designated as innB (incompatible nodulation B), is preceded by a tts box and thus predicted to possess a T3SS-related function. Inoculation assays showed that innB is specifically responsible for the incompatibility with KPS1 but not for Rj4 soybeans (Nguyen et al., 2017). In the present study, we further characterized the innB gene by transcriptional and protein analyses. We also observed infection properties and evaluated its symbiotic roles by inoculating several Vigna species. Our results reveal that innB encodes a novel type III effector that controls symbiosis with Vigna species.
Materials and Methods
Microbiological and Molecular Techniques
The bacterial strains used in this study are listed in Supplementary Table S1. B. elkanii USDA61 and mutant strains were grown at 28°C on arabinose–gluconate (AG) medium (Sadowsky et al., 1987) or peptone salts yeast extract (PSY) medium (Regensburger and Hennecke, 1983) and Escherichia coli strains were grown at 37°C on LB medium (Green and Sambrook, 2012). Antibiotics were added to the media at the following concentrations: for B. elkanii, polymyxin at 50 μg ml-1, kanamycin and streptomycin at 200 μg ml-1; for E. coli, kanamycin and streptomycin at 50 μg ml-1, tetracycline at 10 μg ml-1.
For the construction of InnB-3xFLAG translational fusion, the oligonucleotides 3FLAPstI and 3FLASphI (Supplementary Table S2) were annealed and cloned into the PstI-SphI site of the plasmid pK18mob (Schäfer et al., 1994), generating the plasmid pK18mob3xFLAG. The partial innB fragment was amplified by PCR using primers innB-PstI-fullF and innB-BamHI-fullR and then cloned into pK18mob3xFLAG to generate pInnB7593xFLAG. For the construction of InnB-Cya fusions, the streptomycin-resistance gene (aadA) was amplified by PCR using primers aadAfor and aadArev and then cloned into the DraI sites of the pSLC5 plasmid (Wenzel et al., 2010), generating pSLC5Sm. The innB fragment was amplified by PCR using primers innB-PstI-fullF and innB-BamHI-fullR and cloned into the EcoRI and XbaI sites of pSLC5Sm, generating pInnB759Cya. For complementation, innB and its promoteor region (Accession No. KX499541, Supplementary Data S1) was amplified by PCR using primers BeinnBSacI-InfuF and BeinnBKpnI-InfuR and cloned into the SacI and KpnI sites of the plasmid pBjGroEL4::DsRed2, generating pBjGroEL4::proinnB. The resulting plasmid was mobilized into the innB-deficient mutant BE53 (Nguyen et al., 2017) by conjugation (Krause et al., 2002) using the helper plasmid pRK2013. Integration of the plasmids into the chromosome of B. elkanii strains was confirmed by antibiotic resistance, PCR and sequencing. DsRed- and GusA-tagged B. elkanii strains were obtained by integration of the plasmids pBjGroEL4::DsRed2 (Hayashi et al., 2014) and pCAM120 (Wilson et al., 1995), respectively.
Plant Assays
Seeds of mung bean (V. radiata cv. KPS1), black gram [Vigna mungo (L.) Hepper cv. PI173934] and soybean (G. max cv. Enrei) were surface sterilized and germinated as described previously (Nguyen et al., 2017). One day after transplantation, seedlings were inoculated with B. elkanii strains (1 ml of 107 cells ml-1 per seedling). The plants were grown in a plant growth cabinet (LPH-410SP; NK Systems, Co. Ltd., Osaka, Japan) at 25°C and 70% humidity under a day/night regimen of 16/8 h. The symbiotic phenotypes including nodule number, nodule fresh weight and whole plant fresh weight were examined 8, 15, 30, or 35 days post-inoculation depending on the experiment.
Microscopy
For microscopic analysis, nodules were fixed with 0.1 M cacodylate buffer containing 2.5% (vol/vol) glutaraldehyde in phosphate saline buffer at 4°C overnight. The fixed nodules were embedded in 5% agar and sectioned using a microtome (VT1000s; Leica Biosystems, Germany) and then observed under the microscope (SZX9; Olympus, Japan). GUS staining was performed as described previously (Okazaki et al., 2007).
RNA Extraction and Real-Time RT-PCR
RNAs were extracted from B. elkanii cells as described by Babst et al. (1996). Briefly, B. elkanii strains were grown at 28°C on PSY medium on a rotary shaker at 180 rpm. When OD600 values of the culture reached 0.4, 10 μM of genistein was added and the cultures were sampled at 4 and 24 h, respectively. cDNA synthesis and real-time RT-PCR were performed as described by Yasuda et al. (2016). The RNA isolations and real-time RT-PCR analyses were performed at least twice in triplicate. Transcript levels were normalized to the expression of the housekeeping gene atpD, measured in the same samples (Wen et al., 2016).
Purification and Analysis of Extracellular Proteins
For isolation of extracellular proteins, AG medium was inoculated with 1 : 100 dilution from a B. elkanii preculture and incubated at 28°C for 48 h. Supernatants were recovered from 500 ml of the bacterial cultures by two rounds of centrifugation (4000 ×g at 4°C for 1 h and 8000 ×g at 4°C for 30 min). The supernatants were then lyophilized, and extracellular proteins were extracted as described previously (Okazaki et al., 2009, 2010). Extracellular proteins were separated by 15% SDS-PAGE and stained with Coomassie brilliant blue (CBB) as described previously (Okazaki et al., 2010). For western blot analysis, protein samples were separated on a 4–15 or 4–20% SDS polyacrylamide gel, and identification of the target proteins was carried out as described by Hempel et al. (2009) using an antibody raised against FLAG and NopA. Briefly, the protein samples were transferred to polyvinylidene difluoride (PVDF) membranes (Bio-Rad, United States) and blocked with Western Blot Blocking Buffer (Fish Gelatin) (Takara, Japan). The immunoreaction was detected using an ECL Prime Kit (GE Healthcare, United Kingdom) and a LAS-3000 Luminescent Image Analyzer (Fujifilm, Japan).
Adenylate Cyclase (Cya) Assay
To quantify cAMP levels, root nodules were collected at 18 dpi, immediately frozen in liquid nitrogen and ground to a fine powder. The nodule powder was suspended in a 5× volume of 0.1 M HCl (per nodule weight) and centrifuged. The supernatant was diluted to quantify cAMP concentrations in the detection range and subjected directly to cAMP measurement using a cyclic AMP (direct) enzyme immunoassay (EIA) kit (Cayman Chemical Company, Ann Arbor, MI, United States) according to the manufacturer’s instructions.
Bioinformatic and Statistical Analysis
Amino acid sequences were aligned using MUSCLE or CLUSTAL W algorithms. A phylogenetic tree was constructed by the neighbor-joining method in MEGA 7.0 (Kumar et al., 2016). For statistical analysis, the data were subjected to analysis of variance (ANOVA), and a post hoc test (Fisher’s test at p ≤ 0.05) was done using Minitab statistical software version 16.0.
Results
InnB Is Exclusively Conserved Among Rhizobia
We previously demonstrated that innB of B. elkanii USDA61 was responsible for host-specific nodulation restriction on V. radiata cv. KPS1 (Nguyen et al., 2017). The presence of a conserved tts box motif in the promoter region of innB suggested its T3SS-related function (Supplementary Data S1). In addition, the N-terminal region of InnB exhibits characteristics of T3-secreted proteins: as detailed by Guttman et al. (2002) and Petnicki-Ocwieja et al. (2002), a high percentage of serines and the aliphatic amino acids proline and valine as the third or fourth residues (Supplementary Figure S1).
A BLASTP search revealed that homologs of InnB are present in T3SS-harboring rhizobia, but not in T3SS-harboring pathogens (Figure 1). Because the InnB homologs were highly diverse in length, we divided the selected homologs into high- and low-query-coverage groups and analyzed their phylogenetic relationships separately. Phylogenetic analysis of the high-query-coverage homologs uncovered three clearly distinct branches. InnB and its homologs in B. elkanii USDA76 (WP_018270178, 100% identity) and Bradyrhizobium sp. R5 (SDD80475, 74% identity) were separate from the two groups comprising Mesorhizobium and other Bradyrhizobium strains (Figure 1A). We also discovered three Mesorhizobium species containing InnB homologs, but their similarity to InnB was low. InnB homologs with a low query coverage were split into four different groups: Mesorhizobium strains, B. elkanii USDA61, B. diazoefficiens USDA110 and B. japonicum USDA6, B. diazoefficiens USDA122 and B. japonicum Is-34 (Figure 1B).
