- Nordcee, Department of Biology, University of Southern Denmark, Odense, Denmark
Diverse physiological groups congregate into environmental corrosive biofilms, yet the interspecies interactions between these corrosive physiological groups are seldom examined. We, therefore, explored Fe0-dependent cross-group interactions between acetogens and methanogens from lake sediments. On Fe0, acetogens were more corrosive and metabolically active when decoupled from methanogens, whereas methanogens were more metabolically active when coupled with acetogens. This suggests an opportunistic (win–loss) interaction on Fe0 between acetogens (loss) and methanogens (win). Clostridia and Methanobacterium were the major candidates doing acetogenesis and methanogenesis after four transfers (metagenome sequencing) and the only groups detected after 11 transfers (amplicon sequencing) on Fe0. Since abiotic H2 failed to explain the high metabolic rates on Fe0, we examined whether cell exudates (spent media filtrate) promoted the H2-evolving reaction on Fe0 above abiotic controls. Undeniably, spent media filtrate generated three- to four-fold more H2 than abiotic controls, which could be partly explained by thermolabile enzymes and partly by non-thermolabile constituents released by cells. Next, we examined the metagenome for candidate enzymes/shuttles that could catalyze H2 evolution from Fe0 and found candidate H2-evolving hydrogenases and an almost complete pathway for flavin biosynthesis in Clostridium. Clostridial ferredoxin-dependent [FeFe]-hydrogenases may be catalyzing the H2-evolving reaction on Fe0, explaining the significant H2 evolved by spent media exposed to Fe0. It is typical of Clostridia to secrete enzymes and other small molecules for lytic purposes. Here, they may secrete such molecules to enhance their own electron uptake from extracellular electron donors but indirectly make their H2-consuming neighbors—Methanobacterium—fare five times better in their presence. The particular enzymes and constituents promoting H2 evolution from Fe0 remain to be determined. However, we postulate that in a static environment like corrosive crust biofilms in lake sediments, less corrosive methanogens like Methanobacterium could extend corrosion long after acetogenesis ceased, by exploiting the constituents secreted by acetogens.
Introduction
Steel infrastructure extends for billions of kilometers below ground enabling not only transport and storage of clean water, chemicals, fuels, and sewage, but also protection for telecommunication and electricity cables. Climate change has led to extreme weather conditions like severe storms and rainfall. Urban storm and rainfall management in many countries, especially northern countries like Denmark, involves so-called climate lakes (also known as stormwater ponds or retention ponds) harvesting rainfall at a large scale, thus alleviating stormwater runoff in the cities (Mishra et al., 2020). If the stormwater runoff is improperly detained, underground steel infrastructure could suffer tremendous damage. Damages induced by microbial-induced corrosion (MIC) underground are often discovered too late, leading to environmental and economic devastation. Thus, it is important to predict the lifespan of the material if exposed to microbial communities native to the site where steel structures are located. This would lead to effective replacement and metal recuperation strategies before accidental spills that may be detrimental to the surrounding environment (Usher et al., 2014a; Skovhus et al., 2017; Arriba-Rodriguez et al., 2018).
In this study, we investigate corrosion by microorganisms from the anoxic sediments of a danish climate lake. In such anoxic environments where non-sulfidic conditions prevail, steel was expected to persist unharmed for centuries (Usher et al., 2014a; Skovhus et al., 2017; Arriba-Rodriguez et al., 2018), and yet, researchers showed that certain groups of anaerobes (methanogens and acetogens) strip electrons off the Fe0 surface leading to MIC (Zhang et al., 2003; Mori et al., 2010; Mand et al., 2014, 2016; Kato et al., 2015). Previous studies showed that MIC in non-sulfidic environments is often linked to the presence of acetogens like Clostridium and methanogens like Methanobacterium or Methanosarcinales on the surface of the corroded steel structure (Zhang et al., 2003; Zhu et al., 2003; Mori et al., 2010; Mand et al., 2014, 2016; Kato et al., 2015). It was suggested that Methanosarcinales were indirectly involved in corrosion, growing in a mutualistic relationship with the acetogens (Zhang et al., 2003; Mand et al., 2016). This assumption was based on acetogens producing acetate, which acetotrophic Methanosarcinales methanogens would then consume. In this case, acetogens were expected to be favored by the removal of their metabolic product—acetate. Such a mutualistic association on Fe0 between acetogens and methanogens has not yet been demonstrated experimentally. In contrast, we recently showed that instead of acting cooperatively on Fe0, acetogens and methanogens competed for Fe0 electrons (Palacios et al., 2019). However, the study by Palacios et al. enriched a corrosive community from a coastal marine environment. In this study, we embarked to understand whether similar interactions apply to corrosive communities enriched from an inland climate lake.
Interspecies interactions on Fe0 are scantily examined; here, we investigated the interplay between acetogens (reaction 1) and methanogens (reaction 2) from a climate lake, provided solely with Fe0 as their electron donor.
Theoretically, under standard thermodynamic conditions, methanogens should have an advantage over acetogens when provided with Fe0 as the sole electron donor (Reactions 1 and 2) especially since methanogens, unlike acetogens, are more effective at retrieving abiotic H2 (formed on Fe0) due to their low H2-uptake thresholds (Kotsyurbenko et al., 2001; Drake et al., 2002). Several groups of methanogens could corrode Fe0 independently of acetogenic bacteria, including species of Methanosarcina (Daniels et al., 1987; Belay and Daniels, 1990; Boopathy and Daniels, 1991), Methanobacterium (Belay and Daniels, 1990; Lorowitz et al., 1992; Dinh et al., 2004), and Methanococcus (Mori et al., 2010; Uchiyama et al., 2010; Lienemann et al., 2018; Tsurumaru et al., 2018). The mechanism by which methanogens corrode Fe0 has been debated. Some reports suggest that methanogens retrieve H2 chemically produced at the Fe0 surface (abiotic-H2) (Daniels et al., 1987). Others suggest that methanogens retrieve electrons directly using an unknown electron-uptake mechanism (Dinh et al., 2004; Beese-Vasbender et al., 2015). While others showed that certain methanogens produce extracellular enzymes, which catalyze H2 evolution at the Fe0 surface (Deutzmann et al., 2015; Tsurumaru et al., 2018). The latter mechanism was especially relevant for Methanococcus species, which harbored an unstable genomic island encoding [NiFe]-hydrogenases and the heterodisulfide reductase super complex involved in formate generation (Lienemann et al., 2018; Tsurumaru et al., 2018).
Acetogens often dominate corrosive communities, outcompeting methanogens when concentrations of H2 are high and temperatures are low, presumably due to the higher kinetics (Vmax) of their hydrogenases (Kotsyurbenko et al., 2001). Unlike methanogens, acetogens contain [FeFe]-hydrogenases (Peters et al., 2015), which could retrieve electrons directly from Fe0 for proton reduction to H2 (Mehanna et al., 2008, 2016; Rouvre and Basseguy, 2016). In this study, we were interested to understand the interspecies dynamics on Fe0 between acetogens and methanogens from a climate lake. In the absence of any other terminal electron acceptor but CO2, acetogens and methanogens are assumed to be either competing for food (Fe0 electrons) or cooperating as outlined below:
1. CO2-reducing methanogens (CO2 + 8e– + 8H+ → CH4 + 2H2O/CO2-reducing methanogenesis) may compete (loss/loss) with CO2-reducing acetogens (2CO2 + 8e– + 8H+ → CH3COOH + 2H2O/acetogenesis) for Fe0 electrons.
2. CO2-reducing acetogens (see acetogenesis reaction above) cooperate syntrophically (win/win) with acetoclastic methanogens (CH3COOH → CH4 + CO2/acetoclastic methanogenesis (Zhang et al., 2003; Mand et al., 2016). This type of interaction on Fe0 has never been demonstrated experimentally. Conversely, we recently showed that coastal marine Methanosarcina competed (loss/loss) with acetogens for Fe0 electrons (Palacios et al., 2019).
Here, we used a combination of physiological experiments, inhibition strategies, and molecular analyses to study the acetogens and methanogens from climate lake sediments and disentangle their interactions during Fe0 corrosion. In Fe0 enrichments from climate lake sediments, we witnessed a new type of interaction (a loss–win interaction) between acetogens and methanogens. Furthermore, physiology experiments combined with metagenomics teased apart the role of acetogens and methanogens in Fe0 corrosion and revealed a significant effect of exuded enzymes in promoting H2 evolution at the Fe0 surface.
Materials and Methods
Sample Collection and Enrichment Culture Conditions
Sediment cores were sampled during July 2016 from a small climate lake near a construction site on the University of Southern Denmark Campus, Odense. The salinity of the lake was 0.6 PSU, and gas bubbles (including methane) were continuously released to the water surface while sampling. Sediment cores were sliced in the laboratory. The deeper depth horizon 15–20 cm was used for downstream enrichments. To prepare the original slurries, we used 10 ml of sediment (added with a cutoff syringe) into 50 ml of freshwater media containing 5 g of Fe0 granules. An Fe0-free control was run alongside. The freshwater media was a modified DSM 120 media (DSMZ, 2014) (modifications: 0.6 g/L NaCl, without casitone, without sodium acetate, without methanol, and without Na2S × 9H2O). The enrichment cultures were prepared in 50 ml of blue butyl-rubber-stoppered glass vials with an anoxic headspace of a CO2:N2 gas mix (20:80, v/v). To ensure autotrophic Fe0-oxidizing microorganisms became enriched, we incubated strictly with Fe0 as the electron donor (for more than 3 years and 11 consecutive transfers). Iron was provided as granules: 1 g/10 ml culture (99.98% Thermo Fisher, Germany) or iron coupons (3 cm × 1 cm × 1 mm).
To examine the stability of the enrichments, methane production was monitored during the first five transfers, each time over the course of 3 months or longer (Supplementary Figure S1). Cultures were stable and produced ca. 3 mM methane per gram of Fe0, in all monitored transfers, except for the original slurry, which produced more methane likely due to carry-over organics from the sediment.
When methane production stopped (stationary phase), the enrichments were transferred to fresh media with fresh Fe0 granules. Additionally, we monitored methanogen-specific coenzyme F420 auto-fluorescence via routine microscopy to confirm the presence of methanogens.
All incubations were performed in triplicate (unless otherwise stated), at room temperature (20°C) in the dark, and without shaking.
Most downstream experiments (DNA extractions, SEM, inhibition experiments, and end metabolite determinations) were performed at the fourth transfer on Fe0 (see Figure 1 of our experimental plan). Shuttle experiments were carried out at the 7th transfer on Fe0 and amplicon sequencing at the 11th transfer on Fe0.
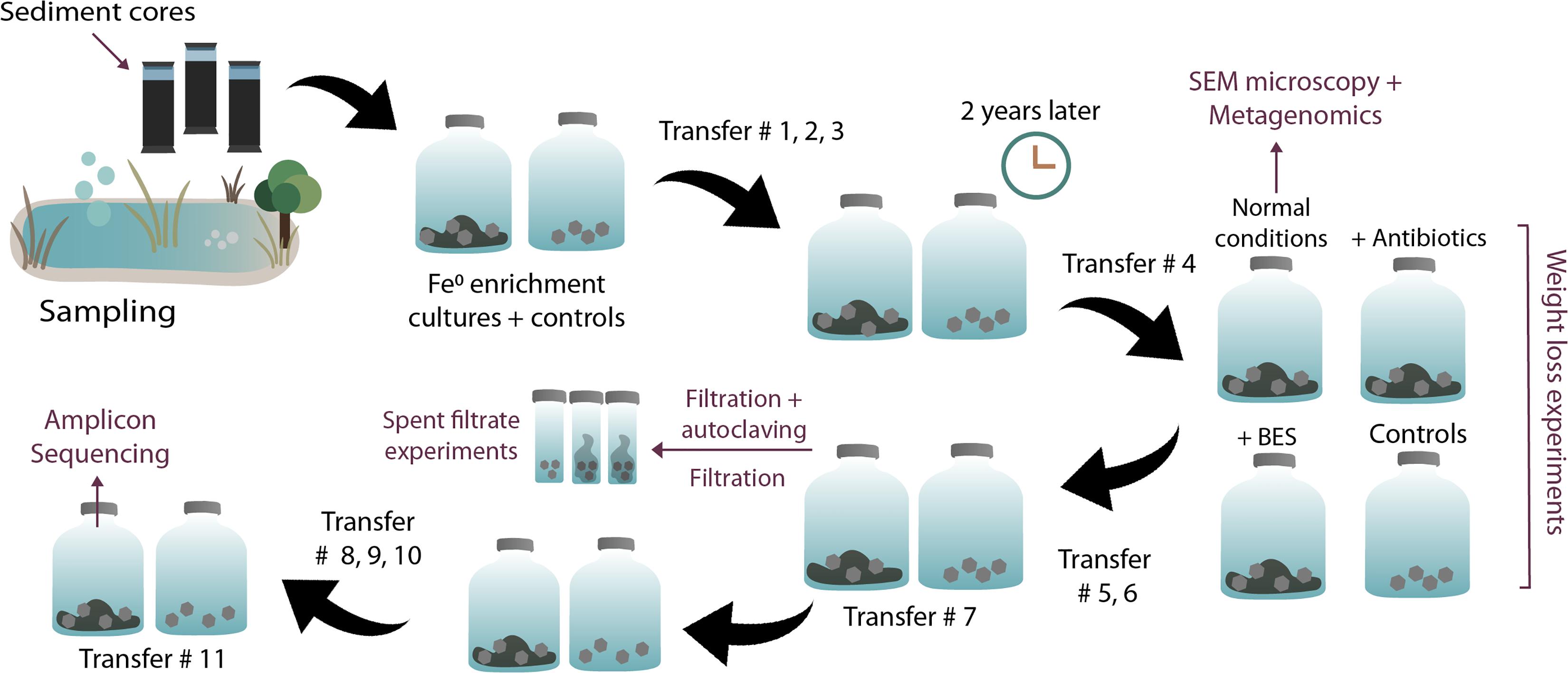
Figure 1. Overview of experimental steps. Enrichments, transfers, and experiments initiated with sediments from a climate lake.
To evaluate whether methanogens alone are corrosive, we blocked bacterial protein synthesis and bacterial cell wall synthesis with 200 μg/ml of kanamycin and 100 μg/ml of ampicillin, respectively (Palacios et al., 2019). To evaluate the corrosive potential of acetogens alone, we inhibited all methanogens with 2 mM 2-bromoethanesulfonate (BES) (Zhou et al., 2011).
