- 1Department of Biology, University of Saskatchewan, Saskatoon, SK, Canada
- 2Department of Plant Sciences, Laval University, CRIV, Quebec City, QC, Canada
Plasmodiophora brassicae is a devastating obligate, intracellular, biotrophic pathogen that causes clubroot disease in crucifer plants. Disease progression is regulated by effector proteins secreted by P. brassicae. Twelve P. brassicae putative effectors (PbPEs), expressed at various stages of disease development [0, 2, 5, 7, 14, 21, and 28 days post inoculation (DPI)] in Arabidopsis and localizing to the plant endomembrane system, were studied for their roles in pathogenesis. Of the 12 PbPEs, seven showed an inhibitory effect on programmed cell death (PCD) as triggered by the PCD inducers, PiINF1 (Phytophthora infestans Infestin 1) and PiNPP1 (P. infestans necrosis causing protein). Showing the strongest level of PCD suppression, PbPE15, a member of the 2-oxoglutarate (2OG) and Fe (II)-dependent oxygenase superfamily and with gene expression during later stages of infection, appears to have a role in tumorigenesis as well as defense signaling in plants. PbPE13 produced an enhanced PiINF1-induced PCD response. Transient expression, in Nicotiana benthamiana leaves of these PbPEs minus the signal peptide (SP) (ΔspPbPEGFPs), showed localization to the endomembrane system, targeting the endoplasmic reticulum (ER), Golgi bodies and nucleo-cytoplasm, suggesting roles in manipulating plant cell secretion and vesicle trafficking. ΔspPbPE13GFP localized to plasma membrane (PM) lipid rafts with an association to plasmodesmata, suggesting a role at the cell-to-cell communication junction. Membrane relocalization of ΔspPbPE13GFP, triggered by flagellin N-terminus of Pseudomonas aeruginosa (flg22 – known to elicit a PAMP triggered immune response in plants), supports its involvement in raft-mediated immune signaling. This study is an important step in deciphering P. brassicae effector roles in the disruption of plant immunity to clubroot disease.
Introduction
Plasmodiophora brassicae is the intracellular obligate biotrophic plant pathogen responsible for clubroot disease in the Brassicaceae. The complex life cycle of P. brassicae can be divided into two infection stages: primary infection of a root hair resulting in secondary zoospore production and secondary infection of cortical tissues by secondary zoospores, leading to the production of resting spores and the characteristic swollen gall or club-shaped root of an infected plant (Rolfe et al., 2016). Secondary infection is crucial to the completion of the P. brassicae life cycle and the production of the next-generation of resting spores.
To facilitate the colonization of a plant root, P. brassicae secretes effector proteins to manipulate or interfere with the pathogen-induced host processes (Schwelm et al., 2015). Putative P. brassicae effector proteins, expressed during primary infection in canola as well as a secondary infection in Arabidopsis, have been identified through transcriptome analysis (Pérez-López et al., 2018, 2020; Chen et al., 2019). While the functional importance of many of these effectors remains unknown, a methyltransferase (PbBSMT) that methylates salicylic acid (SA), thereby disrupting SA-induced host defense pathways and increasing host susceptibility to P. brassicae infection was recently characterized (Ludwig-Müller et al., 2015; Bulman et al., 2019). Further, more recent reports have identified a P. brassicae MAPKKK protein as an elicitor for the generation of ROS and hypersensitive response (HR)-like cell death after transient expression in Nicotiana benthamiana (Jin et al., 2020) and a P. brassicae cysteine protease inhibitor SSPbP53 that targets cruciferous papain-like cysteine proteases to manipulate plant immunity (Pérez-López et al., Unpublished results).
To restrict an infection, plants have developed intricate coordinated networks of defense responses comprised of, pathogen-associated molecular patterns (PAMP)-triggered immunity (PTI), which when triggered results in cell death via the production of reactive oxygen species (ROS) and other mechanisms, and effector-triggered immunity (ETI), that together form the base of stable and long term resistance to pathogens (Hammond-Kosack and Jones, 1996; Jones and Dangl, 2006). Resistance to P. brassicae was first demonstrated in the two Arabidopsis ecotypes, Tsu-0 and Ze-0, with both showing an incompatible interaction to P. brassicae pathotype-e, characterized by a HR and lignification of the cell wall but no characteristic gall structures (Fuchs and Sacristan, 1996). In the evolutionary pathogen–host arms race for compatible interaction, successful pathogens most often target and subvert the tightly interconnected pathways such as protein synthesis, endomembrane trafficking and cellular degradation (autophagy and proteasome-mediated degradation) inside a host cell (Langin et al., 2020). The host counters, starting right at the plasma membrane (PM) with immune receptor activation, vacuolar vesicle trafficking and membrane fusion at the PM (Chinchilla et al., 2006; Teh and Hofius, 2014), endocytic recycling (Chinchilla et al., 2006), secretory pathway defense response (Bartetzko et al., 2009) and endomembrane relocalization of host proteins between membrane compartments (Engelhardt et al., 2012). The endomembrane system is a complex intracellular membrane network comprised of the endoplasmic reticulum (ER), Golgi apparatus, endosomes, vacuoles and PM, all connected via vesicle transport, that plays an important role in cellular homeostasis and signal transduction in response to external stimuli. Endomembrane trafficking and its membrane compartment dynamics are pivotal to limiting pathogen spread within the host and in turn, are often targeted by pathogen effectors to subvert host immunity (Gu et al., 2017).
The importance of nanodomains within the PM in early defense signaling and cell to cell communication has been well-documented in plant cells (Raffaele et al., 2009; Perraki et al., 2014; Gronnier et al., 2017; Sasaki et al., 2018; Albers et al., 2019). However, while PM lipid rafts are important in the activation of the human immune system, several intracellular pathogenic bacteria can hijack these rafts to facilitate entry into the host cell or modulate defense signaling for survival inside the cell (Mañes et al., 2003; Hartlova et al., 2010). The lipid and protein composition of plant lipid rafts are similar between plants and reflect that of animal lipid rafts, suggesting similar functions to those of animal lipid rafts, e.g., signal transduction and cellular trafficking (Morel et al., 2006; Lefebvre et al., 2007). Moreover, it has also been suggested that lipid rafts in root cells may have a role in symbiotic infection in Medicago truncatula (Lefebvre et al., 2007).
From a cDNA library generated from canola galls, we have identified a number of P. brassicae effectors that, by localizing to different sub-compartments of the plant cell endomembrane system, as well as the manipulation of plant-triggered programmed cell death (PCD), suggest a role in a successful P. brassicae infection and colonization of the plant root.
Materials and Methods
Plant Materials and Growth Conditions
Arabidopsis thaliana Columbia-0 plants, for inoculation and expression analysis by RT-PCR, were grown in Sunshine Mix #3 soil (Sun Gro Horticulture Inc., Vancouver, BC, Canada) at 22°C, 16 h light/8 h dark and a light intensity of 100 μmol photons m–2 s–1 in a Conviron E8 growth chamber (CMP6050 control system). N. benthamiana seeds were sown on soil and stratified for 2 days at 4°C before transferring to similar growing conditions as above. Transplanted seedlings were grown in a growth chamber under 16 h light/8 h dark, 25°C and light intensity of 160 μmol photons m–2 s–1 controlled conditions. Transplantation of both A. thaliana and N. benthamiana seedlings was done 10 days after germination.
Pathogen Materials, Inoculum Preparation, and Infection Assay
A single spore isolate of P. brassicae pathotype-3 (Strelkov et al., 2006), obtained from Dr. Gary Peng (AAFC, Saskatoon Research Centre), was propagated through Brassica napus cv. Westar (canola) plants. P. brassicae resting spores were extracted from 2 g of dry canola root galls by first submerging the gall in 0.25% Tween-20 solution for 5–7 min (Pérez-López et al., 2020). The gall was washed with 70% ethanol and twice with ddH2O, prior to grinding in a 10% sucrose solution using a mortar and pestle. The resulting suspension was passed through eight-layered cheesecloth and the filtrate was centrifuged at 100 rpm (Allegra 25R, Beckman Coulter Inc., Germany) for 5 min to remove root tissue debris. The supernatant was centrifuged at 2,500 rpm (Allegra 25R, Beckman Coulter Inc., Germany) for 5 min and the pellet, containing resting spores was washed twice with ddH2O before resuspension in 10 mL ddH2O. Resting spore concentration was determined using a hemocytometer and diluted to 4 × 107 resting spores/mL. For infection studies, 14-day-old A. thaliana seedlings were inoculated at the soil level of the seedling stem with 500 μL of 4 × 107 resting spores/mL. Control seedlings were treated with 500 uL of ddH2O. Each set of plants were grown on separate trays in the same growth chamber. Three independent biological replicates were carried out for both control and treated plants, with each experimental timepoint consisting of 10 or more plants.
Putative Plasmodiophora brassicae Effectors and Their Functional Annotation
Sequences from a cDNA library from 35-day-old canola clubroot galls were screened for P. brassicae putative effectors (PbPEs) using a bioinformatics pipeline (Supplementary Figure 1). Initial trimming of sequences was done using Phred (Ewing and Green, 1998; Ewing et al., 1998) with a quality threshold of 0.05. Vector sequences were identified by multiple sequence alignment using MUSCLE (EMBL-EBI) and removed using crossmatch1. Small (<75 bp) and duplicate sequences were removed using CD-HIT2 with a 97% identity cut-off. Non-redundant cDNA sequences were mapped against the B. napus and P. brassicae genomes using Spliced Transcripts Alignment to a Reference (STAR: Dobin et al., 2013). All sequences that mapped to the P. brassicae genome and those with no hits were translated to putative protein sequences using web server ExPASy tools3 and ORF finder4 and screened against the P. brassicae non-redundant proteome using BlastX (NCBI) with an E-value threshold of 0.001. All P. brassicae positive protein sequences were surveyed with the signal peptide (SP) prediction program SignalP v5.0 (organism group = Eukaryotes)5 with a D-cut-off score above or equal to 0.7 and those sequences with a predicted transmembrane domain (TMD) using TMHMM v2.06 and Phobius7 and/or an ER retention signal motif (ScanProsite web server)8 were excluded from the final list of PbPEs (Supplementary Table 1). Functional annotation of the final PbPEs was carried out using HMMER9 and the rapid functional annotation server PANNZER10 with a Z-score threshold of 0.5. Blast2GO annotations based on functional descriptions with the top 20 hits were also considered and listed. PbPE functional domains were identified using the Conserved Domain Database (CDD)11. The molecular weight, theoretical isoelectric point (pI) and amino acid length of PbPEs were calculated using ProtParam12 and prediction of the subcellular localization of the PbPEs was carried out using LOCALIZER13.
Signal Peptide Validation Using a Yeast Signal Sequence Trap Assay
Plasmodiophora brassicae PE SP functionality was tested in yeast strain YTK12, which is deficient in growth on sucrose or raffinose medium without an active invertase secretory system (Oh et al., 2009). Coding sequences of PbPE SPs were amplified from cDNA with the addition of 5′-EcoR1 and XhoI-3′ restriction sites and cloned, in frame with the SP-deficient invertase gene, into the pSUC2 vector. YTK12 was transformed with the resulting constructs using the Li-Acetate method (Gietz and Woods, 2002) and positive clones, selected on CMD-W media (Yu et al., 2017; Pérez-López et al., 2020), were confirmed by colony PCR. Positive yeast YTK12 transformants were grown on YPRAA selective media to select for invertase secretion (Yu et al., 2017; Pérez-López et al., 2020). For the TTC-(2,3,5-triphenyl tetrazolium chloride)-colorimetric assay, positive yeast YTK12 transformants were grown in YPD media for 36 h and pellets were collected from 1.5 mL cell suspension after centrifugation (Thermo Scientific Sorvall Legend Micro 21R) at 20,000 × g for 2 min. Pellets were washed twice with distilled water before re-suspending in 750 μl sterile distilled water. To this cell suspension 250 μl of 10 mM acetic acid–sodium acetate buffer (pH 4.7) and 500 μl 10% sucrose solution (w/v) was added and incubated at 37°C for 10 min. After centrifugation at 20,000 × g for 1 min, 100 μl of the supernatant was added to 900 μl of 0.1% TTC solution in a glass test tube and incubated at room temperature for 5 min. SP activity was investigated through secreted invertase reduction of the colorless 2,3,5-triphenyl tetrazolium chloride (TTC) to red-colored 1,3,5 triphenyl formazan (TPF) (Pérez-López et al., 2020). The previously identified Arabidopsis secretory protein, low molecular weight cysteine-rich 78 (AtLCR78) (Shahzad et al., 2013; Pérez-López et al., 2020), was used as a positive control in both of these assays.
RNA Extraction, cDNA Synthesis, and Semiquantitative RT-PCR Expression Analysis
Tissue samples from both P. brassicae inoculated and non-inoculated Arabidopsis roots at 0, 2, 5, 7, 14, 21, and 28-DPI, as well as resting spores from dry 35-day old canola galls, were collected in liquid nitrogen. Total RNA was extracted using the phenol-urea-LiCl method as previously described (Missihoun et al., 2011). RNA concentrations were measured using a Thermo NanoDrop 2000C spectrophotometer system (Thermo Fisher Scientific, Waltham, MA, United States). cDNA synthesis, for RT-PCR expression profiling, was carried out using the QuantiTect Reverse Transcription Kit (Qiagen, United States) using 200 ng total RNA. To avoid reaching saturation, semi-quantitative PCR was carried out using a low number of PCR cycles (n = 28). Semiquantitative RT-PCR expression data were generated from unsaturated gel image analysis using VisionWorks LS software14. Relative expression profiles of the PbPEs were measured against the P. brassicae internal control PbRPS17 (AF539801).
Vector Construction and Subcellular Localization of PbPEs
Predicted SP sequences were removed by amplification using appropriate paired AttB1 recombination cloning primers; 3′ to the SP sequence and the 3′ end of the PbPE sequence. After removal of the SP sequence, in planta subcellular localization was determined for each ΔspPbPE. ΔspPbPE cDNA sequences or cellular marker gene sequences were cloned into plant expression binary vectors with (pH7XWG2) or without (pH7WG2) fluorescent tags, using Gateway cloning technology (Thermo Fisher Scientific; Karimi et al., 2002). The cDNA sequence of each ΔspPbPE, sandwiched between attB1 and attB2 recombination sites, was inserted into the entry vector pDONR221/207/Zeo via a BP reaction. From there the ΔspPbPE sequence was added to the C-terminal GFP tagged binary vector pH7FWG2, via an LR reaction, with expression driven by the CaMV 35S promoter.