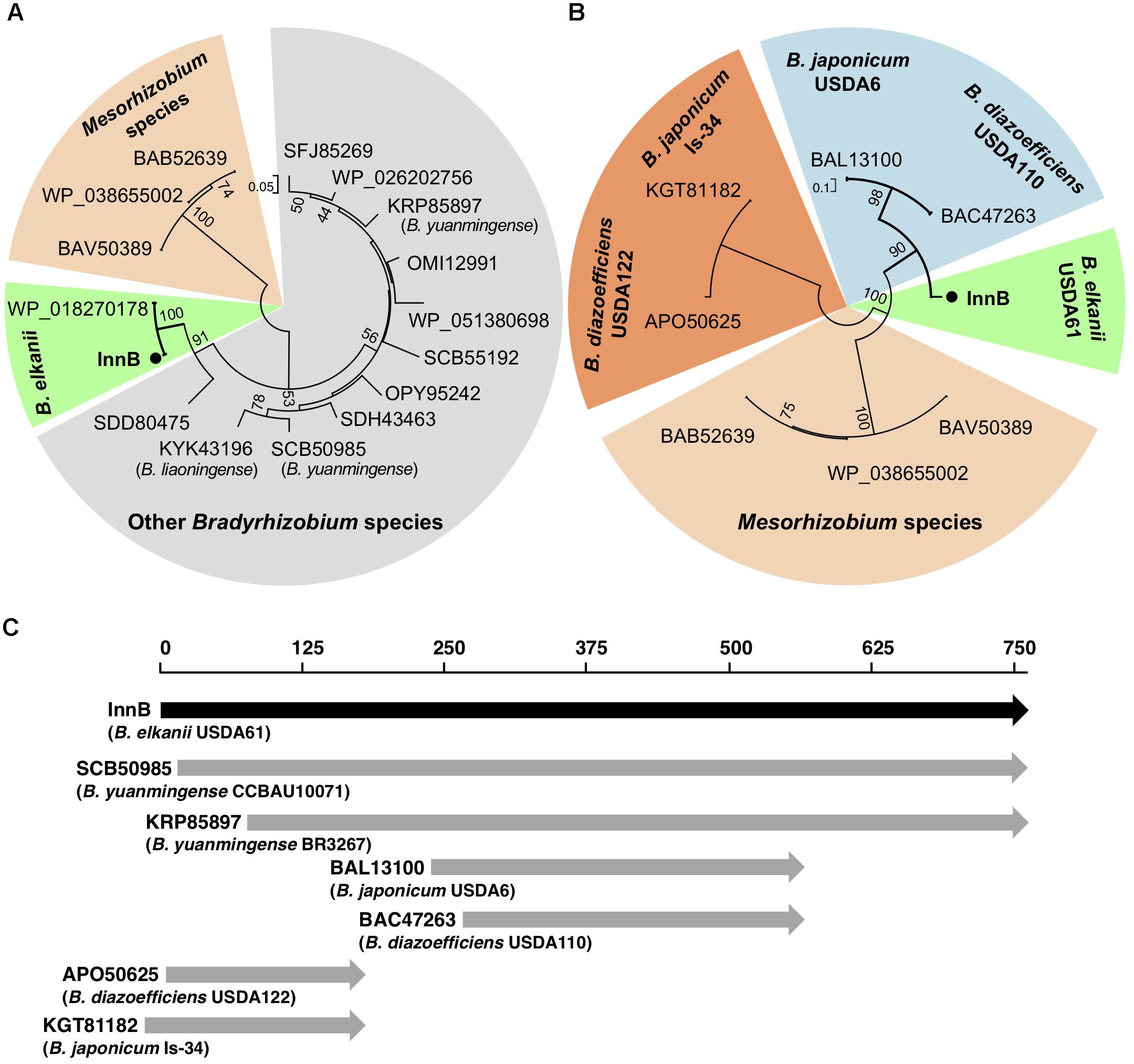
Figure 1. Phylogenetic trees and comparative alignment of InnB and its homologs. (A,B) Phylogenetic trees of homologs having a query coverage of at least 80% (A) and less than 80% (B). (C) Alignment of InnB and its homologs in several rhizobial strains.
An amino acid alignment was conducted using selected homologs, namely, those in B. yuanmingense CCBAU10071 (SCB50985, 72% identity), B. yuanmingense BR3267 (KRP85897, 73% identity, not annotated), B. japonicum USDA6 (BAL13100, 71% identity), B. diazoefficiens USDA110 (BAC47263, 70% identity), B. diazoefficiens USDA122 (APO50625, 70% identity) and B. japonicum Is-34 (KGT81182, 70% identity) (Figure 1C and Supplementary Figure S1). The homologs in two strains, B. yuanmingense CCBAU10071 (SCB50985, query coverage 98%) and BR3267 (KRP85897, query coverage 89%), had many highly conserved amino acids that could be aligned with the InnB amino acid sequence. In contrast, the homologs in B. diazoefficiens and B. japonicum strains with a query coverage lower than 80% aligned with different parts of InnB (Figure 1C and Supplementary Figure S1). Interestingly, the homologs BAL13100 (in USDA6) and BAC47263 (in USDA110) were highly similar to the internal part of InnB, while those in USDA122 (APO50625) and Is-34 (KGT81182) aligned well with the N-terminus of InnB. All four of these homologs lacked amino acids that aligned with the amino acid residues 569 to 759 of InnB (Supplementary Figure S1).
Expression of innB Is Dependent on Genistein and the Transcriptional Regulator TtsI
The presence of a conserved tts box motif in the promoter region of innB suggests that its expression is controlled by TtsI and host-derived flavonoids. We therefore analyzed the expression of innB in the wild-type USDA61 and the ttsI-deficient mutant BEttsI (Okazaki et al., 2013) in the presence and absence of genistein by real-time RT-PCR. Transcriptional levels of innB, nopA, and nodC genes in the wild-type background were increased upon induction with genistein (Figure 2). The innB and nopA showed a very similar expression and induction pattern with significantly higher expression after 24 h (Figure 2 and Supplementary Figure S2). In BEttsI, the expression of innB was completely abolished along with that of nopA, which suggests that innB expression was controlled by genistein and TtsI. Intriguingly, the expression of nodC was slightly reduced in the ttsI mutant background. However, it is unknown how TtsI might affect nodC expression.
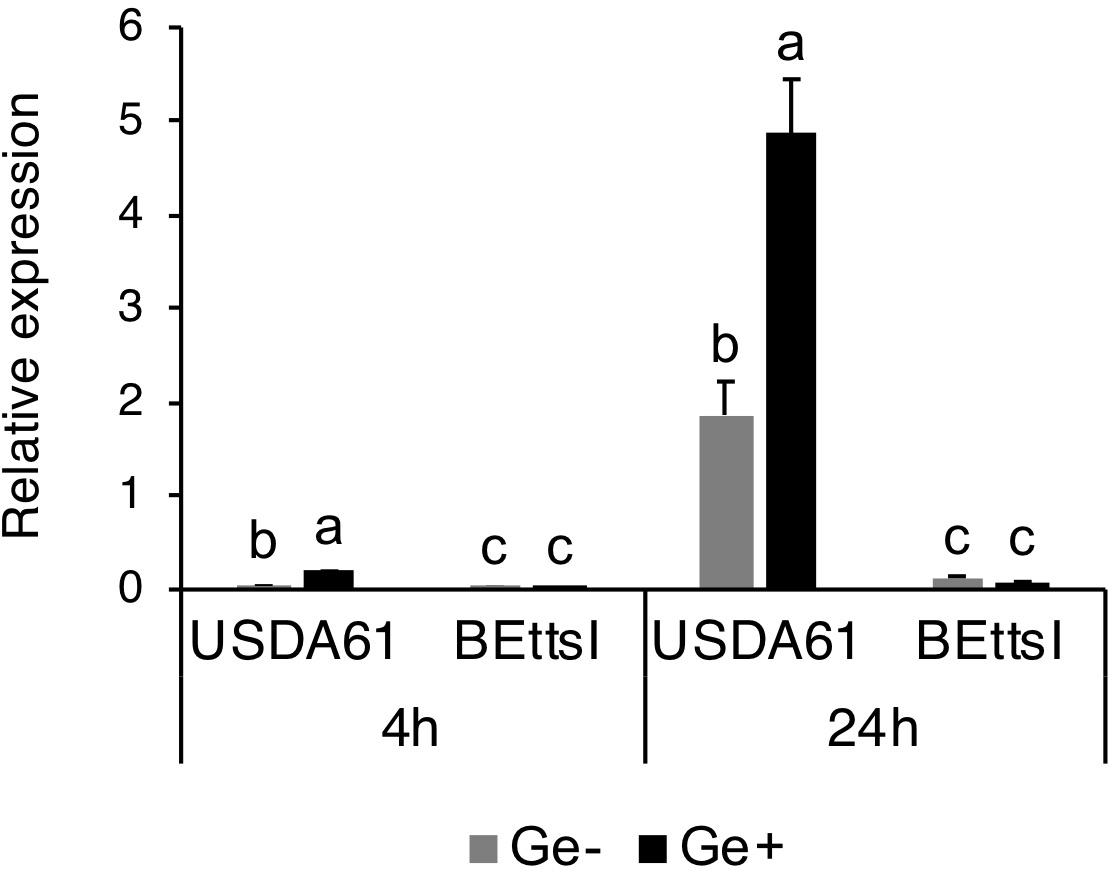
Figure 2. Expression of innB in wild-type and mutant Bradyrhizobium elkanii strains. Real-time RT-PCR was performed using total RNAs isolated from USDA61 and the ttsI-deficient mutant BEttsI grown in the absence (Ge–) or presence (Ge+) of the inducer flavonoid genistein (10 μM) after 4 and 24 h. The expression level of each gene was normalized relative to the atpD gene (ATP synthase) using the ΔΔCt method. The expression data are the mean of triplicates, and the error bars indicate standard deviations. Statistical analysis by Fisher’s method was performed to compare the relative expression levels of innB in USDA61 and BEttsI in the absence/presence of genistein at each timepoint, respectively. Means followed by different letters at the same timepoint are significantly different at the 5% level.
