- 1Center for Discovery and Innovation, Hackensack Meridian Health, Nutley, NJ, United States
- 2Department of Biology, William Paterson University, Wayne, NJ, United States
- 3Department of Medical Sciences, Hackensack Meridian School of Medicine, Nutley, NJ, United States
- 4Lombardi Comprehensive Cancer Center, Georgetown University, Washington, DC, United States
Fungal infections are on the rise, and emergence of drug-resistant Candida strains refractory to treatment is particularly alarming. Resistance to azole class antifungals, which have been extensively used worldwide for several decades, is so high in several prevalent fungal pathogens, that another drug class, the echinocandins, is now recommended as a first line antifungal treatment. However, resistance to echinocandins is also prominent, particularly in certain species, such as Candida glabrata. The echinocandins target 1,3-β-glucan synthase (GS), the enzyme responsible for producing 1,3-β-glucans, a major component of the fungal cell wall. Although echinocandins are considered fungicidal, C. glabrata exhibits echinocandin tolerance both in vitro and in vivo, where a subset of the cells survives and facilitates the emergence of echinocandin-resistant mutants, which are responsible for clinical failure. Despite this critical role of echinocandin tolerance, its mechanisms are still not well understood. Additionally, most studies of tolerance are conducted in vitro and are thus not able to recapitulate the fungal-host interaction. In this study, we focused on the role of cell wall integrity factors in echinocandin tolerance in C. glabrata. We identified three genes involved in the maintenance of cell wall integrity – YPS1, YPK2, and SLT2 – that promote echinocandin tolerance both in vitro and in a mouse model of gastrointestinal (GI) colonization. In particular, we show that mice colonized with strains carrying deletions of these genes were more effectively sterilized by daily caspofungin treatment relative to mice colonized with the wild-type parental strain. Furthermore, consistent with a role of tolerant cells serving as a reservoir for generating resistant mutations, a reduction in tolerance was associated with a reduction in the emergence of resistant strains. Finally, reduced susceptibility in these strains was due both to the well described FKS-dependent mechanisms and as yet unknown, FKS-independent mechanisms. Together, these results shed light on the importance of cell wall integrity maintenance in echinocandin tolerance and emergence of resistance and lay the foundation for future studies of the factors described herein.
Introduction
Invasive fungal infections, in particular candidiasis and candidemia, pose a serious threat to immunocompromised individuals and are associated with high mortality rates (Pfaller and Diekema, 2010). Treatment outcomes critically depend on timely and appropriate use of antifungal therapy. However, therapeutic choices for fungal infections are scarce due to a small number of available drug classes and a rise in antifungal drug resistance (Arastehfar et al., 2020). For several decades, Candida albicans has been the predominant infecting Candida species worldwide and azole drugs were the primary therapy to prevent or treat Candida infections. However, extensive use of azoles has led to an epidemiological shift toward non-albicans Candida species with higher azole tolerance or resistance, such as Candida glabrata (Enoch et al., 2017). Therefore, another drug class, the echinocandins, is currently recommended as first line therapy, especially for suspected non-albicans infections (Perlin, 2011). Alarmingly, however, C. glabrata also shows elevated potential relative to other Candida species to rapidly develop resistance to echinocandins, resulting in difficult-to-treat multidrug-resistant infections (Pham et al., 2014).
Echinocandins target the fungal-specific enzyme 1,3-β-D-glucan synthase (GS), which is responsible for the biosynthesis of a major cell wall polymer and is essential for building and maintaining the fungal cell wall (Perlin, 2015a). Echinocandin resistance is caused by mutations in GS-encoding FKS genes, and these mutations are often associated with therapeutic failure (Shields et al., 2012; Alexander et al., 2014). Although echinocandins are considered fungicidal drugs in Candida spp., including C. glabrata, we have shown that whereas the vast majority of C. glabrata cells do die upon echinocandin exposure in vitro, a small subset of cells survives and shows drug tolerance over a wide range of echinocandin concentrations (Healey and Perlin, 2018). In this context, it is important to note that because echinocandins are cidal in Candida, whereas azoles are static, we use the word “tolerance” not to indicate growth above minimum inhibitory concentrations (MICs), as has been defined for azoles (Berman and Krysan, 2020), but to refer to the ability of cells to survive a given echinocandin concentration that is expected to kill cells, usually at or above the MIC. Under this definition, strains with increased tolerance show improved survival, whereas strains with decreased tolerance show reduced survival, and this can be observed at one or more concentrations of the drug. Additionally, because MIC assays measure not survival but growth, changes in echinocandin tolerance may or may not correspond to changes in echinocandin MICs. In vivo, for example in animal models of infection, tolerance is likewise manifested by an incomplete reduction in fungal burdens upon treatment, i.e., in failure to achieve sterilization (Howard et al., 2011). These tolerant cells likely serve as the cellular reservoir in which resistant mutations arise (Shor and Perlin, 2015); however, despite this key role of drug tolerance in development of drug resistance, the factors underlying echinocandin tolerance in C. glabrata are very poorly understood. Furthermore, most studies looking at drug responses of C. glabrata have been conducted in vitro, providing no information on how drug tolerance is affected in vivo.
The fungal cell wall is the target of echinocandin action, and its structure and stability are key factors regulating resistance and tolerance to these drugs. For instance, fungal cells respond to echinocandin exposure by compensatory increases in cell wall chitin content (Walker et al., 2013), and cells with constitutively increased chitin levels have elevated echinocandin resistance (Lee et al., 2012). Conversely, inhibition of chitin biosynthesis by nikkomycin Z, a chitin synthase inhibitor, sensitizes C. glabrata cells to caspofungin treatment (Healey et al., 2017). Because the cell wall is also a key interface with the environment, such as the host, fungal cells contain several signaling pathways responsible for cell wall maintenance, which detect cell wall damage and activate the cellular processes necessary for cell wall stabilization and repair (Dichtl et al., 2016). However, the importance of these pathways in echinocandin tolerance has not yet been examined.
In this study, we show that four genes that function in cell wall integrity maintenance (SLG1, YPS1, YPK2, and SLT2) contribute to echinocandin tolerance in C. glabrata in vitro. Furthermore, we used our murine model of gastrointestinal (GI) colonization to demonstrate that deletion mutants of YPS1, YPK2, and SLT2 showed increased GI decolonization by caspofungin, reduced incidence of fungal burden rebound, and reduced emergence of fks mutations. Our results identify these cell wall integrity pathways as important contributors to echinocandin tolerance in C. glabrata both in vitro and in vivo and highlight them as potential targets for adjuvant antifungal therapies.
Materials and Methods
Ethics Statement
Mice were housed in the Research Animal Facility Biosafety Level-2 at the Center for Discovery and Innovation, Hackensack Meridian Health (Nutley, NJ, United States). The animal facility follows the Public Health Service and National Institute of Health Policy of Humane Care and Use of Laboratory Animals guide. All experimental protocols were approved by the Center for Discovery and Innovation Institutional Animal Care and Use Committee (IACUC).
Yeast Strains and Media
The C. glabrata strains used in this study were ATCC2001HTL, ATCC90030, and the described deletion mutants. Deletion mutants in the ATCC2001HTL background were provided by Dr. Kuchler (Schwarzmüller et al., 2014), whereas deletion mutants in ATCC90030 background were generated in our lab. Cells were cultured in the standard yeast extract-peptone-dextrose (YPD) medium at 37°C, which is the optimal growth temperature for this species.
Construction of Candida glabrata Deletion Mutants
Deletion mutants were generated in-house using a CRISPR-Cas9 targeted integration replacing the desired ORF by a nourseothricin (NAT)-resistance cassette. The deletion construct containing the NAT-resistance cassette flanked by regions homologous to the locus of interest was amplified from genomic DNA of the deletion mutants in ATCC2001HTL background. All primers used are listed in Supplementary Table 1. Integration of the deletion cassettes was performed using CRISPR as described previously (Shor et al., 2019). Briefly, cells were made competent for electroporation using the Frozen-EZ yeast transformation kit (Zymo Research) according to the manufacturer’s instructions and then electroporated with Cas9-gRNA complex and the DNA containing the deletion construct. Transformants were selected on NAT-containing plates and validated by PCR amplification and sequencing of the targeted locus using external primers (Supplementary Table 1). At least two independent transformants were generated for every deletion mutant. All primers were ordered from Integrated DNA Technologies (Coralville, IA, United States), and all Sanger sequencing of the above-described constructs was done by Genewiz (South Plainfield, NJ, United States).
In vitro Tolerance Assay
Fresh 1 ml YPD cultures of 107 C. glabrata cells were incubated at 37°C with shaking (150 rpm) for 24 h in the presence of a range of micafungin or caspofungin concentrations (0, 0.015, 0.06, 0.25, 1, and 4 μg/ml). After 24 h, 0.1 ml of the appropriate dilutions for each culture was plated onto YPD plates. Colony forming units (CFU) were determined and survival percentage was obtained by normalizing the CFU obtained from cultures treated with the indicated concentration of drug to non-treated controls. At least three biological replicates were performed for every strain and condition.