To evaluate the possible role of spent media enzymes/shuttles in H2 evolution at the Fe0 surface, we withdrew spent media from cultures (at transfer 7) when they have grown on Fe0 for 10 days. Ten milliliters of spent media was then filtered and added to fresh and sterile Fe0 chips. H2 concentration was monitored immediately and after 24 h (n = 3; triplicates). In parallel, we also tested autoclaved spent media filtrate (n = 3; triplicates), which would inform if the activity was due to the release of enzymes (negatively affected by autoclaving) or released shuttles/corrosive molecules (unaffected by autoclaving). At the same time, we ran abiotic controls (Fe0-containing media free of cells; n = 3; triplicates) by exposing fresh Fe0, to 10 ml “spent” media from abiotic controls incubated for 10 days without cells. Abiotic controls show the extent of H2 produced at the Fe0 surface in the absence of biological activity and if any chemicals build up even under abiotic conditions to influence Fe0 corrosion. Other controls were plain cell filtrate (autoclaved, n = 1, and not, n = 1) both incubated without Fe0, informing whether cellular constituents evolve H2 independent of Fe0. We detected little to no H2 in these control experiments, showing that spent media without Fe0 as an electron donor cannot generate H2. All spent filtrate experiments were carried out with 10 ml of cell filtrate added to 1 g of fresh Fe0 and incubated for 24 h at room temperature. H2 monitoring was carried out at the start and after 24 h.
Chemical Analyses
Methane and hydrogen concentrations were analyzed on a Thermo Scientific Trace 1,300 gas chromatograph system coupled to a thermal conductivity detector (TCD). The injector was operated at 150°C and the detector was operated at 200°C with 1.0 ml/min argon as a reference gas. The oven temperature was constant at 70°C. The separation was done on a TG-BOND Msieve 5A column (Thermo Scientific; 30-m length, 0.53-mm i.d., and 20-μm film thickness) with argon as carrier gas at a flow of 25 ml/min. The GC was controlled and automated by Chromeleon software Dionex, Version 7. In our setup, the limit of detection for H2 and CH4 was 5 μM.
Acetate production was measured using the Dionex ICS-1500 Ion Chromatography System (ICS-1500) equipped with the AS50 autosampler and an IonPac AS22 column coupled to a conductivity detector (31 mA). We used 4.5 mM Na2CO3 with 1.4 mM NaHCO3 as eluent for the separation of volatile fatty acids. The run was isothermic at 30°C with a flow rate of 1.2 ml/min. The limit of detection for acetate was 0.1 mM.
Removal of Corrosion Crust and Corrosion Rates
The corrosion crust from the iron coupons was removed with inactivated acid (10% hexamine in 2 M HCl) (Enning and Garrelfs, 2014). Then, the iron coupons were dried with a nitrogen gas stream and weighed.
Scanning Electron Microscopy
Cells were fixed anaerobically on iron coupons by adding 2.5% glutaraldehyde in 0.1 M phosphate buffer (pH 7.3) and incubating at 4°C for 12 h. The corroded coupons were then washed three times with 0.1 M phosphate buffer at 4°C for 10 min. Dehydration was accomplished by a series of anoxic pure ethanol steps (each step, 10 min; 35, 50, 70, 80, 90, 95, and 100% v/v) (Araujo et al., 2003). The coupons were chemically dried with hexamethyldisilazane under a gentle N2 gas stream. Specimens were stored under an N2 atmosphere and analyzed within 18–24 h at the UMASS electron microscopy facility using the FEI Magellan 400 XHR-SEM with a resolution of 5 kV.
DNA Purification From Microbial Enrichments
DNA was extracted from triplicate enrichments grown in 50 ml of media with 5 g of Fe0 as the sole electron donor. Extractions were only carried out on the entire corrosive community (untreated with inhibitors) at the 1-month mark of the 4th transfer (for shotgun metagenome sequencing) and 11th transfer (for amplicon sequencing), when both acetogens and methanogens are sufficiently active according to physiology data.
Before metagenome sequencing, genomic DNA was isolated from the pellets of triplicate enrichments (fourth transfer) using commercially available kits, as previously described (Palacios et al., 2019).
Before amplicon sequencing, genomic DNA was isolated from the cell filtrates of triplicate enrichments (11th transfer) using a FastDNA Spin kit for Soil (MP Biomedicals, United States) with the following modifications: to the Lysing Matrix E tube, we added 500 μl of sample, 480 μl of sodium phosphate buffer, and 120 μl of MT buffer. Bead beating was performed at 6 m/s for 4 × 40 s (Albertsen et al., 2015).
The integrity of genomic DNA was verified on an agarose gel and quantified on a mySPEC spectrophotometer (VWR®/Germany) or Qubit dsDNA HS/BR Assay kit (Thermo Fisher Scientific, United States).
Whole Shotgun Metagenome Sequencing, Assembly, and Analyses
DNA from triplicate biological replicates was pooled before whole metagenome sequencing. Sequencing was carried out commercially on a NovaSeq 6000 system, using an Illumina TrueSeq with a single PCR step (Macrogen/Europe). Unassembled DNA sequences were merged, quality checked, and annotated using the Metagenomics Rapid Annotation (MG-RAST) server (v4.03) with default parameters (Meyer et al., 2008). Illumina True Seq sequencing resulted in 3,723,388 high-quality reads of a total of 4,032,354 with an average length of 249 ± 35 bp (Supplementary Table S1). We compared the metagenomic data with the RefSeq database (Tatusova et al., 2015) available on the MG-RAST platform. The alpha diversity for this shotgun metagenome was 61 species. The rarefaction curve indicated that we recovered most of the prokaryotic diversity in this sample (Supplementary Figure S2). To investigate functional genes in the unassembled shotgun metagenome, sequencing reads were annotated against the KEGG Orthology (KO) reference database. Both taxonomic and functional analyses were performed with the following cutoff parameters: e-value of 1e–5, a minimum identity of 80%, and a maximum alignment length of 15 bp. The unassembled metagenome dataset is available at MG-RAST with this ID: mgm4827981.3.
Before assembling the metagenome, data quality and kmer abundance were estimated using the method of Eitel et al. (2018). The Python and R source code for these steps are available online at https://github.com/wrf/lavaLampPlot. As distinct GC-coverage peaks were clearly visible in the kmer plot, the data were of sufficient quality to continue the assembly. The metagenome was then assembled with MetaSPAdes, a package of the SPAdes v3.14.1 assembler optimized for metagenomes (Nurk et al., 2017), using default parameters. This resulted in 84742 contigs with a contig N50 of 6.7 kb. Most contigs were short and had low coverage, although the largest contig was 489,000 bp. When restricted to contigs over 500 bp, we obtained only 29,455 contigs with a contig N50 of 12 kb, but nonetheless, these accounted for 85% of the assembly.
Binning of the contigs was done manually, using the top BLAST hits to the RefSeq database for each contig, as well as the GC content and coverage. This yielded 10 bins, corresponding to 12 species. Bins for the two predicted archaea were filtered to include only contigs where the best BLAST hit was another archaeon. Proteins were then predicted for all contigs using Prodigal V2.6.3 (Hyatt et al., 2010), using the metagenome mode (option “-p meta”). This gave 1,63,458 predicted proteins in total. Protein counts for each bin are included in the Supplementary Material, Supplementary Table S2. Pathway annotation was done using the KEGG web server BLASTKOALA (Kanehisa et al., 2016) for each bin, selecting “Prokaryotic” annotation mode, using “Genus-Prokaryotes” as the database. Annotations were predicted for >46% of proteins across all bins (see Supplementary Table S2).
All contigs were then uploaded to the MG-RAST server. For the assembled shotgun metagenome, functional gene screening was run on the MG-RAST server in the same fashion as for the unassembled reads (see above). Functional gene screening was also done against the bins annotated with BLASTKOALA. The assembled metagenome dataset is available at MG-RAST with this ID: mgm4916968.3. For the metagenome assembly and annotation, intermediate analyses, code, and commands can be found at https://bitbucket.org/wrf/corrosion-community-2021.
Additionally, we screened the metagenome for hydrogenases by searching for sequence hits with high similarity to those of known [FeFe]-hydrogenases. The resulting 77 genes were then verified for the presence of hydrogenase domains against the most recent CDD/SPARKLE (Conserved Domain Database) database (Marchler-Bauer and Bryant, 2004; Lu et al., 2020) using the batch-blast CD search function on the NCBI platform. At this step, we discarded queries that had not been matched with the hydrogenase superfamily. To conclusively resolve the hydrogenase class, we used the web-based hydrogenase classifier HydDB (Søndergaard et al., 2016).
Amplicon Sequencing and Analyses
DNA from triplicate enrichments (transfer 11) was sequenced commercially (DNASense ApS/Denmark). The 16S rRNA variable gene regions V4 (Bacteria and Archaea) and V3–V5 (Archaea) were amplified. We did not use the standard Illumina primers because they are less successful at retrieving some Archaeal phyla (Brandt and Albertsen, 2018). Therefore, we used a modified primer pair for the V4 region [515FB] GTGYCAGCMGCCGCGGTAA and [806RB] GGACTACNVGGGTWTCTAAT (Apprill et al., 2015), which is better suited for the detection of most Bacteria and Archaea phyla (Brandt and Albertsen, 2018). Additionally, we also amplified the V3–V5 region using archaeal specific primers [Arch−340F] CCCTAHGGGGYGCASCA and [Arch−915R] GWGCYCCCCCGYCAATTC (Pinto and Raskin, 2012).
The amplicons were then primed for sequencing by the addition of adapters. Then, we sequenced pair-end for V4 (2 × 300 bp) and single-end for V3–5 (1 × 300 bp) on an Illumina MiSeq (Illumina, United States) using a MiSeq Reagent kit v3 (Illumina, United States) according to the Illumina protocol (Illumina, 2015). Negative controls (from DNA extraction and PCR amplification) were sequenced alongside samples. As sequencing control, a PhiX control library was spiked in (at 10%) to overcome issues often noticed with low diversity amplicon samples. All raw metagenomic and amplicon sequencing data have been deposited at NCBI under BioProject accession PRJNA713576.
Forward single-end reads for the V3–V5 variable region were cut to 225 bp. All sequence reads (for V3–5 and V4 variable regions) were trimmed, dereplicated, formatted, and chimera filtered with Trimmomatic v. 0.32 (Bolger et al., 2014) using settings SLIDINGWINDOW:5:3 and MINLEN: 225. For the V4 variable region, which was pair-end sequenced, the forward and reverse reads were merged with FLASH v. 1.2.7, settings −m 10 −M 250 (Magoè and Salzberg, 2011). Trimmed and merged reads were processed using the UPARSE workflow, which has better accuracy and generates realistic OTU counts (Edgar, 2013). The dereplicated reads were clustered, with default settings. OTU abundances were estimated using the settings −id 0.97 −maxaccepts 0 −maxrejects 0. Taxonomy was assigned using the RDP classifier (Wang et al., 2007) in QIIME, –confidence 0.8 (Caporaso et al., 2010) and the SILVA database vs. 132 (Quast et al., 2013). The results were analyzed via the Rstudio IDE using the ampvis package v.2.6.4 (Albertsen et al., 2015).
Phylogenetic Tree Construction
Phylogenetic trees were constructed in GENEIOUS (Kearse et al., 2012). The closest related sequences (from type cultures or environmental samples) were identified via the BLASTn (for 16S rRNA gene) or BLASTp (for functional genes) against the RefSeq database at NCBI. Sequences were downloaded as FASTA files and imported to GENEIOUS. Sequences were aligned with MUSCLE (Edgar, 2004) using eight iterations, measuring the distance with kmer 6_6 (iteration 1) and subsequently with pctid_kimura (seven iterations), clustering with UPGMB, and with CLUSTALW for sequence weighting, with - anchor spacing 32, – min. length 24. The protein alignment was then manually verified and refined with MAFFT (Katoh et al., 2002; Katoh and Standley, 2013) using - FFT-NS-I ×1,000 algorithm, the BLOSUM62 scoring matrix, and - gap penalty 1.53. The 16S gene sequence alignments were manually verified and refined with MAFFT using – FFT-NS-I x1000 algorithm, the 200PAM scoring matrix, and – gap penalty 1.53.
Trees were constructed with the GENEIOUS Tree Builder using the Jukes-Cantor genetic distance model, and the Neighbor Joining tree building method with 100 bootstraps (support threshold 50%).
Results and Discussion
Pitted Fe0 Corrosion
We monitored corrosion by a climate lake community transferred only with Fe0 as the electron donor over the course of 3 years. The original slurry amended with Fe0 generated more methane than a slurry without Fe0 (Supplementary Figure S1). Afterward, cultures were transferred (10% transfer) 11 times in fresh media containing only Fe0 as the electron donor. After 11 transfers, cultures continued to generate methane from Fe0.
We monitored a full corrosion time course after circa 2 years, during the fourth transfer on Fe0. The microbial community corroded Fe0 significantly in contrast to cell-free controls according to microscopy observations (Figure 2), gravimetric measurements (Figure 3), and metabolic product buildup (Figure 3).