InnB Is Not Essential for the Secretion of Other Nops
To confirm that InnB is a T3 effector and that mutation of innB does not alter secretion of other Nops, we analyzed the extracellular protein profiles of strains USDA61 and BErhcJ, a mutant defective for T3 protein secretion (Okazaki et al., 2009), and the innB-deficient mutant BE53 in the absence or presence of genistein (10 μM). The proteins from bacterial culture supernatants were extracted and separated by SDS-PAGE (Figure 3). The protein profile of the BErhcJ mutant differed from that of USDA61, with several predicted T3SS-secreted proteins, such as NopA and NopC being absent. In contrast, the protein pattern of BE53 was similar to that of the wild-type USDA61 and secretions of NopA and NopC were detected. These results suggest that the mutation in innB was not essential for the secretion of other Nops. We also examined the secretion of InnB using the FLAG-tagged construct, however, we could not detect the FLAG-tagged InnB in culture supernatants of USDA61 (Supplementary Figure S3).
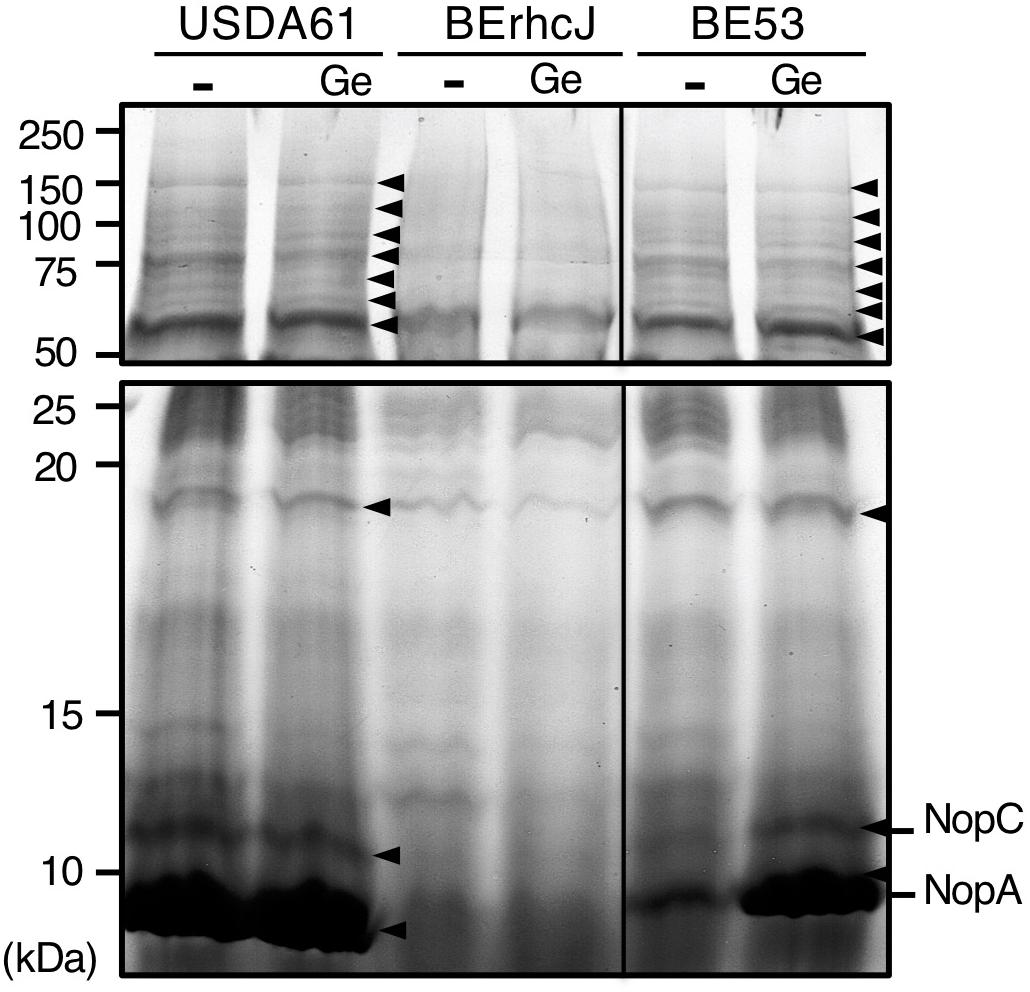
Figure 3. Extracellular protein profiles of B. elkanii strains. USDA61, BErhcJ, and innB-deficient mutant BE53 were cultured in the absence (–) or presence (Ge) of 10 μM genistein. The extracellular proteins were stained with Coomassie brilliant blue (CBB). Proteins were separated by 15% SDS-PAGE. Size-marker molecular masses (kDa) are shown on the left. Arrowheads indicate protein bands that were visible in cultures of USDA61 and BE53 but not BErhcJ.
InnB Is a T3 Effector Translocated Inside Plant Cells
To confirm whether InnB is a T3 effector that functions in host cells, its translocation was analyzed using adenylate cyclase (Cya) as a reporter. In the presence of ATP and a calmodulin-like protein, cAMP production is catalyzed by the Cya enzyme only within eukaryotic cells (Sory and Cornelis, 1994). Once the Cya-fused effector is delivered to the host cell, cAMP production will be detected. In this study, Cya fused to the carboxy terminus of InnB was integrated into the B. elkanii strains USDA61 and BErhcJ, resulting in the mutants BEinnBC and BErhcJinnBC, respectively. The translocation of InnB was confirmed using fresh nodules of the USDA61-compatible cultivar G. max cv. Enrei harvested at 18 dpi. A low level of cAMP was detected in nodules induced by the wild-type USDA61 and the BErhcJinnBC mutant expressing the InnB-Cya fusion (Figure 4). However, the cAMP level was significantly higher in nodules formed by USDA61 containing the InnB-Cya fusion, which suggests that InnB was translocated into the host cell.
