- 1Laboratory of Microbial Electrochemical Systems, Department of Process Engineering and Technology of Polymer and Carbon Materials, Wrocław University of Science and Technology, Wrocław, Poland
- 2Laboratory of Microorganism Biotechnology, Faculty of Technobiology, University of Surabaya, Surabaya, Indonesia
- 3Leibniz Institute for Natural Product Research and Infection Biology – Hans-Knöll-Institute, Jena, Germany
- 4Faculty of Biological Sciences, Friedrich Schiller University, Jena, Germany
Bioelectrochemical systems (BESs) are ruled by a complex combination of biological and abiotic factors. The interplay of these factors determines the overall efficiency of BES in generating electricity and treating waste. The recent progress in bioelectrochemistry of BESs and electrobiotechnology exposed an important group of compounds, which have a significant contribution to operation and efficiency: surface-active agents, also termed surfactants. Implementation of the interfacial science led to determining several effects of synthetic and natural surfactants on BESs operation. In high pH, these amphiphilic compounds prevent the cathode electrodes from biodeterioration. Through solubilization, their presence leads to increased catabolism of hydrophobic compounds. They interfere with the surface of the electrodes leading to improved biofilm formation, while affecting its microarchitecture and composition. Furthermore, they may act as quorum sensing activators and induce the synthesis of electron shuttles produced by electroactive bacteria. On the other hand, the bioelectrochemical activity can be tailored for new, improved biosurfactant production processes. Herein, the most recent knowledge on the effects of these promising compounds in BESs is discussed.
Introduction
In recent decades, bioelectrochemical systems (BESs) have undergone dynamic development and raised increasing interest of the scientific community. The term BESs applies to several types of devices, where microorganisms play the crucial role of carrying out various types of electrochemical reactions. In a microbial fuel cell (MFC), organic matter is oxidized by heterotrophic, electroactive anodic bacteria and converted into electric current (Bennetto et al., 1983). Microbial electrolysis cells (MECs) are based on a similar principle but require additional, small portion of electrical energy input to form hydrogen at the cathode (Liu et al., 2005). The potential difference between anode and cathode electrodes is also used for separation of the ionic species in microbial desalination cells (Cao et al., 2009). Finally, the BESs may be also used for producing valuable chemicals. The processes in which the organic and inorganic compounds are produced with the support of electroactive bacteria are referred to as bioelectrosynthesis and electrofermentation (Rabaey and Rozendal, 2010; Moscoviz et al., 2016).
With these potentials, BESs are in transition to a wide variety of applications. The MFC and MEC technology has already been successfully investigated in scale up experiments and pilot scale studies to treat wastewater (Cusick et al., 2011; Ieropoulos et al., 2016; Hiegemann et al., 2019; Rossi et al., 2019a). A great effort has been made to utilize BES as a biosensing devices (Cui et al., 2019) and even MFC-driven autonomous, self-powered biosensor (Pasternak et al., 2017), or robots (Ieropoulos et al., 2010) have been reported. BES can be also used for biological remediation (Li and Yu, 2015; Ramírez-Vargas et al., 2019) and, as previously noted, as water desalination devices. In lab scale, several organic compounds were synthesized as final products of bioelectrochemical reactions such as alcohols (Mayr et al., 2019), acetate, or acetoin (Patil et al., 2015; Förster et al., 2017).
The BESs are based on microorganisms, which are the principal catalysts in these systems. Very often, the microbial biocatalyst is incorporated in a biofilm in BES. The biofilm carrying out the central reactions requires different functionalities and architectures whether the electron transfer is dominated by direct electron transfer via membrane-bound c-type cytochromes (Holmes et al., 2004; Lovley et al., 2011) or if it is dependent on indirect diffusion-limited electron transfer with soluble redox mediators (Rabaey et al., 2005; Venkataraman et al., 2011). Bioelectrochemical techniques provide a rich interplay of submolecular, molecular, cellular and, community-based mechanisms, which determine the final efficiency of BES-based processes (Yasri et al., 2019). Therefore, multiple elements affecting BES efficiency are being continuously developed and include engineering of membranes (Dizge et al., 2019; Pasternak et al., 2019), electrodes (Chong et al., 2019), microorganisms (Askitosari et al., 2019), design and modeling approaches as well as development of peripheral systems (Gadkari et al., 2018; de Ramón-Fernández et al., 2019; Tsompanas et al., 2019).
The interface between biotic and abiotic elements in such a complex environment is a matter of a particular importance. Therefore, in this mini-review, we will focus on a group of compounds, which can act at the interface of liquid, solid and gas phases, namely surfactants. Surfactants represent a wide group of amphiphilic compounds, which are widely used in the industry. Examples of widely used surfactant groups include alkyl sulfates (anionic), alkyl ammonium chlorides (cationic), betaines (amphoteric), and ethoxylates (non-ionic). Recently, significant scientific attention has been paid to substitute the chemically synthesized surfactants with biosurfactants, which can be produced by microorganisms in situ in various types of bioreactors. Biosurfactant functionalities in living systems have still not been fully understood (Chrzanowski et al., 2012). Examples of the natural roles of biosurfactants include: increasing the surface area and bioavailability of hydrophobic substrates, regulating the attachment, detachment of microorganisms to the surfaces, participating in quorum sensing mechanisms, binding of heavy metals, and antimicrobial activity (Ron and Rosenberg, 2001). The above-mentioned functions may therefore have a crucial impact on the BES performance.
Although synthetic surfactants may have their biological analogs, their functions and applications in biotic and abiotic components of BES may be entirely different. Several types of emerging interactions and applications of synthetic and biosynthesized surfactants in BES were described so far. In high pH, surfactants may prevent the biodeterioration of MFC cathodes (Pasternak et al., 2016). Their presence enhance the bioavailability of hydrophobic substrates (Hwang et al., 2019). They interfere with the surface of the electrodes, which leads to improved biofilm formation (Zhang et al., 2017). Furthermore, they may have synergistic effect with electron shuttles on MFC power performance (Pham et al., 2008). These and other interactions of biosurfactants (Figure 1 and Table 1) along with the possibility of applying BESs for synthesis of these promising and valuable compounds are discussed in this mini-review.
