- 1Centre for Viticulture and Enology, College of Food Science and Nutritional Engineering, China Agricultural University, Beijing, China
- 2Key Laboratory of Viticulture and Enology, Ministry of Agriculture, Beijing, China
- 3College of Public Health, Shaanxi University of Chinese Medicine, Xianyang, China
The levels of unsaturated fatty acids (UFAs) in grape must significantly influence yeast metabolism and the production of aroma compounds. In this work, cDNA microarray technology was applied to analyze the transcriptional discrepancies of wine yeast (commercial wine yeast Lalvin EC1118) fermenting in synthetic grape must supplemented with different concentrations of a mixture of UFAs (including linoleic acid, oleic acid, and α-linolenic acid). The results showed that the initial addition of a high level of UFAs can significantly enrich the intracellular UFAs when compared to a low addition of UFAs and further increase the cell population and most volatiles, including higher alcohols and esters, except for several fatty acids. Microarray analyses identified that 63 genes were upregulated, and 91 genes were downregulated during the different fermentation stages. The up-regulated genes were involved in yeast growth and proliferation, stress responses and amino acid transportation, while the repressed genes were associated with lipid and sterol biosynthesis, amino acid metabolism, TCA cycle regulation, mitochondrial respiration, and stress responses. Unexpectedly, the genes directly related to the biosynthesis of volatile compounds did not vary substantially between the fermentations with the high and low UFA additions. The beneficial aromatic function of the UFAs was ascribed to the increased biomass and amino acid transportation, considering that the incorporation of the additional UFAs in yeast cells maintains high membrane fluidity and increases the ability of the cells to resist deleterious conditions. Our results highlighted the importance of UFAs in the regulation of aroma biosynthesis during wine fermentation and suggested that the improvement of the resistance of yeast to extreme stresses during alcoholic fermentation is essential to effectively modulate and improve the production of aroma compounds. A potential way to achieve this goal could be the rational increase of the UFA contents in grape must.
Introduction
The production of aroma compounds during wine fermentation is largely influenced by the nutrition status of grape must. Even a small change in the must composition and nutrition concentration, such as sugar concentration, nitrogen source (amino acids), vitamins and fatty acids, could result in a significant impact on the profile of aroma compounds (Bell and Henschke, 2005; Luan et al., 2018). In this context, the effect of assimilable nitrogen sources (YAN) on the formation of volatile compounds has been investigated exhaustively because changes in the YAN content (ammonium salts or amino acids) have a direct and specific effect on the aroma quality of wine (Marks et al., 2003; Mendes-Ferreira et al., 2007). In recent years, the importance of unsaturated fatty acids (UFAs) for yeast fermentation performance and volatile formations has been recognized by winemakers. UFAs are required by Saccharomyces cerevisiae to grow under anaerobic conditions. The incorporation of more UFAs into yeast cells can maintain membrane integrity and increase their ability to resist fermentation stresses, such as high sugar and ethanol toxicity (Holcberg and Margalith, 1981; You et al., 2003). In addition, the degree of unsaturation of the cell membrane can influence the activity of membrane-associated enzymes and transporters (such as ATPase and general amino acid permease) and modulate the production of aroma compounds (Calderbank et al., 1984; Rosa and Sa-Correia, 1992). The absence of oxygen during wine fermentation suppresses the fatty acid desaturation of yeast. An alternative to biosynthesis is the uptake of UFAs from grape juice to avoid stuck fermentation (Varela et al., 2012). Several works demonstrated that UFAs influence the production of volatile compounds via their regulation of the formation of precursor acyl-CoA and the expression of related genes (Yoshioka and Hashimoto, 1983; Trotter, 2001; Swiegers et al., 2005; Duan et al., 2015; Rollero et al., 2016). The aromatic functions of UFAs are largely dependent on the type and concentration of UFAs, which might explain why the results reported by different researchers are inconsistent. For example, the addition of oleic acid and ergosterol can increase the production of higher alcohols and acetate esters but inhibit 1-butanol and 1-pentanol (Mauricio et al., 1997). Casu et al. (2016) found that increasing the concentration of linoleic acid was unfavorable for acetate ester formation but improved the production of higher alcohols. Fujii et al. (1997) confirmed that supplementation with linoleic acid can inhibit AATase (alcohol acetyltransferase) activity and reduce acetate ester synthesis. In a study of this synergistic effect, Tween 80, containing 70% oleic acid and 30% palmic acid and stearic acid, was added to improve the content of esters, higher alcohols and volatile fatty acids of wine (Varela et al., 2012).
Grape berries contain 0.15–0.24% (wet weight basis) lipids (Gallander and Peng, 1980), with UFAs being the major components of the total lipids. Linoleic acid (C18:2n6) is the most abundant lipid, followed by oleic (C18:1n9) and α-linolenic acids (C18:3n3). UFA concentrations in grape must change with grape cultivars (Ancín et al., 1998), fermentation technologies such as grape must clarification (Varela et al., 1999) and grape-skin maceration (Valero et al., 1998). Therefore, from the wine production perspective, it is essential to investigate the synergistic effect of UFAs on aroma compound synthesis during wine fermentation. Duan et al. (2015) indicated that rationally increasing the concentrations of UFA mixtures (linoleic, oleic and α-linolenic acids) can improve yeasts growth and most volatile compounds in wine, including higher alcohols, acetate esters (isoamyl acetate and 2-phenylethyl acetate) and ethyl esters. Numerous efforts have been made to characterize the entire gene expression profiles under different nitrogen conditions during vinification (Marks et al., 2003; Mendes-Ferreira et al., 2007; Liu et al., 2018). However, to our knowledge, no related information is available on the response of S. cerevisiae to UFA variation during wine fermentation. In this work, cDNA microarray technology was therefore applied to analyze the transcriptional discrepancies of the wine yeast S. cerevisiae EC1118 fermenting in two different culture media with different levels of UFAS (including linoleic, oleic, and α-linolenic acids), in which various aromatic compound profiles were detected. To facilitate this investigation, a simplified, chemically defined medium (MS300) that resembles the nutrient composition of grape juice was used, which is often employed in the transcriptional research of wine yeasts (Rossignol et al., 2003; Rossouw et al., 2010).
Materials and Methods
Yeast Strain and Culture Media
The commercial S. cerevisiae var. bayanus strain EC1118 (Lallemand Inc., Blagnac, France) was used in this study. It is used for both red and white winemaking worldwide and is considered a fast and robust fermenting strain (Brice et al., 2014). The nitrogen synthetic grape must MS300 was used in this work (Varela et al., 2004). The pH of the medium was adjusted to 3.3. According to previous data by Duan et al. (2015) two UFA mixture concentrations (including linoleic, oleic and α-linolenic acids purchased from Sigma-Aldrich Company, St. Louis, MO, United States) were added to the MS300 medium, and a high UFA concentration medium (with 390 mg/L linoleic, 130 mg/L oleic, and 104 mg/L α-linolenic acids) and a low UFA concentration medium (with 30 mg/L linoleic, 10 mg/L oleic, and 8 mg/L α-linolenic acids) required to ensure normal cell growth and fermentation, considered the control, were obtained.
Fermentation Conditions and Samples
Saccharomyces cerevisiae EC1118 strain was inoculated into 200 mL YEPD medium (10 g/L yeast extract, 20 g/L peptone, 20 g/L glucose) and cultivated for approximately 15 h with shaking (120 rpm) at 28°C. After harvesting and washing twice with sterile water, 5 mL of yeast cells suspension was added into the flask, and the initial viable population was approximately 106 CFU/mL. Before the inoculation, nitrogen was sparged to eliminate oxygen from the medium. The 500 mL flasks with 350 mL MS300 medium were sealed with a fermentation lock, which guarantees carbon dioxide exhaustion and prevents oxygen from entering the flasks to achieve anaerobic conditions. Fermentations were carried out without shaking at 25°C and in triplicate. The fermentation lasted 170 h. The progress of fermentation was monitored daily by measuring the cell density (OD600) and sugar consumption. A total of 25 mL samples were taken from the fermentation flasks with a puncture needle and were immediately centrifuged to collect the cell-free supernatants for the analysis of the main fermentation products and aroma compounds.