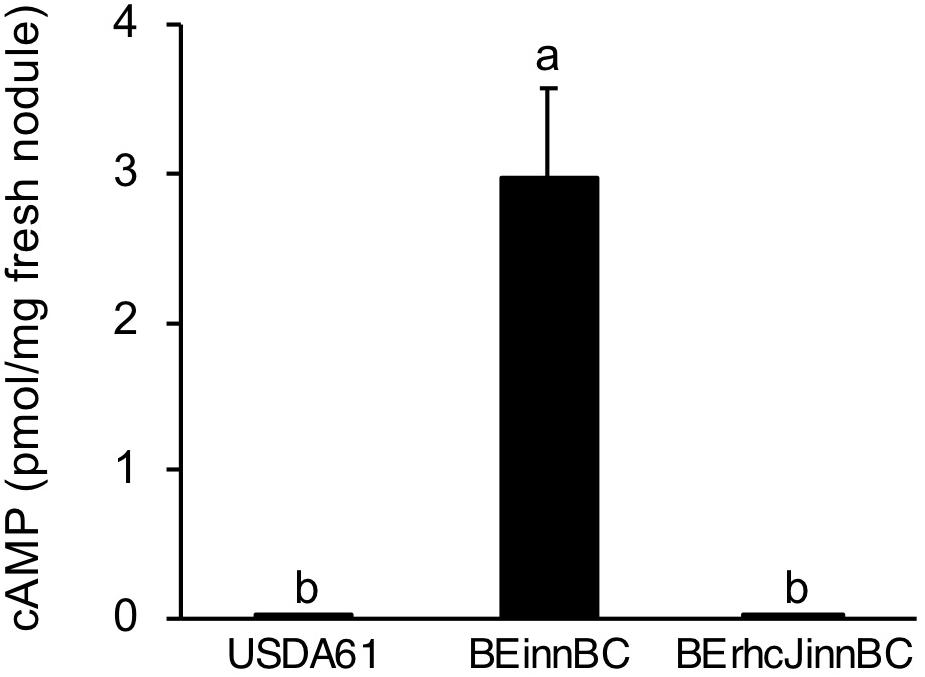
Figure 4. cAMP levels measured in nodules harvested at 18 dpi from the USDA61-compatible plant Glycine max cv. Enrei. Plants were inoculated with the rhizobial strains BEinnBC and BErhcJinnBC, which carry an innB-cya fusion in the USDA61 and BErhcJ background, respectively. The wild-type USDA61 contained no cya reporter. Nodules were randomly collected from at least four plants individually inoculated with each strain. The data are means of triplicates, and the error bars indicate standard deviations. Statistical analysis by Fisher’s method was performed to compare cAMP levels. Means followed by the different letters are significantly different at the 5% level.
InnB Negatively Affects Nodulation of V. radiata
We previously reported that the insertion of a transposon in innB abolishes nodulation incompatibility between USDA61 and KPS1 (Nguyen et al., 2017). To confirm that the symbiotic phenotype was induced by inactivation of innB and not by an additional mutation, we complemented the BE53 mutant with the innB gene and its promoter region. The resulting complemented strain exhibited a symbiotic phenotype similar to wild-type USDA61 (Supplementary Figure S4). These results confirm that innB was responsible for the altered nodulation phenotype on KPS1.
To further characterize the negative impact of InnB on nodulation, we monitored the nodulation process of KPS1 inoculated with DsRed-tagged B. elkanii strains. Early in nodulation (8 dpi) wild-type and mutant induced a similar number of nodule primordia (Figure 5A). However, at later stages (15 and 30 dpi), plants developed a higher number of nodule primordia if infected with the wild-type as compared to the infection with the innB mutant (5- to 20-fold). In contrast, almost no nodules were formed with the wild-type strain but the innB mutant was a very efficient nodulator (Figures 5A–C). In line with that, nodule weight and plant weight were significantly increased with BE53Ds-inoculated plants (Figure 5D). Similar results for the time point 40 dpi were observed previously (Nguyen et al., 2017).
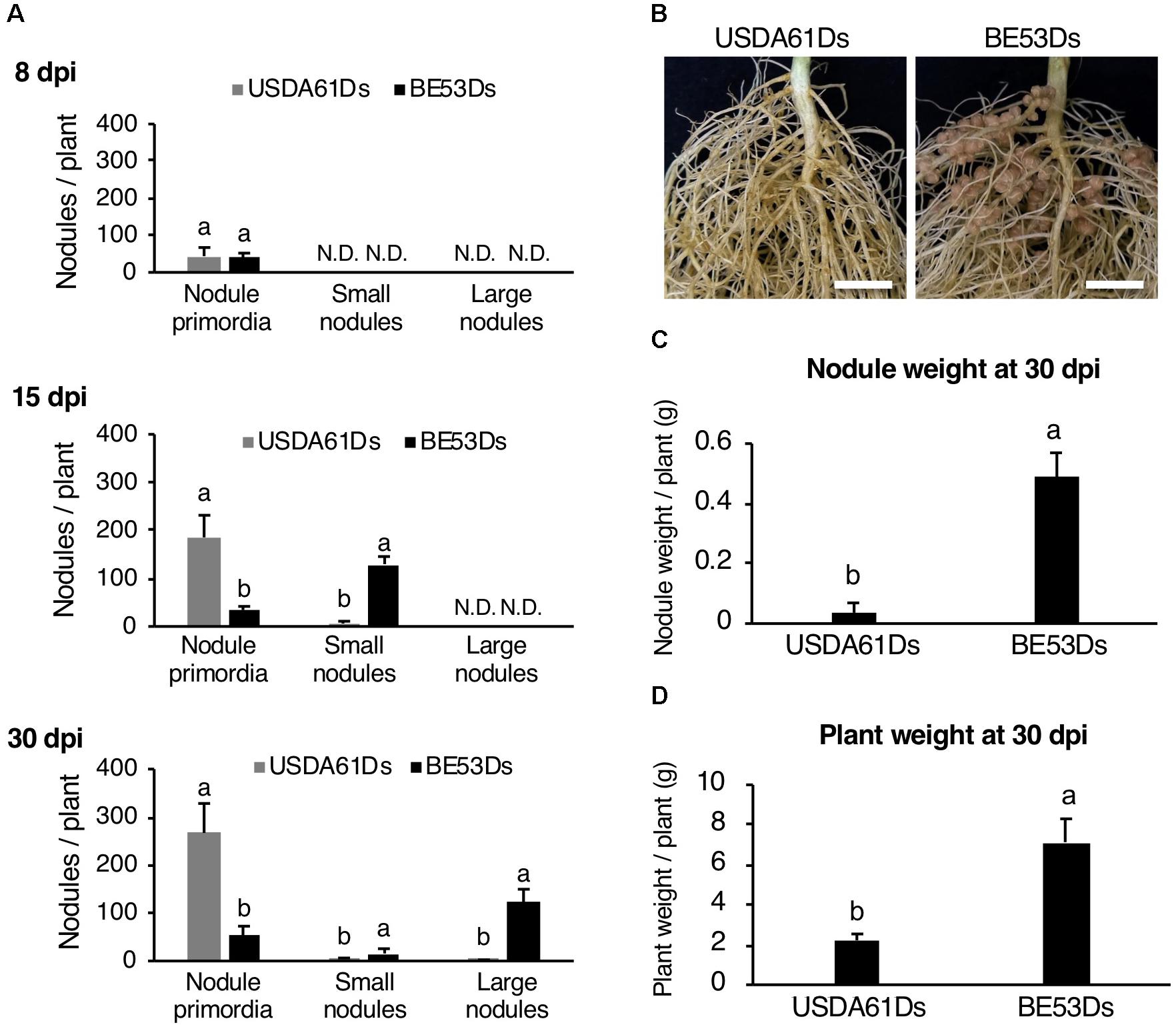
Figure 5. Nodulation properties of Vigna radiata cv. KPS1 inoculated with the B. elkanii USDA61Ds and BE53Ds. (A) The number of nodule primordia and small and large nodules at 8, 15, and 30 dpi. N.D., not detected. (B) Roots of KSP1 plants at 30 dpi; scale bars: 1 cm. (C) Nodule weight and (D) plant weight of KPS1 plants at 30 dpi. The data shown are the means of at least five plants, and the error bars indicate standard deviations. Statistical analysis by Fisher’s method was performed to compare the symbiotic phenotypes obtained with USDA61Ds and BE53Ds. Means followed by different letters are significantly different at the 5% level.
InnB Abolishes Infection and Nodule Organogenesis in V. radiata
To explore the effect of innB on infective properties of B. elkanii, we investigated the infection process in KPS1 plants using GUS-tagged strains of USDA61 and BE53. Examination of early infection events in KPS1 roots revealed that USDA61 and BE53 both infected KPS1 root hairs and formed nodule primordia. However, the number of infected nodule primordia and small nodules was significantly different between USDA61 and BE53 (Figure 6). At 8 dpi, BE53 efficiently infected KPS1 roots, thereby resulting in a higher number of infected nodule primordia than those of the wild-type. Most nodule primordia in KPS1 plants inoculated with USDA61 were uninfected (Figure 6). In contrast to the infection phenotypes observed at 8 dpi, USDA61 induced a higher number of infected nodule primordia at 15 dpi than did BE53G. Many efficiently infected small nodules instead were formed on roots of KPS1 inoculated with BE53 (Figure 6). The small nodules formed by all plants inoculated with BE53 were deep blue, probably because of the high number of active rhizobia inside (Figure 6).