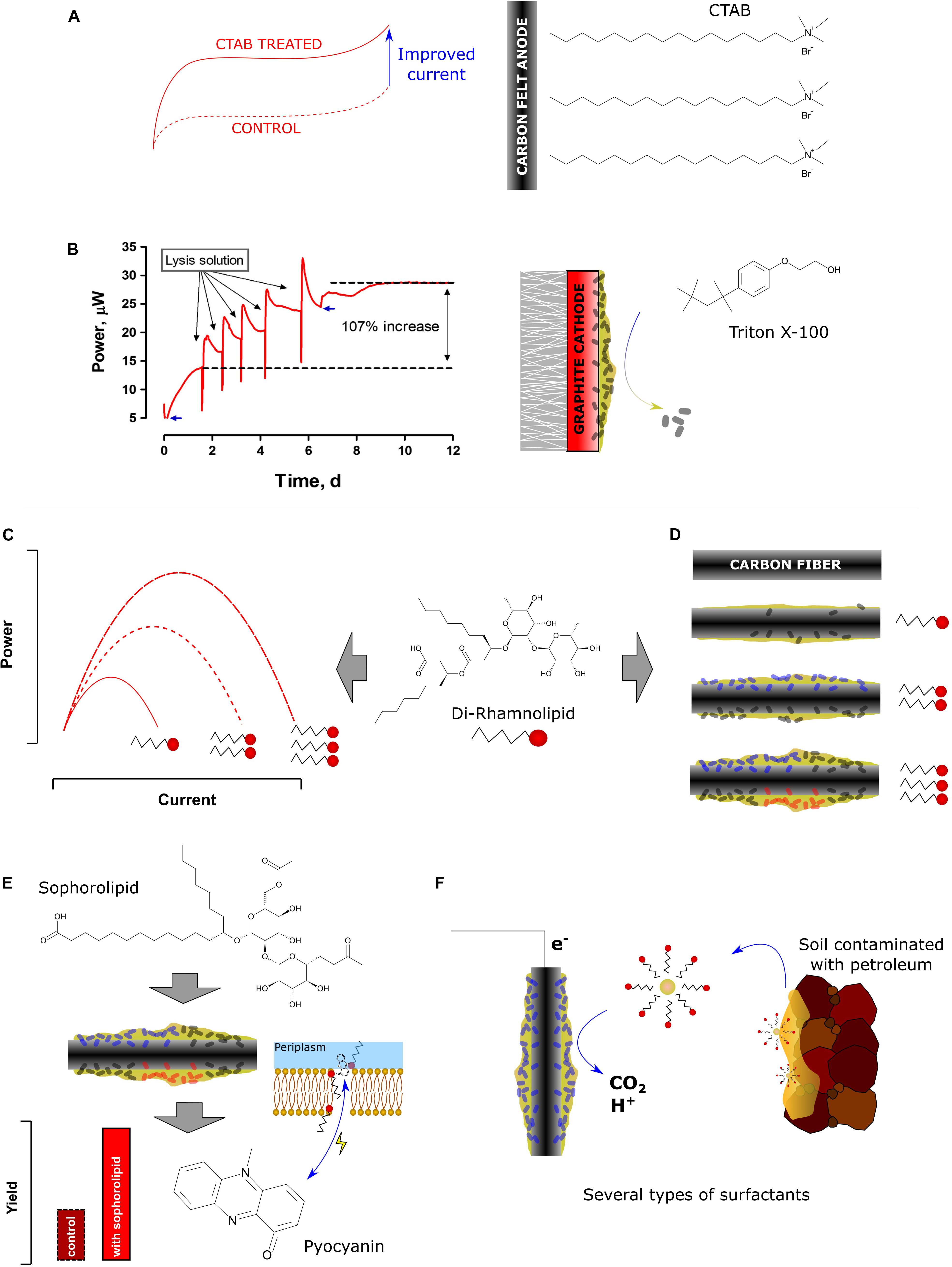
Figure 1. Structures and effects of various synthetic and biological surfactants on bioelectrochemical systems. (A) the effect of hexadecyltrimethylammonium bromide (CTAB) carbon felt surface modification on current output (Guo et al., 2014). (B) Regeneration of power performance by washing biofouled graphite cathodes [adapted from Pasternak et al. (2016) under CCBY4.0 license]. (C) improved power performance (Wen et al., 2010) and (D) biofilm structure (Zhang et al., 2017) and composition (Li et al., 2018) by increasing rhamnolipid concentration. (E) improved pyocyanin yield and cell membrane permeability through addition of sophorolipid (Shen et al., 2014). (F) solubilization of petroleum compounds for bioelectrochemical remediation (Li et al., 2018).
Effects of Surfactants and Biosurfactants on BES Components
Influence on Electron Transfer Mechanisms
One of the main obstacles for efficient mediated extracellular electron transfer is the barrier function of the bacterial cell walls and membranes, which might not allow the mediators to pass (Lovley, 2006). Surfactants may affect the membrane permeability (Sotirova et al., 2008), which is essential for electron shuttling in the mediated electron transfer (MET), and can lead to enhanced electricity generation (Yong et al., 2013). In that light, a handful of experiments showed that the external addition of surfactants like rhamnolipids (Wen et al., 2010), Tween 80 (Ren et al., 2012), SDS (Song et al., 2015), or Triton X-100 (Oluwaseun, 2015) is efficiently increasing the current generation in MFCs (Figure 1C). Notably, Wen et al. (2011) reported that the addition of the non-ionic surfactant Tween 80 in an air-cathode MFC significantly increased the power generation from 21.5 W/m3 (without surfactant) to 187 W/m3 (with surfactant). Many studies also showed that the enhancement of membrane permeability efficiently reduced the internal resistance of the BES, and thus increased the electron transfer efficiency (Yu et al., 2011). An experiment conducted by Shen et al. (2014) proved that the addition of sophorolipid effectively reduced the internal resistance (Rint) by up to ∼ 40%, whereas Cheng et al. (2018) reported the addition of trehalose lipid decreased the Rint about 43% in MFC. The same conclusion has also been obtained from a study conducted by Zheng et al. (2015), in which this parameter decreased by ∼30% for a P. aeruginosa PA01 strain, which endogenously overexpressed rhamnolipid, when compared to the wildtype strain. These mentioned studies indicate the beneficial influence of surfactants on the acceleration of mediated anodic electron transfer and the reduction of energy consumption due to internal resistances in MFCs (Zhang et al., 2017).
