- 1School of Ocean Sciences, Bangor University, Bangor, United Kingdom
- 2School of Natural Sciences, Bangor University, Bangor, United Kingdom
- 3UWA School of Agriculture and Environment, University of Western Australia, Perth, WA, Australia
The fecal indicator organism (FIO) Escherichia coli is frequently used as a general indicator of sewage contamination and for evaluating the success of shellfish cleaning (depuration) processes. To evaluate the robustness of this approach, the accumulation, retention, and depuration of non-pathogenic E. coli, pathogenic E. coli O157:H7 and norovirus GII (NoV GII) RNA were evaluated using a combination of culture-based (E. coli) and molecular methods (E. coli, NoV GII) after exposure of mussels (Mytilus edulis) to water contaminated with human feces. We simulated water contamination after a point-source release from a combined sewer overflow (CSO) where untreated wastewater is released directly into the coastal zone. All three microbiological indicators accumulated rapidly in the mussels, reaching close to maximum concentration within 3 h of exposure, demonstrating that short CSO discharges pose an immediate threat to shellfish harvesting areas. Depuration (72 h) in clean water proved partially successful at removing both pathogenic and non-pathogenic E. coli from shellfish tissue, but failed to eradicate NoV GII RNA. We conclude that current EU standards for evaluating microbiological risk in shellfish are inadequate for protecting consumers against exposure to human norovirus GII found in polluted marine waters.
Introduction
Globally, 18 million tons of marine molluscs are harvested each year with an estimated value of $35 billion, comprising 9% of the value of fisheries worldwide (Wijsman et al., 2019; FAO, 2020). In addition, shellfish consumption is becoming an increasingly important part of the human diet and is seen as an area for expansion and economic growth in many countries (Oehlenschlager, 2012; Silver, 2014; Bostock et al., 2016). Sustainable shellfish production, however, relies on the presence of clean water that is free from both organic and inorganic pollutants (Mohebbi-Nozar et al., 2014; Blanco, 2018; Enuneku et al., 2018). Microbiological contamination of molluscan shellfish can readily occur in areas where coastal waters are polluted with sewage effluent, and agricultural run-off, such that shellfish accumulate large amounts of bacterial or viral pathogens (Bosch et al., 1995; Burkhardt and Calci, 2000; Winterbourn et al., 2016). In many parts of the world, shellfish are grown in areas exposed to treated or untreated wastewater which may also contain human pathogens (e.g., Cryptosporidium, hepatitis A/E, norovirus; Razafimahefa et al., 2020). Therefore, raw and undercooked shellfish can act as vectors of infectious pathogens which can pose a serious risk to human health (Flannery et al., 2014; Santos-Ferreira et al., 2020).
Bacterial species are traditionally used as indicators of fecal contamination of agricultural products, shellfish and shellfish waters (Garcia et al., 2020). This has led to the formulation of legislation based on the measurement of fecal indicator bacteria (EU, 2020). For example, within the European Union (EU), shellfish harvesting areas are classified according to the concentration of fecal indicator bacteria (Escherichia coli) as designated in Annex II of EU Regulation 854/2004 (EU, 2004). However, studies have indicated that monitoring fecal indicator bacteria in shellfish may be a poor indicator of water pollution and the risk of human exposure to pathogens from consuming shellfish (Gerba et al., 1979; Romalde et al., 2002; Younger et al., 2018). While methods for the detection and quantification of pathogens (e.g., Vibrio spp. viruses) in shellfish exist, these have yet to be incorporated into EU legislation due to the lack of a robust evidence base and disagreements over what new standards should be introduced (Lees and Cen WG6 TAG4, 2010; Hassard et al., 2017; EFSA, 2019).
If shellfish are shown to contain high levels of E. coli (>230 CFU 100 g–1 shellfish flesh) then they are deemed unfit for human consumption (Seafish, 2020). At this point, depuration or relaying to a new area is required to reduce contamination levels without further treatment or processing (McLeod et al., 2017a). Depuration is the process of controlled purification in land-based tanks where shellfish are cleansed using flow-through or recirculating water (Richards, 1988). Despite this controlled cleansing, “zero risk” to human health is rarely achievable with some pathogens often proving difficult to eliminate (Hassard et al., 2017; Amoroso et al., 2020). Typically, however, conventional depuration systems are effective at greatly reducing E. coli from shellfish to a standard that complies with current legislation requirements (Muniain-Mujika et al., 2002; Nappier et al., 2008; Love et al., 2010). However, it is unclear whether conventional systems are able to successfully depurate new and emerging pathogens and current legislation is inadequate in this respect, to protect public health. For example, shellfish have recently been implicated in outbreaks of norovirus and hepatitis A, despite undergoing depuration in compliance with EU standards (Diez-Valcarce et al., 2012; Maunula and von Bonsdorff, 2014).
The majority of research on the effectiveness of depuration has been conducted using oysters, as these are usually eaten raw. Less research is available on the effectiveness of depuration on mussels, particularly Mytilus edulis (common mussel), with regard to depuration of bacteria and viruses from mixed contamination sources. The first aim of this study was therefore to compare the accumulation, retention, and depuration of E. coli with pathogenic E. coli O157:H7 and norovirus in a controlled environment over a short time period. The short (24 h), time period was chosen to simulate acute contamination, as observed during combined sewer overflow (CSO) discharges, or other sewage overflow events. These events are known to occur on a frequent basis throughout the UK (Mounce et al., 2014). The second aim of this study was to demonstrate the effectiveness of current UK depuration conditions at the relative removal efficiency of E. coli and norovirus RNA in individual and mixed contamination conditions. Previous studies have largely focused on individual microbial indicators however, it is not known whether they interact in terms of bioaccumulation and subsequent elimination.
Materials and Methods
Cell Culture and Viral Stocks
A human gut isolate of E. coli was obtained from NCIMB, Aberdeen, United Kingdom (NCIMB 12413) as a lyophilised culture (Ni et al., 2016). A human isolate of E. coli serotype O157:H7 verocytotoxin-negative NCTC 12900 was obtained from NCTC, Porton Down, United Kingdom as a lyophilised culture (Bezanson et al., 2012). Lyophilised cultures were resuspended in 0.5 ml nutrient broth (Oxoid Ltd., Basingstoke, United Kingdom), sub-cultured twice onto nutrient agar (Oxoid Ltd.) to single colonies. Overnight broth cultures were diluted to an OD600 value of 0.5; 50 ml of the diluted cultures were centrifuged at 4,000 g for 10 min and resuspended in 1/4-strength Ringers solution (Oxoid Ltd.). Bacterial cultures were quantified by dilution series plating onto selective media and incubating for 24 h at 37°C prior to enumeration: E. coli/coliform harlequin media #008 (Lab M Ltd., Bury, United Kingdom; Pitkanen et al., 2007; Clements et al., 2013) for generic E. coli, CT-SMAC media (Oxoid Ltd) for E. coli O157:H7 (Jordan and Maher, 2006). Toxigenic E. coli O157 was chosen based on its prevalence in shellfish both in the United Kingdom and elsewhere (MacRae et al., 2005; Matulkova et al., 2013; Prakasan et al., 2018), although it should be noted that disease outbreaks directly linked to consumption of E. coli O157 contaminated shellfish are extremely rare. In addition, where they have been linked to outbreaks of gastrointestinal illnesses other pathogens have also been present in shellfish (co-contaminants; e.g., Vibrio parahaemolyticus, Vibrio albensis, Shigella flexneri) (CDC, 2019; CDPH, 2019).
Human-derived norovirus genogroup II (NoV GII.4/2012; Sydney-2012 like variant) positive fecal material was kindly provided by Prof. Ian Goodfellow (University of Cambridge, United Kingdom). This material was aliquoted and quantified using RT-qPCR prior to use in bioaccumulation experiments. The virus was not purified from the fecal material prior to use, however, the solid material was removed by centrifugation. The fecal material contained no detectable E. coli O157. Norovirus was chosen as numerous human disease outbreaks have been directly linked with consumption of norovirus contaminated shellfish (Fusco et al., 2017; Hassard et al., 2017).
Bioaccumulation in Shellfish
E. coli and E. coli O157:H7 were each inoculated to a final concentration of 5 × 106 CFU per 100 ml in 30 l tanks containing aerated natural seawater. These high contamination levels reflect periods when raw untreated sewage is released into UK coastal waters (Campos et al., 2011). Norovirus-positive fecal material was resuspended in a solution of 10% PBS (8 × 109 gc ml–1; gc, genome copies) and inoculated into separate identical 30 l tanks to a final concentration of 2.7 × 105 gc ml–1. E. coli, E. coli O157:H7 and norovirus-positive fecal material in the same quantities were added to a third set of identical tanks. These concentrations reflect high levels of contamination when the risk of bioaccumulation from exposure to raw sewage is greatest (e.g., during a large norovirus outbreak)(Katayama and Vinjé, 2017; Farkas et al., 2018). All tanks were contained in a climate-controlled chamber at 12°C to represent typical UK coastal water conditions (annual mean 12.4°C, min 7°C, max 17°C; Morris et al., 2018). Fresh seawater was obtained from the nearby Menai Straits (53°13′18.7″’ N, 4°9′36.6″’W) and had a salinity of 34‰. The pH was maintained at 7.5–8.0 by daily monitoring with a pH probe. NH4+, NO2– and NO3– were measured colorimetrically every 2 days according to Jones and Willett (2006) with values ranging from 0 to 0.2 mg l–1. The O2 content of the water was measured daily and shown to be fully saturated using a dissolved O2 probe (Hanna Instruments Ltd., Leighton Buzzard, United Kingdom). The room was maintained with a low light intensity to promote shellfish activity (Lee et al., 2008) and was conformed to a microbiological biosafety level 2 containment facility.
Mussels (Mytilus edulis; 4–5 cm shell length) were collected from a Class B shellfish bed in the Menai Strait by a local commercial fishing company. This site has a previous history of microbiological contamination of mussels linked to major foodborne disease outbreaks (Boxman et al., 2016). This is linked to the episodic release of untreated sewage into shellfish harvesting waters from the main wastewater treatment plant that serves the city of Bangor and surrounding region and also from network pumping stations. The release of untreated sewage is often highly episodic and leads to high spatial and temporal fluctuations in E. coli loadings in shellfish (Clements, 2013).
After immediate transfer to the laboratory, the detritus on the shell surface was removed (e.g., barnacles, tubeworm) and the shells briefly cleaned using sterilized seawater (Clements et al., 2013). Any non-viable mussels were discarded (i.e., damaged shells). The mussels (n = 20) were laid in a monolayer in each tank containing viral and bacterial contaminants. Replicate (n = 3) batches of mussels (n = 16 batch–1) were removed from individual tanks every 3 h over a 24 h period for bacterial and viral analysis. Control batches were also removed at 0 h to assess background contaminant levels.