The mCherry-tagged sub-cellular marker gene constructs, in the pBIN20 binary vector backbone, were purchased from the Arabidopsis Biological Resource Centre15. The GUS expression construct, pH7WG2-GUS, was created from pENTR-GUS provided in the gateway cloning kit, with the GUS sequence inserted into pH7WG2 via an LR reaction. The A. thaliana REMORIN 1.3 (AT2G45820.1) sequence was cloned into pH7RWG2, with a C-terminal mRFP fluorescence tag, for co-localization studies. A GFP construct, pH7WG2-GFP, was also generated for use as a negative control for the cell death assay and transient localization studies. All constructs were used to transform Agrobacterium tumefaciens, with positive transformants selected on LB medium supplemented with spectinomycin (100 mg/L), kanamycin (50 mg/L), or rifampicin (50 mg/L) and subsequently used to transform N. benthamiana for transient expression studies. All the constructs used and generated in this study are provided in Supplementary Table 2.
Subcellular localization of PbPEs was determined by transiently expressing the ΔspPbPE-GFP gene fusion-constructs, in A. tumefaciens at a final OD600 of 0.3, together with organelle-specific markers, in N. benthamiana leaves. Subcellular localization of the PEs was recorded 2-3 days after agroinfiltration. The localization of each ΔspPbPE-GFP was visualized with a LSM880 inverted confocal laser scanning microscope (Zeiss, MN, United States) using a 40X water objective at GFP-required wavelengths. GFP and chloroplast autofluorescence was monitored using an Argon laser at 488/500–530 and 488/580–620 nm excitation/emission wavelengths, respectively. The mRFP and mCherry fluorescence tags were monitored using a Helium-Neon laser at 561/600 and 561/630 nm, excitation/emission wavelengths, respectively.
To classify the localization of ΔspPbPE-GFPs at the cell periphery, N. benthamiana leaf segments (leaves) were plasmolyzed in 0.85 M KCl for 15 min before observation under the Zeiss LSM880 microscope using a 40X water objective as outlined above. Flg22 treatment was performed on leaves 2 days post infiltration and confocal images were taken 1 h after flg22 treatment.
Z-stack and time-lapse images were captured to provide further insight into the fluorescence distribution, association and dynamics of ΔspPbPE localization in N. benthamiana leaf epidermal cells. To verify the localization profile for each ΔspPbPE, multiple images were captured from different fluorescence-expressing cells. To avoid overexpression artifacts, transiently expressing cells, with comparatively low fluorescent signals, were imaged for analysis using FIJI ImageJ16. Fluorescence intensity plots were graphed based on the quantitative data measured in arbitrary units (a.u.), obtained from the region of interest of a confocal colocalized image represented by a blue line, using ImageJ. Each fluorescence channel in a colocalized confocal image represents the individual line graph in a fluorescence intensity plot.
Agrobacterium Co-infiltration and Cell Death Assay
Screening of PbPEs for cell death regulation was carried out, with a PCD assay using the inducers PiINF1 elicitin and PiNPP1 from Phytophthora infestans, as previously described (Kelley et al., 2010). ΔspPbPEs were cloned under the control of the CaMV 35S promoter, using gateway cloning, as described above. Transient expression of ΔspPbPEs in N. benthamiana leaves was carried out as described in Sparkes et al. (2006). The third and fourth healthy leaves of 5-week-old N. benthamiana plants were infiltrated on the abaxial side with A. tumefaciens GV3101 (pMP90) strain carrying an inducer of PCD, pGR106-PiNPP1 or pGR106-INF1 plus a ΔspPbPE construct. pH7WG2-GFP was used as the negative control and pART-PiSNE1 (suppressor of necrosis 1) as the positive control for the PCD regulation assays (Kelley et al., 2010).
The PCD assays were carried out using two different methods: (i) a single infiltration of an equal concentration (OD600 of 0.3 for each construct) mixed solution of inducer and ΔspPbPE and (ii) overlapping additions of inducer and ΔspPbPE, where leaves were first infiltrated with the ΔspPbPE 1 day before infiltration with the PCD inducer at a separate, but partially overlapping location, on the same leaf. The overlapping zone of infiltration was the area of co-expression and possible PCD suppression. Suppression or induction of PCD was monitored 5 days post infiltration with PiINF1 and 7 days post infiltration with PiNPP1. The HR index was calculated from the mean percentage necrotic area per total infiltrated area on the leaves using the color threshold for FIJI ImageJ (see text footnote 16). Student’s t-test was conducted to identify statistically significant differences in co-infiltration treatment between GFP and PbPEs with PCD inducers at p = 0.01 and 0.05.
Results
Selection of Plasmodiophora brassicae Candidate Effectors
cDNAs from a full-length cDNA library of total RNAs extracted from P. brassicae-infected canola galls at 35 DPI with P. brassicae resting spores were sequenced and screened for PbPEs. A total of 117 putative secretory protein (effectors)-coding cDNAs (PEs), comprising proteins with an N-terminal SP for secretion out of the pathogen into the plant cell (Supplementary Table 1), a transmembrane domain (TMD) and in some PEs an ER retention signal (HDEL, KDEL) at the C-terminus, were identified (Supplementary Figure 1). Removal of putative membrane located (TMD) proteins and proteins with ER retention signals, resulted in a final list of 52 PbPEs (Supplementary Table 1), representing 44% of the total P. brassicae secretome identified from in silico study at the clubroot gall stage of infection in canola. Based on previously published RNA-seq data (Irani et al., 2018; Pérez-López et al., 2020), functional annotation and preliminary expression data, suggesting likely importance in pathogenesis, 15 PbPE sequences were selected for further study.
Each of the 15 PbPEs contained a predicted N-terminal SP (SignalP v5.0). Using a yeast secretion system (Gietz and Woods, 2002; Oh et al., 2009), SP functionality was established for 14 of the 15 PbPE SPs, with the predicted PbPE16 SP lacking function in both the growth and secretion (red) aspects of the assay (Figure 1). The subcellular membrane localization, in plants, of the 14 PbPEs with functional SPs, was determined.
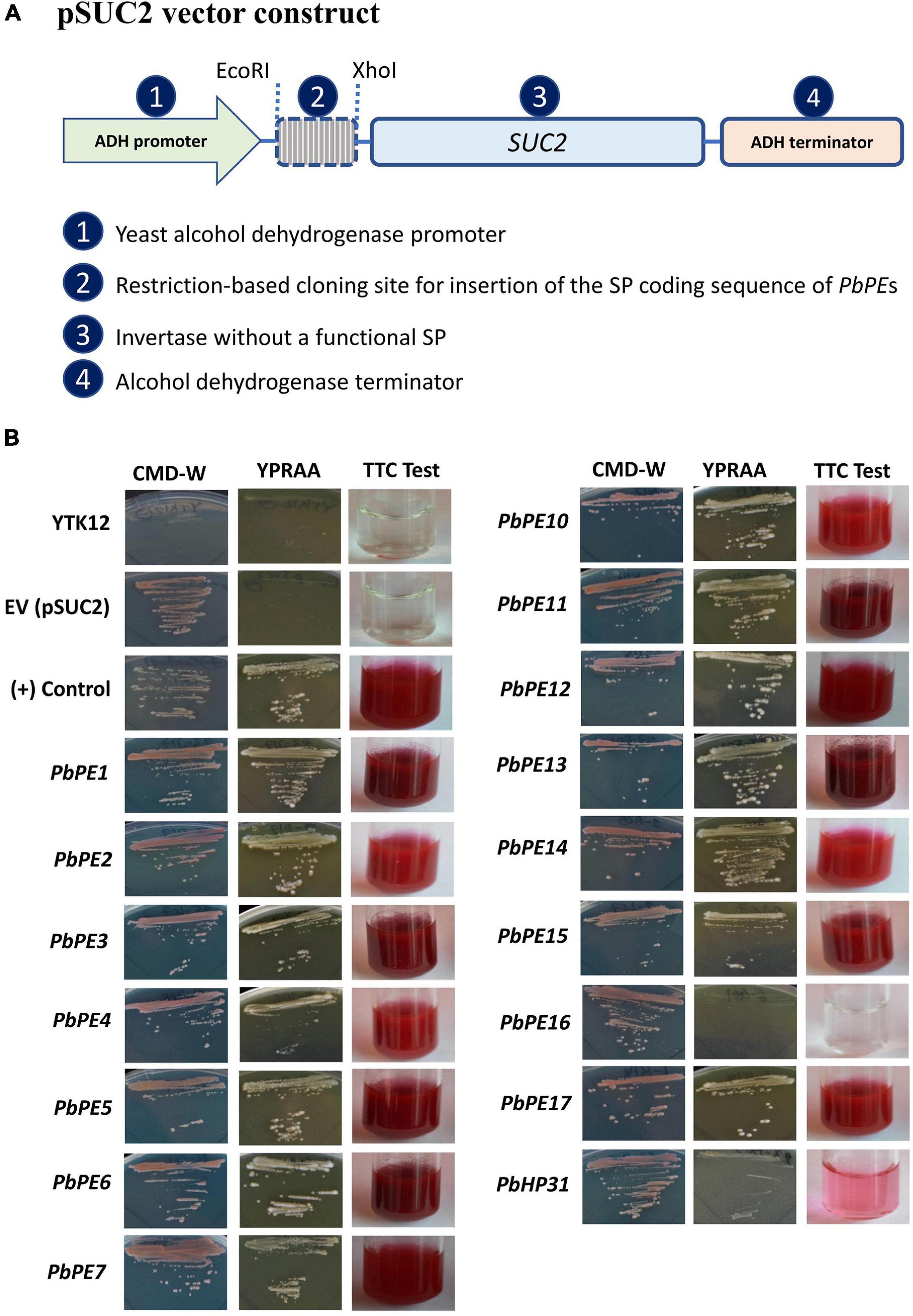
Figure 1. Functional validation of the Plasmodiophora brassicae PE signal peptides with a yeast secretion system. (A) pSUC2 vector construct containing the signal peptide sequences of the P. brassicae PEs cloned in frame with the invertase gene. (B) The invertase negative yeast strain (YTK12) transformed with pSUC2 constructs with a functional signal peptide grew on YPRAA selective media and invertase activity was visualized through reduction of TTC to the red–colored formazan. Intermediate color (pink) was also observed in the TTC test and considered negative in the validation of functional SP. Untransformed YTK12 and YTK12 transformed with empty vector (pSUC2) were negative controls and Arabidopsis secretory protein low molecular weight cysteine-rich 78 (AtLCR78) was the positive control. These images are representative of three independent biological replicates.
PbPEs Targeting to the Endomembrane System of the Plant Cell
To mimic secretion (i.e., processing and cleavage of the PbPE SP during secretion) from the pathogen into the plant cell, each of the 14 PbPE genes was cloned, minus the SP (Δsp), in frame with a green fluorescent protein (GFP) sequence, under the control of a single CaMV 35S promoter. Each of the resulting 14 ΔspPbPE-GFPs was transiently expressed in N. benthamiana leaf epidermal cells.
Of the 14 ΔspPbPE-GFPs, 12 localized to the endomembrane system, with localization to the ER being most prominent. ΔspPbPE-GFPs; ΔspPbPE2GFP, ΔspPbPE10GFP and ΔspPbPE14GFP all localized to the ER and the nucleus in N. benthamiana (Figure 2), whereas ΔspPbPE17GFP, containing three ANK repeats and a predicted BTB domain, localized to both ER and Golgi bodies (Figure 3 and Supplementary Files 1, 2). The ER-mCherry (CD3-959) contains the ER retention signal (HDEL) at the C-terminus was used as a marker for co-localization studies. ΔspPbPE1GFP, also containing three ANK repeats, localized to the ER, Golgi bodies and the nucleus (Figure 4A). ΔspPbPE3GFP localized only to small, mobile, punctate structures, in the plant cytoplasm, that were identified as Golgi bodies after colocalization with the cis-faced Golgi stack marker, GmMan11–49aa-mCherry (CD3-968) (Figure 4B), while ΔspPbPE5GFP, ΔspPbPE6GFP, ΔspPbPE11GFP, and ΔspPbPE12GFP all showed nucleo-cytoplasmic localization with ER fractions in the cell (Supplementary Figure 2). While the possibility cannot be ignored that nuclear localization was the result of diffusion, as seen for GFP alone (Figure 2B), if this was the case then with all selected PbPEs being small, secreted protein-GFPs, one would expect that all PbPE-GFPs would be found in the nucleus.
