- 1Department of Medical Microbiology, School of Medicine, Nanchang University, Nanchang, China
- 2The First Clinical Medical College, Nanchang University, Nanchang, China
- 3Key Laboratory of Tumor Pathogenesis and Molecular Pathology, School of Medicine, Nanchang University, Nanchang, China
Colibacillosis is an economically important infectious disease in poultry, caused by avian pathogenic Escherichia coli (APEC). Salmonella enterica serovar Enteritidis (S. Enteritidis) is a major cause of food-borne diseases in human circulated through poultry-derived products, including meat and chicken eggs. Vaccine control is the mainstream approach for combating these infections, but it is difficult to create a vaccine for the broad-spectrum protection of poultry due to multiple serotypes of these pathogens. Our previous studies have shown that outer membrane vesicles (OMVs) derived from S. enterica serovar Typhimurium mutants with a remodeled outer membrane could induce cross-protection against heteroserotypic Salmonella infection. Therefore, in this study, we further evaluated the potential of broad-spectrum vaccines based on major outer membrane protein (OMP)-deficient OMVs, including ΔompA, ΔompC, and ΔompD, and determined the protection effectiveness of these candidate vaccines in murine and chicken infection models. The results showed that ΔompA led to an increase in the production of OMVs. Notably, ΔompAΔompCΔompD OMVs showed significantly better cross-protection against S. enterica serovar Choleraesuis, S. Enteritidis, APEC O78, and Shigella flexneri 2a than did other omp-deficient OMVs, with the exception of ΔompA OMVs. Subsequently, we verified the results in the chicken model, in which ΔompAΔompCΔompD OMVs elicited significant cross-protection against S. Enteritidis and APEC O78 infections. These findings further confirmed the feasibility of improving the immunogenicity of OMVs by remodeling the outer membrane and provide a new perspective for the development of broad-spectrum vaccines based on OMVs.
Introduction
Salmonella and avian pathogenic Escherichia coli (APEC) are both important pathogens that can cause extended and acute bacterial disease, such as avian colibacillosis and fowl typhoid, and even death in poultry, especially in chickens, worldwide (Barrow, 2007; Whiley and Ross, 2015). In addition, as foodborne pathogens, Salmonella spp. are also linked to human infections, and direct contact with animals or contaminated poultry meat and eggs can also promote infection with Salmonella enterica serovar Enteritidis (S. Enteritidis; Bäumler et al., 2000; Barrow, 2007; Singer, 2015). Therefore, Salmonella infections are a concern for the global poultry industry and a threat to the health and safety of human populations worldwide. The diseases induced by Salmonella and APEC are difficult to control because of numerous serotypes of the pathogens (Gast, 2007; Ebrahimi-Nik et al., 2018). The current strategy mainly relies on the administration of antibiotics against Salmonella and APEC (Feasey, 2012). However, the abuse of antibiotics in the poultry industry has led to their low effectiveness and a serious problem of pollution with antibiotic residues (Cavicchio et al., 2015; Subedi et al., 2018; Weiss et al., 2018). In production practice, immunization with multiple or multivalent vaccines is often necessary to obtain a protective effect against these bacteria. However, there still may be an immune failure, which leads to substantial losses in aquaculture and other industries. Therefore, continuous screening for effective vaccine candidates should be performed as a safer and more effective way to improve the effectiveness of cross-protective immunity.
Outer membrane vesicles (OMVs), which are naturally produced by Gram-negative bacteria, have a spherical structure with a bilayer membrane, which contains mainly biologically active components (Kuehn and Kesty, 2005; Ellis and Kuehn, 2010). Bacterial-derived OMVs are heterogeneous complexes, which contain lipopolysaccharide (LPS), lipoproteins, and outer membrane proteins (OMPs), considered to be the prototype of pathogen-associated molecular patterns (Kuehn and Kesty, 2005). In our previous study, we evaluated the immunogenicity of OMVs derived from S. enterica serovar Typhimurium (S. Typhimurium) with truncated LPS and found that intranasal or intraperitoneal immunization with OMVs from the waaC and rfbP mutants obviously elicited significantly higher cross-reactive IgG levels and provided increased cross-protection against S. enterica serovar Choleraesuis (S. Choleraesuis) and S. Enteritidis compared with OMVs from other truncated LPS mutations (Liu et al., 2016b). Further, flagellin, as the common contaminant in OMV pellets, affected the purification of OMVs, and resulted in non-essential immune responses, thus weakening the protective immune responses produced by OMVs. Therefore, we investigated the characteristics and protective immune responses of OMVs derived from flagellin-deficient strains. The cryo-EM showed that the ΔfliCΔfliB mutations could effectively reduce the contamination of flagellin in the OMVs during density gradient centrifugation, and animal experiments revealed that non-flagellin OMVs provided efficient protection against heterologous S. Choleraesuis and S. Enteritidis (Liu et al., 2016a). Therefore, exploration of OMV-based vaccines may be an effective approach to vaccine development against homologous and heterologous serotypes of enteropathogenic bacteria.
Outer membrane proteins, as the exposed components on the OMV surface, are potential drug and vaccine targets because of their high immunogenicity and safety (Muralinath et al., 2011; Roier et al., 2012). Moreover, OMPs are anchored to the outer membrane and are essential for maintaining the integrity and selective permeability of bacterial outer membranes (Lin et al., 2002). The knockout of several OMPs could affect the synthesis or assembly of OMPs, resulting in different levels of OMP expression (Charlson et al., 2006). The main OMPs in Salmonella are OmpA, OmpC, and OmpD (Grubmeyer et al., 2012). Thus, removing these abundant proteins from the membrane not only leaves more space for recombinant proteins anchored into OMVs as a delivery vaccine but also potentially influences the membrane vesiculation and synthesis of conservative immunogenic OMPs (Meuskens et al., 2017). Therefore, a hypothesis has emerged that remodeling of the outer membrane through deletion of major OMPs may affect the cross-protection with OMVs by affecting the expression of conserved OMPs; the immunogenicity and cross-protection efficacy of OMVs derived from major OMP mutations are subsequently evaluated.
In this study, we constructed various OMP-deficient mutants and assessed the impact of OMP deficiency on the cross-protective efficacy of OMVs in animal experiments. The findings from this study may provide a basis for novel and effective OMV-based broad-spectrum vaccine candidates against reduced infection by Salmonella and APEC in chickens for future application in agricultural production.
Materials and Methods
Construction of Bacterial Mutant Strains and Cell Culture
Strains of Salmonella, Escherichia coli APEC O78, and Shigella flexneri 2a were routinely grown in Luria-Bertani (LB; Théry et al., 2018) broth or on agar at 37°C. To indicate the reproducibility of isolated OMVs derived from omp mutants in different cultures, an acidic low-magnesium minimal medium (acidic MgM), which partially reproduced the intracellular environment, was also used to culture Salmonella for purification of OMVs (Yoon et al., 2009; Bai et al., 2014). The bacterial strains and plasmids used in this study are listed in Table 1. To construct the mutant strains, LB agar with 5% sucrose was employed for sacB gene-based counterselection (Kong et al., 2011). The growth of Δasd strains required 50 mg/ml diaminopimelic acid (DAP; Nakayama et al., 1988). All primers used in the study are listed in Table 2. E. coli was transformed by electroporation, and LB agar plates containing appropriate antibiotics were used to select transformants. We used the suicide plasmid method to construct mutant strains as previously described, and the bacterial strains used were derived from previous experiments (Liu et al., 2016a,b). The resulting PCR product had a terminal A base when using the LA Taq enzyme (TaKaRa, Otsu, Japan), and the amplicon was then inserted into the pYA4278 vector with a terminal T-overhang, generated by AhdI digestion. The same strategy was used to construct pQK014 for ompA gene deletion, pQK015 for ompC gene deletion, and pQK016 for ompD gene deletion. All mutations were introduced into Salmonella Typhimurium K084, resulting in a knocked out flagellin and truncated LPS to improve the cross-proteins of OMVs, generating the K021 mutant (Liu et al., 2016a,b).
Murine macrophage RAW 264.7 cells were cultured at 37°C with 5% CO2 atmosphere in Dulbecco’s modified Eagle’s medium (Gibco BRL, United States) supplemented with 10% fetal bovine serum (HyClone, United States). All experimental protocols were approved by Nanchang University.
Purification and Protein Profiles of OMVs Derived From Mutant Strains of Salmonella
Native OMVs (without any detergent processing) were collected and isolated from culture supernatants of S. Typhimurium UK-1 and its mutants, as described previously (Muralinath et al., 2011). Briefly, 2 L bacterial culture supernatants in the logarithmic growth phase (OD600 = 1) were collected by filtration through a 0.45-μm Steritop bottle-top filter unit (Millipore, Bedford, MA, United States). Then, the vesicles present in the collected supernatants were pelleted by centrifugation (40,000 × g, 4°C, 2 h) and resuspended in Dulbecco’s phosphate-buffered saline (DPBS; Mediatech, Manassas, VA, United States). Further, pelleted OMVs were purified using density gradient centrifugation (200,000 × g, 4°C, overnight) with a discontinuous OptiPrep density gradient medium [20, 25, 30, 35, 40, and 45% OptiPrep in 10 mM HEPES (pH 6.8) with 0.85% NaCl, from top to bottom; Sigma-Aldrich, St. Louis, MO, United States].
The concentration of OMVs was determined based on total protein concentration using a bicinchoninic acid assay (Thermo Pierce, Rockford, IL, United States). Each OMV sample (10 μg, based on the protein content) was analyzed by 12% sodium dodecyl sulfate polyacrylamide gel electrophoresis (SDS-PAGE), and the gels were stained using the GelCode™ blue stain reagent (Thermo Pierce).
Nanoparticle Tracking Analysis
Particle size distribution and concentration of OMVs were determined by nanoparticle tracking analyses (NanoSight NS300, Malvern Instruments, United Kingdom). Purified OMV samples were diluted up to a concentration acceptable for analysis within the recommended range of 100–120 visible particles per frame. Triplicate videos of each sample were taken using the equipped 532-nm green laser, and a high-sensitivity video with camera level of 14 was captured and performed using NanoSight 3.0 or 3.1 software, with a threshold of between 3 and 5. Triplicate video statistics were averaged for each sample. Further, the number of OMVs per milliliter was normalized to colony-forming unit (CFU) per milliliter of each strain to determine the number of OMVs per CFU (White et al., 2018). Approaches for determining size and production met the MISEV 2018 recommendations (Théry et al., 2018).
Animals
Six- to eight-week-old female BALB/c mice were purchased from Dashou Biotechnology Co., Ltd., and were allowed to adapt to their new environment for 1 week before the animal experiments were initiated. Lohmann chickens (1 days old) were purchased from Muxing Poultry Co., Ltd. (Chengdu, China) and were maintained under standard conditions until they were 10 days old. All experiments involving animals were approved by Animal Use Ethical Committee of Nanchang University and were conducted in compliance with the Animal Welfare Act and related regulations (Hansen et al., 2017). The principles stated in the Guide for the Care and Use of Laboratory Animals were followed (National Research Council, 2011).