Shellfish Depuration
After the 24 h accumulation period, the mussels in the remaining tanks were surface cleaned using a multi-agent disinfectant [1% (v/v) Virkon®; Thermo Fisher Scientific United Kingdom Ltd., Loughborough, United Kingdom; Hernandez et al., 2000] and placed into experimental depuration systems consisting of 50 l tanks containing fresh recirculating seawater (15 l min–1) equipped with UV disinfection treatment (Vecton V2 600; TMC Ltd., London, United Kingdom) in the recirculation line. The flow rate was above the minimum industry guidelines (2 complete changes h–1; FSS, 2009). The depth of the water above the mussels (8 cm) reflected recommendations for commercial indoor depuration facilities (FSS, 2009). As single layer of shells was used to maximize water circulation. The UV treatment (25 W) also reflects that in commercial depuration units (Sunnotel et al., 2007; FAO, 2008). Mussels were retained at low density (ca. 6 kg m–2) in these conditions for 5 days at 12°C (industry minimum temperature for depuration is 5°C with maximum shellfish densities of 50 kg m–2; FSS, 2009; McLeod et al., 2017b). Water parameters, including salinity, pH, NH4+, NO3–, NO2–, O2 and temperature were monitored throughout, and maintained as described above. Due to the low shellfish density, at no time in the experiment did any of the water quality parameters fall close to the recommended minimum standard levels (McLeod et al., 2017b). Although higher water temperatures may facilitate the depuration of microbial contaminants, these were not used as it does not reflect current practice within the region (McLeod et al., 2017b). Vibrations in the facility were kept to a minimum and no shells failed to open (Younger et al., 2020). Replicate (n = 9) batches of mussels (n = 16 batch–1) were removed at time 3, 6, 9, 12, 18, 24, 36, 48, and 72 h for bacterial and viral analysis.
Bacterial Enumeration
A sub-sample (n = 6) of the recovered mussels were surface sterilized (as described above), shucked and the tissues homogenized in a sterile blender with stainless steel blades for 60 s. PBS dilutions of homogenized pooled shellfish were assayed for E. coli and E. coli O157:H7 by dilution plating onto selective media as described previously. Microbial numbers were expressed as CFU per 100 g of shellfish flesh. At each accumulation time period, 50 ml water from each tank was retained for bacterial enumeration. Bacteria were enumerated by serial dilution on the same selective media as per shellfish. Bacteria were also enumerated from water at selected time points during depuration to confirm that shellfish were not re-exposed to contamination (data not presented).
DNA and RNA Recovery
Viral and bacterial RNA/DNA recovery from shellfish was carried out according to the ISO 15216-1 method with very minor modifications (ISO, 2017). Mussels (n = 10) were surface sterilized, shucked and the digestive tissues removed and pooled to obtain a final weight of 2 g digestive tissue per replicate sample. Remaining tissues and intravalvular fluid were pooled in the same way to obtain 2 g non-digestive tissue per replicate. Mengovirus vMC0 was used as an extraction control (Winterbourn et al., 2016). The positive controls were derived from homogenates prepared as per the samples but after addition of 1 Lenticule® disc of Norovirus Reference Material (Public Health England, London, United Kingdom). Tissues were macerated and homogenized 1:1 (w/v) in proteinase K solution (100 μg ml–1) to a paste-like consistency prior to shaking incubation (37°C, 300 rev min–1) for 1 h. Digested homogenates were then secondary incubated at 60°C for 15 min, prior to centrifugation (3,000 g, 5 min) and recovering the supernatant. Viral RNA and DNA was extracted from each homogenate using NucliSENS® extraction kits and a MiniMag apparatus (BioMérieux, Craponne, France) from a 500 μl sample volume according to the manufacturer’s protocol with process controls as performed in Lowther et al. (2012) and Winterbourn et al. (2016). RNA/DNA was eluted in RNase-free sterile water and stored at −80°C prior to analysis.
Viral RNA was recovered from water by filtration of 500 ml following the addition of 25 mM MgCl2 at pH 4–6 (Lukasik et al., 2000). Viruses were filtered onto 0.45 μm cellulose nitrate filters (Sartorius AG, Göttingen, Germany). RNA was recovered from filter membranes using the standard phenol-chloroform method described by Griffiths et al. (2000).
Real Time RT-PCR (RT-qPCR) and Real Time PCR (qPCR)
To evaluate E. coli accumulation in different parts of the mussel, a qPCR approach was used. Plate counting was not done on these samples as it was logistically impossible to achieve this within the intensive 3 h sampling periods (i.e., only total E. coli in the mussel tissue was evaluated using standard plate counting).
RT-qPCR for norovirus GI, norovirus GII and Mengovirus (extraction control) and qPCR for E. coli and E. coli O157:H7 was performed using a QuantStudio 6 thermocycler (Applied Biosystems, Foster City, CA, United States). For both E. coli strains, genesig primer/probe proprietary detection kits were utilized (PrimerDesign Ltd., Southampton, United Kingdom) with PrecisionPlus mastermix (PrimerDesign Ltd.) in a total volume of 15 μl, using 5 μl extracted DNA. For norovirus GI and GII, RNA Ultrasense qRT-PCR mix was used with a total volume of 25 μl, using 5 μl (10 ng) extracted RNA. Five hundred nanometer forward primers, IFRGI or IFRGII, were paired with 900 nM reverse primer, NEDG1 or COG2R, and 250 nM probe, TM9GI or IFRG2, as described in Lowther et al. (2012). Negative (water) and positive (positive material) qPCR controls were included in each qPCR/RT qPCR run. The presence of PCR inhibitors was tested using external process controls (mengovirus) or standard positive material for each of the tested pathogens (Lees and Cen WG6 TAG4, 2010; Winterbourn et al., 2016; Farkas et al., 2018). Quantification was estimated by standard curves constructed using serial dilutions of the appropriate RNA or DNA, plotting genome copy number against Cq values and expressed as genome copies per gram of tissue (gc g–1). The limit of quantification was 1 × 102 gc g–1 and the limit of detection was 40 gc g–1 (Winterbourn et al., 2016).
Statistical Analysis
Data were analyzed using PASW Statistics v18 (IBM Corp., Armonk, NY) and Minitab v18 (Minitab Inc., State College, PA). Normality was assessed using a one sample Kolmogorov–Smirnov test (P ≥ 0.05). Data which did not demonstrate normal distributions were log10 transformed. Temporal data were analyzed by two-way analysis of variance (ANOVA) and least significant difference (LSD) Post-hoc test using P < 0.05 as the cut-off for statistical significance.
Results
Accumulation of Pathogenic and Non-pathogenic E. coli in Mussels
Non-pathogenic E. coli accumulated in shellfish tissue from an initial mean concentration of 166 CFU to 1 × 105 CFU 100 g–1 within 3 h of exposure to water containing 5 × 106 CFU E. coli 100 ml–1 seawater (P = 0.030; Figure 1A). This increased further to 3 × 105 CFU 100 g–1 flesh after 6 h of exposure (P = 0.004). Non-pathogenic E. coli remained at this concentration in the mussel flesh, 1 log10 lower than the contamination of the seawater, until the end of the 24 h contamination exposure period. E. coli O157:H7 accumulated in the mussel flesh from an initial starting concentration of zero to approximately 106 CFU 100 g–1 flesh within the first 3 h when exposed to an initial E. coli O157:H7 concentration in the seawater of 5 × 106 CFU 100 ml–1 (P = 0.003; Figure 1A), increasing to 2 × 106 CFU 100 g–1 flesh after 6 h, similar to levels of E. coli O157:H7 in the surrounding water.
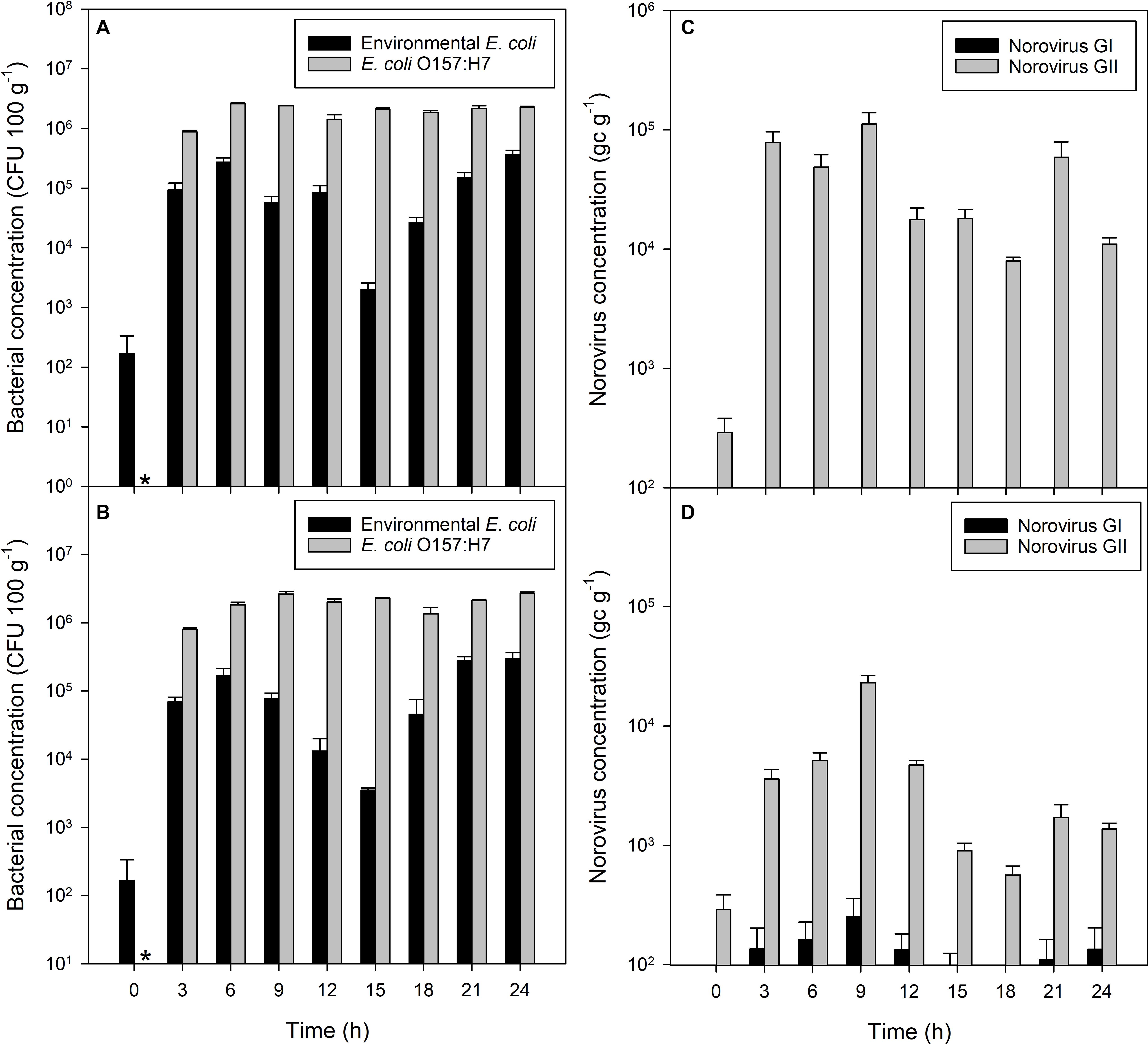
Figure 1. Accumulation of bacterial and viral contaminants in mussel flesh over 24 h of exposure to fecally contaminated seawater. Concentration in mussel flesh of (A) human gut-derived (non-pathogenic) E. coli and human-pathogenic E. coli O157:H7 in bacterial-only contaminated water, (B) human gut derived E. coli and E. coli O157:H7 in mussels exposed to mixed norovirus and E. coli contamination in the water, (C) norovirus GI and GII when exposed to viral-only contaminated water, and (D) norovirus GI and GII in mussels exposed to mixed norovirus and E. coli contaminated water. Values represent means ± SEM (n = 3). The asterisk indicates no E. coli O157:H7 in the mussels prior to contamination.