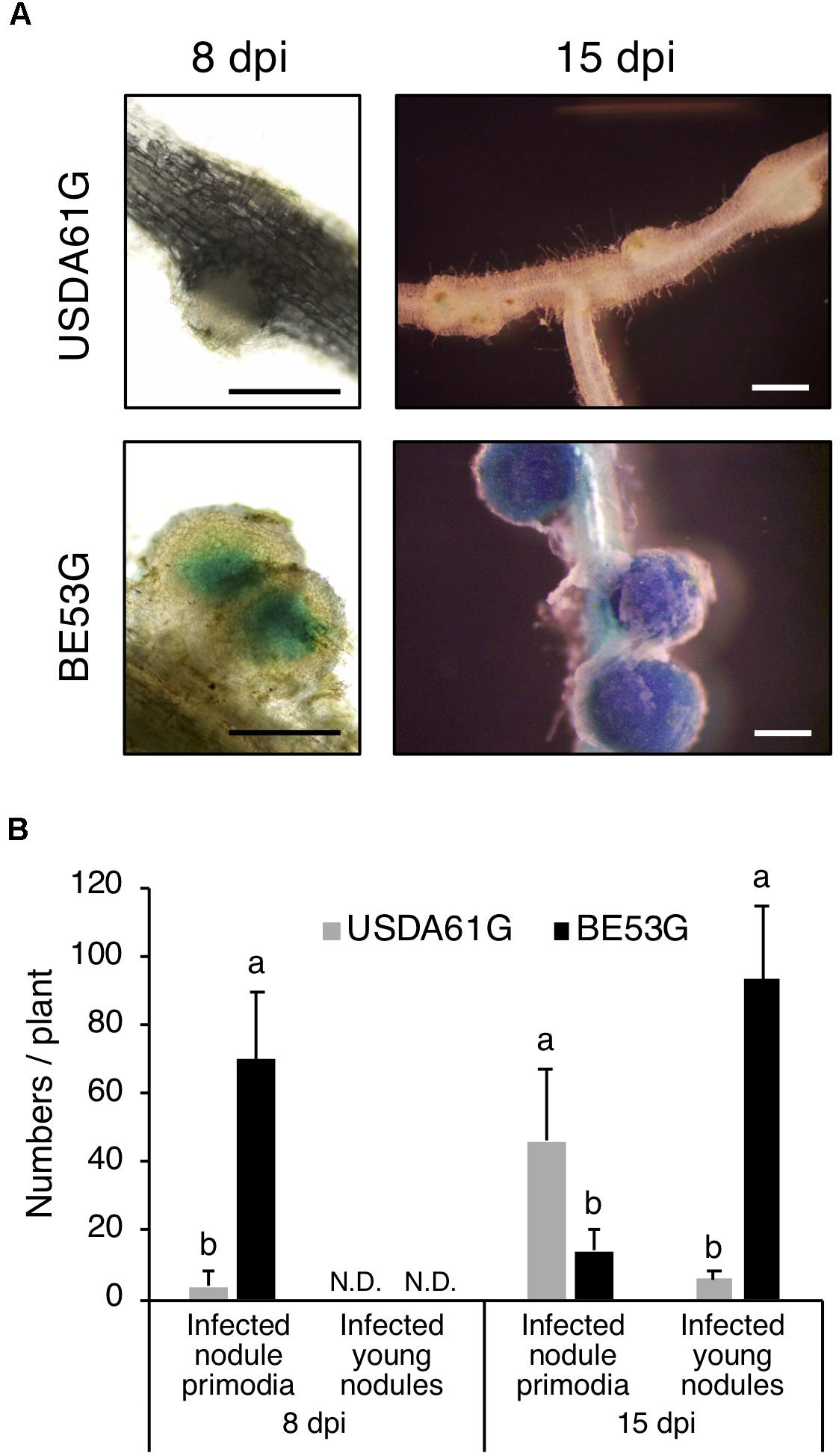
Figure 6. Infection properties of V. radiata cv. KPS1 inoculated with Bradyrhizobium elkaii USDA61G and BE53G. (A) Infected nodule primordia and small nodules. Scale bars: 500 μm. (B) The number of infected nodule primordia and small nodules formed on KPS1 plants. The data shown are means of five plants, and the error bars indicate standard deviations. Means followed by different letters at the same timepoint (dpi) are significantly different at the 5% level.
InnB Promotes Nodulation of V. mungo
To explore the importance of InnB in symbiosis with legumes, we conducted inoculation tests using several Vigna species. Among these species, V. mungo (L.) Hepper cv. PI173934 formed numerous nodules following inoculation with USDA61 (approximately 140 nodules per plant) (Figure 7). In contrast, BErhcJ and BE53 induced significantly lower numbers of nodules, and plant weights were decreased. These observations indicate that the T3SS promotes symbiosis with V. mungo and that InnB is one of the positive effectors for the symbiosis. Intriguingly, BE53 induced a significantly higher number of small nodules than BErhcJ, which suggests that USDA61 harbors an additional positive effector.
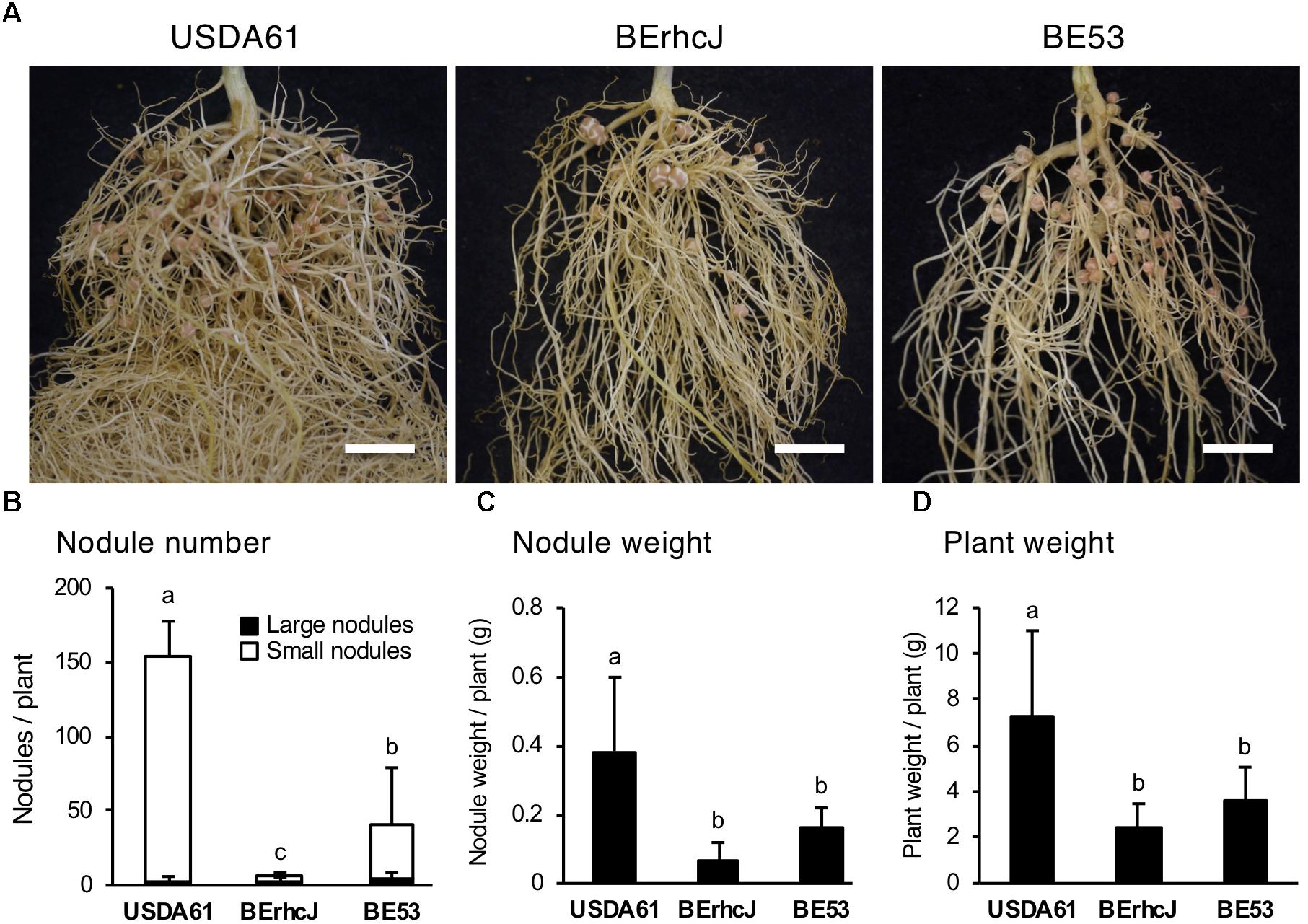
Figure 7. Symbiotic properties of Vigna mungo cv. PI173934 (black gram) inoculated with B. elkanii strains. (A) Roots of black gram inoculated with B. elkanii strains; scale bars: 1 cm. Nodule number (B), fresh nodule weight (C), and fresh plant weight (D) of V. mungo at 35 dpi. White and black bars in (B) represent the number of small (<2 mm) and large (≥2 mm) nodules, respectively. The data shown are the means of at least 10 plants from two independent assays. The error bars indicate standard deviations. Means followed by different letters are significantly different at the 5% level.