Echinocandin Susceptibility Testing
Micafungin (Astellas, Deerfield, IL, United States) and caspofungin (Merck, Rahway, NJ, United States) susceptibility testing was performed using a broth microdilution method following CLSI standards (Clinical and Laboratory Standards Institute (CLSI), 2017) with some modifications. The media used was YPD broth and the final concentrations tested ranged from 0.0035 to 2 μg/ml in two-fold increasing concentrations. MICs were visually read after 24 h of incubation at 37°C and at least three biological replicates were performed.
Murine Model of Candida glabrata GI Colonization
The GI model of C. glabrata colonization was performed as described (Healey et al., 2017) with some modifications. 6-week-old female CF-1 immunocompetent mice (Charles River Laboratories) were treated subcutaneously, daily from day-2 to 15, with 320 mg/kg of piperacillin-tazobactam (PTZ, 8:1 ratio, AuroMedics Pharma LLC, East Windsor, NJ, United States) to clear native intestinal bacterial microbiota. On day 0, mice were inoculated via oral gavage with approximately 1.5⋅108 CFU of C. glabrata in 0.1 ml of PBS. In this manner mice were colonized with ATCC2001HTL, ATCC90030 or their derivative mutants. Daily administration of 20 mg/kg of caspofungin (Merck, Rahway, NJ, United States) or PBS intraperitoneal was initiated on day 3 post inoculation and continued through day 15. Fresh fecal samples were collected every other day throughout the experiment to assess fungal burden in the GI tract.
The experiment comparing ATCC2001HTL and ATCC90030 strains, contained six mice in the caspofungin treated group. Additionally, two mice were in the colonization control group (treated with PBS alone) for each strain. In the experiment studying the effects of cell wall integrity mutants, the caspofungin treated groups contained five mice per deletion mutant; since two independent mutants were analyzed per deletion, ten mice were analyzed in total per one gene deletion. Also for every deletion, one mouse was included as an untreated control (treated with PBS alone). All mice in the PBS alone control groups maintained a constant fungal burden of 106–108 CFU/g of stool.
Determination of in vivo Caspofungin Resistance Development
Caspofungin-resistant colonies were identified through selection of fecal samples on YPD plates supplemented with caspofungin (2 μg/ml), PTZ (16 μg/ml), and chloramphenicol (20 μg/ml), followed by PCR amplification and sequencing of FKS1 and FKS2. Since clinical echinocandin resistance in C. glabrata is nearly always associated to amino acid substitutions in the hotspot regions of FKS1 and FKS2 (Perlin, 2015b), we sequenced a region of 0.8–1 kb containing each hotspot to ensure detection of all mutations associated with a change in susceptibility (Supplementary Table 1).
Non-Candida glabrata Species Identification
When species specific FKS1/2 primers were not successful at PCR amplification, we suspected colonization by a non-C. glabrata species. In this case, we performed a species determination analysis using primers ITS1 and ITS4 (White et al., 1990). One mouse of the untreated control in group yps1Δ was excluded from the study as colonization was established by a non-C. glabrata species, Cyberlindnera fabianii, confirmed by ITS amplification and sequencing (Supplementary Table 1).
Chitin Content Determination
The assessment of cell wall chitin content was performed as described (Costa-de-Oliveira et al., 2013) with some modifications. As the authors recommended, 2.5 μg/ml of calcofluor white (CFW) was used to stain chitin, the mean intensity of fluorescence was detected from three independent experiments using BV-421 filter followed by flow cytometry analysis using FlowJoTM software v10.6.1 (BD Biosciences). Chitin percentage of fluorescence was calculated relative to the parental strain ATCC90030. To confirm that CFW was staining chitin, strains ATCC2001HTL and its chitin synthase deletion mutant (ATCC2001HTL chs3Δ) were included as controls.
Statistical Analysis
Data analyses were performed using GraphPad Prism 8 software. Unpaired t-test was used to determine statistically significant differences for the in vitro tolerance assay, for fungal burdens of colonization between experimental groups (wild-type vs. deletion mutants) and for the differences in chitin content. To establish if the differences in rebound frequency were significant between experimental groups, the Freeman-Halton extension of the Fisher exact probability test was used. In all cases, p-values < 0.05 (two-tailed) were considered statistically significant.
Results
Identification of Four Candidate Genes Whose Deletions Reduce Echinocandin Tolerance
To identify new genes that contribute to C. glabrata echinocandin tolerance, we examined a collection of fifteen C. glabrata deletion mutants (Table 1) generated as part of a systematic deletion collection in the ATCC2001HTL background (Schwarzmüller et al., 2014). These mutants were chosen because they were sensitive to cell wall damaging agents (some to caspofungin) (Schwarzmüller et al., 2014; Rosenwald et al., 2016) and/or because these genes or their orthologs in Saccharomyces cerevisiae were known to function in cell wall maintenance (Table 1). We measured the survival of each knock-out strain after 24-h exposure to micafungin over a wide range of concentrations. As discussed previously (Healey et al., 2017), such survival assays are a better indicator of drug tolerance than the standard MIC assay, which measures growth but not survival and therefore cannot detect non-growing surviving cells that possess the potential for resistance development. Whereas most of the deletion mutants showed no or minor changes in micafungin tolerance (Supplementary Figure 1), four of them – yps1Δ, ypk2Δ, slt2Δ, and slg1Δ – resulted in significantly reduced tolerance to micafungin and caspofungin at one or more concentrations of the drug (Figure 1) and were therefore selected for further studies.
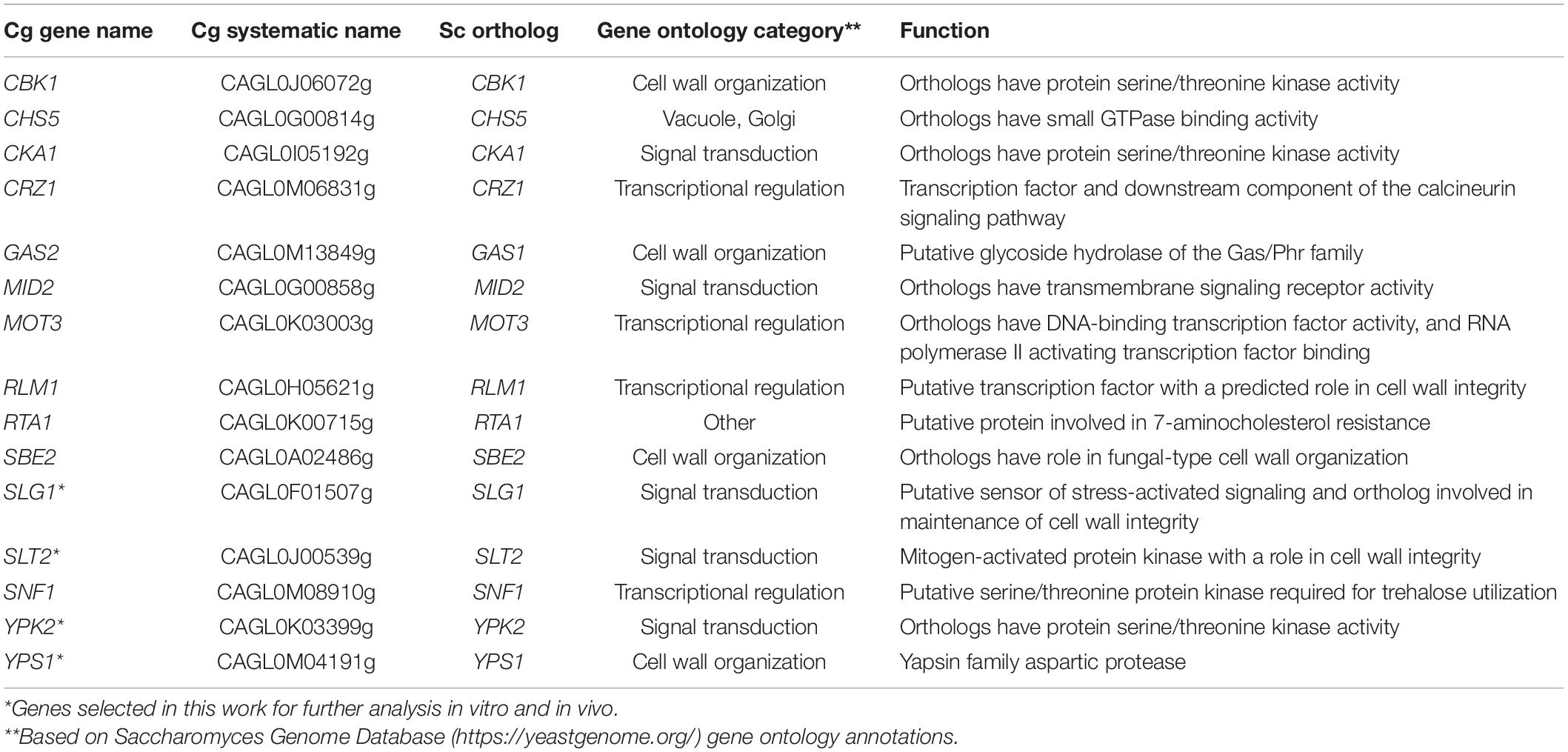
Table 1. Fifteen Candida glabrata genes whose deletion mutants were analyzed for echinocandin tolerance in vitro.