The positive effect has been related mainly to the role of surfactants to form transmembrane channels in the cell membrane. The presence of surfactants enables the reduction of membrane’s resistance, increasing its permeability, accelerating the transport of substances, and enhancing the substrate degradation (Singh et al., 2007). However, the addition of synthetic surfactants to the MFC can also be toxic for the bacteria (Shen et al., 2014). Therefore, a careful study to understand the optimal concentration in the MFC is required. Several studies reported that the effect of surfactants, which were originally produced by bacteria, i.e., biosurfactants such as rhamnolipids, sophorolipids, and trehalose lipids impose less toxicity to the bacteria upon addition. However, while the addition of 20 mg/L trehalose lipid surfactant in an acetate-fed Rhodococcus pyridinivorans-inoculated air cathode-MFC resulted in 1.83 times higher currents density and 5.93 times higher power density than the control, the presence of trehalose lipid above this concentration reduced the bacterial metabolism and integrity, which caused a lower electroactivity than in the control (Cheng et al., 2018). The same trend was also found in the experiment conducted by Shen et al. (2014) when sophorolipids have been added to acetate-fed P. aeruginosa-inoculated air cathode-MFC. Thus, also in case of biosurfactants, a proper concentration to be applied in MFCs needs to be determined beforehand.
The Synergistic Interaction Between Phenazines and Surfactants
An important group of redox compounds studied for natural MET are microbial phenazines, which are produced by Pseudomonas species, in particular by Pseudomonas aeruginosa. Phenazines are synthesized from chorismic acid through phenazine-1-carboxylic acid (PCA) formation, which is further converted into pyocyanin (PYO), 1-hydroxyphenazine (1-HP), and phenazine-carboxyamide (PCN). The synthesis of phenazine is encoded by two homologous operons called operon one and operon two. In P. aeruginosa PAO1 and PA14, operon two showed higher activity when compared to operon one in the phenazine synthesis and current production in oxygen-limited BES (Askitosari et al., 2019). In the environment, phenazines act as a virulence factor of P. aeruginosa by reducing molecular oxygen into reactive oxygen species, which are toxic for other microbial species (Mentel et al., 2009). On the other hand, in co-culture between P. aeruginosa and Enterobacter aerogenes, phenazines can promote synergistic interaction between species and be utilized further for electron discharge (Venkataraman et al., 2011; Schmitz and Rosenbaum, 2018). Concerning the interaction between phenazines and surfactants, in P. aeruginosa, both the endogenous rhamnolipid surfactant and phenazine synthesis are tightly controlled by a complex genetic regulatory network and they are often co-regulated (Abisado et al., 2018).
According to a study conducted by Pham et al. (2008), the non-electrochemically active bacterium Brevibacillus sp. PTH1 was able to generate currents with a combined addition of rhamnolipids up to a concentration of 1 mg/L and phenazine carboxyamide (PCN) produced by Pseudomonas sp. CMR12a in the acetate-fed MFC system. The provision of the phenazine alone did not promote electroactivity. It is likely that the surfactant promoted PCN solubility, which enabled the PCN to cross the peptidoglycan layer of gram-positive Brevibacillus sp. PTH1. Hence, this bacterium was able to employ PCN as electron shuttle through a synergistic interaction with rhamnolipids, eventually enabling these bacteria to discharge electrons to the anode. In another study, reported by Shen et al. (2014), the addition of 40 mg/L sophorolipids led to the increase of pyocyanin production, current density, and power density (1.7 times, ∼2.6 times, and 4 times, respectively, higher than control) in a P. aeruginosa-inoculated air cathode-MFC (Figure 1E). Despite these beneficial effects, the addition of exogenous synthetic or biosurfactants results in higher costs of MFC operation. Therefore, ideally, the biosurfactant should be endogenously produced by the bacteria within the MFC. This should provide physiological and economic advantages for MFC performance. Heterologous production of phenazines to enable electroactivity in Pseudomonas putida KT2440 has recently been achieved (Schmitz et al., 2015; Askitosari et al., 2019). This biotechnologically relevant bacterium has also successfully been engineered for rhamnolipid production in the past (Wittgens et al., 2011; Tiso et al., 2017). A next consequential step would now be the combined tailored production of phenazines and surfactants to evaluate the natural synergism in a controlled manner. One of the studies already showed that an endogenously stimulated surfactant production led to increased cell permeability and enhanced biofilm formation, which are both beneficial to trigger enhanced phenazine production, which in turn increases electroactivity (Zheng et al., 2015). More work is to be expected in this cutting-edge field of microbial electrophysiology.
