- 1PhD Degree Program in Environmental Science, Environmental Science Research Center, Faculty of Science, Chiang Mai University, Chiang Mai, Thailand
- 2Department of Environmental Science, Faculty of Science and Technology, Chiang Mai Rajabhat University, Chiang Mai, Thailand
- 3Department of Biology, Faculty of Science, Research Center in Bioresources for Agriculture, Industry and Medicine, Chiang Mai University, Chiang Mai, Thailand
Paraquat is a non-selective fast-acting herbicide used to control weeds in agricultural crops. Many years of extensive use has caused environmental pollution and food toxicity. This agrochemical degrades slowly in nature, adsorbs onto clay lattices, and may require environmental remediation. Studies have shown that biosynthesized manganese oxide (BioMnOx) successfully degraded toxic synthetic compounds such as bis-phenol A and diclofenac, thus it has potential for paraquat degradation. In this experiment, P. duplex AARL G060 generated low (9.03 mg/L) and high (42.41 mg/L) concentrations of BioMnOx. The precipitated BioMnOx was observed by scanning electron microscopy (SEM), and the elemental composition was identified as Mn and O by energy-dispersive x-ray spectroscopy (EDS). The potential for BioMnOx to act as a catalyst in the degradation of paraquat was evaluated under three treatments: (1) a negative control (deionized water), (2) living alga with low BioMnOx plus hydrogen peroxide, and (3) living alga with high BioMnOx plus hydrogen peroxide. The results indicate that BioMnOx served as a catalyst in the Fenton-like reaction that could degrade more than 50% of the paraquat within 72 h. A kinetic study indicated that paraquat degradation by Fenton-like reactions using BioMnOx as a catalyst can be described by pseudo-first and pseudo-second order models. The pH level of the BioMnOx catalyst was neutral at the end of the experiment. In conclusion, BioMnOx is a viable and environmentally friendly catalyst to accelerate degradation of paraquat and other toxic chemicals.
Introduction
Non-selective herbicides, such as glyphosate and paraquat, were developed based upon their antagonistic effects on non-specific species of weeds and crops. Paraquat (1,1’-dimethyl-4,4’-bipyridinium dichloride) transforms electron flow from the photosystem and inhibits reduction of oxidized nicotinamide adenine dinucleotide phosphate (NADPC) during photosynthesis (Huang et al., 2019). Intensive paraquat usage over decades has led to widespread contamination of soil and water, and the World Health Organization (WHO) set an advisory limit for paraquat in drinking water at 10 μg/L.
Previous studies reported paraquat contamination in many countries worldwide (Huang et al., 2019). A paraquat concentration of 3.95 μg/L was reported in water samples from irrigation channels and rivers in Spain (Fernández et al., 1998). Paraquat residue in surface water in Brazil was found to be 0.279 μg/L (Veríssimo et al., 2018). Paraquat concentrations of 30.69–134.08 μg/L were detected in a stream in Mai Chau province, Vietnam (Thi Hue et al., 2018). Paraquat concentrations of 9.3–87.0 μg/L were reported in surface water in Thailand (Insuwan and Rangsriwatananon, 2017). In Malaysia, 0.6–6.9 μg/L of paraquat residue was detected in water samples (Ismail et al., 2011). These contaminants accumulate in the food chain creating public health hazards for humans, animals, and the environment (Frimpong et al., 2018).
To protect human health from the toxic effects of paraquat, removal is required. Biological methods may provide limited paraquat degradation (e.g., microorganisms utilize and degrade <1% of paraquat in soil particles (Roberts et al., 2002; Huang et al., 2019); therefore, abiological processes may be required to facilitate natural paraquat degradation. Previous studies included various methods for paraquat removal, such as adsorption on modified zeolites, activated carbon, and organoclay (Guégan et al., 2015; Sieliechi and Thue, 2015; Keawkumay et al., 2016; Pukcothanung et al., 2018). On the other hand, physicochemical processes using titanium dioxide, ozone, and various advanced oxidation processes showed potential for paraquat removal and reduction of the physical and chemical effects of this pesticide on the environment and health (Florêncio et al., 2004; Mandal et al., 2010; Wang and Xu, 2012; Deng and Zhao, 2015; Hamad et al., 2016).
The advanced oxidation process (AOP) is a conventional method for paraquat degradation based on the generation of highly reactive and non-selective hydroxyl radicals (∙OH) (Poyatos et al., 2009; Deng and Zhao, 2015; de Guimarães et al., 2016; Vagi and Petsas, 2017; Garrido-Cardenas et al., 2020). The high oxidative power of this radical can oxidize organic compounds to CO2 and H2O in aqueous solution. Fenton’s reaction is an AOP invented in 1894 when Fenton used ferrous ion and hydrogen peroxide as a reagent to improve tartaric acid oxidation (Nidheesh, 2015). Since then, this method has been widely used in wastewater treatment. The chemical process of this reaction is shown in Eqs. (1–3):
The reaction between iron and hydrogen peroxide produces hydroxyl radicals (eq. 1) with high oxidative potential. These radicals attack organic matter in wastewater (eq. 2) and generate oxidation intermediates which are further attacked by hydroxyl radicals to produce CO2 and H2O (eq. 3). However, Fenton’s reaction requires acidic conditions (i.e., a pH value in the range of 3–5 for high performance degradation of paraquat (Bishop et al., 1968; Kiwi et al., 1993; Barbusiñski and Filipek, 2001), and the acidic by-products are not environmentally acceptable. Thus, reactions to proceed at pH levels higher than that Fenton reaction.
Another non-acidic AOP is a “Fenton-like” reaction, a process that operates at nearly neutral pH. The pH value of the run-off effluent from this Fenton-like reaction is environmentally friendly and depends on other metals, such as copper, cobalt, manganese, and chromium, instead of iron (II), as catalysts for H2O2 decomposition (Nidheesh, 2015; Kim et al., 2017). The reaction of transition metals and hydrogen peroxide to generate hydroxyl radicals is shown in Eq. (4).
Various microorganisms can oxidize manganese, including bacteria, fungi, and algae (Greene and Madgwick, 1991; Li et al., 2019; Zhou and Fu, 2020). Previous studies revealed that some microalgae, such as Desmodesmus sp. WR1 and P. duplex AARL G060 increase dissolved oxygen content due to microalgal photosynthesis (Wang et al., 2017; Thongpitak et al., 2019). In addition, microalgae take up CO2 and HCO3– from the culture medium on a cellular level. A decrease in H+ in the culture medium indirectly increased pH levels and generated microenvironments with pH values greater than 9 (Richardson et al., 1988; Richardson and Stolzenbach, 1995; Knauer et al., 1999; Chi et al., 2011). These mechanisms create conditions suitable for Mn oxidation as shown in Eq. (5):
Use of microalgae to generate BioMnOx has several advantages: it is environmentally friendly, and has higher efficiency and lower operation and maintenance costs than those of chemically (Li et al., 2019); however, some microalgae are sensitive to high manganese concentrations (Knauer et al., 1999).
Manganese oxide is a transition metal oxide used as a catalyst in various reactions (Corma et al., 2005; Asgari et al., 2020). It was found to be capable of oxidizing a wide range of recalcitrant compounds, including compounds with phenolic and fluoroquinolonic moieties and some antibacterial compounds (Wu et al., 2017). Manganese oxide has gained attention as a technologically important compound for degradation of toxins in the laboratory and in the field (Kim et al., 2012; Birkner et al., 2013; Robinson et al., 2013; Furgal et al., 2015; Kim et al., 2017). However, Mn oxides are prepared via chemical methods which require high energy and chemical reagents to convert the metal ions into precipitates (Fu et al., 2010; Shaker and Abdalsalm, 2018; Tian et al., 2018).
Recent research demonstrated the application of BioMnOx produced by microorganisms for wastewater treatment (Sabirova et al., 2008; Hennebel et al., 2009; Forrez et al., 2010). Another paper reported that BioMnOx can oxidize As(III) to As(V), which is a preliminary step for As removal (Tani et al., 2004). The removal of 17α-Ethinylestradiol (EE2) by oxidation in flow through bioreactors was achieved up to 57% using a BioMnOx catalyst (Hennebel et al., 2009). In addition, BioMnOx produced by green microalgae was used to degrade phenols and small organic molecules (Wang et al., 2017). For example, diclofenac, a non-steroidal anti-inflammatory drug, was removed by BioMnOx at neutral pH. This was 10-fold faster than removal with chemically synthesized MnOx (Forrez et al., 2010). However, the application of BioMnOx as a catalyst in paraquat degradation was not investigated, so the objective of this experiment was to evaluate the feasibility of using BioMnOx as a catalyst for paraquat (as model pollutant compound) degradation. The BioMnOx was prepared using living microalga P. duplex AARL G060 via a biological reaction process. The results of this study demonstrate the feasibility of applying BioMnOx for removal of harmful agents in the environment.
