- 1Department of Engineering, Graduate School of Integrated Science and Technology, Shizuoka University, Hamamatsu, Japan
- 2Department of Environment and Energy Systems, Graduate School of Science and Technology, Shizuoka University, Hamamatsu, Japan
- 3Green Energy Research Division, Research Institute of Green Science and Technology, Shizuoka University, Hamamatsu, Japan
- 4Julius Kühn Institute (JKI) – Federal Research Centre for Cultivated Plants, Institute for Epidemiology and Pathogen Diagnostics, Braunschweig, Germany
- 5Department Microbial Ecology and Diversity Research, Leibniz Institute DSMZ-German Collection of Microorganisms and Cell Cultures, Braunschweig, Germany
IncP-1 plasmids, first isolated from clinical specimens (R751, RP4), are recognized as important vectors spreading antibiotic resistance genes. The abundance of IncP-1 plasmids in the environment, previously reported, suggested a correlation with anthropogenic pollution. Unexpectedly, qPCR-based detection of IncP-1 plasmids revealed also an increased relative abundance of IncP-1 plasmids in total community DNA from the rhizosphere of lettuce and tomato plants grown in non-polluted soil along with plant age. Here we report the successful isolation of IncP-1 plasmids by exploiting their ability to mobilize plasmid pSM1890. IncP-1 plasmids were captured from the rhizosphere but not from bulk soil, and a high diversity was revealed by sequencing 14 different plasmids that were assigned to IncP-1β, δ, and ε subgroups. Although backbone genes were highly conserved and mobile elements or remnants as Tn501, IS1071, Tn402, or class 1 integron were carried by 13 of the sequenced IncP-1 plasmids, no antibiotic resistance genes were found. Instead, seven plasmids had a mer operon with Tn501-like transposon and five plasmids contained putative metabolic gene clusters linked to these mobile elements. In-depth sequence comparisons with previously known plasmids indicate that the IncP-1 plasmids captured from the rhizosphere are archetypes of those found in clinical isolates. Our findings that IncP-1 plasmids do not always carry accessory genes in unpolluted rhizospheres are important to understand the ecology and role of the IncP-1 plasmids in the natural environment.
Introduction
Plasmids are self-replicating extrachromosomal DNA molecules and some of them are mobile genetic elements (MGEs) mediating the exchange of genetic information between bacterial cells via conjugation, mobilization, or transformation. Broad host range plasmids belonging to the ncompatibility (Inc) groups P/P-1, W, N, Q/P-4, and U/P-6 as well as the more recently proposed PromA (Van der Auwera et al., 2009) are of great interest, owing to their ability to transfer between, and maintain themselves in, bacteria of different taxa (Dröge et al., 2000). Among these, plasmids affiliated to IncP-1 group were shown to efficiently transfer under various environmental conditions (Heuer and Smalla, 2012; Shintani et al., 2014; Klümper et al., 2015). The first IncP-1 plasmids R751 and RP4 were isolated from clinical specimens (Datta et al., 1971; Jobanputra and Datta, 1974). Later, IncP-1 plasmids were reported from diverse environments, e.g., sewage, marine sediment, manure, biofilters, and rhizosphere that were affected by anthropogenic pollutants (Dahlberg et al., 1997; Schlüter et al., 2007; Binh et al., 2008; Bahl et al., 2009; Gomes et al., 2010; Heuer et al., 2012; Brown et al., 2013; Jechalke et al., 2013; Dealtry et al., 2014; Wolters et al., 2015). A wide variety of adaptive traits conferring resistance to antibiotics, disinfectants, heavy metals, and/or degradation of xenobiotics was found as accessory genes in the insertion hot spots of IncP-1 plasmids (Hsu and Bartha, 1979; Top and Springael, 2003; Smets and Barkay, 2005). IncP-1 plasmids were so far mainly reported from polluted environments (Schlüter et al., 2003; Tauch et al., 2003; Heuer et al., 2004; Tennstedt et al., 2005; Kamachi et al., 2006; Smalla et al., 2006). It was assumed that bacterial populations carrying IncP-1 plasmids encoding adaptive traits either increased in abundance due to the plasmid-conferred selective advantages or newly evolved in the environments under selective pressure.
Using improved sequencing technologies, new subgroups of IncP-1 were discovered and the sequence-based information was used to design primer systems that targeted plasmids of the different subgroups (Bahl et al., 2009; Jechalke et al., 2013). PCR-Southern blot hybridization and real-time quantitative PCR (qPCR) allowed detecting and estimating IncP-1 plasmid abundance in total community DNA (TC-DNA) from various environmental settings (Götz et al., 1996; Heuer et al., 2012; Jechalke et al., 2013, 2014). Exogenous plasmid capturing was one efficient way to isolate these plasmids from environmental bacteria, without cultivation of their original host (Smalla et al., 2015). The plasmid capturing by biparental matings is based on the expression of accessory genes provided by the conjugative plasmid, including resistance(s) to antibiotics or heavy metals. The capturing by triparental matings involves a second donor carrying a small mobilizable plasmid and is based on plasmid mobilizing capacity (Smalla et al., 2015). Notably, IncP-1 plasmids were often obtained by both methods, and in triparental matings, IncQ/P-4 plasmids were used as mobilizable plasmids (Smalla et al., 2015). More than 40 IncP-1 plasmids previously sequenced have been exogenously captured; more than 30 were obtained by biparental mating, while only nine were captured by triparental mating (Supplementary Table S1).
Recently, we reported the increase of the relative abundance of IncP-1 plasmids in the rhizosphere of lettuce grown under field conditions in three non-polluted arable soils compared to bulk soil (Jechalke et al., 2014). DNA directly extracted from bulk soil or lettuce rhizosphere (TC-DNA) was analyzed by real time qPCR targeting the IncP-1 specific korB gene. The strongly increased relative abundance of korB copy numbers in the rhizosphere compared to bulk soil was rather unexpected and revealed significant gaps in our present understanding of the ecology and role of IncP-1 plasmids. In order to confirm the findings made with the rhizosphere bacterial communities of field-grown lettuce and to evaluate if the findings can be expanded to other crop plants, several greenhouse experiments were performed with lettuce (Lactuca sativa L.), tomato (Solanum lycopersicum), and potato (Solanum tuberosum) plants. TC-DNA was isolated from both the rhizosphere and the bulk soil, and the relative abundance of IncP-1 plasmids was determined by the ratio of korB copies and 16S rRNA (rrn) gene copies quantified in TC-DNA by qPCR. As a means to capture IncP-1 plasmids, we employed the triparental mating with a mobilizable IncQ/P-4 pSM1890 plasmid. IncP-1 plasmids were successfully captured in Cupriavidus necator from the rhizosphere bacterial communities of lettuce, tomato, and potato but not from bulk soil. A total of 14 IncP-1 plasmids were selected based on prior phenotypic and genotypic characterization. The insights gained from their long read sequencing are reported here.
Materials and Methods
Greenhouse Experiments, Sampling, and Sample Preparation
Lettuce [Lactuca sativa L.; cultivar (cv) Tizian], tomato (Solanum lycopersicum; cv Moneymaker), and potato (Solanum tuberosum; cv Arkola) were planted as seeds or tubers in diluvial sand [DS, arable soil whose characteristics were previously described (Rühlmann and Ruppel, 2005; Schreiter et al., 2014)], and grown under greenhouse conditions (16 h light, 20°C). Plants were watered with 2 g/L Wuxal® Super (AGLUKON Spezialdünger GmbH & Co., KG, Düsseldorf, Germany), with an NPK ratio of 8-8-6 and micro-nutrients. In addition, pots filled with DS and kept unplanted under the same conditions were used as bulk soil controls (four replicates each). Ten (experiment I: lettuce) or six weeks (experiment II: tomato and potato) after transplanting, plants were destructively sampled (four replicate pots) and vigorously shaken to detach loosely bound soil. Greenhouse experiment III was performed with lettuce and tomato plants only to study the relative abundance of IncP-1 plasmids during different plant growth stages. Therefore, four plants sampled six, eight, and ten weeks after transplanting were analyzed.
Rhizosphere microbial fractions were obtained from the entire root system with the tightly adhering soil placed in Stomacher bags after homogenization with 15 mL of 0.85% NaCl using Stomacher 400 Circulator (Seward Ltd., Worthing, United Kingdom). The supernatants were collected, and the Stomacher step was repeated twice. The microbial pellet was obtained from the combined supernatants (45 mL) by centrifugation (16,000 × g, 10 min). The pellet was resuspended in 2 mL 0.85% NaCl, and 1 mL cell suspension was used for the exogenous plasmid isolation experiments. Five-gram bulk soil samples from unplanted pots were processed accordingly. The microbial pellet was harvested by centrifugation.