Higher concentrations of E. coli O157:H7 in mussel flesh were observed than those of non-pathogenic E. coli in the same mussels, despite similar initial seawater concentrations (P < 0.001; Figure 1A). The concentration of E. coli O157:H7 was observed to be approximately 1 log10 higher than E. coli at all time periods during the exposure period.
Bacterial contamination in the digestive tissue was compared to contamination in the general mussel tissue using qPCR (Figure 2A). Both non-pathogenic and pathogenic E. coli were measured in higher quantities in general (non-digestive) mussel flesh, compared to concentrations in the digestive tissue of the same mussel (both P < 0.001). The extent of this difference varied according to time since initial exposure (P < 0.001). After 3 h of exposure, non-digestive tissue contained 9 times more E. coli than digestive tissue, and 3.5 times more E. coli O157:H7 than the digestive tissue. Toward the end of the accumulation period, this ratio decreased. For example, after 18 h of exposure, non-digestive material contained twice the concentration of E. coli and E. coli O157:H7 than digestive tissue.
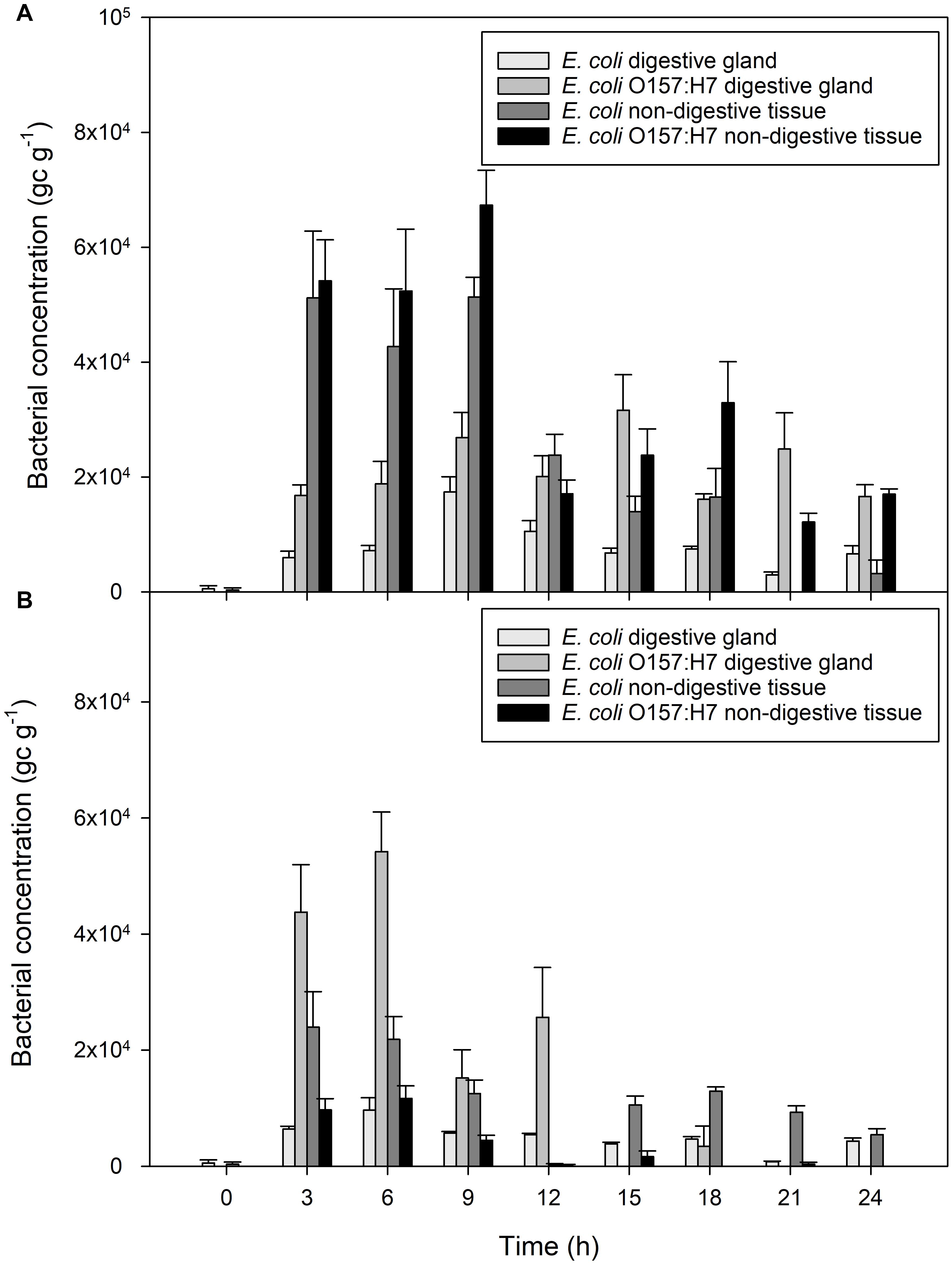
Figure 2. Accumulation of bacterial contaminants in different parts of the mussel over 24 h of exposure to fecally contaminated seawater (A) E. coli and E. coli O157:H7 in mussel digestive tissue or general mussel tissue when exposed to bacterial-only contaminated water, (B) E. coli and E. coli O157:H7 in digestive tissue and non-digestive material of mussels exposed to water containing a mixture of viral and bacterial contamination. Values represent means ± SEM (n = 9).
Endogenous norovirus strains GI and GII (i.e., that naturally present in the mussels before the experiments started) were measured in the mussels exposed only to the two E. coli cultures (i.e., no additional norovirus contamination). Overall, the initial concentrations were low (290 gc g–1) and no further norovirus GI or GII accumulated during the 24 h exposure period to the E. coli-only cultures (P = 0.975 and P = 0.136 for GI and GII, respectively).
Accumulation of Norovirus in Mussels From Viral-Only Contaminated Waters
Norovirus GII concentration in the mussel flesh reached 7.8 × 104 gc g–1 from an initial concentration of 290 gc g–1 within the first 3 h (P < 0.001; Figure 1C). A maximum concentration of norovirus GII of 1.1 × 105 gc g–1 was reached within 9 h. Levels of norovirus GI did not change during the experimental period (P = 0.574). E. coli and E. coli O157:H7 in mussels was monitored, but remained unchanged throughout the 24 h exposure period (data not shown).
Accumulation of E. coli and Norovirus From Co-contaminated Waters
When mussels were exposed to a mixed contamination of norovirus stool sample, non-pathogenic E. coli and E. coli O157:H7, uptake of pathogenic and non-pathogenic E. coli occurred rapidly (Figure 1B). The concentration of non-pathogenic E. coli in the mussel flesh increased from 170 CFU 100 g–1 to 7 × 104 CFU 100 g–1 flesh within the first 3 h (P < 0.003), with a final concentration of 3 × 105 CFU 100 g–1 flesh after 24 h of exposure (P < 0.008; Figure 1B). E. coli O157:H7 was undetected via colony counts at the start of the accumulation period, and increased to 8 × 105 CFU 100 g–1 flesh within the first 3 h, increasing further to a maximum 2.7 × 106 CFU 100 g–1 flesh after 9 h until the end of the 24 h contamination exposure period (Figure 1B). The initial concentration of pathogenic and non-pathogenic E. coli in seawater was 2 × 107 CFU 100 ml–1. At all time periods, the level of E. coli O157:H7 contamination in the mussel flesh was 1 log10 higher than the contamination of the same mussels by non-pathogenic E. coli (P < 0.001).
Mussel digestive tissue contained greater quantities of E. coli O157:H7 than non-digestive tissue from the same mussels over the course of the experiment (P < 0.001). After 3 h of exposure, digestive tissue contained an average of 4.4 × 104 gc g–1 E. coli O157:H7, compared to 9.7 × 103 gc g–1 in non-digestive tissue, a fourfold increase in the tissue compared to non-digestive material (Figure 2B). In contrast, non-digestive tissue contained greater quantities of non-pathogenic E. coli than digestive mussel tissue, during all periods of exposure (P < 0.001; Figure 2B).
Very little norovirus GI was detected in the mussels (117 ± 21 gc g–1) during the experiment and these levels did not change significantly over time (P = 0.329). Norovirus GII concentrations in mussel digestive tissue increased from 290 to 3.6 × 103 gc g–1 during the first 3 h of exposure (P = 0.012; Figure 1D). Contamination in the mussel digestive tissue varied between 2.3 × 105 and 5.2 × 102 gc g–1 over the 24 h exposure period, which is consistent with the level of contamination in the mussels exposed to norovirus only. A small difference in norovirus concentration between mussels exposed to virus only or mixed contamination was observed, with slightly less present in the mixed contamination (GI, P > 0.004; GII, P < 0.016). This level of reduction did not differ between genogroups (P = 0.143).
Depuration of Pathogenic and Non-pathogenic E. coli in Bacterial-Only Contamination
Eighty six percent of the non-pathogenic E. coli was depurated after 24 h. Following this, a slight reduction was also observed between 24 and 36 h. At the end of the 96 h depuration period, the reduction in non-pathogenic E. coli equated to a 87% decrease in concentration in comparison to that present at the start (P = 0.007). In comparison, 40% of the E. coli O157:H7 were depurated within 24 h, with a 48% reduction observed within 36 h. A 72% reduction in E. coli O157:H7 in mussel flesh was achieved in a 96 h period (P < 0.001; Figure 3A).