Analytical Methods
General parameters (glucose, ethanol, glycerol, acetic acid, malic acid, lactic acid, and succinic acid) were determined by high-performance liquid chromatography (HPLC, 1200 series, Agilent Technologies, Inc., Palo Alto, CA, United States) as described by Duan et al. (2015). The system was equipped with an HPX-87H Aminex ion-exchange column (300 mm × 7.8 mm, Bio-Rad Laboratories, Hercules, CA, United States) with 5 mM sulfuric acid as the mobile phase. The volatile compounds of the wines were determined by headspace solid-phase microextraction coupled with gas chromatography-mass spectrometry (HS-SPME-GC-MS) as previously described (Zhang et al., 2011; Xu et al., 2015). An Agilent 6890A equipped with a 5975C MS system and an HP-INNOWAX column (60 m × 0.25 mm × 0.25 μm) was used in this system. The aroma compounds were identified by a comparison of the retention indices (RI) of reference standards and mass spectra that matched in the NIST 08 MS database. The quantification was applied with the calibration curves of aroma standards as described by Xu et al. (2015). Analyses were performed in triplicate. Significant differences of metabolites among the treatments were identified using one-way analysis of variance (ANOVA) followed by Duncan’s test (p < 0.05) (SPSS 17.0, SPSS Inc., Chicago, IL, United States).
Microarray Procedures
For the DNA microarray analyses, the RNA of yeasts in mid-exponential (30 h), early-stationary (87 h) and late-stationary growth phases (123 h) were extracted, corresponding to the time points that 19.4, 67.2, and 88.1% sugars were consumed by yeast in high UFA culture, respectively, and 16.7, 57.6, and 89.9% sugars were consumed in low UFA culture, respectively. Three independent cultures were prepared for the biological repeats. Total RNA was isolated using the hot phenol method (Deed et al., 2011) and assessed by agarose gel electrophoresis and a NanoDrop spectrophotometer ND-1000 (NanoDrop products, DE, United States). The RNA samples were subjected to whole-genomic gene expression profiles (CapitalBio Corporation, Beijing, China). After purification, cDNA and biotin-labeled cRNA syntheses, the cRNA samples were hybridized with Yeast Genome 2.0 (Affymetrix GeneChip) and processed as described by the manufacturer (Affymetrix, CA, United States1). Pretreatments were applied to eliminate the sample variation, including background rectification and normalization. The pretreated data were analyzed with the RMA algorithm. A significance analysis of microarray (SAM) was applied to identify the genes that were differentially expressed between the high UFA and low UFA cultures. The threshold for significance was set to allow a median of one false positive per analysis for a false discovery rate (FDR) of <0.05%. Genes of the yeast in high UFA culture whose expression levels were greater than twofold or less than 0.5-fold, relative to the yeast in low UFA culture, were considered to be induced or repressed, respectively. These genes were further categorized by biological process using the Saccharomyces Genome Database Gene Ontology Slim Mapper tools (SGD GO Slim Mapper2).
Results
Cell Growth, Sugar Consumption, and Major Aroma Compounds in Low and High UFA Cultures
Yeast cell growth (OD600) and sugar consumption in the treatments were monitored during fermentation (Figure 1). To facilitate the comparison, some key kinetic parameters were calculated, such as the duration of fermentation, maximum biomass, time to reach the maximum biomass, maximum specific growth, and rate of fermentation, which are shown in Table 1. In general, a relatively higher level of UFAs improved the yeast population and fermentation activity. The highest cell population (OD600) obtained at late-stationary phase (123 h) in the high UFA fermentation (HUF) was 15.0% higher than that in the low UFA fermentation (LUF). Similarly, the fermentation rate (rate of sugar consumption) in HUF was slightly enhanced in comparison to the LUF (2.06 vs. 1.99 g/L⋅h of maximum fermentation rate). The production profiles of ethanol and glycerol were also determined (Supplementary Figure S1). The results showed that the ethanol concentration did not differ between treatments, while glycerol was higher in the HUF (6.34 ± 0.12 vs. 5.93 ± 0.07 g/L in final samples). No significant differences were found in the number of other metabolites, such as acetic, citric and malic acids, in the final samples of both fermentations (data not shown). We further determined the content of extracellular and intracellular UFAs during fermentation (Figure 2). The data indicated that the UFAs added were rapidly taken up by the cells. No UFAs were detected after fermentation in LUF, while few UFAs remained in the HUF sample. As expected, in both treatments, the concentration of intracellular UFAs increased before 30 h fermentation, after which the values slowly decreased. However, the concentration of particular cellular UFAs was always higher in the HUF than in the LUF samples.

Table 1. Important parameters of yeast fermentation in MS300 media supplemented with high (HUFA) or low (LUFA) concentrations of UFAs.
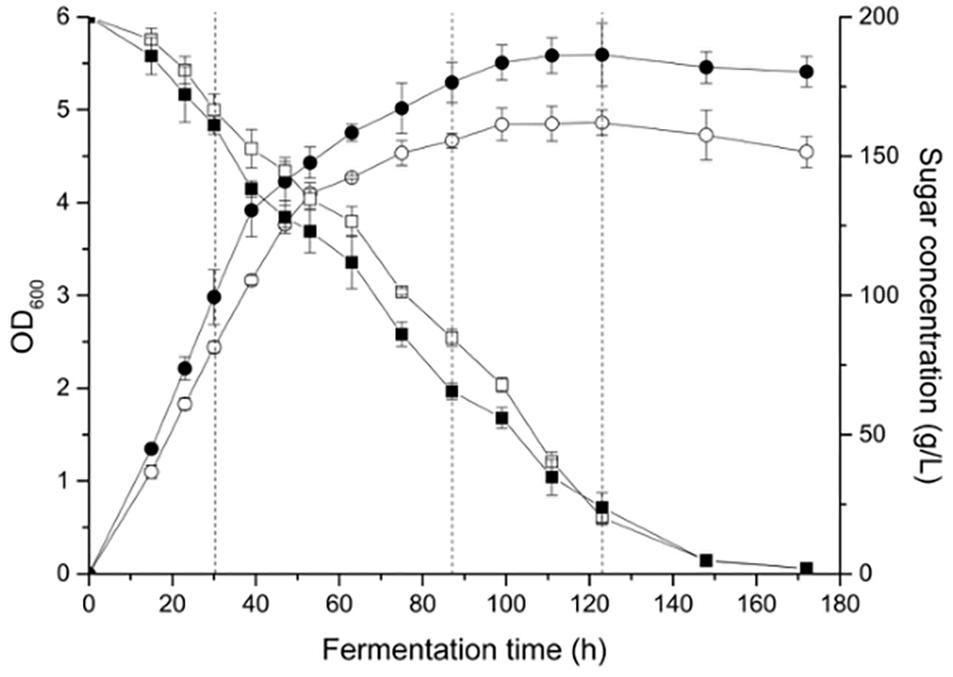
Figure 1. Cell growth and sugar concentration profiles during the fermentation of MS 300 media with high (,
) or low (
,
) UFA concentrations. Data points represent the mean value from triplicate fermentations, and the vertical bars show ±SD. Dashed lines represent the three growth phases in which the microarray analyses were conducted.