Determination of the Relative Abundance of korB in TC-DNA
TC-DNA was extracted and purified from 500 mg of the rhizosphere or bulk soil-derived microbial pellets (four replicates each) as previously described (Jechalke et al., 2013; Blau et al., 2019). Total bacterial 16S rRNA gene (rrn) copy number was determined for the TC-DNA obtained from rhizosphere samples in addition to bulk soil as described in Suzuki et al. (2000). The abundance of korB gene, specific for IncP-1 plasmids, was quantified by TaqManTM-based real-time PCR methods (Jechalke et al., 2013). The relative abundance of IncP-1 plasmids was calculated as ratio of korB gene copy numbers and the rrn copies of the corresponding samples and presented as Log10 values. The CFX 96 Real-time detection system (Bio-Rad Laboratories, Hercules, CA, United States) was used with primer sets, TaqManTM probes, and reference plasmids as previously described (Heuer et al., 2012; Jechalke et al., 2013). The statistical significance was tested by Tukey’s HSD test.
Triparental Exogenous Plasmid Isolation
Triparental matings were performed with rifampicin (Rif) resistant Cupriavidus necator JMP228 as a recipient (which has been successfully used in different experiments as a recipient for capturing IncP-1 plasmids, reviewed in Smalla et al., 2015), Escherichia coli J53 (pSM1890) with the mobilizable IncQ/P-4 plasmid pSM1890 as a second donor (Tietze et al., 1989; Haagensen et al., 2002), and detached cells from rhizosphere and bulk soil samples as donors of mobilizing plasmids as previously described (Smalla et al., 2006). Plasmid pSM1890 encoded GFP and conferred streptomycin (Sm) and gentamicin (Gm) resistances. Overnight cultures of second donor and recipient strains were prepared with lysogeny broth (LB; Roth, Karlsruhe, Germany) supplemented with appropriate antibiotics. The detached cells were activated in 1:10 tryptic soy broth (TSB) medium (Becton Dickinson and Company, Sparks, MD, United States) and gently shaken (150 rpm, 2 h) at room temperature. Recipient, donor, and second donor cells were washed, harvested, and resuspended in 1:10 TSB. A mixture of cells was pipetted onto a Millipore filter (0.22 μm) placed on plate count agar (PCA) (Merck Corp., Kenilworth, NJ, United States) supplemented with cycloheximide (Cyc). After 24 h filter mating at 28°C, cells resuspended from the filters in 1 mL NaCl 0.85% and serial dilutions were plated on PCA with Cyc (200 μg/mL), Rif (50 μg/mL), Km (50 μg/mL), Gm (20 μg/mL), and Sm (50 μg/mL), and incubated for 48 h.
Confirmation of the Transconjugants’ and Plasmids’ Identity and IncP-1 Plasmids Detection and Identification
Genomic DNA was extracted from each overnight culture of potential transconjugants from the lettuce (pTL), tomato (pTT), and potato (pTK) rhizosphere and recipient using Qiagen genomic DNA extraction kit (Qiagen, Hilden, Germany). The presence of oriV region (IncQ/P-4) of pSM1890 was confirmed by PCR with IncQ/P-4 specific primers. BOX-PCR performed as described by Rademaker and De Bruijn (1997) to confirm that the patterns were identical between those of transconjugants and the corresponding recipient. Plasmid DNA extraction from the pSM1890-positive transconjugants was performed as described previously (Smalla et al., 2000). Real-time qPCR was employed for screening the plasmid DNA for korB gene (Heuer et al., 2012; Jechalke et al., 2013). To confirm the presence of IncP-1 plasmids as well as to evaluate their diversity, the korB positive transconjugants were digested with NotI (Thermo Fisher Scientific). The digested plasmid DNA was subjected to 1% agarose gel (w/v) electrophoresis and then Southern-blot analysis was performed with IncP-1 mixed probes (targeting the trfA gene of α, β, ϵ, , and δ subgroups) (Bahl et al., 2009; Wolters et al., 2014). The obtained restriction patterns were compared using GELCOMPAR II version 6.5 (Applied Math, Sint-Martens-Latem, Belgium). The clusters were constructed according to their similarity using Pearson’s correlation coefficients and hierarchical cluster method UPGMA (unweighted pair group method average algorithm means). Based on the restriction pattern comparisons, one representative plasmid was selected for sequencing.
Whole Nucleotide Sequencing of Plasmids and Analyses
Plasmid DNA was extracted from the transconjugants using QIAGEN Plasmid Midi Kit (QIAGEN). SMRTbellTM template library was prepared according to the instructions from Pacific Biosciences, Menlo Park, CA, United States. For preparation of 10 kb libraries 1 to 4 μg of each plasmid DNA was sheared using g-tubesTM (Covaris, Woburn, MA, United States) and DNA was end-repaired and ligated overnight to barcoded SMRTbellTM adapters applying components from the DNA/Polymerase Binding Kit P6 (Pacific Biosciences). Five to eight SMRTbellTM templates were combined equimolar. Samples were either exonuclease-treated for removal of incompletely formed reaction products or subjected to a BluePippinTM Size-Selection (Sage Science, Beverly, MA, United States) for DNA fragments greater than 4 kb. SMRT sequencing was carried out on the PacBio RSII (Pacific Biosciences) taking one 240-min movie for each SMRT cell. Assemblies of the multiplexed plasmid pools have been performed using the HGAP3 Whitelisting protocol within SMRTPipe 2.3.0 applying a genome size of 100 kb and a minimum subread length of 1 kb after demultiplexing using the RS_Subreads.1 protocol contained within SMRT Portal 2.3.0. Whenever possible, plasmids were circularized removing artificial redundancies at the ends of the contigs. Draft annotation was performed using Prokka (Seemann, 2014). The conserved genes in the IncP-1 plasmids were reannotated and named based on those in R751 (Thorsted et al., 1998), except for kfrB (upf54.8 in R751) and kfrC (upf54.4 in R751). Accessory genes including putative metabolic genes and/or transporter genes were subjected to BLAST1 to find similar sequences. Genotypic screening of antibiotic resistance genes in these plasmids was performed by using the comprehensive antibiotic resistance database (CARD) (Alcock et al., 2020). Comparative analyses of the plasmids were performed and visualized by Easyfig ver. 2.2.2 (Sullivan et al., 2011). The nucleotide sequences of genes encoding replication initiation protein (trfA) and relaxase (traI) of 130 selected IncP-1 plasmids (Supplementary Table S3) were aligned using ClustalW (Thompson et al., 1994) and the maximum likelihood method was used for the unrooted trees using MEGA 7 (Kumar et al., 2016). Visualization of plasmid maps was performed using SnapGene2. Plasmid sequences were deposited in GenBank under Accession Numbers MH392232 to MH392246.
Results
Influence of Plant Species and Age on the Relative Abundance of IncP-1 Plasmids
The relative abundance of IncP-1 plasmids (ratio of korB to rrn) quantified by qPCR in TC-DNA showed an approximately one to two orders of magnitude increased relative abundance in the rhizosphere of lettuce and tomato plants compared to bulk soil (t-test, n = 4, p < 0.01 or Tukey’s HSD test, n = 4, p < 0.05), while this was not significant in the potato rhizosphere (Tukey’s HSD test, n = 4, p > 0.05, experiments I and II, Supplementary Figure S1). In addition, korB quantification in TC-DNA from the rhizosphere samples of lettuce and tomato plants collected at six, eight, or ten weeks after transplanting (greenhouse experiment III) revealed that for both plant species the relative abundance of IncP-1 plasmids significantly increased with plant age (Tukey’s HSD test, n = 4, p < 0.05, Figure 1).