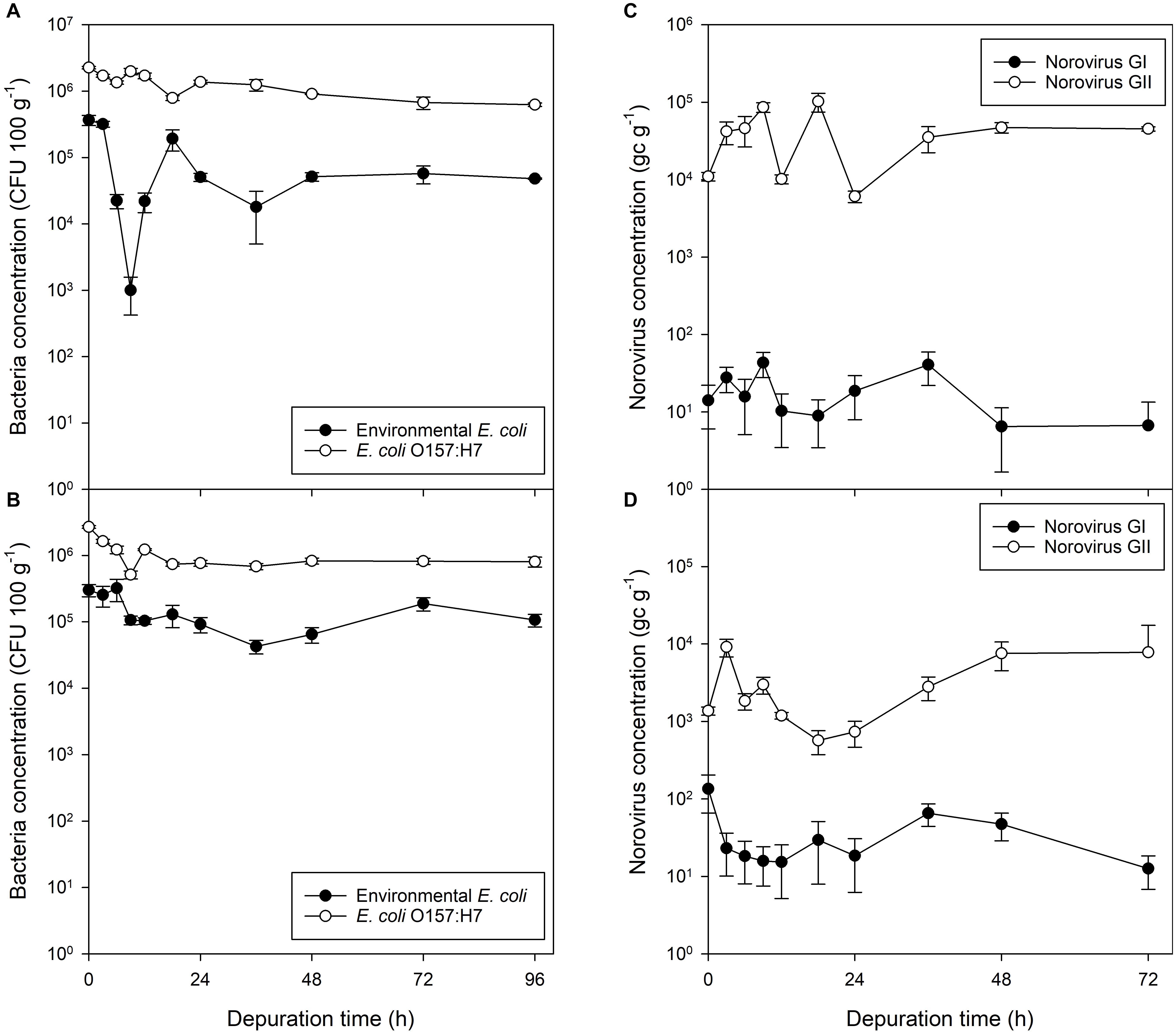
Figure 3. Bacterial and viral contaminants in mussel flesh over a 96 h depuration period after exposure to fecally contaminated seawater. Concentration in mussel flesh of (A) human gut-derived (non-pathogenic) E. coli and human-pathogenic E. coli O157:H7 previously exposed to bacterial-only contaminated water, (B) human gut-derived E. coli and E. coli O157:H7 in mussels exposed to mixed norovirus and E. coli contaminated water, (C) norovirus GI and GII when exposed to viral-only contaminated water, and (D) norovirus GI and GII in mussels previously exposed to water co-contaminated with norovirus and E. coli. Values represent means ± SEM (n = 3).
During depuration, non-digestive tissue contained a lower concentration of E. coli and E. coli O157:H7 than digestive tissue from the same mussels between the start of depuration and 24 h into the depuration process (P < 0.01; Figure 4A). After 48 h of depuration, the concentration of bacterial DNA for both non-pathogenic E. coli and pathogenic E. coli O157:H7 was roughly equivalent between digestive tissue and non-digestive tissue (P = 0.586 and P = 0.803, respectively).
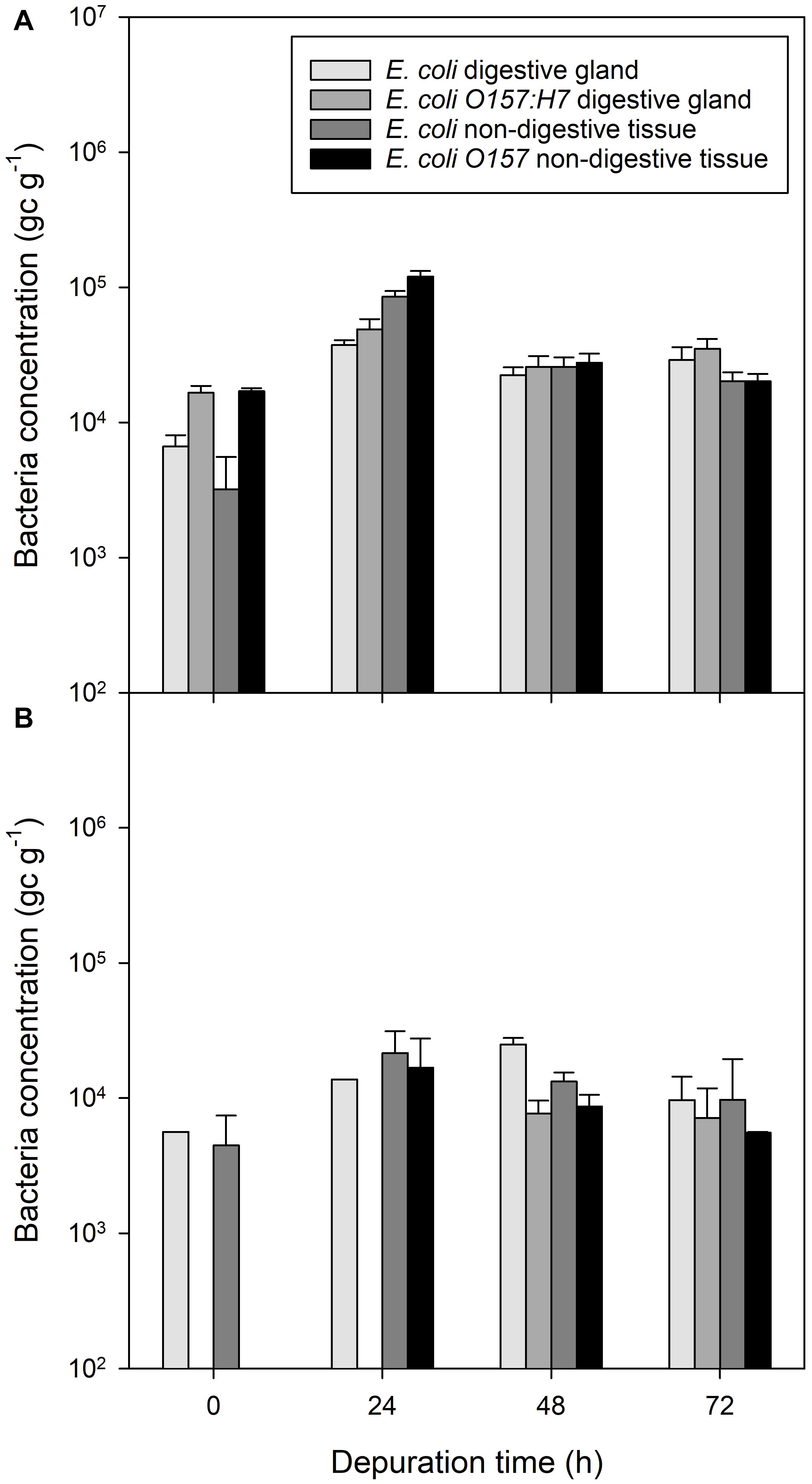
Figure 4. Presence of bacterial contaminants in different mussel tissues over a 96 h depuration period after exposure to fecally contaminated seawater. (A) E. coli and E. coli O157:H7 in mussel digestive tissue or general mussel tissue when previously exposed to bacterial-only contaminated water, (B) E. coli and E. coli O157:H7 in digestive tissue and non-digestive material of mussels previously exposed to water co-contaminated with norovirus and E. coli. Values represent means ± SEM (n = 9). The legend is the same for both panels.
Depuration of Norovirus From Mussels Experimentally Exposed to Norovirus-Only Contamination
Mussels experimentally exposed to norovirus contaminated material for 24 h demonstrated no decline in contamination of either norovirus GI or GII during the 72 h depuration period (Figure 3C). Norovirus GI contamination was at the limit of detection (average across all depuration samples, 19 ± 5 gc g–1), as the stool sample used during accumulation contained only norovirus GII. From the initial low level of norovirus GI contamination there was no change in concentration of GI in the mussel digestive tissue (P = 0.510). Norovirus GII in the digestive tissue continued to increase during the initial depuration period, for the first 18 h of depuration, to a concentration significantly higher than at the start of depuration (P = 0.030). During the 72 h depuration, the total observed decrease in norovirus GII from the start of depuration was 29%, however, this did not prove statistically significant (P = 0.363). The final norovirus concentration in the mussels was 4.5 × 104 gc g–1.
Depuration of Norovirus and E. coli From Mussels Co-contaminated With Faecally-Derived Bacteria and Viruses
Mussels exposed to mixed microbial contamination depurated 70% of their non-pathogenic E. coli during the first 24 h of depuration (P = 0.035), with little further loss seen after this time (Figure 3B). E. coli O157:H7 decreased by a similar proportion (71%; P < 0.001) over 24 h, but there was no subsequent decrease in E. coli O157:H7 between 24 and 96 h (P = 0.808; Figure 3B).
Mussels contaminated with a mixed culture of E. coli, E. coli O157:H7 and norovirus GII were again found to contain levels of norovirus GI (38 ± 12 gc g–1) which did not change during the 72 h depuration period (P = 0.152; Figure 3D). Similarly, the levels of norovirus GII did not change during the first 72 h of depuration (P = 0.087; Figure 3D). Norovirus GII was detected at similar concentrations at the start of depuration for all time points. The final norovirus concentration in the mussels was 7.8 × 103 gc g–1.