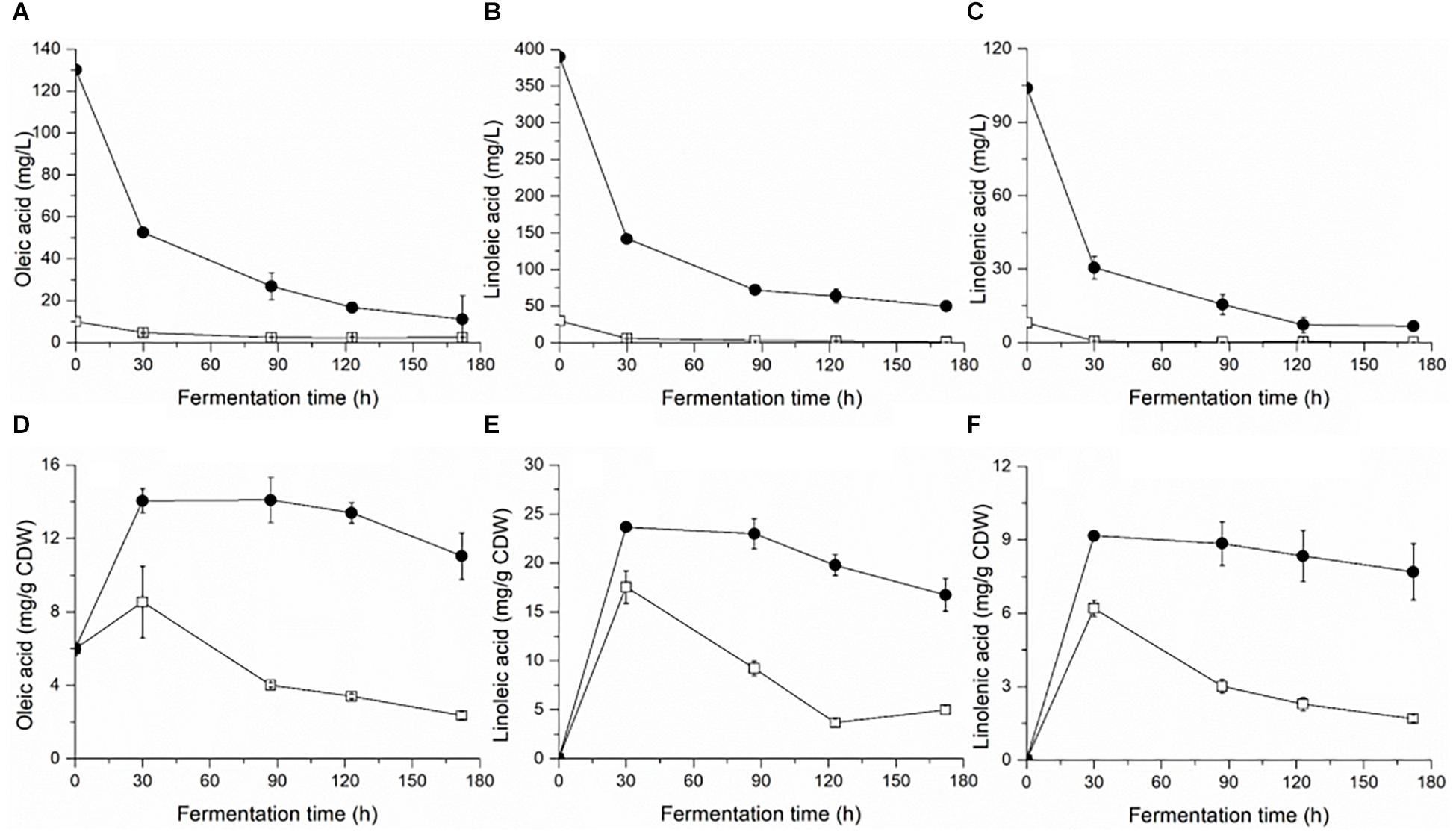
Figure 2. Profiles of extracellular (A–C) and intracellular (D–F) fatty acids during the fermentation of MS300 media with high () and low (
) concentrations of UFAs. Data points represent the mean value from triplicate fermentations, and the vertical bars show ±SD.
To demonstrate the effects of UFAs on the generation of aromatic compounds, the main volatile compounds (higher alcohols, acetate esters, ethyl esters, and fatty acids) produced by yeasts at different fermentation times were determined, as shown in Figures 3, 4. In general, the supply of a relatively higher UFA mixture improved the generation of most aroma compounds, including higher alcohols (2-methyl-1-propanol, 3-methyl-1-butanol, and phenylethyl alcohol) and esters (3-methyl-1-butanol acetate, ethyl acetate, 2-phenylethyl acetate, ethyl hexanoate, ethyl heptanoate, ethyl octanoate, ethyl nonanoate, ethyl decanoate, ethyl dodecanoate, ethyl myristate, and ethyl palmitate). As a result, the total content of higher alcohols, acetate esters and ethyl esters in the final samples of HUF was 45.5, 40.0, and 49.5% higher than those in LUF, respectively. The response of fatty acid formation to the addition of UFA depended to a large extent on the types of fatty acids added. Butanoic acid and octanoic acid showed higher trends, while hexanoic acid, decanoic acid, and dodecanoic acid showed decreased profiles. As a result, no significant difference in total fatty acid content was observed between the two treatments (10.35 ± 0.28 vs. 12.31 ± 0.79 mg/L).
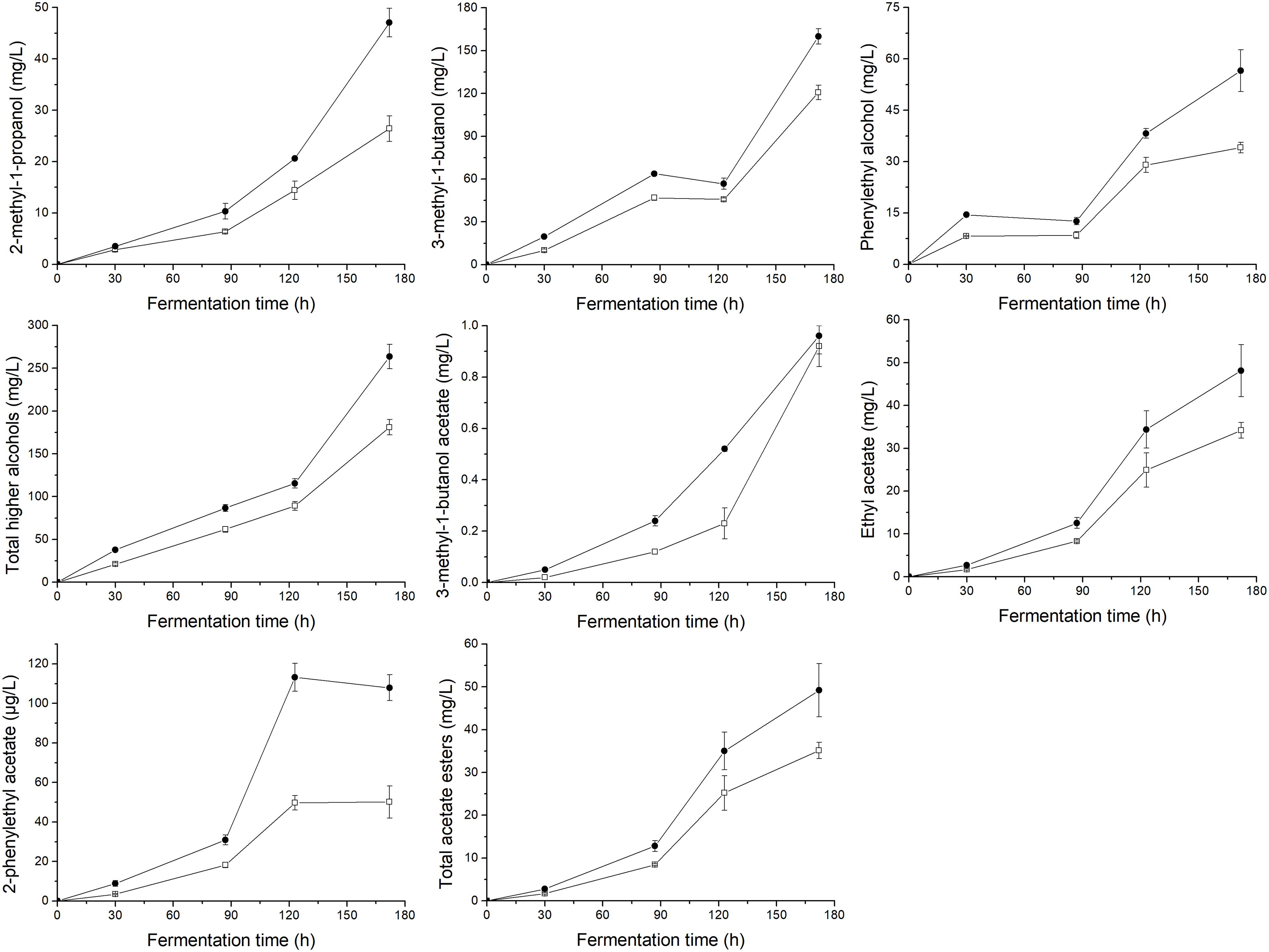
Figure 3. Profiles of the major higher alcohols and total content of higher alcohols, acetate esters and total content of acetate esters during the fermentation of MS300 medium with high () or low (
) UFA concentrations. Data points represent the mean value from triplicate fermentations, and the vertical bars show ±SD.