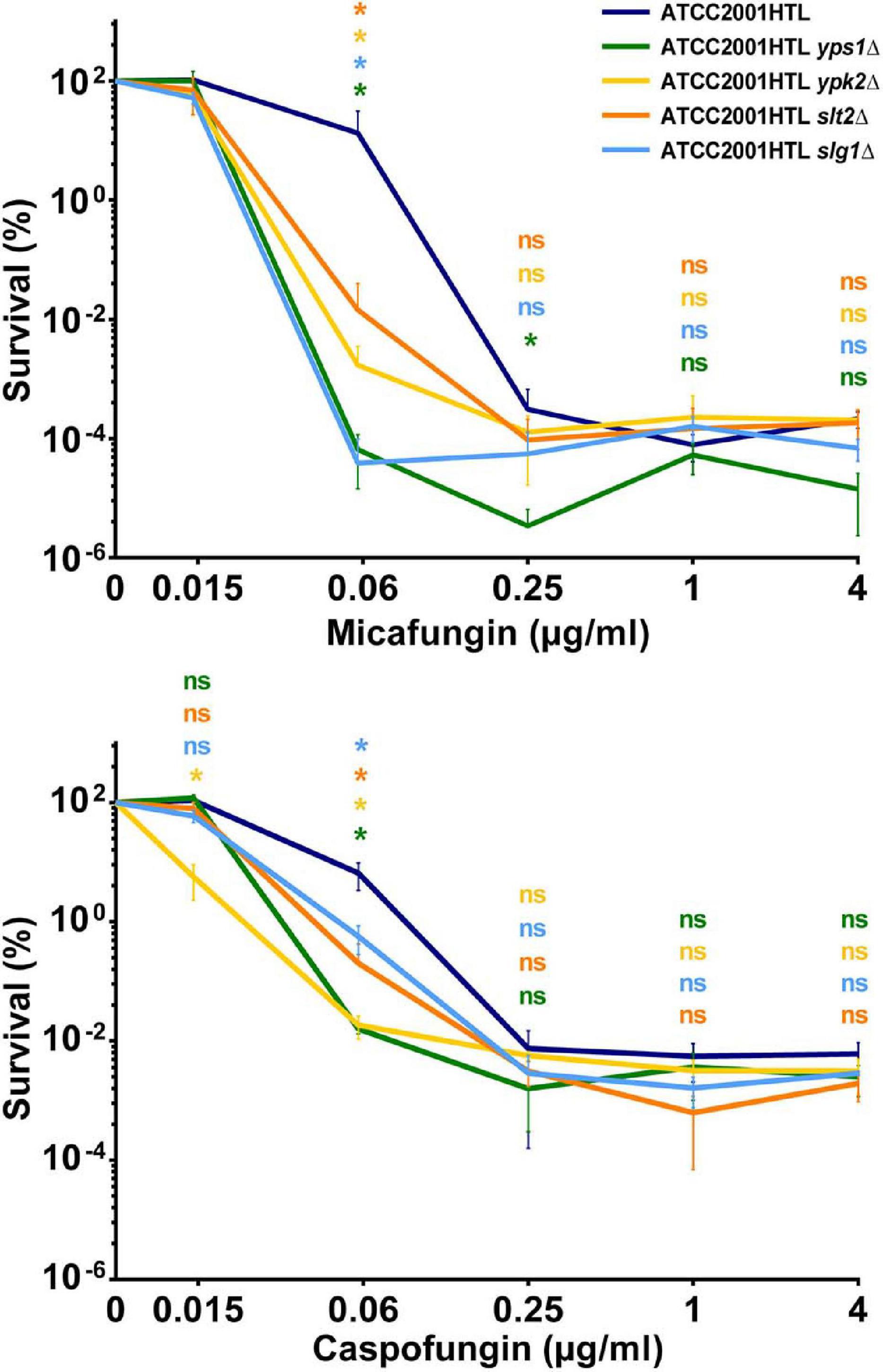
Figure 1. Deletions of genes involved in cell wall maintenance exhibited significantly reduced tolerance to micafungin and caspofungin. Survival percentage was obtained by normalizing the colony forming units (CFU) obtained from cultures treated with the indicated drug concentrations normalized to non-treated controls for each strain. A decrease in drug tolerance was observed in one or more drug concentrations of micafungin or caspofungin in yps1Δ, ypk2Δ, slt2Δ, and slg1Δ mutants after 24 h-drug exposure to a wide range of concentrations. Results were calculated from at least three independent biological replicates. The statistical significance was calculated using an unpaired t-test and is indicated with an asterisk (*) or with n.s. (not significant).
Selection of a Candida glabrata Strain for in vivo Studies of Echinocandin Tolerance
We next wished to investigate the contribution of these genes to echinocandin tolerance in vivo using a mouse model of C. glabrata GI colonization previously developed in our laboratory (Healey et al., 2017). This model recapitulates important aspects of C. glabrata commensalism and emergence of drug resistance because the GI tract is a primary source of Candida colonization and has also been described as a reservoir of antimicrobial resistant microorganisms (Donskey, 2004). Briefly, in this model the mouse GI tract is sterilized using antibiotics and colonized via oral gavage with C. glabrata. After 3 days of colonization, stably colonized mice are exposed to high-dose daily caspofungin (20 mg/kg body weight). In vivo, tolerance is manifested as an initial decline in GI fungal burdens but not true sterilization, whereas resistance, or a reduction in drug susceptibility, is manifested as a rebound of GI burdens to near their initial levels. Our expectation was that strains with reduced echinocandin tolerance in vitro would also show reduced tolerance in vivo, resulting in improved rates of sterilization by the drug and decreased rebound of the fungal burden after treatment. However, we found that we could not test this hypothesis in ATCC2001HTL background, because this strain showed a very low rate of rebound in the GI model, making it impossible to detect a further decrease potentially caused by a mutant (Figure 2). Thus, we performed a pilot GI colonization experiment with a different strain, ATCC90030, which showed a higher level of tolerance and rebound after caspofungin treatment than ATCC2001HTL (Figure 2). As expected, the rebound in fungal burden was accompanied by the emergence of reduced susceptibility to caspofungin in the yeast isolated from mouse fecal pellets, and it was often (though not always) associated with fks mutations. Three different fks mutations were identified, all in the FKS2 gene (S663P, F659L, and P667T) and previously shown to have clinical relevance. Thus, ATCC90030 was chosen as the strain background, in which to test the contribution of YPS1, YPK2, SLT2, and SLG1 to echinocandin tolerance in vivo.