Materials and Methods
Living Microalga Culture of Pediastrum duplex AARL G060
Living green microalga P. duplex AARL G060 was obtained from the Applied Algal Research Laboratory (AARL), Department of Biology, Faculty of Science, Chiang Mai University, Thailand. Thongpitak et al. (2019) demonstrated that P. duplex can generate BioMnOx at high concentrations of Mn, and so this algal strain was selected for use in this study. An axenic culture of microalgal stock was maintained in Jaworski’s Medium under the following condition: 72.51 μE⋅m–2⋅s–1 light intensity from a light emitting diode, 25°C ambient temperature, and continuous shaking at 130 rpm.
Chemicals and Reagents
Chromatographic separation of paraquat was performed by application of high-performance liquid chromatography (HPLC). Paraquat (98% pure) was purchased from MilliporeSigma. Acetonitrile of HPLC grade was purchased from Merck KGaA. All other reagents were analytical grade.
Paraquat Stock Solution
Paraquat stock solution, at a concentration of 1,000 mg/L, was prepared by dissolving 0.6910 g of paraquat in 1,000 mL of DW. The paraquat stock solution was then diluted to the desired concentration using simple dilution methods (Knepil, 1977).
Natural Contaminated Wastewater
Water samples obtained from the surface of a rehabilitated lignite coal-mine reservoir in the northern part of Thailand (500292m E, 1966086m N). Water samples were collected in November and December 2017. Some physico-chemical parameters of the water sample were published elsewhere (Thongpitak et al., 2019). High-density polyethylene bottles of 20 L capacity were filled with natural wastewater and stored at 4°C prior to use; water samples were used to generate BioMnOx using microalgae.
Batch Experiment
Effect of Paraquat on Growth in Pediastrum duplex AARL G060
Living green microalga P. duplex AARL G060 were cultivated in water samples obtained from the rehabilitated reservoir. The microalga was cultivated with nutrients containing NaNO3 (0.09438 g/L), KH2PO4 (0.02606 g/L), CaHCO3 (0.0159 g/L), and MgSO4.7H2O (0.0500 g/L, Thongpitak et al., 2018). The initial concentration of paraquat was 10 mg/L. The initial microalgal optical density was set at 0.2 for each treatment. The batch culture of P. duplex AARL G060 was inoculated into 150 mL of modified medium in DW (LA-Pq, i.e., living microalga with paraquat) to compare to the control (modified medium in DW without paraquat). All treatments were performed in triplicate at ambient temperature and 111.81 mE m–2 s–1 light intensity using LED illumination. The microalga density was determined daily by measuring the OD665 using a Thermo Fisher ScientificTM GENESYSTM 20 Visible Spectrophotometer, and the pH value of the cultures was measured using a Starter 3100 pH Bench (Ohaus, United States). Living microalga cultures in flasks were shaken three times per day by hand to confirm that all algal cells were suspended and to prevent the dissolution of oxygen due to agitation which interferes with Mn oxidation during photosynthesis.
Production of BioMnOx by Pediastrum duplex AARL G060
Manganese concentrations in this experiment were assigned the highest Mn concentrations found in the rehabilitated reservoir (20 mg/L, Thongpitak et al., 2019). The BioMnOx nanoparticles were prepared via photosynthesis of P. duplex AARL G060 and designated as low or high concentration. At low BioMnOx concentration (LA-Low-BioMnOx), the microalga was inoculated in 300 mL of natural wastewater containing a Mn concentration of 20 mg/L. For high BioMnOx concentration (LA-High-BioMnOx), living microalga was inoculated in 900 mL of natural wastewater containing a Mn concentration of 60 mg/L, triple the concentration of low BioMnOx. After six days of cultivation, cell pellets containing BioMnOx were collected by centrifugation at 3,500 rpm for 20 min. Next, samples were washed twice with a phosphate buffer at a pH of 8.00. Then washed with DW to remove residual Mn ions from the microalgal cells. The amount of precipitated BioMnOx that remained on the cell pellets was dissolved with 10 mL of 1 mM EDTA at a pH of 3.40 and analyzed using the same method applied to Mn concentrations in the supernatant (Kenduzler and Turker, 2002; Webster et al., 2011; Thongpitak et al., 2019). The BioMnOx concentration of the microalga was determined by atomic absorption spectroscopy (AAS).
Analysis of BioMnOx Formation Using Scanning Electron Microscope With Energy Dispersive Spectroscopy (SEM-EDS)
The BioMnOx catalyst produced by P. duplex AARL G060 was collected by centrifugation at 4,000 rpm. Cell pellets were fixed with 2.5% glutaraldehyde in 0.1 M of phosphate buffer at a pH of 8.00 overnight at 4°C. The samples were then washed with a phosphate buffer at a pH of 8.00. After that, the samples were dehydrated with an ethanol concentration series. Next step, the sample was mounted on stubs and thereafter gold-sputtered (Michalak et al., 2014). Characterization of BioMnOx was observed using a JEOL-5410LV SEM equipped with an Oxford INCA EDS system to capture the distribution of the elemental composition of MnOx displayed on the microalgal cell walls. The X-ray spectrum of each sample revealed the microelement composition.
Paraquat Degradation by Fenton-Like Reactions Using BioMnOx as a Catalyst
BioMnOx generated by P. duplex AARL G060 for a duration of 6 days, was evaluated for its potential as a catalyst in paraquat degradation. The initial concentration of paraquat was 10 mg/L, which was mixed with the modified medium in DW in preparation for the following three treatments: 1) the control (i.e., the modified medium in DW), 2) the culture containing the living P. duplex AARL G060 with low BioMnOx (LA-Low-BioMnOx), and 3) a culture containing living P. duplex AARL G060 with high BioMnOx (LA-High-BioMnOx). Paraquat degradation was performed in 150 mL of culture in a 250 mL Erlenmeyer flask. Then, 13.5 mL of H2O2 was added to LA-Low-BioMnOx and LA-High-BioMnOx treatments following the method of Fang et al. (2017). During the experiment, samples from each treatment were collected at 0, 3, 6, 12, 24, 48, and 72 h by centrifuging at 3,500 rpm for 15 min to determine the amount of paraquat remaining. The pH was measured, and the paraquat degradation efficiency was calculated using eq. 6:
where C0 is the initial paraquat concentration and Ct is the concentration at time t.
Paraquat Determination by High Performance Liquid Chromatography
The HPLC was operated under the following conditions: the HPLC column was a VertiSep UPS-C18 analytical column (4.6 mm × 250 mm i.d., 5 μm) maintained at a temperature of 30°C. The mobile phase consisted of 0.14 mol sodium chloride and acetonitrile (60:40, v:v). All samples were eluted at a flow rate of 1 mL/min. The column eluent was monitored by HPLC at ambient temperature (25°C) using a Diode-Array Detection (DAD) detector (HP Series 1260, Agilent Technology). The wavelength was fixed at 257 nm. Calibration curves for the analysis were established with a standard solution of paraquat.
Data Analysis
All of the graphs and statistical analyses were completed using MicrosoftTM Excel 2016.
Results
Effect of Paraquat on Growth of P. duplex AARL G060
In this experiment, P. duplex AARL G060 was cultivated in a medium containing of N, P, C, and Mg to measure its growth (Thongpitak et al., 2019) using OD665. The results show that P. duplex AARL G060 grow well in the control medium (Figure 1A). The OD665 increased from 0.17 to 0.24. The growth of microalga also increased the pH level in the cultures; the highest pH level of a control treatment increased from 6.51 to 8.74 by the third day (Figure 1B). Meanwhile, the OD665 of the LA-Pq treatment decreased rapidly after the first day of cultivation (Figure 1A), and the pH level remained stable at approximately 6.80 until the end of the experiment.