Influence on Biofilm Formation and Stability
In BESs, the biofilm plays an essential role in electron transfer between the bacterial cells, as well as in cell-electrode interactions. The microbial ability to form the biofilm is essential in harsh, BES conditions, which are dominated by unfavorable environmental conditions, such as low aeration, substrate limitation, and incidental desiccation. The biofilm formation may be induced by the presence of biosurfactants in MFCs. Such effect was observed by several authors so far. A 96-well plate test revealed that overproduction of rhamnolipids resulted in induced biofilm formation by P. aeruginosa PAO1– the same strain that authors utilized to determine the effect of biosurfactants on MFC performance (Zheng et al., 2015). This may be explained by the amphiphilic nature of biosurfactants, which facilitate the attachment of the hydrophilic bacterial cell to the hydrophobic substratum. The same effect may thus occur at the electrode surface, in particular carbon-based materials, which are hydrophobic. Overall, the positive effect of microbially produced surfactants in initial colonization of surfaces has been long recognized in other fields of microbiology (e.g., tissue infections, agriculture, or microbial corrosion processes). In contrast, larger amounts of biosurfactants will also destabilize biofilms or cell aggregates and promote more planktonic growth. A more recent study stays in line with this hypotheses and revealed some interesting insights into an effect of rhamnolipids on biofilm adhesion and structure (Zhang et al., 2017). The authors have estimated the biofilm thickness on various levels of rhamnolipid present in the anolyte. The addition of 40, 80, and 120 mg/L of rhamnolipid resulted in the biofilm thickness of 2.03, 6.14, and 4.14 μm, respectively, while only weak attachment was observed when the biosurfactant was not present (Figure 1D). A similar trend was observed, when the biomass of the electroactive community was quantified ranging from 0.42 ± 0.06 mg/m3 (control) up to 0.86 ± 0.06 mg/m3. Lastly, the microbial community composition of the biofilm was also affected by the presence of rhamnolipid. The MFC supplemented with 40 mg/L had an increased ratio of potential electroactive species such as Geobacter, Desulfovibrio, Tolumonas, and Aeromonas, reaching 81% when compared to the control (65%). These shifts in microbial community composition may also be related to either tolerance of some groups of bacteria to specific types of surfactants or activation of the quorum sensing mechanisms caused by surfactants which could give a competitive advantages to some of the species.
Improving the Performance of Anode Electrode
The synthetic surfactants have been recently highlighted as compounds, which can react with the surface of both cathode and anode electrodes in MFCs. Guo et al. (2014) have demonstrated a facile method of increasing the hydrophilic properties of carbon felt electrodes by soaking the electrodes a few minutes in 2 mM cetyltrimethylammonium bromide (CTAB) solution (Figure 1A). Such a strategy resulted in improved bioelectrochemical performance of the anodes (Guo et al., 2014). Similar results were demonstrated by Song et al. (2015) who investigated the surface modification with the use of sodium dodecyl sulfate (SDS). They used a chemically pretreated (with sulfuric and chromic acids) and exofoliated graphite powder treated with surfactant and further with nitric acid. Although the authors have used several treatment steps that could lead to an improved hydrophilicity of the anodic surface (such as acid treatment), the only variable was a surfactant concentration. Modulating the quantity of surfactant between 0 and 20 mM resulted in improving the power production by 20% observed for 5 mM SDS and a decreased lag time, while decreased power output was recorded for 10 and 20 mM concentrations of SDS.
Improving the Performance of Cathode Electrode
At high concentrations and pH, the surfactants presence may lead to the death of the bacterial cell through disruption of the cell membrane. This process is known as the alkaline lysis and was used by Pasternak et al. (2016) for improving the performance of deteriorated cathodes (Figure 1B). The biofouling problem of cathodes in BES has been widely reported and leads to the significant decrease of MFC performance (Al Lawati et al., 2019; Noori et al., 2019; Rossi et al., 2019b). In the above mentioned study, the authors recorded nearly 91% drop of power, which clearly resulted from the growth of the biofilm at the graphite-based cathode of the ceramic MFCs. The application of a non-ionic surfactant (Triton X-100) in 0.1% concentration along with 0.2 M NaOH heated to 60°C resulted in immediate recovery of the cathodic performance to the levels exceeding 100% and removing the biofilm from the electrode surface, while NaOH heated to the same temperature had no-effect on power regeneration. Such an approach may be an alternative for more commonly proposed mechanical cleaning methods (Rossi et al., 2018) and help to prevent the biofilm recolonization.
Surfactants and Biosurfactants in BES-Biotechnological Processes
Biosynthesis
Biosurfactants are currently being produced in industrial scale by several commercial companies. Some examples include BASF Cognis (Germany) producing glycolipids, cellobiose lipids and mannosylerythritol lipids, Boruta-Zachem (Poland) producing surfactin, and Ecover (Belgium) producing sophorolipids. However, their synthesis in aerobic microbial processes is often hampered by energy demand of the bioprocess as well as extensive foaming and resulting problems with biomass retention and product recovery leading to losses of efficiency (Marchant and Banat, 2012). Applying oxygen-limited BESs for synthesis of biosurfactants may overcome some of these challenges. The first report, where the rhamnolipid synthesis was observed in MFCs was published in 2008 and their presence allowed Brevibacillus sp. to achieve extracellular electron transfer, as discussed previously (Pham et al., 2008). In another study, Schmitz et al. (2015) have discussed the possibility of using BES along with an engineered P. putida strain to synthesize detergents in oxygen-free or oxygen-limiting conditions. This process is enabled through the engineered production of phenazines as redox mediators in non-electroactive P. putida to enable anaerobic metabolic reactions. Such an oxygen limitation approach could not only lead to developing foaming-limited biotechnological process based on BESs, but potentially can also result in a higher carbon yield of the products (Schmitz et al., 2015). Furthermore, an engineered strain of P. aeruginosa has been used for inducing rhamnolipid production through overexpression of the rhamnosyltransferase gene (rhlA) in a MFC system (Zheng et al., 2015). More recently, the addition of metallic nanoparticles to the cathode electrode resulted in improved power performance and production of biosurfactant (Liu and Vipulanandan, 2017). The authors have recorded significant drop of the surface tension during growth of the biofilm at the anodic potential of −0.3 V and recorded up to 3.14 g/L of crude extracellular lipid products when the Fe-nanoparticles were used. These examples suggest that sustainable, BES-based production of biosurfactants is possible.