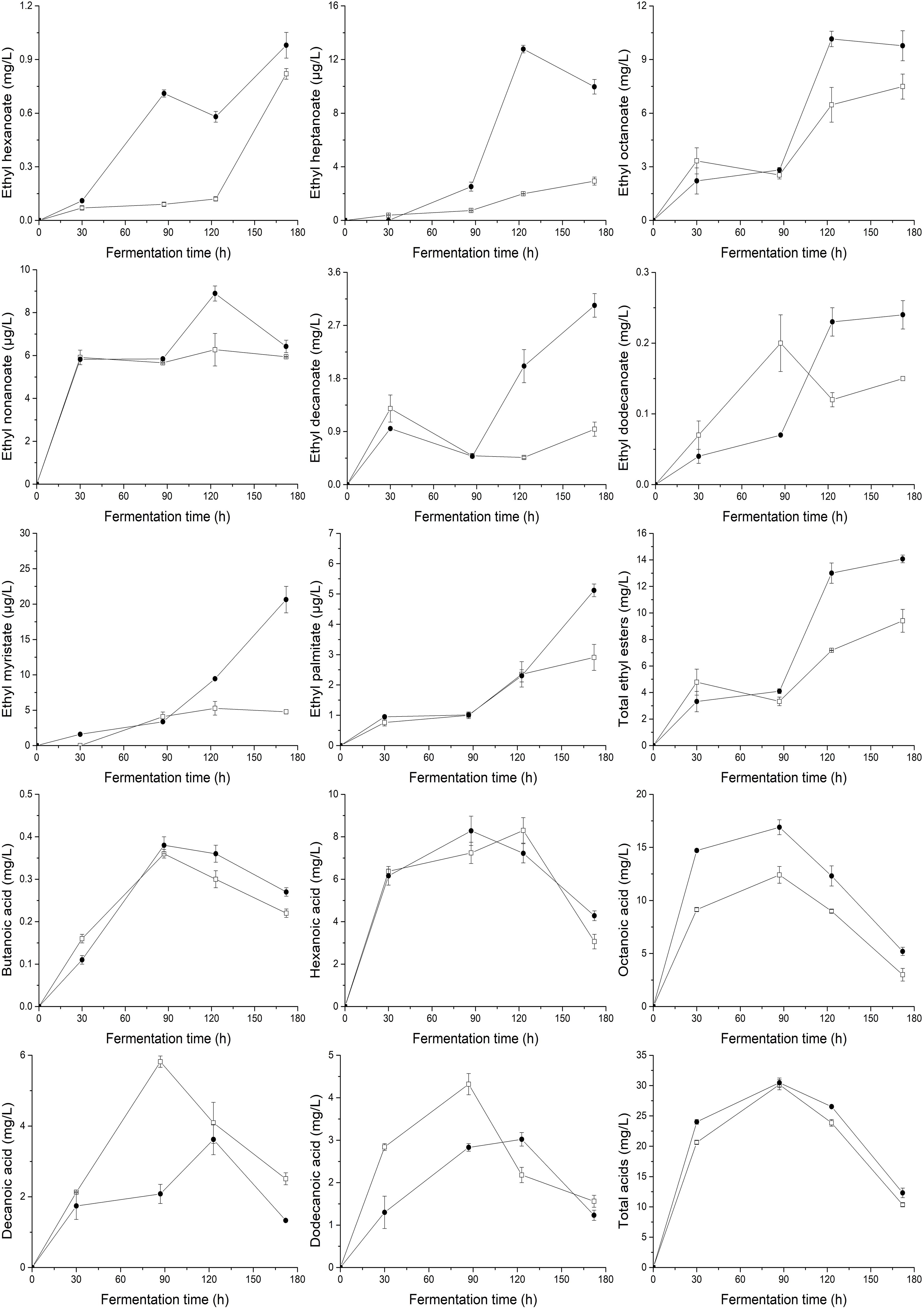
Figure 4. Profiles of major ethyl esters and the total content of ethyl esters, fatty acids and the total content of fatty acids during the fermentation of MS300 media with high () and low (
) UFA concentrations. Data points represent the mean value from triplicate fermentations, and the vertical bars show ±SD.
Analysis of Yeast Global Gene Expression by DNA Microarray
Consistent with the results of Duan et al. (2015), the above data indicated that increasing the UFA content in synthetic grape juice improved yeast growth and the production of most volatiles. To gain insight into the mechanism at the molecular level, a comparative transcriptome analysis using DNA microarray technology was applied. The RNA of yeast in the mid-exponential (30 h), early-stationary (87 h), and late-stationary growth phases (123 h) was used for pair wise comparisons of HUF compared to LUF. In total, 63 genes were upregulated (greater than twofold expression), and 91 were down-regulated (less than 0.5-fold expression), as shown in Tables 2, 3. Most of the affected genes were found in the late-stationary growth phase. To confirm the results of the DNA microarray analyses, six genes were randomly selected, including FAA4, BAP3, CLB1, SPS100, ALD3, and MGA2, and qPCR experiments were performed. Correlation analysis showed that the correlation coefficient between the microarray chip and qPCR determinations exceeded 0.887, indicating that the data obtained by DNA microarray are reliable (Supplementary Figure S2).
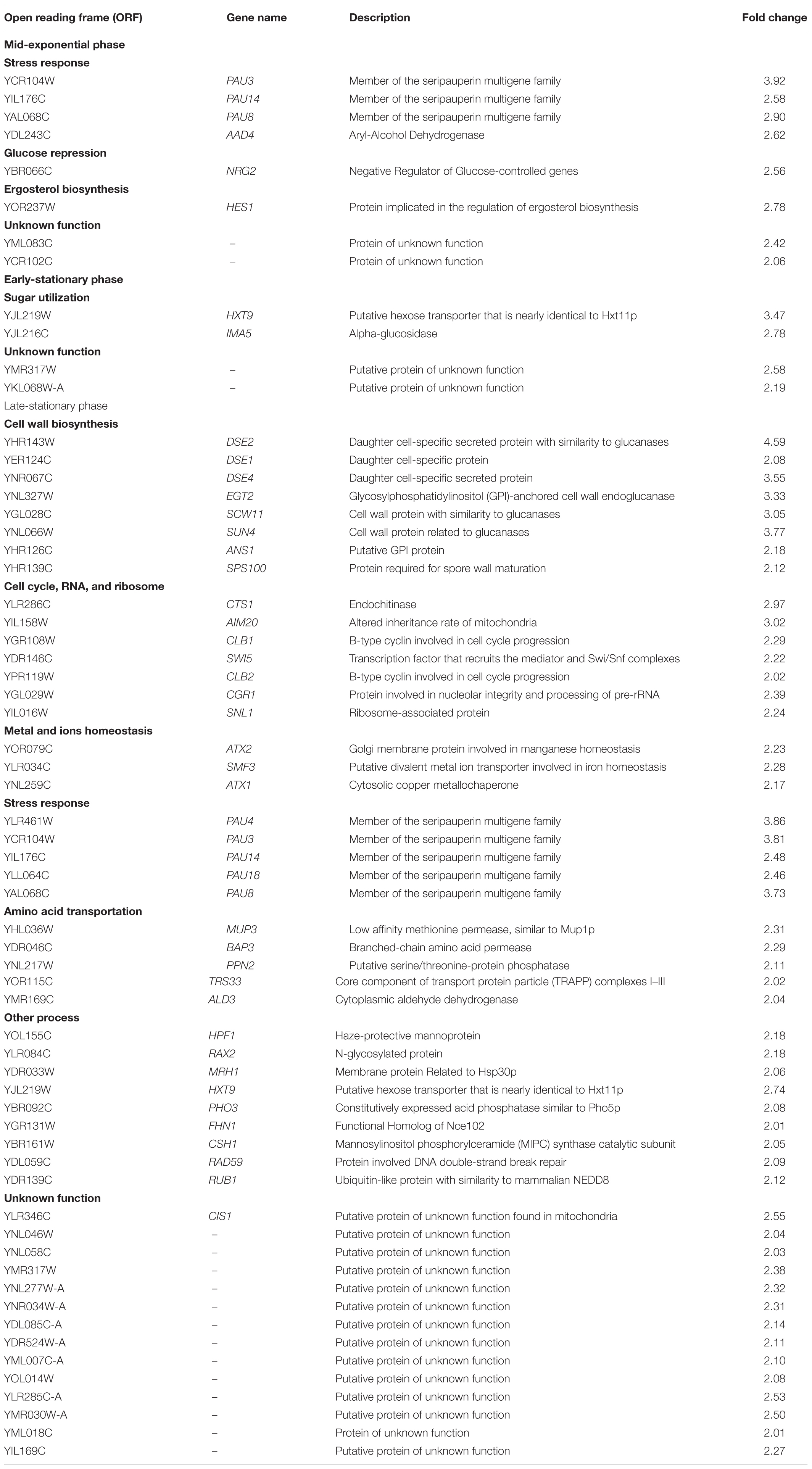
Table 2. Genes upregulated in MS300 media with high vs. low concentrations of UFAs, in different stages of yeast growth categorized by biological process (double or more).