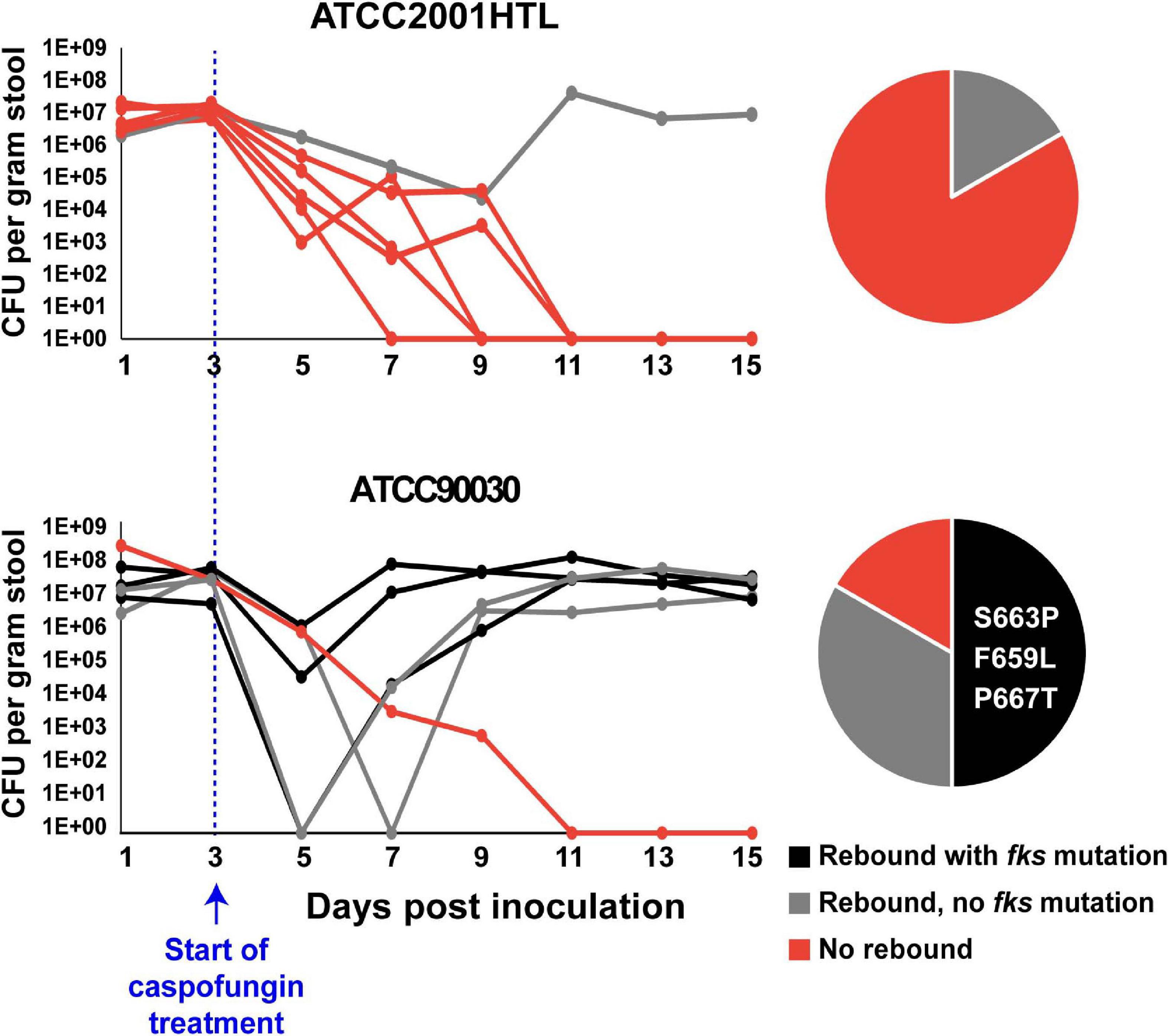
Figure 2. Candida glabrata strain ATCC90030 was more tolerant to caspofungin than strain ATCC2001HTL in the mouse model of gastrointestinal (GI) colonization, leading to a higher rate of rebound and emergence of echinocandin resistant fks-mutants. Six mice per group were colonized with the indicated C. glabrata strains and exposed to daily high-dose caspofungin treatment starting at day 3 post-colonization. CFU per gram of stool were plotted for each day of sample collection, and frequencies for rebound and fks mutations are indicated in pie charts. Zero and three fks-mutants were obtained in ATCC2001HTL and ATCC90030 background, respectively. All obtained mutations were in the FKS2 gene, and the specific amino acid changes are listed in the pie chart.
Deletions of YPS1, YPK2, and SLT2 in ATCC90030 Background Increase the Susceptibility to Echinocandin in vitro
A CRISPR-based approach was used to delete the four genes, separately, in the C. glabrata ATCC90030 strain and replace them with NAT resistance marker. Despite several attempts, we were unable to knock out SLG1. Interestingly, a recent saturation transposon mutagenesis study, conducted in C. glabrata BG2 strain background, indicated that SLG1 confers a fitness defect in C. glabrata (Gale et al., 2020), which may explain our inability to obtain this mutant. If this is the case, the original slg1Δ mutant generated in the ATCC2001HTL background may have a suppressor mutation, which improves fitness. Alternatively, ATCC90030 may be more sensitive to loss of SLG1 than ATCC2001HTL, e.g., due to transcriptional rewiring of the cell wall integrity pathway. For the other three genes, two independent knock-out mutants were generated and analyzed in subsequent assays. In all cases the two mutants showed identical phenotypes, so we present their combined data. Using susceptibility testing, we found that all three mutants resulted in a two- to four-fold reduction in caspofungin and micafungin MIC compared to the ATCC90030 parent strain (Figure 3A). We also performed an in vitro echinocandin tolerance assay using caspofungin to align with our GI colonization model. After 24-h caspofungin exposure, all three mutants showed reduced survival relative to the parent strain (Figure 3B), recapitulating the echinocandin tolerance defect observed in the ATCC2001HTL background (Figure 1) and confirming the roles of these genes in caspofungin tolerance in vitro.
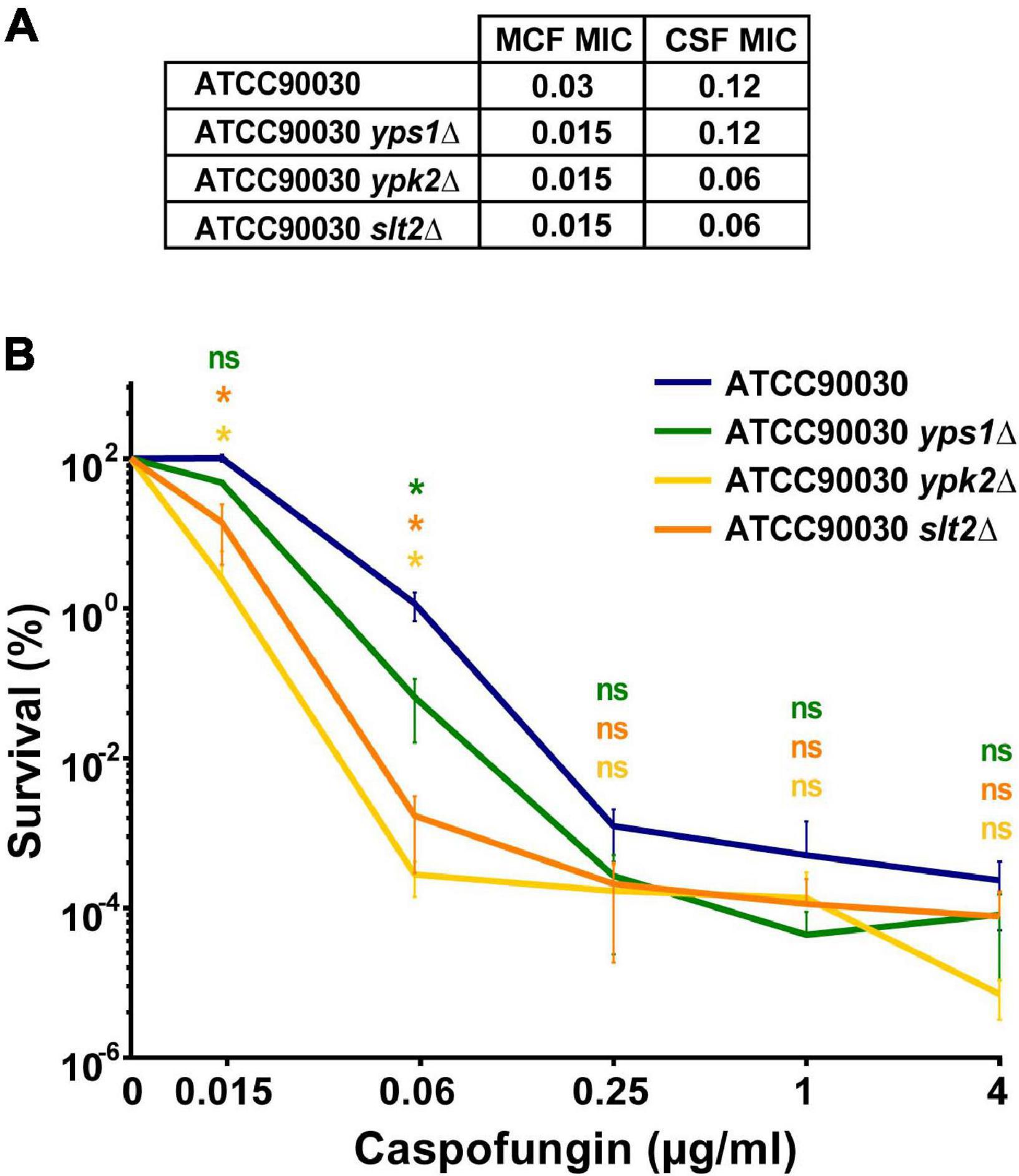
Figure 3. In vitro echinocandin hyper-susceptibility of yps1Δ, ypk2Δ, and slt2Δ was confirmed in the ATCC90030 strain background. (A) Minimum inhibitory concentration (MIC) susceptibility testing to caspofungin and micafungin showed that the deletion mutants had lower or identical MIC-values than the wild-type parental strain. Echinocandin concentrations are shown in μg/ml. (B) The in vitro tolerance assay confirmed that the mutants exhibited reduced drug tolerance compared to the parental ATCC90030 strain. Statistical significance was calculated using an unpaired t-test; p-value ≤ 0.05 is indicated with an asterisk (*), p-value > 0.05 is indicated as n.s. (not significant).