Biodegradation
Surfactants are often used to increase bioavailability of recalcitrant compounds during biodegradation processes (Figure 1F). The study described by Li et al. (2018) showed that the use of surfactants to stimulate the biodegradation of petroleum hydrocarbons in MFCs may affect the taxonomical composition of electroactive biofilms. The authors studied five types of surfactants among which the lecithos – ampholytic surfactant (mainly lecithin) was responsible for the highest power and biodegradation performance. The SDS and β-cyclodextrin (biosurfactant) caused the most selective shifts in bacterial communities. In another study, the presence of Tween 80 resulted in improving the PCB transformation in sediment MFC by 43.5% (Yu et al., 2017). The amphiphilic nature of surfactants was also exploited recently by Hwang et al. (2019), who investigated the biodegradation of bilge water in MFCs. The addition of 100 ppm of anionic SDS resulted in improved power output, which reached 225.3 mW/m2, while the use of non-ionic Triton X-100, resulted in two orders of magnitude lower power performance. The use of surfactants may therefore cause several effects (also negative) in biodegradation-oriented bioelectrochemical techniques and the appropriate studies should always precede their utilization.
Hydrogen Production
Surfactants have also been recognized as methanogenesis inhibiting agents (Jiang et al., 2007). Such a feature may be therefore implemented to improve the hydrogen evolution in MECs. In a recent study, Zhou et al. (2017) have tested SDS, sodium dodecyl benzene sulfonate (SDBS) and rhamnolipids for their influence on hydrogen production. The rhamnolipid addition has boosted the hydrogen yield to 12.90 mg H2/g VSS (volatile suspended solids), which was the maximum value when compared to the other surfactants and several times larger when compared to the controls. The authors claimed that rhamnolipids led to the highest acidification of the activated sludge, which was the fuel and possibly improved membrane permeability of the electroactive biofilm cells, leading to improved electrochemical parameters. Contrary results were described by Ren et al. (2012), who investigated the effect of Tween-80 on power performance of MECs, showing that surfactant concentrations up to 20 mg/L had no significant effect on current generation. Furthermore, an adverse effect was observed when its concentration reached 80 mg/L (Ren et al., 2012).
Concluding Remarks
Surfactants, either synthetic or biological, appear to be a highly reactive group of compounds in terms of their influence on several parameters, which are determining BES performance. Although a relatively rich number of surfactants were examined, only rhamnolipids and sophorolipid were included as representatives of biological surfactants. The recently emerged studies indicate that bio/surfactants may interfere with electron transfer mechanisms, especially electron shuttles such as phenazines, biofilm attachment and survival at the electrodes, as well as biofilm architecture and composition. These advantages make them an ideal target for a novel circular economy approaches in biotechnology, where the waste is being converted into value-added product. The in-situ production of surfactants in BESs would offer a great advantage of ensuring an energy-neutral bioprocess modification, which positively affects the BES performance in substrate utilization and energy production through various mechanisms. Considering the range of the effects when different types of surfactants and biosurfactants were investigated in BES, such process, however, would require careful and accurate control in order to avoid the occurrence of negative phenomena such as toxic effects on the electroactive community. Since contrary effects of different surfactants were observed on similar processes, identifying these effects for specific types of surfactants and comparative studies, as well as focus on the potential negative effects will be the main challenge to make use of their advantages in the future. The application of bio/surfactants in BES could finally lead to extended lifetime of the functional MFC elements affected by biofouling, thus leading to their increased utilization toward waste and wastewater treatment.
Author Contributions
GP conceptualized the scope and outline of the study. GP, TA, and MR performed the literature screening. GP and TA wrote the first draft of the manuscript. MR and GP critically revised the manuscript.
Funding
This work was supported by the Polish National Agency for Academic Exchange – Polish Returns grant (PPN/PPO/2018/1/00038), the National Science Centre, Poland (2019/33/B/NZ9/02774), and subsidy of Department of Process Engineering and Technology of Polymer and Carbon Materials, Wrocław University of Science and Technology. TA would like to thank RISTEK DIKTI (Indonesian Research and Education Foundation) and Deutsche Forschungsgemeinschaft (DFG, German Research Foundation) under grant number AG15671-1.
Conflict of Interest
The authors declare that the research was conducted in the absence of any commercial or financial relationships that could be construed as a potential conflict of interest.
References
Abisado, R. G., Benomar, S., Klaus, J. R., Dandekar, A. A., and Chandler, J. R. (2018). Bacterial quorum sensing and microbial community interactions. mBio 9:e02331–17. doi: 10.1128/mBio.02331-17
Al Lawati, M. J., Jafary, T., Baawain, M. S., and Al-Mamun, A. (2019). A mini review on biofouling on air cathode of single chamber microbial fuel cell; prevention and mitigation strategies. Biocatal. Agric. Biotechnol. 22:101370. doi: 10.1016/j.bcab.2019.101370
Askitosari, T. D., Boto, S. T., Blank, L. M., and Rosenbaum, M. A. (2019). Boosting heterologous phenazine production in Pseudomonas putida KT2440 through the exploration of the natural sequence space. Front. Microbiol. 10:1990. doi: 10.3389/fmicb.2019.01990
Bennetto, H. P., Stirling, J. L., Tanaka, K., and Vega, C. A. (1983). Anodic reactions in microbial fuel cells. Biotechnol. Bioeng. 25, 559–568. doi: 10.1002/bit.260250219
Cao, X., Huang, X., Liang, P., Xiao, K., Zhou, Y., Zhang, X., et al. (2009). A new method for water desalination using microbial desalination cells. Environ. Sci. Technol. 43, 7148–7152. doi: 10.1021/es901950j