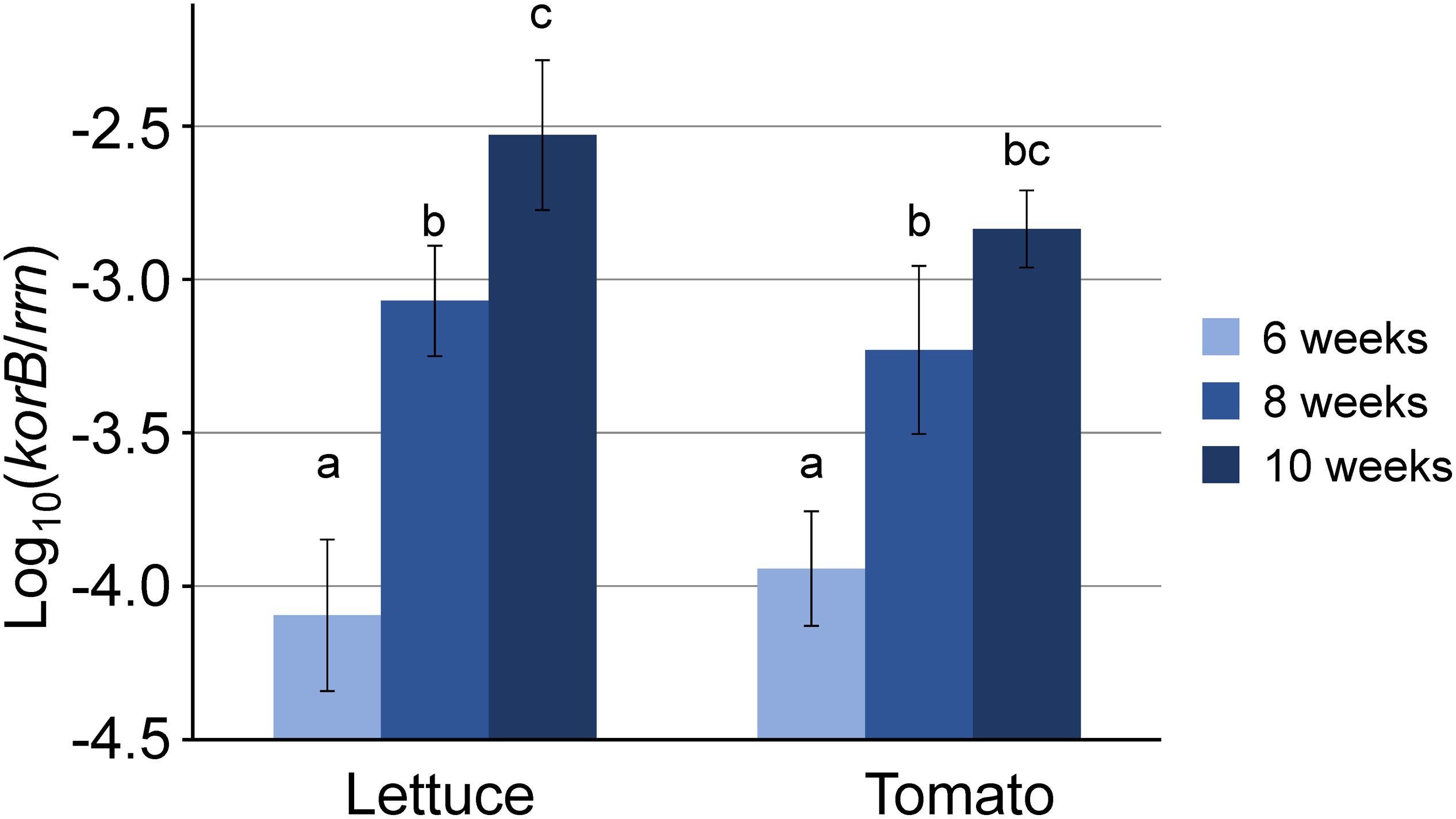
Figure 1. Relative abundance of IncP-1 plasmids log10(korB/rrn) in total community DNA (TC-DNA) from the rhizosphere of lettuce and tomato plants taken at different time points of plant development. a, b, and c indicate the significant differences (Tukey’s HSD test, p < 0.05, n = 4). bc indicates that significant differences were detected between the results of the rhizosphere of lettuce and tomato plants at 6 weeks and that of tomato at 10 weeks, but not between those of lettuce and tomato at 10 weeks.
Exogenous Isolation of IncP-1 Plasmids Based on Their Mobilizing Capacity
From the rhizosphere of lettuce, tomato, and potato, 16, 51, and one IncP-1 plasmids were obtained, respectively, but none was obtained from bulk soil samples. Based on NotI restriction pattern analysis, 14 IncP-1 plasmids were selected, and their complete sequences were obtained. All plasmids captured from the lettuce rhizosphere had a unique restriction pattern, except for pTL50 that was representative of four transconjugants (Supplementary Table S2). In contrast, the restriction patterns of plasmids from the tomato rhizosphere fell into one of a number of groups, i.e., pTT11 was a representative of 21 plasmids, pTT47 of 12, pTT5 of three, and pTT60 of two (Supplementary Table S2). Phenotypic and genotypic screening of transconjugants did not indicate acquired antibiotic resistances.
PacBio Sequence Analysis Revealed That IncP-1 Plasmids From the Rhizosphere Belong to Different Subgroups
The sizes of 14 representative IncP-1 plasmids captured from the rhizosphere of lettuce (pTL8, pTL9, pTL25, pTL16, pTL21, pTL43, pTL50, and pTL52), tomato (pTT5, pTT11, pTT25, pTT47, and pTT60), and potato (pTK9) remarkably varied. Their sizes ranged from 39,671 bp to 107,234 bp for pTL50 and pTL9 plasmids, respectively (Supplementary Table S2). The phylogenetic analysis of the plasmids was performed based on the nucleotide sequence of trfA and traI genes encoding replication initiation protein and relaxase, respectively (Figure 2). The majority of the plasmids from all three rhizospheres (12 out of 14 plasmids) belonged to the IncP-1β subgroup (Figure 2 and Supplementary Table S2). Eleven IncP-1β plasmids were classified as IncP-1β1 subgroup and the pTL8 plasmid belonged to the IncP-1β2 subgroup. Plasmid pTT60 showed >99% nucleotide sequence identity of trfA and traI with the new IncP-1δ group prototype plasmid pAKD4. The only plasmid in this study without any MGEs or accessory genes was the IncP-1ε plasmid pTL50. Eleven plasmids had type II toxin-antitoxin systems (TAs), which were encoded by kluAB genes in their backbone regions (Supplementary Figure S2). Six of them (pTK9, pTT11, pTL16, pTL21, pTL25, and pTL52) had a putative RelEB system encoding riboendonuclease as toxin that cleaves mRNA on translating ribosomes (Christensen et al., 2001). The other five (pTL9, pTL43, pTT5, pTT25, and pTT47) had a putative ParED system encoding inhibitor of DNA gyrase as toxin (Jiang et al., 2002) (Supplementary Figure S2). No previously known antibiotic resistance genes were identified in any captured plasmids by searching the comprehensive antibiotic resistance database (CARD).
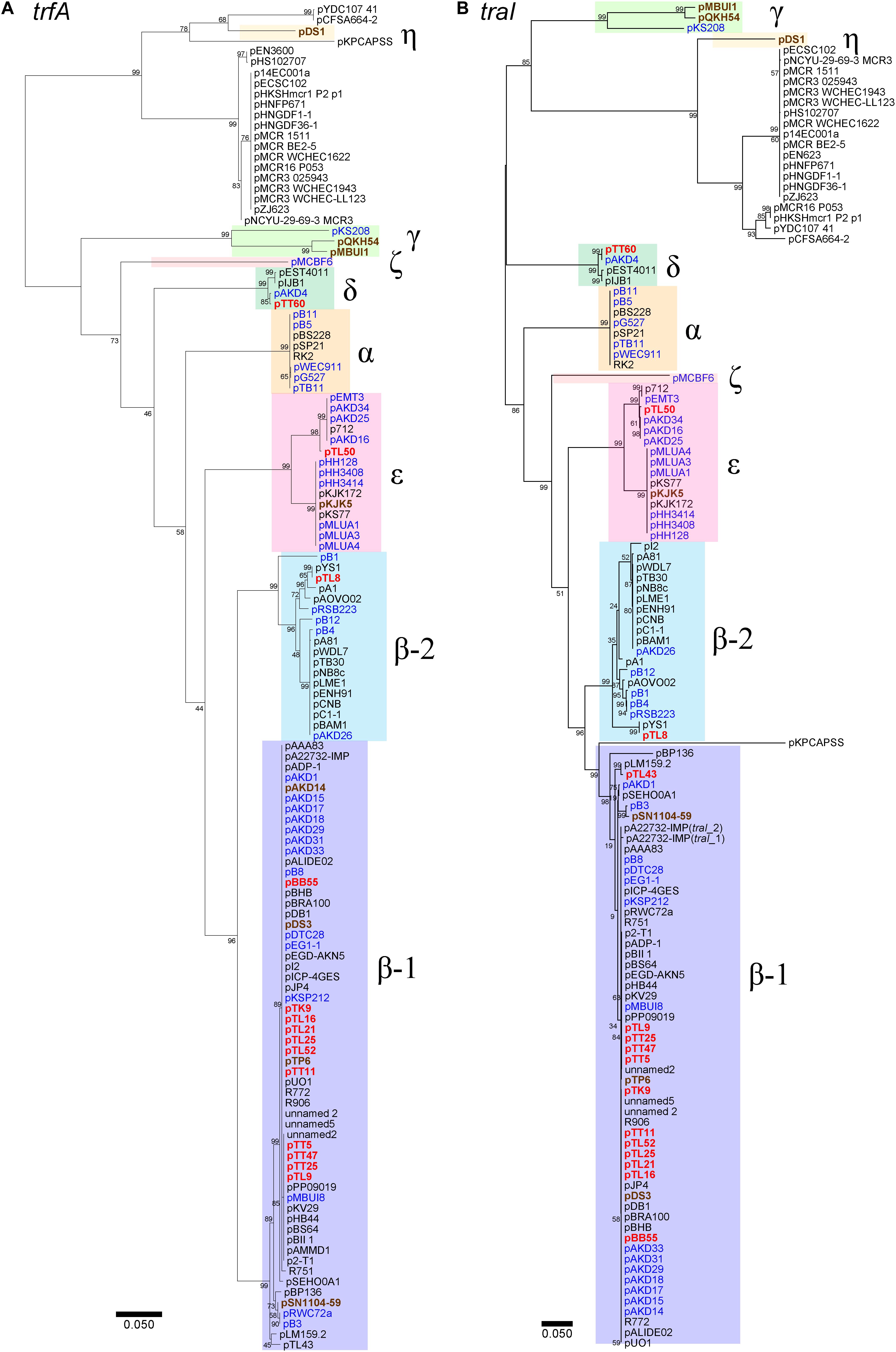
Figure 2. Phylogenetic analysis based on nucleotide sequences of (A) trfA (encoding replication initiation protein) and (B) traI (encoding relaxase) of IncP-1 plasmids. Plasmids with colors indicate that they were obtained by exogenous plasmid capturing, red for the obtained plasmids in the present study, blue for biparental mating, and brown for triparental mating. The accession numbers of other IncP-1 plasmids are listed in Supplementary Table S3. The unrooted trees were inferred by the neighbor joining method using Geneious Prime 2019. Bootstrap values are indicated at each node.