In mussels co-exposed to E. coli, E. coli O157:H7 and norovirus GII, the concentrations of DNA associated with E. coli in the digestive tissue were observed to be similar to E. coli concentration in the non-digestive tissue at the start of depuration (Figure 4B). Non-pathogenic E. coli in the non-digestive tissue increased for the first 24 h of depuration, but the ratio of E. coli in the digestive tissue to non-digestive tissue was lower than observed in the E. coli-only exposed mussels at a ratio of approximately 1:2.
Discussion
Accumulation of E. coli in Mussels
Human pathogenic E. coli O157:H7 accumulated to a higher concentration in mussel flesh compared to the non-pathogenic E. coli strain. Despite similar initial concentrations in the seawater within the experimental exposure system, mussels accumulated E. coli O157:H7 2–10 times more effectively than non-pathogenic E. coli in the same tank. This occurred in both mussels exposed only to E. coli, and mussels co-exposed to both E. coli and norovirus. Mussels were found to accumulate non-pathogenic E. coli to a lower final concentration than that found in the surrounding water, whilst E. coli O157:H7 accumulated to higher concentrations in the shellfish than in the surrounding water. This suggests active accumulation of E. coli O157:H7, which may be due to differential binding of E. coli O157:H7 in the digestive tissue of the mussels. This differential accumulation is also mirrored in Vibrio cholera and V. parahaemolyticus accumulation in mussels and oysters (Collin et al., 2012; Aagesen et al., 2018). This also has parallels with humans where E. coli O157:H7 is known to cause disease in humans via preferential binding to the epithelial cells in the gut due to its high abundance of adhesins and fimbriae (Torres et al., 2005; Vidal et al., 2007). Our result is also consistent with the findings that depuration has a major effect on the bacterial diversity within mussel tissues, preferentially retaining some bacteria at the expense of others (Rubiolo et al., 2018).
Both types of E. coli were found to accumulate in the shellfish within the first 3 h of exposure, in agreement with previous studies of the uptake of E. coli and Vibrio spp. by mussels and other shellfish (Ho and Tam, 2000; McGhee et al., 2008; Herrfurth et al., 2013). In contrast, some other studies have shown E. coli not to be bioaccumulated in shellfish (e.g., Crassostrea virginica) beyond the level of water contamination (Burkhardt and Calci, 2000). This concurs with Polo et al. (2014b) and Younger et al. (2020) who suggest that viral uptake and removal rates from one species cannot be readily translated to another. In the case of shellfish beds becoming exposed to E. coli, we conclude that contamination will affect the E. coli loadings in the shellfish within the first 3 h of any spillage, which is critical when considering the impact of CSO discharges on shellfish (Shibata et al., 2014). However, it should be noted that pulses of sewage contaminated water may take many days to disperse in coastal environments depending on the prevailing currents (Robins et al., 2019). Our data show that environmental E. coli will accumulate to a concentration lower than the contaminating seawater. However, as E. coli O157:H7 is accumulated to a level equivalent to the surrounding seawater, E. coli O157:H7 contamination into the water can represent a much greater risk to surrounding shellfish beds. We also acknowledge that we only used two strains of E. coli. It is known that the human gut microbiome may contain many strains of E. coli which will ultimately enter the environment via wastewater discharge (Richter et al., 2018) and thus potentially accumulate in mussels to different extents (Oliveira et al., 2020). This work could therefore be further extended to investigate the abundance of different E. coli strains present in the mussel microbiome after the discharge of sewage into the marine zone.
Norovirus Accumulation in Mussels
Similar to the fecal coliforms, norovirus GII was also found to accumulate in shellfish digestive tissue within the first 3 h of exposure, in agreement with previous studies demonstrating that norovirus accumulates in oysters within 4 h of exposure (Schwab et al., 1998). The findings from this study suggest that after an initial spike in norovirus GII, the concentration of GII in the digestive tissue decreases slightly over the subsequent 6–12 h. This is most likely due to progressive loss of viral integrity due to enzymatic action within the mussel’s digestive gland (Molloy et al., 2014; Kim et al., 2017).
E. coli Depuration From Mussels
In this study, some of the non-pathogenic E. coli were removed by depuration within the 42 h time period typically used for commercial depuration in the UK. From high contamination levels, E. coli levels were successfully depurated by ca. 90%. However, from these high contamination levels a 90% decrease still represents a bacterial contamination level significantly greater than required under EU legislation for end-point product testing (230 CFU E. coli 100 g–1) (EU, 2004; FSS, 2009). Similarly, the elimination of non-pathogenic E. coli from the mussel flesh during the standard depuration period was similarly poor. We note, however, that the levels of E. coli O157 are reflective of highly sewage polluted waters and the depuration kinetics at low contamination levels requires further work. The same argument follows for Norovirus below.
There are numerous reports of Shiga-toxin-producing Escherichia coli (STEC) contamination of shellfish (Bennani et al., 2011; Baliere et al., 2015), however, surprisingly these have yet to be linked to actual shellfish-borne food poisoning outbreaks. Non-pathogenic E. coli depurated slightly faster in comparison to E. coli O157:H7. In the event of E. coli O157:H7 contamination in shellfish, depuration is likely to remove the non-pathogenic E. coli disproportionately to the more hazardous E. coli O157:H7. End-product testing of shellfish for E. coli will also detect the presence of E. coli O157:H7. However, the infectious dose of E. coli O157 serotypes has been shown to be less than 70 CFU person–1 (Tuttle et al., 1999) and is estimated by Public Health England to be as low at 2 CFU person–1. In the event of mussels being environmentally exposed to E. coli O157 and then depurated, removal of total E. coli spp. to end-point levels of 230 CFU 100 g–1 could result in residual E. coli O157 contamination at dangerous levels in the shellfish, despite passing end-product testing based on current bacteriological criteria. Such a case has been reported in oysters in France (Guyon et al., 2000).
Norovirus Depuration From Mussels
At the initial stages of depuration, norovirus concentrations appeared to increase slightly in the mussel tissue relative to the initial level of concentration. It is likely that this is an artifact of the short accumulation period used. We suggest that this is caused by norovirus being present in the intravalvular fluid and non-digestive tissue material at the start of the depuration period. In the initial stages of depuration, norovirus particles continue to be processed by the mussels from the intravalvular fluid to the digestive tissue, where they bind. This increase in norovirus GII during early stages of depuration was observed in mussels experimentally contaminated with only norovirus and also in mussels exposed to mixed norovirus and E. coli contamination. Previous studies have shown that norovirus is not depurated effectively from oyster digestive tissues (Le Guyader et al., 2006; Ueki et al., 2007; Nappier et al., 2008), and that viruses are reduced at differential rates during depuration when compared with bacteria in mussel tissues (Power and Collins, 1989; De Mesquita et al., 1991). This study shows that over a 4 d depuration period, norovirus GII concentration remained similar to viral concentrations at the start of depuration. A recent study of norovirus depuration in mussels (Mytilus galloprovincialis) showed that low levels of norovirus GII (<103 gc g–1) was successfully removed over a 7 days depuration period (Polo et al., 2014a). Our results show that norovirus GII was not reduced during depuration, in contrast to Polo et al. (2014b), and that a significant concentration of norovirus still remained after 4 days. The study of Polo et al. (2014b) used similar depuration conditions and concentrations (initial levels of murine NoV in shellfish, 4 × 105 gc g–1) but used murine norovirus and Mytilus galloprovincialis which is different from our study. Considering the human infective NoV dose is ca. 10 intact virus particles (Hassard et al., 2017), then these levels may still represent a significant risk to human health. It should be noted, however, that a limitation of our study is that we did not measure infectivity directly. Caution is therefore required when interpreting the RT-qPCR RNA signal in an infectivity context (as a large proportion of the Norovirus particles may have been damaged and therefore not infective; Randazzo et al., 2017). In mussels exposed to mixed microbiological contamination, the period of time in the depuration phase where an increase in norovirus GII was observed was found to be shorter than in mussels exposed only to norovirus. Generally, lower levels of norovirus GII were measured in mussels also exposed to E. coli contamination potentially suggesting competition for binding sites in the digestive gland or that the presence of E. coli had stimulated the release of digestive enzymes. Similar evidence for this depression is also apparent in mammalian systems where bacteria have been shown to reduce norovirus infections, although the mechanisms involved still remain unclear (Hoang et al., 2015; Lee and Ko, 2017; Park et al., 2018).
Whilst the presence of norovirus in mussels appears to have no effect on the depuration efficiency of E. coli O157:H7, non-pathogenic E. coli was depurated less effectively in the presence of norovirus over the UK standard depuration time and the extended 96 h experimental depuration period. This contrasts with a previous study of E. coli in solo or mixed culture with Vibrio cholerae which demonstrated a higher effectiveness of E. coli depuration in M. galloprovincialis in the presence of V. cholerae (Marino et al., 2005). The reduction in efficiency of E. coli depuration in the presence of norovirus could be beneficial in measuring the effectiveness of depuration systems in which depurated shellfish are subject to end-product batch-testing. A reduction in the effectiveness of E. coli depuration as a result of norovirus contamination could result in a failure to reach the required end-point standards (<230 E. coli 100 g–1 shellfish flesh), rendering the product unmarketable.
Previous studies have found that E. coli in sewage contaminated shellfish is almost exclusively restricted to the digestive tract of the shellfish (Dore and Lees, 1995). In contrast, this study revealed that the proportion of E. coli O157:H7 DNA and environmentally derived E. coli found in digestive tissue to non-digestive tissues appear to vary according to the period of time for which the mussels have been exposed to the bacteria, or placed under depuration conditions. At the start of the exposure period, there appears to be a greater concentration of both E. coli strains in the non-digestive tissue than in the digestive tissue. It was hypothesized that this is due to the inclusion of intravalvular fluid in the non-digestive tissue. This includes the volume of water currently being processed by the mussel, which will contain bacteria in the same concentration as the surrounding seawater. The ratio of bacterial DNA in the digestive tissue to non-digestive tissue decreased over time. We ascribe this to high levels of potential bacteriolytic (lysozyme-like) activity in the intravalvular fluid (Allam and Paillard, 1998; Brousseau et al., 2018). However, in the presence of norovirus GII, the ratio of bacterial DNA in the digestive tissue to non-digestive tissue is reversed. In the absence of norovirus, there is initially more E. coli O157:H7 in the non-digestive tissue than in the digestive tissue. In the presence of norovirus, however, more of the E. coli O157:H7 is found in digestive tissue than non-digestive tissue. Norovirus is known to selectively bind to the digestive tissue (Le Guyader et al., 2006).