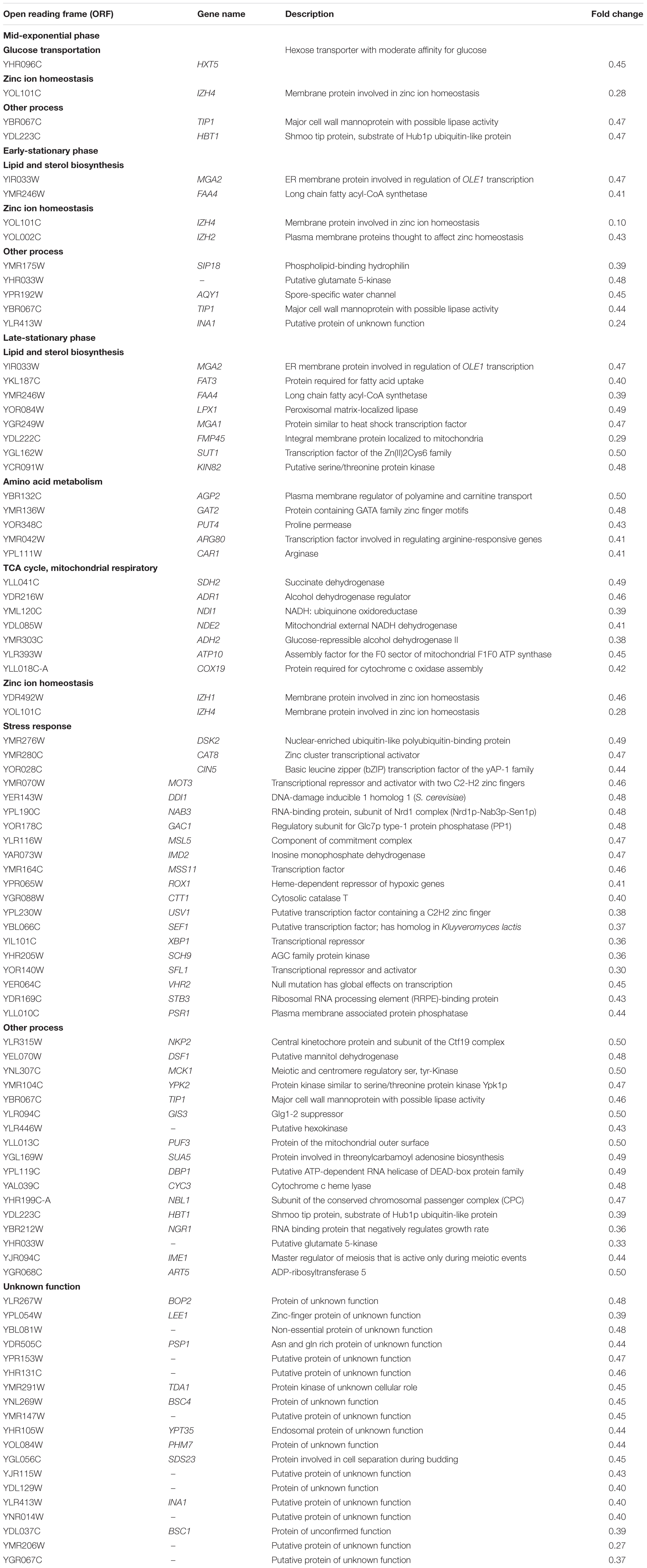
Table 3. Genes downregulated in MS300 media with high vs. low concentrations of UFAs, in different stages of yeast growth categorized by biological process (double or more).
The affected genes were further categorized according to their biological and functional processes as assigned by the Saccharomyces Genome Database (SGD). Eight genes were upregulated, and four genes were downregulated in the mid-exponential growth phase. In addition to two unknown function genes (YML083C and YCR102C), the upregulated genes included PAU3, PAU14, PAU8, and AAD4, which are involved in the processes of stress response. In addition, AAD4 encodes a putative aryl-alcohol dehydrogenase and is involved in the oxidative stress response. NRG2 mediates glucose repression, and HES1 is associated with ergosterol biosynthesis. The downregulated genes included TIP1 (involved in wall protein synthesis), HXT5 (encodes hexose transporter), IZH4 (involved in zinc ion homeostasis), and HBT1 (encodes shmoo tip protein, the substrate of Hub1p ubiquitin-like protein).
Fewer genes were induced in the early-stationary phase compared to the mid-exponential phase, including HXT9 and IMA5, and two unknown function genes (YMR317W and YKL068W-A). HXT9 and IMA5 are involved in sugar transportation and utilization. The expression of genes involved in lipid and fatty acid biosynthesis was downregulated in HUF. Exogenous fatty acids can strongly repress the de novo synthesis of lipids and fatty acids in yeasts, as has been reported by Chirala (1992). Although we did not observe the repression of OLE1 transcription, which encodes delta (9) fatty acid desaturase and is involved in the formation of UFAs, two other genes, MGA2 (encoding an ER membrane protein that regulates OLE1 transcription) and FAA4 (encoding the long chain fatty acyl-CoA synthetase responsible for the importation of long-chain fatty acids), were found to be downregulated. IZH2 and IZH4 were continuously repressed in HUF (still depressed in the late-stationary phase); both genes play an important role in zinc metabolism and homeostasis and exhibit elevated expression in zinc-deficient cells. It has been confirmed that their expression is lipid- and oxygen-dependent and linked with sterol metabolism (Lyons et al., 2004). The down-regulation of these genes might be due to the incorporation of more UFAs into the cell membrane, which disturbs de novo lipid syntheses. The decreased expression of TIP1 was observed in this and the last yeast growth stage of HUF.
Most genes were affected after cells entered the late-stationary phase (51 and 78 genes were upregulated and downregulated, respectively). This could be because the nutritional status at this stage became sterile compared to other stages (Backhus et al., 2001; Marks et al., 2003). The induced genes in this stage were mainly associated with cell wall formation, cell cycle, RNA and ribosome biosynthesis, metal and ion metabolism, stress response, amino acid metabolism, process, and unknown function. The repressed genes were related to lipid, sterol, amino acid, carbohydrate metabolism, stress response, zinc ion homeostasis, process, and unknown function. Cell wall formation, cell cycle, RNA and ribosome biosynthesis are associated with cell growth and proliferation. The high expression of the genes DSE2, DSE1, DSE4, EGT2, SCW1, SUN4, ANS1, SPS100, CTS1, AIM20, CLB1, SWI5, CLB2, CGR1, and SNL1 was correlated with an increase in the cell population in HUF. Members of PAU (PAU3, PAU14, PAU14, PAU8, and PAU18) and the genes MUP3, BAP3, and TRS33, all involved in amino acid transportation, were induced at this stage in HUF. In addition, MUP3 encodes a low affinity methionine permease, and BAP3 encodes a specific branched-chain amino acid permease. Another upregulated gene was ALD3, which encodes cytoplasmic aldehyde dehydrogenase and plays a critical role in the conversion of acetaldehyde to acetyl-CoA during growth on non-fermentable carbon sources, which can be induced in response to ethanol stress (Navarro-Aviño et al., 1999). The upregulation of ALD3 might enable cells to generate more acetyl-CoA for producing esters in HUF. PHO3 (encodes acid phosphatase to hydrolyze thiamine phosphates) was also upregulated by high UFA addition.
Compared to upregulated genes, more genes were repressed in the late-stationary phase. In addition to MGA2 and FAA4 (which were downregulated in the early-stationary phase), the genes FAT3, LPX1, FMP45, SUT1, and MGA1 involved in lipid and sterol metabolism were repressed. FAT3 encodes the transporter protein Fat3p and is responsible for fatty acid uptake; SUT1 encodes the Zn(II)2Cys6 family transcription factor and positively regulates sterol uptake genes under anaerobic conditions; FMP45 encodes an integral membrane protein located in the mitochondria and is required for sporulation and the maintenance of sphingolipid content. These data suggest that de novo synthesis and the metabolism of fatty acids and sterol in cells in the HUF were strongly negatively influenced in the late stationary phase. Additionally, several genes involved in amino acid and nitrogen metabolism were downregulated. It should be noted that these amino acids were not different from those present in the group of upregulated genes. They mainly included AGP2, GAT2, PUT4, ARG80, and CAR1. AGP2 encodes the plasma membrane regulator of polyamine and carnitine transport and can act as a sensor that transduces environmental signals. GAT2 encodes the protein containing the GATA family zinc finger motifs and is repressed by leucine. PUT4 encodes a proline permease with high affinity proline transport. ARG80 encodes a transcription factor and is involved in the regulation of arginine-responsive genes. CAR1 encodes arginase that catabolizes arginine to ornithine and urea and controls the formations of ethyl carbamate (EC). Interestingly, some genes associated with the TCA cycle and mitochondrial respiratory chains were repressed, including SDH2, ADR1, NDI1, NDE2, and ADH2.