Deletions of YPS1, YPK2, and SLT2 Led to Improved Treatment Efficacy, Decreased Emergence of Fungal Burden and Reduced Caspofungin Susceptibility
To determine whether the three mutants under examination also alter echinocandin susceptibility in vivo, we used our C. glabrata GI colonization model (Healey et al., 2017). As described above, the mouse GI tract was sterilized using antibiotics and colonized via oral gavage with strain ATCC90030 or its knock-out derivatives. Stable colonization of the GI tract was established by all strains by day 3 (Figure 4A), at which point the mice were started on high-dose daily caspofungin (20 mg/kg). After 2 days of caspofungin treatment there was a drop in GI fungal burdens in all treated mice independent of genotype. However, at later time points, some mice showed a rebound in fungal burdens due to strains with reduced susceptibility, and this occurred at different rates in mice colonized with the wild-type parent strain compared to the mice colonized with the knock-out mutants (Figure 4B). For instance, for the ATCC90030 wild-type strain, there was a rebound of the fungal burden in nine out of 11 mice (82%), reaching burdens of 105–108 CFU/g of stool by the end of the experiment (15 days post-colonization, 12 days post-treatment) despite daily administration of high-dose caspofungin (Figure 4B). The remaining two mice out of 11 colonized with ATCC90030 eventually showed full sterilization. As expected, rebound was associated with reduced susceptibility to caspofungin in the yeast isolated from fecal pellets, and slightly more than half of the isolated strains contained fks mutations (Figure 4B). In contrast, mice inoculated with cell wall integrity deletion mutants showed significantly higher rates of sterilization and reduced levels of rebound of fungal burden (Figure 4B). For instance, for the yps1Δ mutant, only two out of nine colonized mice showed rebound after treatment, while the remaining seven showed near or full sterilization (Figure 4B, p = 0.02 vs. wild-type strain), and no fks mutations were detected in the two mice with fungal rebound. Similarly, for the ypk2Δ and slt2Δ mutants, three mice showed rebound, while seven and six, respectively, showed near or full sterilization (Figure 4B, p = 0.02 for ypk2Δ and p = 0.03 for slt2Δ). As was the case with the wild-type strain, about half of the yeast strains isolated from mice with fungal rebound contained fks mutations (Figure 4B). Thus, all three cell wall integrity mutants led to reduced echinocandin tolerance, decreased emergence of strains with reduced susceptibility to caspofungin, and improved treatment efficacy in vivo.
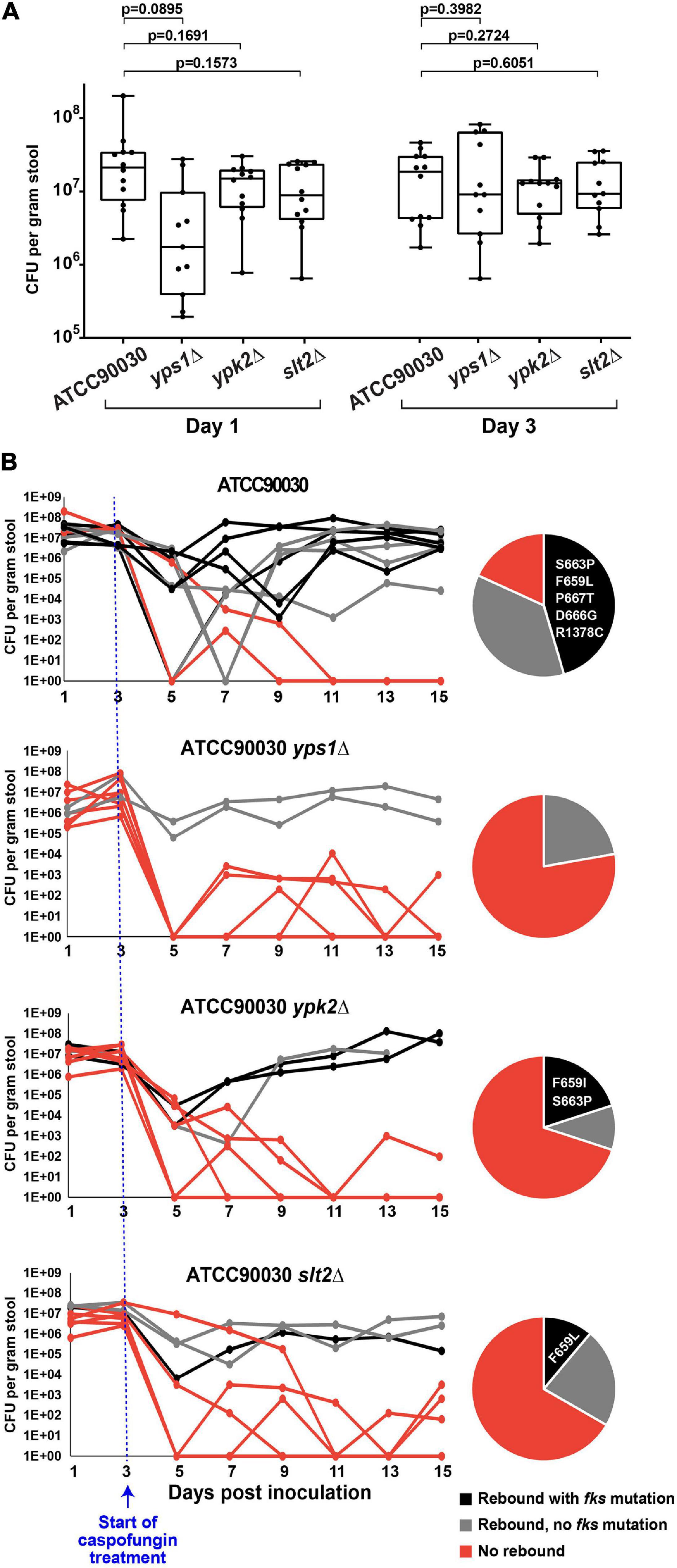
Figure 4. Three cell wall integrity mutants (yps1Δ, ypk2Δ, and slt2Δ) exhibited reduced caspofungin tolerance and decreased emergence of strains with reduced susceptibility to caspofungin in the mouse model of GI colonization. (A) Prior to the start of caspofungin treatment, all mice reached and maintained a fungal burden of 106–108 CFU/g of stool, with non-significant differences between groups. (B) Caspofungin treatment was more effective at sterilizing the GI of mice colonized with the cell wall integrity mutants. Mice colonized with the ATCC90030 parental strain showed a higher rebound of fungal burden relative to the deletion mutants, which was due to strains both with and without fks mutations. All obtained mutations were in the FKS2 gene, and the specific amino acid changes are listed in the pie charts.
Fungal Rebound After Treatment Is Associated With Elevated Caspofungin MICs Due to Both fks-Dependent and Independent Mechanisms
To ask whether fungal rebound was associated with reduced echinocandin susceptibility, we measured the MICs of caspofungin and micafungin in strains isolated from the GI tract of mice with fungal rebound 15 days after colonization (except for one mouse in the ypk2Δ group, which had to be sacrificed on day 13 and for which day 13 colonies were therefore analyzed) and compared them to the MICs of parental strains. As expected, the majority of rebound strains, including all strains carrying fks mutations, showed elevated caspofungin MICs relative to parental strains (Figure 5). Interestingly, many of the rebound strains, including two fks mutants (wild-type D666G and slt2Δ F659L) and most FKS wild-type strains, did not show elevated micafungin MICs, indicating that the change in susceptibility was specific to the drug used for treatment. Two fks mutations were isolated in multiple backgrounds: the fks2-S663P mutation was isolated both in the wild-type and the ypk2Δ strains, whereas the fks2-F659L mutation was isolated both in wild-type and slt2Δ strains. Interestingly, in both cases the MIC of the wild-type strain with the mutation was several-fold higher than the MIC of the mutant with the same mutation (Figure 5), suggesting that cell wall maintenance defects reduce echinocandin tolerance even for strains with mutant GS. Finally, this analysis identified a number of strains lacking fks mutations but nevertheless showing elevated caspofungin MICs (Figure 5), suggesting that these strains had as yet unknown FKS-independent mechanisms leading to reduced drug susceptibility. One mechanism by which Candida cells tolerate cell wall stress is by up-regulation of chitin levels (Reinoso-Martín et al., 2003; Cota et al., 2008), so we examined the chitin content of the non-FKS mutants using CFW staining followed by flow cytometry analysis. However, we found no significant differences in CFW content between non-FKS rebound strains and their parental strains (Supplementary Figure 2), indicating that chitin levels may not be the mechanism underlying their elevated echinocandin MICs.