Immunization Protocol and Challenge
Mice of approximately equal weights were divided into groups (n = 5 each) for intranasal (20 μg OMVs based on protein content in 10 μl DPBS per animal) and intraperitoneal immunizations (5 μg OMVs based on protein content in 100 μl DPBS per animal), which were performed two times without any adjuvant use. The negative control group was served the equivalent volume of DPBS. At 4 weeks, the doses were boosted. To determine the systemic and mucosal immunity induced by OMVs, blood samples of mice were collected via orbital sinus puncture, as well as vaginal secretions by repeatedly flushing the animals with 0.1 ml of PBS buffer at 2-week intervals after the first immunization. The blood serum and the supernatant of a vaginal wash were centrifuged and stored at −80°C for future use. To calculate the rate of protection, 5 weeks after the booster immunization, the mice were challenged with a lethal dose of S. Typhimurium S100 (1.1 × 109 CFU, determined by continuously diluting and then counting on the LB plate) in 20 μl of buffered saline with 0.01% gelatin (BSG) via oral gavage. In another experiment, mice were immunized as above and then challenged with 1.3 × 107 CFU of S. Choleraesuis (∼100-fold LD50), 1.2 × 107 CFU of S. Enteritidis (∼100-fold LD50), 1.4 × 108 CFU of APEC O78 (∼100-fold LD50), and 1.1 × 107 CFU of S. flexneri 2a (∼100-fold LD50) in 20 μl of BSG 5 weeks after the booster immunization by oral gavage. Mice were monitored daily for weight, illness, and signs of obvious discomfort, distress, or pain. Mice that exhibited a weight loss of greater than 20% of their starting weight were euthanized via carbon dioxide followed by cervical dislocation. There were no expected deaths in this mice experiment.
To evaluate the protective efficacy of OMVs in chickens, 10-day-old Lohmann chickens were immunized intranasally (75 μg OMPs or OMVs in 100 μl DPBS per bird) at a 2-week interval as potential immune-protective administration, and 100 μl DPBS was used as a negative control. There were four groups of 20 chickens each (Wang et al., 2019). We marked the day of the first immunization as day 0 and the booster day as week 2. At weeks 1 and 4, 1–2 ml of blood was aseptically drawn from the chicken brachial vein after the first and second immunization. Then, we incubated the collected blood at room temperature for 30 min, removed the blood clot with sterile toothpicks, and centrifuged at 1,000 × g for 15 min. The supernatants were gently removed, and the prepared serum samples were stored at −80°C.
Protection of OMVs in chickens was evaluated in two different experiments. Experiment 1 was performed to determine the protection against wild type APEC O78 strain, and the immunized chickens were challenged via the air sac route with a lethal dose of the wild type APEC O78 strain (1.3 × 108 CFU, ∼100-fold LD50 in chickens), which were suspended in 20 μl of BSG, at 3 weeks after booster administration (week 5). After challenge, the chickens were monitored for 7 days, and the clinical signs scored as follows: none = 0, reluctance to walk = 1, coughing, head shaking, and unwillingness to eat = 2, gasping, or lying down of a long time, or both, accompanied by ruffled feathers = 3, and death = 4 (Grgić et al., 2008). Dead chickens were necropsied immediately on the day of death. At the end of the 7 days of observation period, the surviving chickens were sacrificed and necropsied. Macroscopic lesions in the heart and liver were recorded and scored separately as follows: heart and pericardium (normal = 0, turbid with excessive or cloudy fluid in the pericardial cavity or partial pericarditis = 1, marked pericarditis = 2, and severe pericarditis or death = 3) and liver (normal = 0, small amount of fibrinous exudate = 1, marked perihepatitis = 2, and severe perihepatitis or death = 3; Nagano et al., 2012). Experiment 2 was performed to determine the protection against S. Enteritidis, and the immunized chickens were inoculated orally with 5 ml of DPBS containing 1 × 1010 CFU of S. Enteritidis at 3 weeks after booster immunization. LD50 of S. Enteritidis in chicken was not determined because this strain did not cause the death of chickens in our studies. Therefore, we monitored the fecal shedding of challenge strain to evaluate the protective efficacy against S. Enteritidis in chickens. Feces (5 g) were collected by rectal palpation and then were suspended in tetrathionate broth (TT; Oxiod, United Kingdom) and 10-fold serially-diluted suspensions were plated on brilliant green agar (Oxiod) supplemented with 1 μg/ml novobiocin and 50 μg/ml nalidixic acid (Oxiod). The colonies were counted after 24 h and five colonies were randomly selected for agglutination with anti-S. Enteritidis (ThermoFisher Scientific). The animal experiments were performed twice, and the data were combined for analysis (Figure 1).
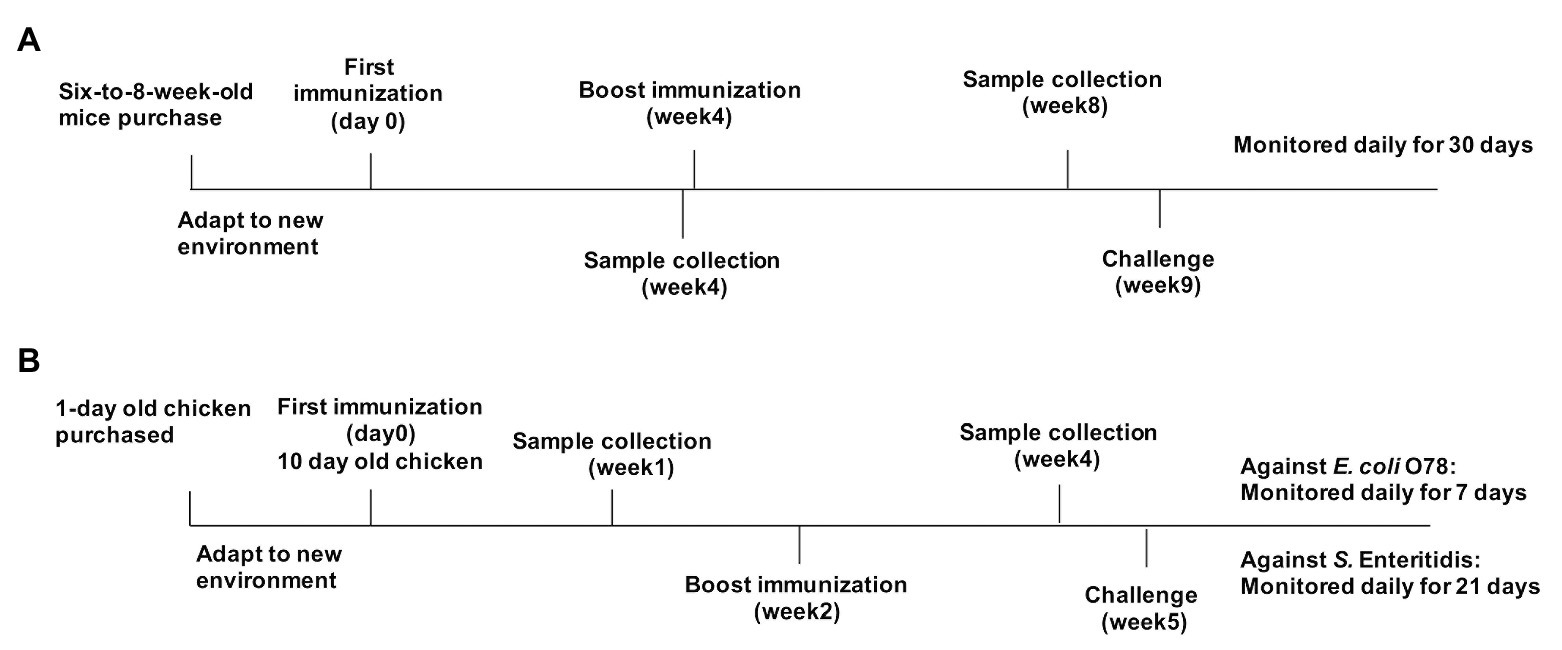
Figure 1. Immunization and challenge protocols for mouse and chicken experiments. (A) Mice were separately immunized with parental outer membrane vesicles (OMVs), OMVs from Δomp mutants, or phosphate-buffered saline (PBS) on day 0, then boosted at week 4, and challenged at 5 weeks after boost immunization. Samples were collected at weeks 4 and 8. (B) Chickens were separately immunized with parental OMVs, K021 OMVs, bacterial OMVs, bacterial outer membrane proteins (OMPs), or PBS (control) on day 0, then boosted at week 2, and challenged at week 5. Blood samples were collected at weeks 1 and 4.
Enzyme-Linked Immunosorbent Assay
Outer membrane proteins were isolated from Salmonella, APEC, and Shigella as previously described (Carlone et al., 1986). IgG and IgA responses of mice were determined using a quantitative ELISA method, and the titers of anti-OMP IgG in chicken serum samples were determined by ELISA as described below. Briefly, NUNC MaxiSorp™ 96-well plates (Thermo Scientific, Waltham, MA, United States) were coated with 2 μg of OMPs from various serotypes of Salmonella, APEC, and Shigella per well in 100 μl of sodium carbonate/bicarbonate coating buffer (pH 9.6). The plates were then incubated at 4°C overnight. To construct standard curves for each antibody isotype and make the experimental data more accurate, plates were coated in triplicate with 2-fold dilutions of appropriate purified mouse Ig isotype standard IgG and IgA (BD Biosciences, San Jose, CA, United States), starting at 0.5 μg/μl. PBS containing 0.1% Tween 20 (PBST) was used to wash the plate and 2% bovine serum albumin was used to block the plate at room temperature. Subsequently, a 100-μl volume of a suitably diluted sample was added to wells and incubated for 1 h at room temperature. After washing the wells, biotinylated goat anti-mouse IgA and IgG (Southern Biotechnology, Inc., Birmingham, AL, United States) were added. The streptavidin-alkaline phosphatase conjugate (Southern Biotechnology, Inc.) was used to develop the protein signals and the p-nitrophenyl phosphate substrate (Sigma-Aldrich) was used to detect the signal of each well. Absorbance was read at 405 nm using an automated ELISA plate reader (iMark; Bio-Rad, United States). The final Ig isotype concentration of the serum samples for quantitative ELISA was calculated using appropriate standard curves, and a log-log regression curve was calculated from at least four dilutions of the isotype standards and used for ELISA.
Opsonophagocytosis Uptake and Serum Bactericidal Activity Assay
The opsonophagocytosis assay was performed in serum samples to determine the function of OMV-raised antibodies, as previously described (Campbell and Edwards, 2000). RAW 264.7 macrophages (5 × 105 cells/well) were seeded in 24-well plates and incubated in RPMI complete medium without antibiotics (500 μl/well; Gibco, United States) for 2 h at 37°C, 5% CO2 atmosphere. To perform opsonization with the antibody and complement system, a serum sample was diluted 1:100 and incubated with bacterial suspensions of 105 CFU of APEC O78 and S. Enteritidis at 37°C for 10 min. To detect phagocytosis, we added the above opsonized bacteria to a macrophage monolayer in 24-well plates at a 1:10 (cells/bacteria) multiplicity of infection. The plates were incubated in an incubator at 37°C with 5% CO2 atmosphere for 30 min to allow phagocytosis of the bacterial cells by macrophages. One hundred microgram of gentamicin per ml cultured for 72 h was used to kill unattached extracellular bacteria, and the plates were washed three times with PBS. The intracellular bacteria were released by immediately lysing the macrophages with 1% Triton X-100 at 37°C for 10 min. The lysates were consecutively diluted 10-fold and plated onto LB plates, which were incubated at 37°C overnight. The numbers of phagocytosed bacteria were calculated by enumerating the colonies on the plates.