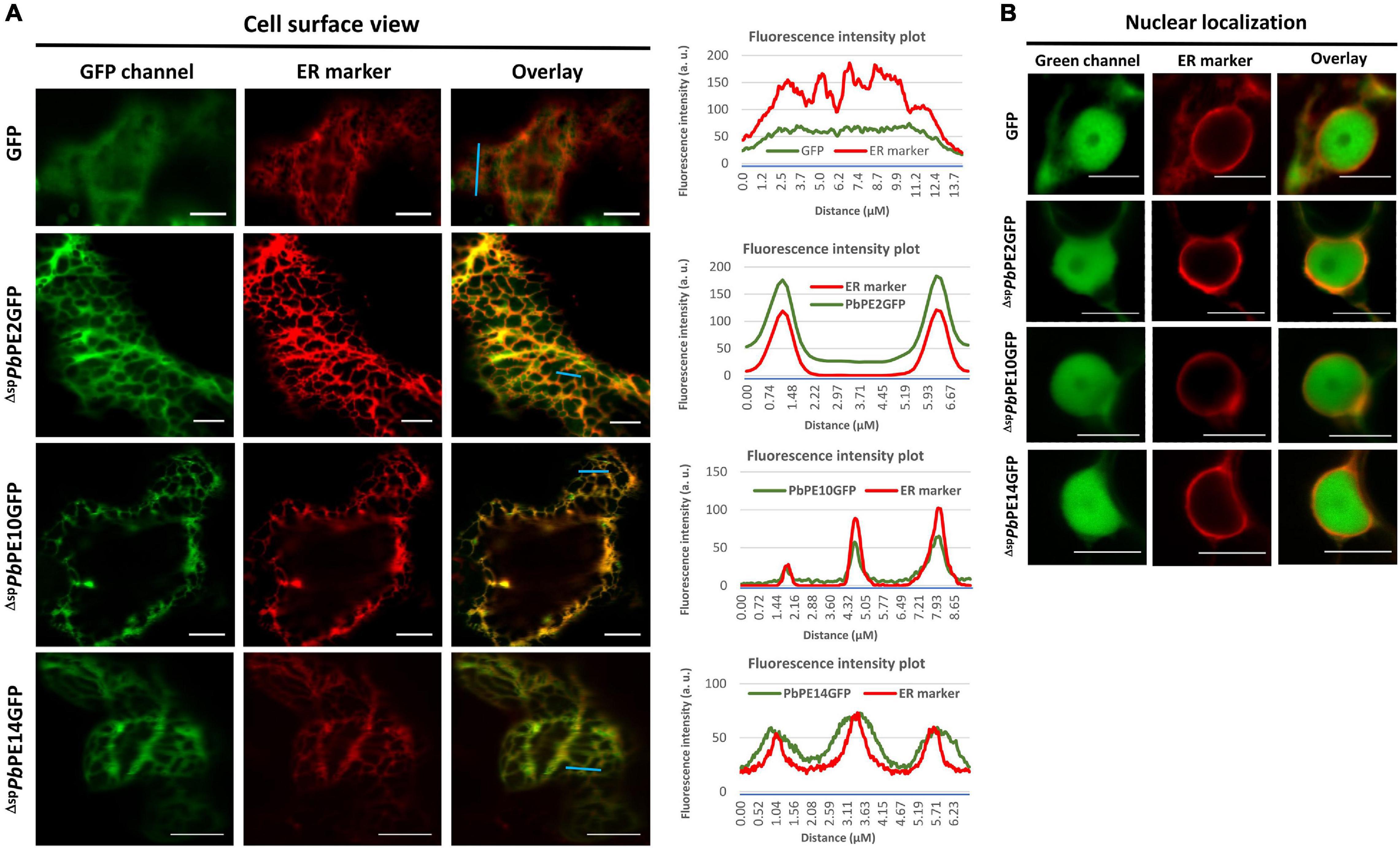
Figure 2. Endomembrane localization of PbPEGFPs upon transient expression in leaf epidermal cells of N. benthamiana. Representative confocal micrographs showing free GFP and nuclear-ER localization of ΔspPbPE2GFP, ΔspPbPE10GFP and ΔspPbPE14GFP in N. benthamiana leaves. (A) Red fluorescence corresponding to the ER marker, mCherry-HDEL (CD3-959) shows the cortical ER pattern and the right panels indicate the merge of green and red fluorescence channels. (B) Confocal images show nuclear and perinuclear localizations of the free GFP and GFP-tagged ΔspPbPEs and mCherry-tagged ER marker, respectively. Fluorescence intensity plots show the representative localization patterns of the FP-tagged proteins along the light blue lines on image overlays. Scale bars = 10 μm.
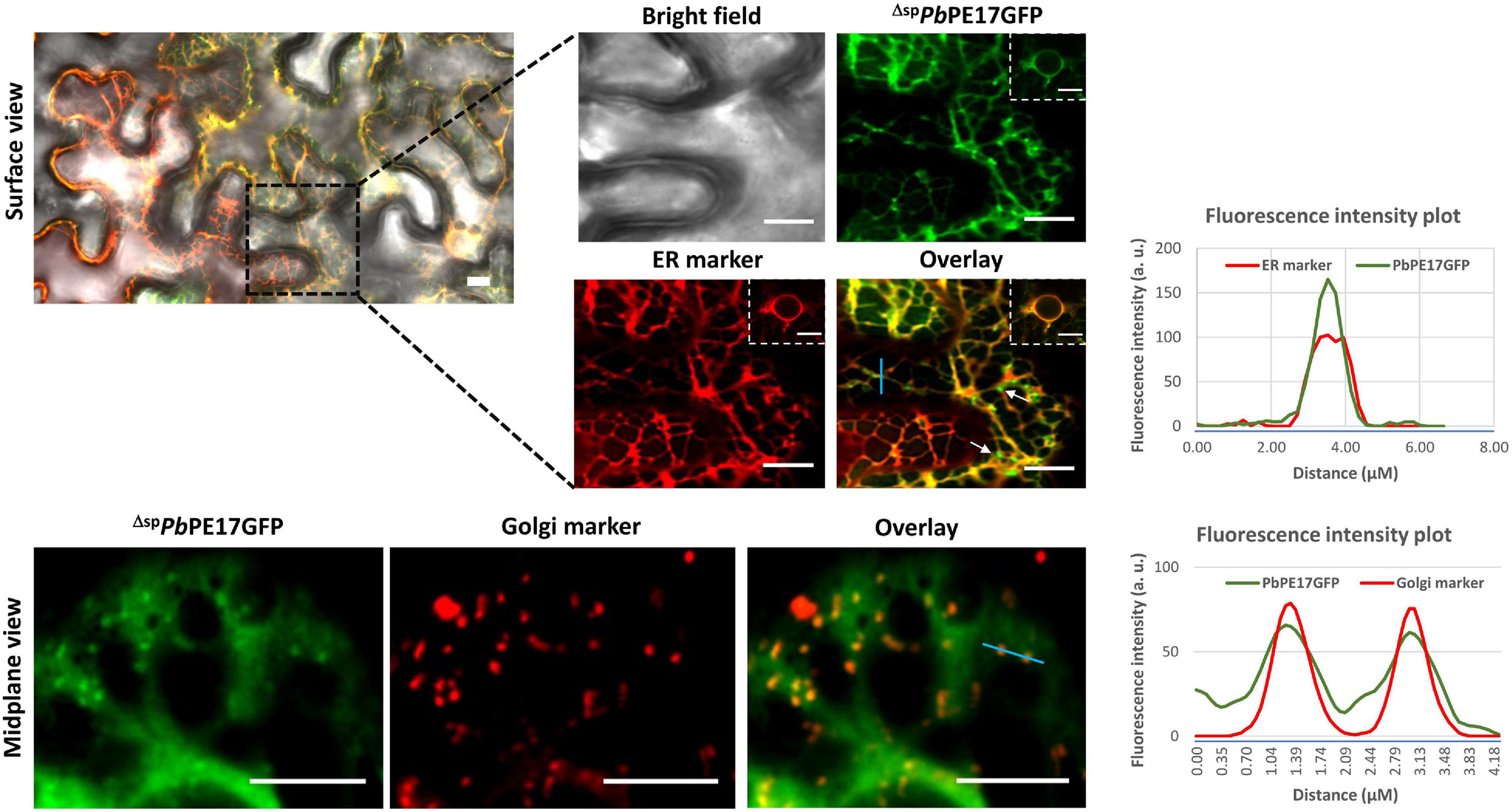
Figure 3. Endomembrane localization of PbPE17GFP upon transient expression in leaf epidermal cells of N. benthamiana. ΔspPbPE17GFP localizes in both ER and Golgi stacks (white arrows) but is excluded from nuclei upon transient expression in N. benthamiana. The concentrated green fluorescence on the face of cortical ER (white arrows) was identified as Golgi stacks by co-localization with the Golgi marker. Surface and mid-plane views of the cell show cortical ER and Golgi localization of the effector, respectively. Black dotted line highlights the zoom in version of the surface images. Inset images showing perinuclear ER localization of ΔspPbPE17GFP in transiently expressed cells. Representative fluorescence intensity plots of co-localization of ΔspPbPE17GFP with ER or Golgi markers are shown along the light blue line on image overlays. Scale bars = 10 μm.
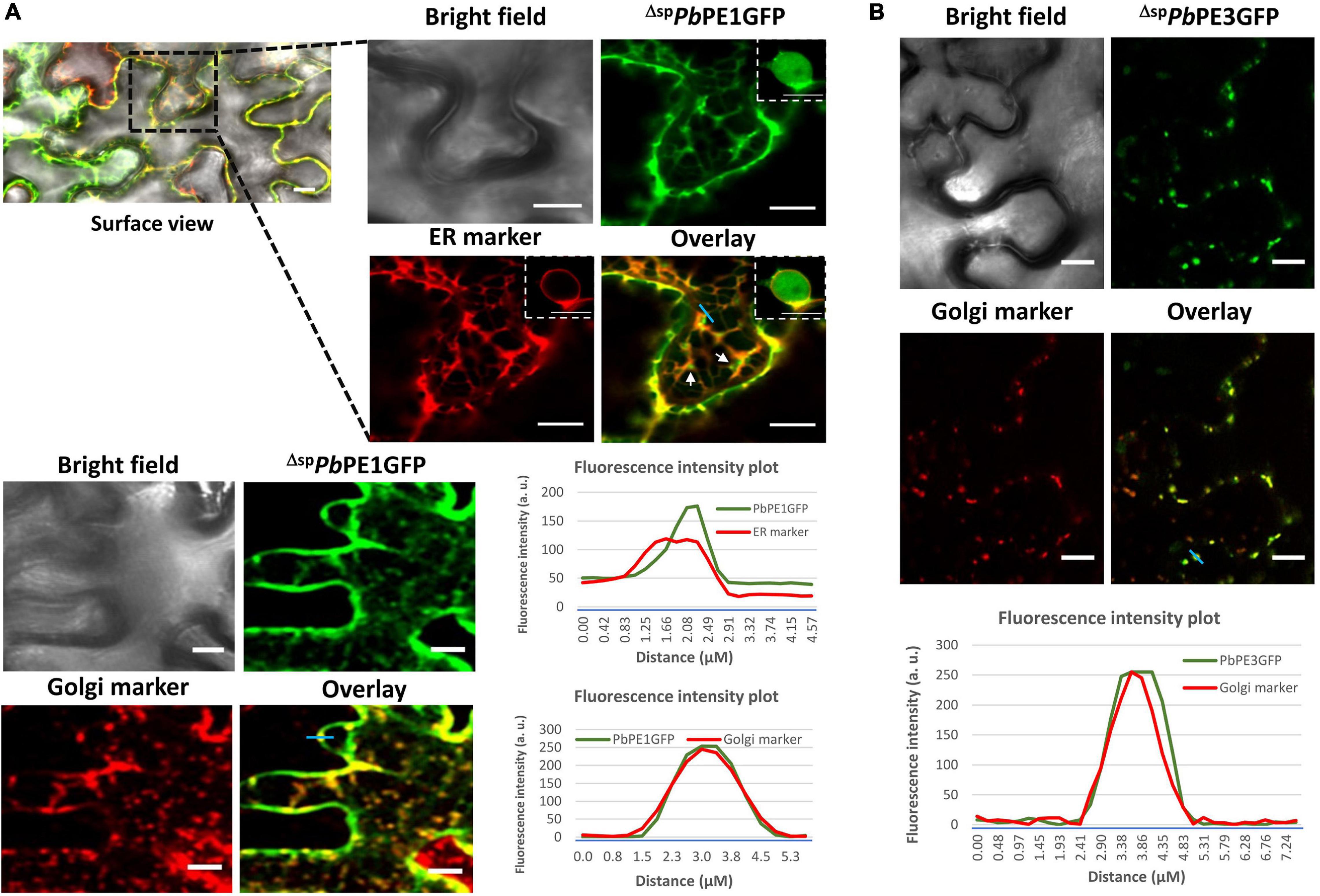
Figure 4. Endomembrane localization of PbPE1GFP and PbPE3GFP upon transient expression in leaf epidermal cells of N. benthamiana. (A) ΔspPbPE1GFP co-localizes with ER and Golgi markers in N. benthamiana leaves. Nuclear localization of ΔspPbPE1GFP was also shown in the inset picture. The concentrated green fluorescence on the face of cortical ER (white arrows) was identified as Golgi stacks by co-localization with the Golgi marker. (B) ΔspPbPE3GFP co-localizes with the cis-faced Golgi stack marker, GmMan11– 49aa-mCherry (CD3-968). Representative fluorescence intensity plots of co-localization of ΔspPbPE1GFP with ER or Golgi markers and ΔspPbPE3GFP with Golgi markers are shown along the light blue line on image overlays. Black dotted line highlights the zoom in version of the surface images. Scale bars = 10 μm.
ΔspPbPE13GFP Localizes to PM Lipid Rafts
PbPE13 is a small hypothetical protein (143 amino acids) of unknown function that is not annotated as a P. brassicae protein in the NCBI database. ΔspPbPE13GFP localized to punctate structures at the cell periphery that co-localized with PM intrinsic protein 2A (AtPIP2A-mCherry CD3-1007) (Figure 5). Co-localization of ΔspPbPE13GFP with the ER marker showed limited ER or perinuclear ER association (Figure 5), however, the punctate arrangements of ΔspPbPE13GFP at the cell periphery co-localized perfectly with a PM lipid raft marker AtREMORIN 1.3 (AtREM1.3) tagged with C-terminal mRFP (Figures 5, 6A). To evaluate the robustness of the PM localization, ΔspPbPE13GFP and AtREM1.3mRFP co-expressing cells were plasmolyzed, resulting in a large fraction of the co-localized signal remaining in the retracting PM, with a small amount retained at the cell wall (Figure 6A). Co-expression of ΔspPbPE13GFP with the known PD marker, plasmodesmata localized callose binding protein 1 (PDCB1-DsRed2) showed co-localization of some of the ΔspPbPE13GFP punctate structures with PDCB1-DsRed2 signals (Figure 6A), suggesting that ΔspPbPE13GFP associates with plasmodesmata.