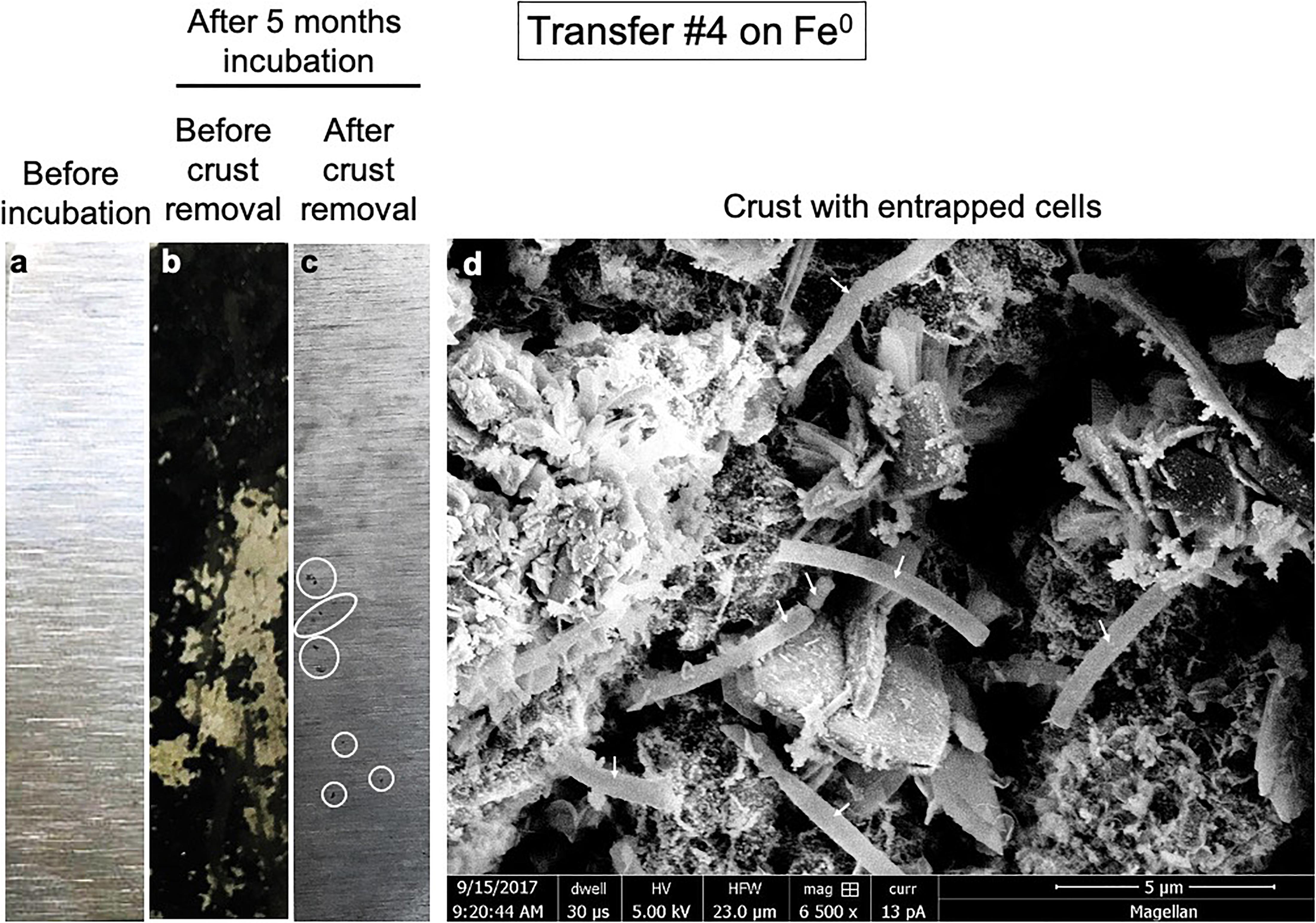
Figure 2. Pitted corrosion by microorganisms. Metallic iron sheets before exposure to cells (a), before crust removal (b), and after crust removal (c). (d) A scanning electron micrograph showing cells entrenched on the metal surface.
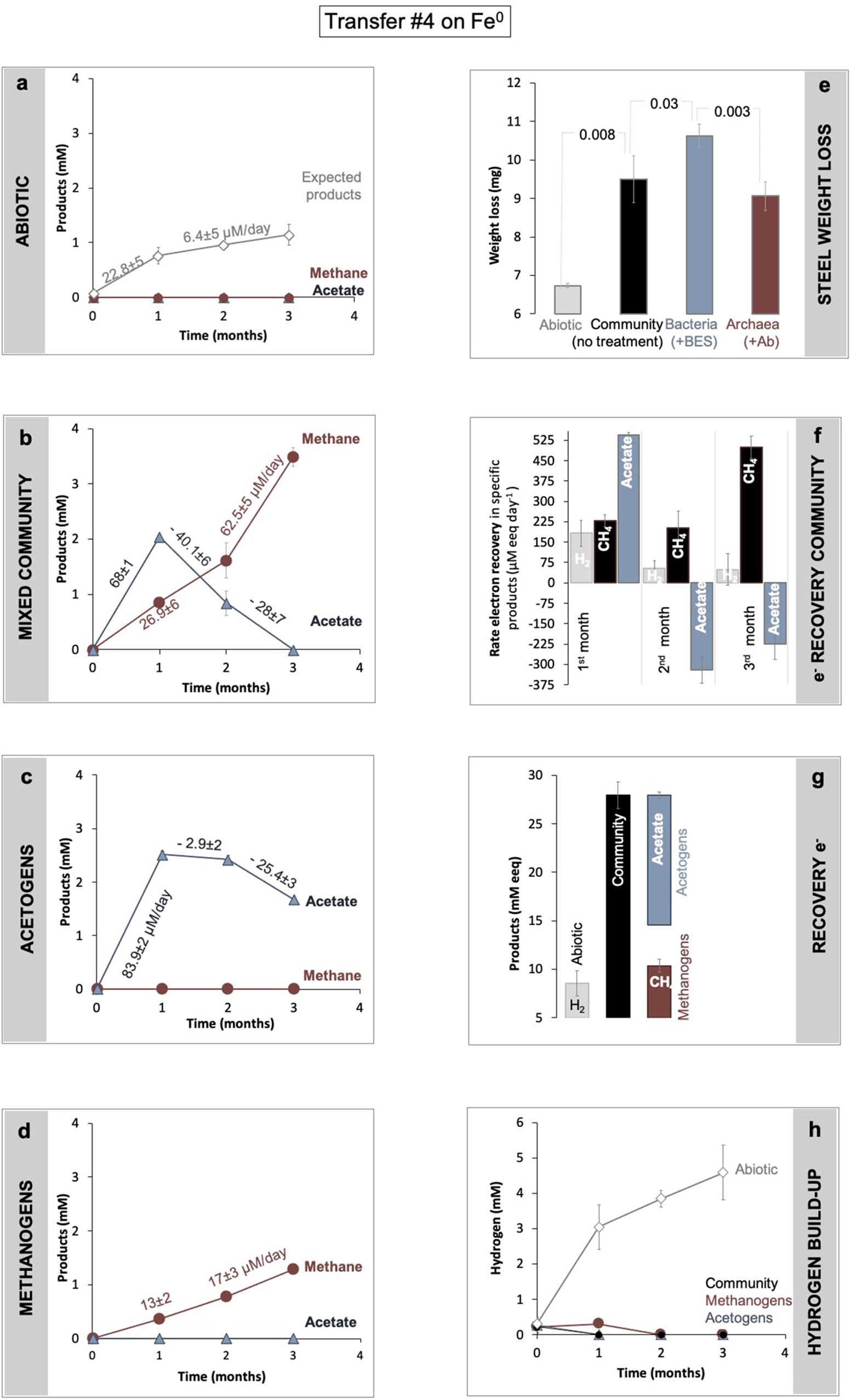
Figure 3. Determination of Fe0 corrosion following product formation (a–d) and weight loss (d). Product formation was monitored in (a) abiotic controls, versus (b) a microbial community from SDU sediments (c) by bacteria alone, after specific inhibition of the methanogens with 2-bromoethanesulfonate (BES), and (d) methanogens alone after inhibition of all bacteria using a mix of antibiotics (Ab). (e) Gravimetric determination of material loss under all four conditions (abiotic, with the mixed community, with acetogens alone, with methanogens alone). For the same incubations, panel (f) shows changes in electron recovery rates over the course of 3 months and (g) total electron recovery into products as mM electron equivalents (eeq) produced from Fe0 under all four different conditions. To calculate electron recoveries, we consider 2 mM electron equivalents/eeq per mol H2 (according to: 2e– + 2H+ → H2) and 8 mM eeq for each mol of methane or acetate (according to: CO2 + 8e– + 8H+ → CH4 + 2H2O; and 2CO2 + 8e– + 8H+ → C2H4O2 + 2H2O). (h) Hydrogen evolution in abiotic controls (mineral media controls) with Fe0, versus incubations with cells (community, acetogens, and methanogens alone) and Fe0. All incubations were run in parallel and in triplicate (n = 3).
Iron sheets incubated for several months with cells from a climate lake developed a black crust. Scanning electron microscopy revealed that the black crust incorporated long blunt-end rod cells encrusted on Fe0 (Figure 2d). The removal of the black crust revealed pitted corrosion underneath (Figure 2).
The lake community induced 41% more Fe0 weight loss, consuming 2.8 ± 0.6 mg more Fe0 (n = 3; p = 0.008) than cell-free controls (Figure 3), according to gravimetric measurements.
Metabolic product buildup showed that the microbial community generated methane and acetate simultaneously once provided with Fe0 (Figure 3), confirming that Fe0 delivers electrons for two types of microbial metabolisms, acetogenesis and methanogenesis. As expected, the abiotic controls with Fe0 showed no traces of microbial metabolic products (methane and acetate) (Figure 3).
During a 3-month-long incubation with Fe0, the corrosive community formed acetate transiently (first month) and ultimately accumulated only methane (Figure 3). During the first month of incubation, acetogenesis rates (68 ± 1 μM acetate/day) surpassed methanogenesis rates (27 ± 6 μM methane/day) (Figure 3), whereas during the last two months of incubation, acetogenesis ceased. At the same time, methanogenesis sped up, achieving rates two-fold (62.5 ± 5.1 μM methane/day) above those predicted (28 ± 7.3 μM methane/day) by acetoclastic methanogenesis (Figure 3). These results show that methanogens did not rely on the acetate generated by acetogens for methanogenesis. Altogether, the microbial community made 3.3-fold more methane (3.5 ± 0.1 mM) than expected (1.1 ± 0.2 mM) from the H2 evolved abiotically (2e– + 2H+ → H2) at the Fe0 surface (Figure 3). These results show that the microbial community employs effective alternative mechanisms (other than abiotic H2) to access electrons from the Fe0 surface. Unraveling these mechanisms is difficult without pure cultures. Nevertheless, we attempted to determine how the microorganisms influence each other’s metabolism and Fe0 corrosion by separating each physiologic group with group-specific inhibitors.
Unraveling Interspecies Interactions on Fe0
In all our enrichments, methane is the final product of the microbial community provided with Fe0 as electron donor and CO2 as the electron acceptor. Thus, methanogens must interact with acetogens, for example, by consuming their metabolic product. Three possible interspecies interactions would ultimately give off only methane:
(a) mutualism (win/win) when methanogens feed on the product of the acetogen, both partners being influenced favorably, one (acetogen) by the removal of metabolic product and the other (methanogen) by the availability of a food substrate.
(b) commensalism (0/win) when acetogens are unaffected by the presence of the methanogens, whereas methanogens are influenced favorably, e.g., by feeding on acetate, the product of acetogenic metabolism.
(c) parasitism/scavenging opportunism (loss/win) when acetogens are negatively influenced by the presence of the methanogens, while methanogens are influenced favorably by the presence of the acetogens.
To test these scenarios, we carried out inhibition experiments to specifically block each metabolic group. Archaeal methanogens were inhibited with 2-bromoethane sulfonate (BES), a coenzyme M analog (Zhou et al., 2011), resulting in favorable conditions for acetogens. Acetogens were inhibited by a cocktail of antibiotics (kanamycin and ampicillin), thus favoring only methanogens. Then, we compared corrosion by each group alone by documenting corrosion via gravimetric measurements and metabolite production (Figure 3).
According to gravimetric measurements, when separated, methanogens and acetogens remained significantly more corrosive than cell-free controls (Figure 3). However, methanogens were only slightly less corrosive than the mixed community (ca. 5% less, n = 3, p = 0.18), whereas acetogens were significantly more corrosive alone (ca. 16% more; n = 3, p = 0.03), denoting that electron uptake from Fe0 by acetogens was negatively affected by the presence of methanogens. Furthermore, when examining acetate metabolism, the acetate buildup was faster when acetogens were alone (Figure 3c, 23% rate increase, n = 3, p = 0.0003) than with methanogens. Thus, the acetogens were more corrosive alone and metabolically better off than within the mixed community (Figure 3). In other words, these results reflect that acetogens are negatively impacted (loss) by the presence of methanogens.
Methanogens, on the other hand, produced three-fold less methane alone than within the mixed community (Figure 3d, n = 3, p = 0.0002). Methanogenesis was overall faster in the presence of acetogens than when methanogens were incubated alone (Figure 3). Nevertheless, the rates of methanogenesis could not be linked to acetate utilization because acetate consumption (28 ± 7 μM/day) did not match methane production (62.5 ± 5 μM/day). This suggests that methanogens are favored (win) by the presence of the acetogens exclusive of acetate released by acetogens.
Altogether, our results show that during Fe0 corrosion, acetogens were negatively (loss) impacted by the presence of methanogens, whereas methanogens were positively (win) impacted, demonstrating a parasitic/opportunistic (loss/win) type of interaction between these two physiologic groups.
A possible negative effect on the acetogens could be due to the alkalinization of the media by protons being dislocated from solution during CO2 conversion to methane. During CO2 conversion by methanogens, the pH often becomes alkaline (Xu et al., 2014). Consequently, we verified the pH change over time. Typically, our cultivation media has a pH of 7.06 ± 0.02. However, after 6 months of incubation, four cultures incubated solely with Fe0 exhibited an alkaline pH of 8.47 ± 0.06. To verify whether alkalinization was dependent on cellular activity, we monitored pH changes in abiotic Fe0 media versus Fe0 media with cells (transfer 10) over the course of 30 days. We noticed a significantly higher alkalinization in media with cells than media without cells starting with day 15 (Figure 4). Typically, CO2-reducing acetogenesis is negatively affected by alkaline conditions (Mohanakrishna et al., 2015), explaining the decrease in acetogenic activity after 1 month. On the other hand, alkaline conditions inhibit acetotrophic methanogenesis while favoring CO2-reducing methanogenesis (Phelps and Zeikus, 1984).