A modified serum bactericidal activity (SBA) assay was performed to evaluate the bactericidal ability of antibodies in serum samples in vitro. The serum was diluted 10-fold with LB medium. We cultured the wild type APEC O78 strain and S. Enteritidis to the logarithmic phase (OD600 = 0.7) and then suspended the cells in PBS at a concentration of 106 CFU/ml. Ninety microliter of the diluted sera was mixed with 10 μl of the bacterial suspensions in the wells of 96-well plates and then incubated at 37°C with shaking at 100 rpm for 1 h. The mixture was consecutively diluted 10-fold and inoculated on plates at 37°C overnight, followed by counting the viable colonies. The survival rates of bacteria in different serum samples were calculated by dividing the CFU counts after serum treatment by the original CFU counts. To make the results more accurate, we independently tested all serum samples three times.
Statistical Analysis
All statistical analyses were performed using the GraphPad Prism 5 software (GraphPad Software, San Diego, CA, United States). One- or two-way analysis of variance was performed followed by Tukey’s post hoc test to determine the significance of differences between the mean values of the experimental and control groups. Values are presented as mean ± standard deviation (SD). Differences in the survival rates among all groups were analyzed using the log-rank sum test. p < 0.05 was indicated statistically significant.
Results
Characterization and Quantification of OMVs
Outer membrane vesicles purified from strains K084, K015, K016, K017, K018, K019, K020, and K021 (all mutations were identified by PCR and gene sequencing, Supplementary Figure 1) were evaluated using protein profile assays. The major OMPs, including OmpA, OmpC, and OmpD, are marked in Figure 2A on the right, which were consistent with previous study (Liu et al., 2016a). Except the corresponding bands of the OmpC, OmpD, and OmpA proteins, the bands of other proteins in the protein profiles were basically the same. The results were consistent with the expected deficiency of certain proteins. Further, cryo-EM data showed that OMVs purified from the ΔompAΔompCΔompD mutant strains were spherical, and no other contaminant was visible, which indicated that deletion of major OMPs did not affect the structure of OMV particles (Supplementary Figure 2). We also determined the particle size of OMVs and enriched fractions during density gradient purification process using nanoparticle tracking analysis (Théry et al., 2018). The results showed that the majority of the produced OMVs derived from omp mutant strains and parental strain had a diameter of 70–90 nm, and the highest concentration of particles was in consecutive fractions 25 and 30% OptiPrep (Supplementary Figures 3, 4), which indicated that the omp knockout mutants did not affect the particle size of OMVs secreted by the bacteria. Further, we observed a statistically significant increase in OMVs release from ΔompA, ΔompAΔompC, ΔompAΔompD, and ΔompAΔompCΔompD compared to that in the parental strain (Figure 2B). The results based on protein quantification of OMVs also confirmed this observation (Figure 2C). Moreover, the results of OMVs cultured in acidic minimal medium (MgM, pH 5.0) showed similar trends as those in LB medium (Supplementary Figure 5). Compared with that of the parental strain, the omp knockout mutants showed certain effects on the OMV yields both in LB and acidic MgM growth conditions, among which the ompA mutant strain had a significantly higher yield than did the parental strain, suggesting that ΔompA might be beneficial for OMV production.
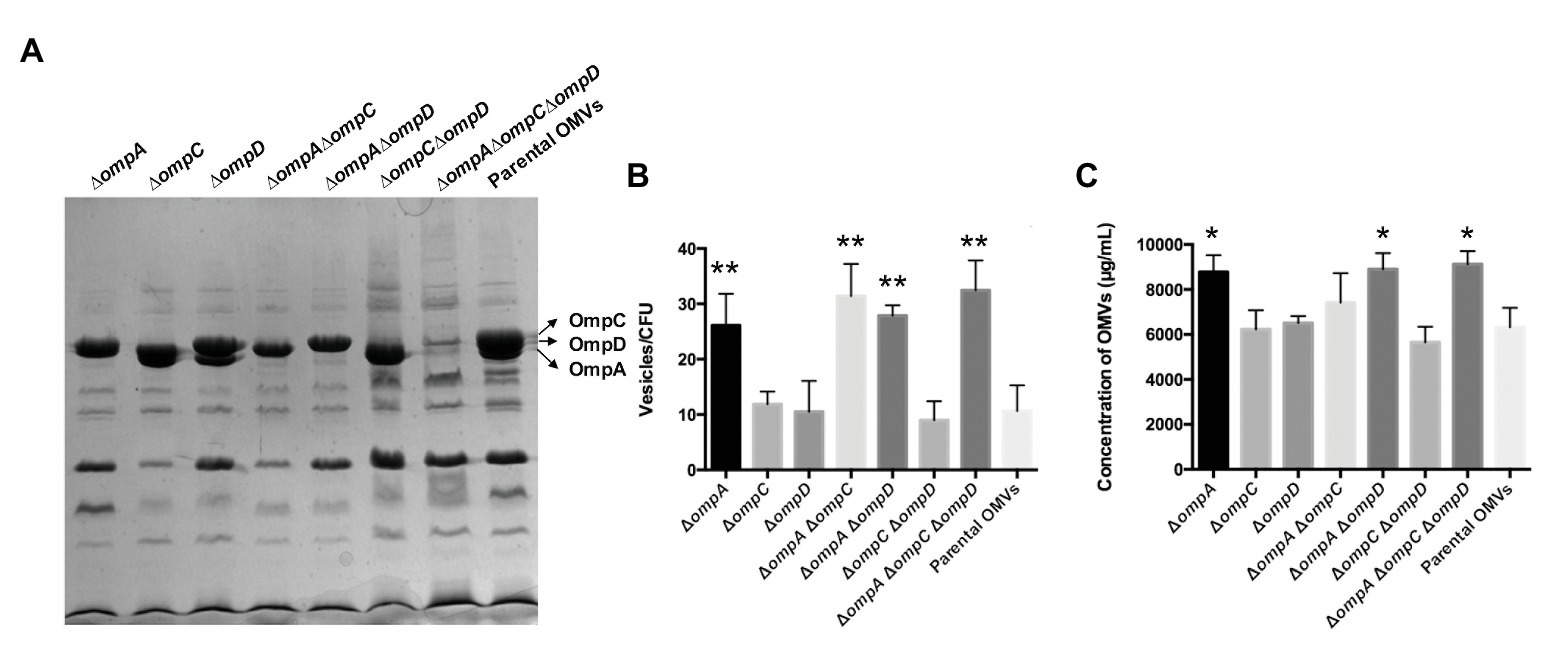
Figure 2. Characterization and quantities of OMVs derived from the ∆omp mutants and parental strain of Salmonella Typhimurium. (A) Protein contents in OMVs from the mutants and parental strain, analyzed by SDS-PAGE. The outer membrane proteins OmpC, OmpD, and OmpA are marked on the right. Strains (from left to right): K015 (∆ompA), K016 (∆ompC), K017 (∆ompD), K018 (∆ompA∆ompC), K019 (∆ompA∆ompD), K020 (∆ompC∆ompD), K021 (∆ompA∆ompC∆ompD), and χ3761 (parent). (B) Numbers of particles per milliliter were normalized to numbers of CFU per milliliter calculated from each mutant and parental strain. (C) Concentrations of OMVs derived from the ∆omp mutants and parental strain (same order as in A). Data are presented as means ± standard deviations (SD) for three independent cultures. *p < 0.05 and **p < 0.01.
IgG and IgA Immune Responses Elicited by OMVs Derived From the ∆omp Mutants and Parental Strain Against S. Typhimurium
To verify the immunogenicity of OMP-deficient OMVs, mice were intranasally and intraperitoneally injected with OMVs purified from the Δomp mutants and the parental strain, and systemic and mucosal antibody levels were measured in the mice. When the mice were intraperitoneally injected, a small bump appeared in their abdominal cavity but disappeared in less than 12 h. All OMVs elicited lower levels of IgG response via the intranasal route than that via the intraperitoneal route at 4 weeks post-immunization, whereas those from the ΔompCΔompD mutant caused a relatively higher production of serum IgG than did the other groups, except the parental OMVs, regardless of the administration route. After booster immunization, a relatively higher IgG level was observed in the group immunized with parental OMVs compared with the other groups; by contrast, the production of IgG from the ΔompCΔompD OMV group was higher than those from the other groups of Δomp mutants (Figures 3A,C). We also determined the levels of anti-OMP IgA in vaginal secretions from the mice as an indicator of mucosal response. After boosting, we could observe a sharp increase in the levels of mucosal IgA, with mice injected with OMVs from the ΔompCΔompD mutant having higher levels than the mice immunized with the other OMVs from Δomp mutants, which was consistent with the effect of IgG level (Figure 3B). Measurements of the IgA responses induced via the intraperitoneal route showed that the values of all groups were lower than 0.5 μg/ml, and no significant difference was observed. At 5 weeks after boost immunization, we infected the mice with 1 × 109 CFU (approximately 2,000 × LD50) of wild type S. Typhimurium strain S100 to monitor the protective efficiency and recorded survival rates. Immunization with OMVs from the parental strain, ΔompCΔompD, and ΔompAΔompCΔompD mutants induced effective protection against the challenge. All the mice in the control (PBS) group succumbed within 10 days after infection with wild type S. Typhimurium strain S100 (Table 3).
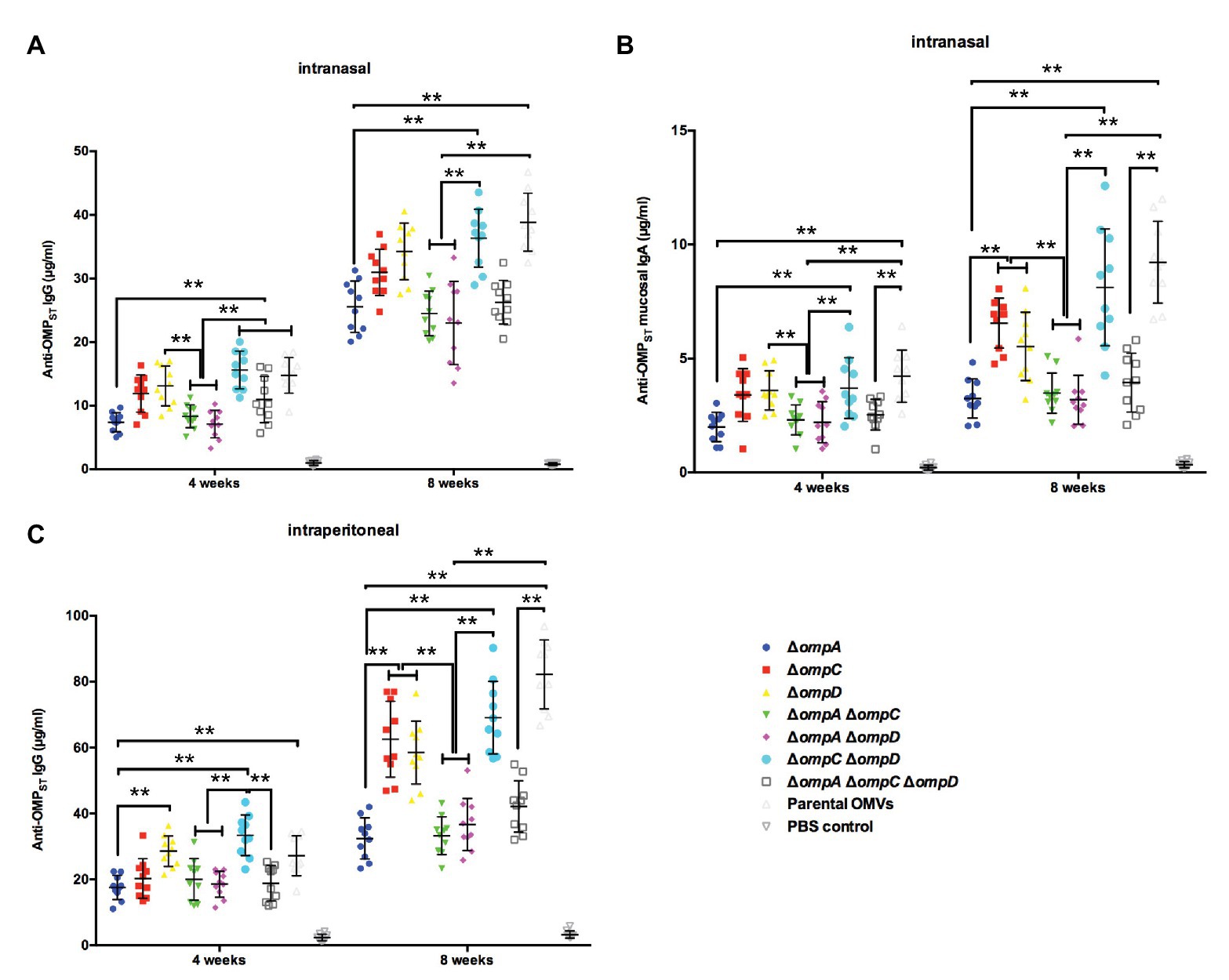
Figure 3. IgG and IgA immune responses in mice immunized with OMVs derived from the parental strain and Δomp mutants. Total amounts of anti-OMPST IgG in the sera of mice immunized with OMVs via the intranasal (A) or intraperitoneal (C) route and total amounts of anti-OMPST IgA in vaginal secretions from mice immunized with OMVs via the intranasal route (B). Samples were collected at 4 and 8 weeks after the first immunization, and PBS-treated mice served as the control group (n = 10 mice per group). The error bars represent variations among all mice in each group. **p < 0.01.