It should be noted that a majority of the stress-response genes were upregulated in LUF compared to HUF at this stage, including DSK2, CAT8 (respond to DNA replication stress), CIN5 (mediates pleiotropic drug resistance and salt tolerance), MOT3 (transcriptional repressor, activator, cellular adjustment to osmotic stress), MSS11 (a transcription factor controlling the activation of FLO11 and STA2 in response to nutritional signals), ROX1 (involved in the hyperosmotic stress resistance), CTT1 (protects from oxidative damage), USV1 (responds to salt stress and cell wall biosynthesis), XBP1 (transcriptional repressor, induced by stress or starvation during mitosis), and PSR1 (plasma membrane associated protein phosphatase and involved in general stress response). These data suggest that yeast cells in LUF might be subjected to more stresses at this stage than those in HUF.
Discussion
It is well known that wine yeast is challenged by simultaneous and sequential stresses during alcoholic fermentation, especially ethanol toxicity. The cellular membrane is the cell structure most affected by ethanol, which causes an increase in its permeability and leads to unfavorable effects, such as the inhibition of sugar, ammonium and amino acid uptake (Diniz et al., 2017). To maintain membrane stability, S. cerevisiae increases the synthesis of UFAs and enriches the plasma membrane UFA content. Alternatively, in the presence of exogenous UFAs, yeast cells can absorb UFAs into the cell membrane directly and improve their resistance to inhospitable environments, which can lead to increased biomass and fermentative activity and consequently modify the production of aroma compounds (Martin et al., 2007; Duan et al., 2015). To better understand the positive aromatic function of UFAs during wine fermentation, in this work, the transcriptional profiles of wine yeast in response to low and high UFA mixture additions were investigated by DNA microarray analyses in synthetic grape medium. The data described indicate that the initial supplementation of a high UFA mixture can promote cell growth and the production of most aromatic compounds, including higher alcohols, acetate esters and ethyl esters, with the exception of several fatty acids (hexanoic acid, decanoic acid, and dodecanoic acid). Microarray analyses identified that sixty-three and ninety-one genes were upregulated (greater than twofold expression) or downregulated (less than 0.5-fold expression), respectively. Because the aim of the study is to reveal the molecular effect of UFAs on aroma compounds, we focused on the groups of genes associated with volatile production in the discussion below, especially the upregulated genes.
The improvement of cell wall formation can increase the resistance of yeast to environmental stresses (Brennan et al., 2013). The expression of genes involved in cell wall formation, cell cycle, RNA, and ribosome biosynthesis was elevated in HUF, which corresponded to an increase in the biomass in HUF. Increasing the cell population in wine fermentation directly promotes the formation of aroma compounds (Varela et al., 2004; Duan et al., 2015). Thus, the beneficial effect of UFAs on aroma compounds observed in this work could be partially ascribed to an increased cell population able to resist deleterious conditions during wine fermentation. PAU genes comprise the largest multiple gene family in S. cerevisiae, with 24 members, which are induced by different stresses, such as low temperature, low oxygen and wine fermentation conditions (Luo and van Vuuren, 2009). In this study, PAU3, PAU14, PAU8, and PAU18 were induced at different stages in HUF, which is consistent with the data of Rossignol et al. (2003), who found that 15 PAU/TIR genes were strongly upregulated during wine alcoholic fermentation. Wilcox et al. (2002) suggested that the function of PAU protein involves sterol transport, which might explain the substantial induction of PAU expression in HUF. Increased biomass can consume more nutrients (such as sterol, ions, copper, and thiamine) and cause nutrition deficiency, which can induce the expression of related functional genes to replenish these compounds (Liu et al., 2018). This could account for the increased expression of several genes (PHO3, ATX1, and ATX2) by high UFA additions. PHO3, which encodes an acid phosphatase, can hydrolyze thiamine phosphates in the periplasmic space and increase cellular thiamine uptake. Thiamine pyrophosphate is a cofactor essential for the activity of pyruvate decarboxylase, and its depletion has a negative effect on yeast carbon metabolism (Hohmann and Meacock, 1998). Increasing the yeast biomass can consume a large amount of thiamine pyrophosphate, resulting in its deficiency in the must (Liu et al., 2018). The upregulation of ATX1 and ATX2, which encode the Mn2+ transporter, implies that the metal could also be limited in the cells (Lin and Culotta, 1996).
Amino acid metabolism is of particular interest from a winemaking perspective, as amino acids serve as the precursors of important volatile aroma compounds (Rossouw et al., 2010). In this study, the expression levels of genes directly related to the formation of aroma compounds, such as BAT1, PDC1, ATF1, EEB1, EHT1, and IAH1, were not significantly different between the two treatments, while the genes encoding amino acid permeases were greatly induced in HUF, for example, BAP3 that encodes a one branched-chain amino acid permease. Branched-chain amino acids (including L-valine, L-leucine, and L-isoleucine) are important flavor precursors in grape must. The elevated expression of BAP2 and BAP3 can enable yeast to transport more extracellular amino acids into the cells to produce higher alcohols and corresponding esters (Hazelwood et al., 2008; Trinh et al., 2010). Thus, the upregulation of BAP3 might be another reason that an increased number of high alcohol and esters were produced in HUF. It should be mentioned that BAP3 is subject to nitrogen catabolite repression (NCR) and is strongly repressed by yeast-preferred nitrogen (such as ammonium and glutamine) but is depressed when the cells are starved for nitrogen (McCusker and Haber, 1990; Magasanik and Kaiser, 2002). The induction of BAP3 in the late-stationary growth phase of HUF could be due to the increased biomass, which causes the nitrogen available for yeast to be deficient in comparison with LUF. The incorporation of abundant UFAs into the cell membrane can help the cells maintain normal membrane fluidity and protect the activity of membrane-associated enzymes and transporters, which might, at least partially, lead to the increased production of aroma compounds. Interestingly, we found that CAR1 and ARG80, which are positively involved in ethyl carbamate (EC) formation, were downregulated in HUF. In grape musts, the catabolism of arginine by wine yeasts can produce ornithine and urea. The secreted urea spontaneously reacts with ethanol to generate EC, which causes different cancers in a variety of test animals (Beland et al., 2005). The disruption of CAR1 can decrease the production of the carcinogen EC during wine fermentation (Schehl et al., 2007). As a result, it is believed that increasing the UFA content in grape must or enriching UFAs in the cell membrane might be a potential way to reduce the formation of EC in wines. This hypothesis merits further study.
The de novo synthesis of lipids and fatty acids is repressed in the presence of exogenous fatty acids (Chirala, 1992), and the absorption of UFAs from the environment can inhibit the biosynthesis of UFAs and FA in yeasts (McDonough et al., 1992). The reduction of medium-chain fatty acids (MCFAs) by exogenous UFAs has been reported by Redon et al. (2011). Similarly, we found that MCFA formation and several genes involved in fatty acid transportation and synthesis were repressed in HUF. It was also found that some genes associated with the TCA cycle and mitochondrial respiration (SDH2, ADR1, NDI1, NDE2, and ADH2) were induced in LUF in comparison with HUF. Currently, we cannot explain these data well. However, the data of Backhus et al. (2001) showed that the wine yeast response to low nitrogen in the late time point of wine fermentation is to switch from a fermentative mode of metabolism to respiration characteristic with a general relief of TCA and respiration genes from glucose repression, which was accompanied by the increased expression of ADH2.
It is important to highlight that some results obtained in this study are not consistent with previous data. For example, the supplementation of single UFAs, such as linoleic acid, oleic acid or α-linolenic acid, can inhibit AATase (alcohol acetyltransferase) activity and reduce acetate ester synthesis (Yoshioka and Hashimoto, 1983; Fujii et al., 1997). The inconsistency might be due to differences in the culture medium or (and) the added UFA compositions (single or combined addition) and concentrations. Additionally, many genes with unknown functions were upregulated (14) or downregulated (19) in HUF vs. LUF. For example, YLR346C is regulated by transcription factors involved in pleiotropic drug resistance, Pdr1p and Yrr1p (Le Crom et al., 2002). The expression of YNR034W-A is regulated by Msn2/Msn4 (Lai et al., 2005). Msn2 and Msn4, which encode stress-responsive transcriptional activators, are activated in response to various stress conditions. These observations highlight the limitation of our understanding of the molecular mechanisms involved in wine yeast survival and metabolism during wine fermentation. Revealing the functions of these genes could help us to rationally control the process of wine fermentation and effectively modulate the formation of aroma compounds.