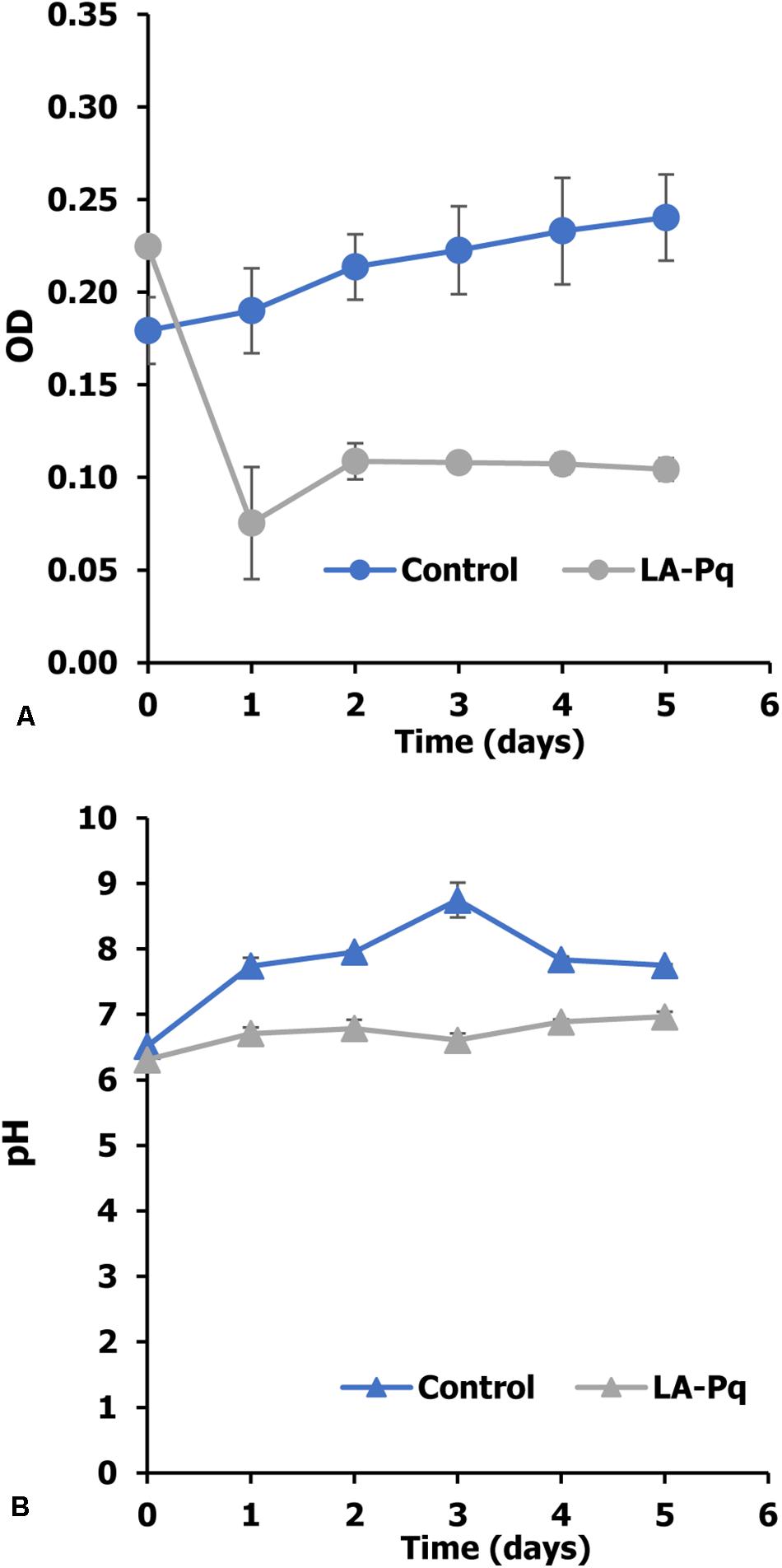
Figure 1. Effect of paraquat on growth of P. duplex AARL G060. (A) Optical density and (B) pH value. LA-Pq: living microalga with paraquat.
Biological Mn Oxide (BioMnOx) Generated by P. duplex AARL G060
The BioMnOx catalyst was generated by P. duplex AARL G060 under the modified medium supplemented with Mn. Living microalgal growth was indicated by OD665). The oxide form of the Mn (MnOx) was achieved when the pH level in culture was raised. The results indicate that P. duplex AARL G060 grow well in both LA-Low-BioMnOx and LA-High-BioMnOx treatments (Figure 2A). The OD665 value increased from 0.20 to 0.26 on the last day, with the OD value of the LA-High-BioMnOx treatment increased from 0.59 to 0.88 on the last day (Figure 2B), and the pH increased in both LA-Low-BioMnOx and LA-High-BioMnOx treatments to a level that supports Mn oxidation. The maximum pH level of LA-Low-BioMnOx treatment was 8.35 on day 3. Similarly, the pH of the LA-High-BioMnOx treatment was 8.01 on day 3. The BioMnOx concentration in LA-Low-BioMnOx treatment was 2.82 mg by day 6 (Figure 2C), and the BioMnOx concentration for the LA-High-BioMnOx treatment was 12.73 mg.
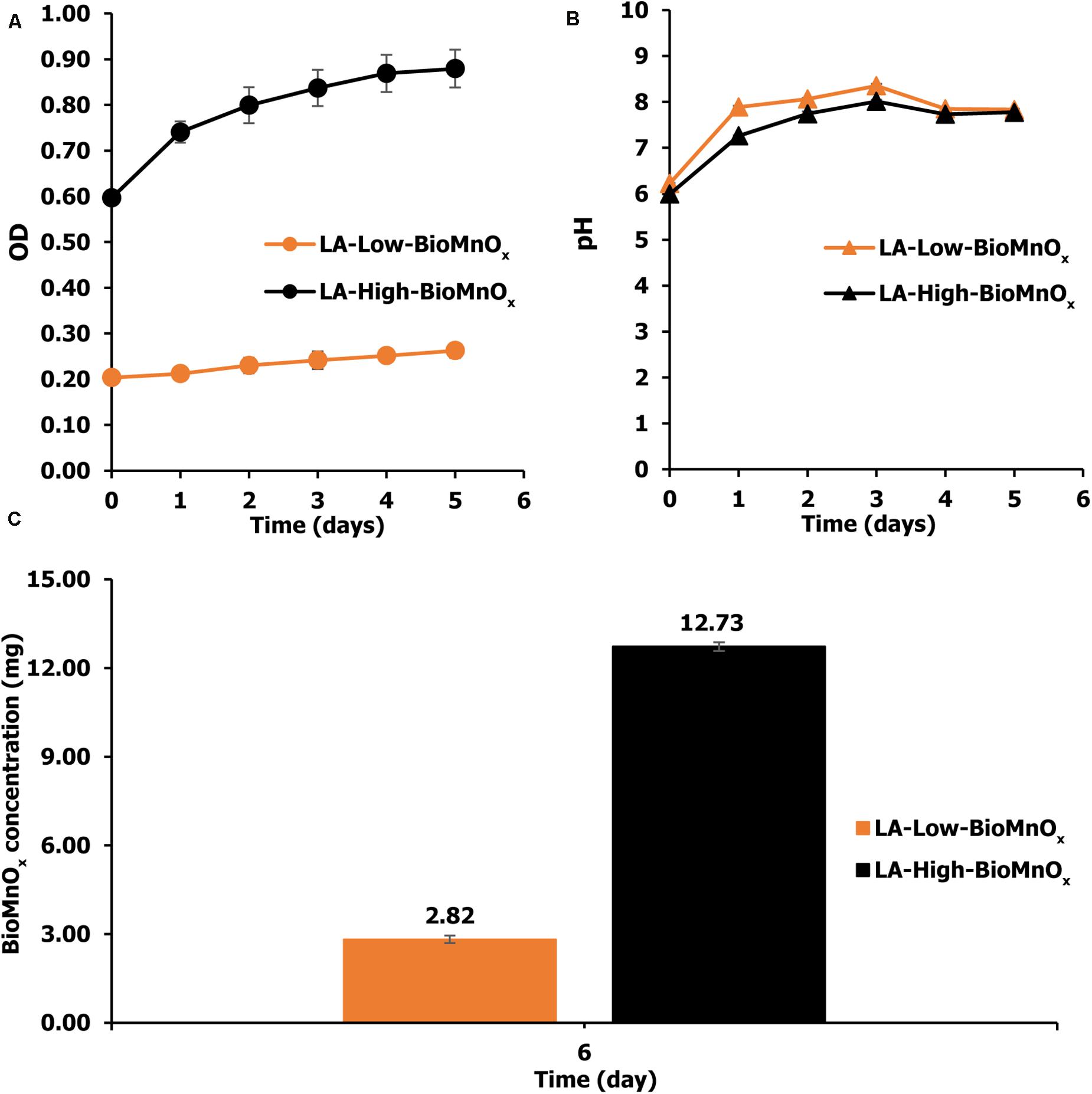
Figure 2. (A) Optical density, (B) pH value, and (C) concentration of BioMnOx generated by P. duplex AARL G060. LA-Low-BioMnOx: living microalga with low BioMnOx and LA-High-BioMnOx: living microalga with high BioMnOx.
Characterization of BioMnOx Catalyst
During the BioMnOx process, the living microalgal culture presented dark brown particles of MnOx on the cell surfaces which were investigated using the SEM. The microphotographs of P. duplex AARL G060 showed that Mn precipitation was not observed on the surface of the cells in the control group (Figure 3A). The cells of P. duplex AARL G060 were smooth and lacked Mn precipitation on cell surfaces. The SEM images of P. duplex AARL G060 with BioMnOx are presented in Figure 3A. The results reveal that after 6 days of Mn generation, dark brown particles appeared in the microalgal culture. The brown deposits were only found as aggregates of algal cells with high levels of Mn. Aggregates of MnOx were observed on cell surfaces in the SEM images shown in Figure 3B. Various sizes and irregular forms of BioMnOx were presented on the microalgal cell surfaces. The relative elemental content of BioMnOx was investigated using EDS. The EDS spectrum of the control treatment indicated the absence of Mn ions on microalgal cell surfaces. Mn was later detected in the EDS spectrum after 6 days of cultivation, and the BioMnOx consisted of Mn and O in the complex (Figure 3C).