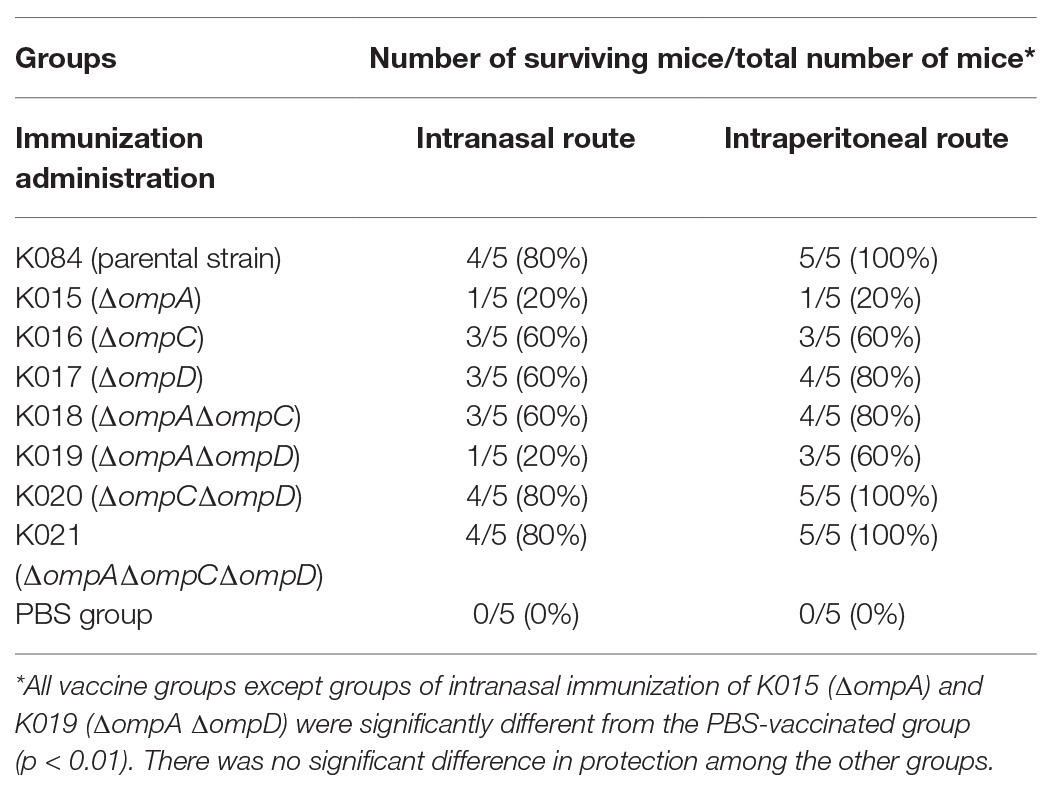
Table 3. Immunization with distinct OMVs with OMP mutations protected mice against oral challenge with S. Typhimurium strain S100.
Cross-Reactive Immune Responses Against Salmonella of Heterologous Serotypes and Other Major Bacterial Pathogens
Our previous studies have reported that truncation of the bacterial outer membrane may continuously expose conserved antigenic proteins to improve the cross-immune effect of OMVs (Liu et al., 2016a,b). Therefore, we evaluated the cross-immune responses of OMVs derived from different OMP mutants. In short, we determined the concentrations of IgG in the mice immunized with OMP-deficient OMVs and in those immunized with OMPs isolated from S. Enteritidis, S. Choleraesuis, APEC O78, and Shigella by a quantitative ELISA to evaluate the effects of cross-immunity. The result for the two routes of immunization showed that the IgG concentrations were roughly the same, as both routes resulted in high IgG levels against OMPs derived from S. Choleraesuis, S. Enteritidis, APEC O78, and Shigella. Regardless of the immunization route, analysis and integration of the data showed that the anti-OMPSC IgG concentrations in the ΔompAΔompCΔompD and ΔompA groups were slightly higher or similar to those in the parental OMV group via intranasal or intraperitoneal immunization, respectively (Figure 4A). Regarding S. Enteritidis, the result of cross-immunity in the ΔompAΔompC group showed that the level was close to that in the group of parental OMVs; only the ΔompAΔompCΔompD group performed better than did the parental OMVs group (Figure 4B). OMVs from the ΔompA, ΔompAΔompC, ΔompAΔompD, and ΔompAΔompCΔompD mutants elicited remarkedly higher levels of serum IgG than did the parental OMVs against OMPs from APEC O78 (Figure 4C). A significant increase in IgG levels against Shigella OMPs was observed in the sera from the mice immunized with OMVs from the ΔompA and ΔompAΔompCΔompD mutants compared with those in the sera from the mice immunized with OMVs from the parental strain (Figure 4D). Both intranasal and intraperitoneal injections resulted in a concentration of IgG that was half that of the homologous group but still showed a good protective efficacy.
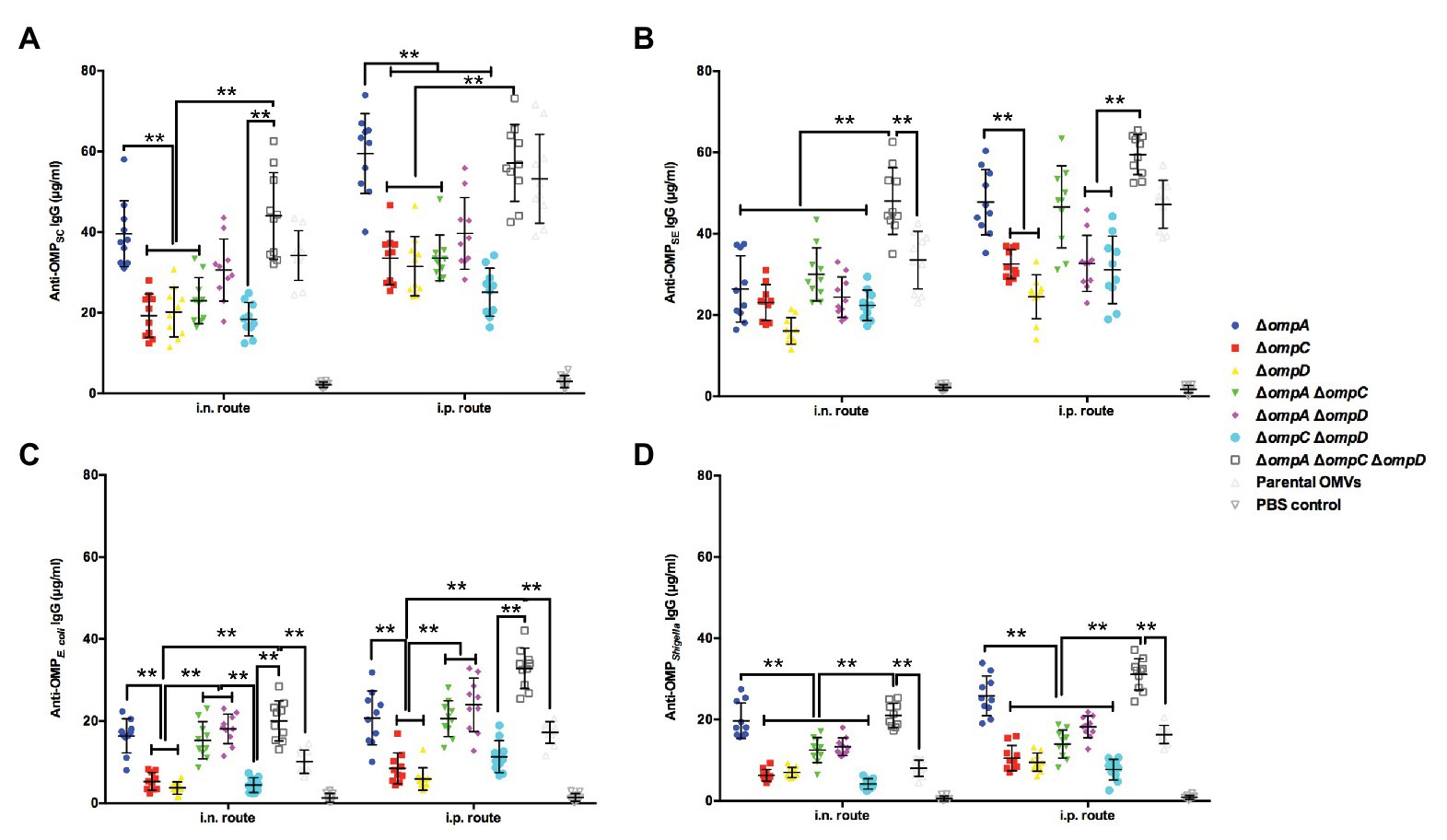
Figure 4. Cross-reactive immune responses induced by OMVs from Δomp mutants against heterologous Salmonella and other intestinal pathogens. Total amounts of anti-OMPSC IgG (A) anti-OMPSE IgG (B) anti-OMPO78 IgG (C) and anti-OMPShigella IgG (D) in the sera of mice (n = 10 per group) immunized with OMVs via the intranasal or intraperitoneal route. The abscissa is the route of immunization, and the ordinate is the concentration of specific IgG. The error bars represent variations among all mice in each group. ** p < 0.01.
Cross-Protection Against Salmonella of Heterologous Serotypes and Other Major Bacterial Pathogen Challenges
To assess cross-protective productivity, mice from different groups were immunized with OMVs from the ΔompA, ΔompAΔompC, ΔompAΔompD, and ΔompAΔompCΔompD mutants via the intraperitoneal and intranasal routes, with the PBS group used as a control. Similar to the experiments on cross-reactivity, mutant strains with a better efficacy were selected to explore their potential as cross-protective vaccines. In the case of Salmonella with different serotypes, the groups immunized with OMVs derived from various OMP mutants all showed good cross-protection, regardless of the route of immunization, compared with that in the PBS group (Figure 5A,B). In particular, the group immunized with ΔompAΔompCΔompD OMVs via the intraperitoneal route showed better or similar protection than did the parental group (Figures 5E,F). Even upon challenge with APEC O78 (Figures 5C,G) and Shigella (Figures 5D,H), ΔompAΔompCΔompD OMVs still provided an established protection efficiency compared with OMVs from the parental strain and other Δomps mutant strain. Irrespective of the wild type strain used as a challenge and the immunization route, OMVs from the ΔompAΔompCΔompD mutant strain remained highly protective, and the survival rate of mice remained above 50%. In the case of the homologous bacterial infection, the survival rate even exceeded 80%.