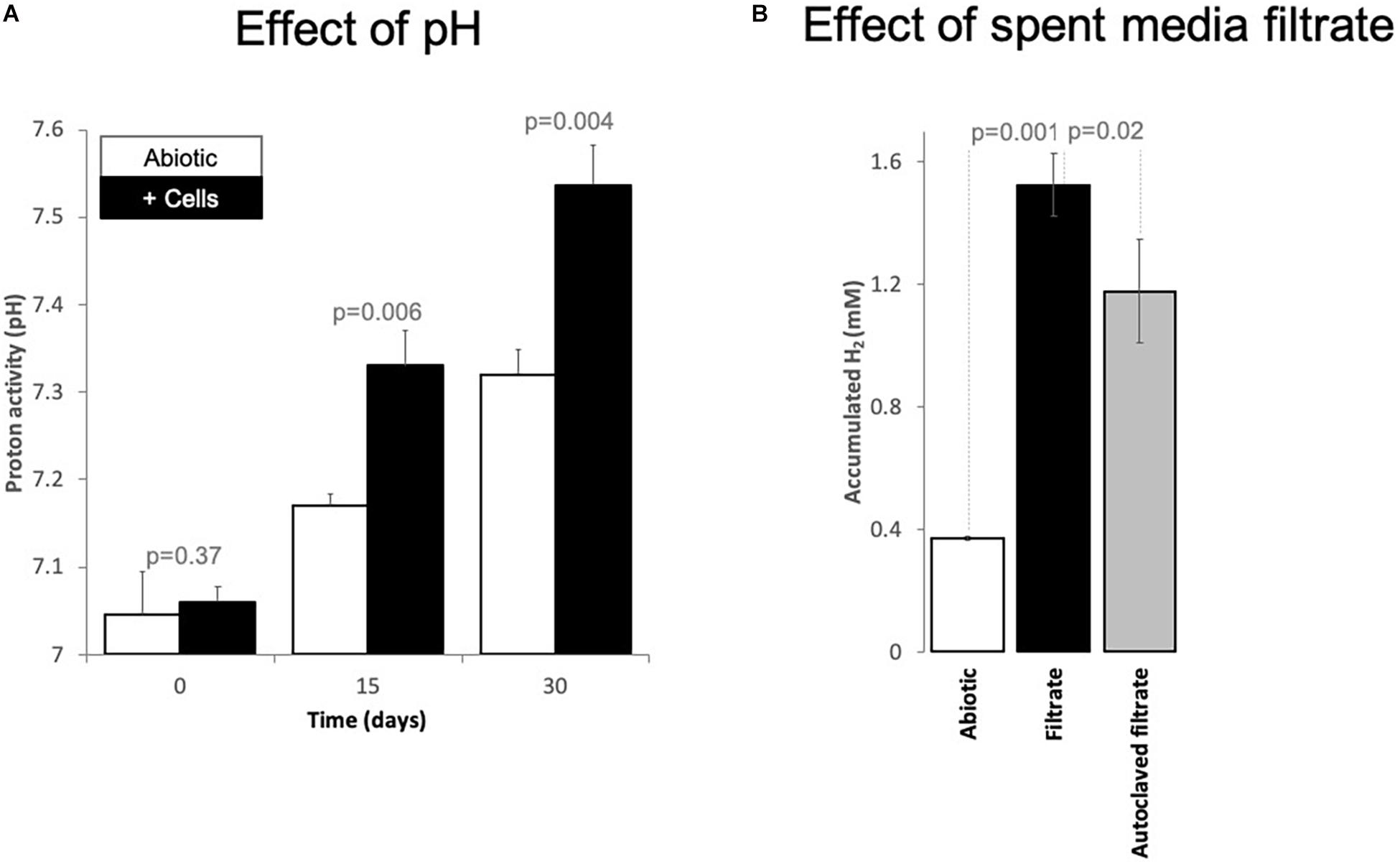
Figure 4. Changes of (A) proton activity (pH) and (B) H2 evolution from Fe0 catalyzed by spent media components. (A) pH changes in abiotic incubations (white bars, mineral media controls) versus incubations with cells (black bars, mixed corrosive community) exposed to Fe0 for 1 month. (B) Changes in H2 evolution from Fe0 when the material got exposed for 24 h to 100% spent media, filtered fresh from a 10-day old Fe0-grown mixed community. Additionally, we exposed Fe0 to spent media from the same culture that has been inactivated by autoclaving. Also, we tested whether 10-day old abiotic spent media induces any changes in H2 evolution from Fe0. All experiments were carried out in parallel and in triplicate (n = 3). Spent media (autoclaved/not) without Fe0 did not produce H2 at all (data not shown, values under the detection limit, n = 1 for each).
A possible positive effect on the methanogens could be due to H2-evolving enzymes being released by acetogens, which reach stationary phase earlier than methanogens. We, therefore, explored whether cell exudates increase H2 buildup from Fe0. For this purpose, we exposed fresh Fe0 to spent media filtrate and compared H2 evolution to that observed with the abiotic filtrate. Ten-day-old spent media filtrate stimulated H2 evolution four-fold compared to abiotic controls (Figure 4). Acetate cannot catalyze H2 evolution from Fe0. Thus, the rise of H2 buildup by spent media may be due to catalytic molecules (enzymes/non-proteinaceous catalysts, e.g., FeS centers) being released in the media by aged cells, thereby promoting electron capture from Fe0 and consequently catalyze H2 buildup. Heat treatment of the spent filtrate led to lower H2 evolution from Fe0, although still three-fold higher than abiotic controls (Figure 4). These results suggest that enzymes have a role in enhancing H2 evolution from Fe0. However, other undefined spent media components are also evidently involved.
In fact, previous studies revealed that H2 evolution could be catalyzed by non-viable cell components (peptides, trace metals), which concentrate, for example, on the surface of electrodes (poised at −600 mV and used as sole electron donor) (Yates et al., 2014).
In our system, the viable media filtrate may contain [FeFe]-hydrogenase and nitrogenase enzymes, which are among the most prevalent enzymes catalyzing proton reduction for H2- production. The non-viable cell components in the spent media filtrate could be catalytic active centers and other organic matter-bound redox-active metal centers.
Corrosive Acetogens and Methanogens
To further investigate the possible interplay between acetogens and methanogens, we investigated the microbial community and their possible metabolic interplay by shotgun metagenomics and amplicon sequencing.
Clostridia and Methanobacteria were enriched with Fe0/CO2 as sole energy sources (Figure 5). We obtained a shotgun metagenome after four transfers when other microorganisms like Bacteroidetes, Deltaproteobacteria, and Methanomicrobia also accompanied the groups above. However, after 11 transfers on Fe0/CO2 -media, only Clostridia and Methanobacteria persisted (Figure 5).
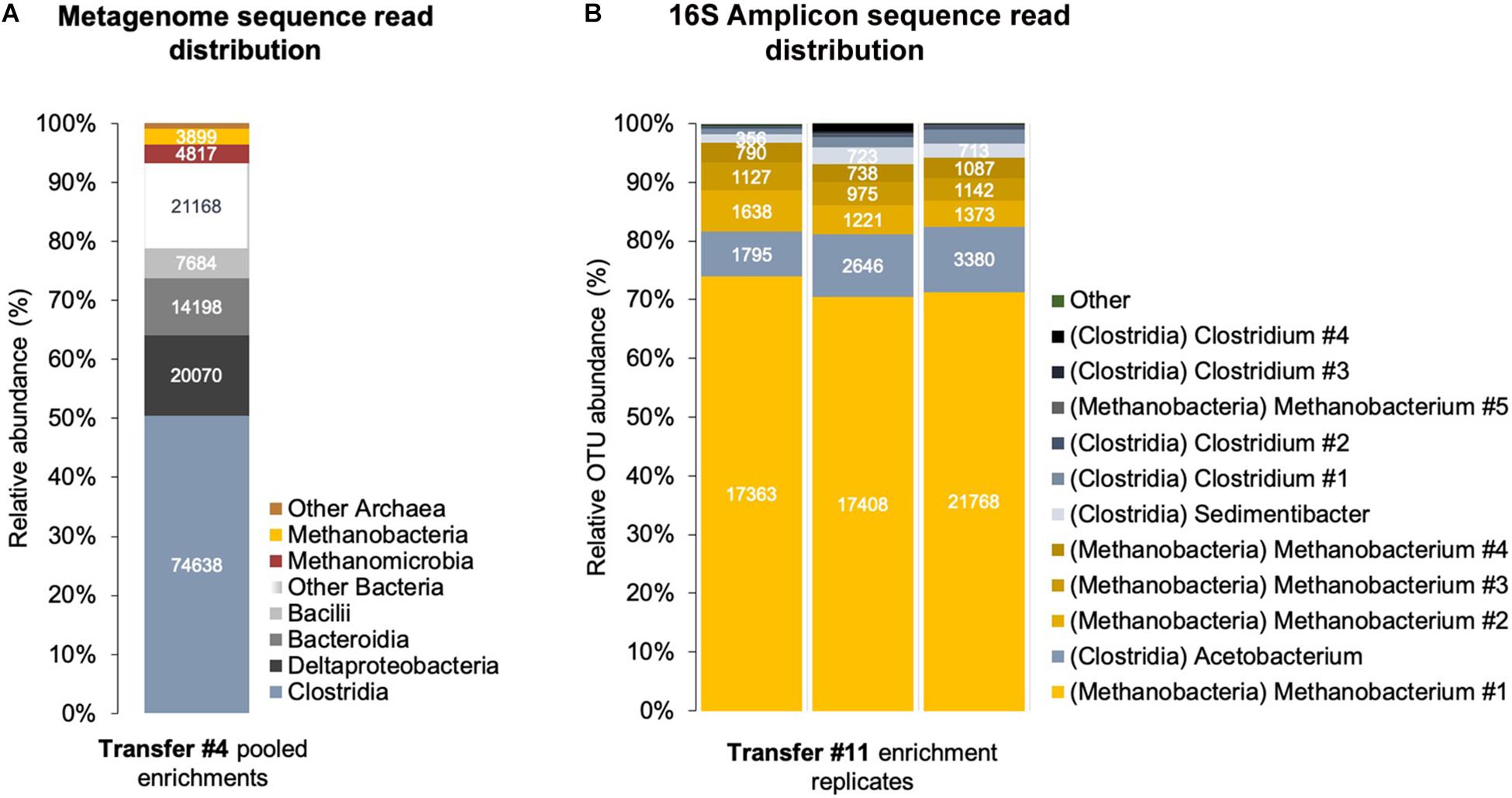
Figure 5. Corrosive microbes from a climate lake enrichment. (A) Relative abundance of classes of Bacteria and Archaea in a shotgun metagenome obtained from a sedimentary lake community transferred four times on Fe0. (B) Relative abundance of OTUs (operational taxonomic units) identified by amplicon sequencing of the V4 region of the 16S rRNA gene in triplicate enrichments.
Sequential transfers with Fe0 under CO2-reducing conditions adapted a community with only two C1-based respiratory metabolisms: CO2-reducing acetogenesis and CO2-reducing methanogenesis. This was apparent in the metagenome from the relative distribution of energy metabolism genes, including C1-carbon metabolism.
Acetogens
At the fourth transfer, we identified several acetogenic genera (Supplementary Table S2) by shotgun metagenomics. The class with the highest relative read abundance was Clostridia (50.5% of assembled prokaryotic reads), followed by Deltaproteobacteria (13.6% of assembled prokaryotic reads) and Bacteroidia/Parabacteroidia (9.6% of assembled prokaryotic reads).
Eight bacterial metagenomes were assembled into bins or metagenome-assembled genomes and then assigned by MG-RAST to one species of Desulfovibrio, one species of Parabacteroides, and six or eight Clostridium species since some bins appear to contain two different strains (Supplementary Table S2). Analyses of the key gene for acetogenesis fhs—formate-tetrahydrofolate ligase (Müller and Frerichs, 2013)—confirmed that five of the bins were inherent acetogens (Figure 6, Supplementary Figure S5, and Supplementary Table S3), four Clostridium and one Parabacteroides. The Parabacteroides fhs was related (93.2% amino acid identity) to that of an uncharacterized Macellibacteroides isolate (Supplementary Table S3).
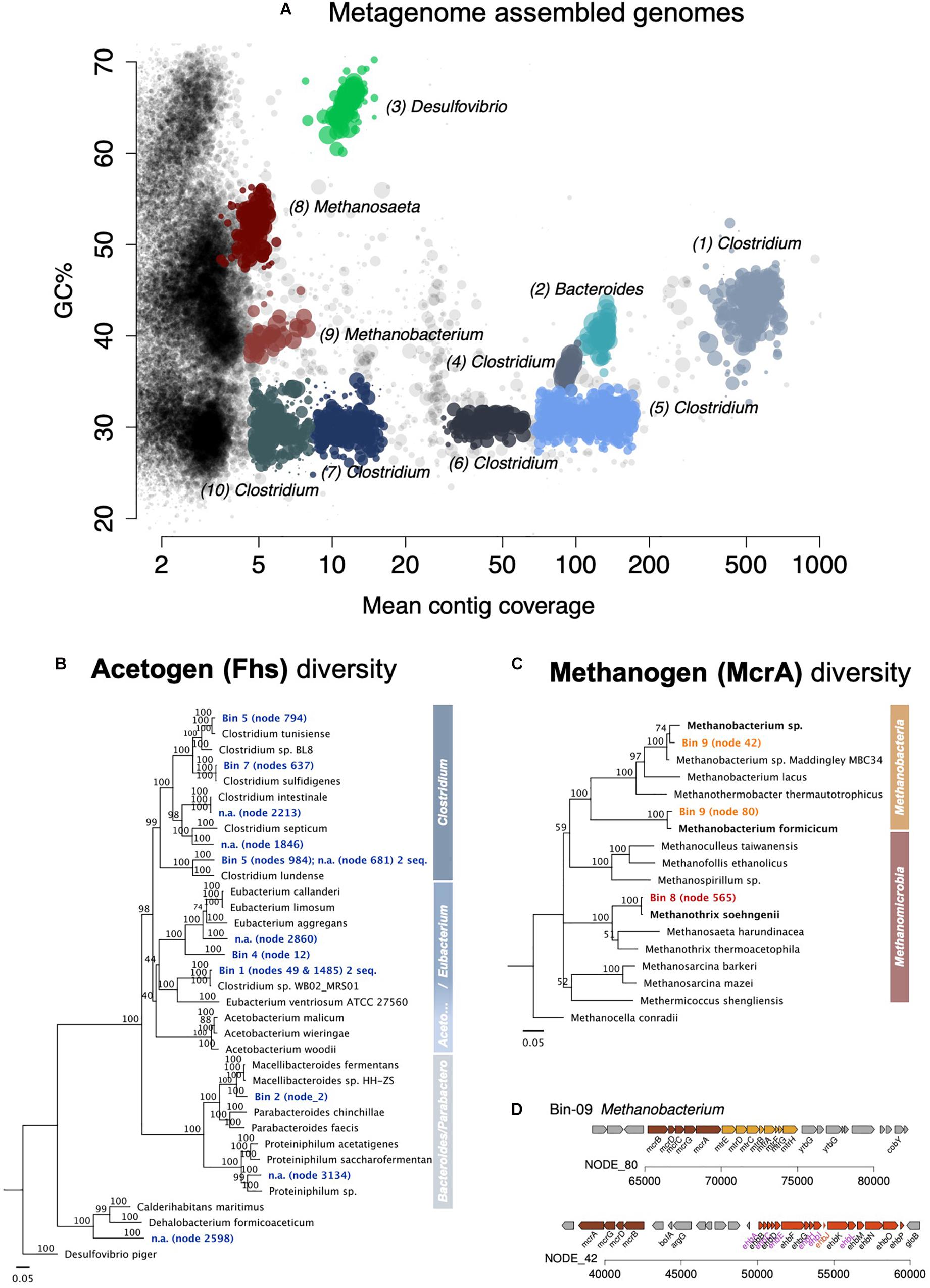
Figure 6. Assembled metagenome bins and the phylogenetic distribution of key genes for acetogenesis and methanogenesis. (A) Overview of all assembled metagenome bins in accordance with the GC content and mean contig coverage. (B) Neighbor Joining tree depicting the diversity of the key protein for acetogenesis Fhs (formate—tetrahydrofolate ligase). The Fhs of a Deltaproteobacteria, Desulfovibrio piger, was used as an outgroup root for this tree. (C) Neighbor Joining tree depicting the diversity of the key protein for methanogenesis McrA (the alpha subunit of the methyl:coenzyme M reductase). The McrA of Methanocella conradii was used as an outgroup root for the tree. Only complete gene sequences were used for the amino acid alignments. A scale of 0.05 means 5% distance. Node numbers show bootstrap values. Values over 70 should be considered significant and supporting the branching point. (D) Two complete mcr operons found in the Methanobacterium-bin (no. 9).