Conclusion and Practical Implications
E. coli abundance in shellfish still represents the primary way of assessing the microbiological quality of shellfish in the European Union. Our study, however, provides strong evidence to show that this approach is fundamentally flawed under current depuration operating conditions. Overall, we have shown under both controlled laboratory and field conditions in UK waters (Winterbourn et al., 2016) that the use of non-pathogenic E. coli as an end-product bacteriological criterion cannot reliably assess the general microbiological risk posed from consuming contaminated shellfish. Further, it cannot be assumed that contaminated shellfish will be rendered safe under standard depuration conditions. While E. coli may be a suitable proxy for some bacteria (Marino et al., 2005), we show that it fails to capture the risk posed by some human pathogenic bacteria and viruses such as E. coli O157:H7 and norovirus GII. This supports previous work on longitudinal or spatial surveys of viral pathogens in shellfish and those performed in other shellfish species at the point of sale (Romalde et al., 2002; Formiga-Cruz et al., 2003; Flannery et al., 2009; Lowther et al., 2018). Further, there are almost no documented cases of E. coli poisoning by shellfish suggesting that new testing standards need to be introduced which better reflect the risk of contracting food poisoning. These standards need to be run alongside routine testing for fecal contamination using for example, E. coli. Alternatively, depuration conditions need to be altered to promote increased depuration rates (e.g., temperature, salinity, feeding regime; McLeod et al., 2017a). Considering the numerous shellfish-borne viral infections in humans (e.g., norovirus, hepatitis A/E; Boxman et al., 2016), we propose that a parallel routine viral test should be introduced alongside routine E. coli testing. Clearly, there is a rationale for choosing norovirus given the numerous shellfish-related disease outbreaks, however, its seasonal nature makes it a poor general indicator of viral contamination (e.g., hepatitis A/E). We therefore propose that human-specific sewage-associated viruses (e.g., adenovirus, crAssphage; Farkas et al., 2018, 2019), may offer an alternative indicator that can be tested alongside norovirus and E. coli. In addition, this can be combined with the F-RNA phage infectivity assays or capsid integrity assays to estimate the likelihood of infective viruses being resent (Flannery et al., 2009; Lowther et al., 2019; Walker et al., 2019). This information is vital if we are to introduce robust quantitative microbial risk assessments (QMRA) and adaptive management practices for commercial shellfisheries.
Data Availability Statement
The raw data supporting the conclusions of this article will be made available by the authors, without undue reservation.
Author Contributions
SM, DJ, and JM designed the research. JS, KC, and MD conducted the research. JS, KC, and DJ collected and analyzed the data. JS and DJ wrote the initial drafts of the manuscript. All authors contributed to the final version of the manuscript.
Funding
This work was supported by the Dŵr Cymru/Welsh Water and James Wilson at Bangor Mussel Producers Ltd. who provided research samples used in the study. Funding was provided by the United Kingdom Natural Environment Research Council (NERC) and the Food Standards Agency (FSA) under the Environmental Microbiology and Human Health (EMHH) Programme (NE/M010996/1). The work was also funded by the European Fisheries Fund (EFF), Bangor Mussel Producers and Dŵr Cymru-Welsh Water under the Shellpath Project. The funders had no role in the study design, data collection and interpretation, or the decision to submit the work for publication. Bangor Mussel Producers provided shellfish samples for use in the experiments. Dŵr Cymru-Welsh Water provided advice on water quality issues pertinent to the experimental study area.
Conflict of Interest
The authors declare that the research was conducted in the absence of any commercial or financial relationships that could be construed as a potential conflict of interest.
References
Aagesen, A. M., Phuvasate, S., Su, Y. C., and Hase, C. C. (2018). Characterizing the adherence profiles of virulent Vibrio parahaemolyticus isolates. Microb. Ecol. 75, 152–162. doi: 10.1007/s00248-017-1025-8
Allam, B., and Paillard, C. (1998). Defense factors in clam extrapallial fluids. Dis. Aquatic Organ. 33, 123–128. doi: 10.3354/dao033123
Amoroso, M. G., Langellotti, A. L., Russo, V., Martello, A., Monini, M., Di Bartolo, I., et al. (2020). Accumulation and depuration kinetics of rotavirus in mussels experimentally contaminated. Food Environ. Virol. 12, 48–57. doi: 10.1007/s12560-019-09413-0
Baliere, C., Rince, A., Thevenot, D., and Gourmelon, M. (2015). Successful detection of pathogenic Shiga-toxin-producing Escherichia coli in shellfish, environmental waters and sediment using the ISO/TS-13136 method. Lett. Appl. Microbiol. 60, 315–320. doi: 10.1111/lam.12386
Bennani, M., Badri, S., Baibai, T., Oubrim, N., Hassar, M., Cohen, N., et al. (2011). First detection of shiga toxin-producing Escherichia coli in shellfish and coastal environments of Morocco. Appl. Biochem. Biotechnol. 165, 290–299. doi: 10.1007/s12010-011-9251-x
Bezanson, G., Delaquis, P., Bach, S., McKellar, R., Topp, E., Gill, A., et al. (2012). Comparative examination of Escherichia coli O157:H7 survival on romaine lettuce and in soil at two independent experimental sites. J. Food Prot. 75, 480–487. doi: 10.4315/0362-028X.JFP-11-306
Blanco, J. (2018). Accumulation of dinophysis toxins in bivalve molluscs. Toxins 10:453. doi: 10.3390/toxins10110453
Bosch, A., Pinto, R. M., and Abad, F. X. (1995). Differential accumulation and depuration of human enteric viruses by mussels. Water Sci. Technol. 31, 447–451. doi: 10.1016/0273-1223(95)00310-J
Bostock, J., Lane, A., Hough, C., and Yamamoto, K. (2016). An assessment of the economic contribution of EU aquaculture production and the influence of policies for its sustainable development. Aquac. Int. 24, 699–733. doi: 10.1007/s10499-016-9992-1
Boxman, I. L. A., Verhoef, L., Vennema, H., Ngui, S. L., Friesema, I. H. M., Whiteside, C., et al. (2016). International linkage of two food-borne hepatitis A clusters through traceback of mussels, the Netherlands, 2012. Euro Surveill. 21:30113. doi: 10.2807/1560-7917.ES.2016.21.3.30113
Brousseau, D. J., Braun, P. C., Harper-Leatherman, A. S., Duemmler, C., Warchol, M., Pacer, E. R., et al. (2018). Antimicrobial activity in the pallial cavity fluids of the ribbed mussel Geukensia demissa from a highly impacted harbor in Western Long Island Sound. J. Shellfish Res. 37, 997–1003. doi: 10.2983/035.037.0510
Burkhardt, W., and Calci, K. R. (2000). Selective accumulation may account for shellfish-associated viral illness. Appl. Environ. Microbiol. 66, 1375–1378. doi: 10.1128/AEM.66.4.1375-1378.2000
Campos, C. J. A., Reese, A., Kershaw, S., and Lee, R. J. (2011). Relationship Between the Microbial Quality of Shellfish Flesh and Seawater in UK Harvesting Areas. Project WT1001. Lowestoft: CEFAS.
CDC. (2019). Multistate Outbreak of Gastrointestinal Illnesses Linked to Oysters Imported from Mexico. Washington DC: Centers for Disease Control and Prevention.
CDPH. (2019). CDPH Warns Consumers not to Eat Raw Oysters from Baja California Sur, Mexico. Sacramento, CA: California Department of Public Health.
Clements, K. (2013). Bacterial Reservoirs in Shellfish and Shellfish Harvesting Waters. Bangor: School of Ocean Sciences, Bangor University. PhD Thesis.
Clements, K., Gimenez, L., Jones, D. L., Wilson, J., and Malham, S. K. (2013). Epizoic barnacles act as pathogen reservoirs on shellfish beds. J. Shellfish Res. 32, 533–538. doi: 10.2983/035.032.0233
Collin, B., Rehnstam-Holm, A. S., Lindmark, B., Pal, A., Wai, S. N., and Hernroth, B. (2012). The origin of vibrio cholerae influences uptake and persistence in the blue mussel Mytilus edulis. J. Shellfish Res. 31, 87–92. doi: 10.2983/035.031.0111
De Mesquita, M. M. F., Evison, L. M., and West, P. A. (1991). Removal of fecal indicator bacteria and bacteriophages from the common mussel Mytilus edulis under artificial depuration conditions. J. Appl. Bacteriol. 70, 495–501.
Diez-Valcarce, M., Kokkinos, P., Soderberg, K., Bouwknegt, M., Willems, K., de Roda-Husman, A. M., et al. (2012). Occurrence of human enteric viruses in commercial mussels at retail level in three European countries. Food Environ. Virol. 4, 73–80. doi: 10.1007/s12560-012-9078-9
Dore, W. J., and Lees, D. N. (1995). Behavior of Escherichia coli and male-specific bacteriophage in environmentally contaminated bivalve molluscs before and after depuration. Appl. Environ. Microbiol. 61, 2830–2834. doi: 10.1128/AEM.61.8.2830-2834.1995
EFSA. (2019). Scientific report on analysis of the European baseline survey of norovirus in oysters. EFSA J. 17:5762. doi: 10.2903/j.efsa.2019.5762
Enuneku, A., Omoruyi, O., Tongo, I., Ogbomida, E., Ogbeide, O., and Ezemonye, L. (2018). Evaluating the potential health risks of heavy metal pollution in sediment and selected benthic fauna of Benin River, Southern Nigeria. Appl. Water Sci. 8:224. doi: 10.1007/s13201-018-0873-9
EU. (2004). Regulation (EC) No 854/2004 of the European Parliament and of the Council of 29 April 2004 Laying Down Specific Rules for the Organisation of Official Controls on Products of Animal Origin Intended for Human Consumption, 854/2004. Brussels: European Union.
EU. (2020). Regulation (EU) 2020/741 of the European Parliament and of the Council of 25 May 2020 on Minimum Requirements for Water Reuse. Brussels: European Union.
FAO (2008). Bivalve Depuration: Fundamental and Practical Aspects. FAO Fisheries Technical Paper 511. Rome: Food and Agriculture Organization of the United Nations.
FAO. (2020). The State of World Fisheries and Aquaculture 2020. Rome: Food and Agriculture Organization of the United Nations.
Farkas, K., Adriaenssens, E. M., Walker, D. I., McDonald, J. E., Malham, S. K., and Jones, D. L. (2019). Critical evaluation of CrAssphage as a molecular marker for human-derived wastewater contamination in the aquatic environment. Food Environ. Virol. 11, 113–119. doi: 10.1007/s12560-019-09369-1
Farkas, K., Cooper, D. M., McDonald, J. E., Malham, S. K., de Rougemont, A., and Jones, D. L. (2018). Seasonal and spatial dynamics of enteric viruses in wastewater and in riverine and estuarine receiving waters. Sci. Total Environ. 634, 1174–1183. doi: 10.1016/j.scitotenv.2018.04.038
Flannery, J., Keaveney, S., and Doré, W. (2009). Use of FRNA bacteriophages to indicate the risk of norovirus contamination in Irish oysters. J. Food Prot. 72, 2358–2362.