Cheng, P., Shan, R., Yuan, H.-R., Dong, G., Deng, L., and Chen, Y. (2018). Improved performance of microbial fuel cells through addition of trehalose lipids. bioRxiv [Preprint]. doi: 10.1101/339267
Chong, P., Erable, B., and Bergel, A. (2019). Effect of pore size on the current produced by 3-dimensional porous microbial anodes: a critical review. Bioresour. Technol. 289:121641. doi: 10.1016/j.biortech.2019.121641
Chrzanowski, Ł, Ławniczak, Ł, and Czaczyk, K. (2012). Why do microorganisms produce rhamnolipids? World J. Microbiol. Biotechnol. 28, 401–419. doi: 10.1007/s11274-011-0854-8
Cui, Y., Lai, B., and Tang, X. (2019). Microbial fuel cell-based biosensors. Biosensors 71, 801–809. doi: 10.3390/bios9030092
Cusick, R. D., Bryan, B., Parker, D. S., Merrill, M. D., Mehanna, M., Kiely, P. D., et al. (2011). Performance of a pilot-scale continuous flow microbial electrolysis cell fed winery wastewater. Appl. Microbiol. Biotechnol. 89, 2053–2063. doi: 10.1007/s00253-011-3130-9
de Ramón-Fernández, A., Salar-García, M. J., Ruiz-Fernández, D., Greenman, J., and Ieropoulos, I. (2019). Modelling the energy harvesting from ceramic-based microbial fuel cells by using a fuzzy logic approach. Appl. Energy 251:113321. doi: 10.1016/j.apenergy.2019.113321
Dizge, N., Unal, B. O., Arikan, E. B., Karagunduz, A., and Keskinler, B. (2019). Recent progress and developments in membrane materials for microbial electrochemistry technologies: a review. Bioresour. Technol. Rep. 8:100308. doi: 10.1016/j.biteb.2019.100308
Förster, A. H., Beblawy, S., Golitsch, F., and Gescher, J. (2017). Electrode-assisted acetoin production in a metabolically engineered Escherichia coli strain. Biotechnol. Biofuels 10:65. doi: 10.1186/s13068-017-0745-9
Gadkari, S., Gu, S., and Sadhukhan, J. (2018). Towards automated design of bioelectrochemical systems: a comprehensive review of mathematical models. Chem. Eng. J. 343, 303–316. doi: 10.1016/j.cej.2018.03.005
Guo, K., Soeriyadi, A. H., Patil, S. A., Prévoteau, A., Freguia, S., Gooding, J. J., et al. (2014). Surfactant treatment of carbon felt enhances anodic microbial electrocatalysis in bioelectrochemical systems. Electrochem. Commun. 39, 1–4. doi: 10.1016/j.elecom.2013.12.001
Hiegemann, H., Littfinski, T., Krimmler, S., Lübken, M., Klein, D., Schmelz, K. G., et al. (2019). Performance and inorganic fouling of a submergible 255 L prototype microbial fuel cell module during continuous long-term operation with real municipal wastewater under practical conditions. Bioresour. Technol. 294:122227. doi: 10.1016/j.biortech.2019.122227
Holmes, D. E., Bond, D. R., O’Neil, R. A., Reimers, C. E., Tender, L. R., and Lovley, D. R. (2004). Microbial communities associated with electrodes harvesting electricity from a variety of aquatic sediments. Microb. Ecol. 48, 178–190. doi: 10.1007/s00248-003-0004-4
Hwang, J. H., Kim, K. Y., Resurreccion, E. P., and Lee, W. H. (2019). Surfactant addition to enhance bioavailability of bilge water in single chamber microbial fuel cells (MFCs). J. Hazard. Mater. 368, 732–738. doi: 10.1016/j.jhazmat.2019.02.007
Ieropoulos, I., Greenman, J., Melhuish, C., and Horsfield, I. (2010). “EcoBot-III: a robot with guts,” in Artificial Life XII: Proceedings of the 12th International Conference on the Synthesis and Simulation of Living Systems, ALIFE 2010 (Cambridge, MA: Massachusetts Institute of Technology Press)Google Scholar
Ieropoulos, I. A., Stinchcombe, A., Gajda, I., Forbes, S., Merino-Jimenez, I., Pasternak, G., et al. (2016). Pee power urinal – microbial fuel cell technology field trials in the context of sanitation. Environ. Sci. Water Res. Technol. 2, 336–343. doi: 10.1039/C5EW00270B
Jiang, S., Chen, Y., Zhou, Q., and Gu, G. (2007). Biological short-chain fatty acids (SCFAs) production from waste-activated sludge affected by surfactant. Water Res. 41, 3112–3120. doi: 10.1016/j.watres.2007.03.039
Li, W. W., and Yu, H. Q. (2015). Stimulating sediment bioremediation with benthic microbial fuel cells. Biotechnol. Adv. 33, 1–12. doi: 10.1016/j.biotechadv.2014.12.011
Li, X., Zhao, Q., Wang, X., Li, Y., and Zhou, Q. (2018). Surfactants selectively reallocated the bacterial distribution in soil bioelectrochemical remediation of petroleum hydrocarbons. J. Hazard. Mater. 344, 23–32. doi: 10.1016/j.jhazmat.2017.09.050
Liu, H., Grot, S., and Logan, B. E. (2005). Electrochemically assisted microbial production of hydrogen from acetate. Environ. Sci. Technol. 39, 4317–4320. doi: 10.1021/es050244p
Liu, J., and Vipulanandan, C. (2017). Effects of Fe, Ni, and Fe/Ni metallic nanoparticles on power production and biosurfactant production from used vegetable oil in the anode chamber of a microbial fuel cell. Waste Manag. 66, 169–177. doi: 10.1016/j.wasman.2017.04.004
Lovley, D. R. (2006). Bug juice: harvesting electricity with microorganisms. Nat. Rev. 4, 497–508. doi: 10.1038/nrmicro1442
Lovley, D. R., Ueki, T., Zhang, T., Malvankar, N. S., Shrestha, P. M., Flanagan, K. A., et al. (2011). Geobacter: the microbe electric’s physiology, ecology, and practical applications. Adv. Microb. Physiol. 59, 1–100. doi: 10.1016/B978-0-12-387661-4.00004-5
Marchant, R., and Banat, I. M. (2012). Microbial biosurfactants: challenges and opportunities for future exploitation. Trends Biotechnol. 30, 558–565. doi: 10.1016/j.tibtech.2012.07.003
Mayr, J. C., Grosch, J. H., Hartmann, L., Rosa, L. F. M., Spiess, A. C., and Harnisch, F. (2019). Resting Escherichia coli as chassis for microbial electrosynthesis: production of chiral alcohols. ChemSusChem 12, 1631–1634. doi: 10.1002/cssc.201900413
Mentel, M., Ahuja, E. G., Mavrodi, D. V., Breinbauer, R., Thomashow, L. S., and Blankenfeldt, W. (2009). Of two make one: the biosynthesis of phenazines. ChemBioChem 10, 2295–2304. doi: 10.1002/cbic.200900323
Moscoviz, R., Toledo-Alarcón, J., Trably, E., and Bernet, N. (2016). Electro-fermentation: how to drive fermentation using electrochemical systems. Trends Biotechnol. 34, 856–865. doi: 10.1016/j.tibtech.2016.04.009
Noori, M. T., Ghangrekar, M. M., Mukherjee, C. K., and Min, B. (2019). Biofouling effects on the performance of microbial fuel cells and recent advances in biotechnological and chemical strategies for mitigation. Biotechnol. Adv. 37:107420. doi: 10.1016/j.biotechadv.2019.107420
Oluwaseun, A. (2015). Bioremediation of Petroleum Hydrocarbons using Microbial Fuel Cells. Ph.D. thesis, University of Westminster Faculty of Science and Technology, London.