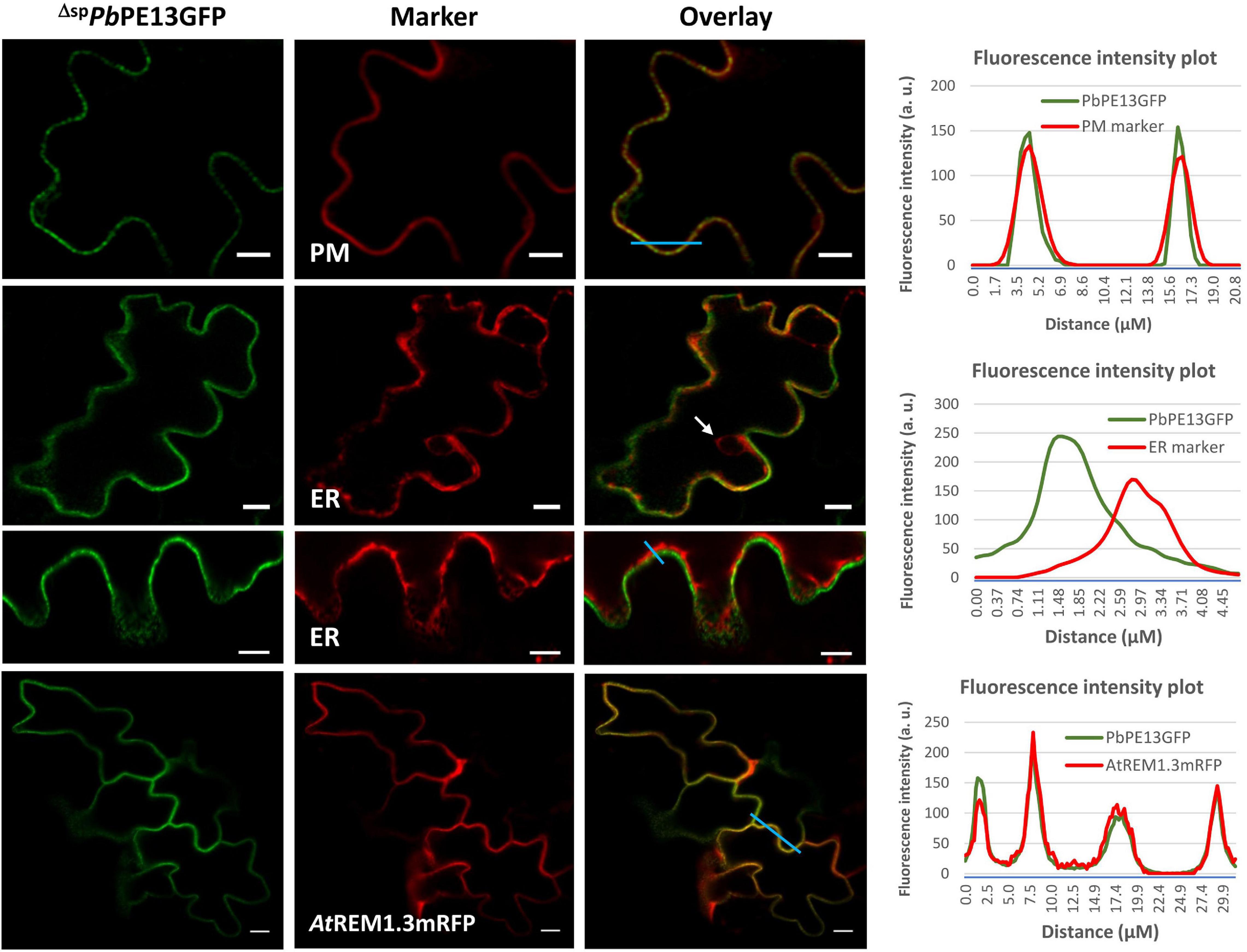
Figure 5. Localization of ΔspPbPE13GFP to plasma membrane (PM) lipid rafts in N. benthamiana leaf cells. PM localization of ΔspPbPE13GFP, together with punctate structures at the cell peri2phery. Co-localization images of ΔspPbPE13GFP and a known PM-marker, mCherry-tagged Arabidopsis aquaporin AtPIP2A (CD3-1007). ΔspPbPE13GFP did not colocalize with perinuclear ER (white arrow). Co-localization of ΔspPbPE13GFP with Arabidopsis REMORIN1.3mRFP in PM lipid rafts. Representative fluorescence intensity plots of co-localization of ΔspPbPE13GFP with PM or ER or Remorin markers are shown along the light blue line on image overlays. Scale bars = 10 μm.
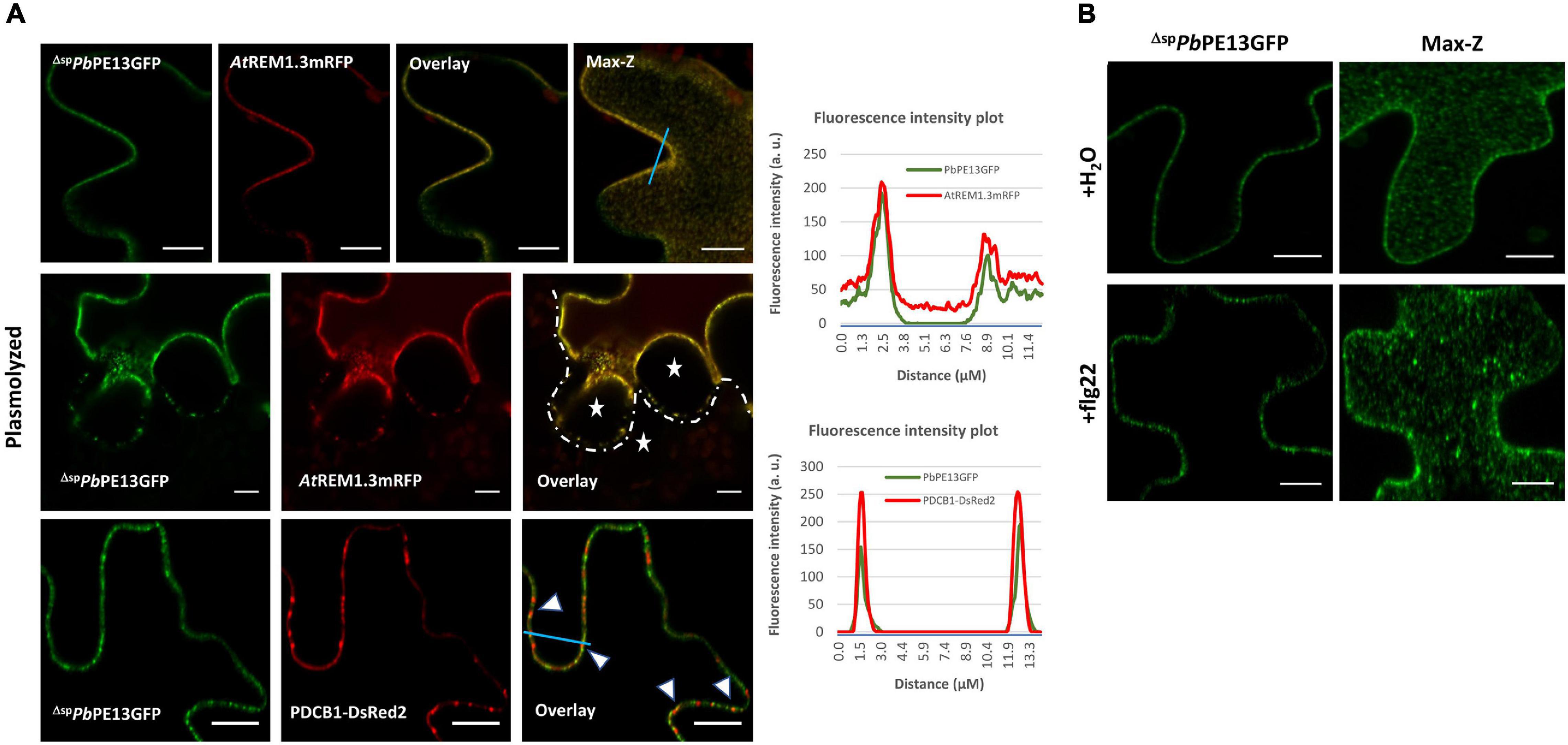
Figure 6. Association of ΔspPbPE13GFP with plasmodesmata (PD) after transient expression in N. benthamiana leaf cells. (A) Close up images and maximum Z-projection of the co-localization of ΔspPbPE13GFP and AtREM1.3mRFP in punctate plasma membrane (PM) structures. Plasmolysis of the co-localized cells shows some retention of fluorescence signal at the cell wall; white stars indicate apoplastic spaces between adjoining cells. Co-localization of ΔspPbPE13GFP with plasmodesmata associated protein PDCB1-DsRed2 at punctate PM structures (white arrowheads). (B) Re-organization of membrane localization of ΔspPbPE13GFP upon flg22 treatment in N. benthamiana leaf cells. Confocal images of surface localization patterns of the effector were monitored upon flg22 treatment and compared with a H2O control. PM localization of ΔspPbPE13GFP, together with punctate structures at the cell periphery using H2O control. flg22 treatment of the ΔspPbPE13GFP transiently expressed leaves shows relocation of fluorescence signal into concentrated and mobile punctate structures as observed in the maximum-Z projection. Representative fluorescence intensity plots of co-localization of ΔspPbPE13GFP with Remorin or PDCB1-DsRed2 markers are shown along the light blue line on image overlays. Scale bars = 10 μm.
Plasmodiophora brassicae primary and secondary zoospores are bi-flagellated, providing motility and facilitating attachment and infection of host cells. To avoid PTI and establish colonization in host plants, P. brassicae must regulate the flg22-FLS2 triggered innate immune responses during infection. Therefore, we investigated the dynamics of ΔspPbPE13GFP localization upon flg22 perception in N. benthamiana. Treatment with flg22, a peptide derived from the flagellin N-terminus of Pseudomonas aeruginosa, triggered the association of NbREM4 and fluorescence distribution due to membrane raft re-organization and compartmentalization within PM lipid rafts (Keinath et al., 2010; Albers et al., 2019). With a concentrated localization of ΔspPbPE13GFP to PM lipid rafts, we wanted to determine if ΔspPbPE13GFP showed a similar localization pattern to NbREM4 upon biotic stress. To do so, we investigated the dynamics of ΔspPbPE13GFP localization upon flg22 perception in N. benthamiana leaf epidermal cells. Maximum-Z projections show concentrated punctate structures due to the compartmentalization of ΔspPbPE13GFP fluorescence in nanodomains of the PM after flg22 treatment (Figure 6B), suggesting that ΔspPbPE13GFP can re-organize PM lipid rafts upon flg22 perception at the PM and may have a regulatory role in flg22/FLS2 triggered endocytosis and PTI response in plants.
PbPEs Targeting the Plant Cell Endomembrane System Are Differentially Expressed During Primary and Secondary Infection of Arabidopsis
Transcript levels for the 12 endomembrane-localizing PbPE genes were determined at 0, 2, 5, 7, 14, 21, and 28 DPI of Arabidopsis with P. brassicae pathotype-3 (Figure 7). Of the 12 PbPE genes, transcripts for seven (PbPE1 to PbPE11) were not found in resting spores (Figure 7A) but were found at various stages of infection, during primary infection, after 2 days – PbPE10, PbPE11 or after 5 days – PbPE5, PbPE6; or during secondary infection, after 7 days – PbPE1, PbPE2, PbPE3. Once initiated, all 12 PbPE genes showed continuous and increasing transcript levels up to 21 DPI, after which most showed decreased levels. Transcript for remorin-associated PbPE13 was initially identified during primary infection, at 5 DPI, with no increase between 5 and 7 DPI, before peaking at 21 DPI (Figure 7). PbPE10 was the only PbPE to show a bimodal transcript level; increased transcript at 5 DPI, during primary infection and again at 21 DPI, during late secondary infection (Figure 7).
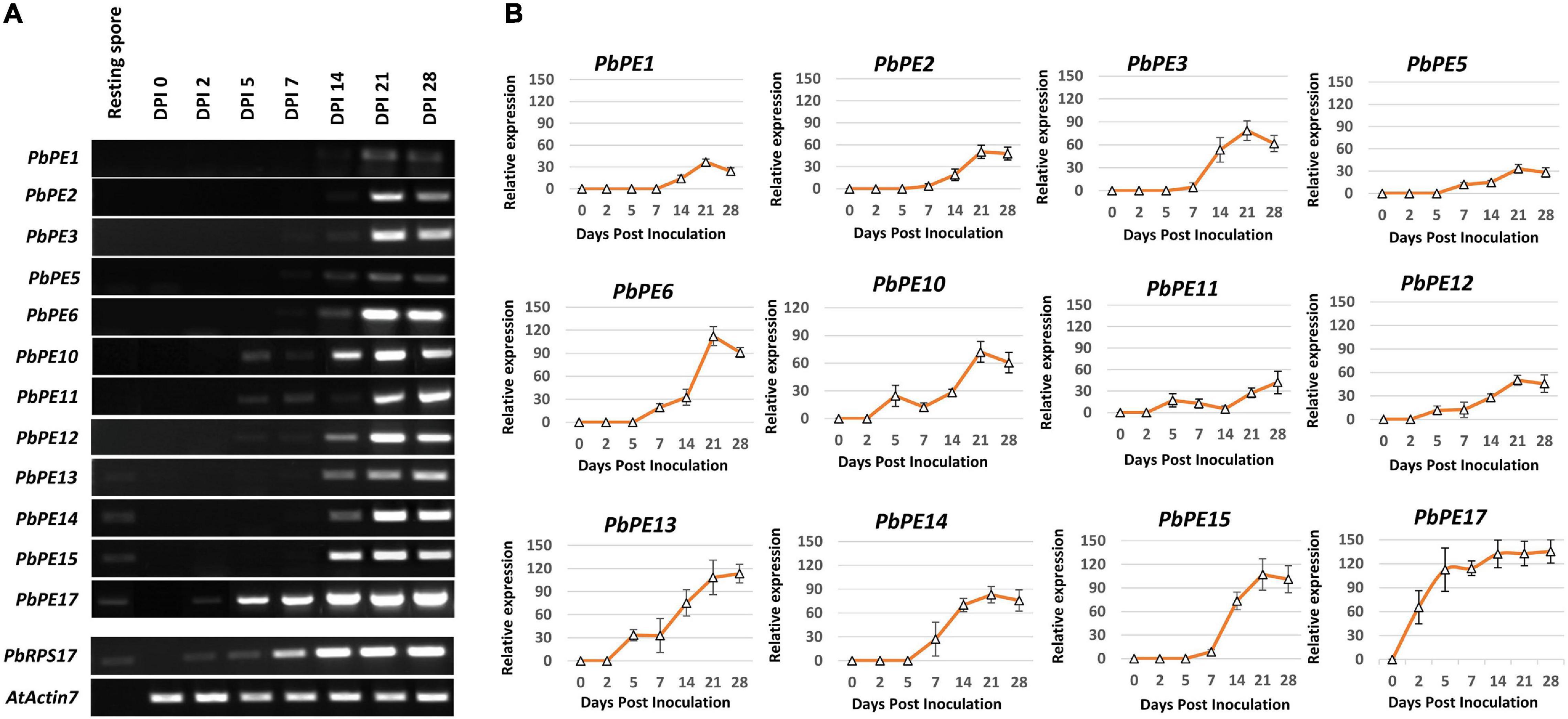
Figure 7. Gene expression profiles of plant endomembrane-localized P. brassicae PEs in the root sample of Arabidopsis plants infected with P. brassicae pathotype-3. (A) Semi-quantitative RT-PCR gene expression patterns of P. brassicae PE candidates at different time points; resting spore, 0, 2, 5, 7, 14, 21, and 28-days post inoculation (DPI). P. brassicae internal control, PbRPS17 (AF539801) and Arabidopsis positive control, AtACTIN7 (At5g09810). (B) Mean (±SD) relative expression profiles of candidate P. brassicae PEs. The relative expression of all P. brassicae genes was determined with respect to that of PbRPS17 set at 100. n = 3 independent biological replicates.