Flannery, J., Rajko-Nenow, P., Winterbourn, J. B., Malham, S. K., and Jones, D. L. (2014). Effectiveness of cooking to reduce Norovirus and infectious F-specific RNA bacteriophage concentrations in Mytilus edulis. J. Appl. Microbiol. 117, 564–571. doi: 10.1111/jam.12534
Formiga-Cruz, M., Allard, A. K., Conden-Hansson, A. C., Henshilwood, K., Hernroth, B. E., Jofre, J., et al. (2003). Evaluation of potential indicators of viral contamination in shellfish and their applicability to diverse geographical areas. Appl. Environ. Microbiol. 69, 1556–1563. doi: 10.1128/AEM.69.3.1556-1563.2003
FSS (2009). Guidance for Inspection of Shellfish Purification Systems for Local Food Authorities. Aberdeen: Food Standards Scotland.
Fusco, G., Di Bartolo, I., Cioffi, B., Ianiro, G., Palermo, P., Monini, M., et al. (2017). Prevalence of foodborne viruses in mussels in Southern Italy. Food Environ. Virol. 9, 187–194. doi: 10.1007/s12560-016-9277-x
Garcia, A. V., de Sa, S. H. G., Silva, G. D., Ballesteros, J. E. M., Barbieri, E., de Sousa, R. L. M., et al. (2020). Microbiological quality of shellfish and evaluation of compact dry EC for detecting total coliforms and Escherichia coli. Acta Aliment. 49, 32–39. doi: 10.1556/066.2020.49.1.5
Gerba, C. P., Goyal, S. M., Labelle, R. L., Cech, I., and Bodgan, G. F. (1979). Failure of indicator bacteria to reflect the occurrence of enteroviruses in marine waters. Am. J. Public Health 69, 1116–1119. doi: 10.2105/AJPH.69.11.1116
Griffiths, R. I., Whiteley, A. S., O’Donnell, A. G., and Bailey, M. J. (2000). Rapid method for coextraction of DNA and RNA from natural environments for analysis of ribosomal DNA- and rRNA-based microbial community composition. Appl. Environ. Microbiol. 66, 5488–5491. doi: 10.1128/AEM.66.12.5488-5491.2000
Guyon, R., Dorey, F., Collobert, J. F., Foret, J., Goubert, C., Mariau, V., et al. (2000). Detection of Shiga toxin-producing Escherichia coli O157 in shellfish (Crassostrea gigas). Sci. Aliments 20, 457–465. doi: 10.3166/sda.20.457-466
Hassard, F., Sharp, J. H., Taft, H., LeVay, L., Harris, J. P., McDonald, J. E., et al. (2017). Critical review on the public health impact of Norovirus contamination in shellfish and the environment: A UK perspective. Food Environ. Virol. 9, 123–141. doi: 10.1007/s12560-017-9279-3
Hernandez, A., Martro, E., Matas, L., Martin, M., and Ausina, V. (2000). Assessment of in-vitro efficacy of 1% Virkon (R) against bacteria, fungi, viruses and spores by means of AFNOR guidelines. J. Hosp. Infect. 46, 203–209. doi: 10.1053/jhin.2000.0818
Herrfurth, D., Oeleker, K., Pund, R. P., Strauch, E., Schwartz, K., Kleer, J., et al. (2013). Uptake and localization of Vibrio cholerae, Vibrio parahaemolyticus, and Vibrio vulnificus in blue mussels (Mytilus edulis) of the Baltic Sea. J. Shellfish Res. 32, 855–859. doi: 10.2983/035.032.0329
Ho, B. S. W., and Tam, T. Y. (2000). Natural depuration of shellfish for human consumption: a note of caution. Water Res. 34, 1401–1406. doi: 10.1016/S0043-1354(99)00256-0
Hoang, P. M., Cho, S., Kim, K. E., Byun, S. J., Lee, T. K., and Lee, S. (2015). Development of Lactobacillus paracasei harboring nucleic acid-hydrolyzing 3D8 scFv as a preventive probiotic against murine norovirus infection. Appl. Microbiol. Biotechnol. 99, 2793–2803. doi: 10.1007/s00253-014-6257-7
ISO. (2017). Microbiology of the Food Chain – Horizontal Method for Determination of Hepatitis A Virus and Norovirus using Real-Time RT-PCR–Part 1: Method for Quantification. Method 15216-1:2017. Geneva: International Organization for Standardization.
Jones, D. L., and Willett, V. B. (2006). Experimental evaluation of methods to quantify dissolved organic nitrogen (DON) and dissolved organic carbon (DOC) in soil. Soil Biol. Biochem. 38, 991–999. doi: 10.1016/j.soilbio.2005.08.012
Jordan, K. N., and Maher, M. M. (2006). Sensitive detection of Escherichia coli O157:H7 by conventional plating techniques. J. Food Prot. 69, 689–692. doi: 10.4315/0362-028
Katayama, H., and Vinjé, J. (2017). “Norovirus and other Calicivirus,” in Global Water Pathogen Project, eds J. B. Rose and B. Jiménez-Cisneros (Paris: UNESCO), doi: 10.14321/waterpathogens.14X-69.3.689
Kim, K. I., Kim, Y. C., Kwon, W. J., and Jeong, H. D. (2017). Evaluation of blue mussel Mytilus edulis as vector for viral hemorrhagic septicemia virus (VHSV). Dis. Aquatic Organ. 126, 239–246. doi: 10.3354/dao03180
Le Guyader, F. S., Loisy, F., Atmar, R. L., Hutson, A. M., Estes, M. K., Ruvoen-Clouet, N., et al. (2006). Norwalk virus-specific binding to oyster digestive tissues. Emerg. Infect. Dis. 12, 931–936.
Lee, H., and Ko, G. (2017). New perspectives regarding the antiviral effect of vitamin A on norovirus using modulation of gut microbiota. Gut Microbes 8, 616–620. doi: 10.1080/19490976.2017.1353842
Lee, R., Lovatelli, A., and Ababouch, L. (2008). Bivalve Depuration: Fundamental and Practical Aspects. FAO Fisheries Technical Paper No. 511. Rome: Food and Agriculture Organization of the United Nations.
Lees, D., and Cen WG6 TAG4. (2010). International standardisation of a method for detection of human pathogenic viruses in molluscan shellfish. Food Environ. Virol. 2, 146–155. doi: 10.1007/s12560-010-9042-5
Love, D. C., Lovelace, G. L., and Sobsey, M. D. (2010). Removal of Escherichia coli, Enterococcus fecalis, coliphage MS2, poliovirus, and hepatitis A virus from oysters (Crassostrea virginica) and hard shell clams (Mercinaria mercinaria) by depuration. Int. J. Food Microbiol. 143, 211–217. doi: 10.1016/j.ijfoodmicro.2010.08.028
Lowther, J. A., Cross, L., Stapleton, T., Gustar, N. E., Walker, D. I., Sills, M., et al. (2019). Use of F-specific RNA bacteriophage to estimate infectious norovirus levels in oysters. Food Environ. Virol. 11, 247–258. doi: 10.1007/s12560-019-09383-3
Lowther, J. A., Gustar, N. E., Powell, A. L., Hartnell, R. E., and Lees, D. N. (2012). Two-year systematic study to assess norovirus contamination in oysters from commercial harvesting areas in the United Kingdom. Appl. Environ. Microbiol. 78, 5812–5817. doi: 10.1128/AEM.01046-12
Lowther, J. A., Gustar, N. E., Powell, A. L. O., Brien, S., and Lees, D. N. (2018). A one-year survey of norovirus in UK oysters collected at the point of sale. Food Environ. Virol. 10, 278–287. doi: 10.1007/s12560-018-9338-4
Lukasik, J., Scott, T. M., Andryshak, D., and Farrah, S. R. (2000). Influence of salts on virus adsorption to microporous filters. Appl. Environ. Microbiol. 66, 2914–2920. doi: 10.1128/AEM.66.7.2914-2920.2000
MacRae, M., Hamilton, C., Strachan, N. J. C., Wright, S., and Ogden, I. D. (2005). The detection of Cryptosporidium parvum and Escherichia coli O157 in UK bivalve shellfish. J. Microbiol. Methods 60, 395–401. doi: 10.1016/j.mimet.2004.10.017
Marino, A., Lombardo, L., Fiorentino, C., Orlandella, B., Monticelli, L., Nostro, A., et al. (2005). Uptake of Escherichia coli, Vibrio cholerae non-O1 and Enterococcus durans by, and depuration of mussels (Mytilus galloprovincialis). Int. J. Food Microbiol. 99, 281–286. doi: 10.1016/j.ijfoodmicro.2004.09.003
Matulkova, P., Gobin, M., Taylor, J., Oshin, F., O’Connor, K., and Oliver, I. (2013). Crab meat: a novel vehicle for E. coli O157 identified in an outbreak in South West England, August 2011. Epidemiol. Infect. 141, 2043–2050.
Maunula, L., and von Bonsdorff, C. H. (2014). Emerging and re-emerging enteric viruses causing multinational foodborne disease outbreaks. Future Virol. 9, 301–312. doi: 10.2217/fvl.13.128
McGhee, T. J., Morris, J. A. Jr., Noble, R. T., and Fowler, P. K. (2008). Comparative microbial dynamics in Crassostrea virginica (Gmelin 1791) and Crassostrea ariakensis (Fujita 1913). J. Shellfish Res. 27, 559–565.
McLeod, C., Polo, D., Le Saux, J. C., and Le Guyader, F. S. (2017b). Evaluating the Effectiveness of Depuration in Removing Norovirus from Oysters. Report FS101068. London: Food Standards Agency.