Pasternak, G., Greenman, J., and Ieropoulos, I. (2016). Regeneration of the power performance of cathodes affected by biofouling. Appl. Energy 173, 431–437. doi: 10.1016/j.apenergy.2016.04.009
Pasternak, G., Greenman, J., and Ieropoulos, I. (2017). Self-powered, autonomous biological oxygen demand biosensor for online water quality monitoring. Sens. Actuators B Chem. 244, 815–822. doi: 10.1016/j.snb.2017.01.019
Pasternak, G., Yang, Y., Santos, B. B., Brunello, F., Hanczyc, M. M., and Motta, A. (2019). Regenerated silk fibroin membranes as separators for transparent microbial fuel cells. Bioelectrochemistry 126, 146–155. doi: 10.1016/j.bioelechem.2018.12.004
Patil, S. A., Arends, J. B. A., Vanwonterghem, I., Van Meerbergen, J., Guo, K., Tyson, G. W., et al. (2015). Selective enrichment establishes a stable performing community for microbial electrosynthesis of acetate from CO2. Environ. Sci. Technol. 49, 8833–8843. doi: 10.1021/es506149d
Pham, T. H., Boon, N., Aelterman, P., Clauwaert, P., De Schamphelaire, L., Vanhaecke, L., et al. (2008). Metabolites produced by Pseudomonas sp. enable a gram-positive bacterium to achieve extracellular electron transfer. Appl. Microbiol. Biotechnol. 77, 1119–1129. doi: 10.1007/s00253-007-1248-6
Rabaey, K., Boon, N., Höfte, M., and Verstraete, W. (2005). Microbial phenazine production enhances electron transfer in biofuel cells. Environ. Sci. Technol. 39, 3401–3408. doi: 10.1021/es048563o
Rabaey, K., and Rozendal, R. A. (2010). Microbial electrosynthesis – revisiting the electrical route for microbial production. Nat. Rev. Microbiol. 8, 706–716. doi: 10.1038/nrmicro2422
Ramírez-Vargas, C. A., Arias, C. A., Carvalho, P., Zhang, L., Esteve-Núñez, A., and Brix, H. (2019). Electroactive biofilm-based constructed wetland (EABB-CW): a mesocosm-scale test of an innovative setup for wastewater treatment. Sci. Total Environ. 659, 796–806. doi: 10.1016/j.scitotenv.2018.12.432
Ren, L., Tokash, J. C., Regan, J. M., and Logan, B. E. (2012). Current generation in microbial electrolysis cells with addition of amorphous ferric hydroxide, Tween 80, or DNA. Int. J. Hydrogen Energy 37, 16943–16950. doi: 10.1016/j.ijhydene.2012.08.119
Ron, E. Z., and Rosenberg, E. (2001). Natural roles of biosurfactants. Environ. Microbiol. 3, 229–236. doi: 10.1046/j.1462-2920.2001.00190.x
Rossi, R., Jones, D., Myung, J., Zikmund, E., Yang, W., Gallego, Y. A., et al. (2019a). Evaluating a multi-panel air cathode through electrochemical and biotic tests. Water Res. 148, 51–59. doi: 10.1016/j.watres.2018.10.022
Rossi, R., Wang, X., Yang, W., and Logan, B. E. (2019b). Impact of cleaning procedures on restoring cathode performance for microbial fuel cells treating domestic wastewater. Bioresour. Technol. 290:121759. doi: 10.1016/j.biortech.2019.121759
Rossi, R., Yang, W., Zikmund, E., Pant, D., and Logan, B. E. (2018). In situ biofilm removal from air cathodes in microbial fuel cells treating domestic wastewater. Bioresour. Technol. 265, 200–206. doi: 10.1016/j.biortech.2018.06.008
Schmitz, S., Nies, S., Wierckx, N., Blank, L. M., and Rosenbaum, M. A. (2015). Engineering mediator-based electroactivity in the obligate aerobic bacterium Pseudomonas putida KT2440. Front. Microbiol. 6:284. doi: 10.3389/fmicb.2015.00284
Schmitz, S., and Rosenbaum, M. A. (2018). Boosting mediated electron transfer in bioelectrochemical systems with tailored defined microbial cocultures. Biotechnol. Bioeng. 115, 2183–2193. doi: 10.1002/bit.26732
Shen, H. B., Yong, X. Y., Chen, Y. L., Liao, Z. H., Si, R. W., Zhou, J., et al. (2014). Enhanced bioelectricity generation by improving pyocyanin production and membrane permeability through sophorolipid addition in Pseudomonas aeruginosa-inoculated microbial fuel cells. Bioresour. Technol. 167, 490–494. doi: 10.1016/j.biortech.2014.05.093
Singh, A., Van Hamme, J. D., and Ward, O. P. (2007). Surfactants in microbiology and biotechnology: part 2. Application aspects. Biotechnol. Adv. 25, 99–121. doi: 10.1016/j.biotechadv.2006.10.004
Song, Y. C., Kim, D. S., Woo, J. H., Subha, B., Jang, S. H., and Sivakumar, S. (2015). Effect of surface modification of anode with surfactant on the performance of microbial fuel cell. Int. J. Energy Res. 39, 860–868. doi: 10.1002/er.3284
Sotirova, A. V., Spasova, D. I, Galabova, D. N., Karpenko, E., and Shulga, A. (2008). Rhamnolipid-biosurfactant permeabilizing effects on gram-positive and gram-negative bacterial strains. Curr. Microbiol. 56, 639–644. doi: 10.1007/s00284-008-9139-3