Of the Clostridium bins, only two showed high fhs-amino acid identity to actual Clostridium species in culture (Supplementary Table S3). Clostridium bin five included two fhs, both with high sequence similarity with the fhs in Clostridium species, one with 99.5% to C. tunisiense, the other 99.2% to C. lundense. Also, Clostridium bin seven carried two fhs, one 99.8% identical in amino acid residues to C. sulfidigenes, and the other 90.6% identical to the fhs of a Sedimentibacter. Nevertheless, the best represented Clostridium bins in the metagenome, bins 1 and 4, had uncharacteristic Clostridium fhs genes that were instead assigned to other clostridial genera. So, the fhs of Clostridium bin 1 showed a high amino acid sequence similarity to that of Clostridium sp. WB02 MRS01, which does not fall under Clostridium genus sensu stricto and is known as Lacrimispora celerecrescens (>99.2% amino acid identity), whereas the fhs of Clostridium bin 4 was far related to Sedimentibacter saalensis (80.5% amino acid identity).
Overall, class Clostridia showed the best representation of the Wood Ljungdahl pathway (Figure 7 and Supplementary Table S5).
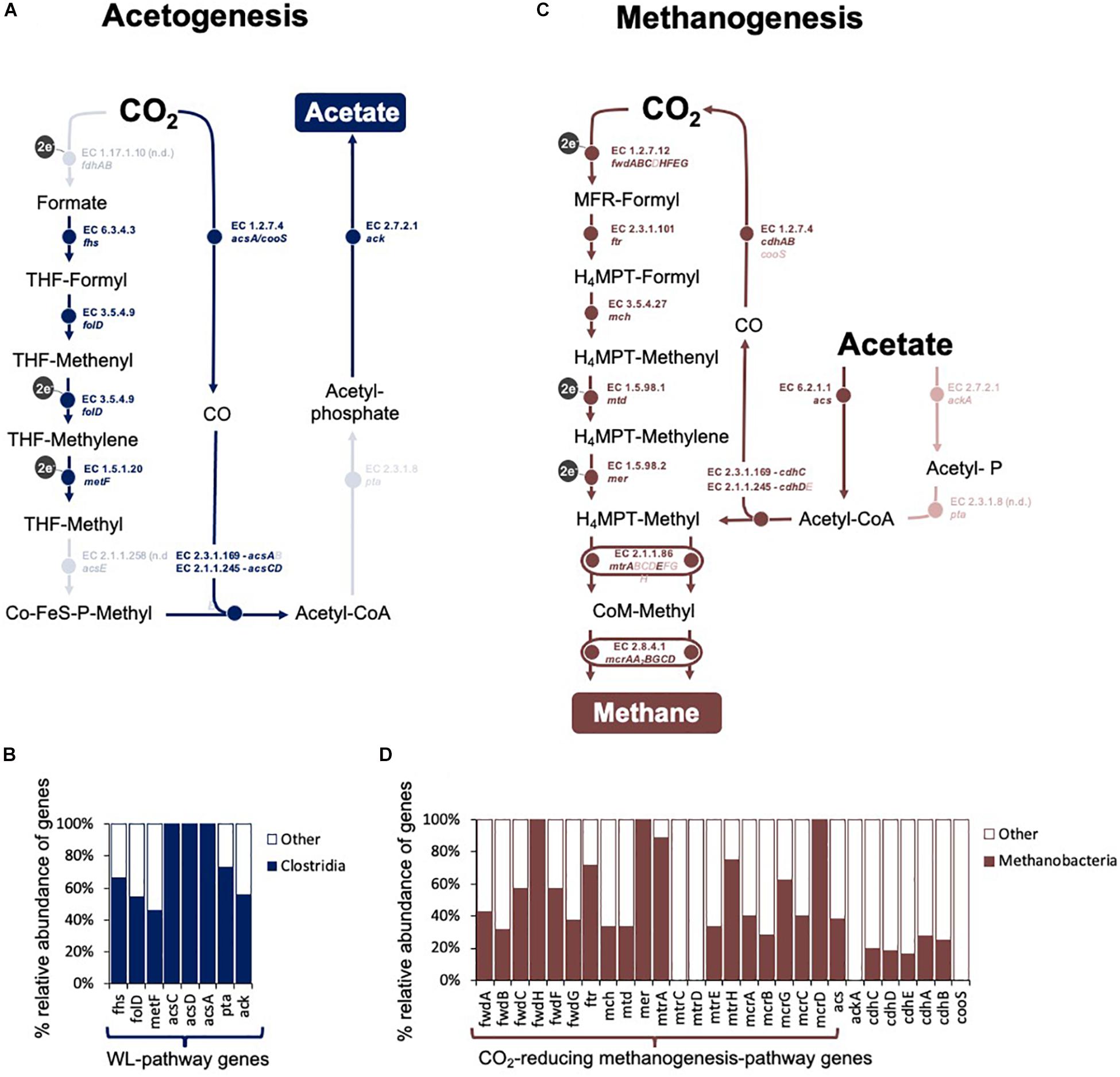
Figure 7. Overview of CO2-reducing acetogenesis and methanogenesis in an assembled metagenome obtained at the fourth transfer of the Fe0-corrosive enrichment. Only reactions involved in C-modification during (A,C) acetogenesis and (B,D) methanogenesis are presented. (A) The Wood Ljungdahl pathway in the Clostridial metagenome. It includes: fdh operon (formate dehydrogenase), fhs (formate–tetrahydrofolate ligase), folD (methenyltetrahydrofolate cyclohydrolase), metF (methylenetetrahydrofolate reductase), acsE (5-methyltetrahydrofolate:corrinoid/iron-sulfur protein Co-methyltransferase), acs (CO-methylating acetyl-CoA synthase), acsCD (5-methyltetrahydrosarcinapterin:corrinoid/iron-sulfur protein CO-methyltransferase), acsA/cooS (Ni CO dehydrogenase), pta (phosphate acetyltransferase), and ack (acetate kinase). (B) Relative distribution of the genes for the WL-pathway in Clostridia. (C) Methanogenesis pathway in the Methanobacteria metagenome. It includes: fmd/fwd operon (formylmethanofuran dehydrogenase), ftr (formate–tetrahydrofolate ligase), mch (N5, N10-methenyltetrahydromethanopterin cyclohydrolase), mtd (F420-dependent N5, N10-methylene-H4MPT dehydrogenase), mer (N5, N10-methylene-H4MPT reductase), mtr operon (coenzyme M methyltransferase), mcr operon (methyl:coenzyme M reductase), hdrA1B1C1 (ferredoxin:CoB—CoM heterosulfide reductase), mvhAGD (-[NiFe]-hydrogenase, eha operon (energy-converting hydrogenase A), ehb operon (energy-converting hydrogenase B), and frhABG (F420-reducing hydrogenase) for CO2-reducing methanogenesis, plus cdhED (acetyl-CoA decarbonylase), cdhC (CO-methylating acetyl-CoA synthase), acs (acetyl-CoA synthetase), ackA (acetate kinase), and pta (phosphotransacetylase) for accetotrophic methanogenesis. (D) Relative distribution of the genes for the CO2-reducing methanogenesis pathway and acetoclastic methanogenesis pathways in Methanobacteria. Lighter shades show steps that had no hits for either Clostridia or Methanobacteria.
At the 11th transfer on Fe0, the lake community became entirely governed by bacteria of the class Clostridia according to amplicon sequencing of the V4 region of the 16S rRNA gene. We could not detect any Proteobacteria and Bacteroidia/Parabacteroidia during this latter transfer, indicating that only Clostridia species survived. We could not match the V4 region to previously identified bins because neither included a complete 16S rRNA gene.
Clostridia of the genus Clostridium were best represented in this enrichment’s metagenome after four transfers (36%, Supplementary Figure S5) and persisted in the enrichments after 11 transfers. Clostridium includes several species of CO2-reducing acetogens that use H2 as an electron donor (Bengelsdorf et al., 2018) but also electrodes (Nevin et al., 2011) and Fe0 (Monroy et al., 2011). Besides, Clostridium was previously enriched on Fe0 from environments such as scraped bicycles thrown in a Dutch channel (Philips et al., 2019), rice paddies (Kato et al., 2015), or oilfield production waters (Ma et al., 2019). These studies, along with ours, suggest that Clostridium is a likely corrosive acetogen when Fe0 becomes available in their environment.
Clostridia of the genus Acetobacterium were not represented in the metagenome after four transfers but were the main amplicon OTU detected after 11 transfers (Figure 5 and Supplementary Table S5). Nevertheless, whether this organism is a true Acetobacterium or a Clostridium remains to be determined. Phylogenetic assignment by short 16S amplicon sequences, although effective for most organisms, is ineffective for phylogenetic assignment of Clostridium species, which fall out of their family when a 16S classification is used (Wiegel et al., 2006) and require up to 46 marker genes for proper phylogenetic classification, which does not include the 16S rRNA gene (Yu et al., 2019).
Still, Acetobacterium is another genus within Clostridia that includes at least nine species of CO2-reducing acetogens (Bengelsdorf et al., 2018). A new species can even utilize Fe0 as a sole electron donor (Philips et al., 2019). Still, generally Acetobacterium species have not been effective at using Fe0 (Kato et al., 2015) or electrodes (Nevin et al., 2011) as electron donors. Yet, Acetobacterium species are often enriched on cathodes (Marshall et al., 2012, 2013; Labelle et al., 2014; Patil et al., 2015) and sometimes on steel (Mand et al., 2014; Philips et al., 2019). Moreover, they have been found in corrosive biofilms on steel structures from oil production facilities (Vigneron et al., 2016). It is believed that they were non-corrosive and rather feeding syntrophically. Thus, the exact role of Acetobacterium in Fe0 corrosion remains to be determined.
Essentially, the class Clostridia includes the majority of the CO2-reducing acetogens (50 out of 61 described CO2-reducing acetogens) (Bengelsdorf et al., 2018), some of which are known Fe0 corroders and were also the main contenders at acetogenesis in our Fe0-dependent enrichments according to the distribution of the key gene for acetogenesis (Supplementary Figure S5).
Methanogens
When the corrosive community reached the fourth transfer on Fe0, using shotgun metagenomics, we identified two major methanogenic groups: Methanomicrobia (48% of Archaea; 3.2% of assembled prokaryotic reads, 1 assembled bin) and Methanobacteria (38.5% of Archaea; 2.6% of assembled prokaryotic reads, 1 assembled bin). The Methanobacteria metagenome reads provided the best coverage of the CO2-reducing methanogenesis pathway (Supplementary Table S6 and Figure 7).
We could assemble two bins or metagenome-assembled genomes, one Methanomicrobia related to Methanosaeta/Methanothrix, and the other a Methanobacteria related to Methanobacterium/Methanothermobacter. MG-RAST assigned the Methanobacteria bin to Methanothermobacter, a former Methanobacterium (Wasserfallen et al., 2000). However, analyses of the key gene for methanogenesis (mcrA—for methyl coenzyme M reductase) designated the bin to a Methanobacterium (Figure 6 and Supplementary Table S3). In fact, the two mcrAs in the Methanobacteria-bin 9 were more similar to the mcrA of described Methanobacterium species, one being 98% identical to the mcrA of M. formicicum, the other 96.6% to Methanobacterium sp. NBRC 105039. Both were only distantly related to the mcrA of Methanothermobacter thermoautotrophicus with 89.3 and 85.5% amino acid identity, respectively. The mcrA of Methanosaeta/Methanothrix bin eight was very closely related to that of Methanothrix shoehngenii (99.5% amino acid identity).
At the 11th transfer on Fe0, the lake community was represented solely by Methanobacterium according to amplicon sequencing of both the V4 and V3–V5 regions of the 16S rRNA gene. We could not detect any Methanomicrobia during this latter transfer, indicating that only Methanobacterium species survived 11 transfers on Fe0. Unfortunately, we could not match the V4 or V3–V5 regions to previously identified Methanobacterium bins because they did not contain a 16S rRNA gene.
Methanobacterium/Methanothermobacter are often associated with biofilms formed on Fe0 infrastructure like corroded sea-submerged railway lines (Usher et al., 2014b) or oil pipelines and oil facility infrastructure (Duncan et al., 2009; Lenchi et al., 2013; Liang et al., 2014; Okoro et al., 2017; Suarez et al., 2019; Lahme et al., 2020; Su et al., 2020). Sometimes, Methanobacterium/Methanothermobacter are implicated in severe corrosion (Lahme et al., 2020). Other times are thought to help form a protective layer against corrosion (in’t Zandt et al., 2019). Several corrosive Methanobacterium have been isolated from various environments, like North sea coastal sediments (Dinh et al., 2004) or an oil storage tank in Japan (Uchiyama et al., 2010), and several Methanothermobacter species (formerly known as thermophilic Methanobacterium) have been previously shown capable of Fe0 corrosion in pure culture [e.g., M. thermoautotrophicum (Daniels et al., 1987; Karr, 2013) or M. thermoflexus (Mayhew et al., 2016)]. On the other hand, a Methanothermobacter strain isolated from a corroded oil pipeline could not corrode Fe0 alone and required partnering with other microorganisms (Davidova et al., 2012).
Recently, Dutch researchers suggested that Methanobacteriales, including Methanobacterium and Methanothermobacter, have anticorrosive properties (in’t Zandt et al., 2019). It has been afterward debated whether Methanobacterium/Methanothermobacter induce Fe0 corrosion. However, in our enrichments, methanogens, best represented by Methanobacterium/Methanothermobacter, have an active role in Fe0 corrosion within the microbial community, upholding Fe0-dependent methanogenesis months after acetogenic activity ceased (Figure 3).