PbPEs Regulate the PTI Response in Plants
The effect of the 12 secreted endomembrane targeting P. brassicae PEs on the plant PTI response was assessed using a cell death assay with PiINF1 (P. infestans Infestin 1) and PiNPP1 (P. infestans necrosis causing protein 1) as inducers of PCD (Figure 8). Agroinfiltration of PiNPP1 or PiINF1 with a GFP control construct induced PCD of N. benthamiana leaf cells (Figure 8A). PiINF1 is a more potent inducer of PCD, with necrotic lesions prominent 5 days post infiltration with PiINF1 + GFP, compared to PiNPP1, with necrotic lesions visible 7 days post infiltration with PiNPP1 + GFP. The induction or suppression of PCD by each PbPE was measured by the mean percentage of necrotic area within the infiltrated zone (Figure 8B).
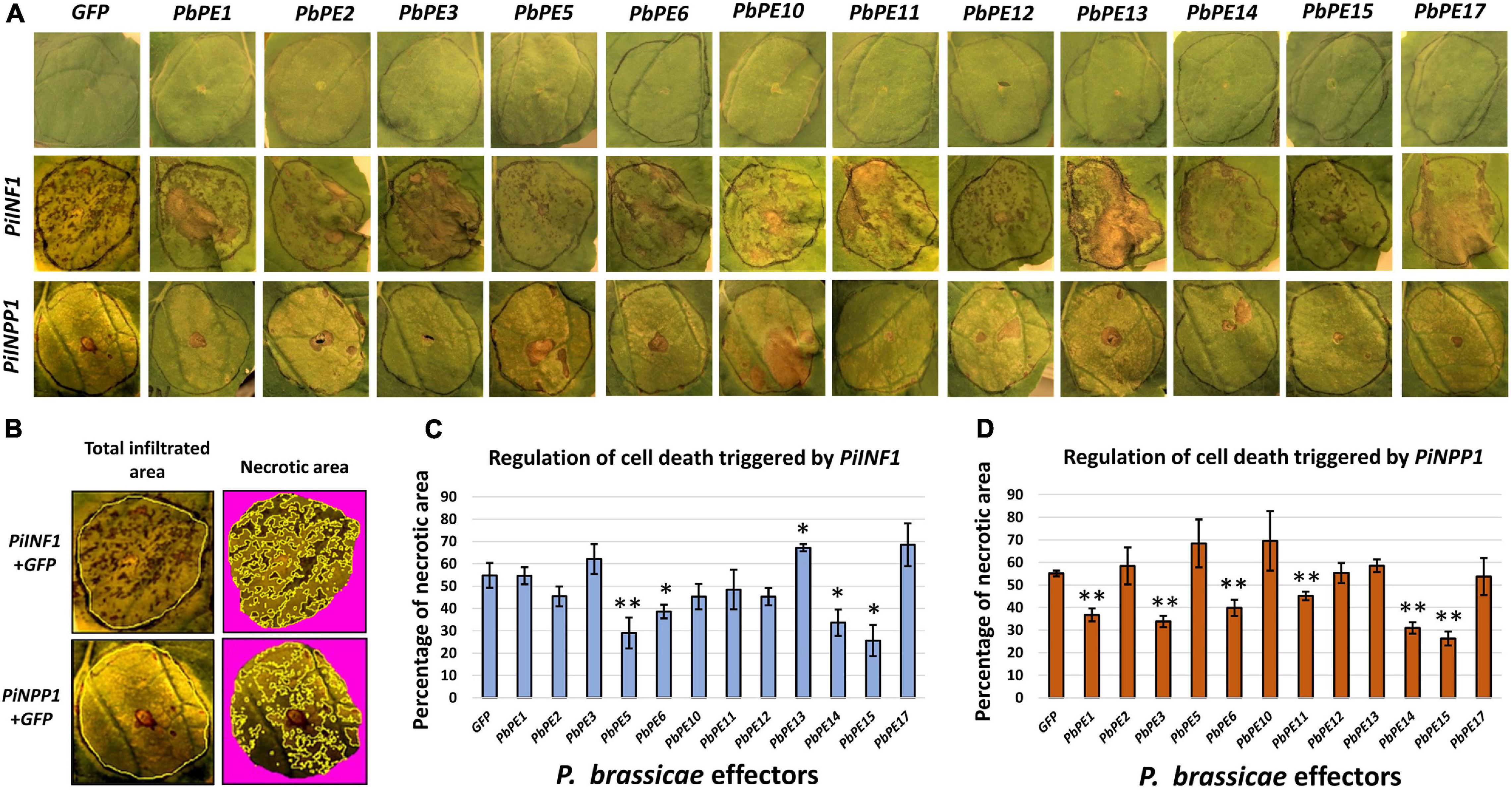
Figure 8. Endomembrane-localized P. brassicae PEs regulate cell death triggered by cell death inducers after transient expression in N. benthamiana. Agrobacterium strains carrying P. brassicae PE gene-vector constructs were co-infiltrated with or without cell death inducers, into leaves of N. benthamiana, to determine their effect on the regulation of cell death. GFP and cell death inducers (PiINF1 and PiNPP1) were used as negative and positive controls, respectively. (A) Visual observation of N. benthamiana leaves infiltrated with P. brassicae effectors and cell death inducers. Infiltration with GFP or P. brassicae PEs alone did not result in cell death (1st row). Cell death phenotypes were scored 5 (PiINF1) and 7 (PiNPP1) days post infiltration after co-infiltration with P. brassicae PEs. (B) Quantitative measurement of cell death in necrotic areas, within the total infiltrated areas on the leaves, was calculated with ImageJ utilizing color threshold measures. Yellow lines indicate the selected regions for calculating total infiltrated areas and necrotic areas on the leaves. Mean percentage of necrotic areas of leaves co-infiltrated with (C) PiINF1 or (D) PiNPP1 and P. brassicae PEs. Each column represents a quantitative measure of the HR index in relation to the mean percentage of the necrotic areas of leaves and standard deviation for an effector of P. brassicae. *P-value < 0.05 and **P-value < 0.01. PiINF1 = infestin 1 elicitin of P. infestens, PiNPP1 = necrosis causing protein of P. infestens. N = three independent biological replicates.
PiINF1-induced PCD was significantly suppressed by PbPE5, PbPE6, PbPE14, and PbPE15, whereas co-expression of PbPE13 and PiINF1 resulted in an enhanced PCD response in N. benthamiana leaves (Figure 8C). PbPE3, PbPE6, PbPE11, PbPE14, and PbPE15 significantly suppressed PiNPP1-triggered PCD (Figure 8D), with PbPE15, a putative member of the 2-oxoglutarate (2OG) and Fe (II)-dependent oxygenase superfamily, showing the strongest level of suppression of both inducers (Figures 8C,D). PbPE15GFP shows nucleo-cytoplasmic localization with ER fractions after transient expression in N. benthamiana cells (Figure 9A). To validate PCD inhibition by PbPE15, an overlapping method of PCD inhibition, using the PCD inducer PiNPP1 with PbPE15, was carried out (Figures 9B,D). The P. infestans suppressor of necrosis 1 (PiSNE1), a secreted effector of the hemibiotrophic oomycete P. infestans, suppresses necrosis mediated by Nep-like proteins (NLPs) during the biotrophic infection phase and was used as a positive suppressor of cell death (Kelley et al., 2010).
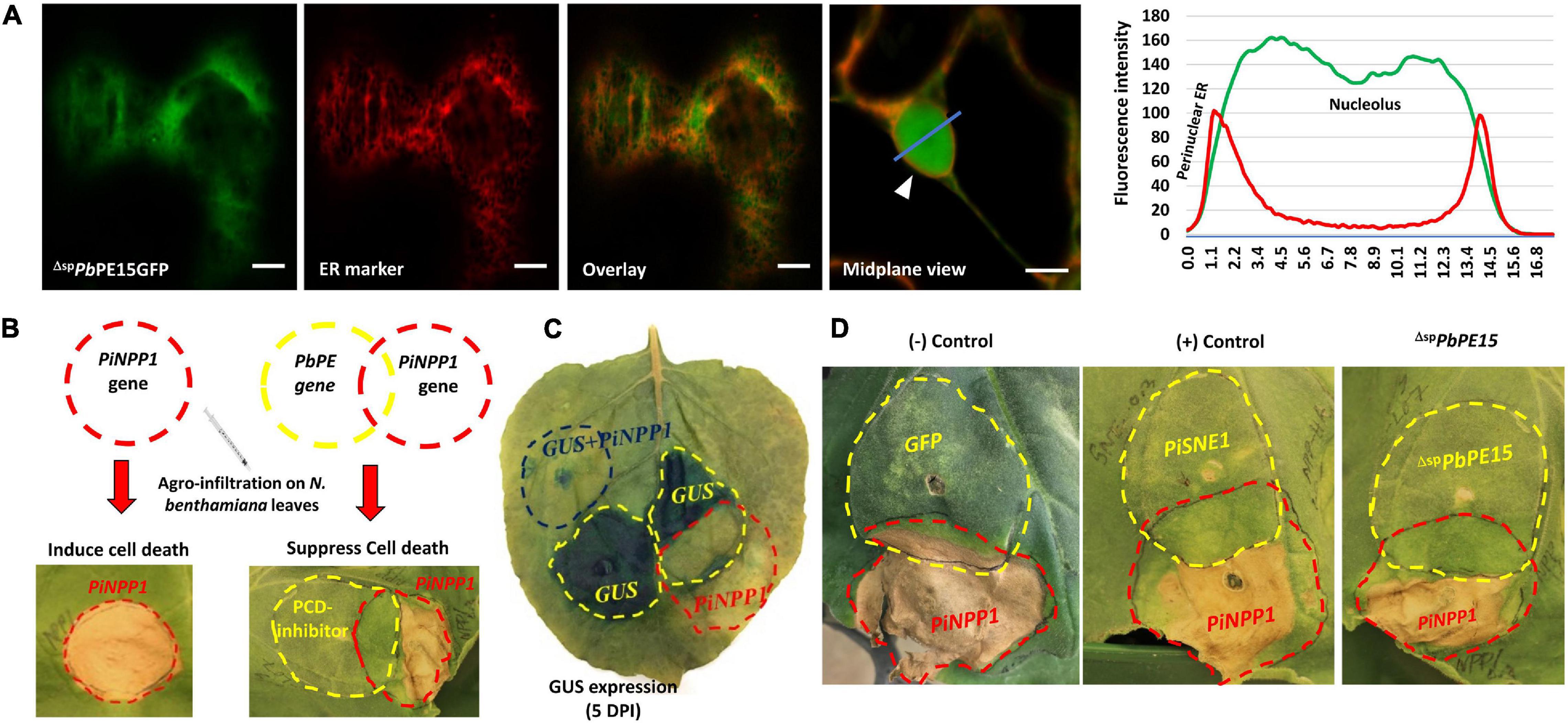
Figure 9. ΔspPbPE15 inhibits PCD induced by PiNPP1. (A) ΔspPbPE15GFP co-localize with an ER marker (CD3-959) in N. benthamiana leaf epidermal cells. ΔspPbPE15GFP shows nucleo-cytoplasmic localization with some ER fraction patterning after transient expression in N. benthamiana leaves. The surface view of the cell indicates cortical ER localization of ΔspPbPE15GFP whereas, the mid-plane view of the merged images shows nuclear localization of the PE. White arrowheads indicate perinuclear ER. Fluorescence intensity plots on the right show the nuclear and perinuclear localization patterns of the FP-tagged proteins along the blue line on the image overlays. Scale bars = 10 μm. (B) Schematic of programmed cell death (PCD) suppression by Agrobacterium co-infiltration of PCD inducer (PiNPP1) and P. brassicae PE gene/cell death regulator. (C) Validation of the overlapping co-infiltration method for agro-infiltration with PCD inducers and P. brassicae PE gene/cell death regulators. (D) P. brassicae putative cell death inhibitor ΔspPbPE15 results in inhibition of PiNPP1-induced PCD. An OD600 of 0.3 was used for all co-infiltration studies. The overlapping regions of the PCD test were identified as positive for the presence of necrosis or negative for the absence of necrosis. The GFP gene was used as a negative control whereas PiSNE1 was used as a positive control. GUS = β-glucuronidase, PiNPP1 = necrosis causing protein of P. infestens, PiSNE1 = suppressor of necrosis 1 protein of P. infestens. The images are representative of three independent biological replicates.
PbPE6 and PbPE14 also showed significant suppression of both PiINF1- and PiNPP1-triggered PCD (Figures 8C,D). PbPE2, PbPE10, PbPE12 and PbPE17 had limited or no suppression effect on either PiINF1- or PiNPP1-triggered PCD (Figures 8C,D). PbPEs localizing to the different compartments of the plant endomembrane system along with their significant regulatory roles in modulating PTI response indicate the functional importance of these effectors in plant pathogenesis during infection.
Discussion
A successful plant immune response against biotic stress requires a well-organized array of intracellular processes by the host, including signal transduction, endomembrane trafficking of cellular cargo to pathogen invasion sites in the process of the execution of PTI and ETI responses, resulting in some degree of resistance to the stress (Gu et al., 2017). As an intracellular biotroph, P. brassicae manipulates the host metabolism to its benefit while also avoiding recognition by host cells. In this study, we initially identified 52 PbPEs from root galls of P. brassicae infected canola (B. napus), a subset of which were investigated further based on their localizations to the plant endomembrane system. Here, we report the impact of twelve endomembrane-targeting PbPEs on cell death regulation in N. benthamiana. An overall schematic summarizing possible functions of the endomembrane-targeting PbPEs during P. brassicae pathogenesis is presented (Figure 10).