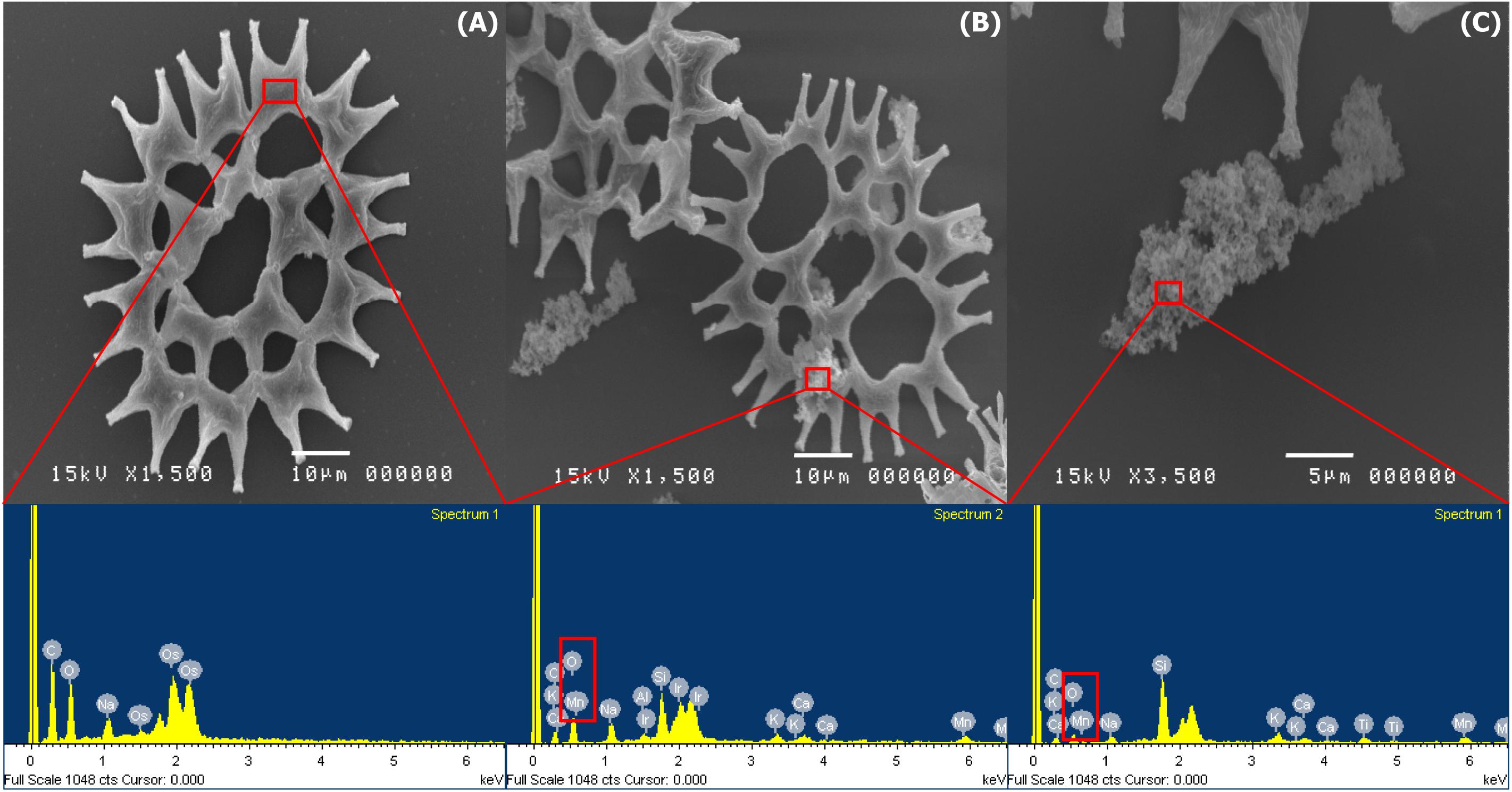
Figure 3. SEM microphotographs and EDS spectrums of BioMnOx on the cell surface of P. duplex AARL G060. (A) Control and EDS spectra of selected area on cell surface. (B,C) Microalga cell and aggregate of BioMnOx in culture and EDS spectra of selected area on day 6.
Paraquat Degradation by Fenton-Like Reactions Using BioMnOx as a Catalyst
The efficiency of paraquat degradation was studied starting with an initial paraquat concentration of 10 mg/L. The effect of BioMnOx loading on paraquat degradation efficiency shows that the LA-High-BioMnOx-H2O2 treatment has higher paraquat removal efficiency than that of LA-Low-BioMnOx-H2O2 treatment (Figure 4A). This treatment degraded paraquat from 100 to 35.24% within 12 h of contact time and degraded up to 54.64% within 72 h. The results show that catalyst loading had a considerable effect on paraquat degradation efficiency, and that paraquat degrading efficiency improved as the loading increased. The LA-Low-BioMnOx-H2O2 treatment degraded paraquat from 100 to 83.84% within 12 h of contact time. Meanwhile, the control (DW) treatment maintained fairly stable paraquat concentrations. The pH level was above 5 from the initial time to the last hour of contact time (Figure 4B), and the HPLC chromatogram shows that the paraquat was degraded (Figure 5).
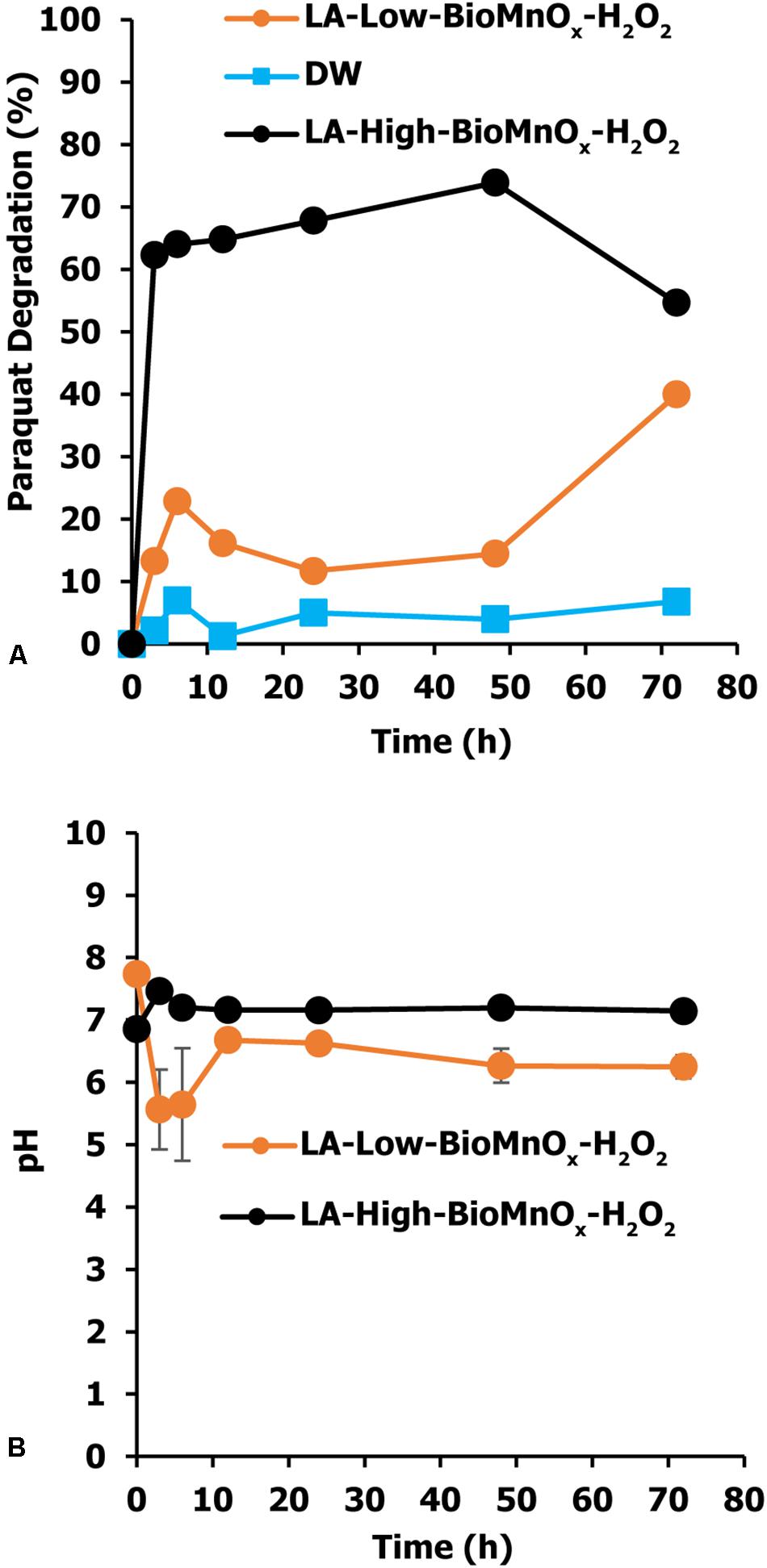
Figure 4. Paraquat degradation by Fenton-like reaction using BioMnOx as catalyst. (A) Percent paraquat degradation and (B) pH level in paraquat degradation. LA-Low-BioMnOx-H2O2, living microalga with low BioMnOx plus H2O2; DW, deionized water (control); LA, living microalga; CSMnOx-H2O2, chemical synthesis of MnOx plus H2O2; LA-High-BioMnOx, living microalga with high BioMnOx plus H2O2.