Possible Mechanism of Electron Uptake During Fe0 Corrosion
Methanogens showed four to five times higher rates in the presence of acetogens than alone, when methanogenesis rates were fully explained by abiotic H2. It is therefore unlikely that methanogens in this corrosive microbiome have an inherent mechanism of electron uptake from Fe0. Indeed, we found no evidence in the metagenome that methanogens had the potential to accelerate electron uptake from Fe0 as they did not present the MIC island specific to highly corrosive methanogens (Tsurumaru et al., 2018). [NiFe]-hydrogenases on the MIC island of methanogens promotes corrosion only when encoded on the genomic island (MIC island) between secretory proteins, which apparently help the hydrogenase on its way out of the cell to the extracellular electron donor (Tsurumaru et al., 2018). Hydrogenotrophic methanogens also contain an enzyme supercomplex (Mvh/Hdr: methyl viologen reducing hydrogenase/heterodisulfide reductase) required for energy metabolism, which can function outside the cells evolving H2 or formate from Fe0 (Lienemann et al., 2018). Nonetheless, there is no supporting evidence that the Hdr supercomplex gets excreted or that it has a particular role in corrosion by methanogens since all hydrogenotrophic methanogens contain this supercomplex, but not all are corrosive.
Methanogens in this corrosive microbiome appear to benefit from the presence of acetogens by mechanisms that could not be fully explained by acetate turnover.
To further explain the methanogens’ dependency on acetogens, we looked into possible strategies acetogens may use to enhance extracellular electron retrieval from Fe0, which would also benefit neighboring cells. These are:
(1) enzymes (e.g., hydrogenases) lowering the activation energy for Fe0 electron oxidation to form H2 (Deutzmann et al., 2015; Rouvre and Basseguy, 2016; Lienemann et al., 2018).
(2) cell-associated metals and peptides may act as organometallic catalysts lowering the activation energy for the H2 evolution reaction. In fact, streamlined organometallic molecules (e.g., diiron oxadithiolate) mimicked the chemistry of the H2 evolution reaction by hydrogenase enzymes (Song et al., 2005). Interestingly, biocathodes with killed cell biomass retained peptides and metals on the cathode surface promoting H2 evolution (Yates et al., 2014). These previous observations together mean that organometallic-rich cell deposits on the Fe0 surface may act similar to hydrogenases in promoting the H2 evolution reaction.
(3) shuttles (e.g., flavins) may cycle electrons between Fe0 and cells and induce corrosion. Shuttles (e.g., flavins) have been involved in extracellular electron transfer in many Firmicutes including Clostridia (Light et al., 2018), but their role in corrosion and whether they could be used by methanogens have, to our knowledge, never been reported.
In our enrichments, cell filtrate from a 10-day-old culture stimulated four-fold the H2 evolution reaction from Fe0 and three-fold when heat inactivated (Figure 4). Thus, the spent media filtrate contains excreted thermolabile and non-thermolabile catalysts that promote the H2 evolution reaction. The thermolabile cell exudates could be enzymes or shuttles (e.g., flavins) while the non-thermolabile constituents could be metal/peptide cell debris.
Because thermolabile constituents from the media (e.g., hydrogenases, nitrogenases) partially explained enhanced H2 evolution from Fe0, we screened the assembled metagenome for enzymes and shuttles.
Enzymes: In biological systems, H2-evolving reactions are typically catalyzed by ferredoxin-dependent hydrogenases (EC: 1.12.7.2 like the diiron-containing Clostridial hydrogenases ([FeFe]-hydrogenases). The [FeFe]-hydrogenases of acetogens have characteristic high H2 evolution rates (Adams, 1990) and take up electrons directly from Fe0 (Mehanna et al., 2008; Rouvre and Basseguy, 2016). Other H2-evolving enzymes are nitrogenases, which release H2 as a side product of the dinitrogen fixing reaction and have been confirmed by genome-wide transcriptomics to play a role in H2 evolution in Clostridium species (Calusinska et al., 2015). Both [FeFe]-hydrogenase and nitrogenases were well represented in the Clostridia in this corrosive microbiome (Figure 8 and Supplementary Figure S6). In fact, [FeFe]-hydrogenase enzymes (classes A–C) could be matched to all six Clostridium bins and also to one Parabacteroides bin (Figure 8).
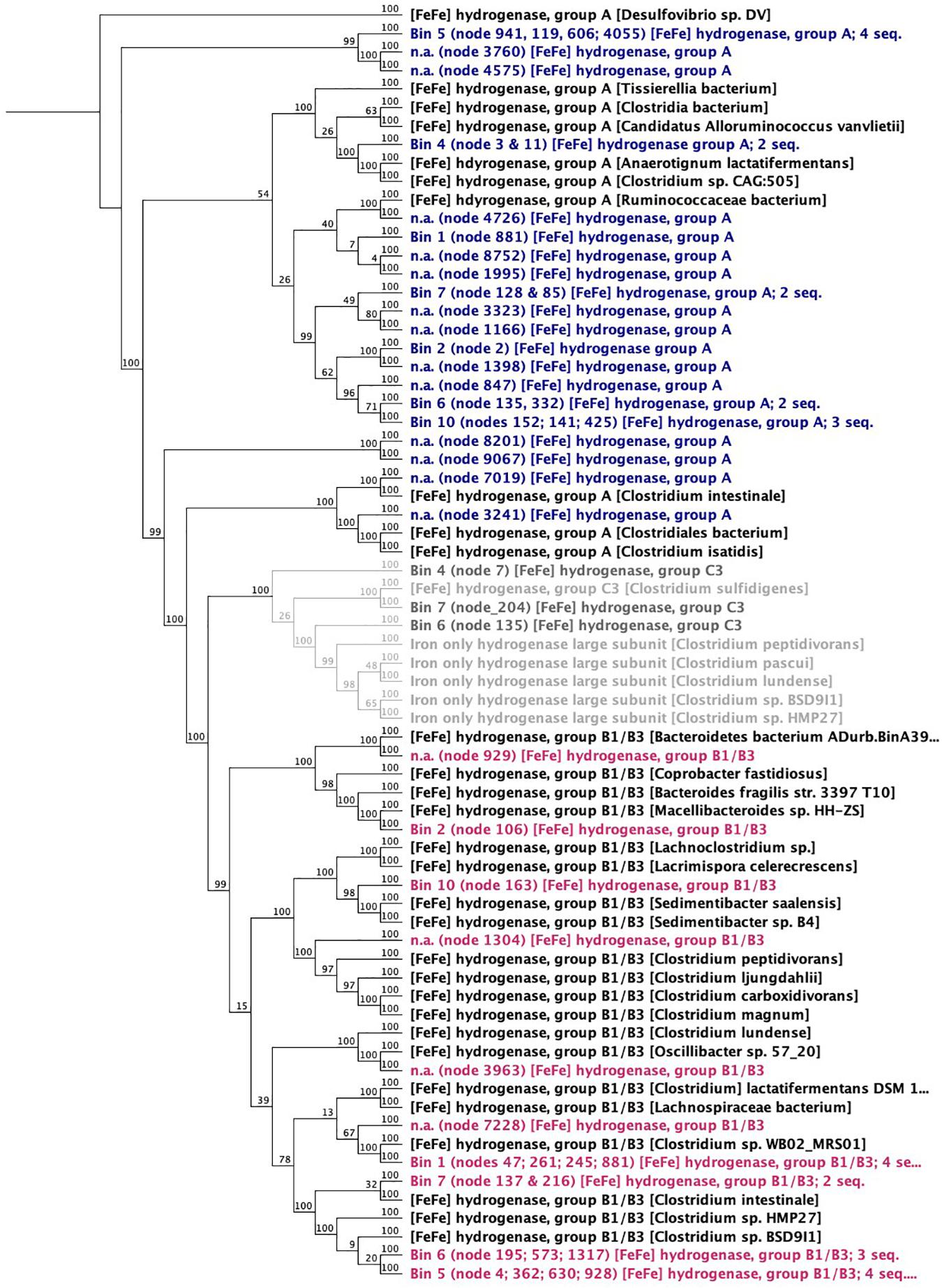
Figure 8. Neighbor joining dendrogram of amino acid sequences of [FeFe]-hydrogenases. Numbers close to nodes indicate bootstrap probability (%) based on 100 replicates. The outgroup root is a Deltaproteobacteria—a Desulfovibrio sp.
One possible candidate for effective H2 evolution from Fe0 is the ferredoxin-dependent hydrogenases (hydrogen-evolving hydrogenase HydA; EC:1.12.7.2). When screening the metagenome, HydA was found exclusively (100%) in Clostridia (87% affiliated to Clostridium and 7% to Eubacterium).
Since Clostridium excrete enzymes (and toxins) extracellularly to carry out lytic functions (Revitt-Mills et al., 2015), some may have evolved the capacity to excrete hydrogenases, promoting access to insoluble food sources like Fe0.
Shuttles: Other possible secreted constituents in the spent media are flavins, which can be used as shuttles between cells and Fe0. Although reports are scarce (Fuller et al., 2014), Clostridia can produce and excrete flavins for extracellular electron transfer. In this corrosive microbiome, we could identify almost a full pathway for the biosynthesis of riboflavin, FMN and FAD in Clostridia (Supplementary Figure S7). It is therefore possible that Clostridia stimulates electron uptake from Fe0 also by using flavin shuttles. However, it is unclear how flavins may stimulate methanogenic rates, since, to our knowledge, there are no reports that flavins act as shuttles to promote methanogenesis.
In other words, the results above could explain why the metabolism of methanogens favorably influenced acetogens. It is most likely that Methanobacterium methanogens became stimulated by extracellular Clostridial enzymes, which promote, without discrimination, any H2/CO2 metabolisms in the enrichment. Once outside the cell, extracellular Clostridial enzymes capture Fe0 electrons to evolve H2. H2 is then a common resource. Thus, excreted and freely available catalytic molecules that promote the H2 evolution reaction on Fe0 support a methanogen/win–acetogen/loss scenario (Figure 9).
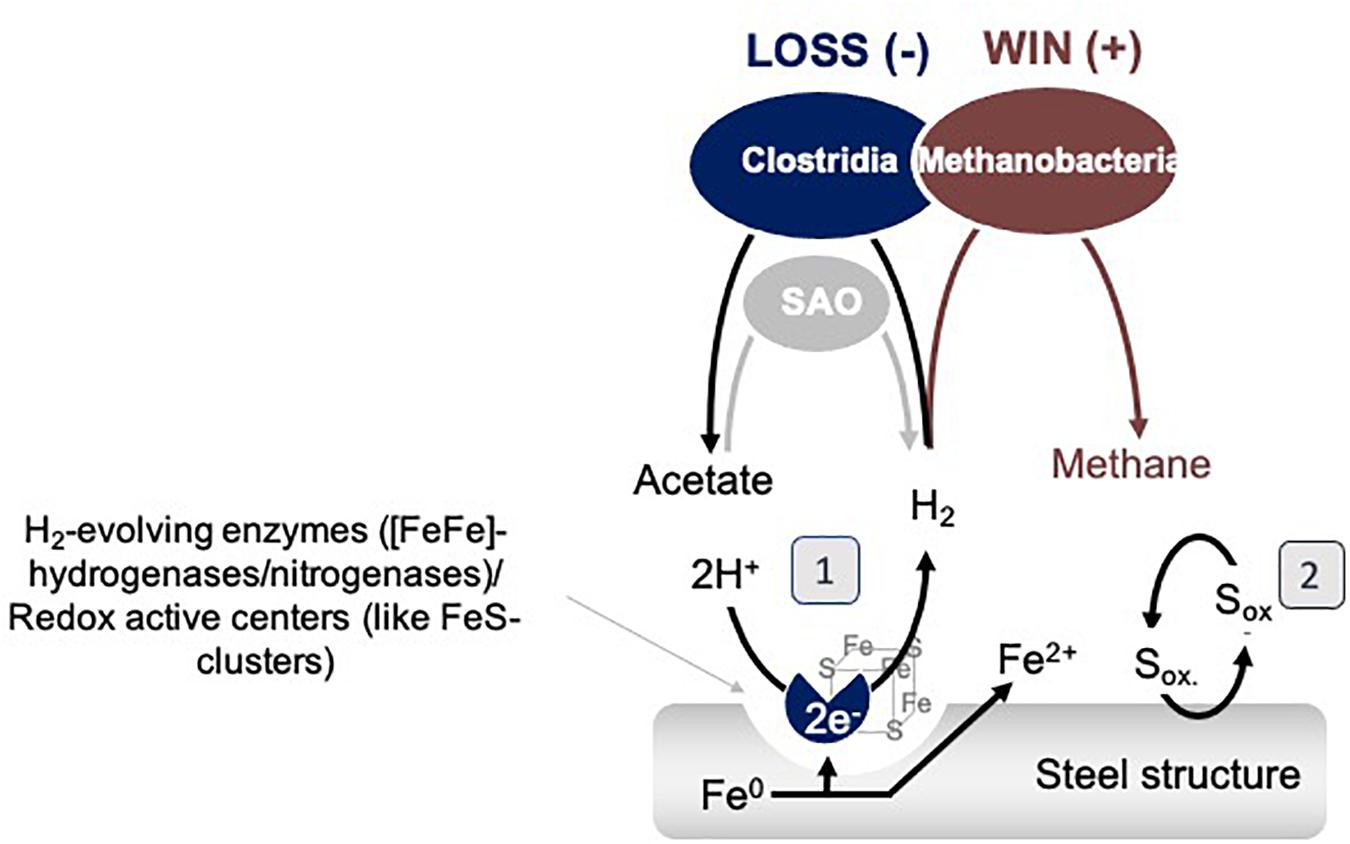
Figure 9. Model interspecies interactions on Fe0 between Clostridia and Methanobacteria. SAO represents syntrophic acetate oxidizing bacteria. (1) represents a mechanism based on catalytic constituents released by cells which could promote the H2 evolution reaction, and (2) represents a shuttle based mechanism of interaction.
Possible explanations for why Methanobacterium become even more favored by the termination of acetogenesis are that: (1) during the late stationary, Clostridial cells lyse, releasing more enzymes/redox-active proteins and thereby promoting H2 evolution and the growth of hydrogenotrophic methanogens, or (2) some Clostridia switch to syntrophic acetate oxidation (SAO) engaging Methanobacterium further as a syntrophic partner (Figure 9).
Additional examinations of the interactions at the Fe0 surface and the identity of the thermolabile and non-thermolabile constituents that promote H2 evolution from Fe0 remain to be resolved in future studies.
The bottle experiments presented here mimic the static freshwater environment of lake sediment conditions, informing about the type of microorganisms and interactions that may accelerate corrosion in such environments. However, we cannot juxtapose these bottle results to a natural environment, where other environmental factors may play a role. Therefore, in situ studies of corrosion in such environments are necessary and could be guided by a better understanding of the corrosive species present in these environments.
Conclusion
Interspecies interactions on Fe0 have been insufficiently investigated. Here, we bring evidence for a win–loss type of interaction on Fe0 between methanogens (esp. Methanobacterium) and acetogens (Clostridia) enriched from climate lake sediments.