Conclusion
The results of the present work indicate that adding high contents of an UFA mixture into synthetic grape medium increased cell growth and the production of most yeast-derived volatile compounds compared to the low UFA-added culture, including higher alcohols and the corresponding esters, with the exception of several fatty acids. Sixty three and ninety one genes were identified by microarray analyses to be upregulated or downregulated, respectively, during alcoholic fermentation. Most of the upregulated genes were involved in yeast growth and proliferation, stress response, and nitrogen compound transportation. There were no genes directly involved in the formation of higher alcohols, and esters were found to be significantly upregulated in HUF vs. LUF. The improvement of aroma compounds in HUF is ascribed to the increased resistance of yeast to various stresses due to the incorporation of more UFAs into cells and the increased biomass and amino acid transportation. Our results highlighted the importance of UFAs in the regulation of aroma biosynthesis during wine fermentation and suggested that improving the resistance of yeast to extreme stresses is essential to effectively manipulate and improve the production of aroma compounds.
Author Contributions
C-QD and G-LY designed the experiments. L-LD and P-TL conducted the experiments. L-LD, P-TL, and G-LY analyzed the experimental data and wrote the manuscript.
Funding
This work was supported financially by the China Agriculture Research System (CARS-29) and Chinese Universities Scientific Fund (2017SP003).
Conflict of Interest Statement
The authors declare that the research was conducted in the absence of any commercial or financial relationships that could be construed as a potential conflict of interest.
Supplementary Material
The Supplementary Material for this article can be found online at: https://www.frontiersin.org/articles/10.3389/fmicb.2019.01115/full#supplementary-material
Footnotes
References
Ancín, C., Ayestarán, B., García, A., and Garrido, J. (1998). Evolution of fatty acid contents in Garnacha and Viura musts during fermentation and the aging of wine. Z. Lebensm. Unters. F. A 206, 143–147. doi: 10.1007/s002170050230
Backhus, L. E., Derisi, J. D., Brown, P. O., and Bisson, L. F. (2001). Functional genomic analysis of a commercial wine strain of Saccharomyces cerevisiae under differing nitrogen conditions. FEMS Yeast Res. 1, 111–125. doi: 10.1016/s1567-1356(01)00019-8
Beland, F. A., Benson, R. W., Mellick, P. W., Kovatch, R. M., Roberts, D. W., Fang, J. L., et al. (2005). Effect of ethanol on the tumorigenicity of urethane (ethyl carbamate) in B6C3F1 mice. Food Chem. Toxicol. 43, 1–19. doi: 10.1016/j.fct.2004.07.018
Bell, S. J., and Henschke, P. A. (2005). Implications of nitrogen nutrition for grapes, fermentation and wine. Aust. J. Grape Wine Res. 11, 242–295. doi: 10.1111/j.1755-0238.2005.tb00028.x
Brennan, T. C., Krömer, J. O., and Nielsen, L. K. (2013). Physiological and transcriptional responses of Saccharomyces cerevisiae to d-limonene show changes to the cell wall but not to the plasma membrane. Appl. Environ. Microbiol. 79, 3590–3600. doi: 10.1128/AEM.00463-13
Brice, C., Sanchez, I., Tesnière, C., and Blondin, B. (2014). Assessing the mechanisms responsible for differences between nitrogen requirements of Saccharomyces cerevisiae wine yeasts in alcoholic fermentation. Appl. Environ. Microbiol. 80, 1330–1339. doi: 10.1128/AEM.03856-13
Calderbank, J., Keenan, M. H., Rose, A. H., and Holman, G. D. (1984). Accumulation of amino acids by Saccharomyces cerevisiae Y185 with phospholipids enriched in different fatty-acyl residues: a statistical analysis of data. J. Gen. Microbiol. 130, 2817–2824.
Casu, F., Pinu, F. R., Fedrizzi, B., Greenwood, D. R., and Villas-Boas, S. G. (2016). The effect of linoleic acid on the Sauvignon blanc fermentation by different wine yeast strains. FEMS Yeast Res. 16:fow050. doi: 10.1093/femsyr/fow050
Chirala, S. S. (1992). Coordinated regulation and inositol-mediated and fatty acid-mediated repression of fatty acid synthase genes in Saccharomyces cerevisiae. P. Natl. Acad. Sci. U.S.A. 89, 10232–10236. doi: 10.1073/pnas.89.21.10232
Deed, N. K., van Vuuren, H. J. J., and Gardner, R. C. (2011). Effects of nitrogen catabolite repression and di-ammonium phosphate addition during wine fermentation by a commercial strain of S. cerevisiae. Appl. Microbiol. Biotechnol. 89, 1537–1549. doi: 10.1007/s00253-011-3084-y
Diniz, R. H. S., Villada, J. C., Alvim, M. C. T., Vidigal, P. M. P., Vieira, N. M., Lamas-Maceiras, M., et al. (2017). Transcriptome analysis of the thermotolerant yeast Kluyveromyces marxianus CCT 7735 under ethanol stress. Appl. Microbiol. Biotechnol. 101, 6969–6980. doi: 10.1007/s00253-017-8432-0
Duan, L. L., Shi, Y., Jiang, R., Yang, Q., Wang, Y. Q., Liu, P. T., et al. (2015). Effects of adding unsaturated fatty acids on fatty acid composition of Saccharomyces cerevisiae and major volatile compounds in wine. S. Afr. J. Enol. Vitic. 36, 285–295.
Fujii, T., Kobayashi, O., Yoshimoto, H., Furukawa, S., and Tamai, Y. (1997). Effect of aeration and unsaturated fatty acids on expression of the Saccharomyces cerevisiae alcohol acetyltransferase gene. Appl. Environ. Microbiol. 63, 910–915.
Gallander, J. F., and Peng, A. C. (1980). Lipid and fatty acid compositions of different grape types. Am. J. Enol. Vitic. 31, 24–27.
Hazelwood, L. A., Daran, J., van Maris, A. J., Pronk, J. T., and Dickinson, J. R. (2008). The Ehrlich pathway for fusel alcohol production: a century of research on Saccharomyces cerevisiae metabolism. Appl. Environ. Microbiol. 74, 2259–2266. doi: 10.1128/aem.02625-07
Hohmann, S., and Meacock, P. A. (1998). Thiamin metabolism and thiamin diphosphate-dependent enzymes in the yeast Saccharomyces cerevisiae: genetic regulation. Biochem. Biophys. Acta 1385, 201–219. doi: 10.1016/s0167-4838(98)00069-7
Holcberg, I., and Margalith, P. (1981). Alcoholic fermentation by immobilized yeast at high sugar concentrations. Euro. J. Appl. Microbiol. Biotech. 13, 133–140. doi: 10.1007/bf00703041
Lai, L. C., Kosorukoff, A. L., Burke, P. V., and Kwast, K. E. (2005). Dynamical remodeling of the transcriptome during short-term anaerobiosis in Saccharomyces cerevisiae: differential response and role of Msn2 and/or Msn4 and other factors in galactose and glucose media. Mol. Cell Biol. 25, 4075–4091. doi: 10.1128/mcb.25.10.4075-4091.2005
Le Crom, S., Devaux, F., Marc, P., Zhang, X., Moye-Rowley, W. S., and Jacq, C. (2002). New insights into the pleiotropic drug resistance network from genome-wide characterization of the YRR1 transcription factor regulation system. Mol. Cell Biol. 22, 2642–2649. doi: 10.1128/mcb.22.8.2642-2649.2002
Lin, S. J., and Culotta, V. C. (1996). Suppression of oxidative damage by Saccharomyces cerevisiae ATX2, which encodes a manganese trafficking protein that localizes to Golgi-like vesicles. Mol. Cell. Biol. 16, 6303–6312. doi: 10.1128/mcb.16.11.6303
Liu, P., Wang, Y., Ye, D., Duan, L., Duan, C., and Yan, G. (2018). Effect of the addition of branched-chain amino acids to non-limited nitrogen synthesis grape must on volatile compounds and global gene expression during alcoholic fermentation. Aust. J. Grape Wine R. 24, 197–205. doi: 10.1111/ajgw.12313
Luan, Y., Zhang, B., Duan, C., and Yan, G. (2018). Effects of different pre-fermentation cold maceration time on aroma compounds of Saccharomyces cerevisiae co-fermentation with Hanseniaspora opuntiae or Pichia kudriavzevii. LWT-Food Sci. Technol. 92, 177–186. doi: 10.1016/j.lwt.2018.02.004
Luo, Z., and van Vuuren, H. J. J. (2009). Functional analyses of PAU genes in Saccharomyces cerevisiae. Microbiology 155, 4036–4049. doi: 10.1099/mic.0.030726-0
Lyons, T. J., Villa, N. Y., Regalla, L. M., Kupchak, B. R., Vagstad, A., and Eide, D. J. (2004). Metalloregulation of yeast membrane steroid receptor homologs. P. Natl. Acad. Sci. USA. 101, 5506–5511. doi: 10.1073/pnas.0306324101
Magasanik, B., and Kaiser, C. A. (2002). Nitrogen regulation in Saccharomyces cerevisiae. Gene 290, 1–18. doi: 10.1016/s0378-1119(02)00558-9
Marks, V. D., van der Merwe, G. K., and van Vuuren, H. J. (2003). Transcriptional profiling of wine yeast in fermenting grape juice: regulatory effect of diammonium phosphate. FEMS Yeast Res. 3, 269–287. doi: 10.1016/s1567-1356(02)00201-5
Martin, C. E., Oh, C., and Jiang, Y. (2007). Regulation of long chain unsaturated fatty acid synthesis in yeast. Biochem. Biophys. Acta 1771, 271–285. doi: 10.1016/j.bbalip.2006.06.010
Mauricio, J. C., Moreno, J., Zea, L., Ortega, J. M., and Medina, M. (1997). The effects of grape must fermentation conditions on volatile alcohols and esters formed by Saccharomyces cerevisiae. J. Sci. Food Agric. 75, 155–160. doi: 10.3390/foods7090147
McCusker, J. H., and Haber, J. E. (1990). Mutations in Saccharomyces cerevisiae which confer resistance to several amino acid analogs. Mol. Cell. Biol. 10, 2941–2949. doi: 10.1128/mcb.10.6.2941
McDonough, V. M., Stukey, J. E., and Martin, C. E. (1992). Specificity of unsaturated fatty acid-regulated expression of the Saccharomyces cerevisiae OLE1 gene. J. Biol. Chem. 267, 5931–5936.