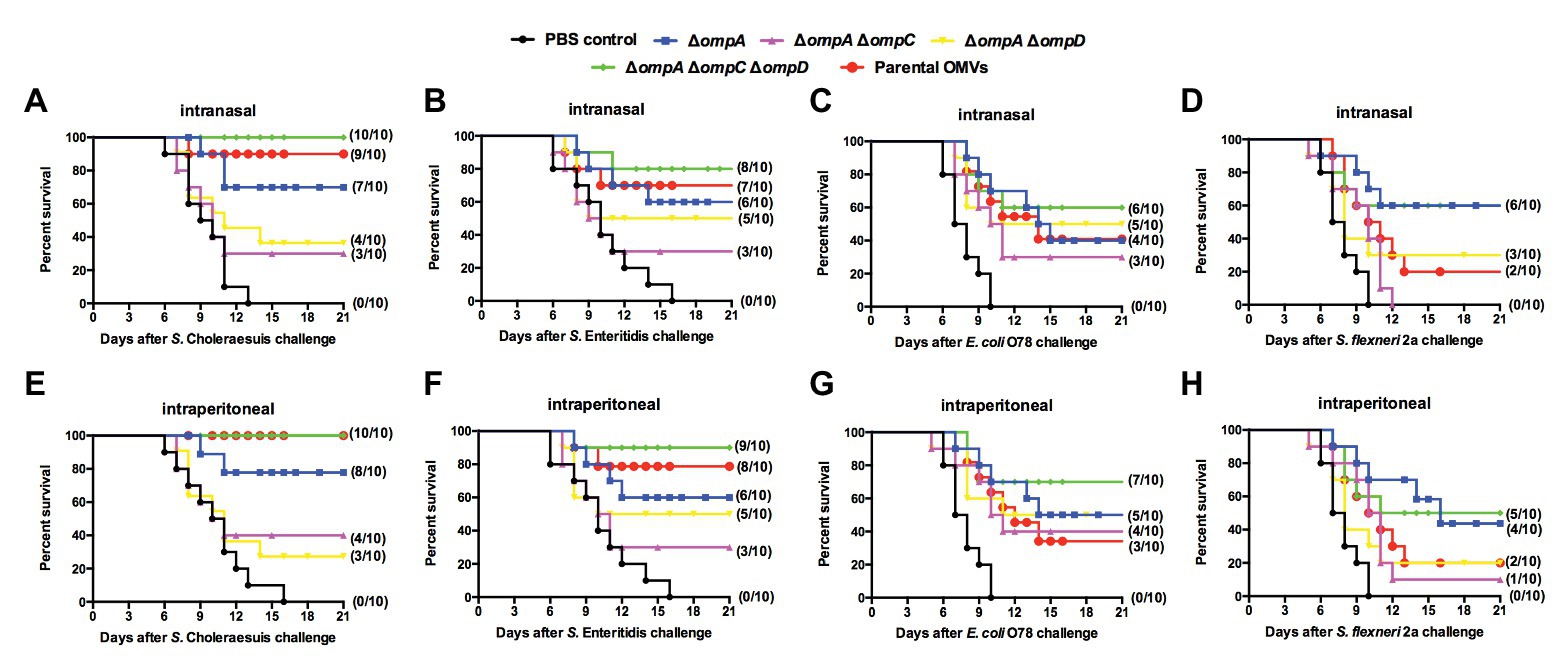
Figure 5. Cross-protection induced by OMVs from Δomp mutants against challenge with heterologous-serotype Salmonella and other intestinal pathogens. Mice were immunized intranasally (A–D) or intraperitoneally (E–H) with OMVs isolated from the indicated Salmonella mutants. The immunized mice were orally challenged with S. Choleraesuis (A,E) S. Enteritidis (B,F) APEC O78 (C, G) and Shigella flexneri 2a (D,H) 9 weeks after the first immunization. After the challenge, mortality was monitored every 3 weeks. The number of surviving mice/total number of mice (n = 10 per group) is shown in parentheses.
Cross-Immunogenicity and Protection Effects of omp-Deficient OMVs Against S. Enteritidis Challenge in the Chicken Model
Based on the results of the previous experiments in the mouse model and the current status of S. Enteritidis as a major food-borne pathogen, we further studied the immunity of mutant vaccine to S. Enteritidis in a chicken model. To verify the cross-immunogenicity of OMVs from the ΔompAΔompCΔompD mutant, which could induce high levels of cross-protection and high survival rates after the challenge in mice, chickens were intranasally immunized with purified OMVs from strain K021 and the parental strain (K084), as well as with OMPs isolated from S. Enteritidis, as potential immune-protective injections. At weeks 1 and 4, the anti-OMPSE IgG was at a high level in the ΔompAΔompCΔompD OMVs group, which was consistent with that of SE OMPs group (Figure 6A). In the period between week 1 and week 4, the bacterial survival rates declined, and at week 4, levels of ΔompAΔompCΔompD OMVs group were significantly decreased compared with the SE OMP group, which indicated that booster immunization with OMVs from the ΔompAΔompCΔompD mutant could significantly enhance SBA and display a long-term bacterial killing or growth suppression effect (Figure 6B). Compared with that of the PBS- and SE OMP-immunized groups, a significantly higher capability for opsonophagocytosis was shown at all time points (weeks 1 and 4) by the parental OMV- and ΔompAΔompCΔompD OMV-immunized groups (Figure 6C). Immunized chickens in the different groups were challenged via the air sac route with 1 × 1010 CFU of SE at week 5 to evaluate the cross-protective efficiency. As shown in Figure 6D, a significant level of reduction in shedding was observed in the ΔompAΔompCΔompD OMVs-immunized group of chickens, and birds were negative by day 17 post-challenge. Moreover, the ΔompAΔompCΔompD OMVs-immunized group could shed higher levels of the challenge strain compared with that the parental OMVs immunized group and SE OMP-immunized group (Figure 6D).
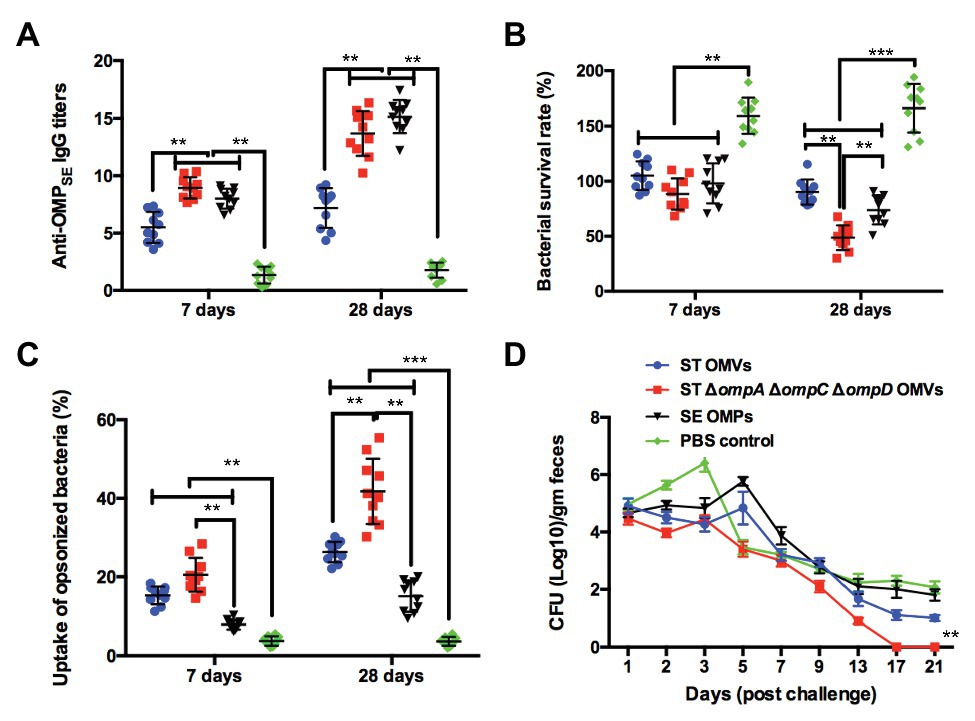
Figure 6. Cross-immunogenic protection with Δomp OMVs against S. Enteritidis challenge in the chicken model. (A) Titers of anti-OMP IgG in the sera of chickens immunized with OMVs, OMPs, or PBS. (B) Bacterial survival after incubation at 37°C for 1 h with serum samples from PBS-, S. Enteritidis OMP-, S. Typhimurium OMV-, and K021 OMV-immunized chickens. (C) The phagocytosis rate of the phagocytic bacteria was obtained by comparing the phagocytosis of macrophage RAW 264.7 on the inoculated macrophages. (D) Chickens were challenged with 1010 CFU of S. Enteritidis at 3 weeks after the boost immunization. Fecal shedding of the challenge strain in animal feces, and each point on the graph represents mean fecal shedding along with the corresponding standard deviation for each group of animal for the day of fecal sampling. The error bars represent variations among all mice in each group. **p < 0.01 and ***p < 0.001.
Cross-Immunogenicity and Protection of omp-Deficient OMVs Against APEC O78 Challenge in the Chicken Model
Further, we also determined the cross-immunogenicity of ΔompAΔompCΔompD OMVs to APEC O78 in chickens and evaluated their cross-protective efficacy. At the first week, the production of anti-OMPO78 IgG in all experimental groups exhibited levels significantly higher than that in the PBS group. At week 4, the titers of anti-OMPO78 IgG in the ΔompAΔompCΔompD OMVs group showed a similar trend as in the O78 OMP group, and were significantly higher than those in the parental group (Figure 7A). In addition, SBA assay showed that the bacterial survival rate of all experimental groups was significantly lower than that of PBS group at the first week; all experimental groups maintained this trend at the 4th week, although the ΔompAΔompCΔompD OMVs group showed significantly lower bacterial survival rate than O78 OMP group (Figure 7B). Further, the bacterial uptake rate in the ΔompAΔompCΔompD OMVs-immunized group was significantly higher than that in the O78 OMP group (Figure 7C). The post-challenge survival rate in the ΔompAΔompCΔompD OMVs group approached 60%, higher than other groups, even in the O78 OMP group (Figure 7D). Moreover, immunization with ΔompAΔompCΔompD OMVs provided significant reductions in clinical score compared to the parental and PBS groups. Similarly, liver lesion score was lesser in the ΔompAΔompCΔompD OMVs immunized chickens than in the control and PBS groups (Figure 7E). Although there was no significant difference in the heart lesion score among the experimental groups, slightly lower score was observed in the ΔompAΔompCΔompD OMV-immunized group compared with parental and O78 OMP groups. The relatively decreased clinical scores and liver lesion scores demonstrated that immunization of ΔompAΔompCΔompD OMVs could lower the severity of perihepatitis induced by APEC O78 in the birds (Nagano et al., 2012). Taken together, these data clearly demonstrated the feasibility of using ΔompAΔompCΔompD OMVs as a broad-spectrum vaccine.