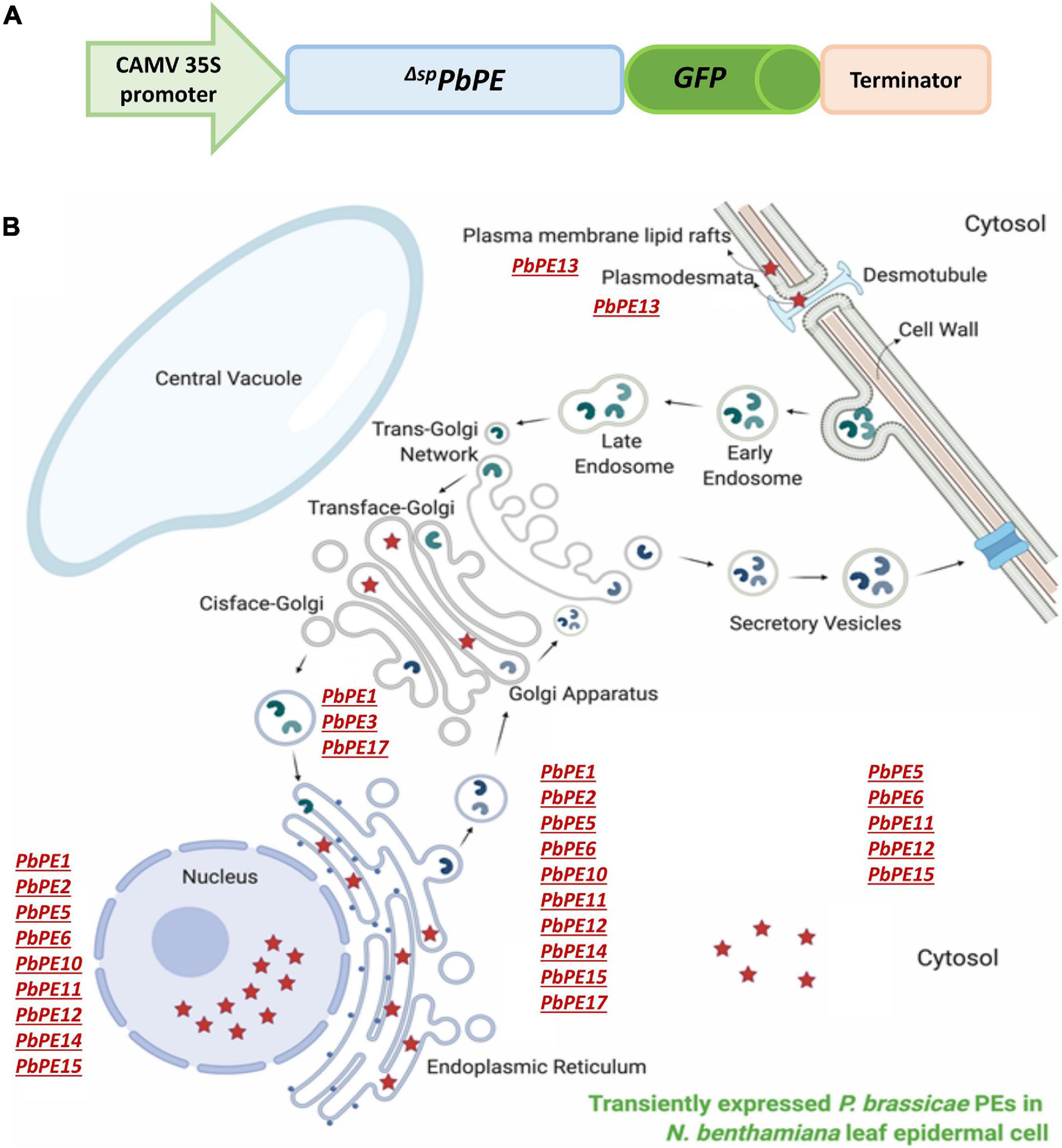
Figure 10. Schematics of the plant endomembrane localization of P. brassicae PEs after transient expression in N. benthamiana leaves. (A) Generation of PH7FWG2 vector-PE gene constructs for subcellular localization (not scaled). (B) Schematic of the localization of transiently expressed PbPEs in distinct sub-compartments in a host cell. Red stars denote P. brassicae PEs.
The ER is the gateway to the cell’s secretory pathway, hosting the co-translational translocation of secretory proteins, protein folding, quality control (QC) system and ER stress response. The interconnected tubular network of the ER can extend throughout the cytoplasm from early endosome to PM, to cell-to-cell junctions, facilitating communication and the regulation of adaptive biotic stress responses. Due to both the receptive and the responsive nature of the ER (Breeze et al., 2020), it is a common subcellular target of pathogen effectors to manipulate host immunity and to hijack the secretory pathway to enable completion of the pathogen life cycle (McLellan et al., 2013; Jing et al., 2016; Fan et al., 2018; Meisrimler et al., 2019; Tsai et al., 2019). In oomycetes up to 17% of the effector secretome targets ER localized host proteins, e.g., ER-localized NAC transcription factors and ER luminal binding immunoglobulin proteins (BiPs; Khan et al., 2018a). ER proteins, such as the NAC transcription factors, are translocated to the nucleus to regulate gene expression, possibly carrying the pathogen effector protein with them into the nucleus (McLellan et al., 2013; Duan et al., 2017; Meisrimler et al., 2019).
Of the 12 endomembrane-targeting PbPEs identified in this study, nine (PbPE1, PbPE2, PbPE5, PbPE6, PbPE10, PbPE11, PbPE12, PbPE14, and PbPE15) showed ER, as well as nuclear localization in N. benthamiana leaf cells. Six of these effectors were found to suppress either PiINF1 and/or PiNPP1-triggered PCD. The most significant inhibition of PCD was observed with ΔspPbPE15, a predicted member of the 2OG and Fe (II)-dependent oxygenase superfamily. A tobacco rattle virus (TRV)-based, host-induced gene silencing (HIGS) of 2OG-Fe(II) oxygenase compromised the pathogenesis of Rhizoctonia solani in tomato plants (Ghosh et al., 2020). Furthermore, treating P. brassicae infected A. thaliana, with an inhibitor of oxoglutaric acid-dependent dioxygenase, resulted in a decreased severity of clubroot formation (Päsold et al., 2013). As a predicted member of this superfamily, it would be appropriate for ΔspPbPE15 to have a role in the processes leading to clubroot formation, including inhibitory effects on defense signaling in plants.
Several studies have previously shown that ER localization of pathogen effectors result in suppression of the pathogen-induced ER stress response and manipulation of trafficking routes to facilitate infection (Qiang et al., 2012; Jing et al., 2016; Fan et al., 2018). The RXLR effector, PcAvr3a12 from Phytophthora capsici, targets and inhibits a novel ER-localized plant peptidyl-prolyl cis-trans isomerase (PPIase), FKBP15-2, to facilitate infection by suppressing ER stress-mediated immunity (Fan et al., 2018). Similarly, the effector PsAvh262, from the soybean pathogen Phytophthora sojae, suppresses ER stress-triggered cell death and aids P. sojae infection by stabilizing plant ER luminal BiPs, thereby preventing them from participating in the ER-localized unfolded protein-related pro-survival response (Qiang et al., 2012; Jing et al., 2016). The inhibition of the ER-stress related defense mechanism can also result from the blocking of translocation of NAC transcription factors from the ER to the nucleus by RXLR effectors (McLellan et al., 2013; Meisrimler et al., 2019).
While PbPE3 localized specifically to the plant Golgi bodies, suggesting a role in manipulating plant cell vesicle trafficking, as seen in Bartetzko et al. (2009), two P. brassicae ankyrin (ANK) repeat-containing proteins, ΔspPbPE1GFP and ΔspPbPE17GFP, localized to both ER and Golgi, with ΔspPbPE1GFP additionally showing nuclear localization. ANK repeat-containing proteins, present in all eukaryotes and some prokaryotes (Mosavi et al., 2004), have diverse functions including roles in signal transduction, vesicular trafficking, disease resistance, reactive oxygen production, biotic and abiotic stress responses, cell cycle regulation, and control of gene transcription (Mou et al., 2013; Böttner et al., 2009; Li and Chye, 2004; Sakamoto et al., 2008; Yang et al., 2008; Ge and Shao, 2011; Yuan et al., 2013; Sharma and Pandey, 2016). ANK domains form molecular scaffolds for protein-protein interactions and in some intracellular pathogens, ANK repeat-containing proteins are essential virulence factors, for example in animals, the Legionella pneumophila AnkX protein prevents the fusion of the L. pneumophila-containing vacuole with late endosomes in infected macrophages as well as interfering with microtubule-dependent transport of ER-derived vesicles (Pan et al., 2008). A number of ANK repeat-containing effector proteins have been identified in the P. brassicae transcriptome, being expressed in both the primary and secondary infection stages in host plants (Chen et al., 2019; Pérez-López et al., 2020).
In a plant–pathogen interaction, the PM is one of the first barriers to infection by many pathogens. Located within the PM are distinct pattern recognition receptors (PRRs) that recognize microbial patterns and induce PTI responses to terminate or contain an infection (Hammond-Kosack and Jones, 1996; Jones and Dangl, 2006). PRRs are often located in cholesterol-rich lipid microdomains or lipid rafts, dynamically assembled and disassembled within the PM (Vieira et al., 2010) and targeted by some protozoan pathogens that have developed methods to escape PRR recognition (Mañes et al., 2003; Hartlova et al., 2010). Plasmodium falciparum, the causative pathogen for malaria, enters targeted cells via lipid rafts, whereas depleting lipid rafts of their cholesterol content was found to prevent infection (Lauer et al., 2000; Samuel et al., 2001). Other PM or ER-localized proteins that reside in close proximity of plasmodesmata, may prove to be targets of P. brassicae effectors to enhance pathogen cell to cell movement (Ueki and Citovsky, 2014; Sun et al., 2019).
Interestingly, ΔspPbPE13GFP, highly expressed during the secondary infection stage of P. brassicae infection, localized to PM lipid rafts associated with plasmodesmata (PD). Significant lipid enrichment in the raft arrangement around PD, compared with the neighboring PM, providing a perfect medium for immune signaling and cell to cell communication by many glycosylphosphatidylinositol (GPI)-anchored and PD-localized proteins, has previously been reported (Malinsky et al., 2013; Grison et al., 2015; Sager and Lee, 2018; Sasaki et al., 2018; Jaillais and Ott, 2020). The lipid raft marker Remorin has been detected in association with PD, where they are thought to modulate the PD size exclusion limit (SEL) and regulate pathogen movement across the raft enriched PM (Raffaele et al., 2009; Perraki et al., 2014; Gronnier et al., 2017; Sasaki et al., 2018). In N. benthamiana, the Pseudomonas syringae effector HopZ1a, which interferes with early immune signaling at the PM in plants, interacts with the lipid raft localized protein REMORIN4 (NbREM4) (Albers et al., 2019). Similarly in Arabidopsis, the P. syringae effector HopF2, which suppresses the PTI response by interacting with the BAK1 receptor at the PM, is also associated with the remorins AtREM1.2 and AtREM1.3 (Zhou et al., 2014; Khan et al., 2018b). Recently, cell to cell movement of P. brassicae plasmodial structures, as tracked by PbBMST movement, has been reported during infection (Badstöber et al., 2020). The association of ΔspPbPE13GFP with remorins linked with PD suggests a role in pathogen spread, possibly through regulation of the plant PTI response. The compartmentalization of ΔspPbPE13GFP fluorescence at the PM remorin-PD nanodomains, after flg22 treatment, supports this suggestion. flg22 interacts with Flagellin Sensing-2 (FLS-2), associated with lipid rafts and triggers the endocytosis of the FLS2-flg22 complex into endosomes that are sorted at the trans-Golgi network and targeted for degradation, probably in the vacuole (Jelenska et al., 2017). A similar pattern of fluorescence distribution, due to membrane raft reorganization, has been observed for GFP-NbREM4 and FLS2, after flg22 treatment (Keinath et al., 2010; Albers et al., 2019). An increase in B. rapa FLS2 (BraA09g021780.3C) transcript levels has been observed after infection with a virulent strain (SCCD-52) of P. brassicae (Fu et al., 2019). The association of ΔspPbPE13GFP with this complex suggests that it may have a role in hijacking the endocytosis response and redirecting the process toward a root-wide distribution of the pathogen.
None of the PbPEs, in this study, contain HDEL/KDEL ER retention signals. As such, the localization of these PbPEs to the endomembrane system would be the result of yet to be identified self-contained signals or host-mediated modification such as lipidation via S−palmitoylation, N−myristoylation or prenylation, previously reported for correct subcellular localization, membrane association and translocation from one cellular compartment to another, of pathogen effectors (Hicks and Galán, 2013; Escoll et al., 2016; Popa et al., 2016). Likewise, none of the PbPEs that also localized to the nucleus (nine of the 12) contain any recognized nuclear localization signals (NLSs). While it is possible that nuclear localization resulted from the overexpression and diffusion of PbPE-GFP to the nucleus, as was seen for GFP alone (Figure 2B; Wang and Brattain, 2007), it is probable that the PbPEs were either targeted through yet to be identified self-contained signals (Savada and Bonham-Smith, 2013; Bourgeois et al., 2020; Tessier et al., 2020) or, due to the contiguous nature of the ER with the perinuclear membrane, localization resulted from the translocation or diffusion of PbPE-GFPs from the ER lumen into the nucleus.
All of these studies were carried out in a heterologous (tobacco) system and we have to recognize that PbPE localization could be a result of the system, as well, studying effector function in isolation may not reflect the true function when studied in combination with other effectors during a natural and spontaneous P. brassicae infection in host plants. Identifying P. brassicae PEs and deciphering their roles in the regulation of plant immunity during disease development will be important for understanding plant–pathogen interactions during clubroot establishment and the design of effective control strategies against this devastating pathogen.
Data Availability Statement
The original contributions presented in the study are included in the article/ Supplementary Material, further inquiries can be directed to the corresponding author.
Author Contributions
MH, EP-L, CT, YW, and PB-S designed the research, and wrote and edited the manuscript. MH performed the research. All authors contributed to the article and approved the submitted version.
Funding
This work was supported by the Saskatchewan Agriculture Development Fund (SADF #20160138) and the Saskatchewan Canola Development Commission (SaskCanola #20160138) funding to PB-S, YW, and CT.
Conflict of Interest
The authors declare that the research was conducted in the absence of any commercial or financial relationships that could be construed as a potential conflict of interest.