Acetogens were more effective Fe0 corroders and acetate producers when decoupled from methanogens with the help of a specific inhibitor. Methanogens, on the other hand, became less effective methane producers when decoupled from acetogens with a specific inhibitor.
When both groups were together, the intact community exhibited metabolic rates beyond what abiotic H2 could explain. Since Clostridia are known to release molecules and enzymes extracellularly, we tested whether extracellular enzymes/shuttles may mediate H2 evolution from Fe0. Undeniably, filtered spent media filtrate promoted H2 evolution from Fe0 four-fold compared to abiotic controls, partially via thermolabile constituents (enzymes/flavins), partially via undefined non-thermolabile constituents. We screened the metagenome for potential enzyme candidates and identified Clostridial [FeFe] hydrogenases, known to be effective in retrieving electrons directly from Fe0. Additionally, we found an almost complete flavin-biosynthesis pathway in Clostridia. We could not find genomic evidence for enzymatic mediated electron uptake typical of certain methanogens (the MIC island was absent). Besides, it was puzzling that methanogenic rates jumpstarted (above what is expected from abiotic H2) only when methanogens co-existed with acetogens, especially when acetogens ceased their activity. The higher rates could be somewhat explained by the late stationary release of extracellular Clostridial hydrogenases that promote H2 evolution. Another explanation is the metabolic reversal of some Clostridial metabolism from CO2-reducing acetogenesis to acetate oxidation when the Clostridia would have to be coupled syntrophically to the methanogens. These suggestions need to be further validated experimentally.
Some studies suggested that methanogens like Methanobacteria may have a protective role during corrosion (in’t Zandt et al., 2019). Here, we show that such methanogens can upkeep corrosion in biofilms where acetogens are present, even after acetogenesis ceases. Therefore, we recommend that the corrosive effects of methanogens should be investigated not only in pure culture but also in consortia with acetogenic partners before suggesting they have a protective anticorrosive role.
Data Availability Statement
The datasets presented in this study can be found in online repositories. The names of the repository/repositories and accession number(s) can be found in the article/Supplementary Material.
Author Contributions
A-ER and PP developed the idea. PP carried out all wet-lab experimental work and some of the analyses and drafted the manuscript. A-ER carried out most of the data analyses and wrote the manuscript. WF carried out the metagenome assembly and some of the analyses. All authors contributed to editing the last version of the manuscript.
Funding
This is a contribution to a Sapere Aude Danish Research Council grant awarded to A-ER (grant number 4181-00203). WF has been funded by a Villum experiment grant no. 00028022.
Conflict of Interest
The authors declare that the research was conducted in the absence of any commercial or financial relationships that could be construed as a potential conflict of interest.
Acknowledgments
We would like to thank Oona Snoeyenbos-West, Carolin Löscher, Satoshi Kawaichi, and Christian Furbo Reeder for help with sampling and valuable discussions. We would also like to thank Joy Ward for help with preparing samples for scanning electron microscopy and we would like to recognize the University of Massachusetts electron microscopy facility that provided access and training of PP on the FEI Magellan XHR-SEM.
Supplementary Material
The Supplementary Material for this article can be found online at: https://www.frontiersin.org/articles/10.3389/fmicb.2021.638282/full#supplementary-material
References
Adams, M. W. W. (1990). The structure and mechanism of iron-hydrogenases. Biochim. Biophys. Acta 1020, 115–145. doi: 10.1016/0005-2728(90)90044-5
Albertsen, M., Karst, S. M., Ziegler, A. S., Kirkegaard, R. H., and Nielsen, P. H. (2015). Back to basics–the influence of DNA extraction and primer choice on phylogenetic analysis of activated sludge communities. PLoS One 10:e0132783. doi: 10.1371/journal.pone.0132783
Apprill, A., McNally, S., Parsons, R., and Weber, L. (2015). Minor revision to V4 region SSU rRNA 806R gene primer greatly increases detection of SAR11 bacterioplankton. Aquat. Microb. Ecol. 75, 129–137. doi: 10.3354/ame01753
Araujo, J. C., Téran, F. C., Oliveira, R. A., Nour, E. A. A., Montenegro, M. A. P., Campos, J. R., et al. (2003). Comparison of hexamethyldisilazane and critical point drying treatments for SEM analysis of anaerobic biofilms and granular sludge. J. Electron. Microsc. 52, 429–433. doi: 10.1093/jmicro/52.4.429
Arriba-Rodriguez, L., Villanueva-Balsera, J., Ortega-Fernandez, F., and Rodriguez-Perez, F. (2018). Methods to evaluate corrosion in buried steel structures: a review. Metals 8:334. doi: 10.3390/met8050334
Beese-Vasbender, P. F., Grote, J.-P., Garrelfs, J., Stratmann, M., and Mayrhofer, K. J. J. (2015). Selective microbial electrosynthesis of methane by a pure culture of a marine lithoautotrophic archaeon. Bioelectrochemistry 102, 50–55. doi: 10.1016/j.bioelechem.2014.11.004
Belay, N., and Daniels, L. (1990). Elemental metals as electron sources for biological methane formation from COz. Antonie Van Leeuwenhoek 57, 1–7. doi: 10.1007/bf00400329
Bengelsdorf, F. R., Beck, M. H., Erz, C., Hoffmeister, S., Karl, M. M., Riegler, P., et al. (2018). Bacterial anaerobic synthesis gas (syngas) and CO2+ H2 fermentation. Adv. Appl. Microbiol. 103, 143–221. doi: 10.1016/bs.aambs.2018.01.002
Bolger, A. M., Lohse, M., and Usadel, B. (2014). Trimmomatic: a flexible trimmer for Illumina sequence data. Bioinformatics 30, 2114–2120. doi: 10.1093/bioinformatics/btu170
Boopathy, R., and Daniels, L. (1991). Effect of pH on anaerobic mild steel corrosion by methanogenic bacteria. Appl. Environ. Microbiol. 57, 2104–2108. doi: 10.1128/AEM.57.7.2104-2108.1991
Brandt, J., and Albertsen, M. (2018). Investigation of detection limits and the influence of DNA extraction and primer choice on the observed microbial communities in drinking water samples using 16S rRNA gene amplicon sequencing. Front. Microbiol. 9:2140. doi: 10.3389/fmicb.2018.02140
Calusinska, M., Hamilton, C., Monsieurs, P., Mathy, G., Leys, N., Franck, F., et al. (2015). Genome-wide transcriptional analysis suggests hydrogenase- and nitrogenase-mediated hydrogen production in Clostridium butyricum CWBI 1009. Biotechnol. Biofuels 8:27. doi: 10.1186/s13068-015-0203-5
Caporaso, J. G., Kuczynski, J., Stombaugh, J., Bittinger, K., Bushman, F. D., Costello, E. K., et al. (2010). QIIME allows analysis of high-throughput community sequencing data. Nat. Methods 7, 335–336.
Daniels, L., Belay, N., Rajagopal, B., and Weimer, P. (1987). Bacterial methanogenesis and growth from CO2 with elemental iron as the sole source of electrons. Science 237, 509–511. doi: 10.1126/science.237.4814.509
Davidova, I. A., Duncan, K. E., Perez-Ibarra, B. M., and Suflita, J. M. (2012). Involvement of thermophilic archaea in the biocorrosion of oil pipelines. Environ. Microbiol. 14, 1762–1771. doi: 10.1111/j.1462-2920.2012.02721.x
Deutzmann, J. S., Sahin, M., and Spormann, A. M. (2015). Extracellular enzymes facilitate electron uptake in biocorrosion and bioelectrosynthesis. mBio 6:e00496-15. doi: 10.1128/mBio.00496-15
Dinh, H. T., Kuever, J., Mußmann, M., Hassel, A. W., Stratmann, M., and Widdel, F. (2004). Iron corrosion by novel anaerobic microorganisms. Nature 427, 829–832. doi: 10.1038/nature02321
Drake, H. L., Küsel, K., and Matthies, C. (2002). Ecological consequences of the phylogenetic and physiological diversities of acetogens. Antonie Van Leeuwenhoek 81, 203–213. doi: 10.1023/A:1020514617738
DSMZ (2014). 120. Methanosarcina Medium. Available online at: https://www.dsmz.de/microorganisms/medium/pdf/DSMZ_Medium120.pdf (accessed March 10, 2021).
Duncan, K. E., Gieg, L. M., Parisi, V. A., Tanner, R. S., Tringe, S. G., Bristow, J., et al. (2009). Biocorrosive thermophilic microbial communities in alaskan north slope oil facilities. Environ. Sci. Technol. 43, 7977–7984. doi: 10.1021/es9013932
Edgar, R. C. (2004). MUSCLE: multiple sequence alignment with high accuracy and high throughput. Nucleic Acids Res. 32, 1792–1797. doi: 10.1093/nar/gkh340
Edgar, R. C. (2013). UPARSE: highly accurate OTU sequences from microbial amplicon reads. Nat. Methods 10, 996–998. doi: 10.1038/nmeth.2604
Eitel, M., Francis, W. R., Varoqueaux, F., Daraspe, J., Osigus, H. J., Krebs, S., et al. (2018). Comparative genomics and the nature of placozoan species. PLoS Biol. 16:e2005359. doi: 10.1371/journal.pbio.2005359
Enning, D., and Garrelfs, J. (2014). Corrosion of iron by sulfate-reducing bacteria: new views of an old problem. Appl. Environ. Microbiol. 80, 1226–1236. doi: 10.1128/AEM.02848-13
Fuller, S. J., McMillan, D. G. G., Renz, M. B., Schmidt, M., Burke, I. T., and Stewart, D. I. (2014). Extracellular electron transport-mediated Fe (III) reduction by a community of alkaliphilic bacteria that use flavins as electron shuttles. Appl. Environ. Microbiol. 80, 128–137. doi: 10.1128/aem.02282-13
Hyatt, D., Chen, G.-L., LoCascio, P. F., Land, M. L., Larimer, F. W., and Hauser, L. J. (2010). Prodigal: prokaryotic gene recognition and translation initiation site identification. BMC Bioinformatics 11:119. doi: 10.1186/1471-2105-11-119
Illumina, I. (2015). 16S Metagenomic Sequencing Library Preparation, part# 15044223 Rev. B 1213, 1214. Available online at: https://support.illumina.com/documents/documentation/chemistry_documentation/16s/16s-metagenomic-library-prep-guide-15044223-b.pdf (accessed March 10, 2021).
in’t Zandt, M. H., Kip, N., Frank, J., Jansen, S., van Veen, J. A., Jetten, M. S. M., et al. (2019). High-level abundances of Methanobacteriales and Syntrophobacterales may help to prevent corrosion of metal sheet piles. Appl. Environ. Microbiol. 85:e01369-19. doi: 10.1128/AEM.01369-19
Kanehisa, M., Sato, Y., and Morishima, K. (2016). BlastKOALA and GhostKOALA: KEGG tools for functional characterization of genome and metagenome sequences. J. Mol. Biol. 428, 726–731. doi: 10.1016/j.jmb.2015.11.006
Karr, E. A. (2013). Examination of metal corrosion by Desulfomicrobium thermophilum, Archaeoglobus fulgidus, and Methanothermobacter thermautotrophicus. Bios 84, 59–64. doi: 10.1893/0005-3155-84.2.59
Kato, S., Yumoto, I., and Kamagata, Y. (2015). Isolation of acetogenic bacteria that induce biocorrosion by utilizing metallic iron as the sole electron donor. Appl. Environ. Microbiol. 81, 67–73. doi: 10.1128/AEM.02767-14
Katoh, K., Misawa, K., Kuma, K., and Miyata, T. (2002). MAFFT: a novel method for rapid multiple sequence alignment based on fast Fourier transform. Nucleic Acids Res. 30, 3059–3066. doi: 10.1093/nar/gkf436
Katoh, K., and Standley, D. M. (2013). MAFFT multiple sequence alignment software version 7: improvements in performance and usability. Mol. Biol. Evol. 30, 772–780. doi: 10.1093/molbev/mst010
Kearse, M., Moir, R., Wilson, A., Stones-Havas, S., Cheung, M., Sturrock, S., et al. (2012). Geneious Basic: an integrated and extendable desktop software platform for the organization and analysis of sequence data. Bioinformatics 28, 1647–1649. doi: 10.1093/bioinformatics/bts199
Kotsyurbenko, O. R., Glagolev, M. V., Nozhevnikova, A. N., and Conrad, R. (2001). Competition between homoacetogenic bacteria and methanogenic archaea for hydrogen at low temperature. FEMS Microbiol. Ecol. 38, 153–159. doi: 10.1111/j.1574-6941.2001.tb00893.x
Labelle, E. V., Marshall, C. W., Gilbert, J. A., and May, H. D. (2014). Influence of acidic pH on hydrogen and acetate production by an electrosynthetic microbiome. PLoS One 9:e0109935. doi: 10.1371/journal.pone.0109935
Lahme, S., Mand, J., Longwell, J., Smith, R., and Enning, D. (2020). Severe corrosion of carbon steel in oil field produced water can be linked to methanogenic archaea containing a special type of [NiFe] hydrogenase. Appl. Environ. Microbiol. 1, 2–7. doi: 10.1128/AEM.01819-20
Lenchi, N., Inceoglu, Ö., Kebbouche-Gana, S., Gana, M. L., Llirós, M., Servais, P., et al. (2013). Diversity of microbial communities in production and injection waters of algerian oilfields revealed by 16S rRNA Gene Amplicon 454 pyrosequencing. PLoS One 8:e0066588. doi: 10.1371/journal.pone.0066588
Liang, R., Grizzle, R. S., Duncan, K. E., McInerney, M. J., and Suflita, J. M. (2014). Roles of thermophilic thiosulfate-reducing bacteria and methanogenic archaea in the biocorrosion of oil pipelines. Front. Microbiol. 5:89. doi: 10.3389/fmicb.2014.00089
Lienemann, M., Deutzmann, J. S., Milton, R. D., Sahin, M., and Spormann, A. M. (2018). Mediator-free enzymatic electrosynthesis of formate by the Methanococcus maripaludis heterodisulfide reductase supercomplex. Bioresour. Technol. 254, 278–283. doi: 10.1016/j.biortech.2018.01.036
Light, S. H., Su, L., Rivera-Lugo, R., Cornejo, J. A., Louie, A., Iavarone, A. T., et al. (2018). A flavin-based extracellular electron transfer mechanism in diverse Gram-positive bacteria. Nature 562, 140–144. doi: 10.1038/s41586-018-0498-z
Lorowitz, W. H., Nagle, D. P., and Tanner, R. S. (1992). Anaerobic oxidation of elemental metals coupled to methanogenesis by Methanobacterium thermoautotrophicum. Environ. Sci. Technol. 26, 1606–1610. doi: 10.1021/es00032a018
Lu, S., Wang, J., Chitsaz, F., Derbyshire, M. K., Geer, R. C., Gonzales, N. R., et al. (2020). CDD/SPARCLE: the conserved domain database in 2020. Nucleic Acids Res. 48, D265–D268.