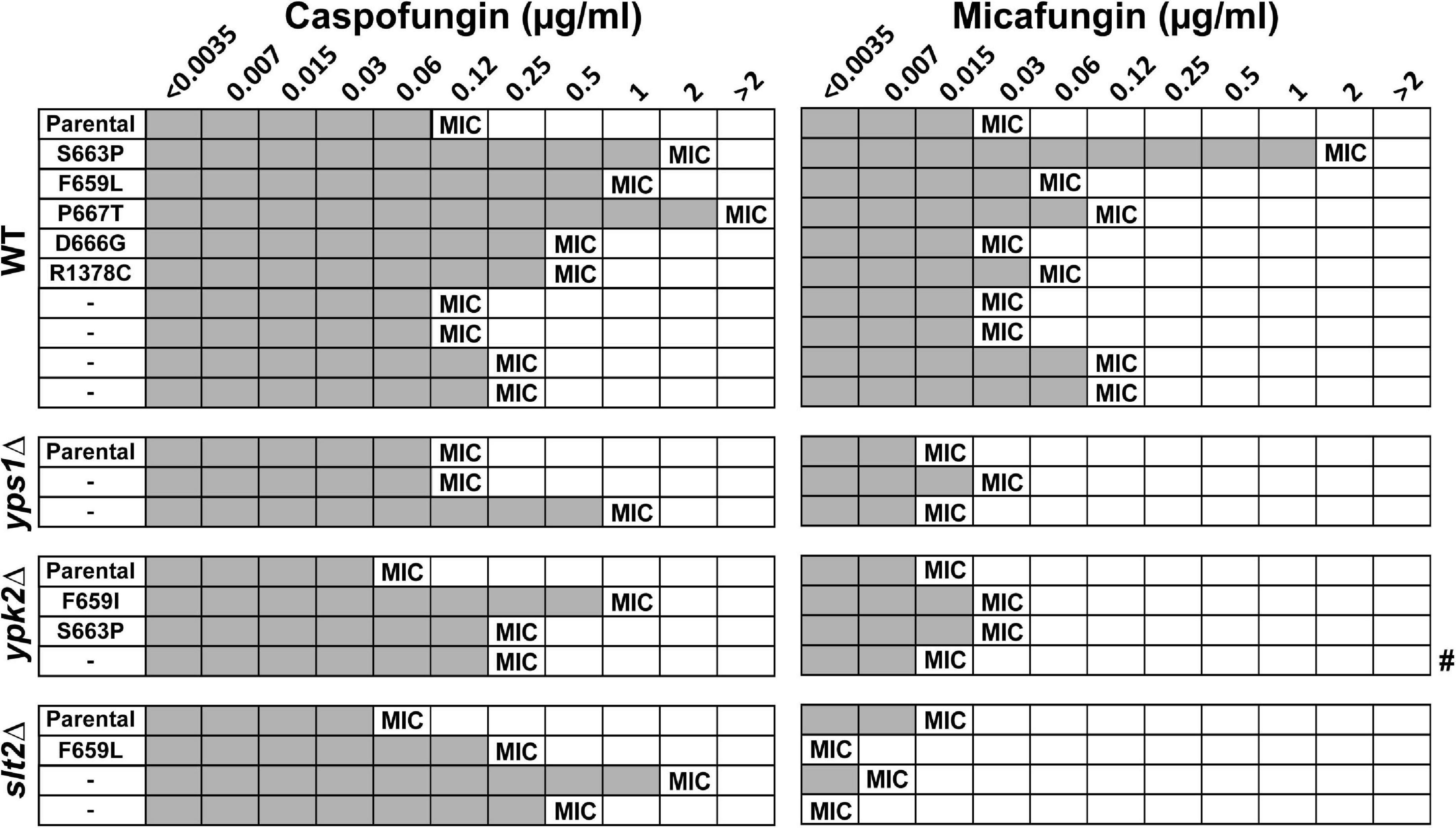
Figure 5. Fungal rebound was predominantly due to strains with elevated MICs to caspofungin but not necessarily micafungin. All strains with fks mutations showed reduced susceptibility to caspofungin relative to the parental strain. Gray shading indicates visible growth in drug-containing media, and the MIC is defined as the lowest concentration at which there is at least a 50% decrease in growth. # indicates one mouse in the ypk2Δ group, which had to be sacrificed on day 13 and for which day 13 colonies not day 15 were therefore analyzed.
Discussion
In this study, we assessed the contribution of several C. glabrata cell wall integrity genes to echinocandin tolerance in vitro and in vivo and to emergence of strains with reduced drug susceptibility in C. glabrata colonizing the mouse GI tract. We show that deletion of four genes involved in the maintenance of cell wall integrity, SLG1, YPS1, YPK2, and SLT2, increased the susceptibility of C. glabrata to two echinocandin class drugs, micafungin and caspofungin. Consistent with that finding, deletion mutants of YPS1, YPK2, and SLT2 were more efficiently eliminated from the mouse GI system by high-dose caspofungin treatment, with an associated reduction in the emergence of mutants with decreased susceptibility to caspofungin, including resistant mutants.
Cell wall integrity in both C. glabrata and closely related, well studied budding yeast S. cerevisiae is controlled protein kinase C (PKC), which upon cell wall damage triggers a Mitogen Activated Protein (MAP) kinase cascade that culminates with the phosphorylation and activation of Slt2p MAP kinase (Reinoso-Martín et al., 2003; Cota et al., 2008). Activated Slt2p phosphorylates and activates multiple downstream target proteins implicated in the expression of cell wall-related genes, including GS subunit FKS2 (Jung and Levin, 1999; de Nobel et al., 2000). Thus, the mechanistic role of SLT2 in cell wall integrity has been extensively studied in S. cerevisiae, and its importance has also been examined in C. glabrata. For instance, the C. glabrata slt2Δ strain was shown to have decreased tolerance to several cell wall damaging agents, such as congo red, CFW, and micafungin (Miyazaki et al., 2010). Furthermore, overexpression of SLT2 has been linked to an increase in chitin content and incomplete in vitro killing by caspofungin in C. glabrata and in S. cerevisiae (Reinoso-Martín et al., 2003; Cota et al., 2008). Thus, the reduced caspofungin and micafungin MIC of the slt2Δ mutants is consistent with a decreased expression of one or both FKS genes and/or other cell wall components controlled by this pathway. Interestingly, our data also show a genetic interaction between slt2Δ and fks2-F659L mutation, wherein this mutation raised the micafungin MIC of the wild-type strain but reduced the micafungin MIC of the slt2Δ mutant. This difference suggests that the effect of this mutation on the drug-target interaction may be dependent on the expression level of the target (GS), which is regulated by SLT2. Overall, the results presented here reinforce the importance of SLT2 in echinocandin tolerance in C. glabrata and provide the first evidence for a role of this gene in echinocandin tolerance in C. glabrata colonizing a mammalian host and in emergence of drug resistance in vivo.
Like SLT2, YPK2 encodes a protein kinase; however, significantly less information is available about Ypk2p function. Ypk2p and its closely related ortholog Ypk1p have been studied in S. cerevisiae, where deletion of YPK1 results in slow growth, whereas ypk2Δ shows no apparent phenotypic defects (Chen et al., 1993). However, S. cerevisiae cells deleted for both genes are inviable, indicating that these genes have functionally redundant roles in cell viability. Interestingly, the cells carrying inactivating mutations in both YPK1 and YPK2 lyse rapidly unless placed in osmotically supportive medium (Roelants et al., 2002). Furthermore, the inviability of the ypk1Δ ypk2Δ mutant is rescued by overexpression of EXG1, a major exo-1,3-beta-glucanase of the cell wall, supporting a role of these genes in cell wall integrity. Indeed, genetic analyses have placed YPK1 and YPK2 in a cell wall integrity pathway that acts in parallel with the PKC1-dependent pathway (Roelants et al., 2002). Our results suggest that in C. glabrata at least Ypk2p is also necessary for the maintenance of cell wall integrity, contributing to echinocandin tolerance both in vitro and in the animal host.
Yps1p is a member of the yapsin protein family, which are GPI-linked aspartyl proteases with established roles in cell wall integrity and in virulence (Krysan et al., 2005). In S. cerevisiae the yapsin family contains five members, whereas C. glabrata has eleven yapsins, showing an expansion of this family in C. glabrata. Yapsin functions have been predominantly studied in S. cerevisiae, where deletion mutants have defects in cell wall glucan incorporation (but not in glucan synthesis), with mannan and chitin levels increased as a compensatory response (Krysan et al., 2005). The quintuple yapsin mutant is sensitive to cell wall-disrupting agents, showing dramatically altered cell wall composition and temperature-induced lysis. Phenotypic analysis of individual yapsin deletion mutants in S. cerevisiae highlighted the important role of ScYps1p in cell wall maintenance: ScYps1 was shown to promote glucan incorporation and/or retention within the cell wall in response to cell wall stress and remodeling, acting as part of the transcriptional response to cell wall stress mediated by the Pkc1 signaling cascade (Heinisch et al., 1999). In agreement with these data, Scyps1Δ mutant is sensitive to caspofungin, congo red, and caffeine, and its overexpression during cell wall stress helps maintain cell wall glucan homeostasis (Krysan et al., 2005). C. glabrata yapsins are less well studied, but CgYps1p seems to have a role in cell wall regulation in the context of maintaining pH homeostasis, helping reduce total beta-glucan levels in the cell wall in acidic environments (Bairwa and Kaur, 2011). In vivo models have shown that yapsins are essential for C. glabrata virulence in a murine model of systemic infection (Kaur et al., 2007) as well as responsible for epithelial cell damage in an experimental oral infection model with C. albicans (Albrecht et al., 2006). In agreement with the consensus role of yapsins in cell wall integrity maintenance, our study demonstrates that CgYps1 is necessary for echinocandin tolerance both in vitro and in vivo, and for emergence of echinocandin resistance in vivo.