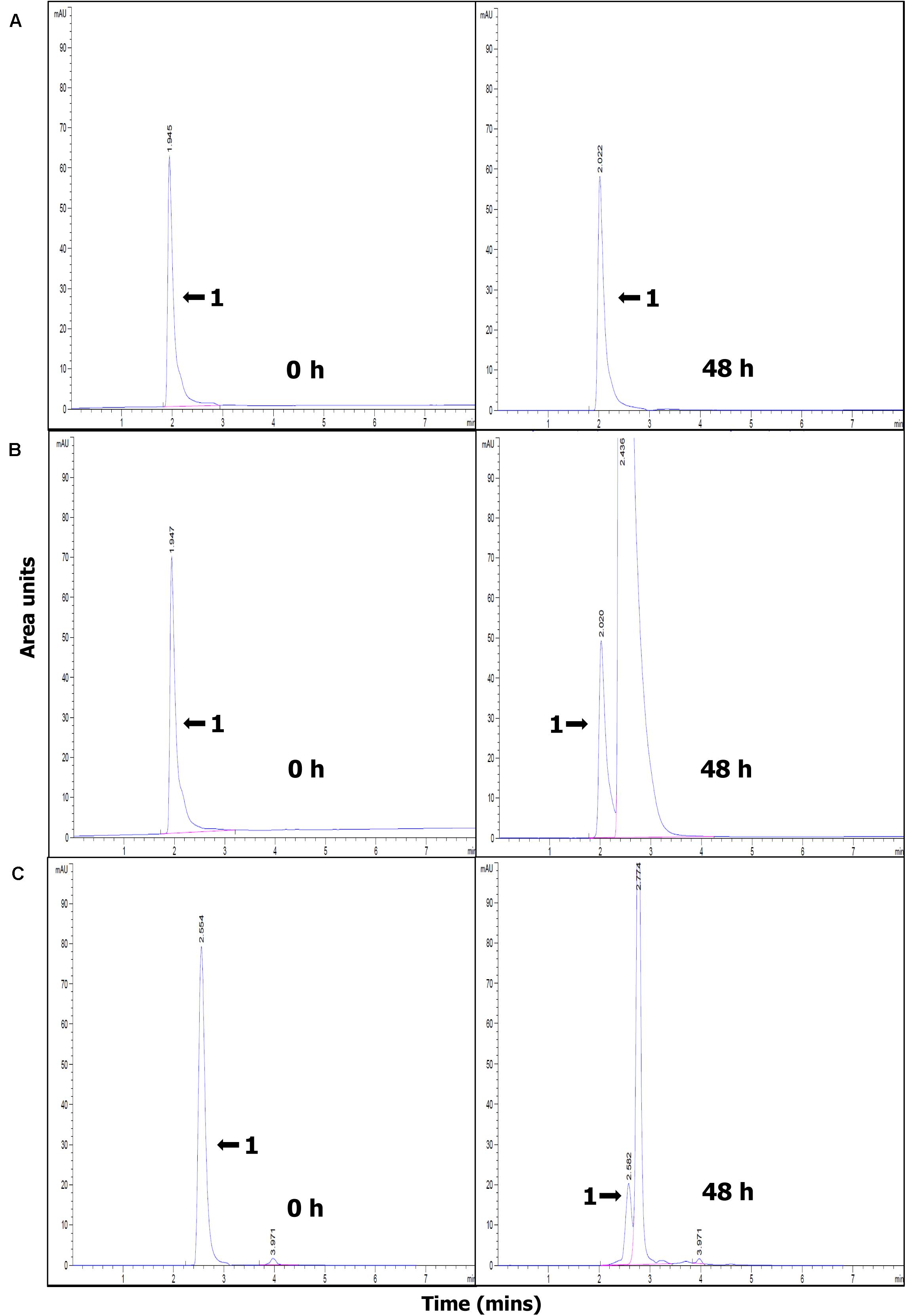
Figure 5. HPLC chromatogram showing paraquat degradation at 0 and 48 h. (A) DW (control), (B) LA-Low-BioMnOx-H2O2, and (C) LA-High-BioMnOx-H2O2 (the arrow with the number 1 indicates the peak representing paraquat). DW, deionized water; LA-Low-BioMnOx-H2O2, living microalga with low BioMnOx plus H2O2; LA-High-BioMnOx, living microalga with high BioMnOx plus H2O2.
Kinetic Study of Paraquat Degradation
A kinetic study of paraquat degradation in a Fenton-like reaction using BioMnOx as a catalyst was performed, and the pseudo-first order and pseudo-second-order kinetic models were applied to model the kinetics of paraquat degradation under different BioMnOx concentrations. Each reaction proceeded at different rates. The pseudo first-order model is represented by Eq. (7):
where C0 and Ct represents are initial paraquat concentration and concentration at time t, k1 is the rate of degradation (constant) and the time t. Events for the pseudo-second-order model were described by Eq. (8):
in which, k2 is the constant rate of degradation.
The results of the kinetic study indicate that paraquat degradation in LA-Low-BioMnOx-H2O2 treatment was fast during the initial period of 0–6 h, and the highest R2 value for this treatment was described as pseudo-first order and pseudo-second order kinetic model (Table 1). This first phase showed that the kinetic rate constants k1 and k2 were 0.0441 h–1 and 0.006 L.mg.h–1, respectively. The degradation rates in first period were faster than paraquat degradation in the Fenton-like reactions. For the LA-High-BioMnOx-H2O2 treatment, paraquat degradation was highest during the first period (0–6 h). This treatment could be described as pseudo-first order model because the k1 value was 0.2012 h–1. Higher constant rates revealed in this treatment demonstrated paraquat degradation efficiency higher than that of the LA-Low-BioMnOx-H2O2 treatment. Meanwhile, in a later period (6–72 h), the reaction of both treatments showed low degradation rates, which indicates a slower reaction.
Discussion
The results from this study indicate that paraquat affects the growth of P. duplex AARL G060. The growth of microalga in LA-Pq treatment decreased with time. A previous study reported that paraquat accepted electrons from photosystem I, thus preventing electron transport to NADPH, and this action may block photosynthesis (Sétif, 2015; Reczek et al., 2017; Huang et al., 2019). Free radicals also react with oxygen, yielding superoxide anions, which led to the formation of hydrogen peroxide and hydroxyl radicals. The hydroxyl radicals caused changes in the ultrastructure of the cells and damaged the DNA in the microalgae (Qian et al., 2009; Zhang et al., 2014).
Living P. duplex AARL G060 converted Mn ions into solid Mn via photosynthesis and the oxidation process. After 6 days of cultivation, BioMnOx was recorded on cell walls of the microalga. Photosynthesis of the living microalga increased the pH level of the water and produced oxygen. The oxygen was released to the environment to oxidize Mn ions and turn them into MnOx on the microalgal cell surfaces, appearing as a dark brown solid aggregates (Taguchi, 1976; Richardson et al., 1988; Renger and Wydrzynski, 1991; Richardson and Stolzenbach, 1995; Bohutskyi et al., 2016). The results are consistent with previous studies which found that Scenedesmus subspicatus oxidized Mn, and MnO was observed both intracellularly and extracellularly (Knauer et al., 1999). In addition, the green microalga Desmodesmus sp. WR1 generated 13 mg/L of BioMnOx after 3 days from an initial Mn concentration of 30 mg/L (Wang et al., 2017). The different quantities of Mn oxide depend on the level of oxygen production, the pH value, the number and size of microalgal cells, and the growth rate of each microalga species. For bacteria, previous studies revealed that the Mn-oxidizing bacterium Aeromonas hydrophila strain DS02 had a high tolerance for Mn(II) stress and generated up to 240 mg/L of Mn oxide in 6 days. The BioMnOx coupled with peroxymonosulfate (PMS) activation degraded 99.5% of 2,4-dimethylaniline within 80 min (Zhang et al., 2019). Stuetz et al. (1996) reported that algal-bacterial oxidation by Haemaetococcus sp., Chlamydomonas sp., and Chorella sp. generated 210 ± 0.04, 170 ± 0.05, and 180 ± 0.05 mg/L of BiOMnOx in 30 days, respectively (Table 2).
The application of chemical paraquat degradation methods discovered that the chemical process is a Fenton-like reaction that relies on metals, such as cerium, chromium, manganese, cobalt, and copper, to directly decompose H2O2 into ∙OH (Bokare and Choi, 2014; Nidheesh, 2015). A biological process characteristic of a Fenton-like reaction was also involved in the generation of the biocatalyst BioMnOx by living microalga. The MnO2 generated showed potential as a strong oxidant that can transform aqueous pollutants according to (eq. 9):
Then, a Fenton-like reaction, involving Mn ions proceeded as shown in Eqs. (10–13):
Interconversion between Mn2+ and Mn4+ through intermediate Mn3+ species should allow the Mn-catalyzed Fenton-like activation of H2O2. The advantages of MnOx oxidation have received intensive attention due to the large application area and environmental friendliness (Zhang et al., 2014).