The Diversity of IncP-1β Subgroup Driven by MGE
The comparison of the sequences beyond the backbone genes revealed that the diversity of the set of plasmids studied here was driven by MGEs and accessory genes (Figure 3 and Supplementary Table S2). The two smallest IncP-1β1 plasmids originating both from the tomato rhizosphere, pTT5 and pTT47 (43,580 and 43,582 bp), are likely identical. Interestingly, they consisted only of the plasmid backbone genes except for the presence of IS1071 linked to a gene coding for a hypothetical protein. Furthermore, sequence analysis showed that the parA gene was lost from both plasmids, likely due to the insertion of IS1071 at this site (Supplementary Figure S2).
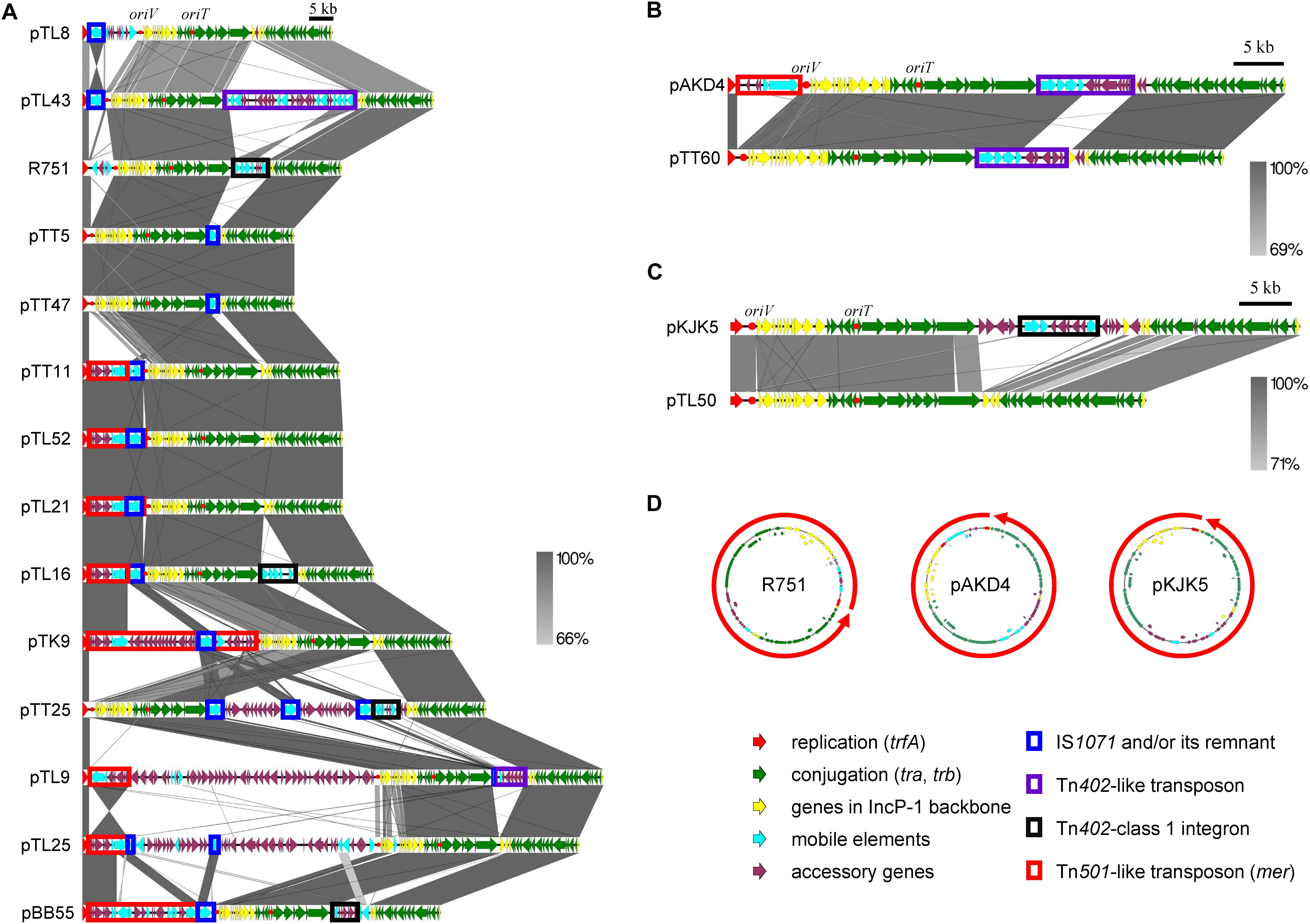
Figure 3. Comparisons of the whole genetic structure for the captured plasmids. (A) IncP-1β with representative plasmids R751 and pBB55 from manure soil, (B) IncP-1δ with pAKD4, and (C) IncP-1ε with pKJK5. trfA was set as the right end of the linear plasmid maps, and the start points and directions of the representative plasmids in the maps are shown by red arrows in panel (D). Block arrows with colors indicate coding sequences (CDSs) and their predicted functions (red for replication, green for conjugation, yellow for other genes in IncP-1 backbone, light blue for genes related to mobile genetic element, and magenta for accessory genes). Homologous regions are indicated by frame areas. The key mobile genetic elements (IS1071, Tn402, Tn402-integron, and Tn501) are shown by colored rectangular shapes that are shown below the figure.
Tn501-Like Transposon
The Tn501-like transposon linked to a mer operon was detected on seven of the 11 IncP-1β1 plasmids studied here (Figure 3A) and the transconjugants harboring these plasmids indeed grew on a mercury chloride-containing medium (data not shown). In all plasmids with Tn501 the insertion site was between trfA and oriV. However, a closer sequence comparison revealed a few distinct differences that were caused mainly by the insertion site of IS1071 and truncation of the Tn501 tnpATn501 coding for the transposase (Figure 4A and Supplementary Text).
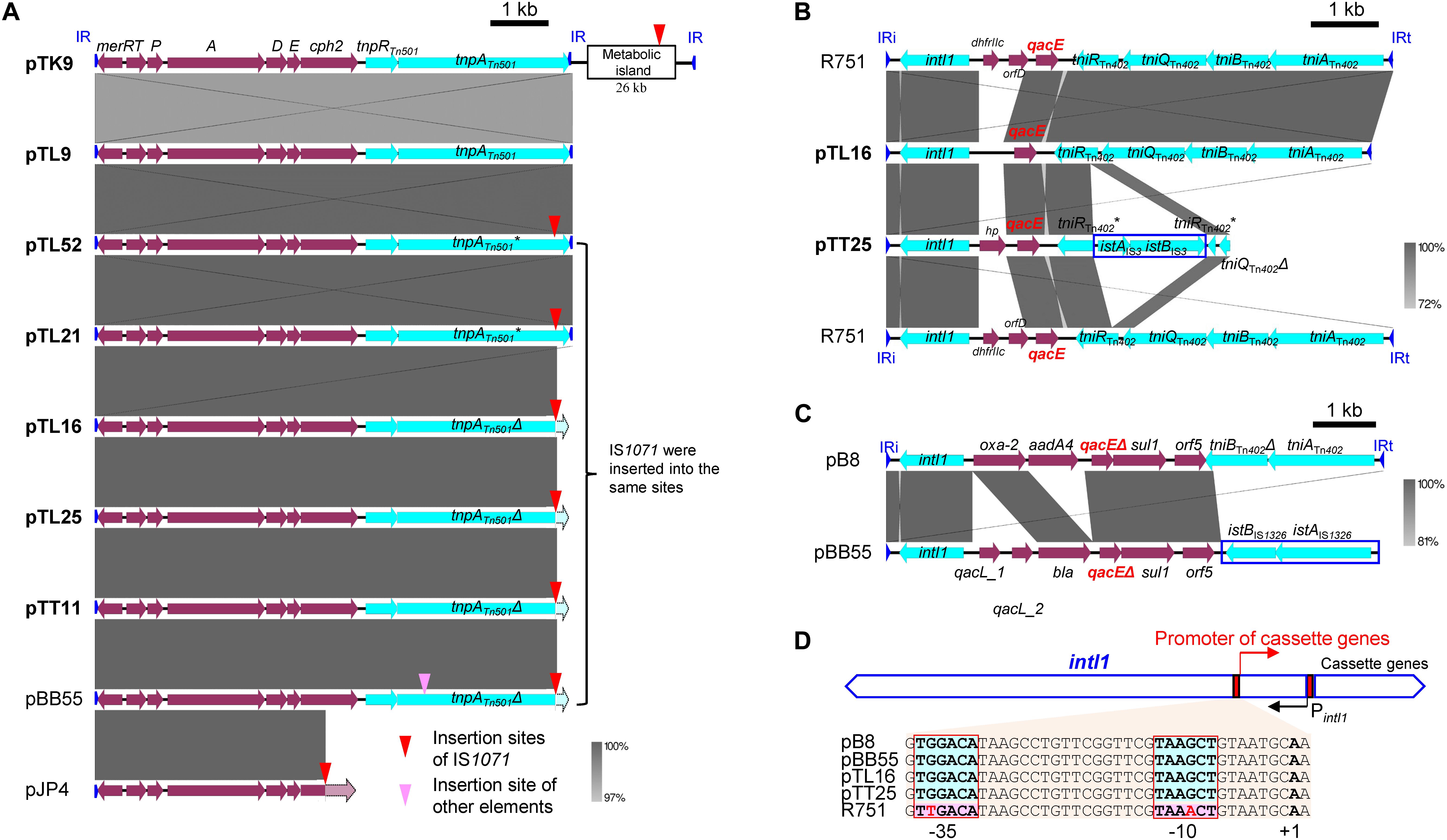
Figure 4. Comparisons of the genetic structure for the key mobile genetic elements (MGEs), Tn501 (A) and Tn402-class 1 integrons (B,C). CDSs and homologous regions are indicated by block arrows and framed areas, respectively. Inverted repeats (IRs) are shown by blue triangles. * or Δ indicates that the gene was disrupted or truncated by other MGEs, respectively. (A) Comparisons of Tn501 containing the mer operon found in IncP-1β-1 plasmids with pJP4, which has a mer operon. The insertion sites of IS1071 are pointed by red triangles. pTK9 had another inverted repeat of Tn501 in downstream of the metabolic island with IS1071 insertion. pBB55 had another insertion in the tnpATn501 gene shown by pink triangles. (B) Comparisons of Tn402-class 1 integron like regions in pTL16 and pTT25 with that in R751. IS3-family element is shown as a rectangle. (C) Comparisons of Tn402-class 1 integron-like regions in pBB55 (captured from manure soil) with those in pB8. IS1326-family element is shown by a rectangle. (D) Comparisons of promoters for gene cassettes in the Tn402-class 1 integrons. Red boxes show –35 and –10 region of the gene cassette and +1 indicates transcription start point. The boxes with light blue indicate PcW promoter (pB8, pBB55, pTL25, and pTT25), while those with pink indicate PcS promoter in R751.