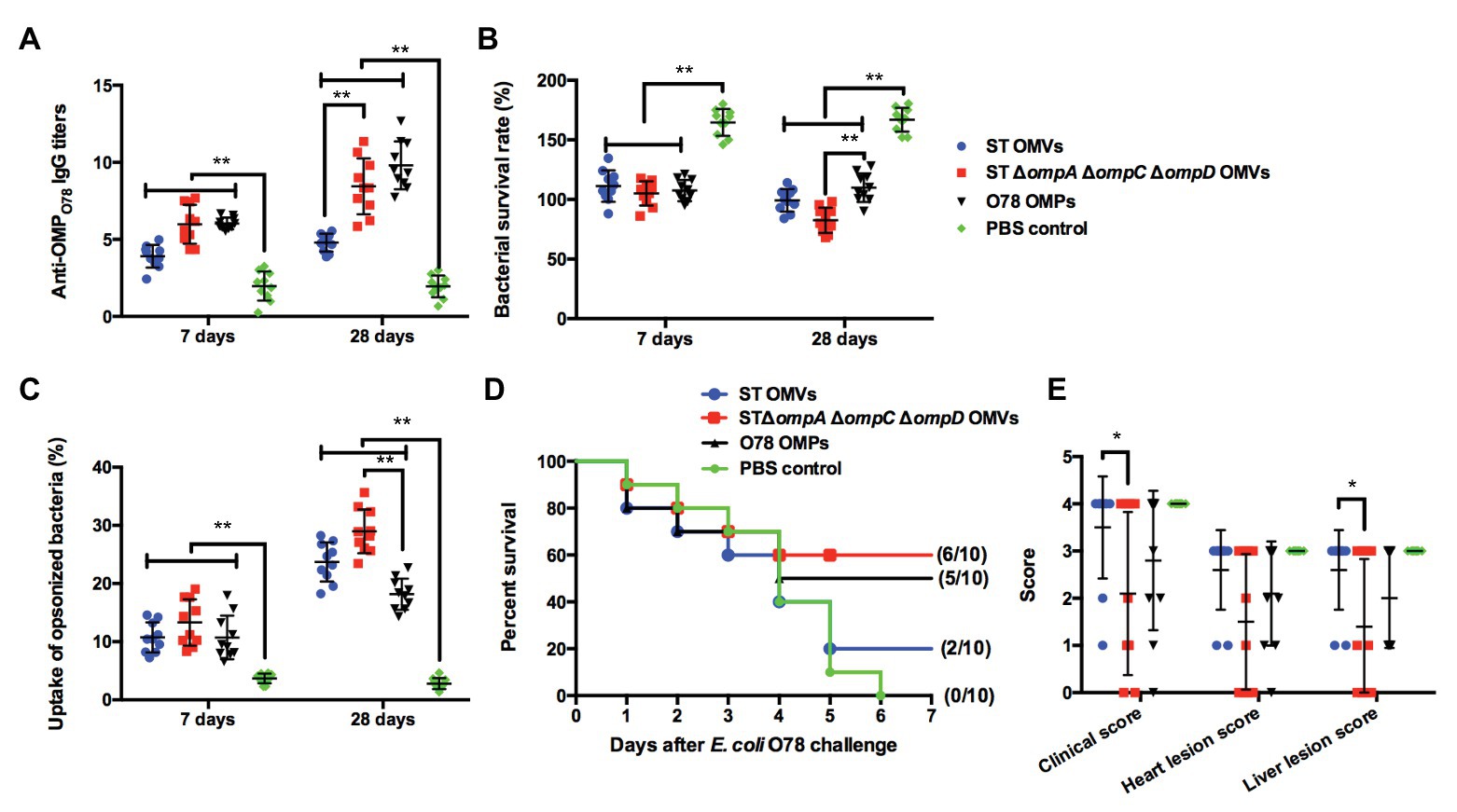
Figure 7. Cross-immunogenic protection with Δomp OMVs against APEC O78 challenge in the chicken model. (A) Titers of anti-OMPSE IgG in the sera of chickens intramuscularly immunized with purified OMVs from strain K021, the parental strain (K084), as well as with O87 OMPs and PBS. (B) Bacterial survival after incubation at 37°C for 1 h with serum samples from PBS-, O87 OMP-, S. Typhimurium OMV-, and K021 OMV-immunized chickens. (C) The phagocytosis rate of the phagocytic bacteria was obtained by comparing the phagocytosis of macrophage RAW264.7 on the inoculated macrophages. (D) Chickens were challenged with 108 CFU of APEC O78 at 3 weeks after the boost immunization, and mortality was monitored for 7 days after the challenge. (E) Clinical features and tissues were scored for signs of colibacillosis. The error bars represent variations among all mice in each group. *p < 0.05 and **p < 0.01.
Discussion
Outer membrane vesicle-based vaccines show several potential advantages over live attenuated vaccines, including a high immunogenicity without replication, a higher safety, and better intrinsic adjuvant effects, thus being a novel choice to be used against bacterial infections (Roy et al., 2010, 2011; McConnell et al., 2011; Mitra et al., 2012). For example, OMVs from Neisseria meningitidis, which contains three highly immunogenic proteins, have been used as a licensed vaccine in children older than 2 months in Europe (Villena et al., 2018). However, until now, there is no licensed OMV vaccine for the protection against avian diseases. OMVs are composed of OMPs, LPS, lipoproteins, and some other components (Kuehn and Kesty, 2005). Our previous studies have demonstrated that OMVs derived from a ΔfliCΔfljB mutant could induce the production of cross-reactive antibodies but did not affect the production of OMVs (Liu et al., 2016a), whereas deletion of the rfbP gene, which induces a lack of full length O-antigen in LPS, could result in remodeling of the outer membrane structure and, thus, enhance the production of OMVs (Liu et al., 2016b). Based on these previous findings, we continued studying the effects of knockout of several major OMPs (OmpA, OmpC, and OmpD) on the cross-protection with OMVs and testing our hypothesis that remodeling of the outer membrane might affect the cross-protection with OMVs. Our results showed that ΔompA could increase the yield of OMVs, and ΔompAΔompCΔompD OMVs provided good cross-protection against heterologous Salmonella and APEC in chickens, which was in line with our hypothesis.
The formation of OMVs is an important means of resistance of bacteria to diverse external environments, and various triggers, including iron limitation, antibiotics, signaling molecules, DNA-damaging agents, and hydrophobic compounds, can induce membrane vesicle formation through membrane blebbing or explosive cell lysis (McBroom et al., 2006; Toyofuku et al., 2019). The production of vesicles varies depending on the growth period and the availability of nutrients, and vesicle-related enzymes may contribute to the elimination of nutrients. The yield of OMVs is a critical factor that limits their application for clinical treatment. The knockout of OMPs may affect the biosynthesis of the outer membrane and thus the yield of OMVs (van de Waterbeemd et al., 2010; Nevermann et al., 2019). Our results also support this hypothesis, as ΔompA, ΔompAΔompD, and ΔompAΔompCΔompD mutations could increase the OMV production (Figure 2B). On the contrary, the ΔompCΔompD mutant produced fewer OMVs than did the parental strain. We speculated that the knockout of the ompA gene might affect the formation of the outer membrane, increasing the outer membrane vesiculation and content.
The development of an ideal vaccine to control bacterial infections should take systemic, mucosal, and cellular immunity into consideration (Pasetti et al., 2003; Capozzo et al., 2004). Therefore, both intranasal and intraperitoneal routes of administration to mice were chosen to evaluate the mucosal and systemic immunogenicity of OMVs isolated from OMP-deficient S. Typhimurium. Researchers have paid much attention to the intranasal route for vaccination (Partidos, 2000), which has previously been shown to be a good choice to elicit active systemic and mucosal immunity (Kiyono and Fukuyama, 2004; Holmgren and Czerkinsky, 2005). The intraperitoneal route, which can induce a strong humoral immunity, is also a standard route of immunization of mice with purified OMVs (Alaniz et al., 2007; Schild et al., 2009; Baker et al., 2017). In our previous study, both intranasal and intraperitoneal routes resulted in good immunogenic effects. Moreover, intranasal immunization may be a safer route for vaccination with OMVs (Liu et al., 2016a). Consequently, these two routes of immunization were used to assess the immunogenicity and protection ability of OMVs in this study. As shown in Figures 3, 5, although the ability of intranasal route to induce IgG production was not as strong as that of intraperitoneal route, intranasal route can effectively induce mucosal immune response, and intranasal OMV immunization could also elicit cross-protection similar to that of intraperitoneal immunization. This also confirmed that the intranasal route may be the preferred immunization strategy for OMVs as vaccines.
To assess the cross-protection provided by OMVs derived from OMP-deficient S. Typhimurium, it is necessary to ensure that the amount of various OMVs we immunized was consistent. With the deletion of the OMVs from the main OMPs, a question we considered was whether the cross-protection of ΔompAΔompCΔompD OMVs achieved is a function of the estimated OMVs amount as indicated by protein concentration measurement. Therefore, we analyzed the particle size and distribution of each fractions of various OMP-deficient OMVs obtained by density gradient centrifugation using NTA, and found that the size and appearance of OMVs secreted by different OMP mutations were similar (Supplementary Figures 3, 4), which preliminarily indicated that the amount of OMVs can be accurately estimated by protein concentration measurement. And that, based on the cross-protection data of the chicken model, OMVs derived from OMP mutations stimulated mice to produce the same or even higher immune protection compared with the OMPs isolated from heterologous pathogen against this heterologous pathogen infection (Figures 6, 7). This also indicated that the cross-protection is not caused by the amount of immunized OMVs, but more likely by an increase in the expressed cross-immunogenic antigens. Taken together, these results confirmed that the number of OMVs from various OMP mutations that we immunized into mice is consistent, and the results of cross-protection induced by ΔompAΔompCΔompD OMVs are also reliable.
In this study, S. Choleraesuis, S. Enteritidis, APEC O78, and S. flexneri 2a, which had been isolated from infected animals, were chosen to assess the immune efficiency of purified OMVs in mice. Owing to the deficiency of bacterial protective OMPs, the IgG levels against these pathogenic bacteria were higher in all groups than in the negative control group, especially in the ΔompAΔompCΔompD OMVs group, wherein the levels were also obviously higher than those in the parental group (Figures 4A–D). Furthermore, in the mouse model, ΔompAΔompCΔompD OMVs also provided appreciable protection against heterologous serotypes of Salmonella, including S. Choleraesuis and S. Enteritidis, APEC O78, and even human pathogenic S. flexneri 2a (Figure 5), which can cause significant diarrheal disease and mortality in humans (Groisman and Ochman, 1993). Recently, studies have reported that the O-antigen of Shigella was synthesized in Salmonella to construct a cross-protective vaccine to multiple disease-predominant S. flexneri serotypes, which suggested that Shigella and Salmonella had similar O-antigen synthesis pathways (Dharmasena et al., 2017; Su et al., 2019). Meanwhile, this fact might partly explain the cross-protection by these OMP-deficient OMVs against Shigella infection and also suggested that we could recombine the O-antigen of Shigella into OMVs to construct a cross-protective vaccine against predominant causative agents of shigellosis.