Supplementary Material
The Supplementary Material for this article can be found online at: https://www.frontiersin.org/articles/10.3389/fmicb.2021.651279/full#supplementary-material
Supplementary Figure 1 | Identification and selection of P. brassicae putative effectors (PbPEs) from B. napus clubroot gall cDNA library. Bioinformatics tools and parameters used in a signal peptide prediction pipeline to identify PEs of P. brassicae. (A) Prediction of P. brassicae secretome – cDNA sequences from a 35 DPI canola gall cDNA library were trimmed and filtered for the removal of duplicate sequences. Non-redundant sequence reads were then mapped against the B. napus and P. brassicae genomes to identify pathogen specific sequences using Spliced Transcripts Alignment to a Reference (STAR: Dobin et al., 2013). P. brassicae secretome sequences were identified from a BlastX search against the P. brassicae non-redundant proteome and positive hits were then searched by SignalP 4.1, with a D-cut-off score above or equal to 0.5, for the presence of a signal peptide (SP). (B) Identification of PbPE candidates – P. brassicae secretory sequence reads with transmembrane domain(s) and ER retention signals (HDEL, KDEL) were removed from the list. The final group of PbPE candidates was identified after signal peptide prediction using SignalP 5.0 (http://www.cbs.dtu.dk/services/SignalP-5.0/) with a D-cut-off score above or equal to 0.7. “n” denotes the number of reads belonging to a specific group in the pipeline. Encircled numbers specify a step in the pipeline and its detailed information. DPI = days post inoculation, STAR = spliced transcripts alignment to a reference, TMD = transmembrane domain, ER = endoplasmic reticulum.
Supplementary Figure 2 | Nucleo-cytoplasmic localization with ER fractions of ΔspPbPE5GFP, ΔspPbPE6GFP, ΔspPbPE11GFP, and ΔspPbPE12GFP in N. benthamiana leaf epidermal cells. The PbPEs show nucleo-cytoplasmic localization with some accumulation and distribution in the ER. Co-localization of ΔspPbPE5GFP, ΔspPbPE6GFP, ΔspPbPE11GFP and ΔspPbPE12GFP with the ER marker (CD3-959) in N. benthamiana leaf epidermal cells. Confocal images show nuclear localization of the effectors excluding nucleolar localization. Surface views of the cell show cortical ER association of the effector in N. benthamiana. White arrowheads indicate punctate structures of PbPE localization. Scale bars = 10 μm.
Supplementary Table 1 | List of Plasmodiophora brassicae effectors and their functional details. All the predictions and calculations done with the PbPE sequences using different web servers are mentioned in the materials and methods. ¥ denotes GenBank accession number and the number range in bracket indicates nucleotide range of the protein coding sequence (CDS). Domain information of the proteins were identified using conserved domain database (CDD) search. C-site = AA = amino acid, Ch = chloroplast, cleavage site, mito = mitochondria, MW = molecular weight, NA = not available, nuc = nucleus, nt = nucleotide, and pI = isoelectric point.
Supplementary Table 2 | List of plasmid constructs used in this study.
Supplementary File 1 | Time-lapse of ΔspPbPE17GFP colocalization with ER marker. Red fluorescence corresponding to the ER marker, mCherry-HDEL (CD3-959) shows the cortical ER pattern and the yellow signal indicates the merge of green and red fluorescence channels.
Supplementary File 2 | Time-lapse of ΔspPbPE17GFP colocalization with Golgi marker. Yellow fluorescence indicates the merge of punctate green fluorescence from ΔspPbPE17GFP co-localizes with the cis-faced Golgi stack marker, GmMan11–49aa-mCherry (CD3-968) in plants.
Footnotes
- ^ https://www.ebi.ac.uk/Tools/msa/muscle/
- ^ http://weizhongli-lab.org/cd-hit/
- ^ https://www.expasy.org/tools/
- ^ https://www.genscript.com/sms2/orf_find.html
- ^ http://www.cbs.dtu.dk/services/SignalP-5.0/
- ^ http://www.cbs.dtu.dk/services/TMHMM/
- ^ https://www.ebi.ac.uk/Tools/pfa/phobius/
- ^ https://prosite.expasy.org/scanprosite/
- ^ https://www.ebi.ac.uk/Tools/hmmer/
- ^ http://ekhidna2.biocenter.helsinki.fi/sanspanz/
- ^ https://www.ncbi.nlm.nih.gov/Structure/cdd/wrpsb.cgi
- ^ https://web.expasy.org/protparam/
- ^ http://localizer.csiro.au/
- ^ https://www.fishersci.nl/shop/products/software-vision-works-ls-complete-software/12568355
- ^ https://abrc.osu.edu/
- ^ https://imagej.net/Fiji
References
Albers, P., Üstün, S., Witzel, K., Kraner, M., and Börnke, F. (2019). A remorin from Nicotiana benthamiana interacts with the Pseudomonas Type-III effector protein HopZ1a and is phosphorylated by the immune-related kinase PBS1. Mol. Plant Microbe Interact. 32, 1229–1242. doi: 10.1094/MPMI-04-19-0105-R
Badstöber, J., Gachon, C., Ludwig-Müller, J., Sandbichler, A. M., and Neuhauser, S. (2020). Demystifying biotrophs: FISHing for mRNAs to decipher plant and algal pathogen-host interaction at the single cell level. Sci. Rep. 10:14269. doi: 10.1038/s41598-020-70884-4
Bartetzko, V., Sonnewald, S., Vogel, F., Hartner, K., Stadler, R., Hammes, U. Z., et al. (2009). The Xanthomonas campestris pv. vesicatoria type III effector protein XopJ inhibits protein secretion: evidence for interference with cell wall-associated defense responses. Mol. Plant Microbe Interactions 22, 655–664. doi: 10.1094/MPMI-22-6-0655
Böttner, S., Iven, T., Carsjens, C. S., and Dröge-Laser, W. (2009). Nuclear accumulation of the ankyrin repeat protein ANK1 enhances the auxin-mediated transcription accomplished by the bZIP transcription factors BZI-1 and BZI-2. Plant J. 58, 914–926. doi: 10.1111/j.1365-313X.2009.03829.x
Bourgeois, B., Hutten, S., Gottschalk, B., Hofweber, M., Richter, G., Sternat, J., et al. (2020). Nonclassical nuclear localization signals mediate nuclear import of CIRBP. Proc. Natl. Acad. Sci. USA 117, 8503–8514. doi: 10.1073/pnas.1918944117
Breeze, E., Vale, V., McLellan, H., Godiard, L., Grant, M., and Frigerio, L. (2020). The plant endoplasmic reticulum is both receptive and responsive to pathogen effectors. BioRxiv [Preprint]. Available Online at: https://doi.org/10.1101/2020.06.09.142141.
Bulman, S., Richter, F., Marschollek, S., Benade, F., Jülke, S., and Ludwig-Müller, J. (2019). Arabidopsis thaliana expressing PbBSMT, a gene encoding a SABATH-type methyltransferase from the plant pathogenic protist Plasmodiophora brassicae, show leaf chlorosis and altered host susceptibility. Plant Biol. 21, (Suppl. 1), 120–130. doi: 10.1111/plb.12728
Chen, W., Li, Y., Yan, R., Xu, L., Ren, L., Liu, F., et al. (2019). Identification and characterization of Plasmodiophora brassicae primary infection effector candidates that suppress or induce cell death in host and nonhost plants. Phytopathology 109, 1689–1697. doi: 10.1094/PHYTO-02-19-0039-R
Chinchilla, D., Bauer, Z., Regenass, M., Boller, T., and Felix, G. (2006). The Arabidopsis receptor kinase FLS2 binds flg22 and determines the specificity of flagellin perception. Plant Cell 18, 465–476. doi: 10.1105/tpc.105.036574
Dobin, A., Davis, C. A., Schlesinger, F., Drenkow, J., Zaleski, C., Jha, S., et al. (2013). STAR: ultrafast universal RNA-seq aligner. Bioinformatics 29, 15–21. doi: 10.1093/bioinformatics/bts635
Duan, M., Zhang, R., Zhu, F., Zhang, Z., Gou, L., Wen, J., et al. (2017). A lipid-anchored NAC transcription factor is translocated into the nucleus and activates Glyoxalase I expression during drought stress. Plant Cell 29, 1748–1772. doi: 10.1105/tpc.17.00044
Engelhardt, S., Boevink, P. C., Armstrong, M. R., Ramos, M. B., Hein, I., and Birch, P. R. (2012). Relocalization of late blight resistance protein R3a to endosomal compartments is associated with effector recognition and required for the immune response. Plant Cell 24, 5142–5158. doi: 10.1105/tpc.112.104992
Escoll, P., Mondino, S., Rolando, M., and Buchrieser, C. (2016). Targeting of host organelles by pathogenic bacteria: a sophisticated subversion strategy. Nat. Rev. Microbiol. 14, 5–19. doi: 10.1038/nrmicro.2015.1
Ewing, B., and Green, P. (1998). Base-calling of automated sequencer traces using phred. II. error probabilities. Genome Res. 8, 186–194. doi: 10.1101/gr.8.3.186
Ewing, B., Hillier, L., Wendl, M. C., and Green, P. (1998). Base-calling of automated sequencer traces using phred. i. accuracy assessment. Genome Res. 8, 175–185. doi: 10.1101/gr.8.3.175
Fan, G., Yang, Y., Li, T., Lu, W., Du, Y., Qiang, X., et al. (2018). A Phytophthora capsici RXLR effector targets and inhibits a plant PPIase to suppress endoplasmic reticulum-mediated immunity. Mol. Plant 11, 1067–1083. doi: 10.1016/j.molp.2018.05.009
Fu, P., Piao, Y., Zhan, Z., Zhao, Y., Pang, W., Li, X., et al. (2019). Transcriptome profile of Brassica rapa L. reveals the involvement of jasmonic acid, ethylene, and brassinosteroid signaling pathways in clubroot resistance. Agronomy 9:589. doi: 10.3390/agronomy9100589
Fuchs, H., and Sacristan, M. D. (1996). Identification of a gene in Arabidopsis thaliana controlling resistance to clubroot (Plasmodiophora brassicae) and characterization of the resistance response. Mol. Plant Microbe Interact. 9, 91–97. doi: 10.1094/MPMI-9-0091
Ge, J., and Shao, F. (2011). Manipulation of host vesicular trafficking and innate immune defence by Legionella Dot/Icm effectors. Cell Microbiol. 13, 1870–1880. doi: 10.1111/j.1462-5822.2011.01710.x
Ghosh, S., Kant, R., Pradhan, A., and Jha, G. (2020). RS_CRZ1, a C2H2-type transcription factor is required for pathogenesis of Rhizoctonia solani AG1-IA in tomato. Mol. Plant Microbe Interact. 34, 26–38. doi: 10.1094/MPMI-05-20-0121-R
Gietz, R. D., and Woods, R. A. (2002). Transformation of yeast by lithium acetate/single-stranded carrier DNA/polyethylene glycol method. Methods Enzymol. 350, 87–96. doi: 10.1016/S0076-6879(02)50957-5
Grison, M. S., Brocard, L., Fouillen, L., Nicolas, W., Wewer, V., Dörmann, P., et al. (2015). Specific membrane lipid composition is important for plasmodesmata function in Arabidopsis. Plant Cell 27, 1228–1250. doi: 10.1105/tpc.114.135731
Gronnier, J., Crowet, J. M., Habenstein, B., Nasir, M. N., Bayle, V., Hosy, E., et al. (2017). Structural basis for plant plasma membrane protein dynamics and organization into functional nanodomains. eLife 6:e26404. doi: 10.7554/eLife.26404
Gu, Y., Zavaliev, R., and Dong, X. (2017). Membrane trafficking in plant immunity. Mol. Plant 10, 1026–1034. doi: 10.1016/j.molp.2017.07.001
Hammond-Kosack, K. E., and Jones, J. D. (1996). Resistance gene-dependent plant defense responses. Plant Cell 8, 1773–1791. doi: 10.1105/tpc.8.10.1773
Hartlova, A., Cerveny, L., Hubalek, M., Krocova, Z., and Stulik, J. (2010). Membrane rafts: a potential gateway for bacterial entry into host cells. Microbiol. Immunol. 54, 237–245. doi: 10.1111/j.1348-0421.2010.00198.x
Hicks, S. W., and Galán, J. E. (2013). Exploitation of eukaryotic subcellular targeting mechanisms by bacterial effectors. Nat. Rev. Microbiol. 11, 316–326. doi: 10.1038/nrmicro3009
Irani, S., Trost, B., Waldner, M., Nayidu, N., Tu, J., Kusalik, A. J., et al. (2018). Transcriptome analysis of response to Plasmodiophora brassicae infection in the Arabidopsis shoot and root. BMC Genom. 19:23. doi: 10.1186/s12864-017-4426-7
Jaillais, Y., and Ott, T. (2020). The nanoscale organization of the plasma membrane and its importance in signaling: a proteolipid perspective. Plant Physiol. 182, 1682–1696. doi: 10.1104/pp.19.01349
Jelenska, J., Davern, S. M., Standaert, R. F., Mirzadeh, S., and Greenberg, J. T. (2017). Flagellin peptide flg22 gains access to long-distance trafficking in Arabidopsis via its receptor. FLS2. J. Exp. Bot. 68, 1769–1783. doi: 10.1093/jxb/erx060
Jin, C., Liao, R., Zheng, J., Fang, Y., Wang, W., Fan, J., et al. (2020). MAPKKK7 from Plasmodiophora brassicae regulates low-light-dependent Nicotiana benthamiana immunity. Phytopathology doi: 10.1094/PHYTO-08-20-0323-R Online ahead of print.