Tn402/Class 1 Integrons
Complete or remnants of Tn402-like transposons were found in the four IncP-1β1 plasmids, pTL9, pTL16, pTL43, pTT25, and in the IncP-1δ plasmid pTT60 (Figure 3A and Supplementary Figure S3). Despite the differences in the backbone of the two plasmid subgroups, the integration site was always between traC and parA. In pTL16 and pTT25, the complete or the remnant of the Tn402-like transposon was linked to a class 1 integron, which was highly similar to the constellation in the prototype plasmid R751 (Figure 4B). In contrast to R751, the class 1 integrons on pTL16 and pTT25 did not carry any gene cassettes conferring antibiotic resistances but an intact qacE gene instead (Figure 4B). In R751, the class 1 integron is linked to a qacE in addition to an antibiotic resistance gene cassette (dhfrII, Figure 4B). Notably, nucleotide sequences of the promoters of gene cassettes on the integrons in pTL16, pTT25, and R751 were different; as shown in Figure 4D, the former two were identical with PcW promoters, while that in R751 was identical with PcS promoter (Labbate et al., 2008; Chamosa et al., 2017; Ghaly et al., 2017).
IS Elements
Different IS elements were identified in this set of IncP-1 plasmids, but by far the most frequently detected IS element was IS1071 (Figure 3A and Supplementary Figure S3). This IS element, or its remnant, was detected in all IncP-1β plasmids, and in eight plasmids, IS1071 was integrated between trfA and oriV (Figure 3A and Supplementary Figure S3). In the remaining plasmids, it was inserted between traC and parA (Figure 3A and Supplementary Figure S3). In seven plasmids, a complete IS1071 was detected, and in three plasmids, the IS1071 also contained direct repeats (Supplementary Table S2). In pTT11 a ΔtnpAIS1071 frame shift was detected in the IS1071 integrated into the Tn501 (Supplementary Table S2).
Metabolic Genes of IncP-1β Plasmids
Five IncP-1β plasmids captured from potato or lettuce rhizosphere carried putative metabolic gene clusters or even complete operons and therefore were larger than most of the remaining plasmids. In pTK9, the only plasmid captured from potato rhizosphere, a complete set for catechol degradation genes also related to chloroaniline degradation was inserted between trfA and oriV (Figure 3A and Supplementary Figure S3). The metabolic genes were localized downstream of the mer operon/Tn501 followed by IS1071 (Figure 3A and Supplementary Figure S3). Interestingly, another Tn501 inverted repeat was found downstream of IS1071 (Supplementary Figure S3). The IncP-1β2 plasmid pTL8 carried a chlorite dismutase gene inserted between trfA-oriV in a Tn3-like transposon downstream from a complete IS1071 with direct repeat (Supplementary Figure S3). Plasmid pTL9 carries a remarkably large fragment (50 kb) between trfA and oriV, showing high identity (>99%) with the genomic DNA of Cupriavidus basilensis strains X1 and 4G11 (Supplementary Figure S3). This region contained putative genes for glycolate and pyruvate metabolisms (50 kp). In addition, this plasmid carried putative molybdenum transporter genes modEAGC inserted between traC and parA linked to Tn402 (Supplementary Figure S3). Putative novel dioxygenases (Rieske type) and C4-organic transporters were identified in pTL25 downstream of the mer operon/Tn501 and two remnant IS1071 copies (Supplementary Figure S3). In pTL43, the insertion of extradiol dioxygenase genes into a complete Tn402 was observed between traC and parA (Supplementary Figure S3). The metabolic genes were linked to three IS21, two of which were identical (Supplementary Figure S3).
A very curious observation was that a large proportion of plasmid pTL21 seemed to have lost a large metabolic island. pTL21 had been considered to carry putative polyaromatic hydrocarbon (PAH) ring hydroxylating dioxygenase gene, because a PCR product was detected with primers for PAH-rhdα (Ding et al., 2010). In-depth analyses in the assembly process of pTL21 revealed that only a smaller proportion of pTL21 carried the above gene as a part of nag operon, named as pTL21∗(Supplementary Text). pTL21∗ was 81,088 bp in size and carried a complete nag operon, which was integrated into IS1071 linked to Tn501-mer operon (Supplementary Text and Supplementary Figure S4). The putative naphthalene degradative (nag) genes were flanked by the two copies of IS1071 in pTL21∗, which might have been lost by homologous recombination between them (Supplementary Figure S4). This was a possible reason that the majorly assembled plasmid (i.e., pTL21) did not carry the nagAg operon.
Discussion
Quantification of IncP-1 plasmids in TC-DNA revealed that remarkably, these plasmids increased in relative abundance in the rhizosphere of lettuce and tomato plants along with the plant age. These results obtained under greenhouse conditions confirmed our previous data on the rhizosphere of lettuce grown under field conditions in three different soils (Jechalke et al., 2014). However, the increase in relative abundance in the rhizosphere of lettuce compared to bulk soil was stronger under field conditions (more than two orders of magnitude) compared to the pot experiment. These plant species-dependent differences were likely due to the differences in amount of root exudates and deposits (Neumann et al., 2014) shaping the plant species-dependent bacterial community. Although we did not perform amplicon sequencing of 16S rRNA genes amplified from rhizosphere TC-DNA of lettuce, tomato, and potato in the present project, this was done for lettuce and potato both grown under field conditions in DS soil. The rhizosphere microbiome was distinct (unpublished) likely due to differences in exudate composition.
The exogenous plasmid capturing methods have a limitation in so far as the original host(s) of the obtained plasmids is not determined (Smalla et al., 2015). Several potential hosts of IncP-1 plasmids including Pseudomonas, Variovorax, and Burkholderia were previously reported as rhizosphere responders (genera with a significant increase in relative abundance in the rhizosphere compared to bulk soil) of lettuce grown in DS under field conditions (Schreiter et al., 2014). Currently, except for one isolate, the IncP-1 plasmids were not successfully detected by PCR of bacterial isolates obtained from the rhizosphere of lettuce and tomato (data not shown). This was probably because the original hosts of IncP-1 plasmids were not cultivable or because they did not belong to the dominant bacterial isolates. To identify the original hosts of the obtained plasmids, further in-depth analysis with HiC method as reported in Stalder et al. (2019) will be necessary.
In sewage sludge or manure, high concentrations of pollutants likely provide a selective advantage of carrying IncP-1 plasmids because they typically carried multiple antibiotic resistance genes (Schlüter et al., 2007). In contrast, the rhizosphere samples studied here originated from plants grown in unpolluted soil.