Discussion
We previously identified five novel B. elkanii genes that are responsible for the incompatibility with V. radiata KPS1. One of the genes, innB, encodes a hypothetical protein that contains a tts box in its promoter region and thus seems to possess T3SS-related functions (Nguyen et al., 2017). As previously reported, the innB mutant BE53 can nodulate KPS1 but not Rj4 soybean (Nguyen et al., 2017), which indicates that InnB causes a highly specific incompatibility with KPS1 but not with Rj4 plants. In the present study, we analyzed the innB gene by examining gene expression, protein secretion and symbiotic phenotypes.
Homologs of the InnB protein were found in T3SS-harboring rhizobia, but not in other plant-associated bacteria. Other rhizobial T3-secreted proteins, such as NopC, NopI, NopL and NopP, have also been identified as Rhizobium-specific and have no homologs in plant or animal pathogens (Deakin and Broughton, 2009; Jiménez-Guerrero et al., 2015). These reports imply that InnB has a symbiosis-specific function. Notably, homologs in B. japonicum (strains USDA6 and Is-34) and B. diazoefficiens (strains USDA110 and USDA122) are highly similar to either the N-terminal or internal region of InnB (Figure 1 and Supplementary Figure S1). Our preliminary experiment in agreement with Sugawara et al. (2018) showed that USDA110 was not incompatible with KPS1, thus suggesting that the homolog of USDA110 (BAC47263) lacks a functional domain for inducing incompatibility. In contrast, InnB homologs in other bradyrhizobia, such as B. elkanii strain USDA76 and B. yuanmingense strains CCBAU10071 and BR3267, showed a high degree of query coverage, suggesting that these proteins have common functions in symbiosis.
Similar to other nop genes, the transcription of innB was found to be regulated by flavonoids and the transcriptional regulator TtsI. In addition, the different transcriptional levels of nodC in the absence and presence of genistein in the wild-type and ttsI mutant suggest that nodC expression is slightly reduced in a TtsI mutant background (Figure 2 and Supplementary Figure S2). The mechanism underlying the reduced nodC expression remains unclear. Notably, the transcriptional levels of nopA and nodC are lower than that of innB, suggesting that they might function differently in protein secretion and nodulation process (Supplementary Figure S2). Although transcription of tts genes were clearly inducible by genistein, the addition of genistein did not significantly change the extracellular protein patterns of B. elkanii strains (Figure 3). These results suggest that the T3SS is activated without genistein under the tested conditions. Therefore, unidentified regulators or inducers are likely be involved in the regulation of tts genes in B. elkanii.
An analysis of extracellular proteins confirmed that InnB is not essential for the secretion of other proteins. It has been shown that the mutations of genes encoding T3SS components, such as nopA and nopB, significantly affect the secretion of other secreted proteins and nodule formation (Deakin et al., 2005). In our experiment, Nops, such as NopA, NopC and NopX, were visible in both BE53 and USD61 extracellular protein samples, but not in those of T3SS-deficient BErhcJ (Figure 3), thus indicating that InnB is a secreted protein and not a component of the secretion apparatus.
The InnB protein, which was predicted to have a molecular mass of approximately 83.11 kDa, was not clearly detected by SDS-PAGE (Figure 3). To further confirm the secretion of innB, we constructed a 3xFLAG-tagged innB. The generated construct was transferred to either USAD61 or a ttsI mutant, generating BEinnBF and BEttsIinnBF, respectively. However, we could not detect InnB fragment in the culture supernatant of both strains by western blotting (Supplementary Figure S3B). Notably, the InnB homolog in B. diazoefficiens USDA110 was also not detected in the extracellular proteins of USDA110 (Hempel et al., 2009). It is likely that the InnB protein was secreted at quite low levels or secreted InnB proteins undergo a certain modification or degradation.
The translocation of InnB into soybean nodule cells was verified by a Cya reporter assay (Figure 4). Translocation of rhizobial T3 effectors such as NGR234 NopP (Schechter et al., 2010), NopC (Jiménez-Guerrero et al., 2015), HH103 NopL (Jiménez-Guerrero et al., 2017), and USDA110 NopE (Wenzel et al., 2010) into host cells has been similarly confirmed by Cya assays. The InnB-induced cAMP accumulation observed in our study was much lower than that of other Nops. This result may reflect the lower secretion and translocation of InnB compared with other Nops.
In V. radiata, USDA61 induced numerous nodule primordia, but the presence of InnB halted the subsequent nodulation process. While BE53 induced a higher number of infected small nodules on KPS1 roots at 15 dpi, the number of nodule primordia formed by USDA61 increased continuously until 30 dpi (Figures 5, 6). The failure of small nodule formation correlates well with an increase in nodule primordia formation (Figure 5). Taken together, KPS1 halted USDA61 infection and nodule organogenesis before small nodule formation due to the presence of InnB. Probably, the InnB secreted into host cells interacts specifically with Vigna resistance (R) genes and triggers immune responses (effector-triggered immunity), thus resulting in host defense responses and consequently halting infection and nodulation by USDA61.
Although nodulation on KPS1 by USDA61 was heavily restricted, a few nodules were occasionally observed on the roots (Figure 5). According to previous research, USDA61 can infect host legumes such as Arachis hypogaea (Ibáñez and Fabra, 2011) and Aeschynomene spp. (Bonaldi et al., 2011) via “crack entry” (intercellular infection). In soybean, infection via crack entry to form nodules is mediated by the T3SS of B. elkanii and may induce a defense response in Rj4 plants (Okazaki et al., 2013; Yasuda et al., 2016). However, T3SS-mediated crack entry infection is rare and weak and only results in the formation of several small nodules or bumps (Yasuda et al., 2016). In contrast, rhizobial infection through infection threads (ITs) can induce a stronger host defense that is completely able to block nodule formation (Yasuda et al., 2016). We observed that USDA61, but not the innB-deficient mutant, failed to induce root hair curling and only weakly infected KPS1 roots via ITs; nevertheless, a few large nodules occasionally formed at the base of lateral roots (Supplementary Figure S5). In addition, most USDA61-induced nodules were more poorly infected than those induced by BE53 (Supplementary Figure S5). These observations imply that the T3 effector-triggered host defense occasionally fails to completely block the nodule formation of USDA61-infected cells. In these cases, the USDA61 infection may occur via crack entry of the lateral roots of KPS1.
In V. mungo, BE53 induced less nodules than USDA61, indicating that the InnB plays a beneficial role in the symbiosis with this species. It was reported that the V. mungo plants were nodulated by Bradyrhizobium spp. (closely related with B. yuanmingense) but not by B. japonicum or B. diazoefficiens (Appunu et al., 2009). Notably, InnB homologs with a high coverage ratio were specifically found in Bradyrhizobium spp., but not in B. japonicum or B. diazoefficiens (Figure 1). These suggest that the symbionts of V. mungo specifically possess InnB for enhancing the interaction with this host plants. Intriguingly, the number of BE53-formed small nodules is higher than that formed by infection with BErhcJ, implying the presence of an additional positive effector. Further studies are required to elucidate the specific functions of the Rhizobium-specific T3 effector InnB and the additional effector in nodulation among different legumes.