Ma, L., Zhou, L., Ruan, M.-Y., Gu, J.-D., and Mu, B.-Z. (2019). Simultaneous methanogenesis and acetogenesis from the greenhouse carbon dioxide by an enrichment culture supplemented with zero-valent iron. Renew. Energy 132, 861–870. doi: 10.1016/j.renene.2018.08.059
Magoè, T., and Salzberg, S. L. (2011). FLASH: fast length adjustment of short reads to improve genome assemblies. Bioinformatics 27, 2957–2963. doi: 10.1093/bioinformatics/btr507
Mand, J., Park, H. S., Jack, T. R., and Voordouw, G. (2014). The role of acetogens in microbially influenced corrosion of steel. Front. Microbiol. 5:268. doi: 10.3389/fmicb.2014.00268
Mand, J., Park, H. S., Okoro, C., Lomans, B. P., Smith, S., Chiejina, L., et al. (2016). Microbial methane production associated with carbon steel corrosion in a Nigerian oil field. Front. Microbiol. 6:1538. doi: 10.3389/fmicb.2015.01538
Marchler-Bauer, A., and Bryant, S. H. (2004). CD-Search: protein domain annotations on the fly. Nucleic Acids Res. 32, W327–W331.
Marshall, C. W., Ross, D. E., Fichot, E. B., Norman, R. S., and May, H. D. (2012). Electrosynthesis of commodity chemicals by an autotrophic microbial community. Appl. Environ. Microbiol. 78, 8412–8420. doi: 10.1128/AEM.02401-12
Marshall, C. W., Ross, D. E., Fichot, E. B., Norman, R. S., and May, H. D. (2013). Long-term operation of microbial electrosynthesis systems improves acetate production by autotrophic microbiomes. Environ. Sci. Technol. 47, 6023–6029. doi: 10.1021/es400341b
Mayhew, L. E., Lau, G. E., and Templeton, A. S. (2016). Distinct geochemistries of water-basalt-Fe0 reactions in the presence versus absence of CO2-driven microbial methanogenesis. Chem. Geol. 428, 92–105. doi: 10.1016/j.chemgeo.2016.02.028
Mehanna, M., Basseguy, R., Delia, M. L., Girbal, L., Demuez, M., and Bergel, A. (2008). New hypotheses for hydrogenase implication in the corrosion of mild steel. Electrochim. Acta 54, 140–147. doi: 10.1016/j.electacta.2008.02.101
Mehanna, M., Rouvre, I., Delia, M.-L., Feron, D., Bergel, A., and Basseguy, R. (2016). Discerning different and opposite effects of hydrogenase on the corrosion of mild steel in the presence of phosphate species. Bioelectrochemistry 111, 31–40. doi: 10.1016/j.bioelechem.2016.04.005
Meyer, F., Paarmann, D., D’Souza, M., Olson, R., Glass, E., Kubal, M., et al. (2008). The metagenomics RAST server – a public resource for the automatic phylogenetic and functional analysis of metagenomes. BMC Bioinformatics 9:386. doi: 10.1186/1471-2105-9-386
Mishra, B. K., Chakraborty, S., Kumar, P., and Saraswat, C. (2020). “Urban stormwater management: practices and governance,” in Sustainable Solutions for Urban Water Security: Innovative Studies, eds B. K. Mishra, S. Chakraborty, P. Kumar, and C. Saraswat (Cham: Springer), 115–146. doi: 10.1007/978-3-030-53110-2_6
Mohanakrishna, G., Seelam, J. S., Vanbroekhoven, K., and Pant, D. (2015). An enriched electroactive homoacetogenic biocathode for the microbial electrosynthesis of acetate through carbon dioxide reduction. Faraday Discuss. 183, 445–462. doi: 10.1039/c5fd00041f
Monroy, O. A. R., Gayosso, M. J. H., Ordaz, N. R., Olivares, G. Z., and Ramírez, C. J. (2011). Corrosion of API XL 52 steel in presence of Clostridium celerecrescens. Mater. Corros. 62, 878–883. doi: 10.1002/maco.200905582
Mori, K., Tsurumaru, H., and Harayama, S. (2010). Iron corrosion activity of anaerobic hydrogen-consuming microorganisms isolated from oil facilities. J. Biosci. Bioeng. 110, 426–430. doi: 10.1016/j.jbiosc.2010.04.012
Nevin, K. P., Hensley, S. A., Franks, A. E., Summers, Z. M., Ou, J., Woodard, T. L., et al. (2011). Electrosynthesis of organic compounds from carbon dioxide is catalyzed by a diversity of acetogenic microorganisms. Appl. Environ. Microbiol. 77, 2882–2886. doi: 10.1128/AEM.02642-10
Nurk, S., Meleshko, D., Korobeynikov, A., and Pevzner, P. A. (2017). MetaSPAdes: a new versatile metagenomic assembler. Genome Res. 27, 824–834. doi: 10.1101/gr.213959.116
Okoro, C. C., Samuel, O., and Lin, J. (2017). Substrate availability, pH, and temperature influence Methanogenesis and mild steel corrosion. Geomicrobiol. J. 34, 729–736. doi: 10.1080/01490451.2016.1257662
Palacios, P. A., Snoeyenbos-West, O., Löscher, C. R., Thamdrup, B., and Rotaru, A.-E. (2019). Baltic Sea methanogens compete with acetogens for electrons from metallic iron. ISME J. 13, 3011–3023. doi: 10.1038/s41396-019-0490-0
Patil, S. A., Arends, J. B. A., Vanwonterghem, I., van Meerbergen, J., Guo, K., Tyson, G. W., et al. (2015). Selective enrichment establishes a stable performing community for microbial electrosynthesis of acetate from CO2. Environ. Sci. Technol. 49, 8833–8843. doi: 10.1021/es506149d
Peters, J. W., Schut, G. J., Boyd, E. S., Mulder, D. W., Shepard, E. M., Broderick, J. B., et al. (2015). [FeFe]- and [NiFe]-hydrogenase diversity, mechanism, and maturation. Biochim. Biophys. Acta 1853, 1350–1369. doi: 10.1016/j.bbamcr.2014.11.021
Phelps, T. J., and Zeikus, J. G. (1984). Influence of pH on terminal carbon metabolism in anoxic sediments from a mildly acidic lake. Appl. Environ. Microbiol. 48, 1088–1095. doi: 10.1128/aem.48.6.1088-1095.1984
Philips, J., Monballyu, E., Georg, S., De Paepe, K., Prévoteau, A., Rabaey, K., et al. (2019). An Acetobacterium strain isolated with metallic iron as electron donor enhances iron corrosion by a similar mechanism as Sporomusa sphaeroides. FEMS Microbiol. Ecol. 95:fiy222. doi: 10.1093/femsec/fiy222
Pinto, A. J., and Raskin, L. (2012). PCR biases distort bacterial and archaeal community structure in pyrosequencing datasets. PLoS One 7:e43093. doi: 10.1371/journal.pone.0043093
Quast, C., Pruesse, E., Yilmaz, P., Gerken, J., Schweer, T., Yarza, P., et al. (2013). The SILVA ribosomal RNA gene database project: improved data processing and web-based tools. Nucleic Acids Res. 41, D590–D596. doi: 10.1093/nar/gks1219
Revitt-Mills, S. A., Rood, J. I., and Adams, V. (2015). Clostridium perfringens extracellular toxins and enzymes: 20 and counting. Microbiol. Aust. 36, 114–117. doi: 10.1071/ma15039
Rouvre, I., and Basseguy, R. (2016). Exacerbation of the mild steel corrosion process by direct electron transfer between [Fe-Fe]-hydrogenase and material surface. Corros. Sci. 111, 199–211. doi: 10.1016/j.corsci.2016.05.005
Skovhus, L. T., Enning, D., and Lee, J. S. (2017). Microbiologically Influenced Corrosion in the Upstream Oil and Gas Industry. Boca Raton, FL: CRC Press.
Søndergaard, D., Pedersen, C. N. S., and Greening, C. (2016). HydDB: a web tool for hydrogenase classification and analysis. Sci. Rep. 6:34212.
Song, L.-C., Yang, Z.-Y., Bian, H.-Z., Liu, Y., Wang, H.-T., Liu, X.-F., et al. (2005). Diiron oxadithiolate type models for the active site of iron-only hydrogenases and biomimetic hydrogen evolution catalyzed by Fe2 (μ-SCH2OCH2S-μ)(CO) 6. Organometallics 24, 6126–6135. doi: 10.1021/om0507373
Su, H., Tang, R., Peng, X., Gao, A., and Han, Y. (2020). Corrosion behavior and mechanism of carbon steel influenced by interior deposit microflora of an in-service pipeline. Bioelectrochemistry 132:107406. doi: 10.1016/j.bioelechem.2019.107406
Suarez, E. M., Lepkova, K., Kinsella, B., and Machuca, L. L. (2019). Aggressive corrosion of steel by a thermophilic microbial consortium in the presence and absence of sand. Int. Biodeterior. Biodegrad. 137, 137–146. doi: 10.1016/j.ibiod.2018.12.003
Tatusova, T., Ciufo, S., Federhen, S., Fedorov, B., McVeigh, R., O’Neill, K., et al. (2015). Update on RefSeq microbial genomes resources. Nucleic Acids Res. 43, D599–D605. doi: 10.1093/nar/gku1062
Tsurumaru, H., Ito, N., Mori, K., Wakai, S., Uchiyama, T., Iino, T., et al. (2018). An extracellular [NiFe] hydrogenase mediating iron corrosion is encoded in a genetically unstable genomic island in Methanococcus maripaludis. Sci. Rep. 8:15149. doi: 10.1038/s41598-018-33541-5
Uchiyama, T., Ito, K., Mori, K., Tsurumaru, H., and Harayama, S. (2010). Iron-corroding methanogen isolated from a crude-oil storage tank. Appl. Environ. Microbiol. 76, 1783–1788. doi: 10.1128/AEM.00668-09
Usher, K. M., Kaksonen, A. H., Cole, I., and Marney, D. (2014a). Critical review: microbially influenced corrosion of buried carbon steel pipes. Int. Biodeterior. Biodegrad. 93, 84–106. doi: 10.1016/j.ibiod.2014.05.007
Usher, K. M., Kaksonen, A. H., and MacLeod, I. D. (2014b). Marine rust tubercles harbour iron corroding archaea and sulphate reducing bacteria. Corros. Sci. 83, 189–197. doi: 10.1016/j.corsci.2014.02.014
Vigneron, A., Alsop, E. B., Chambers, B., Lomans, B. P., Head, I. M., and Tsesmetzis, N. (2016). Complementary microorganisms in highly corrosive biofilms from an offshore oil production facility. Appl. Environ. Microbiol. 82, 2545–2554. doi: 10.1128/AEM.03842-15
Wang, Q., Garrity, G. M., Tiedje, J. M., and Cole, J. R. (2007). Naive Bayesian classifier for rapid assignment of rRNA sequences into the new bacterial taxonomy. Appl. Environ. Microbiol. 73, 5261–5267. doi: 10.1128/aem.00062-07
Wasserfallen, A., Nölling, J., Pfister, P., Reeve, J., and De Macario, E. C. (2000). Phylogenetic analysis of 18 thermophilic Methanobacterium isolates supports the proposals to create a new genus, Methanothermobacter gen. nov., and to reclassify several isolates in three species, Methanothermobacter thermautotrophicus comb. nov., Methanothermobacter wolfeii comb. nov., and Methanothermobacter marburgensis sp. nov. Int. J. Syst. Evol. Microbiol. 50, 43–53. doi: 10.1099/00207713-50-1-43
Wiegel, J., Tanner, R., and Rainey, F. A. (2006). “An Introduction to the Family Clostridiaceae,” in The Prokaryotes: Volume 4: Bacteria: Firmicutes, Cyanobacteria, eds M. Dworkin, S. Falkow, E. Rosenberg, K.-H. Schleifer, and E. Stackebrandt (New York, NY: Springer), 654–678. doi: 10.1007/0-387-30744-3_20
Xu, H., Wang, K., and Holmes, D. E. (2014). Bioelectrochemical removal of carbon dioxide (CO2): an innovative method for biogas upgrading. Bioresour. Technol. 173, 392–398. doi: 10.1016/j.biortech.2014.09.127
Yates, M. D., Siegert, M., and Logan, B. E. (2014). Hydrogen evolution catalyzed by viable and non-viable cells on biocathodes. Int. J. Hydrogen Energy 39, 16841–16851. doi: 10.1016/j.ijhydene.2014.08.015
Yu, H. Y., Meade, A., and Liu, S. J. (2019). Phylogeny of Clostridium spp. based on conservative genes and comparisons with other trees. Microbiology 88, 469–478. doi: 10.1134/S002626171904012X
Zhang, T., Fang, H. H. P., and Ko, B. C. B. (2003). Methanogen population in a marine biofilm corrosive to mild steel. Appl. Microbiol. Biotechnol. 63, 101–106. doi: 10.1007/s00253-003-1396-2
Zhou, Z., Meng, Q., and Yu, Z. (2011). Effects of methanogenic inhibitors on methane production and abundances of methanogens and cellulolytic bacteria in in vitro ruminal cultures. Appl. Environ. Microbiol. 77, 2634–2639. doi: 10.1128/aem.02779-10
Keywords: microbial influenced corrosion, acetogens, methanogens, interspecies interactions, iron corrosion, Methanobacterium, Clostridium
Citation: Palacios PA, Francis WR and Rotaru A-E (2021) A Win–Loss Interaction on Fe0 Between Methanogens and Acetogens From a Climate Lake. Front. Microbiol. 12:638282. doi: 10.3389/fmicb.2021.638282
Received: 05 December 2020; Accepted: 29 March 2021;
Published: 13 May 2021.
Edited by:
Kylie Allen, Virginia Tech, United StatesReviewed by:
Katharine J. Thompson, University of British Columbia, CanadaErin Field, East Carolina University, United States
Copyright © 2021 Palacios, Francis and Rotaru. This is an open-access article distributed under the terms of the Creative Commons Attribution License (CC BY). The use, distribution or reproduction in other forums is permitted, provided the original author(s) and the copyright owner(s) are credited and that the original publication in this journal is cited, in accordance with accepted academic practice. No use, distribution or reproduction is permitted which does not comply with these terms.
*Correspondence: Amelia-Elena Rotaru, YXJvdGFydUBiaW9sb2d5LnNkdS5kaw==