Most unexpected was the high diversity of IncP-1 plasmids captured. In contrast, only one dominant IncP-1 plasmid type was captured from a soil treated with manure spiked with doxycycline (Blau et al., 2019) by biparental exogenous isolation as previously described (Blau et al., 2018; Supplementary Text). Forty plasmids captured from this soil (from three soil replicates) displayed identical restriction patterns (data not shown). One representative of these plasmids, pBB55, was sequenced in the same PacBio sequencing run as the 14 IncP-1 plasmids from the plant rhizosphere and represented a multi-resistance plasmid carrying a tet(C) gene conferring resistance to doxycycline but also others such as macrolide resistance genes msr(E) and mph(E), mer genes conferring resistance to mercury, aminoglycoside resistance genes aph(3″)-Ib and aph(6)-Id, beta lactamase gene blaOXA-2, and emrE multidrug efflux protein genes (Supplementary Figure S5, GenBank accession no. MH392232). These genes were contained by several transposons and integrons, and they were inserted into the two insertion hot spots (Supplementary Figure S5) like the other obtained IncP-1β plasmids (Supplementary Figure S3). One of them was between trfA and oriV, in which tet(C), msr(E), and mph(E) genes were contained by a composite transposon of IS26s, aph(3″)-Ib and aph(6)-Id were contained by a Tn3-like transposon, and mer genes were contained by Tn501 remnant with IS1071 (Supplementary Figure S5). The other was between parA and upf30.5, in which emrE was in Tn402-class 1 integron remnant (with IS1326) in addition with sulfonamide resistance gene sul1 and quaternary ammonium compound resistance gene qacEΔ (Supplementary Figure S5). We propose that plasmid diversity observed under strong selective conditions including high pollutants and/or antibiotic exposure is lower due to the increased relative abundance of host populations carrying IncP-1 plasmids that provide fitness advantages by their accessory genes. In contrast, the plasmids from the rhizosphere of plants grown in unpolluted soils showed higher diversity, and none of them carried previously known antibiotic resistance genes by searching in CARD. The presence of highly diverse IncP-1 plasmids suggested that the role of IncP-1 plasmids in unpolluted rhizosphere might be not only be conferring resistance to antibiotics, disinfectants, heavy metals, and/or degradation of xenobiotics (Hsu and Bartha, 1979; Top and Springael, 2003; Smets and Barkay, 2005) but also providing genetic flexibility of microbes in rhizosphere by a high rate of gene acquisition and loss via various IncP-1 plasmids.
The present study points to the important role of plasmid-localized MGEs in the acquisition of accessory genes and plasmid evolution.
Tn501 was always linked to mercury resistance in seven of the sequenced IncP-1 plasmids (Figure 3A and Supplementary Figure S3). Lilley and Bailey (1997) reported that plasmids with mercury resistance genes were isolated from the rhizosphere of sugar beets grown in arable field soils. However, in their report, the mercury resistance operon was carried by large Pseudomonas plasmids (Lilley and Bailey, 1997). To date, we can only speculate about the widespread dissemination of mercury resistance genes, and mercury resistance might provide a fitness advantage to their hosts. The amount of mercury compounds in soil might be due to pedogenesis, their presence in organic fertilizers (sewage sludge, manure), or their usage as fungicide, e.g., for treating seeds.
The class 1 integrons were similarly localized on the IncP-1 plasmids from unpolluted rhizosphere as those present on the prototype IncP-1β1 plasmid, R751 found in clinical isolate (Jobanputra and Datta, 1974). Sequence comparison of the class 1 integron identified in pTL16 from the lettuce rhizosphere displayed a high similarity to that in R751, except that the latter plasmid contained two additional gene cassettes with the dhfr gene conferring the trimethoprim resistance that initially led to its isolation. The class 1 integron of both plasmids carried an intact qacE gene, instead of qacEΔ1, which is presently far more frequently distributed (Ghaly et al., 2017). Indeed, the integron in pBB55 carried the qacEΔ1 gene (Figure 4C), which was also similarly found in another IncP-1β plasmid, pB8, obtained by biparental plasmid capturing from an activated sludge (Schlüter et al., 2005). pTL16 seems to carry an ancestral class 1 integron, although loss of the antibiotic resistance gene cassettes localized on R751 cannot be excluded. Ghaly et al. (2017) reported an Enterobacter cloacae isolated from baby spinach leaves that also carried a class 1 integron inserted into a Tn402 localized on an IncP-1β plasmid, pOP-I. They argued that plants eaten raw might be an important transmission route and a likely link from the environment to humans. Further, the promoters of these integrons in pTL16, pTT25, and also in pBB55 and pB8 were identical to “weak” PcW promoters (Figure 4D), which are considered to be “ancestral” promoters conserved in chromosomal integrons of “environmental” strains, while that in R751 was “strong” PcS promoter, which is common in “clinical” integron cassette arrays and considered to be an “evolved” promoter (Labbate et al., 2008; Chamosa et al., 2017; Ghaly et al., 2017). These facts indicate that the IncP-1 plasmids obtained from the rhizosphere possessed relatively ancestral types of class 1 integrons.
In five plasmids, MGEs were linked to metabolic degradative genes. The in-depth exploration of the metabolic genes linked to the IncP-1 plasmid captured from the rhizosphere and their contribution to host fitness deserves further studies. The products of these genes might be advantageous for the survival of host(s) of these plasmids in rhizosphere environments. As for pTL21, here we can only speculate that during growth of transconjugant, the presence of the nag operon was a metabolic burden and disadvantageous under the growth conditions on nutrient media. This might well present a general strategy to lower the metabolic burden of the population. In the rhizosphere, the presence of the nagAg operon might not represent a disadvantage due to lower growth rates and the presence of aromatic compounds in the root exudates (Neumann et al., 2014; Windisch et al., 2017).
pTL50 assigned to the IncP-1ε subgroup could represent the first archetype of the IncP-1ε group only carrying core genes (Figure 3C). Recently, we isolated numerous IncP-1ε plasmids conferring sulfadiazine or tetracycline resistance from bacteria of manure, digestates of biogas plants, and manure-treated soils by exogenous plasmid capturing (Heuer et al., 2012; Wolters et al., 2015). Similar to pKJK5, originally isolated from manure-treated soils (Bahl et al., 2007), all these plasmids carried class 1 integrons with tet(A) gene and their diversity was proposed to be driven by different sets of other gene cassettes (Heuer et al., 2012; Jechalke et al., 2014; Wolters et al., 2015). In contrast, IncP-1ε plasmids originating from mercury or 2,4-D polluted soils including pAKD34, pAKD25, pAKD16, and pEMT3 did not contain class 1 integrons but mer genes or metabolic genes (Sen et al., 2011; Sen et al., 2013; Li et al., 2016). pTL50 can be an archetype plasmid of them as a vector for genes in these polluted environments.
Conclusion
The discovery that bacterial populations carrying IncP-1 plasmids are increased in relative abundance in the rhizosphere of plants grown in unpolluted soil in a plant species-dependent manner is of importance as these self-transmissible plasmids might represent important shuttles of genes conferring adaptive traits or MGEs ready to recruit them. Here we showed that the exogenously captured IncP-1 plasmids exhibited an unexpectedly high diversity. As described in Brown et al. (2013), it is still unclear whether the IncP-1 plasmids with a few or no accessory genes are advantageous for their hosts. They might be advantageous because they allow rapid and dynamic acquisition or loss of accessory genes due to the presence of MGEs that were identical or highly similar to the ones found on the clinical IncP-1 plasmids conferring multiple antibiotic resistances.
Data Availability Statement
The datasets presented in this study can be found in online repositories. The names of the repository/repositories and accession number(s) can be found in the article/Supplementary Material.
Author Contributions
KS conceived, designed, and supervised the study. MS, EN, TE, IW, CS, BB, and JO performed the experiments and data analyses. MS, EN, KB, SJ, BB, JO, and KS wrote, reviewed, and edited the manuscript. All authors contributed to manuscript revision, read, and approved the submitted version.
Funding
This study was partly supported by JSPS KAKENHI Grant numbers 15H05618, 15KK0278, 19H02869, and 19H05686 to MS.
Conflict of Interest
The authors declare that the research was conducted in the absence of any commercial or financial relationships that could be construed as a potential conflict of interest.
Supplementary Material
The Supplementary Material for this article can be found online at: https://www.frontiersin.org/articles/10.3389/fmicb.2020.590776/full#supplementary-material
Footnotes
References
Alcock, B. P., Raphenya, A. R., Lau, T. T. Y., Tsang, K. K., Bouchard, M., Edalatmand, A., et al. (2020). CARD 2020: antibiotic resistome surveillance with the comprehensive antibiotic resistance database. Nucleic Acids Res. 48, D517–D525.
Bahl, M., Hansen, L., Goesmann, A., and Sørensen, S. (2007). The multiple antibiotic resistance IncP-1 plasmid pKJK5 isolated from a soil environment is phylogenetically divergent from members of the previously established alpha, beta and delta sub-groups. Plasmid 58, 31–43. doi: 10.1016/j.plasmid.2006.11.007
Bahl, M. I., Burmølle, M., Meisner, A., Hansen, L. H., and Sørensen, S. J. (2009). All IncP-1 plasmid subgroups, including the novel epsilon subgroup, are prevalent in the influent of a Danish wastewater treatment plant. Plasmid 62, 134–139. doi: 10.1016/j.plasmid.2009.05.004
Binh, C. T., Heuer, H., Kaupenjohann, M., and Smalla, K. (2008). Piggery manure used for soil fertilization is a reservoir for transferable antibiotic resistance plasmids. FEMS Microbiol. Ecol. 66, 25–37. doi: 10.1111/j.1574-6941.2008.00526.x
Blau, K., Bettermann, A., Jechalke, S., Fornefeld, E., Vanrobaeys, Y., Stalder, T., et al. (2018). The Transferable Resistome of Produce. mBio 9:e01300-18.