In summary, we have presented evidence that the innB gene of B. elkanii encodes a novel T3 effector involved in the control of symbiosis with Vigna species. The conservation of InnB homologs among rhizobia suggests a symbiosis-specific function for innB, especially in interactions between bradyrhizobia and Vigna spp.
Author Contributions
HN and SO designed the research and wrote the paper. HN performed the research and analyzed the data. SR, MY, and MG contributed new reagents and analytic tools.
Funding
This work was supported in part by JSPS KAKENHI (Grant Nos. JP15KK0276 and JP16H04889) and JSPS Bilateral Program (Joint Research Projects) (Grant No. 16932112).
Conflict of Interest Statement
The authors declare that the research was conducted in the absence of any commercial or financial relationships that could be construed as a potential conflict of interest.
Acknowledgments
We thank K. Suzuki for her kind help.
Supplementary Material
The Supplementary Material for this article can be found online at: https://www.frontiersin.org/articles/10.3389/fmicb.2018.03155/full#supplementary-material
References
Appunu, C., N’Zoue, A., Moulin, L., Depret, G., and Laguerre, G. (2009). Vigna mungo, V. radiata and V. unguiculata plants sampled in different agronomical–ecological–climatic regions of India are nodulated by Bradyrhizobium yuanmingense. Syst. Appl. Microbiol. 32, 460–470. doi: 10.1016/J.SYAPM.2009.05.005
Babst, M., Hennecke, H., and Fischer, H.-M. (1996). Two different mechanisms are involved in the heat-shock regulation of chaperonin gene expression in Bradyrhizobium japonicum. Mol. Microbiol. 19, 827–839. doi: 10.1046/j.1365-2958.1996.438968.x
Bonaldi, K., Gargani, D., Prin, Y., Fardoux, J., Gully, D., Nouwen, N., et al. (2011). Nodulation of Aeschynomene afraspera and A. indica by photosynthetic Bradyrhizobium sp. strain ORS285: the nod-dependent versus the nod-independent symbiotic interaction. Mol. Plant Microbe. Interact. 24, 1359–1371. doi: 10.1094/MPMI-04-11-0093
Broughton, W. J., Jabbouri, S., and Perret, X. (2000). Keys to symbiotic harmony. J. Bacteriol. 182, 5641–5652. doi: 10.1128/JB.182.20.5641-5652.2000
Cullimore, J. V., Ranjeva, R., and Bono, J.-J. (2001). Perception of lipo-chitooligosaccharidic Nod factors in legumes. Trends Plant Sci. 6, 24–30. doi: 10.1016/S1360-1385(00)01810-0
Deakin, W. J., and Broughton, W. J. (2009). Symbiotic use of pathogenic strategies: rhizobial protein secretion systems. Nat. Rev. Microbiol. 7, 312–320. doi: 10.1038/nrmicro2091
Deakin, W. J., Marie, C., Saad, M. M., Krishnan, H. B., and Broughton, W. J. (2005). NopA is associated with cell surface appendages produced by the type III secretion system of Rhizobium sp. strain NGR234. Mol. Plant Microbe. Interact. 18, 499–507. doi: 10.1094/MPMI-18-0499
Faruque, O. M., Miwa, H., Yasuda, M., Fujii, Y., Kaneko, T., Sato, S., et al. (2015). Identification of Bradyrhizobium elkanii genes involved in incompatibility with soybean plants carrying the Rj4 allele. Appl. Environ. Microbiol. 81, 6710–6717. doi: 10.1128/AEM.01942-15
Göttfert, M., Röthlisberger, S., Kündig, C., Beck, C., Marty, R., and Hennecke, H. (2001). Potential symbiosis-specific genes uncovered by sequencing a 410-kilobase DNA region of the Bradyrhizobium japonicum chromosome. J. Bacteriol. 183, 1405–1412. doi: 10.1128/JB.183.4.1405-1412.2001
Green, M. R., and Sambrook, J. (2012). Molecular Cloning?: A Laboratory Manual. Cold Spring Harbor, NY: Cold Spring Harbor Laboratory Press.
Guttman, D. S., Vinatzer, B. A., Sarkar, S. F., Ranall, M. V., Kettler, G., and Greenberg, J. T. (2002). A functional screen for the type III (Hrp) secretome of the plant pathogen Pseudomonas syringae. Science 295, 1722–1726. doi: 10.1126/science.295.5560.1722
Hayashi, M., Shiro, S., Kanamori, H., Mori-Hosokawa, S., Sasaki-Yamagata, H., Sayama, T., et al. (2014). A thaumatin-like protein, Rj4, controls nodule symbiotic specificity in soybean. Plant Cell Physiol. 55, 1679–1689. doi: 10.1093/pcp/pcu099
Hempel, J., Zehner, S., Göttfert, M., and Patschkowski, T. (2009). Analysis of the secretome of the soybean symbiont Bradyrhizobium japonicum. J. Biotechnol. 140, 51–58. doi: 10.1016/j.jbiotec.2008.11.002
Hueck, C. J. (1998). Type III protein secretion systems in bacterial pathogens of animals and plants. Microbiol. Mol. Biol. Rev. 62, 379–433.
Ibáñez, F., and Fabra, A. (2011). Rhizobial Nod factors are required for cortical cell division in the nodule morphogenetic programme of the Aeschynomeneae legume Arachis. Plant Biol. 13, 794–800. doi: 10.1111/j.1438-8677.2010.00439.x
Jiménez-Guerrero, I., Pérez-Montaño, F., Medina, C., Ollero, F. J., and López-Baena, F. J. (2015). NopC is a Rhizobium-specific type 3 secretion system effector secreted by Sinorhizobium (Ensifer) fredii HH103. PLoS One 10:e0142866. doi: 10.1371/journal.pone.0142866
Jiménez-Guerrero, I., Pérez-Montaño, F., Medina, C., Ollero, F. J., and López-Baena, F. J. (2017). The Sinorhizobium (Ensifer) fredii HH103 nodulation outer protein NopI is a determinant for efficient nodulation of soybean and cowpea plants. Appl. Environ. Microbiol. 83, e02770-16. doi: 10.1128/AEM.02770-16
Krause, A., Doerfel, A., and Göttfert, M. (2002). Mutational and transcriptional analysis of the type III secretion system of Bradyrhizobium japonicum. Mol. Plant Microbe. Interact. 15, 1228–1235. doi: 10.1094/MPMI.2002.15.12.1228
Krishnan, H. B., Lorio, J., Kim, W. S., Jiang, G., Kim, K. Y., DeBoer, M., et al. (2003). Extracellular proteins involved in soybean cultivar-specific nodulation are associated with pilus-like surface appendages and exported by a type III protein secretion system in Sinorhizobium fredii USDA257. Mol. Plant Microbe. Interact. 16, 617–625. doi: 10.1094/MPMI.2003.16.7.617
Kumar, S., Stecher, G., and Tamura, K. (2016). MEGA7: molecular evolutionary genetics analysis version 7.0 for bigger datasets. Mol. Biol. Evol. 33, 1870–1874. doi: 10.1093/molbev/msw054
López-Baena, F. J., Vinardell, J. M., Pérez-Montaño, F., Crespo-Rivas, J. C., Bellogín, R. A., Espuny, M. D. R., et al. (2008). Regulation and symbiotic significance of nodulation outer proteins secretion in Sinorhizobium fredii HH103. Microbiology 154, 1825–1836. doi: 10.1099/mic.0.2007/016337-0
Madsen, L. H., Tirichine, L., Jurkiewicz, A., Sullivan, J. T., Heckmann, A. B., Bek, A. S., et al. (2010). The molecular network governing nodule organogenesis and infection in the model legume Lotus japonicus. Nat. Commun. 1, 1–12. doi: 10.1038/ncomms1009
Marie, C., Deakin, W. J., Ojanen-Reuhs, T., Diallo, E., Reuhs, B., Broughton, W. J., et al. (2004). TtsI, a key regulator of rhizobium species NGR234 is required for type III-dependent protein secretion and synthesis of rhamnose-rich polysaccharides. Mol. Plant Microbe Interact. 958, 958–966. doi: 10.1094/MPMI.2004.17.9.958