Clinical echinocandin resistance in C. glabrata is strictly associated with amino acid substitutions in specific hotspot regions of integral membrane proteins Fks1p and Fks2p (Perlin, 2015b), and most of these mutations directly correlate with clinical failure (Garcia-Effron et al., 2009; Shields et al., 2012; Alexander et al., 2014). All fks mutations described in this work occurred in the FKS2 gene, which is in agreement with the previous observations that echinocandin resistance is more frequently associated with mutation in FKS2 in C. glabrata (Arendrup and Perlin, 2014). Notably, seven isolates out of nine harbored fks mutations (F659I, F659L, S663P, D666G, and P667T) that also have been identified among human clinical isolates, and several of these have been associated with clinical failure (Garcia-Effron et al., 2009; Alexander et al., 2014). Modifications of FKS2 at positions F659 or R1378 have also been previously described (Shields et al., 2012; Bienvenu et al., 2019); however, as far as we know, the F659I and R1378C mutations are novel amino acid changes associated with reduced susceptibility identified for the first time in this work. Finally, the rebound in fungal burden was not always associated with fks mutations, indicating additional mechanisms that will be explored in future studies. The clinical significance of this finding is intriguing but unclear. Whereas compensatory increases in cell wall chitin content have been linked to increased echinocandin tolerance (Reinoso-Martín et al., 2003; Krysan et al., 2005; Cota et al., 2008), we found no significant differences in the chitin levels of these strains compared to their parental strain. The elevated MICs of rebound strains could also be linked to changes in plasma membrane lipid composition, similar to what we have demonstrated for Aspergillus fumigatus, where two membrane sphingolipids, dihydrosphingosine and phytosphingosine, rendered GS insensitive to echinocandins (Satish et al., 2019). Supporting the sphingolipid hypothesis, whereas all isolates with fks mutations showed reduced susceptibility to caspofungin (elevated caspofungin MICs), this was not the case for micafungin. Indeed, several strains lacking fks mutations and one strain containing an fks mutation (wild-type background, fks2-D666G) had higher MIC values to caspofungin than their respective parental strains, but unaltered, or sometimes decreased, MICs to micafungin. This phenomenon was reminiscent of the so-called “CRS-MIS phenotype” observed in a set of C. glabrata mutants derived in vitro that showed caspofungin reduced susceptibility (CRS) combined with micafungin increased susceptibility (MIS) (Healey et al., 2012). The CRS-MIS phenotype was shown to be connected to changes in membrane sphingolipids modulating the echinocandin-GS interaction. Thus, it is possible that some or all mutants isolated in our study that show a CRS-MIS-like phenotype also have altered membrane sphingolipid composition, resulting in these particular changes in GS sensitivity to echinocandin class drugs.
In summary, the experiments presented here evaluate echinocandin tolerance and emergence of strains than are less susceptible to killing by caspofungin in C. glabrata colonizing the animal host, uncovering an important role of multiple cell wall integrity maintenance mechanisms in these processes. These experiments lay the foundation for future work examining other components of these pathways, the mechanistic details underpinning echinocandin tolerance, and identification of new ways to manipulate these mechanisms to enhance the efficacy of drug treatment and prevent emergence of resistance.
Data Availability Statement
The original contributions presented in the study are included in the article/Supplementary Material, further inquiries can be directed to the corresponding authors.
Ethics Statement
The animal study was reviewed and approved by Center for Discovery and Innovation Institutional Animal Care and Use Committee (IACUC).
Author Contributions
RG-R, RH, and AC performed the experiments. RG-R and ES performed the data analysis. RG-R, KH, ES, and DP designed the experiments. RG-R, ES, and DP wrote the manuscript. ES and DP supervised, coordinated, and directed the project. All authors contributed to the article and approved the submitted version.
Funding
This work was supported by NIH 5R01AI109025 to DSP.
Conflict of Interest
DP receives research support and/or serves on advisory boards for Amplyx, Cidara, Scynexis, N8 Medical, Matinas, Merck, Regeneron, and Pfizer. RG-R, RH, AC, KH, and ES declare that the research was conducted in the absence of any commercial or financial relationships that could be construed as a potential conflict of interest.
Acknowledgments
We thank Kuchler lab (Medical University Vienna, Max F. Perutz Laboratories, Department of Medical Biochemistry, Vienna, Austria) for sharing the fifteen C. glabrata gene deletion strains. We also thank Christopher Quinteros for technical support.
Supplementary Material
The Supplementary Material for this article can be found online at: https://www.frontiersin.org/articles/10.3389/fmicb.2021.702779/full#supplementary-material
References
Albrecht, A., Felk, A., Pichova, I., Naglik, J. R., Schaller, M., de Groot, P., et al. (2006). Glycosylphosphatidylinositol-anchored proteases of Candida albicans target proteins necessary for both cellular processes and host-pathogen interactions. J. Biol. Chem. 281, 688–694. doi: 10.1074/jbc.M509297200
Alexander, B. D., Johnson, M. D., Pfeiffer, C. D., Jiménez-Ortigosa, C., Catania, J., Booker, R., et al. (2014). Increasing echinocandin resistance in Candida glabrata: clinical failure correlates with presence of FKS mutations and elevated minimum inhibitory concentrations. Clin Infect Dis. (2013) 56(12):1724-32. doi: 10.1093/cid/cit136. Epub 2013 Mar 13. Erratum Clin. Infect. Dis. 58:754.
Arastehfar, A., Gabaldón, T., Garcia-Rubio, R., Jenks, J. D., Hoenigl, M., Salzer, H. J. F., et al. (2020). Drug-resistant fungi: an emerging challenge threatening our limited antifungal armamentarium. Antibiotics (Basel) 9:877. doi: 10.3390/antibiotics9120877
Arendrup, M. C., and Perlin, D. S. (2014). Echinocandin resistance: an emerging clinical problem? Curr. Opin. Infect. Dis. 27, 484–492. doi: 10.1097/QCO.0000000000000111
Bairwa, G., and Kaur, R. (2011). A novel role for a glycosylphosphatidylinositol-anchored aspartyl protease, CgYps1, in the regulation of pH homeostasis in Candida glabrata. Mol. Microbiol. 79, 900–913. doi: 10.1111/j.1365-2958.2010.07496.x
Berman, J., and Krysan, D. J. (2020). Drug resistance and tolerance in fungi. Nat. Rev. Microbiol. 18, 319–331. doi: 10.1038/s41579-019-0322-2
Bienvenu, A. L., Leboucher, G., and Picot, S. (2019). Comparison of fks gene mutations and minimum inhibitory concentrations for the detection of Candida glabrata resistance to micafungin: a systematic review and meta-analysis. Mycoses 62, 835–846. doi: 10.1111/myc.12929
Chen, P., Lee, K. S., and Levin, D. E. (1993). A pair of putative protein kinase genes (YPK1 and YPK2) is required for cell growth in Saccharomyces cerevisiae. Mol. Gen. Genet. 236, 443–447. doi: 10.1007/BF00277146
Clinical and Laboratory Standards Institute (CLSI) (2017). Performance Standards for Antifungal Susceptibility Testing of Yeasts, 1st Edn. Annapolis Junction, MD: CLSI.