The biological catalyst in the LA-Low-BioMnOx treatment easily degraded paraquat in first period (0–6 h). The kinetics of the Fenton-like reaction during this initial period was described as pseudo-first and pseudo-second order kinetic models. During this period, the Fenton-like reaction used Mn ions which could increase reaction rates. During the last period (6–72 h), this treatment showed slower reaction rates and a decrease in paraquat degradation. The reason for this phenomenon is unclear but may be explained as follows. First, when P. duplex AARL G060 synthesized BioMnOx, Mn particles were generated both intracellularly and extracellularly (Thongpitak et al., 2019). Next, in two degradation steps, Mn3+ and Mn4+ from Mn oxide on cell surfaces reacted with H2O2 and generated OH which degrades paraquat. After that, when the microalga died, intercellular metal ions were released into solution. The Mn ions most abundant in solution were water-soluble Mn2+ and Mn3+ compounds. In aerobic neutral pH conditions, the oxidation of Mn2+ to Mn4+ and interconversion between Mn2+ and Mn4+ via intermediate Mn3+ species enabled the Mn-catalyzed Fenton-like activation of H2O2 (Bokare and Choi, 2014).
The results of the LA-High-BioMnOx treatment also demonstrated the ability to degrade paraquat in solution by degrading more than 50% of the paraquat within 72 h. In addition, the amount of BioMnOx catalyst produced had a positive effect on process efficiency. The initial period (0–6 h) of this treatment showed a high kinetic rate constant (0.2012 h–1). This degradation percentage of paraquat in LA-High-BioMnOx was higher than that of LA-Low-BioMnOx treatment during this period. During the last period (6–72 h), the kinetic rate constant was lower than the first period and also indicated that degradation rates stabilized in this phase. Even for the amount of BioMnOx catalyst loading observed, the results are consistent with previous studies that attributed catalyst loading to an increase in the active substances; which, in turn, form more active radicals to contact the target pollutant (Fang et al., 2017; Abdellah et al., 2018; Sabouni and Gomaa, 2019), consistent with previous studies. Application of manganese oxide removed approximately 78% of bisphenol A within 168 h (Wang et al., 2017). Moreover, manganese oxide was used as act a catalyst in a Fenton-like reaction to completely degrade methylene blue in a short duration of 20 min (Kim et al., 2017). In addition, the co-synthesized Fe3O4-MnO2 nano-complex removed up to 96.8% of acid orange 7. The MnO catalyst performed better and degraded more toxin than either Fe3O4 or MnO2 alone.
Paraquat degradation efficiency depended on the dispersions of the catalyst. The highly aggregated BiOMnOx on microalgal cell surfaces are shown in Figure 3. The nanoparticle catalyst may be well-dispersed that could support paraquat degradation. The size of BioMnOx nanoparticles varied according to different Mn ion concentrations, microalgae concentrations, and cultivation time. Other than using viable microalgal cells, cell free extracts were also possible to biosynthesize for nano MnO (Kumar et al., 2017). In Kumar’s study, the size of nanoparticles was varied by alteration of metal ion concentrations, cell free extract concentrations, metal ion volume, cell free extract volume, and incubation temperature.
The observed paraquat degradation by the Fenton-like LA-High-BiOMnOx-H2O2 treatment after 72 h decreased. Regeneration of the catalyst could help degradation efficiency remain nearly constant. Cao et al. (2009) revealed a highly effective method for recovering the iron catalyst from Fenton and Fenton-like reactions in which samples were dewatered, dried, and baked at 350–400°C for 20–30 min. For the Mn catalyst, Poyraz et al. (2016) studied the recycling of manganese oxide cathodes for lithium-based batteries and found that thermal regeneration was a suitable method for recycling catalysts.
The HPLC profiles showed rapid disappearance of the peak corresponding to paraquat, accompanied by the appearance of new peaks. Although degradation products of paraquat were not evaluated in this study, degradation products from AOPs were suggested elsewhere. The degradation products may correspond to demethylation products such as 4,40-bipyridine and monopyridone and hydroxylation or oxidative ring cleavage products such as 4-carboxy-1-methyl-pyridinium ion, 4-picolinic acid, and hydroxyl-4-picolinic acid, in accordance with paraquat degradation by UV-ozonation (Kearney et al., 1988), or degradation products such as 4-carboxy-1-methylpyridinium ion, paraquat pyridine, and paraquat dipyridone as suggested by Florêncio et al. (2004) who studied paraquat degradation by titanium dioxide photodegradation. And Burrows et al. (2002) showed that irradiation of paraquat in the presence of oxygen led to formation of 4,4-bipyridyl and 4-picolinic acid. The degradation products of paraquat via Mn catalyzed Fenton-like reaction were not investigated in this study, but this issue should be considered in future studies.
The Fenton-like reactions using BiOMnOx as a catalyst to remove contaminants from real-life full-scale wastewater provides a viable environmentally friendly alternative technology for the treatment of industrial wastewater.
Conclusion
Based on the results of this study, P. duplex AARL G060 was found to be a potent strain of living green microalgae for BioMnOx production. The BioMnOx served as a biocatalyst that degraded 54.64% of the paraquat in aqueous solution, and the pH level of this operation was above 5. This result demonstrates that BioMnOx is an environmentally friendly alternative catalyst to remove toxins in wastewater. The insights gained from this experiment will be used to develop better treatments for degradation of toxins and remediation of wastewater.
Data Availability Statement
All datasets generated for this study are included in the article/Supplementary Material.
Author Contributions
All authors listed have made a substantial, direct and intellectual contribution to the work, and approved it for publication.
Funding
This research was partially supported by the Chiang Mai University and The Graduate School and the Research Center in Bioresources for Agriculture, Industry, and Medicine, Chiang Mai University.
Conflict of Interest
The authors declare that the research was conducted in the absence of any commercial or financial relationships that could be construed as a potential conflict of interest.
Supplementary Material
The Supplementary Material for this article can be found online at: https://www.frontiersin.org/articles/10.3389/fmicb.2020.575361/full#supplementary-material
References
Abdellah, M. H., Nosier, S. A., El-Shazly, A. H., and Mubarak, A. A. (2018). Photocatalytic decolorization of methylene blue using TiO2/UV system enhanced by air sparging. Alexandria Eng. J. 57, 3727–3735. doi: 10.1016/j.aej.2018.07.018
Asgari, G., Seidmohammadi, A., Esrafili, A., Faradmal, J., Noori Sepehr, M., and Jafarinia, M. (2020). The catalytic ozonation of diazinon using nano-MgO@CNT@Gr as a new heterogenous catalyst: the optimization of effective factors by response surface methodology. RSC Adv. 10, 7718–7731. doi: 10.1039/c9ra10095d
Barbusiñski, K., and Filipek, K. (2001). Use of Fenton’s reagent for removal of pesticides from industrial wastewater. Polish J. Environ. Stud. 10, 207–212.
Birkner, N., Nayeri, S., Pashaei, B., Najafpour, M. M., Casey, W. H., and Navrotsky, A. (2013). Energetic basis of catalytic activity of layered nanophase calcium manganese oxides for water oxidation. Proc. Natl. Acad. Sci. U.S.A. 110, 8801–8806. doi: 10.1073/pnas.1306623110
Bishop, D. F., Stern, G., Fleischman, M., and Marshall, L. S. (1968). Hydrogen peroxide catalytic oxidation of refractory organics in municipal waste waters. Ind. Eng. Chem. Process Des. Dev. 7, 110–117. doi: 10.1021/i260025a022
Bohutskyi, P., Kligerman, D., Byers, N., Nasr, L. K., Cua, C., Chow, S., et al. (2016). Effects of inoculum size, light intensity, and dose of anaerobic digestion centrate on growth and productivity of Chlorella and Scenedesmus microalgae and their poly-culture in primary and secondary wastewater. Chemosphere 19, 278–290. doi: 10.1016/j.algal.2016.09.010
Bokare, A. D., and Choi, W. (2014). Review of iron-free Fenton-like systems for activating H2O2 in advanced oxidation processes. J. Hazard Mater. 275, 121–135. doi: 10.1016/j.jhazmat.2014.04.054
Burrows, H. D., Canle, L. M., Santaballa, J. A., and Steenken, S. (2002). Reaction pathways and mechanisms of photodegradation of pesticides. J. Photochem. Photobiol. B Biol. 67, 71–108. doi: 10.1016/s1011-1344(02)00277-4
Cao, G., Sheng, M., Niu, M., Feng, W., Fei, Y. L., and Li, D. (2009). Regeneration and reuse of iron catalyst for Fenton-like reactions. J. Hazard Mater. 172, 1446–1449. doi: 10.1016/j.jhazmat.2009.08.010
Chi, Z., O’Fallon, J. V., and Chen, S. (2011). Bicarbonate produced from carbon capture for algae culture. Trends Biotechnol. 29, 537–541. doi: 10.1016/j.tibtech.2011.06.006
Corma, A., Garcia, H., and Leyva, A. (2005). Catalytic activity of palladium supported on single wall carbon nanotubes compared to palladium supported on activated carbon: study of the Heck and Suzuki couplings, aerobic alcohol oxidation and selective hydrogenation. J. Mol. Catal. A Chem. 230, 97–105. doi: 10.1016/j.molcata.2004.11.030
de Guimarães, B. S., Bernardes, A. A., Salcedo, G. M., Caldas, S. S., Jorge, M. B., Bianchini, A., et al. (2016). Photocatalytic degradation for treating multipesticide residues using [Ru(bipy)3]Cl2-Doped TiO2/SiO2 based on surface response methodology. J. Braz. Chem. Soc. 27, 2256–2263.