Avian pathogenic Escherichia coli O78 infections result in a great harm to young chickens, causing avian colibacillosis and even deaths, reducing the profit of the breeding industry. Although S. Enteritidis infection does not cause death in poultry, S. Enteritidis is a major cause of food-borne diseases, and poultry-derived products, including meat and chicken eggs, are considered a major source of this pathogen in human infection (Bäumler et al., 2000). Vaccine could be useful for preventing S. Enteritidis circulation in chickens and diminishes its transmission to humans. Therefore, we also evaluated the cross-protection of the OMP-deficient OMVs against S. Enteritidis and APEC O78 in a chicken model. The results showed that ΔompAΔompCΔompD OMVs provided an immune effect, which was significantly higher than the immune effects of OMPs from S. Enteritidis and APEC O78. Thus, the data indicated that the ΔompAΔompCΔompD OMVs could improve the cross-protection effectiveness against APEC O78 and feces shedding against S. Enteritidis (Figures 6, 7). Thus, OMVs derived from the ΔompAΔompCΔompD mutations might also be effective and promising adjuvants in a vaccine design for chickens to prevent infections.
Currently, it is not clear whether the enhanced cross-protection induced by OMVs can be attributed to the conserved OMPs or to other conserved antigens that are shared among these pathogens, such as the LPS core oligosaccharide moiety that is possessed by various Gram-negative bacteria (Yang et al., 2013). Previous study reported that conserved LPS oligosaccharides in OMVs also contribute to inducing protective immunity (Lorenzo et al., 2015). However, these sugar epitopes may provide limited broad-spectrum protection against smooth Gram-negative bacterial infections (Francis and Shenep, 1985; Siber and Warren, 1985). Therefore, we can preliminarily speculate that the deletion of major OMPs might have increased the expression levels of conserved OMPs or other conserved antigens, which are typically found in the four enteropathogenic bacteria in this study, leading to the enhancement of cross-protection. Nevertheless, the existing data could not clarify which component causes the variation in immunogenicity. In the future, we will advance our research via direct identification, using proteomic analysis, of the conserved antigens that lead to the increase of cross-protection by OMVs. Moreover, OMVs can encapsulate antigenic components of other pathogens and serve as a delivery platform to induce desired pathogen-specific immune responses, thus expanding the application of OMVs as vaccines (Gnopo et al., 2017). Therefore, our future efforts would focus on OMVs as a multiple antigen platform to encapsulate conserved antigens or other O-antigens for the development of a broad-spectrum protective vaccine against avian pathogenic bacteria.
In conclusion, we discovered that the deletion of the ompA gene could enhance the production of OMVs and that OMVs derived from the ΔompAΔompCΔompD mutations might be an effective and promising vaccine candidate for chickens to prevent S. Enteritidis and APEC O78 infections. OMP-deficient OMVs may not only be nominated as an effective vaccine candidate but may also present a novel vaccine adjuvant, which can be formulated with other vaccine components to elicit multi-serotype cross-protection responses and control infection with heterologous bacterial serotypes.
Data Availability Statement
The raw data supporting the conclusions of this article will be made available by the authors, without undue reservation.
Ethics Statement
The animal study was reviewed and approved by Animal Use Ethical Committee of Nanchang University.
Author Contributions
QL and XH conceived and designed the experiments. YC, KJ, BL, HY, HR, and JW performed the experiments. YC and KJ analyzed the data. YC, KJ, XH, and QL wrote the manuscript. All authors contributed to the article and approved the submitted version.
Funding
This study was supported by the National Natural Science Foundation of China (31760261, 31660035, and 32060040), the Science and Technology Research Project of Jiangxi Provincial Education Department (60224), and Key research and development projects of Jiangxi Natural Science Foundation (20192BBG70067).
Conflict of Interest
The authors declare that the research was conducted in the absence of any commercial or financial relationships that could be construed as a potential conflict of interest.
Acknowledgments
We thank Accdon (www.accdon.com) for its linguistic assistance during the preparation of this manuscript.
Supplementary Material
The Supplementary Material for this article can be found online at: https://www.frontiersin.org/articles/10.3389/fmicb.2020.588952/full#supplementary-material
Supplementary Figure 1 | Identification of outer membrane protein (OMP) mutations by PCR. Strains include K015 (∆ompA), K016 (∆ompC), K017 (∆ompD), K018 (∆ompA∆ompC), K019 (∆ompA∆ompD), K020 (∆ompC∆ompD), and K021 (∆ompA∆ompC∆ompD).
Supplementary Figure 2 | Cryo-EM imaging of outer membrane vesicles (OMVs). OMVs derived from the ∆ompA∆ompC∆ompD mutant strains of Salmonella Typhimurium were visualized using cyro-EM. The red arrows indicate the visible OMVs.
Supplementary Figure 3 | Distribution of OMV sizes from the ∆omp mutants and parental strain, binned in 10-nm increments. n = 3, mean ± SD.
Supplementary Figure 4 | Graph representing particle numbers and mean particle size (Théry et al., 2018) for the OMVs derived from the ∆omp mutants and parental strain in various OptiPrep density gradient medium fractions, after NTA measurement. n = 3, mean ± SD.
Supplementary Figure 5 | Numbers of particles per milliliter were normalized to numbers of CFU per milliliter calculated from each mutant and parental strain cultured in acidic MgM growth condition.
Abbreviations
APEC, Avian pathogenic Escherichia coli; BSG, Buffered saline with 0.01% gelatin; CFU, Colony-forming units; DPBS, Dulbecco’s phosphate-buffered saline; LB, Luria-Bertani; LPS, Lipopolysaccharide; OMP, Outer membrane protein; OMV, Outer membrane vesicle; PBS, Phosphate-buffered saline; PBST, Phosphate-buffered saline containing 0.1% Tween 20; SBA, Serum bactericidal activity; SDS-PAGE, Sodium dodecyl sulfate polyacrylamide gel electrophoresis.
References
Alaniz, R. C., Deatherage, B. L., Lara, J. C., and Cookson, B. T. (2007). Membrane vesicles are immunogenic facsimiles of Salmonella Typhimurium that potently activate dendritic cells, prime B and T cell responses, and stimulate protective immunity in vivo. J. Immunol. 179, 7692–7701. doi: 10.4049/jimmunol.179.11.7692
Bai, J., Kim, S. I., Ryu, S., and Yoon, H. (2014). Identification and characterization of outer membrane vesicle-associated proteins in Salmonella enterica serovar Typhimurium. Infect. Immun. 82, 4001–4010. doi: 10.1128/iai.01416-13
Baker, S. M., Davitt, C. J. H., Motyka, N., Kikendall, N. L., Russell-Lodrigue, K., Roy, C. J., et al. (2017). A Burkholderia pseudomallei outer membrane vesicle vaccine provides cross protection against inhalational glanders in mice and non-human primates. Vaccine 5:49. doi: 10.3390/vaccines5040049
Barrow, P. A. (2007). Salmonella infections: immune and non-immune protection with vaccines. Avian Pathol. 36, 1–13. doi: 10.1080/03079450601113167
Bäumler, A. J., Hargis, B. M., and Tsolis, R. M. (2000). Tracing the origins of Salmonella outbreaks. Science 287, 50–52. doi: 10.1126/science.287.5450.50
Campbell, J. R., and Edwards, M. S. (2000). Cytokines enhance opsonophagocytosis of type III group B Streptococcus. J. Perinatol. 20, 225–230. doi: 10.1038/sj.jp.7200360
Capozzo, A. V., Cuberos, L., Levine, M. M., and Pasetti, M. F. (2004). Mucosally delivered Salmonella live vector vaccines elicit potent immune responses against a foreign antigen in neonatal mice born to naive and immune mothers. Infect. Immun. 72, 4637–4646. doi: 10.1128/IAI.72.8.4637-4646.2004
Carlone, G. M., Thomas, M. L., Rumschlag, H. S., and Sottnek, F. O. (1986). Rapid microprocedure for isolating detergent-insoluble outer-membrane proteins from Haemophilus species. J. Clin. Microbiol. 24, 330–332. doi: 10.1128/jcm.24.3.330-332.1986
Cavicchio, L., Dotto, G., Giacomelli, M., Giovanardi, D., Grilli, G., Franciosini, M. P., et al. (2015). Class 1 and class 2 integrons in avian pathogenic Escherichia coli from poultry in Italy. Poult. Sci. 94, 1202–1208. doi: 10.3382/ps/pev095
Charlson, E. S., Werner, J. N., and Misra, R. (2006). Differential effects of yfgL mutation on Escherichia coli outer membrane proteins and lipopolysaccharide. J. Bacteriol. 188, 7186–7194. doi: 10.1128/jb.00571-06
Dharmasena, M. N., Osorio, M., Takeda, K., Stibitz, S., and Kopecko, D. J. (2017). Stable chromosomal expression of Shigella flexneri 2a and 3a O-antigens in the live Salmonella oral vaccine vector Ty21a. Clin. Vaccine Immunol. 24:e00181–17. doi: 10.1128/cvi.00181-17
Ebrahimi-Nik, H., Bassami, M. R., Mohri, M., Rad, M., and Khan, M. I. (2018). Bacterial ghost of avian pathogenic E. coli (APEC) serotype O78:K80 as a homologous vaccine against avian colibacillosis. PLoS One 13:e0194888. doi: 10.1371/journal.pone.0194888
Ellis, T. N., and Kuehn, M. J. (2010). Virulence and immunomodulatory roles of bacterial outer membrane vesicles. Microbiol. Mol. Biol. Rev. 74:81. doi: 10.1128/mmbr.00031-09
Feasey, N. A. (2012). Invasive non-typhoidal Salmonella disease: an emerging and neglected tropical disease in Africa. Lancet 379, 2489–2499. doi: 10.1016/S0140-6736(11)61752-2
Francis, G., and Shenep, J. L. (1985). Failure of monoclonal antibodies to core glycolipid to bind intact smooth strains of Escherichia coli. J. Infect. Dis. 151, 1005–1011. doi: 10.1093/infdis/151.6.1005
Gast, R. K. (2007). Serotype-specific and serotype-independent strategies for preharvest control of food-borne Salmonella in poultry. Avian Dis. 51, 817–828. doi: 10.1637/8090-081807.1
Gnopo, Y. M. D., Watkins, H. C., Stevenson, T. C., DeLisa, M. P., and Putnam, D. (2017). Designer outer membrane vesicles as immunomodulatory systems—reprogramming bacteria for vaccine delivery. Adv. Drug Deliv. Rev. 114, 132–142. doi: 10.1016/j.addr.2017.05.003
Grgić, H., Hunter, D. B., Hunton, P., and Nagy, E. (2008). Pathogenicity of infectious bronchitis virus isolates from Ontario chickens. Can. J. Vet. Res. 72, 403–410.