Tiso, T., Zauter, R., Tulke, H., Leuchtle, B., Li, W. J., Behrens, B., et al. (2017). Designer rhamnolipids by reduction of congener diversity: production and characterization. Microb. Cell Fact. 16:225. doi: 10.1186/s12934-017-0838-y
Tsompanas, M. A., You, J., Wallis, L., Greenman, J., and Ieropoulos, I. (2019). Artificial neural network simulating microbial fuel cells with different membrane materials and electrode configurations. J. Power Sources 436:226832. doi: 10.1016/j.jpowsour.2019.226832
Venkataraman, A., Rosenbaum, M. A., Perkins, S. D., Werner, J. J., and Angenent, L. T. (2011). Metabolite-based mutualism between Pseudomonas aeruginosa PA14 and Enterobacter aerogenes enhances current generation in bioelectrochemical systems. Energy Environ. Sci. 4, 4550–4559. doi: 10.1039/c1ee01377g
Wen, Q., Kong, F., Ma, F., Ren, Y., and Pan, Z. (2011). Improved performance of air-cathode microbial fuel cell through additional Tween 80. J. Power Sources 196, 899–904. doi: 10.1016/j.jpowsour.2010.09.009
Wen, Q., Kong, F., Ren, Y., Cao, D., Wang, G., and Zheng, H. (2010). Improved performance of microbial fuel cell through addition of rhamnolipid. Electrochem. Commun. 12, 1710–1713. doi: 10.1016/j.elecom.2010.10.003
Wittgens, A., Tiso, T., Arndt, T. T., Wenk, P., Hemmerich, J., Müller, C., et al. (2011). Growth independent rhamnolipid production from glucose using the non-pathogenic Pseudomonas putida KT2440. Microb. Cell Fact. 10:80. doi: 10.1186/1475-2859-10-80
Yasri, N., Roberts, E. P. L., and Gunasekaran, S. (2019). The electrochemical perspective of bioelectrocatalytic activities in microbial electrolysis and microbial fuel cells. Energy Rep. 5, 1116–1136. doi: 10.1016/j.egyr.2019.08.007
Yong, Y. C., Yu, Y. Y., Yang, Y., Liu, J., Wang, J. Y., and Song, H. (2013). Enhancement of extracellular electron transfer and bioelectricity output by synthetic porin. Biotechnol. Bioeng. 110, 408–416. doi: 10.1002/bit.24732
Yu, H., Wan, H., Feng, C., Yi, X., Liu, X., Ren, Y., et al. (2017). Microbial polychlorinated biphenyl dechlorination in sediments by electrical stimulation: the effect of adding acetate and nonionic surfactant. Sci. Total Environ. 580, 1371–1380. doi: 10.1016/j.scitotenv.2016.12.102
Yu, Y. Y., Chen, H. L., Yong, Y. C., Kim, D. H., and Song, H. (2011). Conductive artificial biofilm dramatically enhances bioelectricity production in Shewanella-inoculated microbial fuel cells. Chem. Commun. 47, 12825–12827. doi: 10.1039/c1cc15874k
Zhang, Y., Jiang, J., Zhao, Q., Gao, Y. Z., Wang, K., Ding, J., et al. (2017). Accelerating anodic biofilms formation and electron transfer in microbial fuel cells: role of anionic biosurfactants and mechanism. Bioelectrochemistry 117, 48–56. doi: 10.1016/j.bioelechem.2017.06.002
Zheng, T., Xu, Y. S., Yong, X. Y., Li, B., Yin, D., Cheng, Q. W., et al. (2015). Endogenously enhanced biosurfactant production promotes electricity generation from microbial fuel cells. Bioresour. Technol. 197, 416–421. doi: 10.1016/j.biortech.2015.08.136
Zhou, A., Zhang, J., Cai, W., Sun, R., Wang, G., Liu, W., et al. (2017). Comparison of chemosynthetic and biological surfactants on accelerating hydrogen production from waste activated sludge in a short-cut fermentation-bioelectrochemical system. Int. J. Hydrogen Energy 42, 9044–9050. doi: 10.1016/j.ijhydene.2016.02.075
Keywords: biosurfactant, surfactant, microbial fuel cell, bioelectrochemistry, anode, cathode, BES
Citation: Pasternak G, Askitosari TD and Rosenbaum MA (2020) Biosurfactants and Synthetic Surfactants in Bioelectrochemical Systems: A Mini-Review. Front. Microbiol. 11:358. doi: 10.3389/fmicb.2020.00358
Received: 07 November 2019; Accepted: 18 February 2020;
Published: 13 March 2020.
Edited by:
Amelia-Elena Rotaru, University of Southern Denmark, DenmarkReviewed by:
Sunil A. Patil, Indian Institute of Science Education and Research Mohali, IndiaDeepak Pant, Flemish Institute for Technological Research, Belgium
Copyright © 2020 Pasternak, Askitosari and Rosenbaum. This is an open-access article distributed under the terms of the Creative Commons Attribution License (CC BY). The use, distribution or reproduction in other forums is permitted, provided the original author(s) and the copyright owner(s) are credited and that the original publication in this journal is cited, in accordance with accepted academic practice. No use, distribution or reproduction is permitted which does not comply with these terms.
*Correspondence: Grzegorz Pasternak, Z3J6ZWdvcnoucGFzdGVybmFrQHB3ci5lZHUucGw=