Deng, Y., and Zhao, R. (2015). Advanced oxidation processes (AOPs) in wastewater treatment. Curr. Pollut. Rep. 1, 167–176. doi: 10.1007/s40726-015-0015-z
Fang, Z., Zhang, D., Liu, K., Fan, J., and Zhao, J. Y. Z. W. (2017). Fenton-like oxidation of azo dye in aqueous solution using magnetic Fe3O4 -MnO2 nanocomposites as catalysts. Water Sci. Eng. 10, 326–333. doi: 10.1016/j.wse.2017.10.005
Fernández, M., Ibáñez, M., Picó, Y., and Mañes, J. (1998). Spatial and temporal trends of paraquat, diquat, and difenzoquat contamination in water from marsh areas of the Valencian community (Spain). Arch. Environ. Contam. Toxicol. 35, 377–384. doi: 10.1007/s002449900391
Florêncio, M. H., Pires, E., Castro, A. L., Nunes, M. R., Borges, C., and Costa, F. M. (2004). Photodegradation of diquat and paraquat in aqueous solutions by titanium dioxide: evolution of degradation reactions and characterisation of intermediates. Chemosphere 55, 345–355. doi: 10.1016/j.chemosphere.2003.11.013
Forrez, I., Carballa, M., Verbeken, K., Vanhaecke, L., Ternes, T., Boon, N., et al. (2010). Diclofenac oxidation by biogenic manganese oxides. Environ. Sci. Technol. 44, 3449–3454. doi: 10.1021/es9027327
Frimpong, J. O., Ofori, E. S. K., Yeboah, S., Marri, D., Offei, B. K., Apaatah, F., et al. (2018). Evaluating the impact of synthetic herbicides on soil dwelling macrobes and the physical state of soil in an agro-ecosystem. Ecotoxicol. Environ. Saf. 156, 205–215. doi: 10.1016/j.ecoenv.2018.03.034
Fu, J., He, Z., Wang, H., Liang, W., and Guo, C. (2010). Preparation of chemical manganese dioxide from manganese sulfate. Min. Sci. Technol. 20, 877–881. doi: 10.1016/s1674-5264(09)60299-4
Furgal, K. M., Meyer, R. L., and Bester, K. (2015). Removing selected steroid hormones, biocides and pharmaceuticals from water by means of biogenic manganese oxide nanoparticles in situ at ppb levels. Chemosphere 136, 321–326. doi: 10.1016/j.chemosphere.2014.11.059
Garrido-Cardenas, J. A., Esteban-García, B., Agüera, A., Sánchez-Pérez, J. A., and Manzano-Agugliaro, F. (2020). Wastewater treatment by advanced oxidation process and their worldwide research trends. Int. J. Environ. Res. Public Health 17:170. doi: 10.3390/ijerph17010170
Greene, A. C., and Madgwick, J. C. (1991). Microbial formation of manganese oxides. Appl. Environ. Microbiol. 57, 1114–1120. doi: 10.1128/aem.57.4.1114-1120.1991
Guégan, R., Giovanela, M., Warmont, F., and Motelica-Heino, M. (2015). Nonionic organoclay: a ‘Swiss Army knife’ for the adsorption of organic micro-pollutants? J. Colloid Interface Sci. 437, 71–79. doi: 10.1016/j.jcis.2014.09.043
Hamad, D., Dhib, R., and Mehrvar, M. (2016). Photochemical degradation of aqueous polyvinyl alcohol in a continuous UV/H2O2 process: experimental and statistical analysis. J. Polym. Environ. 24, 72–83. doi: 10.1007/s10924-016-0750-2
Hennebel, T., De Gusseme, B., Boon, N., and Verstraete, W. (2009). Biogenic metals in advanced water treatment. Trends Biotechnol. 27, 90–98. doi: 10.1016/j.tibtech.2008.11.002
Huang, Y., Zhan, H., Bhatt, P., and Chen, S. (2019). Paraquat degradation from contaminated environments: current achievements and perspectives. Front. Microbiol. 10:1754. doi: 10.3389/fmicb.2019.01754
Insuwan, W., and Rangsriwatananon, K. (2017). Removal of paraquat from aqueous solutions onto zeolite LTL. Eng. J. 21, 15–23. doi: 10.4186/ej.2017.21.2.15
Ismail, B. S., Sameni, M., and Halimah, M. (2011). Evaluation of herbicide pollution in the Kerian ricefields of Perak, Malaysia. World Appl. Sci. J. 15, 05–13.
Kearney, P. C., Muldoon, M. T., Somich, C. J., Ruth, J. M., and Voaden, D. J. (1988). Biodegradation of ozonated atrazine as a wastewater disposal system. J. Agric. Food Chem. 36, 1301–1306. doi: 10.1021/jf00084a044
Keawkumay, C., Rakmae, S., Rongchapo, W., Suppakarn, N., Prayoonpokarach, S., and Wittayakun, J. (2016). Adsorption of paraquat and pirimiphos-methyl by montmorillonite modified with tetradecylammonium chloride and intragallery templating method. Adsorpt. Sci. Technol. 35, 357–371. doi: 10.1177/0263617416677351
Kenduzler, E., and Turker, A. R. (2002). Determination of iron, manganese and zinc in water samples by flame atomic absorption spectrophotometry after preconcentration with solid-phase extraction onto Ambersorb 572. Anal. Sci. 18, 917–921. doi: 10.2116/analsci.18.917
Kim, D. G., Jiang, S., Jeong, K., and Ko, S. O. (2012). Removal of 17α-Ethinylestradiol by biogenic manganese oxides produced by the Pseudomonas putida strain MnB1. Water Air Soil Pollut. 223, 837–846. doi: 10.1007/s11270-011-0906-6
Kim, E. J., Oh, D., Lee, C. S., Gong, J., Kim, J., and Chang, Y. S. (2017). Manganese oxide nanorods as a robust Fenton-like catalyst at neutral pH: crystal phase-dependent behavior. Catal. Today 282, 71–76. doi: 10.1016/j.cattod.2016.03.034
Kiwi, J., Pulgarin, C., Peringer, P., and Grätzel, M. (1993). Beneficial effects of homogeneous photo-Fenton pretreatment upon the biodegradation of anthraquinone sulfonate in waste water treatment. Appl. Catal. B Environ. 3, 85–99. doi: 10.1016/0926-3373(93)80070-t
Knauer, K., Jabusch, T., and Sigg, L. (1999). Manganese uptake and Mn(II) oxidation by the alga Scenedesmus subspicatus. Aquat. Sci. 61, 44–58. doi: 10.1007/pl00001321
Knepil, J. (1977). A short, simple method for the determination of paraquat in plasma. Clin. Chim. Acta 79, 387–390. doi: 10.1016/0009-8981(77)90433-8
Kumar, V., Singh, K., Panwar, S., and Mehta, S. K. (2017). Green synthesis of manganese oxide nanoparticles for the electrochemical sensing of p-nitrophenol. Int. Nano Lett. 7, 123–131. doi: 10.1007/s40089-017-0205-3
Li, Y., Xu, Z., Ma, H., and Hursthouse, A. S. (2019). Removal of manganese (II) from acid mine wastewater: a review of the challenges and opportunities with special emphasis on. Water 11:2493. doi: 10.3390/w11122493
Mandal, T., Maity, S., Dasgupta, D., and Datta, S. (2010). Advanced oxidation process and biotreatment: their roles in combined industrial wastewater treatment. Desalination 250, 87–94. doi: 10.1016/j.desal.2009.04.012
Michalak, I., Marycz, K., Basiñska, K., and Chojnacka, K. (2014). Using SEM-EDX and ICP-OES to investigate the elemental composition of green macroalga Vaucheria sessilis. Sci. World J. 2014:891928. doi: 10.1155/2014/891928
Nidheesh, P. V. (2015). Heterogeneous fenton catalysts for the abatement of organic pollutants from aqueous solution: a review. RSC Adv. 5, 40552–40577. doi: 10.1039/c5ra02023a
Poyatos, J. M., Muñio, M. M., Almecija, M. C., Torres, J. C., Hontoria, E., and Osorio, F. (2009). Advanced oxidation processes for wastewater treatment: state of the art. Water Air Soil Pollut. 205:187.