Groisman, E. A., and Ochman, H. (1993). Cognate gene clusters govern invasion of host epithelial-cells by Salmonella-Typhimurium and Shigella-flexneri. EMBO J. 12, 3779–3787. doi: 10.1002/j.1460-2075.1993.tb06056.x
Grubmeyer, C., Hansen, M. R., Fedorov, A. A., and Almo, S. C. (2012). Structure of Salmonella Typhimurium OMP synthase in a complete substrate complex. Biochemistry 51, 4397–4405. doi: 10.1021/bi300083p
Hansen, B. C., Gografe, S., Pritt, S., Jen, K. C., McWhirter, C. A., Barman, S. M., et al. (2017). Ensuring due process in the IACUC and animal welfare setting: considerations in developing noncompliance policies and procedures for institutional animal care and use committees and institutional officials. FASEB J. 31, 4216–4225. doi: 10.1096/fj.201601250R
Holmgren, J., and Czerkinsky, C. (2005). Mucosal immunity and vaccines. Nat. Med. 11(Suppl. 4), S45–S53. doi: 10.1038/nm1213
Kiyono, H., and Fukuyama, S. (2004). NALT- versus Peyer’s-patch-mediated mucosal immunity. Nat. Rev. Immunol. 4, 699–710. doi: 10.1038/nri1439
Kong, Q., Six, D. A., Roland, K. L., Liu, Q., Gu, L., Reynolds, C. M., et al. (2011). Salmonella synthesizing 1-monophosphorylated lipopolysaccharide exhibits low endotoxic activity while retaining its immunogenicity. J. Immunol. 187, 412–423. doi: 10.4049/jimmunol.1190051
Kuehn, M. J., and Kesty, N. C. (2005). Bacterial outer membrane vesicles and the host-pathogen interaction. Genes Dev. 19, 2645–2655. doi: 10.1101/gad.1299905
Lin, J., Huang, S., and Zhang, Q. (2002). Outer membrane proteins: key players for bacterial adaptation in host niches. Microbes Infect. 4, 325–331. doi: 10.1016/s1286-4579(02)01545-9
Liu, Q., Liu, Q., Yi, J., Liang, K., Hu, B., Zhang, X., et al. (2016a). Outer membrane vesicles from flagellin-deficient Salmonella enterica serovar Typhimurium induce cross-reactive immunity and provide cross-protection against heterologous Salmonella challenge. Sci. Rep. 6:34776. doi: 10.1038/srep34776
Liu, Q., Liu, Q., Yi, J., Liang, K., Liu, T., Roland, K. L., et al. (2016b). Outer membrane vesicles derived from Salmonella Typhimurium mutants with truncated LPS induce cross-protective immune responses against infection of Salmonella enterica serovars in the mouse model. Int. J. Med. Microbiol. 306, 697–706. doi: 10.1016/j.ijmm.2016.08.004
Lorenzo, F. D., Silipo, A., Lanzetta, R., Parrilli, M., and Molinaro, A. (2015). “Bacterial lipopolysaccharides: an overview of their structure, biosynthesis and immunological activity” in carbohydrate chemistry: State of the Art and challenges for drug development. 57–89.
McBroom, A. J., Johnson, A. P., Vemulapalli, S., and Kuehn, M. J. (2006). Outer membrane vesicle production by Escherichia coli is independent of membrane instability. J. Bacteriol. 188, 5385–5392. doi: 10.1128/JB.00498-06
McConnell, M. J., Rumbo, C., Bou, G., and Pachon, J. (2011). Outer membrane vesicles as an acellular vaccine against Acinetobacter baumannii. Vaccine 29, 5705–5710. doi: 10.1016/j.vaccine.2011.06.001
Meuskens, I., Michalik, M., Chauhan, N., Linke, D., and Leo, J. C. (2017). A new strain collection for improved expression of outer membrane proteins. Front. Cell. Infect. Microbiol. 7:464. doi: 10.3389/fcimb.2017.00464
Mitra, S., Barman, S., Nag, D., Sinha, R., Saha, D. R., and Koley, H. (2012). Outer membrane vesicles of Shigella boydii type 4 induce passive immunity in neonatal mice. FEMS Immunol. Med. Microbiol. 66, 240–250. doi: 10.1111/j.1574-695X.2012.01004.x
Muralinath, M., Kuehn, M. J., Roland, K. L., and Curtiss, R. 3rd. (2011). Immunization with Salmonella enterica serovar Typhimurium-derived outer membrane vesicles delivering the pneumococcal protein PspA confers protection against challenge with Streptococcus pneumoniae. Infect. Immun. 79, 887–894. doi: 10.1128/iai.00950-10
Nagano, T., Kitahara, R., and Nagai, S. (2012). An attenuated mutant of avian pathogenic Escherichia coli serovar O78: a possible live vaccine strain for prevention of avian colibacillosis. Microbiol. Immunol. 56, 605–612. doi: 10.1111/j.1348-0421.2012.00482.x
Nakayama, K., Kelly, S. M., and Curtiss, R. (1988). Construction of an ASD+ expression-cloning vector—stable maintenance and high-level expression of cloned genes in a Salmonella vaccine strain. Nat. Biotechnol. 6, 693–697. doi: 10.1038/nbt0688-693
National Research Council (2011). Guide for the care and use of laboratory animals. 8th Edn. Washington, DC: National Academies Press.
Nevermann, J., Silva, A., Otero, C., Oyarzun, D. P., Barrera, B., Gil, F., et al. (2019). Identification of genes involved in biogenesis of outer membrane vesicles (OMVs) in Salmonella enterica Serovar Typhi. Front. Microbiol. 10:104. doi: 10.3389/fmicb.2019.00104
Partidos, C. D. (2000). Intranasal vaccines: forthcoming challenges. Pharm. Sci. Technol. Today 3, 273–281. doi: 10.1016/s1461-5347(00)00281-9
Pasetti, M. F., Barry, E. M., Losonsky, G., Singh, M., Medina-Moreno, S. M., Polo, J. M., et al. (2003). Attenuated Salmonella enterica serovar Typhi and Shigella flexneri 2a strains mucosally deliver DNA vaccines encoding measles virus hemagglutinin, inducing specific immune responses and protection in cotton rats. J. Virol. 77, 5209–5217. doi: 10.1128/jvi.77.9.5209-5217.2003
Roier, S., Leitner, D. R., Iwashkiw, J., Schild-Pruefert, K., Feldman, M. F., Krohne, G., et al. (2012). Intranasal immunization with nontypeable haemophilus influenzae outer membrane vesicles induces cross-protective immunity in mice. PLoS One 7:e42664. doi: 10.1371/journal.pone.0042664
Roy, N., Barman, S., Ghosh, A., Pal, A., Chakraborty, K., Das, S. S., et al. (2010). Immunogenicity and protective efficacy of Vibrio cholerae outer membrane vesicles in rabbit model. FEMS Immunol. Med. Microbiol. 60, 18–27. doi: 10.1111/j.1574-695X.2010.00692.x
Roy, K., Hamilton, D. J., Munson, G. P., and Fleckenstein, J. M. (2011). Outer membrane vesicles induce immune responses to virulence proteins and protect against colonization by enterotoxigenic Escherichia coli. Clin. Vaccine Immunol. 18, 1803–1808. doi: 10.1128/CVI.05217-11
Schild, S., Nelson, E. J., Bishop, A. L., and Camilli, A. (2009). Characterization of Vibrio cholerae outer membrane vesicles as a candidate vaccine for cholera. Infect. Immun. 77, 472–484. doi: 10.1128/IAI.01139-08
Siber, G. R., and Warren, S. A. K. S. (1985). Cross-reactivity of rabbit antibodies to lipopolysaccharides of Escherichia coli J5 and other Gram-negative bacteria. J. Infect. Dis. 152, 954–964. doi: 10.1093/infdis/152.5.954
Singer, R. S. (2015). Urinary tract infections attributed to diverse ExPEC strains in food animals: evidence and data gaps. Front. Microbiol. 6:28. doi: 10.3389/fmicb.2015.00028
Su, H., Liu, Q., Wang, S., Curtiss, R. 3rd, and Kong, Q. (2019). Regulated delayed Shigella flexneri 2a O-antigen synthesis in live recombinant Salmonella enterica Serovar Typhimurium induces comparable levels of protective immune responses with constitutive antigen synthesis system. Theranostics 9, 3565–3579. doi: 10.7150/thno.33046
Subedi, M., Luitel, H., Devkota, B., Bhattarai, R. K., Phuyal, S., Panthi, P., et al. (2018). Antibiotic resistance pattern and virulence genes content in avian pathogenic Escherichia coli (APEC) from broiler chickens in Chitwan, Nepal. BMC Vet. Res. 14:113. doi: 10.1186/s12917-018-1442-z
Théry, C., Witwer, K. W., Aikawa, E., Alcaraz, M. J., Anderson, J. D., Andriantsitohaina, R., et al. (2018). Minimal information for studies of extracellular vesicles 2018 (MISEV2018): a position statement of the International Society for Extracellular Vesicles and update of the MISEV2014 guidelines. J. Extracell. Vesicles 7:1535750. doi: 10.1080/20013078.2018.1535750
Toyofuku, M., Nomura, N., and Eberl, L. (2019). Types and origins of bacterial membrane vesicles. Nat. Rev. Microbiol. 17, 13–24. doi: 10.1038/s41579-018-0112-2
van de Waterbeemd, B., Streefland, M., van der Ley, P., Zomer, B., van Dijken, H., Martens, D., et al. (2010). Improved OMV vaccine against Neisseria meningitidis using genetically engineered strains and a detergent-free purification process. Vaccine 28, 4810–4816. doi: 10.1016/j.vaccine.2010.04.082
Villena, R., Safadi, M. A. P., Valenzuela, M. T., Torres, J. P., Finn, A., and O’Ryan, M. (2018). Global epidemiology of serogroup B meningococcal disease and opportunities for prevention with novel recombinant protein vaccines. Hum. Vaccin. Immunother. 14, 1042–1057. doi: 10.1080/21645515.2018.1458175
Wang, H., Liang, K., Kong, Q., and Liu, Q. (2019). Immunization with outer membrane vesicles of avian pathogenic Escherichia coli O78 induces protective immunity in chickens. Vet. Microbiol. 236:108367. doi: 10.1016/j.vetmic.2019.07.019
Weiss, D., Wallace, R. M., Rwego, I. B., Gillespie, T. R., Chapman, C. A., Singer, R. S., et al. (2018). Antibiotic-resistant Escherichia coli and class 1 integrons in humans, domestic animals, and wild primates in rural Uganda. Appl. Environ. Microbiol. 84:e01632–18. doi: 10.1128/AEM.01632-18
Whiley, H., and Ross, K. (2015). Salmonella and eggs: from production to plate. Int. J. Environ. Res. Public Health 12, 2543–2556. doi: 10.3390/ijerph120302543
White, D. W., Elliott, S. R., Odean, E., Bemis, L. T., and Tischler, A. D. (2018). Mycobacterium tuberculosis Pst/SenX3-RegX3 regulates membrane vesicle production independently of ESX-5 activity. mBio 9:e00778–18. doi: 10.1128/mBio.00778-18
Yang, Y., Oishi, S., Martin, C. E., and Seeberger, P. H. (2013). Diversity-oriented synthesis of inner core oligosaccharides of the lipopolysaccharide of pathogenic Gram-negative bacteria. J. Am. Chem. Soc. 135, 6262–6271. doi: 10.1021/ja401164s
Keywords: outer membrane vesicle, broad-spectrum vaccine, Salmonella Enteritidis, Escherichia coli APEC O78, outer membrane protein
Citation: Chen Y, Jie K, Li B, Yu H, Ruan H, Wu J, Huang X and Liu Q (2020) Immunization With Outer Membrane Vesicles Derived From Major Outer Membrane Protein-Deficient Salmonella Typhimurium Mutants for Cross Protection Against Salmonella Enteritidis and Avian Pathogenic Escherichia coli O78 Infection in Chickens. Front. Microbiol. 11:588952. doi: 10.3389/fmicb.2020.588952
Edited by:
George Grant, University of Aberdeen, United KingdomReviewed by:
Jeanette Mary Claire Robertson, Queen’s University Belfast, United KingdomSimon Swift, The University of Auckland, New Zealand
Copyright © 2020 Chen, Jie, Li, Yu, Ruan, Wu, Huang and Liu. This is an open-access article distributed under the terms of the Creative Commons Attribution License (CC BY). The use, distribution or reproduction in other forums is permitted, provided the original author(s) and the copyright owner(s) are credited and that the original publication in this journal is cited, in accordance with accepted academic practice. No use, distribution or reproduction is permitted which does not comply with these terms.
*Correspondence: Xiaotian Huang, xthuang@ncu.edu.cn; Qiong Liu, p19890528@126.com
†These authors have contributed equally to this work