Blau, K., Jacquiod, S., Sørensen, S. J., Su, J.-Q., Zhu, Y.-G., Smalla, K., et al. (2019). Manure and doxycycline affect the bacterial community and its resistome in lettuce rhizosphere and bulk soil. Front. Microbiol. 10:725. doi: 10.3389/fmicb.2019.00725
Brown, C. J., Sen, D., Yano, H., Bauer, M. L., Rogers, L. M., Van Der Auwera, G. A., et al. (2013). Diverse broad-host-range plasmids from freshwater carry few accessory genes. Appl. Environ. Microbiol. 79, 7684–7695. doi: 10.1128/aem.02252-13
Chamosa, L. S., Alvarez, V. E., Nardelli, M., Quiroga, M. P., Cassini, M. H., and Centron, D. (2017). Lateral antimicrobial resistance genetic transfer is active in the open environment. Sci. Rep. 7:513.
Christensen, S. K., Mikkelsen, M., Pedersen, K., and Gerdes, K. (2001). RelE, a global inhibitor of translation, is activated during nutritional stress. Proc. Natl. Acad. Sci. U.S.A. 98, 14328–14333. doi: 10.1073/pnas.251327898
Dahlberg, C., Linberg, C., Torsvik, V. L., and Hermansson, M. (1997). Conjugative plasmids isolated from bacteria in marine environments show various degrees of homology to each other and are not closely related to well-characterized plasmids. Appl. Environ. Microbiol. 63, 4692–4697. doi: 10.1128/aem.63.12.4692-4697.1997
Datta, N., Hedges, R. W., Shaw, E. J., Sykes, R. B., and Richmond, M. H. (1971). Properties of an R Factor from Pseudomonas aeruginosa. J. Bacteriol. 108, 1244–1249.
Dealtry, S., Ding, G. C., Weichelt, V., Dunon, V., Schlüter, A., Martini, M. C., et al. (2014). Cultivation-independent screening revealed hot spots of IncP-1, IncP-7 and IncP-9 plasmid occurrence in different environmental habitats. PLoS One 9:e89922. doi: 10.1371/journal.pone.0089922
Ding, G. C., Heuer, H., Zühlke, S., Spiteller, M., Pronk, G. J., Heister, K., et al. (2010). Soil type-dependent responses to phenanthrene as revealed by determining the diversity and abundance of polycyclic aromatic hydrocarbon ring-hydroxylating dioxygenase genes by using a novel PCR detection system. Appl. Environ. Microbiol. 76, 4765–4771. doi: 10.1128/aem.00047-10
Dröge, M., Pühler, A., and Selbitschka, W. (2000). Phenotypic and molecular characterization of conjugative antibiotic resistance plasmids isolated from bacterial communities of activated sludge. Mol. Gen. Genet. 263, 471–482. doi: 10.1007/s004380051191
Ghaly, T. M., Chow, L., Asher, A. J., Waldron, L. S., and Gillings, M. R. (2017). Evolution of class 1 integrons: mobilization and dispersal via food-borne bacteria. PLoS One 12:e0179169. doi: 10.1371/journal.pone.0179169
Gomes, N. C., Flocco, C. G., Costa, R., Junca, H., Vilchez, R., Pieper, D. H., et al. (2010). Mangrove microniches determine the structural and functional diversity of enriched petroleum hydrocarbon-degrading consortia. FEMS Microbiol. Ecol. 74, 276–290. doi: 10.1111/j.1574-6941.2010.00962.x
Götz, A., Pukall, R., Smit, E., Tietze, E., Prager, R., Tschäpe, H., et al. (1996). Detection and characterization of broad-host-range plasmids in environmental bacteria by PCR. Appl. Environ. Microbiol. 62, 2621–2628. doi: 10.1128/aem.62.7.2621-2628.1996
Haagensen, J. A. J., Hansen, S. K., Johansen, T., and Molin, S. (2002). In situ detection of horizontal transfer of mobile genetic elements. FEMS Microbiol. Ecol. 42, 261–268. doi: 10.1111/j.1574-6941.2002.tb01016.x
Heuer, H., Binh, C. T., Jechalke, S., Kopmann, C., Zimmerling, U., Krögerrecklenfort, E., et al. (2012). IncP-1epsilon plasmids are important vectors of antibiotic resistance genes in agricultural systems: diversification driven by class 1 integron gene cassettes. Front. Microbiol. 3:2. doi: 10.3389/fmicb.2012.00002
Heuer, H., and Smalla, K. (2012). Plasmids foster diversification and adaptation of bacterial populations in soil. FEMS Microbiol. Rev. 36, 1083–1104. doi: 10.1111/j.1574-6976.2012.00337.x
Heuer, H., Szczepanowski, R., Schneiker, S., Pühler, A., Top, E. M., and Schlüter, A. (2004). The complete sequences of plasmids pB2 and pB3 provide evidence for a recent ancestor of the IncP-1beta group without any accessory genes. Microbiology 150, 3591–3599. doi: 10.1099/mic.0.27304-0
Hsu, T. S., and Bartha, R. (1979). Accelerated mineralization of two organophosphate insecticides in the rhizosphere. Appl. Environ. Microbiol. 37, 36–41. doi: 10.1128/aem.37.1.36-41.1979
Jechalke, S., Dealtry, S., Smalla, K., and Heuer, H. (2013). Quantification of IncP-1 plasmid prevalence in environmental samples. Appl. Environ. Microbiol. 79, 1410–1413. doi: 10.1128/aem.03728-12
Jechalke, S., Schreiter, S., Wolters, B., Dealtry, S., Heuer, H., and Smalla, K. (2014). Widespread dissemination of class 1 integron components in soils and related ecosystems as revealed by cultivation-independent analysis. Front. Microbiol. 4:420. doi: 10.3389/fmicb.2013.00420
Jiang, Y., Pogliano, J., Helinski, D. R., and Konieczny, I. (2002). ParE toxin encoded by the broad-host-range plasmid RK2 is an inhibitor of Escherichia coli gyrase. Mol. Microbiol. 44, 971–979. doi: 10.1046/j.1365-2958.2002.02921.x
Jobanputra, R. S., and Datta, N. (1974). Trimethoprim R-factors in enterobacteria from clinical specimens. J. Med. Microbiol. 7, 169–177. doi: 10.1099/00222615-7-2-169
Kamachi, K., Sota, M., Tamai, Y., Nagata, N., Konda, T., Inoue, T., et al. (2006). Plasmid pBP136 from Bordetella pertussis represents an ancestral form of IncP-1beta plasmids without accessory mobile elements. Microbiology 152, 3477–3484. doi: 10.1099/mic.0.29056-0
Klümper, U., Riber, L., Dechesne, A., Sannazzarro, A., Hansen, L. H., Sørensen, S. J., et al. (2015). Broad host range plasmids can invade an unexpectedly diverse fraction of a soil bacterial community. ISME J. 9, 934–945. doi: 10.1038/ismej.2014.191
Kumar, S., Stecher, G., and Tamura, K. (2016). MEGA7: molecular evolutionary genetics analysis version 7.0 for bigger datasets. Mol. Biol. Evol. 33, 1870–1874. doi: 10.1093/molbev/msw054
Labbate, M., Roy Chowdhury, P., and Stokes, H. W. (2008). A class 1 integron present in a human commensal has a hybrid transposition module compared to Tn402: evidence of interaction with mobile DNA from natural environments. J. Bacteriol. 190, 5318–5327. doi: 10.1128/jb.00199-08
Li, X., Wang, Y., Brown, C. J., Yao, F., Jiang, Y., Top, E. M., et al. (2016). Diversification of broad host range plasmids correlates with the presence of antibiotic resistance genes. FEMS Microbiol. Ecol. 92:fiv151. doi: 10.1093/femsec/fiv151
Lilley, A. K., and Bailey, M. J. (1997). The acquisition of indigenous plasmids by a genetically marked Pseudomonad population colonizing the sugar beet phytosphere is related to local environmental conditions. Appl. Environ. Microbiol. 63, 1577–1583. doi: 10.1128/aem.63.4.1577-1583.1997
Neumann, G., Bott, S., Ohler, M. A., Mock, H. P., Lippmann, R., Grosch, R., et al. (2014). Root exudation and root development of lettuce (Lactuca sativa L. cv. Tizian) as affected by different soils. Front. Microbiol. 5:2. doi: 10.3389/fmicb.2014.00002
Rademaker, J., and De Bruijn, F. (1997). Characterization and Classification of Microbes by Rep-PCR Genomic Fingerprinting and Computer Assisted Pattern Analysis. DNA Markers: Protocols, Applications and Overviews. New York, NY: John Wiley & Sons, 151–171.