Poyraz, A. S., Huang, J., Cheng, S., Bock, D. C., Wu, L., Zhu, Y., et al. (2016). Effective recycling of manganese oxide cathodes for lithium based batteries. Green Chem. 18, 3414–3421. doi: 10.1039/c6gc00438e
Pukcothanung, Y., Siritanon, T., and Rangsriwatananon, K. (2018). The efficiency of zeolite Y and surfactant-modified zeolite Y for removal of 2,4-dichlorophenoxyacetic acid and 1,1’-dimethyl-4,4’-bipyridinium ion. Micropor. Mesopor. Mater. 258, 131–140. doi: 10.1016/j.micromeso.2017.08.035
Qian, H., Chen, W., Sun, L., Liu, W., and Fu, Z. (2009). Inhibitory effects of paraquat on photosynthesis and the response to oxidative stress in Chlorella vulgaris. Ecotoxicology 18, 537–543. doi: 10.1007/s10646-009-0311-8
Reczek, C. R., Birsoy, K., Kong, H., Martínez-Reyes, I., Wang, T., Gao, P., et al. (2017). A CRISPR screen identifies a pathway required for paraquat-induced cell death. Nat. Chem. Biol. 13, 1274–1279. doi: 10.1038/nchembio.2499
Renger, G., and Wydrzynski, T. (1991). The role of manganese in photosynthetic water oxidation. Biol. Met. 4, 73–80. doi: 10.1007/bf01135382
Richardson, L. L., Aguilar, C., and Nealson, K. H. (1988). Manganese oxidation in pH and O2 microenvironments produced by phytoplankton. Limnol. Oceanogr. 33, 352–363. doi: 10.4319/lo.1988.33.3.0352
Richardson, L. L., and Stolzenbach, K. D. (1995). Phytoplankton cell size and the development of microenvironments. FEMS Microbiol. Ecol. 16, 185–191. doi: 10.1111/j.1574-6941.1995.tb00282.x
Roberts, T. R., Dyson, J. S., and Lane, M. C. G. (2002). Deactivation of the biological activity of paraquat in the soil environment: a review of long-term environmental fate. J. Agric. Food Chem. 50, 3623–3631. doi: 10.1021/jf011323x
Robinson, D. M., Go, Y. B., Mui, M., Gardner, G., Zhang, Z., Mastrogiovanni, D., et al. (2013). Photochemical water oxidation by crystalline polymorphs of manganese oxides: structural requirements for catalysis. J. Am. Chem. Soc. 135, 3494–3501. doi: 10.1021/ja310286h
Sabirova, J. S., Cloetens, L. F. F., Vanhaecke, L., Forrez, I., Verstraete, W., and Boon, N. (2008). Manganese-oxidizing bacteria mediate the degradation of 17α-ethinylestradiol. Microb. Biotechnol. 1, 507–512. doi: 10.1111/j.1751-7915.2008.00051.x
Sabouni, R., and Gomaa, H. (2019). Photocatalytic degradation of pharmaceutical micro-pollutants using ZnO. Environ. Sci. Pollut. Res. 26, 5372–5380. doi: 10.1007/s11356-018-4051-2
Sétif, P. (2015). Electron-transfer kinetics in cyanobacterial cells: methyl viologen is a poor inhibitor of linear electron flow. Biochim. Biophys. Acta Bioenerg. 1847, 212–222. doi: 10.1016/j.bbabio.2014.10.008
Shaker, K. S., and Abdalsalm, A. H. (2018). Synthesis and characterization nano structure of MnO2 via chemical method. Engr. Tech. J. 36, 946–950. doi: 10.30684/etj.36.9a.1
Sieliechi, J. M., and Thue, P. S. (2015). Removal of paraquat from drinking water by activated carbon prepared from waste wood. Desalin. Water Treat. 55, 986–998. doi: 10.1080/19443994.2014.922504
Stuetz, R. M., Greene, A. C., and Madgwick, J. C. (1996). Microalgal-facilitated bacterial oxidation of manganese. J. Ind. Microbiol. 16, 267–273. doi: 10.1007/bf01570033
Taguchi, S. (1976). Relationship between photosynthesis and cell size of marine diatom. J. Phycol. 12, 185–189. doi: 10.1111/j.1529-8817.1976.tb00499.x
Tani, Y., Miyata, N., Ohashi, M., Ohnuki, T., Seyama, H., Iwahori, K., et al. (2004). Interaction of inorganic arsenic with biogenic manganese oxide produced by a Mn-oxidizing fungus, strain KR21-2. Environ. Sci. Technol. 38, 6618–6624. doi: 10.1021/es049226i
Thi Hue, N., Nguyen, T. P. M., Nam, H., and Hoang Tung, N. (2018). Paraquat in surface water of some streams in Mai Chau province, the northern Vietnam: concentrations, profiles, and human risk assessments. J. Chem. 2018:8521012.
Thongpitak, J., Pekkoh, J., and Pumas, C. (2018). Simple medium formulation for manganese remediation by green microalga Pediastrum duplex AARLG060. Chiang Mai J. Sci. 45, 1247–1256.
Thongpitak, J., Pekkoh, J., and Pumas, C. (2019). Remediation of manganese-contaminated coal-mine water using bio-sorption and bio-oxidation by the microalga Pediastrum duplex (AARLG060): a laboratory-scale feasibility study. Front. Microbiol. 10:2605. doi: 10.3389/fmicb.2019.02605
Tian, N., Tian, X., Nie, Y., Yang, C., Zhou, Z., and Li, Y. (2018). Biogenic manganese oxide: an efficient peroxymonosulfate activation catalyst for tetracycline and phenol degradation in water. Chem. Eng. J. 352, 469–476. doi: 10.1016/j.cej.2018.07.061
Vagi, M. C., and Petsas, A. S. (2017). “Advanced oxidation processes for the removal of pesticides from wastewater: recent review and trends,” in Proceedings of the 15th International Conference on Environmental Science and Technology, Rhodes.
Veríssimo, G., Moreira, J., and Meyer, A. (2018). Paraquat contamination in surface waters of a rural stream in the mountain region in the state of Rio De Janeiro southeastern Brazil. J. Environ. Toxicol. Stud. 2:111.
Wang, J. L., and Xu, L. J. (2012). Advanced oxidation processes for wastewater treatment: formation of hydroxyl radical and application. Crit. Rev. Environ. Sci. Technol. 42, 251–325. doi: 10.1080/10643389.2010.507698
Wang, R., Wang, S., Tai, Y., Tao, R., Dai, Y., Guo, J., et al. (2017). Biogenic manganese oxides generated by green algae Desmodesmus sp. WR1 to improve bisphenol A removal. J. Hazard. Mater. 339, 310–319. doi: 10.1016/j.jhazmat.2017.06.026
Webster, R. E., Dean, A. P., and Pittman, J. K. (2011). Cadmium exposure and phosphorus limitation increases metal content in the freshwater alga Chlamydomonas reinhardtii. Environ. Sci. Technol. 45, 7489–7496. doi: 10.1021/es200814c
Wu, R., Wu, H., Jiang, X., Shen, J., Faheem, M., Sun, X., et al. (2017). The key role of biogenic manganese oxides in enhanced removal of highly recalcitrant 1,2,4-triazole from bio-treated chemical industrial wastewater. Environ. Sci. Pollut. Res. 24, 10570–10583. doi: 10.1007/s11356-017-8641-1
Zhang, W., Liu, M., Zhang, P., Yu, F., Lu, S., Li, P., et al. (2014). Effects of paraquat on photosynthetic pigments, antioxidant enzymes, and gene expression in Chlorella pyrenoidosa under mixotrophic compared with autotrophic conditions. Arch. Environ. Contam. Toxicol. 67, 593–600. doi: 10.1007/s00244-014-0067-x
Zhang, Y., Tang, Y., Qin, Z., Luo, P., Ma, Z., Tan, M., et al. (2019). A novel manganese oxidizing bacterium-Aeromonas hydrophila strain DS02: Mn(II) oxidization and biogenic Mn oxides generation. J. Hazard Mater. 367, 539–545. doi: 10.1016/j.jhazmat.2019.01.012
Keywords: microalgae, photosynthesis, bio-oxidation, herbicide, catalyst
Citation: Thongpitak J, Pumas P and Pumas C (2020) Paraquat Degradation by Biological Manganese Oxide (BioMnOx) Catalyst Generated From Living Microalga Pediastrum duplex AARL G060. Front. Microbiol. 11:575361. doi: 10.3389/fmicb.2020.575361
Received: 23 June 2020; Accepted: 24 August 2020;
Published: 15 September 2020.
Edited by:
Dayanand Kalyani, Royal Institute of Technology, SwedenReviewed by:
Sujit Jagtap, University of Illinois at Urbana-Champaign, United StatesUmesh Uttamrao Jadhav, Savitribai Phule Pune University, India
Gajanan Ghodake, Dongguk University Seoul, South Korea
Copyright © 2020 Thongpitak, Pumas and Pumas. This is an open-access article distributed under the terms of the Creative Commons Attribution License (CC BY). The use, distribution or reproduction in other forums is permitted, provided the original author(s) and the copyright owner(s) are credited and that the original publication in this journal is cited, in accordance with accepted academic practice. No use, distribution or reproduction is permitted which does not comply with these terms.
*Correspondence: Chayakorn Pumas, Y2hheWFrb3JuLnB1bWFzQGdtYWlsLmNvbQ==