Marie, C., Deakin, W. J., Viprey, V., Kopciñska, J., Golinowski, W., Krishnan, H. B., et al. (2003). Characterization of Nops, nodulation outer proteins, secreted via the type III secretion system of NGR234. Mol. Plant Microbe Interact. 16, 743–751. doi: 10.1094/MPMI.2003.16.9.743
Miwa, H., and Okazaki, S. (2017). How effectors promote beneficial interactions. Curr. Opin. Plant Biol. 38, 148–154. doi: 10.1016/j.pbi.2017.05.011
Nguyen, H., Miwa, H., Kaneko, T., Sato, S., and Okazaki, S. (2017). Identification of Bradyrhizobium elkanii genes involved in incompatibility with Vigna radiata. Genes 8:374. doi: 10.3390/genes8120374
Okazaki, S., Hattori, Y., and Saeki, K. (2007). The Mesorhizobium loti purB gene is involved in infection thread formation and nodule development in Lotus japonicus. J. Bacteriol. 189, 8347–8352. doi: 10.1128/JB.00788-07
Okazaki, S., Kaneko, T., Sato, S., and Saeki, K. (2013). Hijacking of leguminous nodulation signaling by the rhizobial type III secretion system. Proc. Natl. Acad. Sci. U.S.A. 110, 17131–17136. doi: 10.1073/pnas.1302360110
Okazaki, S., Okabe, S., Higashi, M., Shimoda, Y., Sato, S., Tabata, S., et al. (2010). Identification and functional analysis of type III effector proteins in Mesorhizobium loti. Mol. Plant Microbe Interact. 23, 223–234. doi: 10.1094/MPMI-23-2-0223
Okazaki, S., Zehner, S., Hempel, J., Lang, K., and Göttfert, M. (2009). Genetic organization and functional analysis of the type III secretion system of Bradyrhizobium elkanii. FEMS Microbiol. Lett. 295, 88–95. doi: 10.1111/j.1574-6968.2009.01593.x
Oldroyd, G. E. D., Murray, J. D., Poole, P. S., and Downie, J. A. (2011). The rules of engagement in the legume-rhizobial symbiosis. Annu. Rev. Genet. 45, 119–144. doi: 10.1146/annurev-genet-110410-132549
Pérez-Montaño, F., Jiménez-Guerrero, I., Acosta-Jurado, S., Navarro-Gómez, P., Ollero, F. J., Ruiz-Sainz, J. E., et al. (2016). A transcriptomic analysis of the effect of genistein on Sinorhizobium fredii HH103 reveals novel rhizobial genes putatively involved in symbiosis. Sci. Rep. 6, 1–12. doi: 10.1038/srep31592
Perret, X., Staehelin, C., and Broughton, W. J. (2000). Molecular basis of symbiotic promiscuity. Microbiol. Mol. Biol. Rev. 64, 180–201. doi: 10.1128/MMBR.64.1.180-201.2000
Petnicki-Ocwieja, T., Schneider, D. J., Tam, V. C., Chancey, S. T., Shan, L., Jamir, Y., et al. (2002). Genomewide identification of proteins secreted by the Hrp type III protein secretion system of Pseudomonas syringae pv. tomato DC3000. Proc. Natl. Acad. Sci. U.S.A. 99, 7652–7657. doi: 10.1073/pnas.112183899
Radutoiu, S., Madsen, L. H., Madsen, E. B., Jurkiewicz, A., Fukai, E., Quistgaard, E. M. H., et al. (2007). LysM domains mediate lipochitin-oligosaccharide recognition and Nfr genes extend the symbiotic host range. EMBO J. 26, 3923–3935. doi: 10.1038/sj.emboj.7601826
Regensburger, B., and Hennecke, H. (1983). RNA polymerase from Rhizobium japonicum. Arch. Microbiol. 135, 103–109. doi: 10.1007/BF00408017
Sadowsky, M. J., Tully, R. E., Cregan, P. B., and Keyser, H. H. (1987). Genetic diversity in Bradyrhizobium japonicum serogroup 123 and its relation to genotype-specific nodulation of soybean. Appl. Environ. Microbiol. 53,2624–2630.
Sánchez, C., Iannino, F., Deakin, W. J., Ugalde, R. A., and Lepek, V. C. (2009). Characterization of the Mesorhizobium loti MAFF303099 type-three protein secretion system. Mol. Plant Microbe Interact. 22, 519–528. doi: 10.1094/MPMI-22-5-0519
Schäfer, A., Tauch, A., Jäger, W., Kalinowski, J., Thierbach, G., and Pühler, A. (1994). Small mobilizable multi-purpose cloning vectors derived from the Escherichia coli plasmids pK18 and pK19: selection of defined deletions in the chromosome of Corynebacterium glutamicum. Gene 145, 69–73. doi: 10.1016/0378-1119(94)90324-7
Schechter, L. M., Guenther, J., Olcay, E. A., Jang, S., and Krishnan, H. B. (2010). Translocation of NopP by Sinorhizobium fredii USDA257 into Vigna unguiculata root nodules. Appl. Environ. Microbiol. 76, 3758–3761. doi: 10.1128/AEM.03122-09
Sory, M.-P., and Cornelis, G. R. (1994). Translocation of a hybrid YopE-adenylate cyclase from Yersinia enterocolitica into HeLa cells. Mol. Microbiol. 14, 583–594. doi: 10.1111/j.1365-2958.1994.tb02191.x
Staehelin, C., and Krishnan, H. B. (2015). Nodulation outer proteins: double-edged swords of symbiotic rhizobia. Biochem. J. 470, 263–274. doi: 10.1042/BJ20150518
Sugawara, M., Takahashi, S., Umehara, Y., Iwano, H., Tsurumaru, H., Odake, H., et al. (2018). Variation in bradyrhizobial NopP effector determines symbiotic incompatibility with Rj2-soybeans via effector-triggered immunity. Nat. Commun. 9:3139. doi: 10.1038/s41467-018-05663-x
Viprey, V., Del Greco, A., Golinowski, W., Broughton, W. J., and Perret, X. (1998). Symbiotic implications of type III protein secretion machinery in Rhizobium. Mol. Microbiol. 28, 1381–1389. doi: 10.1046/j.1365-2958.1998.00920.x
Wassem, R., Kobayashi, H., Kambara, K., Le Quéré, A., Walker, G. C., Broughton, W. J., et al. (2008). TtsI regulates symbiotic genes in Rhizobium species NGR234 by binding to tts boxes. Mol. Microbiol. 68, 736–748. doi: 10.1111/j.1365-2958.2008.06187.x
Wen, S., Chen, X., Xu, F., and Sun, H. (2016). Validation of reference genes for real-time quantitative PCR (qPCR) analysis of Avibacterium paragallinarum. PLoS One 11:e0167736. doi: 10.1371/journal.pone.0167736
Wenzel, M., Friedrich, L., Göttfert, M., and Zehner, S. (2010). The type III-secreted protein NopE1 affects symbiosis and exhibits a calcium-dependent autocleavage activity. Mol. Plant Microbe Interact. 23, 124–129. doi: 10.1094/MPMI-23-1-0124
Wilson, K. J., Sessitsch, A., Corbo, J. C., Giller, K. E., Akkermans, A. D. L., and Jefferson, R. A. (1995). β-Glucuronidase (GUS) transposons for ecological and genetic studies of rhizobia and other Gram-negative bacteria. Microbiology 141, 1691–1705. doi: 10.1099/13500872-141-7-1691
Keywords: symbiosis, type III secretion system, effector, Bradyrhizobium elkanii, Vigna species
Citation: Nguyen HP, Ratu STN, Yasuda M, Göttfert M and Okazaki S (2018) InnB, a Novel Type III Effector of Bradyrhizobium elkanii USDA61, Controls Symbiosis With Vigna Species. Front. Microbiol. 9:3155. doi: 10.3389/fmicb.2018.03155
Received: 15 September 2018; Accepted: 05 December 2018;
Published: 18 December 2018.
Edited by:
Kiwamu Minamisawa, Tohoku University, JapanReviewed by:
Christian Staehelin, Sun Yat-sen University, ChinaFrancisco Javier López-Baena, Universidad de Sevilla, Spain
Copyright © 2018 Nguyen, Ratu, Yasuda, Göttfert and Okazaki. This is an open-access article distributed under the terms of the Creative Commons Attribution License (CC BY). The use, distribution or reproduction in other forums is permitted, provided the original author(s) and the copyright owner(s) are credited and that the original publication in this journal is cited, in accordance with accepted academic practice. No use, distribution or reproduction is permitted which does not comply with these terms.
*Correspondence: Shin Okazaki, c29rYXpha2lAY2MudHVhdC5hYy5qcA==