Jing, M., Guo, B., Li, H., Yang, B., Wang, H., Kong, G., et al. (2016). A Phytophthora sojae effector suppresses endoplasmic reticulum stress-mediated immunity by stabilizing plant binding immunoglobulin proteins. Nat. Commun. 7:11685. doi: 10.1038/ncomms11685
Jones, J. D., and Dangl, J. L. (2006). The plant immune system. Nature 444, 323–329. doi: 10.1038/nature05286
Karimi, M., Inzé, D., and Depicker, A. (2002). GATEWAY vectors for Agrobacterium-mediated plant transformation. Trends Plant Sci. 7, 193–195. doi: 10.1016/S1360-1385(02)02251-3
Keinath, N. F., Kierszniowska, S., Lorek, J., Bourdais, G., Kessler, S. A., Shimosato-Asano, H., et al. (2010). PAMP (pathogen-associated molecular pattern)-induced changes in plasma membrane compartmentalization reveal novel components of plant immunity. J. Biol. Chem. 285, 39140–39149. doi: 10.1074/jbc.M110.160531
Kelley, B. S., Lee, S. J., Damasceno, C. M., Chakravarthy, S., Kim, B. D., Martin, G. B., et al. (2010). A secreted effector protein (SNE1) from Phytophthora infestans is a broadly acting suppressor of programmed cell death. Plant J. 62, 357–366. doi: 10.1111/j.1365-313X.2010.04160.x
Khan, M., Seto, D., Subramaniam, R., and Desveaux, D. (2018a). Oh, the places they’ll go! a survey of phytopathogen effectors and their host targets. Plant J. 93, 651–663. doi: 10.1111/tpj.13780
Khan, M., Youn, J. Y., Gingras, A. C., Subramaniam, R., and Desveaux, D. (2018b). In planta proximity dependent biotin identification (BioID). Sci. Rep. 8:9212. doi: 10.1038/s41598-018-27500-3
Langin, G., Gouguet, P., and Üstün, S. (2020). Microbial effector proteins – a journey through the proteolytic landscape. Trends Microbiol. 28, 523–535. doi: 10.1016/j.tim.2020.02.010
Lauer, S., VanWye, J., Harrison, T., McManus, H., Samuel, B. U., Hiller, N. L., et al. (2000). Vacuolar uptake of host components, and a role for cholesterol and sphingomyelin in malarial infection. EMBO J. 19, 3556–3564. doi: 10.1093/emboj/19.14.3556
Lefebvre, B., Furt, F., Hartmann, M. A., Michaelson, L. V., Carde, J. P., Sargueil-Boiron, F., et al. (2007). Characterization of lipid rafts from Medicago truncatula root plasma membranes: a proteomic study reveals the presence of a raft-associated redox system. Plant Physiol. 144, 402–418. doi: 10.1104/pp.106.094102
Li, H. Y., and Chye, M. L. (2004). Arabidopsis Acyl-CoA-binding protein ACBP2 interacts with an ethylene-responsive element-binding protein, AtEBP, via its ankyrin repeats. Plant Mol. Biol. 54, 233–243. doi: 10.1023/B:PLAN.0000028790.75090.ab
Ludwig-Müller, J., Jülke, S., Geiß, K., Richter, F., Mithöfer, A., Šola, I., et al. (2015). A novel methyltransferase from the intracellular pathogen Plasmodiophora brassicae methylates salicylic acid. Molecular Plant Pathology 16, 349–364. doi: 10.1111/mpp.12185
Malinsky, J., Opekarová, M., Grossmann, G., and Tanner, W. (2013). Membrane microdomains, rafts, and detergent-resistant membranes in plants and fungi. Annu. Rev. Plant Biol. 64, 501–529. doi: 10.1146/annurev-arplant-050312-120103
Mañes, S., del Real, G., and Martínez-A, C. (2003). Pathogens: raft hijackers. Nat. Rev. Immunol. 3, 557–568. doi: 10.1038/nri1129
McLellan, H., Boevink, P. C., Armstrong, M. R., Pritchard, L., Gomez, S., Morales, J., et al. (2013). An RxLR effector from Phytophthora infestans prevents re-localisation of two plant NAC transcription factors from the endoplasmic reticulum to the nucleus. PLoS Pathog. 9:e1003670. doi: 10.1371/journal.ppat.1003670
Meisrimler, C. N., Pelgrom, A., Oud, B., Out, S., and Van den Ackerveken, G. (2019). Multiple downy mildew effectors target the stress-related NAC transcription factor LsNAC069 in lettuce. Plant J. 99, 1098–1115. doi: 10.1111/tpj.14383
Missihoun, T. D., Schmitz, J., Klug, R., Kirch, H. H., and Bartels, D. (2011). Betaine aldehyde dehydrogenase genes from Arabidopsis with different sub-cellular localization affect stress responses. Planta 233, 369–382. doi: 10.1007/s00425-010-1297-4
Morel, J., Claverol, S., Mongrand, S., Furt, F., Fromentin, J., Bessoule, J. J., et al. (2006). Proteomics of plant detergent-resistant membranes. Mol. Cell. Proteomics 5, 1396–1411. doi: 10.1074/mcp.M600044-MCP200
Mosavi, L. K., Cammett, T. J., Desrosiers, D. C., and Peng, Z. Y. (2004). The ankyrin repeat as molecular architecture for protein recognition. Protein Sci. 13, 1435–1448. doi: 10.1110/ps.03554604
Mou, S., Liu, Z., Guan, D., Qiu, A., Lai, Y., and He, S. (2013). Functional analysis and expressional characterization of rice ankyrin repeat-containing protein, OsPIANK1, in basal defense against Magnaporthe oryzae attack. PLoS One 8:e59699. doi: 10.1371/journal.pone.0059699
Oh, S. K., Young, C., Lee, M., Oliva, R., Bozkurt, T. O., Cano, L. M., et al. (2009). In planta expression screens of Phytophthora infestans RXLR effectors reveal diverse phenotypes, including activation of the Solanum bulbocastanum disease resistance protein Rpi-blb2. Plant Cell 21, 2928–2947. doi: 10.1105/tpc.109.068247
Pan, X., Lührmann, A., Satoh, A., Laskowski-Arce, M. A., and Roy, C. R. (2008). Ankyrin repeat proteins comprise a diverse family of bacterial type IV effectors. Science 320, 1651–1654. doi: 10.1126/science.1158160
Päsold, S., and Ludwig−Müller, J. (2013). Reduction of clubroot (Plasmodiophora brassicae) formation in Arabidopsis thaliana after treatment with prohexadione-calcium, an inhibitor of oxoglutaric acid-dependent dioxygenases. Plant Pathol. 62, 1357–1365. doi: 10.1111/ppa.12049
Pérez-López, E., Hossain, M. M., Tu, J., Waldner, M., Todd, C. D., Kusalik, A. J., et al. (2020). Transcriptome analysis identifies Plasmodiophora brassicae secondary infection effector candidates. J. Eukaryot. Microbiol. 67, 337–351. doi: 10.1111/jeu.12784
Pérez-López, E., Waldner, M., Hossain, M., Kusalik, A. J., Wei, Y., Bonham-Smith, P. C., et al. (2018). Identification of Plasmodiophora brassicae effectors - a challenging goal. Virulence 9, 1344–1353. doi: 10.1080/21505594.2018.1504560
Perraki, A., Binaghi, M., Mecchia, M. A., Gronnier, J., German-Retana, S., Mongrand, S., et al. (2014). StRemorin1.3 hampers potato virus X TGBp1 ability to increase plasmodesmata permeability, but does not interfere with its silencing suppressor activity. FEBS Lett. 588, 1699–1705. doi: 10.1016/j.febslet.2014.03.014
Popa, C. M., Tabuchi, M., and Valls, M. (2016). Modification of bacterial effector proteins inside eukaryotic host cells. Front. Cell. Infection Microbiol. 6:73. doi: 10.3389/fcimb.2016.00073
Qiang, X., Zechmann, B., Reitz, M. U., Kogel, K. H., and Schäfer, P. (2012). The mutualistic fungus Piriformospora indica colonizes Arabidopsis roots by inducing an endoplasmic reticulum stress-triggered caspase-dependent cell death. Plant Cell 24, 794–809. doi: 10.1105/tpc.111.093260
Raffaele, S., Bayer, E., Lafarge, D., Cluzet, S., German Retana, S., Boubekeur, T., et al. (2009). Remorin, a solanaceae protein resident in membrane rafts and plasmodesmata, impairs potato virus X movement. Plant Cell 21, 1541–1555. doi: 10.1105/tpc.108.064279
Rolfe, S. A., Strelkov, S. E., Links, M. G., Clarke, W. E., Robinson, S. J., Djavaheri, M., et al. (2016). The compact genome of the plant pathogen Plasmodiophora brassicae is adapted to intracellular interactions with host Brassica spp. BMC Genom. 17:272. doi: 10.1186/s12864-016-2597-2
Sager, R. E., and Lee, J. Y. (2018). Plasmodesmata at a glance. J. Cell Sci. 131:jcs209346. doi: 10.1242/jcs.209346
Sakamoto, H., Matsuda, O., and Iba, K. (2008). ITN1, a novel gene encoding an ankyrin-repeat protein that affects the ABA-mediated production of reactive oxygen species and is involved in salt-stress tolerance in Arabidopsis thaliana. Plant J. 56, 411–422. doi: 10.1111/j.1365-313X.2008.03614.x
Samuel, B. U., Mohandas, N., Harrison, T., McManus, H., Rosse, W., Reid, M., et al. (2001). The role of cholesterol and glycosylphosphatidylinositol-anchored proteins of erythrocyte rafts in regulating raft protein content and malarial infection. J. Biol. Chem. 276, 29319–29329. doi: 10.1074/jbc.M101268200
Sasaki, N., Takashima, E., and Nyunoya, H. (2018). Altered subcellular localization of a tobacco membrane raft-associated remorin protein by tobamovirus infection and transient expression of viral replication and movement proteins. Front. Plant Sci. 9:619. doi: 10.3389/fpls.2018.00619
Savada, R. P., and Bonham-Smith, P. C. (2013). Charge versus sequence for nuclear/nucleolar localization of plant ribosomal proteins. Plant Mol. Biol. 81, 477–493. doi: 10.1007/s11103-013-0017-4
Schwelm, A., Fogelqvist, J., Knaust, A., Jülke, S., Lilja, T., Bonilla-Rosso, G., et al. (2015). The Plasmodiophora brassicae genome reveals insights in its life cycle and ancestry of chitin synthases. Sci. Rep. 5:11153. doi: 10.1038/srep11153
Shahzad, Z., Ranwez, V., Fizames, C., Marquès, L., Le Martret, B., Alassimone, J., et al. (2013). Plant defensin type 1 (PDF1): protein promiscuity and expression variation within the Arabidopsis genus shed light on zinc tolerance acquisition in Arabidopsis halleri. New Phytol. 200, 820–833. doi: 10.1111/nph.12396
Sharma, M., and Pandey, G. K. (2016). Expansion and function of repeat domain proteins during stress and development in plants. Front. Plant Sci. 6:1218. doi: 10.3389/fpls.2015.01218
Sparkes, I. A., Runions, J., Kearns, A., and Hawes, C. (2006). Rapid, transient expression of fluorescent fusion proteins in tobacco plants and generation of stably transformed plants. Nat. Protoc. 1, 2019–2025. doi: 10.1038/nprot.2006.286
Strelkov, S., Tewari, J. P., and Smith-Degenhardt, E. (2006). Characterization of Plasmodiophora brassicae populations from Alberta, Canada. Can. J. Plant Pathol. 28, 467–474. doi: 10.1080/07060660609507321
Sun, Y., Huang, D., and Chen, X. (2019). Dynamic regulation of plasmodesmatal permeability and its application to horticultural research. Hortic. Res. 6:47. doi: 10.1038/s41438-019-0129-3
Teh, O. K., and Hofius, D. (2014). Membrane trafficking and autophagy in pathogen-triggered cell death and immunity. J. Exp. Bot. 65, 1297–1312. doi: 10.1093/jxb/ert441
Tessier, T. M., MacNeil, K. M., and Mymryk, J. S. (2020). Piggybacking on classical import and other non-classical mechanisms of nuclear import appear highly prevalent within the human proteome. Biology 9:188. doi: 10.3390/biology9080188
Tsai, A. Y., English, B. C., and Tsolis, R. M. (2019). Hostile takeover: hijacking of endoplasmic reticulum function by T4SS and T3SS effectors creates a niche for intracellular pathogens. Microbiol. Spectrum 7. doi: 10.1128/9781683670285.ch23
Ueki, S., and Citovsky, V. (2014). Plasmodesmata-associated proteins: can we see the whole elephant? Plant Signaling Behav. 9:e27899. doi: 10.4161/psb.27899
Vieira, F. S., Corrêa, G., Einicker-Lamas, M., and Coutinho-Silva, R. (2010). Host-cell lipid rafts: a safe door for micro-organisms? Biol. Cell 102, 391–407. doi: 10.1042/BC20090138
Wang, R., and Brattain, M. G. (2007). The maximal size of protein to diffuse through the nuclear pore is larger than 60kDa. FEBS Lett. 581, 3164–3170. doi: 10.1016/j.febslet.2007.05.082
Yang, X., Sun, C., Hu, Y., and Lin, Z. (2008). Molecular cloning and characterization of a gene encoding RING zinc finger ankyrin protein from drought-tolerant Artemisia desertorum. J. Biosci. 33, 103–112. doi: 10.1007/s12038-008-0026-7
Yu, Y., Xiao, J., Zhu, W., Yang, Y., Mei, J., Bi, C., et al. (2017). Ss-Rhs1, a secretory Rhs repeat-containing protein, is required for the virulence of Sclerotinia sclerotiorum. Mol. Plant Pathol. 18, 1052–1061. doi: 10.1111/mpp.12459
Yuan, X., Zhang, S., Liu, S., Yu, M., Su, H., Shu, H., et al. (2013). Global analysis of ankyrin repeat domain C3HC4-type RING finger gene family in plants. PLoS One 8:e58003. doi: 10.1371/journal.pone.0058003
Keywords: Plasmodiophora brassicae, effectors, endomembrane, programmed cell death, pathogen-associated molecular pattern
Citation: Hossain MM, Pérez-López E, Todd CD, Wei Y and Bonham-Smith PC (2021) Endomembrane-Targeting Plasmodiophora brassicae Effectors Modulate PAMP Triggered Immune Responses in Plants. Front. Microbiol. 12:651279. doi: 10.3389/fmicb.2021.651279
Received: 09 January 2021; Accepted: 31 May 2021;
Published: 01 July 2021.
Edited by:
Georgios Tzelepis, Swedish University of Agricultural Sciences, SwedenReviewed by:
Aiping Zheng, Sichuan Agricultural University, ChinaJutta Ludwig-Müller, Technische Universität Dresden, Germany
Copyright © 2021 Hossain, Pérez-López, Todd, Wei and Bonham-Smith. This is an open-access article distributed under the terms of the Creative Commons Attribution License (CC BY). The use, distribution or reproduction in other forums is permitted, provided the original author(s) and the copyright owner(s) are credited and that the original publication in this journal is cited, in accordance with accepted academic practice. No use, distribution or reproduction is permitted which does not comply with these terms.
*Correspondence: Peta C. Bonham-Smith, cGV0YS5ib25oYW1zQHVzYXNrLmNh