Rühlmann, J., and Ruppel, S. (2005). Effects of organic amendments on soil carbon content and microbial biomass – results of the long-term box plot experiment in Grossbeeren. Arch. Agron. Soil Sci. 51, 163–170. doi: 10.1080/03650340400026651
Schlüter, A., Heuer, H., Szczepanowski, R., Forney, L. J., Thomas, C. M., Pühler, A., et al. (2003). The 64 508 bp IncP-1beta antibiotic multiresistance plasmid pB10 isolated from a waste-water treatment plant provides evidence for recombination between members of different branches of the IncP-1beta group. Microbiology 149, 3139–3153. doi: 10.1099/mic.0.26570-0
Schlüter, A., Heuer, H., Szczepanowski, R., Poler, S. M., Schneiker, S., Pühler, A., et al. (2005). Plasmid pB8 is closely related to the prototype IncP-1β plasmid R751 but transfers poorly to Escherichia coli and carries a new transposon encoding a small multidrug resistance efflux protein. Plasmid 54, 135–148. doi: 10.1016/j.plasmid.2005.03.001
Schlüter, A., Szczepanowski, R., Pühler, A., and Top, E. M. (2007). Genomics of IncP-1 antibiotic resistance plasmids isolated from wastewater treatment plants provides evidence for a widely accessible drug resistance gene pool. FEMS Microbiol. Rev. 31, 449–477. doi: 10.1111/j.1574-6976.2007.00074.x
Schreiter, S., Ding, G.-C., Heuer, H., Neumann, G., Sandmann, M., Grosch, R., et al. (2014). Effect of the soil type on the microbiome in the rhizosphere of field-grown lettuce. Front. Microbiol. 5:144. doi: 10.3389/fmicb.2014.00144
Seemann, T. (2014). Prokka: rapid prokaryotic genome annotation. Bioinformatics 30, 2068–2069. doi: 10.1093/bioinformatics/btu153
Sen, D., Brown, C. J., Top, E. M., and Sullivan, J. (2013). Inferring the evolutionary history of IncP-1 plasmids despite incongruence among backbone gene trees. Mol. Biol. Evol. 30, 154–166. doi: 10.1093/molbev/mss210
Sen, D., Van Der Auwera, G. A., Rogers, L. M., Thomas, C. M., Brown, C. J., and Top, E. M. (2011). Broad-host-range plasmids from agricultural soils have IncP-1 backbones with diverse accessory genes. Appl. Environ. Microbiol. 77, 7975–7983. doi: 10.1128/aem.05439-11
Shintani, M., Matsui, K., Inoue, J., Hosoyama, A., Ohji, S., Yamazoe, A., et al. (2014). Single-cell analyses revealed transfer ranges of IncP-1, IncP-7, and IncP-9 plasmids in a soil bacterial community. Appl. Environ. Microbiol. 80, 138–145. doi: 10.1128/aem.02571-13
Smalla, K., Haines, A. S., Jones, K., Krögerrecklenfort, E., Heuer, H., Schloter, M., et al. (2006). Increased abundance of IncP-1beta plasmids and mercury resistance genes in mercury-polluted river sediments: first discovery of IncP-1beta plasmids with a complex mer transposon as the sole accessory element. Appl. Environ. Microbiol. 72, 7253–7259. doi: 10.1128/aem.00922-06
Smalla, K., Heuer, H., Götz, A., Niemeyer, D., Krögerrecklenfort, E., and Tietze, E. (2000). Exogenous isolation of antibiotic resistance plasmids from piggery manure slurries reveals a high prevalence and diversity of IncQ-like plasmids. Appl. Environ. Microbiol. 66, 4854–4862. doi: 10.1128/aem.66.11.4854-4862.2000
Smalla, K., Jechalke, S., and Top, E. M. (2015). Plasmid detection, characterization and ecology. Microbiol. Spectr. 3, LAS–0038–2014. doi: 10.1128/microbiolspec.PLAS-0038-2014
Smets, B. F., and Barkay, T. (2005). Horizontal gene transfer: perspectives at a crossroads of scientific disciplines. Nat. Rev. Microbiol. 3, 675–678. doi: 10.1038/nrmicro1253
Stalder, T., Press, M. O., Sullivan, S., Liachko, I., and Top, E. M. (2019). Linking the resistome and plamidome to the microbiome. ISME J. 13, 2437–2446. doi: 10.1038/s41396-019-0446-4
Sullivan, M. J., Petty, N. K., and Beatson, S. A. (2011). Easyfig: a genome comparison visualizer. Bioinformatics 27, 1009–1010. doi: 10.1093/bioinformatics/btr039
Suzuki, T., Muroga, Y., Takahama, M., and Nishimura, Y. (2000). Roseigium denhamense gen. nov., sp. nov. and Roseibium hemelinense sp. nov., aerobic bacteriochlorophyll-containing bacteria isolated from the east and west coasts of Australia. Int. J. Syst. Evol. Microbiol. 50(Pt 6), 2151–2156. doi: 10.1099/00207713-50-6-2151
Tauch, A., Schlüter, A., Bischoff, N., Goesmann, A., Meyer, F., and Pühler, A. (2003). The 79,370-bp conjugative plasmid pB4 consists of an IncP-1beta backbone loaded with a chromate resistance transposon, the strA-strB streptomycin resistance gene pair, the oxacillinase gene bla(NPS-1), and a tripartite antibiotic efflux system of the resistance-nodulation-division family. Mol. Genet. Genom. 268, 570–584. doi: 10.1007/s00438-002-0785-z
Tennstedt, T., Szczepanowski, R., Krahn, I., Pühler, A., and Schlüter, A. (2005). Sequence of the 68,869 bp IncP-1alpha plasmid pTB11 from a waste-water treatment plant reveals a highly conserved backbone, a Tn402-like integron and other transposable elements. Plasmid 53, 218–238. doi: 10.1016/j.plasmid.2004.09.004
Thompson, J. D., Higgins, D. G., and Gibson, T. J. (1994). CLUSTAL W: improving the sensitivity of progressive multiple sequence alignment through sequence weighting, position-specific gap penalties and weight matrix choice. Nucleic Acids Res. 22, 4673–4680. doi: 10.1093/nar/22.22.4673
Thorsted, P. A., Macartney, D. P., Akhtar, P., Haines, A. S., Ali, N., Davidson, P., et al. (1998). Complete sequence of the IncP beta plasmid R751: implications for evolution and organisation of the IncP backbone. J. Mol. Biol. 282, 969–990. doi: 10.1006/jmbi.1998.2060
Tietze, E., Tschäpe, H., and Voigt, W. (1989). Characterization of new resistance plasmids belonging to incompatibility group IncQ. J. Basic Microbiol. 29, 695–706. doi: 10.1002/jobm.3620291013
Top, E. M., and Springael, D. (2003). The role of mobile genetic elements in bacterial adaptation to xenobiotic organic compounds. Curr. Opin. Biotechnol. 14, 262–269. doi: 10.1016/s0958-1669(03)00066-1
Van der Auwera, G. A., Król, J. E., Suzuki, H., Foster, B., Van Houdt, R., Brown, C. J., et al. (2009). Plasmids captured in C. metallidurans CH34: defining the PromA family of broad-host-range plasmids. Antonie Van Leeuwenhoek 96, 193–204. doi: 10.1007/s10482-009-9316-9
Windisch, S., Bott, S., Ohler, M.-A., Mock, H.-P., Lippmann, R., Grosch, R., et al. (2017). Rhizoctonia solani and bacterial inoculants stimulate root exudation of antifungal compounds in lettuce in a soil-type specific manner. Agronomy 7:44. doi: 10.3390/agronomy7020044
Wolters, B., Kyselkova, M., Krögerrecklenfort, E., Kreuzig, R., and Smalla, K. (2014). Transferable antibiotic resistance plasmids from biogas plant digestates often belong to the IncP-1epsilon subgroup. Front. Microbiol. 5:765. doi: 10.3389/fmicb.2014.00765
Keywords: lettuce-, tomato-, and potato rhizosphere, korB qPCR, IncP-1 plasmid, PacBio sequencing
Citation: Shintani M, Nour E, Elsayed T, Blau K, Wall I, Jechalke S, Spröer C, Bunk B, Overmann J and Smalla K (2020) Plant Species-Dependent Increased Abundance and Diversity of IncP-1 Plasmids in the Rhizosphere: New Insights Into Their Role and Ecology. Front. Microbiol. 11:590776. doi: 10.3389/fmicb.2020.590776
Received: 03 August 2020; Accepted: 15 October 2020;
Published: 27 November 2020.
Edited by:
Clay Fuqua, Indiana University Bloomington, United StatesReviewed by:
Christopher Karl Yost, University of Regina, CanadaHui Li, Institute of Applied Ecology (CAS), China
Copyright © 2020 Shintani, Nour, Elsayed, Blau, Wall, Jechalke, Spröer, Bunk, Overmann and Smalla. This is an open-access article distributed under the terms of the Creative Commons Attribution License (CC BY). The use, distribution or reproduction in other forums is permitted, provided the original author(s) and the copyright owner(s) are credited and that the original publication in this journal is cited, in accordance with accepted academic practice. No use, distribution or reproduction is permitted which does not comply with these terms.
*Correspondence: Kornelia Smalla, a29ybmVsaWEuc21hbGxhQGp1bGl1cy1rdWVobi5kZQ==
†These authors have contributed equally to this work