Costa-de-Oliveira, S., Silva, A. P., Miranda, I. M., Salvador, A., Azevedo, M. M., Munro, C. A., et al. (2013). Determination of chitin content in fungal cell wall: an alternative flow cytometric method. Cytometry A 83, 324–328. doi: 10.1002/cyto.a.22250
Cota, J. M., Grabinski, J. L., Talbert, R. L., Burgess, D. S., Rogers, P. D., Edlind, T. D., et al. (2008). Increases in SLT2 expression and chitin content are associated with incomplete killing of Candida glabrata by caspofungin. Antimicrob. Agents Chemother. 52, 1144–1146. doi: 10.1128/AAC.01542-07
de Nobel, H., Ruiz, C., Martin, H., Morris, W., Brul, S., Molina, M., et al. (2000). Cell wall perturbation in yeast results in dual phosphorylation of the Slt2/Mpk1 MAP kinase and in an Slt2-mediated increase in FKS2-lacZ expression, glucanase resistance and thermotolerance. Microbiology (Reading) 146(Pt 9), 2121–2132. doi: 10.1099/00221287-146-9-2121
Dichtl, K., Samantaray, S., and Wagener, J. (2016). Cell wall integrity signalling in human pathogenic fungi. Cell Microbiol. 18, 1228–1238. doi: 10.1111/cmi.12612
Donskey, C. J. (2004). The role of the intestinal tract as a reservoir and source for transmission of nosocomial pathogens. Clin. Infect. Dis. 39, 219–226. doi: 10.1086/422002
Enoch, D. A., Yang, H., Aliyu, S. H., and Micallef, C. (2017). The changing epidemiology of invasive fungal infections. Methods Mol. Biol. 1508, 17–65. doi: 10.1007/978-1-4939-6515-1_2
Gale, A. N., Sakhawala, R. M., Levitan, A., Sharan, R., Berman, J., Timp, W., et al. (2020). Identification of essential genes and fluconazole susceptibility genes in Candida glabrata by profiling hermes transposon insertions. G3 (Bethesda) 10, 3859–3870. doi: 10.1534/g3.120.401595
Garcia-Effron, G., Lee, S., Park, S., Cleary, J. D., and Perlin, D. S. (2009). Effect of Candida glabrata FKS1 and FKS2 mutations on echinocandin sensitivity and kinetics of 1,3-beta-D-glucan synthase: implication for the existing susceptibility breakpoint. Antimicrob. Agents Chemother. 53, 3690–3699. doi: 10.1128/AAC.00443-09
Healey, K. R., Katiyar, S. K., Raj, S., and Edlind, T. D. (2012). CRS-MIS in Candida glabrata: sphingolipids modulate echinocandin-Fks interaction. Mol. Microbiol. 86, 303–313. doi: 10.1111/j.1365-2958.2012.08194.x
Healey, K. R., Nagasaki, Y., Zimmerman, M., Kordalewska, M., Park, S., Zhao, Y., et al. (2017). The gastrointestinal tract is a major source of echinocandin drug resistance in a murine model of Candida glabrata colonization and systemic dissemination. Antimicrob. Agents Chemother. 61:e01412-17. doi: 10.1128/AAC.01412-17
Healey, K. R., and Perlin, D. S. (2018). Fungal resistance to echinocandins and the MDR phenomenon in Candida glabrata. J. Fungi (Basel) 4:105. doi: 10.3390/jof4030105
Heinisch, J. J., Lorberg, A., Schmitz, H. P., and Jacoby, J. J. (1999). The protein kinase C-mediated MAP kinase pathway involved in the maintenance of cellular integrity in Saccharomyces cerevisiae. Mol. Microbiol. 32, 671–680. doi: 10.1046/j.1365-2958.1999.01375.x
Howard, S. J., Livermore, J., Sharp, A., Goodwin, J., Gregson, L., Alastruey-Izquierdo, A., et al. (2011). Pharmacodynamics of echinocandins against Candida glabrata: requirement for dosage escalation to achieve maximal antifungal activity in neutropenic hosts. Antimicrob. Agents Chemother. 55, 4880–4887. doi: 10.1128/AAC.00621-11
Jung, U. S., and Levin, D. E. (1999). Genome-wide analysis of gene expression regulated by the yeast cell wall integrity signalling pathway. Mol. Microbiol. 34, 1049–1057. doi: 10.1046/j.1365-2958.1999.01667.x
Kaur, R., Ma, B., and Cormack, B. P. (2007). A family of glycosylphosphatidylinositol-linked aspartyl proteases is required for virulence of Candida glabrata. Proc. Natl. Acad. Sci. U.S.A. 104, 7628–7633. doi: 10.1073/pnas.0611195104
Krysan, D. J., Ting, E. L., Abeijon, C., Kroos, L., and Fuller, R. S. (2005). Yapsins are a family of aspartyl proteases required for cell wall integrity in Saccharomyces cerevisiae. Eukaryot Cell 4, 1364–1374. doi: 10.1128/EC.4.8.1364-1374.2005
Lee, K. K., Maccallum, D. M., Jacobsen, M. D., Walker, L. A., Odds, F. C., Gow, N. A., et al. (2012). Elevated cell wall chitin in Candida albicans confers echinocandin resistance in vivo. Antimicrob. Agents Chemother. 56, 208–217. doi: 10.1128/AAC.00683-11
Miyazaki, T., Inamine, T., Yamauchi, S., Nagayoshi, Y., Saijo, T., Izumikawa, K., et al. (2010). Role of the Slt2 mitogen-activated protein kinase pathway in cell wall integrity and virulence in Candida glabrata. FEMS Yeast Res. 10, 343–352. doi: 10.1111/j.1567-1364.2010.00611.x
Perlin, D. S. (2011). Current perspectives on echinocandin class drugs. Future Microbiol. 6, 441–457. doi: 10.2217/fmb.11.19
Perlin, D. S. (2015b). Mechanisms of echinocandin antifungal drug resistance. Ann. N. Y. Acad. Sci. 1354, 1–11. doi: 10.1111/nyas.12831
Perlin, D. S. (2015a). Echinocandin resistance in candida. Clin. Infect. Dis. 61, (Suppl. 6) S612–S617. doi: 10.1093/cid/civ791
Pfaller, M. A., and Diekema, D. J. (2010). Epidemiology of invasive mycoses in North America. Crit. Rev. Microbiol. 36, 1–53. doi: 10.3109/10408410903241444
Pham, C. D., Iqbal, N., Bolden, C. B., Kuykendall, R. J., Harrison, L. H., Farley, M. M., et al. (2014). Role of FKS mutations in Candida glabrata: MIC values, echinocandin resistance, and multidrug resistance. Antimicrob. Agents Chemother. 58, 4690–4696. doi: 10.1128/AAC.03255-14
Reinoso-Martín, C., Schüller, C., Schuetzer-Muehlbauer, M., and Kuchler, K. (2003). The yeast protein kinase C cell integrity pathway mediates tolerance to the antifungal drug caspofungin through activation of Slt2p mitogen-activated protein kinase signaling. Eukaryot Cell 2, 1200–1210. doi: 10.1128/ec.2.6.1200-1210.2003
Roelants, F. M., Torrance, P. D., Bezman, N., and Thorner, J. (2002). Pkh1 and Pkh2 differentially phosphorylate and activate Ypk1 and Ykr2 and define protein kinase modules required for maintenance of cell wall integrity. Mol. Biol. Cell 13, 3005–3028. doi: 10.1091/mbc.e02-04-0201
Rosenwald, A. G., Arora, G., Ferrandino, R., Gerace, E. L., Mohammednetej, M., Nosair, W., et al. (2016). Identification of genes in Candida glabrata conferring altered responses to caspofungin, a cell wall synthesis inhibitor. G3 (Bethesda) 6, 2893–2907. doi: 10.1534/g3.116.032490
Satish, S., Jiménez-Ortigosa, C., Zhao, Y., Lee, M. H., Dolgov, E., Krüger, T., et al. (2019). Stress-induced changes in the lipid microenvironment of β-(1,3)-d-Glucan synthase cause clinically important echinocandin resistance in Aspergillus fumigatus. mBio 10:e00779-19. doi: 10.1128/mBio.00779-19
Schwarzmüller, T., Ma, B., Hiller, E., Istel, F., Tscherner, M., Brunke, S., et al. (2014). Systematic phenotyping of a large-scale Candida glabrata deletion collection reveals novel antifungal tolerance genes. PLoS Pathog. 10:e1004211. doi: 10.1371/journal.ppat.1004211
Shields, R. K., Nguyen, M. H., Press, E. G., Kwa, A. L., Cheng, S., Du, C., et al. (2012). The presence of an FKS mutation rather than MIC is an independent risk factor for failure of echinocandin therapy among patients with invasive candidiasis due to Candida glabrata. Antimicrob. Agents Chemother. 56, 4862–4869. doi: 10.1128/AAC.00027-12
Shor, E., and Perlin, D. S. (2015). Coping with stress and the emergence of multidrug resistance in fungi. PLoS Pathog. 11:e1004668. doi: 10.1371/journal.ppat.1004668
Shor, E., Schuyler, J., and Perlin, D. S. (2019). A novel, drug resistance-independent, fluorescence-based approach to measure mutation rates in microbial pathogens. mBio 10:e00120-19. doi: 10.1128/mBio.00120-19
Walker, L. A., Gow, N. A., and Munro, C. A. (2013). Elevated chitin content reduces the susceptibility of Candida species to caspofungin. Antimicrob. Agents Chemother. 57, 146–154. doi: 10.1128/AAC.01486-12
Keywords: fungal infections, Candida glabrata, echinocandins, glucan synthase, cell wall integrity, YPS1, YPK2, SLT2
Citation: Garcia-Rubio R, Hernandez RY, Clear A, Healey KR, Shor E and Perlin DS (2021) Critical Assessment of Cell Wall Integrity Factors Contributing to in vivo Echinocandin Tolerance and Resistance in Candida glabrata. Front. Microbiol. 12:702779. doi: 10.3389/fmicb.2021.702779
Received: 29 April 2021; Accepted: 09 June 2021;
Published: 30 June 2021.
Edited by:
Miguel Cacho Teixeira, University of Lisbon, PortugalReviewed by:
Dominique Sanglard, University of Lausanne, SwitzerlandAlessandra Da Silva Dantas, University of Exeter, United Kingdom
Copyright © 2021 Garcia-Rubio, Hernandez, Clear, Healey, Shor and Perlin. This is an open-access article distributed under the terms of the Creative Commons Attribution License (CC BY). The use, distribution or reproduction in other forums is permitted, provided the original author(s) and the copyright owner(s) are credited and that the original publication in this journal is cited, in accordance with accepted academic practice. No use, distribution or reproduction is permitted which does not comply with these terms.
*Correspondence: Rocio Garcia-Rubio, rocio.garcirubio@gmail.com; Erika Shor, erika.shor@hmh-cdi.org