Mendes-Ferreira, A., Olmo, M. D., Garcóa-Martinez, J., Jiménez- Martí, E., Mendes-Faia, A., Pérez-Ortín, J. E., et al. (2007). Transcriptional response of Saccharomyces cerevisiae to different nitrogen concentrations during alcoholic fermentation. Appl. Environ. Microbiol. 73, 3049–3060.
Navarro-Aviño, J. P., Prasad, R., Miralles, V. J., Benito, R. M., and Serrano, R. (1999). A proposal for nomenclature of aldehyde dehydrogenases in Saccharomyces cerevisiae and characterization of the stress-inducible ALD2 and ALD3 genes. Yeast 15, 829–842. doi: 10.1002/(sici)1097-0061(199907)15:10a<829::aid-yea423>3.0.co;2-9
Redon, M., Guillamon, J. M., Mas, A., and Rozes, N. (2011). Effect of growth temperature on yeast lipid composition and alcoholic fermentation at low temperature. Eur. Food Res. Technol. 232, 517–527. doi: 10.1007/s00217-010-1415-3
Rollero, S., Mouret, J., Sanchez, I., Camarasa, C., Ortiz-Julien, A., Sablayrolles, J., et al. (2016). Key role of lipid management in nitrogen and aroma metabolism in an evolved wine yeast strain. Microb. Cell Fact. 15:32. doi: 10.1186/s12934-016-0434-6
Rosa, M. F., and Sa-Correia, I. (1992). Ethanol tolerance and activity of plasma membrane ATPase in Kluyveromyces marxianus and Saccharomyces cerevisiae. Enzyme Microb. Tech. 14, 23–27. doi: 10.1016/0141-0229(92)90021-f
Rossignol, T., Dulau, L., Julien, A., and Blondin, B. (2003). Genome-wide monitoring of wine yeast gene expression during alcoholic fermentation. Yeast 20, 1369–1385. doi: 10.1002/yea.1046
Rossouw, D., van den Dool, A. H., Jacobson, D., and Bauer, F. F. (2010). Comparative transcriptomic and proteomic profiling of industrial wine yeast strains. Appl. Environ. Microbiol. 76, 3911–3923. doi: 10.1128/AEM.00586-10
Schehl, B., Senn, T., Lachenmeier, D. W., Rodicio, R., and Heinisch, J. J. (2007). Contribution of the fermenting yeast strain to ethyl carbamate generation in stone fruit spirits. Appl. Microbiol. Biotechnol. 74, 843–850. doi: 10.1007/s00253-006-0736-4
Swiegers, J. H., Bartowsky, E. J., Henschke, P. A., and Pretorius, I. S. (2005). Yeast and bacterial modulation of wine aroma and flavour. Aust. J. Grape Wine R. 11, 139–173. doi: 10.1111/j.1755-0238.2005.tb00285.x
Trinh, T. T. T., Woon, W. Y., Yu, B., Curran, P., and Liu, S. Q. (2010). Effect of L-isoleucine and L-phenylalanine addition on aroma compound formation during longan juice fermentation by a co-culture of Saccharomyces cerevisiae and Williopsis saturnus. S. Afr. J. Enol. Vitic. 31, 116–124.
Trotter, P. J. (2001). The genetics of fatty acid metabolism in Saccharomyces cerevisiae. Annu. Rev. Genet. 21, 97–119.
Valero, E., Millan, M. C., Mauricio, J. C., and Ortega, J. (1998). Effect of grape skin maceration on sterol, phospholipid, and fatty acid contents of Saccharomyces cerevisiae during alcoholic fermentation. Am. J. Enol. Vitic. 49, 119–124.
Varela, C., Pizarro, F., and Agosin, E. (2004). Biomass content governs fermentation rate in nitrogen-deficient wine musts. Appl. Environ. Microbiol. 70, 3392–3400. doi: 10.1128/aem.70.6.3392-3400.2004
Varela, C., Torrea, D., Schmidt, S., Ancin-Azpilicueta, C., and Henschke, P. (2012). Effect of oxygen and lipid supplementation on the volatile composition of chemically defined medium and Chardonnay wine fermented with Saccharomyces cerevisiae. Food Chem. 135, 2863–2871. doi: 10.1016/j.foodchem.2012.06.127
Varela, F., Calderon, F., Gonzalez, M. C., Colomo, B., and Suarez, J. A. (1999). Effect of clarification on the fatty acid composition of grape must and the fermentation kinetics of white wines. Eur. Food Res. Technol. 209, 439–444. doi: 10.1007/s002170050523
Wilcox, L. J., Balderes, D. A., Wharton, B., Tinkelenberg, A. H., Rao, G., and Sturley, S. L. (2002). Transcriptional profiling identifies two members of the ATP-binding-cassette transporter superfamily required for sterol uptake in yeast. J. Biol. Chem. 277, 32466–32472. doi: 10.1074/jbc.m204707200
Xu, X. Q., Cheng, G., Duan, L. L., Jiang, R., Pan, Q. H., Duan, C. Q., et al. (2015). Effect of training systems on fatty acids and their derived volatiles in Cabernet Sauvignon grapes and wines of the north foot of Mt. Tianshan. Food Chem. 181, 198–206. doi: 10.1016/j.foodchem.2015.02.082
Yoshioka, K., and Hashimoto, N. (1983). Cellular fatty acid and ester formation by brewers’ yeast. Agric. Biol. Chem. 47, 2287–2294. doi: 10.1080/00021369.1983.10865955
You, K. M., Rosenfield, C. L., and Knipple, D. C. (2003). Ethanol tolerance in the yeast Saccharomyces cerevisiae is dependent on cellular oleic acid content. Appl. Environ. Microbiol. 69, 1499–1503. doi: 10.1128/aem.69.3.1499-1503.2003
Keywords: unsaturated fatty acids, microarray analyses, Saccharomyces cerevisiae, volatile aroma compounds, wine
Citation: Yan G-L, Duan L-L, Liu P-T and Duan C-Q (2019) Transcriptional Comparison Investigating the Influence of the Addition of Unsaturated Fatty Acids on Aroma Compounds During Alcoholic Fermentation. Front. Microbiol. 10:1115. doi: 10.3389/fmicb.2019.01115
Received: 16 April 2018; Accepted: 02 May 2019;
Published: 22 May 2019.
Edited by:
Patricia Lappe-Oliveras, Universidad Nacional Autónoma de México, MexicoReviewed by:
Paola Branduardi, University of Milano-Bicocca, ItalyMaria Angelica Ganga, Universidad de Santiago de Chile, Chile
Copyright © 2019 Yan, Duan, Liu and Duan. This is an open-access article distributed under the terms of the Creative Commons Attribution License (CC BY). The use, distribution or reproduction in other forums is permitted, provided the original author(s) and the copyright owner(s) are credited and that the original publication in this journal is cited, in accordance with accepted academic practice. No use, distribution or reproduction is permitted which does not comply with these terms.
*Correspondence: Chang-Qing Duan, ZHVhbmNocUB2aXAuc2luYS5jb20=
†These authors have contributed equally to this work