McLeod, C., Polo, D., Le Saux, J. C., and Le Guyader, F. S. (2017a). Depuration and relaying: a review on potential removal of Norovirus from oysters. Compr. Rev. Food Sci. Food Safety 16, 692–706. doi: 10.1111/1541-4337.12271
Mohebbi-Nozar, S. L., Ismail, W. R., Zakaria, M. P., Mortazavi, M. S., Zahed, M. A., and Jahanlu, A. (2014). Health Risk of PCBs and DDTs in Seafood from Southern Iran. Hum. Ecol. Risk Assess. 20, 1164–1176. doi: 10.1080/10807039.2013.838121
Molloy, S. D., Pietrak, M. R., Bouchard, D. A., and Bricknell, I. (2014). The interaction of infectious salmon anaemia virus (ISAV) with the blue mussel, Mytilus edulis. Aquac. Res. 45, 509–518. doi: 10.1111/j.1365-2109.2012.03254.x
Morris, D. J., Pinnegar, J. K., Maxwell, D. L., Dye, S. R., Fernand, L. J., Flatman, S., et al. (2018). Over 10 million seawater temperature records for the United Kingdom Continental Shelf between 1880 and 2014 from 17 Cefas (United Kingdom government) marine data systems. Earth Syst. Sci. Data 10, 27–51. doi: 10.5194/essd-10-27-2018
Mounce, S. R., Shepherd, W., Sailor, G., Shucksmith, J., and Saul, A. J. (2014). Predicting combined sewer overflows chamber depth using artificial neural networks with rainfall radar data. Water Sci. Technol. 69, 1326–1333. doi: 10.2166/wst.2014.024
Muniain-Mujika, I., Girones, R., Tofino-Quesada, G., Calvo, M., and Lucena, F. (2002). Depuration dynamics of viruses in shellfish. Int. J. Food Microbiol. 77, 125–133. doi: 10.1016/S0168-1605(02)00052-1
Nappier, S. P., Graczyk, T. K., and Schwab, K. J. (2008). Bioaccumulation, retention, and depuration of enteric viruses by Crassostrea virginica and Crassostrea ariakensis oysters. Appl. Environ. Microbiol. 74, 6825–6831. doi: 10.1128/AEM.01000-08
Ni, Y., Lynch, M. J., Modic, M., Whalley, R. D., and Walsh, J. L. (2016). A solar powered handheld plasma source for microbial decontamination applications. J. Phys. D Appl. Physics 49:355203. doi: 10.1088/0022-3727/49/35/355203
Oehlenschlager, J. (2012). Seafood: nutritional benefits and risk aspects. Int. J. Vitamin Nutr. Res. 82, 168–176. doi: 10.1024/0300-9831/a000108
Oliveira, A. M. S., Barauna, R. A., Marcon, D. J., Lago, L. A. B., Silva, A., Lusio, J., et al. (2020). Occurrence, antibiotic-resistance and virulence of E. coli strains isolated from mangrove oysters (Crassostrea gasar) farmed in estuaries of Amazonia. Mar. Pollut. Bull. 157:111302. doi: 10.1016/j.marpolbul.2020.111302
Park, J. H., Lee, J. W., Choi, H., Jung, S. K., Kim, J. S., Kim, K. W., et al. (2018). Survival of Escherichia coil harboring nucleic acid-hydrolyzing 3D8 scFv during RNA virus infection. Regul. Toxicol. Pharmacol. 94, 286–292. doi: 10.1016/j.yrtph.2018.02.012
Pitkanen, T., Paakkari, P., Miettinen, I. T., Heinonen-Tanski, H., Paulin, L., and Hanninen, M. L. (2007). Comparison of media for enumeration of coliform bacteria and Escherichia coli in non-disinfected water. J. Microbiol. Methods 68, 522–529. doi: 10.1016/j.mimet.2006.10.007
Polo, D., Alvarez, C., Diez, J., Darriba, S., Longa, A., and Romalde, J. L. (2014a). Viral elimination during commercial depuration of shellfish. Food Control 43, 206–212. doi: 10.1016/j.foodcont.2014.03.022
Polo, D., Feal, X., Varela, M. F., Monteagudo, A., and Romalde, J. L. (2014b). Depuration kinetics of murine norovirus in shellfish. Food Res. Int. 64, 182–187. doi: 10.1016/j.foodres.2014.06.027
Power, U. F., and Collins, J. K. (1989). Differential depuration of poliovirus, Escherichia-coli, and a coliphage by the common mussel, Mytilus-edulis. Appl. Environ. Microbiol. 55, 1386–1390.
Prakasan, S., Prabhakar, P., Lekshmi, M., Nayak, B. B., and Kumar, S. (2018). Isolation of Shiga toxin-producing Escherichia coli harboring variant Shiga toxin genes from seafood. Veterinary World 11, 379–385. doi: 10.14202/vetworld.2018.379-385
Randazzo, W., Piqueras, J., Rodríguez-Díaz, J., Aznar, R., and Sánchez, G. (2017). Improving efficiency of viability-qPCR for selective detection of infectious HAV in food and water samples. J. Appl. Microbiol. 124, 958–964. doi: 10.1111/jam.13519
Razafimahefa, R. M., Ludwig-Begall, L. F., and Thiry, E. (2020). Cockles and mussels, alive, alive, oh: the role of bivalve molluscs as transmission vehicles for human norovirus infections. Transbound. Emerg. Dis. 67, 9–25. doi: 10.1111/tbed.13165
Richards, G. P. (1988). Microbial purification of shellfish - a review of depuration and relaying. J. Food Prot. 51, 218–251. doi: 10.4315/0362-028X-51.3.218
Richter, T. K. S., Michalski, J. M., Zanetti, L., Tennant, S. M., Chen, W. H., and Rasko, D. A. (2018). Responses of the human gut Escherichia coli population to pathogen and antibiotic disturbances. mSystems 3:e00047-18. doi: 10.1128/mSystems.00047-18
Robins, P. E., Farkas, K., Cooper, D., Malham, S. K., and Jones, D. L. (2019). Viral dispersal in the coastal zone: a method to quantify water quality risk. Environ. Int. 126, 430–442. doi: 10.1016/j.envint.2019.02.042
Romalde, J. L., Area, E., Sánchez, G., Ribao, C., Torrado, I., Abad, X., et al. (2002). Prevalence of enterovirus and hepatitis A virus in bivalve molluscs from Galicia (NW Spain): inadequacy of the EU standards of microbiological quality. Int. J. Food Microbiol. 74, 119–130. doi: 10.1016/s0168-1605(01)00744-9
Rubiolo, J. A., Lozano-Leon, A., Rodriguez-Souto, R., Rodriguez, N. F., Vieytes, M. R., and Botana, L. M. (2018). The impact of depuration on mussel hepatopancreas bacteriome composition and predicted metagenome. Antonie Van Leeuwenhoek Int. J. Gen. Mol. Microbiol. 111, 1117–1129. doi: 10.1007/s10482-018-1015-y
Santos-Ferreira, N., Mesquita, J. R., and Rivadulla, E. (2020). Norovirus contamination of sea urchins (Paracentrotus lividus): Potential food risk for consumers. Food Control 111:107041. doi: 10.1016/j.foodcont.2019.107041
Schwab, K. J., Neill, F. H., Estes, M. K., Metcalf, T. G., and Atmar, R. L. (1998). Distribution of norwalk virus within shellfish following bioaccumulation and subsequent depuration by detection using RT-PCR. J. Food Prot. 61, 1674–1680. doi: 10.4315/0362-028X-61.12.1674
Shibata, T., Kojima, K., Lee, S. A., and Furumai, H. (2014). Model evaluation of faecal contamination in coastal areas affected by urban rivers receiving combined sewer overflows. Water Sci. Technol. 70, 430–436. doi: 10.2166/wst.2014.225
Silver, J. (2014). From fishing to farming: shellfish aquaculture expansion and the complexities of ocean space on Canada’s west coast. Appl. Geogr. 54, 110–117. doi: 10.1016/j.apgeog.2014.07.013
Sunnotel, O., Snelling, W. J., McDonough, N., Browne, L., Moore, J. E., Dooley, J. S. G., et al. (2007). Effectiveness of standard UV depuration at inactivating Cryptosporidium parvum recovered from spiked pacific oysters (Crassostrea gigas). Appl. Environ. Microbiol. 73, 5083–5087. doi: 10.1128/AEM.00375-07
Torres, A. G., Zhou, X., and Kaper, J. B. (2005). Adherence of diarrheagenic Escherichia coli strains to epithelial cells. Infect. Immun. 73, 18–29. doi: 10.1128/IAI.73.1.18-29.2005
Tuttle, J., Gomez, T., Doyle, M. P., Wells, J. G., Zhao, T., Tauxe, R. V., et al. (1999). Lessons from a large outbreak of Escherichia coli O157:H7 infections: insights into the infectious dose and method of widespread contamination of hamburger patties. Epidemiol. Infect. 122, 185–192. doi: 10.1017/S0950268898001976
Ueki, Y., Shoji, M., Suto, A., Tanabe, T., Okimura, Y., Kikuchi, Y., et al. (2007). Persistence of caliciviruses in artificially contaminated oysters during depuration. Appl. Environ. Microbiol. 73, 5698–5701. doi: 10.1128/AEM.00290-07
Vidal, M., Escobar, P., Prado, V., Hormazabal, J. C., and Vidal, R. (2007). Distribution of putative adhesins in Shiga toxin-producing Escherichia coli (STEC) strains isolated from different sources in Chile. Epidemiol. Infect. 135, 688–694. doi: 10.1017/S0950268806007102
Walker, D. I., Cross, L. J., Stapleton, T. A., Jenkins, C. L., Lees, D. N., and Lowther, J. A. (2019). Assessment of the applicability of capsid-integrity assays for detecting infectious norovirus inactivated by heat or UV irradiation. Food Environ. Virol. 11, 229–237. doi: 10.1007/s12560-019-09390-4
Wijsman, J. W. M., Troost, K., Fang, J., and Roncarati, A. (2019). “Global production of marine bivalves. Trends and challenges,” in Goods and Services of Marine Bivalves, eds A. Smaal, J. Ferreira, J. Grant, J. Petersen, and Ø Strand (Cham: Springer), doi: 10.1007/978-3-319-96776-9_2
Winterbourn, J. B., Clements, K., Lowther, J. A., Malham, S. K., McDonald, J. E., and Jones, D. L. (2016). Use of Mytilus edulis biosentinels to investigate spatial patterns of norovirus and faecal indicator organism contamination around coastal sewage discharges. Water Res. 105, 241–250. doi: 10.1016/j.watres.2016.09.002
Younger, A. D., Alves, M. T., Taylor, N. G. H., Lowther, J., Baker-Austin, C., Campos, C. J. A., et al. (2018). Evaluation of the protection against norovirus afforded by E. coli monitoring of shellfish production areas under EU regulations. Water Sci. Technol. 78, 1010–1022. doi: 10.2166/wst.2018.357
Keywords: shellfish handling, mussel (Mytilus edulis), food safety, microbiological standard, risk assessment, STEC, norovirus
Citation: Sharp JH, Clements K, Diggens M, McDonald JE, Malham SK and Jones DL (2021) E. coli Is a Poor End-Product Criterion for Assessing the General Microbial Risk Posed From Consuming Norovirus Contaminated Shellfish. Front. Microbiol. 12:608888. doi: 10.3389/fmicb.2021.608888
Received: 21 September 2020; Accepted: 18 January 2021;
Published: 19 February 2021.
Edited by:
Gloria Sánchez Moragas, Institute of Agrochemistry and Food Technology (IATA), SpainReviewed by:
Leena Maunula, University of Helsinki, FinlandWalter Randazzo, University of Valencia, Spain
Copyright © 2021 Sharp, Clements, Diggens, McDonald, Malham and Jones. This is an open-access article distributed under the terms of the Creative Commons Attribution License (CC BY). The use, distribution or reproduction in other forums is permitted, provided the original author(s) and the copyright owner(s) are credited and that the original publication in this journal is cited, in accordance with accepted academic practice. No use, distribution or reproduction is permitted which does not comply with these terms.
*Correspondence: Davey L. Jones, ZC5qb25lc0BiYW5nb3IuYWMudWs=